- 1State Key Laboratory of Quality Research in Chinese Medicine and School of Pharmacy, Macau University of Science and Technology, Macau, China
- 2School of Basic Medicine, Guizhou University of Traditional Chinese Medicine, Guiyang, China
- 3Department of Psychiatry, Huashan Hospital, Fudan University, Shanghai, China
- 4School of Biosciences, University of Nottingham, Loughborough, United Kingdom
Epigenetics is defined as the heritable alterations of gene expression without changes to the coding sequence of DNA. These alterations are mediated by processes including DNA methylation, histone modifications, and non-coding RNAs mechanisms. Vascular aging consists of both structural and functional changes in the vasculature including pathological processes that drive progression such as vascular cell senescence, inflammation, oxidation stress, and calcification. As humans age, these pathological conditions gradually accumulate, driven by epigenetic alterations, and are linked to various aging-related diseases. The development of drugs targeting a spectrum of epigenetic processes therefore offers novel treatment strategies for the targeting of age-related diseases. In our previous studies, we identified HDAC4, JMJD3, Fra-1, and GATA4 as potential pharmacological targets for regulating vascular inflammation, injury, and senescence.
Introduction
In the 19th century, the father of modern medicine William Osler stated, “a man is only as old as his arteries.” During vascular aging, pathological processes drive changes in the structure and function of blood vessels including dysregulation in vascular homeostasis and vascular remodeling, leading to lumen dilation, vascular stiffness, and thickening. At the molecular level dysregulation in vascular homeostasis is promoted by vascular cell senescence, widespread inflammation, oxidation stress, and calcification (Ding et al., 2018). It is now widely recognized that vascular aging is intimately linked with cardiovascular diseases (CVD) including atherosclerosis (AS), hypertension, coronary heart disease, and stroke (Lakatta and Levy, 2003b). Aside from high mortality rates, CVD also leads to reduce quality of life in afflicted individuals and high burden on society and families (Van Camp, 2014).
Epigenetics is defined as processes that governs the expression of a gene(s) without altering the sequence of coding DNA. These heritable changes in expression are controlled by distinct chemical modifications to bases present in DNA including DNA methylation and histone modification in addition to non-coding RNA (ncRNA) mechanisms. In healthy tissues, normal gene expression occurs as a result of interactions between genetic and environmental factors viz. smoking, obesity, or alcohol consumption, which can cause dysregulation in cellular homeostasis, having negative impacts on health. Epigenetics changes can explained many of the interaction between genes and environment cues and can explain altered risk of developing diseases in humans (Feinberg, 2018). Indeed, as age increases the cumulative effects of stress and environmental impacts promotes the gradual accumulation of epigenetic changes in tissues. These epigenetic changes could serve by increasing an individuals susceptibility and risk of developing chronic diseases. Fortunately, many of these epigenetic modifications can be reversed, and targeting the respective enzymes that control methylation or histone modifications has been proposed as useful drug target in the treatment of age-related diseases. Therefore, in the current review, coverage of vascular aging and associated epigenetics processes will be covered. In addition, a summary of the main pathological drivers of vascular cell senescence, inflammation, oxidation stress, and calcification will be provided. A better perspective of epigenetic changes that occur during vascular aging will help to better understand the process of vascular damage that occurs with age. This information could be used to better development therapeutics or strategies to delay or treat aging-related cardiovascular diseases (Sen et al., 2016).
Vascular Aging
Vascular aging is characterized by changes in both structural and functional elements associated with blood vessels. Over the course of time, vascular aging leads to lumen dilation, vascular stiffness, and thickening, these changes being largely driven by pathological processes including vascular cell senescence, widespread inflammation, oxidation stress, and tissue calcification (Ding et al., 2018). The structural and functional changes of blood vessels that occurs during vascular aging are shown in Figure 1.
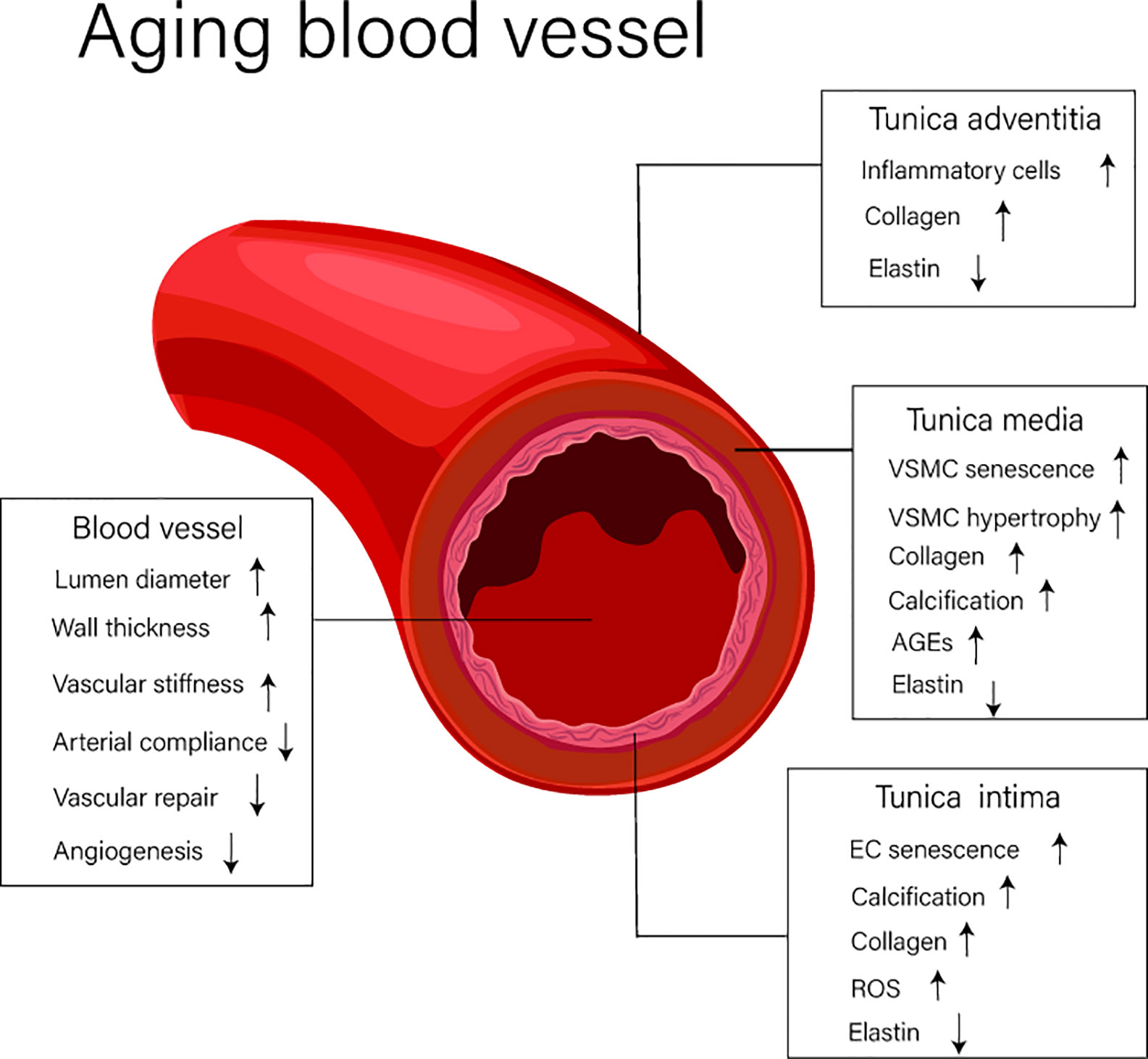
Figure 1 The structure and functional changes of blood vessels. EC, endothelial cell; VSMC, vascular smooth muscle cell; AGEs, advanced glycation end products; ROS, reactive oxygen species.
Structural Changes
Blood vessels (excluding capillaries) are composed of distinct anatomical features comprising the intima, media, and adventitia. The intima is largely composed of endothelial cells (ECs) and is the first defensive layer important in mitigating the development of vascular diseases. The media consists of vascular smooth muscle cells (VSMCs), elastic fibers, and extracellular matrix, and the adventitia, the outermost layer, is composed of loose connective tissue. This region is consists of thick collagen fibers and disordered elastin fibers. It is widely known that during aging, significant change occur in the intima, and this alters the function properties of this layer and, importantly, how the intima interacts with adjacent regions like the media (Lakatta et al., 2009). These changes promote altered responses to luminal dilation, vascular stiffness, and the thickening of blood vessels. Indeed, research has shown that the diameter of the aorta of elderly people, those over 65 years of age, increases by 15–20% compared with that of tissues from younger individuals (Lakatta, 2003). In addition, increased thickening of the arterial wall, largely driven by thickening of the arterial intima and media (Lakatta and Levy, 2003a), is associated with increased abundance of hypertrophic smooth muscle cells. Other common structural changes associated with aged blood vessels include elastin breaks, increased collagen abundance, and elevated levels of advanced glycation end products (AGEs). Combined these changes have a dramatic impact on the severity of vascular aging (Lakatta, 2003; Lakatta and Levy, 2003a).
Functional Changes
Increased arterial stiffness, decreased arterial compliance (AC), reductions in vascular repair, and diminished capacity to control processes like angiogenesis are important features of aging blood vessels. Arterial stiffness and decreased AC can be attributed to smooth muscle cell hypertrophy, arterial calcification, and ECM remolding. One of the key reasons for the decline in the ability of vessels to repair tissue damage is thought to be due to vascular endothelial cell senescence (Lakatta and Levy, 2003a; Novella et al., 2012).
Pathological Process During Vascular Aging and Cardiovascular Diseases
Aging blood vessels promote the development of vascular diseases and in turn accelerates the process of vascular aging. Numerous epidemiological studies indicate that lipid levels, diabetes, sedentary lifestyles, and various genetic factors increase the risk of coronary heart disease, hypertension, heart failure, and stroke. Underpining these changes are distinct biochemical and physiological changes that drive changes in the cardiovascular system. In recent times, the conventional cardiovascular continuum (CCC) in 2006 (Dzau et al., 2006a; Dzau et al., 2006b) has proposed that CVD begins with risk factors and progresses to terminal stage cardiac disease through a series of steps. The main characteristics of CCC are viewed as coronary artery atherosclerosis (AS), leading to coronary stenosis, and myocardial ischemia and myocardial infarction. However, one aspect not considered by the CCC criteria is the role of aging, especially vascular aging in the occurrence and development of CVD. Therefore, in 2010, the aging cardiovascular continuum (ACC) suggested that a key component of CVD should include aging (O’Rourke et al., 2010). Therefore, the ACC describes the stages of cardiovascular disease (CVD) as vascular aging, which promotes aorta dilation and sclerosis. In turn, these pathoglogical changes lead to heart failure, terminal stage cardiac disease, and death. In essence, the basic characteristics proposed by the ACC are the progressive degradation of the proximal aorta with arterial dilatation and sclerosis that has an adverse effects on the heart. If we consider that aortic pulsation, then the repetitive stretching and relaxation of arteries has occured 3 billion times in elderly people over 80 years old; it is clear that aging is an important factor in this process. As such, aging should be viewed as a risk factor for CVD, since it explains 50% of all clinical CVD cases in senior citizen (Cunha et al., 2017).
Vascular aging and CVD have several common pathological features when viewed at the molecular and cellular levels viz. increased oxidative stress, a pro-inflammatory environment, dysregulation in cell signaling, and altered response to infiltrating immune cell types. These feactures, as mentioned, provide sufficient conditions in the aged artery for the development of cardiovascular disease. For example, arterial aging and atherosclerosis have similar structural and biochemical characteristics. Indeed, research has shown that abnormal plasma cholesterol levels of young people is related to arterial wall thickening, VSMCs, collagen proliferation, and collagen deposition. These changes are similar to those observed in elderly people with altered cholesterol levels (McGill et al., 2008; Wang et al., 2010a). However, some animal studies show that plasma lipid levels do not change with aging; however, the prevalence, severity, and negative impact of atherosclerosis still increase in aged animals (Eto et al., 2008). These observations suggesting other molecular events are also important in driving CVD. One clue then may be that many proteins that are highly expressed in atherosclerotic tissues are also increased in aging arterial walls such as MFG-E8 and MMPs (Fu et al., 2009).
The pathological processes assocociated with vascular aging are characterized by vascular cell senescence, widespread inflammation, oxidation stress, and calcification, and these processes are summarized in Figure 2. As ECs age, the cells become flattened and enlarged, the rates of proliferation is diminished, and rates of apoptosis increased. In addition, enrichment of the surrounding environment with inflammatory mediators including interleukins-6 and tumour necrosis factor-α results in the decreased ability to control vascular repair and angiogenesis. Similalrly, the numbers of hypertrophic VSMCs begin to increase, as do the rates of cell proliferation and migration, and combined, this cellular transition drives extracellular matrix (ECM) remolding. Critically, as rates of inflammation increases over time, so do changes in cell populations of EC and VSMCs, and this, in turn, stimulates vascular aging and ultimately the endothelium damage. A widely recognized key driver of chronic inflammation in the vasculariture, especially during aging, is the renin-angiotensin II (Ang II) signaling pathway. Changes in this signaling system initiates a cascde of events leading to the activation of downstream pro-inflammatory transcription factors like nuclear factor kappa beta (NF-kB), the production of reactive oxygen species (ROS) leading to oxidative damage, and the induction of endoplasmic reticulum (ER) stress. ER and sarcoplasmic reticulum are important pools of calcium; therefore, this could promote dysregulation in Ca2+ signaling. Collectively, these molecular events accelerate vascular remodeling (Cavallaro et al., 2000; Lakatta et al., 2009; Wang et al., 2010a; Wang et al., 2010b; Krebs et al., 2015). Under normal physiological conditions, ROS are produced in the vascular system through two routes, the mitochondrial and non-mitochondrial pathways. Importantly, in healthy or younger cells, oxidative stress is controlled by cells having the capacity to remove excess ROS via antioxidant system, and this could potentially reduce the rates of oxidative damage to mtDNA, respiratory chain complex proteins, and other important cellular components, therefore maintaining adequate cellular and tissue redox homeostasis (Kadlec et al., 2016). However, during aging, mitochondrial function can diminish, and this results in a cumulative increase of mitochondrial damage and excessive ROS production. Consequently, increased levels of ROS are generated via active oxygen release mechanism, resulting in further damage to the mitochondrial outer membrane and causing membranes to rupture, intracellular calcium overload, DNA damage, and the release of pro-apoptotic proteins like cytochrome C. This cascade ultimately leads to the induction of apoptosis or necrosis and further amplifies damage to aged or aging tissues fueling age-related degenerative diseases (Mikhed et al., 2015).
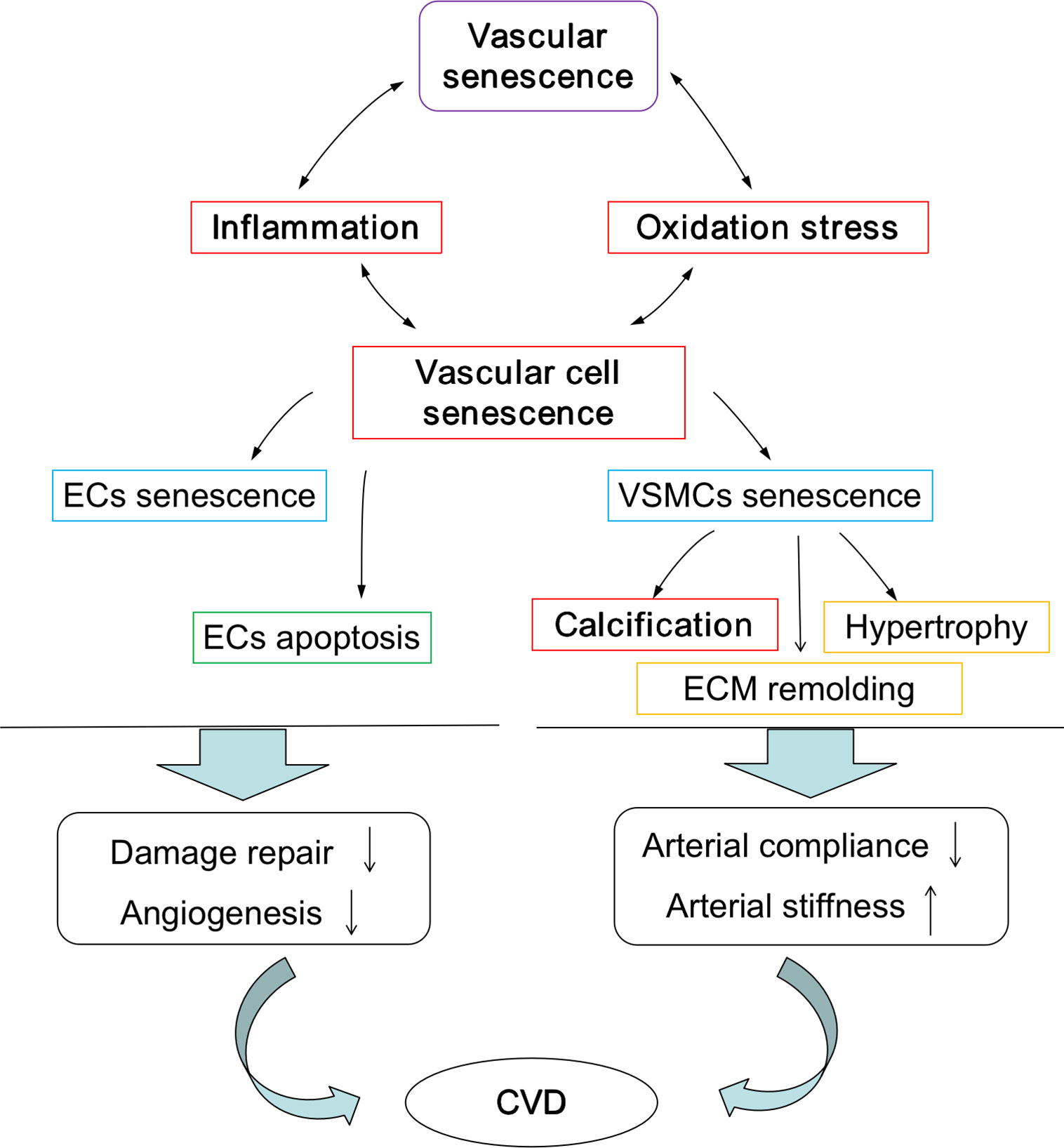
Figure 2 Pathological process during vascular aging. Pathological process promote the development of vascular aging and vascular aging accelerates the pathological process. EC, endothelial cell; VSMC, vascular smooth muscle cell; ECM, extracellular matrix; CVD, cardiovascular diseases.
Tissue calcification is also common in the aged cardiovascular system, and researchers have demonstrated that senescent VSMCs are associated with a calcification phenotype. Aging VSMCs produce appreciable levels of calpain-1, and these promote blood vessels calcification, a process commonly referred to as biomineralization (Jiang et al., 2012). Calcification activates tissue transglutaminase (TG2) and upregulates calcification promoter genes such as the osteoblast transcription factor Runt-related transcription factor 2 (Runx2) and bone morphogenetic protein-2 (BMP-2). These systems participate in driving arterial calcification and sclerosis in the aging blood vessel wall. It is now realized that vascular calcification is an important cause of the increase in the incidence and mortality of cardiovascular diseases (Leopold, 2013) and that calcification, VSMCs hypertrophy, ECM remolding promote arterial stiffness and decreased AC.
Epigenetic Control of Gene Expression
Epigenetics is the heritable alterations of gene expression without changing the DNA sequence and determines whether a gene is turned on or off (Handy et al., 2011). Mounting evidence shows that epigenetic mechanisms play an important role in phenotypes and behavioral changes. Indeed, these mechanisms go someway to explain why twins who grew up in different locations and under differing environmental conditions, yet having the same genetic background, can have differences in lifespans or altered risks of chronic diseases such as diabetes or hypertension (Fraga et al., 2005; Tan et al., 2013). Similarly, dietary influences like caloric restriction can delay the occurrence of age-dependent diseases via epigenetic mechanisms. Again, these examples exemplify the intimate association between how environmental factors can drive changes in epigenetic events that control gene expression (Maegawa et al., 2017).
DNA Methylation
DNA methylation, the direct addition of a methyl group to the 5th carbon atom of cytosine, is one the most widely recognised epigenetic mechanims known to regulate gene expression. CpG islands, characterized as short interspersed DNA sequences that are GC-rich regions. In mammalian cells, most of these CpG regions can be methylated (Jeltsch, 2008). Importantly, de novo DNA methylation status is governed by a family of enzymes known as DNA methyltransferases that include DNMT3a and DNMT3b, along with DNMT1 that is important during replication (Okano et al., 1999). The activities of these enzymes are readily influenced by environmental cues making DNA methylation a dynamic process in cells and tissues (Tsaprouni et al., 2014). Generally speaking, high methylation rates in the gene promoter region of genes inhibit gene expression. Inhibition in this instance is caused by altered (reduced) transcription factors binding to promoters consensus regions or by reducing the recruitment of chromatin modifying enzymes (Kohli and Zhang, 2013). To date, a wide range of abnormal DNA methylation patterns have been characterized in aged cells, with many seen in various age-related diseases (Hai and Zuo, 2016).
Histone Modifications
Histone modifications is another important epigenetic mechanism that regulates gene transcription by changing how histones proteins interact with DNA. Changes in DNA histone interactions is important in controlling gene expression during processes such as replication, transcription, and repair. Common mechanisms of histone modification include methylation, acetylation, phosphorylation, and ubiquitination. Unlike DNA methylation, the effect of histone modifications on gene expression may vary due to the specific type of chemical modifications (Shahbazian and Grunstein, 2007). Enzymes that regulate histone modifications include histone deacetylase (HDAC), histone methyltransferase, and histone acetyltransferase, and it is widely known that these enzymes play an important roles in the process of vascular aging (Calvanese et al., 2009).
ncRNA Mechanisms
ncRNA is RNA that lacks the capacity to code for a protein. Examples of ncRNA include microRNA (miRNA), long ncRNA (lncRNA), and small interfering RNA (siRNA). Although ncRNA has no direct affect on chromatin structure, it does play an important role in post-transcriptional control of gene expression (Gurha and Marian, 2013). Genome-wide RNA sequencing shows that ncRNAs are differentially expressed in both senescent and normal cells (Wu et al., 2015; Anderson et al., 2016).
Do Epigenetics Mechanisms Contribute to Vascular Aging?
The following introduces the pathological steps that are involved in the epigenetic regulation of vascular aging. A summary of these events and epigenetic targets are shown in Table 1.
Are Epigenetic Mechanisms Involved in the Regulation of Vascular Cell Senescence?
NAD-dependent deacetylase sirtuin-1 (SIRT1), is the most thoroughly studied member of the Nuclear-localized type III histone deacetylases (Situin) family. This protein is involved at multiple levels during the stages of vascular aging, and plays an important role vascular cell senescence as demonstrated by the following observations: (1) the expression of SIRT1 in VSMCs of mice is decreased with advancing age; (2) smooth muscle-specific knockout of SIRT1 in animals promotes Angiotensin II (Ang II) induced vascular senescence (Chen et al., 2016); (3) SIRT1 is important in the deacetylation of histone H4K16, and that this process inhibits senescence of ECs and is protective against vascular aging (Wan et al., 2014); (4) SIRT1 can increase ECs Kruppel-like factor 2 (KLF2) expression, which causes vascular ECs to enter a “vaso-protective” state (Gracia-Sancho et al., 2010); (5) energy restriction promotes increased SIRT1 expression in VSMCs and tissues and fights abdominal aortic aneurysm (Liu et al., 2016); and finally, (6) nuclear-localized SIRT6 protects telomeres in vascular ECs, tempering reductions in replication capacity and premature cell senescence following DNA damage (Cardus et al., 2013).
ncRNAs, especially miRNAs, are involved in vascular cell senescence. miRNAs are short single-stranded ribonucleic acids that can negatively regulate gene expression by base-pairing to target mRNA and causing mRNA cleavage or translation repression (Carthew and Sontheimer, 2009). For example, the expression of miR-217 in ECs is seen to increase with age and can inhibit the expression of SIRT1. Subsequent reductions in the expression of SIRT1 increases the levels of senescent and dysfunction ECs. Interestingly, miR-217 inhibition reportedly slows the rates of cellular senescence in ECs (Menghini et al., 2009). Similarly, miR-145 and miR-143 can regulate the phenotypic transition of smooth muscle cells during vascular aging and during the differentiation of smooth muscle. The miR-143 and miR-145 work together targeted transcription factors network, such as Klf4 (Kruppel-like factor 4) and myocardin and Elk-1 (member of ETS oncogene family) and, on the one hand, form a positive feedback mechanism to promote the differentiation of smooth muscle cells, and on the other hand, repress the proliferation of smooth muscle cells (Cordes et al., 2009). The lncRNA H19 is expressed in the adult endothelium and is reduced with advanced age. H19 inhibits the STAT3 signaling pathway, a key pathway regulating endothelial cell senescence (Hofmann et al., 2019). Other lcRNAs such as MEG3 prevent miR-128–dependent Girdin down regulation and inhibits vascular endothelial cell senescence (Lan et al., 2019). More recently, our research group has shown that Fos-related antigen 1 (Fra-1) plays an important role in Ang II–induced vascular senescence. Fra-1 expression is dramatically increased in Ang II–induced rat aortic endothelial cell (RAEC) senescence (Yang et al., 2019).
Epigenetic Regulation of Inflammation in Vascular Aging
Typically, an individuals inflammatory status increases with age (Fulop et al., 2018). Studies on the methylation patterns in the human genome have shown that hypermethylation of DNA appears to correlated with chronic inflammation associated with many aging-related diseases (Cutolo et al., 2014). For example, in pathophysiological conditions such as atherosclerosis, hypomethylation of the promoter region of monocyte chemoattractant protein-1 (MCP-1) leads to increased expression of MCP-1 and promotes the recruitment of inflammatory cells accelerating the disease process (Liu et al., 2012).
In other research, the activation of SIRT1 in smooth muscle cells reduces the stiffness of blood vessels; a process associate with crosstalk with the NF-κB inflammatory signaling pathway and the inhibition of inflammatory signaling (Fry et al., 2016). Loss of functional SIRT1 resulted in hyperacetylated of NF-KB and drives increased transcription of pro-inflammatory genes. Similarly, cytoplasmic SIRT2 induces the deacetylate of p65 Lys310 and regulates NF-κB–dependent gene expression viz. reduces transcription of pro-inflammation genes (Rothgiesser et al., 2019). These finding showing that acetylation status of NF-κB is an important driver of rates of inflammation in cells and tissues. Additional support comes from the knowledge that the inhibition of histone deacetylases (HDAC) increases tumor necrosis factor α (TNF-α) levels, the activation of the NF-κB signaling pathway, and resultant increase in IL-8 expression (Ashburner et al., 2001). Other members of the HDAC family such as HDAC3 have been shown to inhibit the activation of macrophages. In macrophages lacking HDAC3, there is an increased response to stimulation by interleukin 4 (IL-4) that likely drives increased rates of inflammation in blood vessel leading to aging (Mullican et al., 2011). In addition, our research on vascular aging points to a possible role of HDAC4 in mitigating inflammatory responses in the vascular system. HDAC4 appears to play an essential role in vascular inflammation by regulates Ang II–induced autophagy via the activation of FoxO3a deacetylation (Yang et al., 2018), work recently communicated as an editorial focusing on cardiovascular epigenetics (Tarun and Antoniades, 2018). Additional research from our group has identified histone demethylase Jumonji domain-containing protein 3 (JMJD3) as a key epigenetic regulator of the inflammatory response in cells (Liu et al., 2018). JMJD3 playing a pivitol role in rheumatoid synovial hyperplasia in rheumatoid arthritis (RA) (Jia et al., 2018a; Wu et al., 2019). Moreover, evidence also points to potential roles for JMJD3 in vascular remodeling (Luo et al., 2018) and in the regulation of the transcription factor GATA4. GATA4 functioning in inflammation persistence and angiogenesis in rheumatoid arthritis (RA) (Jia et al., 2018).
Other mechanisms linking epigenetic processes and inflammatory status include the ncRNA molecule, miR-155. miR-155 is a positive regulator of vascular inflammation, and is abundant in activated macrophages and monocytes, and potentially leaves blood vessels in a chronic inflammatory state. Increased levels of miR-155 induce the expression of MCP-1, and could encourage the recruitment of monocytes to vascular tissues thus exacerbating the inflammatory response (Wu et al., 2014). In contrast, miR-194 has opposing effects in that this miRNA can inhibit inflammation. While the mechanisms for this still required additional research, it is known that miR-194 overexpression can inhibit tumor necrosis factor receptor-associated factor 6 (TRAF6), and this reduces the production of monocyte inflammatory factors (Tian et al., 2015).
Regulation of Oxidation Stress in Vascular Aging
Links between vascular aging, DNA methylation patterns and oxidative stress are also seen in blood vessels. Regulation in the expression of endothelial nitric oxide synthase (eNOS), which encodes an endogenous nitric oxide synthase and is a source of the vasoactive molecule nitric oxide (NO), is altered by methylation status. Lower methylation patterns in the promoter region of eNOS allow for gene transcription and is therefore important in the production of NO and associated physiological processes involving this gaseous signaling molecule. In pathological situations hypermethylation in the eNOS promoter region inhibits eNOS expression and causes diminished levels of NO (Chan et al., 2004). Other proteins such as p66Shc, a protein associated with endothelial dysfunction, are also regulated via methylation status. The p66Shc protein is important in signaling systems linked to the production of hydrogen peroxide (H2O2), and the gene encoding for this protein is known to contains a large number of methylation sites. Modification of the methylation pattern is thus seen as a means to control the expression of this protein (Ventura et al., 2002; Cencioni et al., 2013).
Potential roles of SIRT1 in mitigating oxidative stress in smooth muscle cells has recently been proposed and suggested to be important in reducing blood vessels stiffness (Fry et al., 2016). Similarly, expression of SIRT3 a protein known to decline in tissues by as much as 40% by the age of 65 also appears to be important in mitigating oxidative stress. In cells lacking SIRT3, the activity of the mitochondrial antioxidant enzyme superoxide dismutase 2 (SOD2) is impaired due to hyperacetylation. Consequently, elevated mitochondrial O2• and diminished endothelial NO are observed, leading to an imbalance of redox homeostasis in blood vessels (Dikalova et al., 2017). Building on this area of research are roles for miRNA and associated impacts on redox systems linked to vascular ageing. To date, miR-210 has recently been demonstrated to reduce the overproduction of mitochondrial reactive oxygen species (ROS) (Ma et al., 2018).
Epigenetics Systems and Calcification in Vascular Aging
SIRT1 can inhibit vascular remodeling, stiffness, and functions in protection against atherosclerosis and vascular calcification in mice and is indicative that SIRT1 has protective roles in vascular injury diseases (Winnik et al., 2015). Evidence to support protective roles come from several important pieces of research. Firstly, that in vitro culture of VSMCs using media containing high levels of phosphate (Pi) stimulates cell senescence and calcification. These changes related to the down-regulation of SIRT1 expression and the activation of p21(WAF1/Cip1). Activation of p21(WAF1/Cip1) drives replicative senescent in VSMC cells, a process that can be reversed in cells in which p21(WAF1/Cip1) has been knockdown using molecular approaches. Moreover, loss of functional p21(WAF1/Cip1) abolishes Pi induced senescence and calcification in VSCMs. Thirdly, knockdown of SIRT1 in cells promotes a transformation to a calcification phenotype and promotes Pi-induced VSMC senescence calcification. Interestingly, treatment of cells with the SIRT1 induce resveratrol activates the protein and inhibits VSMC calcification (Takemura et al., 2011). In allied areas of research, the expression of the miRNA molecule miR-34a is elevated in the aorta of aged mice and is associated with rates of calcification. miR-34a is downregulating by SIRT1, this serving to temper miR-34a induced VSMCs calcification (Badi et al., 2018). Futhermore, the lncRNA-ES3/miR-34c-5p/BMF axis has recently been shown to regulate high-glucose-induced VSMCs calcification/senescence (Lin et al., 2019), and miR-135a, miR-714, miR-762, and miR-712 are involved in VSMC calcification by disrupting Ca2+ efflux proteins like NCX1, PMCA1, and NCKX4 (Gui et al., 2012).
Epigenetics Regulation and Treatment of Vascular Aging and CVD
As discussed in the previous sections, vascular aging contributes to cardiovascular disease(s) including atherosclerosis, hypertension, coronary heart diseases, and stroke. Vascular aging encompasses many biochemical and physiological changes associated with vascular remodeling, vascular homeostasic imbalance, vascular cell senescence, a pro-inflammation state and increased rates of oxidative stress, and tissue calcification. The interplay between each of these conditions is complex and makes the development of robust treatment strategies targetting vascular aging challenging. Indeed, the targeting of a single vascular cell type or population or a specific signaling system is difficult. However, the recognized association between epigenetic regulation of multiple gene targets coding for proteins regulating biochemical or physiological processes linked to vascular aging may be achievable. Epigenetic targets could serve as appropriate therapeutic targets suitable for the management of vascular aging and related diseases in humans. These treatments, if explored and developed further, may be more effective, span several risk factors linked to the development of vascular aging, and would have the added benefit that they are reversible processes. Such systems could be exploited in the future development of novel therapeutics. For example, our research has demonstrated that JMJD3 could be a useful therapeutic target. JMJD3 is a crucial epigenetic regulator involved in the inflammatory response to LPS in macrophages. JMJD3 expression is controlled by the transcription factor Sp-1 and is responsible for changes in the expression of cystathionine gamma-lyase (CSE). This system negatively regulates the inflammatory response in cells and tissues and reduce the progression of rheumatoid arthritis (RA). Moreover, deficiency of JMJD3 reduces neointima formation after vascular injury by inhibits the Nox4-autophagy signaling pathway. These observations suggesting that JMJD3 may represent a novel target for the development of new anti-inflammatory therapeutics for treating RA, the prevention and treatment of intima hyperplasia-related vascular diseases, and other pro-inflammatory conditions (Liu et al., 2018; Jia et al., 2018a; Luo et al., 2018; Wu et al., 2019). Likewise, the transcription factor GATA4, a key regulator of angiogenesis and persistence of inflammation in RA may also hold promise as a therapeutic target (Jia et al., 2018). We also show that Fos-related antigen 1 (Fra-1) plays a novel and key role in promoting vascular aging by directly binding and activating the target proteins p21(WAF1/Cip1) and p16(INK4A) protein signaling systems. Intervention of Fra-1 is a potential strategy for the prevention of aging-related cardiovascular disorders (Yang et al., 2019).
The development of epigenetically targeted therapeutics has received considerable attention over the last decade and has lead to the identification of several important epigenetic modified protein inhibitors including the FDA-approved molecule azacytidine and various inhibitors of DNMT1, HDAC, and histone acetyltransferases (HAT) (Voelter-Mahlknecht, 2016). These therapeutics could be adopted for use in the treatment of specific CVD conditions. To date, epidrugs-based therapeutics for the treatment of CVD mainly include compounds widely used in the clinical that function through epigenetics-related mechanisms, numerous natural compounds, and various newly synthesized molecules. Our group has reported on the anti-inflammation effects of the HDAC4 inhibitor Tasquinimod and its use in the treatment of vascular inflammation-related diseases (Yang et al., 2018). Other molecules of interest include common statins used to lower serum cholesterol to prevent major cardiovascular problems. Some statins may function as HDAC inhibitor (Voelter-Mahlknecht, 2016). Likewise, trichostatin A, an inhibitor of HDAC, prevents ventricular remodeling by inhibiting TNF-α transcription and by promoting cardiomyocyte survival by enhancing Akt phosphorylation (Zhang et al., 2012). In addition, in experimental models of myocardial infarction and atherosclerosis, the HDAC inhibitor sodium butyrate inhibits NF-κB signal transduction and the production of inflammatory molecules including TNF-α, interleukin-6, vascular cell adhesion molecule-1, and intercellular adhesion molecule-1, pointing to potential pharmacological effects (Hu et al., 2014). The natural product curcumin functions as an HAT inhibitor in rodent models of heart failure preserving systolic function and preventing ventricular hypertrophy (Pan et al., 2013). Similarly, molecules like folic acid and B vitamins are DNMT inhibitors and deficiencies in folic acid causes global DNA hypomethylation that is associated with increased risk of CVD including coronary heart disease, atherosclerosis, and anemia (Kim et al., 2007; McNulty et al., 2008). The common analgesic, acetylsalicylic acid appears to reduces ATP-binding cassette transporter A1 gene methylation rates in the pathophysiology conditions associated with coronary heart disease and thus points to a potentially new therapeutic strategy for this disease (Guay et al., 2014). Likewise, the molecule 5- aza-2-deoxycytidinede (DAC), an inhibitor of DNMT that can reverse rates of DNA methylation, has been shown to re-active genes silenced by hypermethylation viz. estrogen receptors α and β in normal ECs and smooth muscle. Importantly, the failure of some estrogen therapies to protect cardiac tissues from damage could be due to epigenetic silencing of the female estrogen receptor. The discovery of natural products that can be used to alter SIRT1 activity in cells has also gained some interest. SIRT1 expression and activity can reduce during senescence-related diseases and re-activation of SIRT1 in tissues may offer new opportunities in the development of future drug candidates. Indeed, one potential drug for disease intervention, is the stilbene resveratrol, an activator of SIRT1. Resveratrol has been repeatedly shown to effectively delay vascular senescence in mice, and to improve cardiometabolic health (da Luz et al., 2012; Pollack and Crandall, 2013; Kim et al., 2018). Finally, rapamycin-induced miR-30a down-regulation is mediated via the targeting of beclin1 and can inhibit the senescence of VSMCs (Tan et al., 2019). It is clear that more research is needed to further address the anti-aging effects of many of these molecules and in the discovery of other compounds that could be used to manipulate epigenetic systems in mammalian cells and tissues. In particular, pharmacologist should focus on research to assess the role of other types of epigenetic targeting drugs. Identified molecules could then drive developments in combined approaches to treat CVD using epigenetic-based therapeutics coupled with hormone replacement therapy (Schiano et al., 2015).
Conclusion and Prospects
Our understanding of the molecular mechanism controlling the epigenetic regulation of gene expression has progressed significantly over the last two decades. However, much has still to be learnt, and our perceived ideas of epigenetic modulation and its manipulation in vivo is far more complicated than previously thought. Aging is an irreversible biological process while epigenetic alternations are reversible and may offer novel treatment strategies in patients with age-related CVD. New methods and experimental research techniques are needed to facilitate the manipulation of epigenetic processes in cells and tissues. Indeed, recently a newly emerging epigenetic mechanism involving RNA methylation has been reported (Yue et al., 2015); however, its role in vascular biology is not yet clear and requires further research. Another important problem to be solved in the future is how to manipulate histone modification in specific tissues like the vascular endothelium. This problem is critical because systemic inhibition or activation of HDAC, or other epigenetic enzymes may cause adverse reactions (Heerboth et al., 2014). Building on these advances will be the ability to monitor epigenetic changes in cells, this will be critical in making advances in this field. Interestingly, the progressive development of single-cell sequencing and single-cell epigenetic technologies like scATAC-seq, scDNase-seq, and scChic-seq can be used to study the mode of epigenetic regulation at the single-cell level, and these technologies will offers exciting opportunities in the near future (Nawy, 2014; Ku et al., 2019). In particular, these systems will aid in the development of more elaborate models of epigenetic regulation and will allow for the development of more accurately therapeutics for use in epigenetic research. With regards to vascular aging, a good start here would be research on newer epigenetic pharmaceuticals, developed using drug repurposing approaches; a safe and low-cost way to support future vascular drug discovery (Xu et al., 2019). In addition, since epigenetic mechanisms work in concert to regulate gene networks, there may also be requirements for the development of epigenetic “cocktail” therapies that can be exploited to target a spectrum of age-related genes for treating age-related diseases.
Author Contributions
All authors contributed to the article and approved the submitted version.
Funding
This work was supported by the National Natural Science Foundation of China (81973320), Macau Science and Technology Development fund (FDCT) (067/2018/A2,033/2017/AMJ, 0007/2019/AKP, 0052/2020/A), and the Key Laboratory Program of the Education Commission of Shanghai Municipality (no. ZDSYS14005).
Conflict of Interest
The authors declare that the research was conducted in the absence of any commercial or financial relationships that could be construed as a potential conflict of interest.
References
Anderson, K. M., Anderson, D. M., McAnally, J. R., Shelton, J. M., Bassel-Duby, R., Olson, E. N. (2016). Transcription of the non-coding RNA upperhand controls Hand2 expression and heart development. Nature 539 (7629), 433–436. doi: 10.1038/nature20128
Ashburner, B. P., Westerheide, S. D., Baldwin, A. S., Jr (2001). The p65 (RelA) subunit of NF-kappaB interacts with the histone deacetylase (HDAC) corepressors HDAC1 and HDAC2 to negatively regulate gene expression. Mol. Cell Biol. 21 (20), 7065–7077. doi: 10.1128/MCB.21.20.7065-7077.2001
Badi, I., Mancinelli, L., Polizzotto, A., Ferri, D., Zeni, F., Burba, I., et al (2018). miR-34a Promotes Vascular Smooth Muscle Cell Calcification by Downregulating SIRT1 (Sirtuin 1) and Axl (AXL Receptor Tyrosine Kinase). Arterioscler. Thromb. Vasc. Biol. 38 (9), 2079–2090. doi: 10.1161/ATVBAHA.118.311298
Calvanese, V., Lara, E., Kahn, A., Fraga, M. F. (2009). The role of epigenetics in aging and age-related diseases. Ageing Res. Rev. 8 (4), 268–276. doi: 10.1016/j.arr.2009.03.004
Cardus, A., Uryga, A. K., Walters, G., Erusalimsky, J. D. (2013). SIRT6 protects human endothelial cells from DNA damage, telomere dysfunction, and senescence. Cardiovasc. Res. 97 (3), 571–579. doi: 10.1093/cvr/cvs352
Carthew, R. W., Sontheimer, E. J. (2009). Origins and Mechanisms of miRNAs and siRNAs. Cell 136 (4), 642–655. doi: 10.1016/j.cell.2009.01.035
Cavallaro, U., Castelli, V., Del Monte, U., Soria, M. R. (2000). Phenotypic alterations in senescent large-vessel and microvascular endothelial cells. Mol. Cell Biol. Res. Commun. 4 (2), 117–121. doi: 10.1006/mcbr.2000.0263
Cencioni, C., Spallotta, F., Martelli, F., Valente, S., Mai, A., Zeiher, A. M., et al. (2013). Oxidative stress and epigenetic regulation in ageing and age-related diseases. Int. J. Mol. Sci. 14 (9), 17643–17663. doi: 10.3390/ijms140917643
Chan, Y., Fish, J. E., D’Abreo, C., Lin, S., Robb, G. B., Teichert, A. M., et al. (2004). The cell-specific expression of endothelial nitric-oxide synthase: a role for DNA methylation. J. Biol. Chem. 279 (33), 35087–35100. doi: 10.1074/jbc.M405063200
Chen, H. Z., Wang, F., Gao, P., Pei, J. F., Liu, Y., Xu, T. T., et al. (2016). Age-Associated Sirtuin 1 Reduction in Vascular Smooth Muscle Links Vascular Senescence and Inflammation to Abdominal Aortic Aneurysm. Circ. Res. 119 (10), 1076–1088. doi: 10.1161/CIRCRESAHA.116.308895
Cordes, K. R., Sheehy, N. T., White, M. P., Berry, E. C., Morton, S. U., Muth, A. N., et al. (2009). miR-145 and miR-143 regulate smooth muscle cell fate and plasticity. Nature 460 (7256), 705–710. doi: 10.1038/nature08195
Cunha, P. G., Boutouyrie, P., Nilsson, P. M., Laurent, S. (2017). Early Vascular Ageing (EVA): Definitions and Clinical Applicability. Curr. Hypertens. Rev. 13 (1), 8–15. doi: 10.2174/1573402113666170413094319
Cutolo, M., Paolino, S., Pizzorni, C. (2014). Possible contribution of chronic inflammation in the induction of cancer in rheumatic diseases. Clin. Exp. Rheumatol. 32 (6), 839–847. doi: 10.3177/jnsv.47.385
da Luz, P. L., Tanaka, L., Brum, P. C., Dourado, P. M., Favarato, D., Krieger, J. E., et al. (2012). Red wine and equivalent oral pharmacological doses of resveratrol delay vascular aging but do not extend life span in rats. Atherosclerosis 224 (1), 136–142. doi: 10.1016/j.atherosclerosis.2012.06.007
Dikalova, A. E., Itani, H. A., Nazarewicz, R. R., McMaster, W. G., Flynn, C. R., Uzhachenko, R., et al. (2017). Sirt3 Impairment and SOD2 Hyperacetylation in Vascular Oxidative Stress and Hypertension. Circ. Res. 121 (5), 564–574. doi: 10.1161/CIRCRESAHA.117.310933
Ding, Y. N., Tang, X., Chen, H. Z., Liu, D. P. (2018). Epigenetic Regulation of Vascular Aging and Age-Related Vascular Diseases. Adv. Exp. Med. Biol. 1086, 55–75. doi: 10.1007/978-981-13-1117-8_4
Dzau, V. J., Antman, E. M., Black, H. R., Hayes, D. L., Manson, J. E., Plutzky, J., et al. (2006a). The cardiovascular disease continuum validated: clinical evidence of improved patient outcomes: part I: Pathophysiology and clinical trial evidence (risk factors through stable coronary artery disease). Circulation 114 (25), 2850–2870. doi: 10.1161/CIRCULATIONAHA.106.655688
Dzau, V. J., Antman, E. M., Black, H. R., Hayes, D. L., Manson, J. E., Plutzky, J., et al. (2006b). The cardiovascular disease continuum validated: clinical evidence of improved patient outcomes: part II: Clinical trial evidence (acute coronary syndromes through renal disease) and future directions. Circulation 114 (25), 2871–2891. doi: 10.1161/CIRCULATIONAHA.106.655761
Eto, H., Miyata, M., Shirasawa, T., Akasaki, Y., Hamada, N., Nagaki, A., et al. (2008). The long-term effect of angiotensin II type 1a receptor deficiency on hypercholesterolemia-induced atherosclerosis. Hypertens. Res. 31 (8), 1631–1642. doi: 10.1291/hypres.31.1631
Feinberg, A. P. (2018). The Key Role of Epigenetics in Human Disease Prevention and Mitigation. N. Engl. J. Med. 378 (14), 1323–1334. doi: 10.1056/NEJMra1402513
Fraga, M. F., Ballestar, E., Paz, M. F., Ropero, S., Setien, F., Ballestar, M. L., et al. (2005). Epigenetic differences arise during the lifetime of monozygotic twins. Proc. Natl. Acad. Sci. U. S. A. 102 (30), 10604–10609. doi: 10.1073/pnas.0500398102
Fry, J. L., Al Sayah, L., Weisbrod, R. M., VanRoy, I., Weng, X., Cohen, R. A., et al. (2016). Vascular Smooth Muscle Sirtuin-1 Protects Against Diet-Induced Aortic Stiffness. Hypertension 68 (3), 775–784. doi: 10.1161/HYPERTENSIONAHA.116.07622
Fu, Z., Wang, M., Gucek, M., Zhang, J., Wu, J., Jiang, L., et al. (2009). Milk fat globule protein epidermal growth factor-8: a pivotal relay element within the angiotensin II and monocyte chemoattractant protein-1 signaling cascade mediating vascular smooth muscle cells invasion. Circ. Res. 104 (12), 1337–1346. doi: 10.1161/CIRCRESAHA.108.187088
Fulop, T., Larbi, A., Dupuis, G., LePage, A., Frost, E. H., Cohen, A. A., et al. (2018). Immunosenescence and Inflamm-Aging As Two Sides of the Same Coin: Friends or Foes? Front. Immunol. 8:1960. doi: 10.3389/fimmu.2017.01960
Gracia-Sancho, J., Villarreal, G., Jr, Zhang, Y., García-Cardeña, G. (2010). Activation of SIRT1 by resveratrol induces KLF2 expression conferring an endothelial vasoprotective phenotype. Cardiovasc. Res. 85 (3), 514–519. doi: 10.1093/cvr/cvp337
Guay, S. P., Légaré, C., Houde, A. A., Mathieu, P., Bossé, Y., Bouchard, L. (2014). Acetylsalicylic acid, aging and coronary artery disease are associated with ABCA1 DNA methylation in men. Clin. Epigenet. 6 (1):14. doi: 10.1186/1868-7083-6-14
Gui, T., Zhou, G., Sun, Y., Shimokado, A., Itoh, S., Oikawa, K., et al. (2012). MicroRNAs that target Ca(2+) transporters are involved in vascular smooth muscle cell calcification. Lab. Invest. 92 (9), 1250–1259. doi: 10.1038/labinvest.2012.85
Gurha, P., Marian, A. J. (2013). Noncoding RNAs in cardiovascular biology and disease. Circ. Res. 113 (12), e115–e120. doi: 10.1161/CIRCRESAHA.113.302988
Hai, Z., Zuo, W. (2016). Aberrant DNA methylation in the pathogenesis of atherosclerosis. Clin. Chim. Acta 456, 69–74. doi: 10.1016/j.cca.2016.02.026
Handy, D. E., Castro, R., Loscalzo, J. (2011). Epigenetic modifications: basic mechanisms and role in cardiovascular disease. Circulation 123 (19), 2145–2156. doi: 10.1161/CIRCULATIONAHA.110.956839
Heerboth, S., Lapinska, K., Snyder, N., Leary, M., Rollinson, S., Sarkar, S. (2014). Use of epigenetic drugs in disease: an overview. Genet. Epigenet. 6, 9–19. doi: 10.4137/GEG.S12270
Hofmann, P., Sommer, J., Theodorou, K., Kirchhof, L., Fischer, A., Li, Y., et al. (2019). Long non-coding RNA H19 regulates endothelial cell aging via inhibition of STAT3 signalling. Cardiovasc. Res. 115 (1), 230–242. doi: 10.1093/cvr/cvy206
Hu, X., Zhang, K., Xu, C., Chen, Z., Jiang, H. (2014). Anti-inflammatory effect of sodium butyrate preconditioning during myocardial ischemia/reperfusion. Exp. Ther. Med. 8 (1), 229–232. doi: 10.3892/etm.2014.1726
Jeltsch, A. (2008). Reading and writing DNA methylation. Nat. Struct. Mol. Biol. 15 (10), 1003–1004. doi: 10.1038/nsmb1008-1003
Jia, W., Wu, W., Yang, D., Xiao, C., Su, Z., Huang, Z., et al. (2018a). Histone demethylase JMJD3 regulates fibroblast-like synoviocyte-mediated proliferation and joint destruction in rheumatoid arthritis. FASEB J. 32 (7), 4031–4042. doi: 10.1096/fj.201701483R
Jia, W., Wu, W., Yang, D., Xiao, C., Huang, M., Long, F., et al. (2018b). GATA4 regulates angiogenesis and persistence of inflammation in rheumatoid arthritis. Cell Death Dis. 9 (5), 503. doi: 10.1038/s41419-018-0570-5
Jiang, L., Zhang, J., Monticone, R. E., Telljohann, R., Wu, J., Wang, M., et al. (2012). Calpain-1 regulation of matrix metalloproteinase 2 activity in vascular smooth muscle cells facilitates age-associated aortic wall calcification and fibrosis. Hypertension 60 (5), 1192–1199. doi: 10.1161/HYPERTENSIONAHA.112.196840
Kadlec, A. O., Chabowski, D. S., Ait-Aissa, K., Gutterman, D. D. (2016). Role of PGC-1α in Vascular Regulation: Implications for Atherosclerosis. Arterioscler. Thromb. Vasc. Biol. 36 (8), 1467–1474. doi: 10.1161/ATVBAHA.116.307123
Kim, J., Kim, J. Y., Song, K. S., Lee, Y. H., Seo, J. S., Jelinek, J., et al. (2007). Epigenetic changes in estrogen receptor beta gene in atherosclerotic cardiovascular tissues and in-vitro vascular senescence. Biochim. Biophys. Acta 1772 (1), 72–80. doi: 10.1016/j.bbadis.2006.10.004
Kim, E. N., Kim, M. Y., Lim, J. H., Kim, Y., Shin, S. J., Park, C., et al. (2018). The protective effect of resveratrol on vascular aging by modulation of the renin-angiotensin system. Atherosclerosis 270, 123–131. doi: 10.1016/j.atherosclerosis.2018.01.043
Kohli, R. M., Zhang, Y. (2013). TET enzymes, TDG and the dynamics of DNA demethylation. Nature 502 (7472), 472–479. doi: 10.1038/nature12750
Krebs, J., Agellon, L. B., Michalak, M. (2015). Ca(2+) homeostasis and endoplasmic reticulum (ER) stress: An integrated view of calcium signaling. Biochem. Biophys. Res. Commun. 460 (1), 114–121. doi: 10.1016/j.bbrc.2015.02.004
Ku, W. L., Nakamura, K., Gao, W., Cui, K., Hu, G., Tang, Q., et al. (2019). Single-cell chromatin immunocleavage sequencing (scChIC-seq) to profile histone modification. Nat. Methods 16 (4), 323–325. doi: 10.1038/s41592-019-0361-7
Lakatta, E. G., Levy, D. (2003a). Arterial and cardiac aging: major shareholders in cardiovascular disease enterprises: Part I: aging arteries: a “set up” for vascular disease. Circulation 107 (1), 139–146. doi: 10.1161/01.cir.0000048892.83521.58
Lakatta, E. G., Levy, D. (2003b). Arterial and cardiac aging: major shareholders in cardiovascular disease enterprises: Part II: the aging heart in health: links to heart disease. Circulation 107 (2), 346–354. doi: 10.1161/01.cir.0000048893.62841.f7
Lakatta, E. G., Wang, M., Najjar, S. S. (2009). Arterial aging and subclinical arterial disease are fundamentally intertwined at macroscopic and molecular levels. Med. Clin. North Am. 93 (3), 583–Contents. doi: 10.1016/j.mcna.2009.02.008
Lakatta, E. G. (2003). Arterial and cardiac aging: major shareholders in cardiovascular disease enterprises: Part III: cellular and molecular clues to heart and arterial aging. Circulation 107 (3), 490–497. doi: 10.1161/01.cir.0000048894.99865.02
Lan, Y., Li, Y. J., Li, D. J., Li, P., Wang, J. Y., Diao, Y. P., et al. (2019). Long noncoding RNA MEG3 prevents vascular endothelial cell senescence by impairing miR-128-dependent Girdin downregulation. Am. J. Physiol. Cell Physiol. 316 (6), C830–C843. doi: 10.1152/ajpcell.00262.2018
Leopold, J. A. (2013). Vascular calcification: an age-old problem of old age. Circulation 127 (24), 2380–2382. doi: 10.1161/CIRCULATIONAHA.113.003341
Lin, X., Zhan, J. K., Zhong, J. Y., Wang, Y. J., Wang, Y., Li, S., et al. (2019). lncRNA-ES3/miR-34c-5p/BMF axis is involved in regulating high-glucose-induced calcification/senescence of VSMCs. Aging (Albany N. Y.) 11 (2), 523–535. doi: 10.18632/aging.101758
Liu, X. L., Zhang, P. F., Ding, S. F., Wang, Y., Zhang, M., Zhao, Y. X., et al. (2012). Local gene silencing of monocyte chemoattractant protein-1 prevents vulnerable plaque disruption in apolipoprotein E-knockout mice. PLoS One 7 (3), e33497. doi: 10.1371/journal.pone.0033497
Liu, Y., Wang, T. T., Zhang, R., Fu, W. Y., Wang, X., Wang, F., et al. (2016). Calorie restriction protects against experimental abdominal aortic aneurysms in mice. J. Exp. Med. 213 (11), 2473–2488. doi: 10.1084/jem.20151794
Liu, S., Wang, X., Pan, L., Wu, W., Yang, D., Qin, M., et al. (2018). Endogenous hydrogen sulfide regulates histone demethylase JMJD3-mediated inflammatory response in LPS-stimulated macrophages and in a mouse model of LPS-induced septic shock. Biochem. Pharmacol. 149, 153–162. doi: 10.1016/j.bcp.2017.10.010
Luo, X., Yang, D., Wu, W., Long, F., Xiao, C., Qin, M., et al. (2018). Critical role of histone demethylase Jumonji domain-containing protein 3 in the regulation of neointima formation following vascular injury. Cardiovasc. Res. 114 (14), 1894–1906. doi: 10.1093/cvr/cvy176
Ma, X., Wang, J., Li, J., Ma, C., Chen, S., Lei, W., et al. (2018). Loading MiR-210 in Endothelial Progenitor Cells Derived Exosomes Boosts Their Beneficial Effects on Hypoxia/Reoxygeneation-Injured Human Endothelial Cells via Protecting Mitochondrial Function. Cell Physiol. Biochem. 46 (2), 664–675. doi: 10.1159/000488635
Maegawa, S., Lu, Y., Tahara, T., Lee, J. T., Madzo, J., Liang, S., et al. (2017). Caloric restriction delays age-related methylation drift. Nat. Commun. 8 (1), 539. doi: 10.1038/s41467-017-00607-3
McGill, H. C., Jr, McMahan, C. A., Gidding, S. S. (2008). Preventing heart disease in the 21st century: implications of the Pathobiological Determinants of Atherosclerosis in Youth (PDAY) study. Circulation 117 (9), 1216–1227. doi: 10.1161/CIRCULATIONAHA.107.717033
McNulty, H., Pentieva, K., Hoey, L., Ward, M. (2008). Homocysteine, B-vitamins and CVD. Proc. Nutr. Soc 67 (2), 232–237. doi: 10.1017/S0029665108007076
Menghini, R., Casagrande, V., Cardellini, M., Martelli, E., Terrinoni, A., Amati, F., et al. (2009). MicroRNA 217 modulates endothelial cell senescence via silent information regulator 1. Circulation 120 (15), 1524–1532. doi: 10.1161/CIRCULATIONAHA.109.864629
Mikhed, Y., Daiber, A., Steven, S. (2015). Mitochondrial Oxidative Stress, Mitochondrial DNA Damage and Their Role in Age-Related Vascular Dysfunction. Int. J. Mol. Sci. 16 (7), 15918–15953. doi: 10.3390/ijms160715918
Mullican, S. E., Gaddis, C. A., Alenghat, T., Nair, M. G., Giacomin, P. R., Everett, L. J., et al. (2011). Histone deacetylase 3 is an epigenomic brake in macrophage alternative activation. Genes Dev. 25 (23), 2480–2488. doi: 10.1101/gad.175950.111
Novella, S., Heras, M., Hermenegildo, C., Dantas, A. P. (2012). Effects of estrogen on vascular inflammation: a matter of timing. Arterioscler. Thromb. Vasc. Biol. 32 (8), 2035–2042. doi: 10.1161/ATVBAHA.112.250308
Okano, M., Bell, D. W., Haber, D. A., Li, E. (1999). DNA methyltransferases Dnmt3a and Dnmt3b are essential for de novo methylation and mammalian development. Cell 99 (3), 247–257. doi: 10.1016/s0092-8674(00)81656-6
O’Rourke, M. F., Safar, M. E., Dzau, V. (2010). The Cardiovascular Continuum extended: aging effects on the aorta and microvasculature. Vasc. Med. 15 (6), 461–468. doi: 10.1177/1358863X10382946
Pan, M. H., Lai, C. S., Wu, J. C., Ho, C. T. (2013). Epigenetic and disease targets by polyphenols. Curr. Pharm. Des. 19 (34), 6156–6185. doi: 10.2174/1381612811319340010
Pollack, R. M., Crandall, J. P. (2013). Resveratrol: therapeutic potential for improving cardiometabolic health. Am. J. Hypertens. 26 (11), 1260–1268. doi: 10.1093/ajh/hpt165
Rothgiesser, K. M., Erener, S., Waibel, S., Lüscher, B., Hottiger, M. O. (2019). Correction: SIRT2 regulates NF-κB-dependent gene expression through deacetylation of p65 Lys310 (doi:10.1242/jcs.073783). J. Cell Sci. 132 (8), jcs232801. doi: 10.1242/jcs.232801
Schiano, C., Vietri, M. T., Grimaldi, V., Picascia, A., De Pascale, M. R., Napoli, C. (2015). Epigenetic-related therapeutic challenges in cardiovascular disease. Trends Pharmacol. Sci. 36 (4), 226–235. doi: 10.1016/j.tips.2015.02.005
Sen, P., Shah, P. P., Nativio, R., Berger, S. L. (2016). Epigenetic Mechanisms of Longevity and Aging. Cell 166 (4), 822–839. doi: 10.1016/j.cell.2016.07.050
Shahbazian, M. D., Grunstein, M. (2007). Functions of site-specific histone acetylation and deacetylation. Annu. Rev. Biochem. 76, 75–100. doi: 10.1146/annurev.biochem.76.052705.162114
Takemura, A., Iijima, K., Ota, H., Son, B. K., Ito, Y., Ogawa, S., et al. (2011). Sirtuin 1 retards hyperphosphatemia-induced calcification of vascular smooth muscle cells. Arterioscler. Thromb. Vasc. Biol. 31 (9), 2054–2062. doi: 10.1161/ATVBAHA.110.216739
Tan, Q., Christiansen, L., Thomassen, M., Kruse, T. A., Christensen, K. (2013). Twins for epigenetic studies of human aging and development. Ageing Res. Rev. 12 (1), 182–187. doi: 10.1016/j.arr.2012.06.004
Tan, P., Wang, H., Zhan, J., Ma, X., Cui, X., Wang, Y., et al. (2019). Rapamycinߛinduced miRߛ30a downregulation inhibits senescence of VSMCs by targeting Beclin1. Int. J. Mol. Med. 43 (3), 1311–1320. doi: 10.3892/ijmm.2019.4074
Tarun, A., Antoniades, C. (2018). The era of cardiovascular epigenetics: histone deacetylases and vascular inflammation. Cardiovasc. Res. 114 (7), 928–930. doi: 10.1093/cvr/cvy099
Tian, H., Liu, C., Zou, X., Wu, W., Zhang, C., Yuan, D. (2015). MiRNA-194 Regulates Palmitic Acid-Induced Toll-Like Receptor 4 Inflammatory Responses in THP-1 Cells. Nutrients 7 (5), 3483–3496. doi: 10.3390/nu7053483
Tsaprouni, L. G., Yang, T. P., Bell, J., Dick, K. J., Kanoni, S., Nisbet, J., et al. (2014). Cigarette smoking reduces DNA methylation levels at multiple genomic loci but the effect is partially reversible upon cessation. Epigenetics 9 (10), 1382–1396. doi: 10.4161/15592294.2014.969637
Van Camp, G. (2014). Cardiovascular disease prevention. Acta Clin. Belg. 69 (6), 407–411. doi: 10.1179/2295333714Y.0000000069
Ventura, A., Luzi, L., Pacini, S., Baldari, C. T., Pelicci, P. G. (2002). The p66Shc longevity gene is silenced through epigenetic modifications of an alternative promoter. J. Biol. Chem. 277 (25), 22370–22376. doi: 10.1074/jbc.M200280200
Voelter-Mahlknecht, S. (2016). Epigenetic associations in relation to cardiovascular prevention and therapeutics. Clin. Epigenet. 8, 4. doi: 10.1186/s13148-016-0170-0
Wan, Y. Z., Gao, P., Zhou, S., Zhang, Z. Q., Hao, D. L., Lian, L. S., et al. (2014). SIRT1-mediated epigenetic downregulation of plasminogen activator inhibitor-1 prevents vascular endothelial replicative senescence. Aging Cell 13 (5), 890–899. doi: 10.1111/acel.12247
Wang, M., Khazan, B., Lakatta, E. G. (2010a). Central Arterial Aging and Angiotensin II Signaling. Curr. Hypertens. Rev. 6 (4), 266–281. doi: 10.2174/157340210793611668
Wang, M., Monticone, R. E., Lakatta, E. G. (2010b). Arterial aging: a journey into subclinical arterial disease. Curr. Opin. Nephrol. Hypertens. 19 (2), 201–207. doi: 10.1097/MNH.0b013e3283361c0b
Winnik, S., Auwerx, J., Sinclair, D. A., Matter, C. M. (2015). Protective effects of sirtuins in cardiovascular diseases: from bench to bedside. Eur. Heart J. 36 (48), 3404–3412. doi: 10.1093/eurheartj/ehv290
Wu, X. Y., Fan, W. D., Fang, R., Wu, G. F. (2014). Regulation of microRNA-155 in endothelial inflammation by targeting nuclear factor (NF)-κB P65. J. Cell Biochem. 115 (11), 1928–1936. doi: 10.1002/jcb.24864
Wu, C. L., Wang, Y., Jin, B., Chen, H., Xie, B. S., Mao, Z. B. (2015). Senescence-associated Long Non-coding RNA (SALNR) Delays Oncogene-induced Senescence through NF90 Regulation. J. Biol. Chem. 290 (50), 30175–30192. doi: 10.1074/jbc.M115.661785
Wu, W., Qin, M., Jia, W., Huang, Z., Li, Z., Yang, D., et al. (2019). Cystathionine-γ-lyase ameliorates the histone demethylase JMJD3-mediated autoimmune response in rheumatoid arthritis. Cell Mol. Immunol. 16 (8), 694–705. doi: 10.1038/s41423-018-0037-8
Xu, S., Kamato, D., Little, P. J., Nakagawa, S., Pelisek, J., Jin, Z. G. (2019). Targeting epigenetics and non-coding RNAs in atherosclerosis: from mechanisms to therapeutics. Pharmacol. Ther. 196, 15–43. doi: 10.1016/j.pharmthera.2018.11.003
Yang, D., Xiao, C., Long, F., Su, Z., Jia, W., Qin, M., et al. (2018). HDAC4 regulates vascular inflammation via activation of autophagy. Cardiovasc. Res. 114 (7), 1016–1028. doi: 10.1093/cvr/cvy051
Yang, D., Xiao, C., Long, F., Wu, W., Huang, M., Qu, L., et al. (2019). Fra-1 plays a critical role in angiotensin II-induced vascular senescence. FASEB J. 33 (6), 7603–7614. doi: 10.1096/fj.201801671RRRR
Yue, Y., Liu, J., He, C. (2015). RNA N6-methyladenosine methylation in post-transcriptional gene expression regulation. Genes Dev. 29 (13), 1343–1355. doi: 10.1101/gad.262766.115
Keywords: vascular aging, epigenetics, cell senescence, inflammation, oxidation stress, calcification
Citation: Ding Q, Shao C, Rose P and Zhu YZ (2020) Epigenetics and Vascular Senescence–Potential New Therapeutic Targets? Front. Pharmacol. 11:535395. doi: 10.3389/fphar.2020.535395
Received: 15 February 2020; Accepted: 31 August 2020;
Published: 29 September 2020.
Edited by:
Cheng Xue Helena Qin, Monash University, AustraliaReviewed by:
Hou-Zao Chen, Chinese Academy of Medical Sciences, ChinaChristina Yan Ru Tan, Agency for Science, Technology and Research (A*STAR), Singapore
Copyright © 2020 Ding, Shao, Rose and Zhu. This is an open-access article distributed under the terms of the Creative Commons Attribution License (CC BY). The use, distribution or reproduction in other forums is permitted, provided the original author(s) and the copyright owner(s) are credited and that the original publication in this journal is cited, in accordance with accepted academic practice. No use, distribution or reproduction is permitted which does not comply with these terms.
*Correspondence: Yi Zhun Zhu, yzzhu@must.edu.mo; Chunhong Shao, chunhongshao@fudan.edu.cn