NRP2 as an Emerging Angiogenic Player; Promoting Endothelial Cell Adhesion and Migration by Regulating Recycling of α5 Integrin
- 1School of Biological Sciences, University of East Anglia, Norwich Research Park, Norwich, United Kingdom
- 2Gut Microbes and Health, Quadram Institute Bioscience, Norwich Research Park, Norwich, United Kingdom
- 3Faculty of Medicine and Health Sciences, University of East Anglia, Norwich Research Park, Norwich, United Kingdom
Angiogenesis relies on the ability of endothelial cells (ECs) to migrate over the extracellular matrix via integrin receptors to respond to an angiogenic stimulus. Of the two neuropilin (NRP) orthologs to be identified, both have been reported to be expressed on normal blood and lymphatic ECs, and to play roles in the formation of blood and lymphatic vascular networks during angiogenesis. Whilst the role of NRP1 and its interactions with integrins during angiogenesis has been widely studied, the role of NRP2 in ECs is poorly understood. Here we demonstrate that NRP2 promotes Rac-1 mediated EC adhesion and migration over fibronectin (FN) matrices in a mechanistically distinct fashion to NRP1, showing no dependence on β3 integrin (ITGB3) expression, or VEGF stimulation. Furthermore, we highlight evidence of a regulatory crosstalk between NRP2 and α5 integrin (ITGA5) in ECs, with NRP2 depletion eliciting an upregulation of ITGA5 expression and disruptions in ITGA5 cellular organization. Finally, we propose a mechanism whereby NRP2 promotes ITGA5 recycling in ECs; NRP2 depleted ECs were found to exhibit reduced levels of total ITGA5 subunit recycling compared to wild-type (WT) ECs. Our findings expose NRP2 as a novel angiogenic player by promoting ITGA5-mediated EC adhesion and migration on FN.
Introduction
Neuropilins (NRPs) are single non-tyrosine kinase receptors belonging to a family of type I transmembrane glycoproteins (MW ∼130–140 kDa) (Soker et al., 1998). To date, two NRP orthologs have been identified in vertebrates, NRP1 and NRP2, both of which share a very similar domain structure and an overall 44% amino acid homology (Pellet-Many et al., 2008; Zachary, 2014). Their expression and function have been identified in many cell types, including nerve cells, endothelial cells (ECs), epithelial cells, immune cells, osteoblasts and tumor cells (Bielenberg et al., 2004; Zachary, 2014). In ECs, it is believed that NRPs play an essential role in sprouting angiogenesis and lymphogenesis through the selective binding to members of the vascular endothelial growth factor (VEGF) family. Following this complex formation, NRPs function as co-receptors with VEGFRs to enhance the VEGF-induced activation of many intracellular pathways (Prahst et al., 2008). As such, the functions of NRP have been implicated in influencing cell adhesion, migration and permeability during angiogenesis, under both physiological and pathological conditions (Fukasawa et al., 2007; Valdembri et al., 2009; Ellison et al., 2015). Studies have, however, provided evidence to suggest that NRPs can mediate ligand signaling independently of VEGFRs, in addition to regulating VEGFRs independently of VEGF binding. Based on early transgenic mouse studies in this field, it was originally speculated that NRP1 is mainly expressed on arteries, arterioles and capillaries, whereas NRP2 is expressed on veins, venules and lymphatic vessels (Herzog et al., 2001; Yuan et al., 2002). However, subsequent studies have revealed that both NRPs are expressed in normal blood and lymphatic ECs, and both play essential roles in forming blood and lymphatic vasculature networks (Bouvree et al., 2012; Jurisic et al., 2012; Mucka et al., 2016). Despite this, investigations into elucidating the roles of endothelial NRPs during angiogenesis have, for the most part, focused on NRP1 (Zachary, 2014). With regard to NRP2, studies have instead focused on annotating a role in cancer cells, where its upregulation is consistent with cancer progression in a number of cell types [e.g., neuroblastomas (Fakhari et al., 2002), non-small cell lung carcinoma [NSCLC] (Kawakami et al., 2002), human prostate carcinoma, melanoma (Bielenberg et al., 2004), lung cancer (Tomizawa et al., 2001; Kawakami et al., 2002; Lantuejoul et al., 2003), myeloid leukemia (Vales et al., 2007), breast cancer (Bachelder et al., 2001) and pancreatic cancer (Fukahi et al., 2004)]. Interestingly, Favier et al. (2006) recapitulated this upregulation of NRP2 observed in cancer cells by overexpressing NRP2 in human microvascular ECs (hMVECs), and found that cell survival in these ECs was significantly increased following stimulation with either VEGF-A- or VEGF-C. Furthermore, NRP2 knockdown significantly inhibited both VEGF-A- and VEGFC-induced migration, suggesting that NRP2 as a potential pharmacological target. Crucially, this work highlighted the importance of understanding the cross-talk between NRP2 and other receptors (mainly VEGF, integrins and plexins) in ECs, which will aid in the design of novel drugs to better control the mechanisms underlying angiogenesis and lymphangiogenesis in autoimmune diseases and tumor development (Favier et al., 2006). We have previously described a link between NRP1 and the β3-integrin (ITGB3) subunit during VEGF-induced angiogenesis. We reported that complete loss of the Itgb3 gene enhanced EC permeability through the upregulation of VEGF-VEGFR2-ERK1/2 signaling (Robinson et al., 2004), and that NRP1 and ERK1/2 expression was elevated in ITGB3-NULL ECs. Subsequent targeting of NRP1 in ITGB3-NULL mice revealed a significant inhibition of VEGF-induced angiogenesis compared to WT mice, indicating that the elevation of angiogenesis in the absence of the Itgb3 gene is dependent on NRP1 expression (Robinson et al., 2009; Ellison et al., 2015). In this study we aimed to investigate whether NRP2 shares a similar interaction with ITGB3 or other integrins in ECs.
Results
NRP2 Function Is Not Regulated by ITGB3 During VEGFR2-Mediated Signaling or Migration Over FN Matrices
We previously showed the involvement of NRP1 during VEGF-stimulated angiogenesis to be dependent upon ITGB3 in ECs (Ellison et al., 2015). Due to the structural homology between NRP1 and NRP2 (Zachary, 2014), we decided to first consider whether, like NRP1, NRP2’s function shares a dependency on ITGB3 during VEGF-mediated angiogenic responses in ECs. To investigate this, we isolated mouse lung microvascular endothelial cells (mLMECs) from both wild-type (WT) and ITGB3-heterozygous (β3HET) mice and immortalized them with polyoma-middle-T antigen (PyMT) by retroviral transduction. As in previous studies, we decided to use β3HET cells for these analyses, rather than β3-integrin knockout (β3NULL) cells, because we have shown they are a good model for studying the role of αvβ3-integrin in cell migration, whilst evading changes arising from the complete loss of the integrin on both alleles (e.g., up-regulated VEGFR2 expression) (Ellison et al., 2015; Atkinson et al., 2018). DNA was extracted from multiple immortalized lines and analyzed by PCR to confirm their genetic status as either WT or β3HET cells (Supplementary Figure S1A). We subsequently confirmed the EC identity of each immortalized line by examining the expression of EC markers including VE-Cadherin (Sivarapatna et al., 2015; Ikuno et al., 2017), in addition to quantifying the expression of ITGB3 in β3HET ECs. Each clone was confirmed to express canonical EC markers, and all β3HET ECs were confirmed to express approximately 50% ITGB3 compared to their WT counterparts (Supplementary Figures S1B–D). We reported previously that in β3HET ECs, NRP1 expression is upregulated, and that NRP1 appeared only to play a role in post development angiogenesis when ITGB3 expression is reduced (Ellison et al., 2015). Western blot quantification of NRP2 expression between WT and β3HET protein lysates showed a similar elevation in NRP2 expression in β3HET ECs (Figure 1A), suggesting the existence of a regulatory nexus between NRP2 and ITGB3, which we felt warranted further exploration.
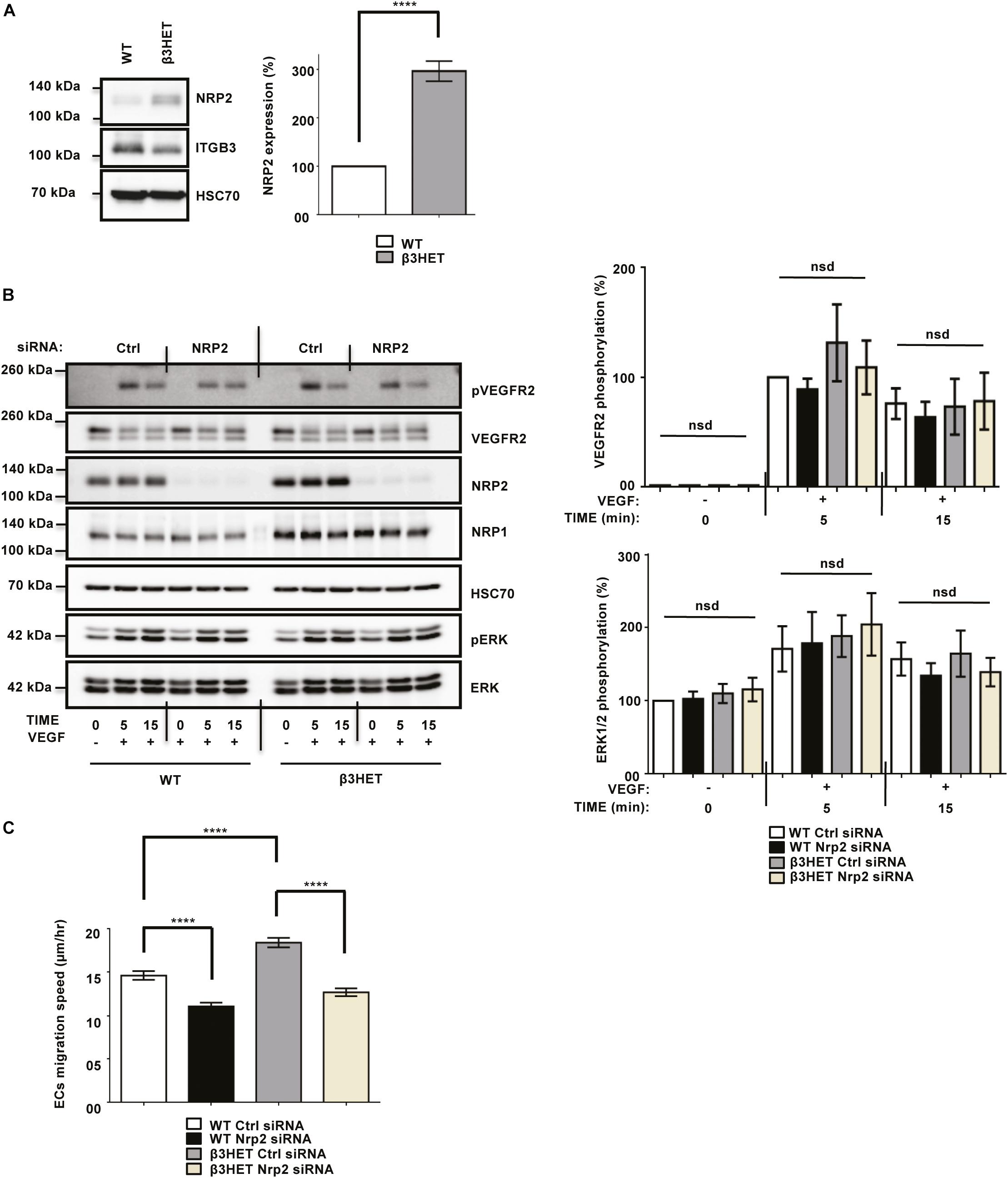
Figure 1. NRP2 is not regulated by ITGB3 during VEGFR2-mediated signaling or during migration over FN. (A): WT and β3HET ECs were plated onto FN and incubated for 48 h at 37°C and 5% CO2. EC extracts were immunoblotted using antibodies to NRP2, ITGB3 and HSC70. N = 3 independent experiments, ****P < 0.0001. (B,C): siRNA-transfected ECs were plated onto FN and incubated for 48 h at 37°C and 5% CO2. ECs were subsequently starved in serum-free media and stimulated with VEGF (30 ng/ml) for the indicated timepoints. EC extracts were immunoblotted using antibodies to phospho-VEGFR2, VEGFR2, NRP2, NRP1, HSC70, phospho-ERK and ERK. N = 6 independent experiments, P > 0.05 (nsd). Left panel shows representative Western blot images, right panel shows densitometric analysis of band intensities normalized against HSC70 and obtained using ImageJTM. Asterisks indicate statistical significance, nsd indicates no significant difference from unpaired two-tailed t-tests. (C): siRNA-transfected ECs were seeded onto FN and incubated for 3 h at 37°C and 5% CO2. Fixed images were taken every 10 min for 15 h at 37°C and 5% CO2 using an inverted Zeiss Axiovert microscope with one-phase contrast. Random migration speed was quantified using the ImageJTM plugin mTrackJ in μm/hour. N = 4 independent experiments, n ≥ 30 ECs per experimental condition, ****P < 0.0001.
As NRP2 has been shown to regulate VEGF-induced signaling in both human lymphatic (Caunt et al., 2008), and lymphatic microvascular ECs, we examined whether NRP2 regulates proangiogenic signaling responses to VEGF and if the effects are dependent on ITGB3. Using NRP2 specific siRNA (Supplementary Figure S1E) versus a control siRNA transfected into our WT and β3HET ECs, we measured differences in VEGFR2 and ERK phosphorylation over a time course of 15 min. We observed only marginally attenuated VEGFR2 and ERK phosphorylation by Western blot analysis in response to NRP2 silencing and, importantly, saw no differences between WT and β3HET ECs (Figure 1B), suggesting that ITGB3 does not regulate NRP2 dependent VEGF induced signaling in our cells.
To explore the possibility of a regulatory axis between NRP2 and ITGB3 further, we examined cellular migration. Angiogenesis relies on the ability of ECs to respond to angiogenic stimuli by migrating over an extracellular matrix (ECM). We have shown previously that NRP1 plays a role in promoting EC migration over fibronectin (FN) matrices, but only when ITGB3 levels are reduced (Ellison et al., 2015). Independent studies have also described NRP2 silencing to inhibit migration of human microvascular ECs (Favier et al., 2006) and human lymphatic ECs (Caunt et al., 2008). We therefore chose to examine the effects of NRP2 depletion on EC migration over FN in our mLMECs, and to determine whether any effect is dependent upon ITGB3 expression. To achieve this, control and NRP2 siRNA transfected WT and β3HET ECs were plated on FN and random migration speed was measured by time-lapse microscopy over 15 h. As previously reported, we observed β3HET ECs migrate faster over a FN matrix than WT ECs (Ellison et al., 2015). However, unlike NRP1, whilst depletion of NRP2 significantly reduced EC migration speed, it did so independently of ITGB3 expression (Figure 1C). We therefore conclude that ITGB3 does not regulate NRP2 function during these angiogenic processes.
Depletion of NRP2 in ECs Disrupts Adhesion to FN Matrices
Whilst the upregulation of NRP2 expression we observe in β3HET ECs suggests a regulatory crosstalk between NRP2 and ITGB3 (Figure 1A), NRP2 regulated signaling and migration show no dependence on ITGB3. As NRP2 depletion significantly impaired the ability of ECs to migrate over FN (Figure 1C), but not to undergo proliferation (Supplementary Figure S2A) we next chose to directly examine the effect of NRP2 depletion on cell adhesion to FN. To do this we compared the relative number of cells adhered to 96-well plates pre-coated with FN for either 15 or 30 min. At both timepoints significantly fewer cells adhered to FN following NRP2 siRNA treatment compared to control siRNA treated ECs (Figure 2A and Supplementary Figure S2B). Migration is dependent on the ability of cells to adhere to a matrix via the formation of FA complexes that continuously cycle through phases of assembly and disassembly. FAs comprise a module of recruited intracellular proteins, such as paxillin and integrins, that link to the actin cytoskeleton to mediate mechanical changes in the cell (Small et al., 1998; Webb et al., 2002; Valdembri and Serini, 2012; De Pascalis and Etienne-Manneville, 2017). Whilst NRP2 depletion had no effect on total or phosphorylated levels of paxillin in our ECs (Figure 2B), we did observe it to negatively impact the rate of focal adhesion (FA) turnover itself. FA assembly and disassembly were monitored in ECs treated with either control or NRP2 siRNAs, transfected with paxillin-GFP, by time-lapse microscopy for 30 min over a FN matrix. Silencing of NRP2 in mLMECs significantly reduced the rate of both FA assembly and disassembly compared to control siRNA treated cells (Figure 2C). We also measured FA number and size distribution in ECs using two different NRP2 siRNAs by immunolabelling for endogenous paxillin in cells that had adhered to FN for 90 min, a time that allows for mature FAs to form (Ridley et al., 2003; Schiller et al., 2013; Atkinson et al., 2018). Despite no significant difference in average cell area (Supplementary Figure S2C), FAs were significantly fewer in number and smaller in average size in NRP2 siRNA treated cells compared to control siRNA treated ECs (Figure 2D, Supplementary Figure S2D), suggesting NRP2 depletion inhibits FA maturation. Finally, we considered whether NRP2 promotes EC adhesion and migration on FN by regulating Rac1 activation. Rac1 is a small Rho GTPase that mediates cell motility following integrin engagement, regulating leading edge cytoskeletal dynamics and promoting the formation of FAs (Nobes and Hall, 1995; Price et al., 1998; Suzuki-Inoue et al., 2001; Ridley, 2011). Using a recombinant PBD-domain protein (PAK-1) fused to glutathione-magnetic beads, we captured the relative abundance of active Rac1-GTP in control and NRP2 siRNA treated ECs stimulated for 180 min on FN. Compared to our control siRNA treated ECs, NRP2 depleted ECs exhibited a significantly reduced level of active Rac1 (Figure 2E), suggesting that NRP2 promotes EC adhesion and migration on FN by regulating Rac1 activation.
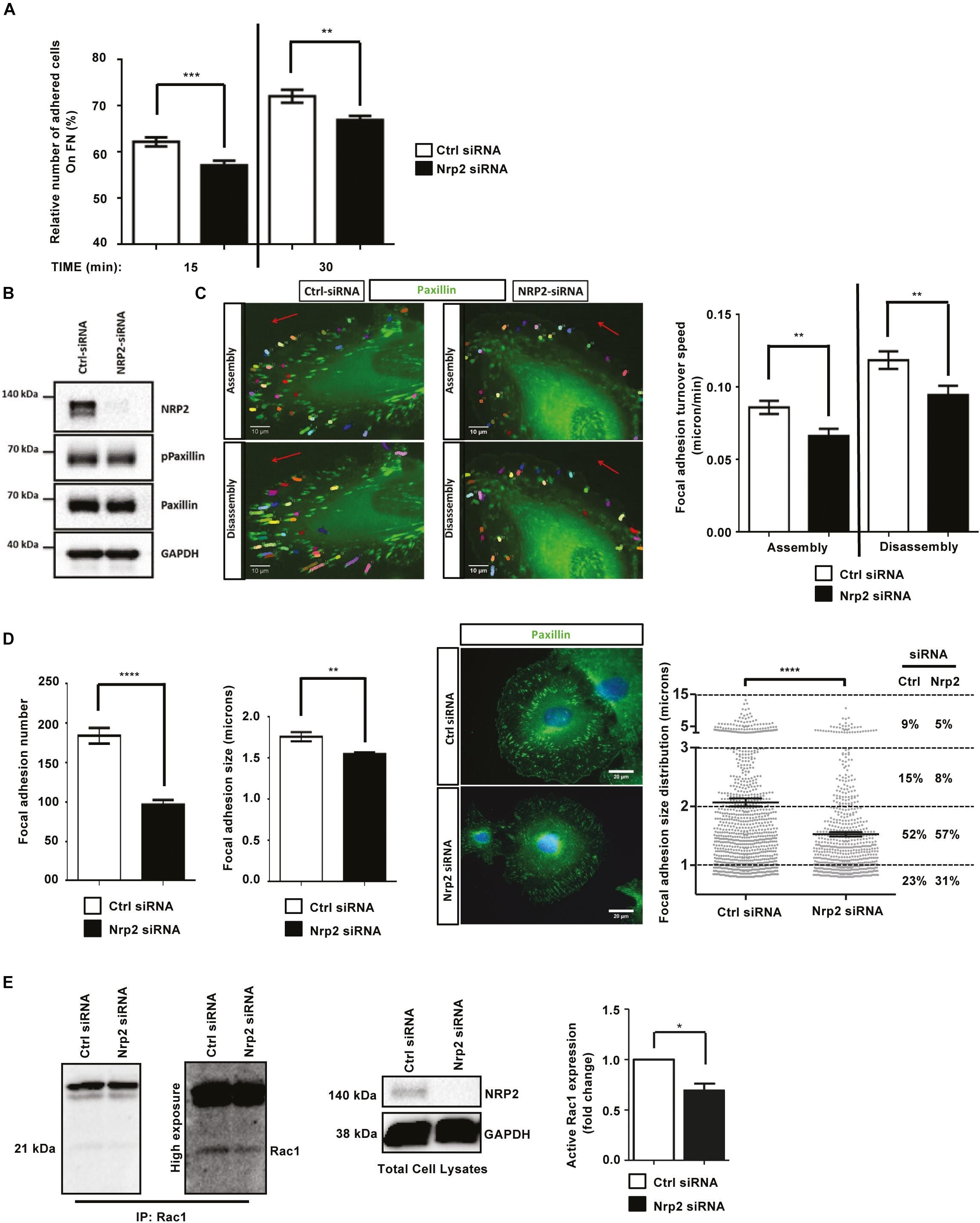
Figure 2. Depletion of NRP2 in ECs disrupts migration and adhesion to FN matrices by affecting Rac1 activation (A): siRNA-transfected ECs were seeded onto FN coated 96-well plates pre-blocked with 5% bovine serum albumin (BSA), and incubated for either 15 or 30 min at 37°C and 5% CO2. ECs were subsequently fixed in 4% PFA and stained with methylene blue. Absorbance was read at 630 nm. Data was normalized to the relative number of the total cells seeded in a 3-h incubation control plate. N = 3 independent experiments, **P < 0.01, ***P < 0.001. Asterisks indicate statistical significance from unpaired two-tailed t-tests. (B) siRNA-transfected ECs were plated onto FN and incubated for 48 h at 37°C and 5% CO2. EC lysates were immunoblotted using antibodies to NRP2, phospho-paxillin, paxillin and GAPDH. (C) siRNA-transfected ECs were double transfected with a GFP paxillin construct, seeded onto FN and incubated for 48 h at 37°C and 5% CO2. ECs were fixed in a Ludin chamber and live imaged at 37°C and 5% CO2 using an inverted Axiovert microscope in which an individual cell was captured every one minute for 30 min (left panels). Focal adhesion (FA) assembly and disassembly speeds were analyzed using the ImageJTM plugin mTrackJ in μm/min. In each respective bar, n ≥ 100 FAs, **P < 0.01 (right panel). (D) siRNA-transfected ECs were seeded onto FN and incubated for 90 min at 37°C and 5% CO2 before being fixed and stained for paxillin. Images were taken using a Zeiss AxioImager M2 microscope at 63x magnification. FA number and size was quantified using ImageJTM software as previously described by Lambert et al. (2020). A FA size lower detection limit was set at 0.8 microns. The center panel shows representative images for fixed ECs transfected either with control or NRP2 siRNA. Image quantification for FA number and size is shown in the left panel. Quantification performed on mean data from n ≥ 25 ECs over N = 3 independent experiments. % FA size distribution analysis is shown in the right panel on mean data from n ≥ 25 ECs over N = 3 independent experiments. Asterisks indicate statistical significance from an unpaired two-tailed t-test. (E) siRNA-transfected ECs were seeded onto FN and incubated for 180 min at 37°C and 5% CO2. EC extracts were immunoprecipitated by incubation with 10 μg Rac1 assay reagent (PAK-1 PBD magnetic beads) for 45 min at 4°C with gentle agitation. Immunoprecipitated complexes were subjected to Western blot analysis using antibodies against Rac1. Left panel: low and high exposure images showing active Rac1 levels in control and NRP2 siRNA transfected lysate. Middle panel: NRP2 depletion was confirmed by Western blot analysis using antibodies against NRP2 and GAPDH. Right panel: densitometric analysis of band intensities normalized against GAPDH and obtained using ImageJTM. Asterisks indicate statistical significance from an unpaired two-tailed t-test.
NRP2 Regulates ITGA5 Expression in ECs
Cells adhere to the ECM via heterodimeric integrin receptors, which are recruited to FA complexes during cell migration (Avraamides et al., 2008; Goel et al., 2012) and which activate Rho family GTPases such as Rac1 (Price et al., 1998; Suzuki-Inoue et al., 2001). α5β1 integrin is the principle FN binding integrin in ECs (Valdembri et al., 2009; Valdembri and Serini, 2012), and has been previously described as being upregulated during developmental angiogenesis to promote EC migration and survival (Kim et al., 2002; Goodman and Picard, 2012). Studies have also shown NRP1, through its cytoplasmic SEA motif, to specifically promote α5β1 integrin-mediated EC adhesion to FN matrices in a VEGF independent fashion (Valdembri et al., 2009; Cao et al., 2013). As NRP2 depletion significantly impaired mLMEC migration and adhesion on FN, and given the structural homology shared between NRP1 and NRP2, we considered whether a similar association exists between NRP2 and α5β1 integrin. First, we examined whether NRP2 knockdown regulated the expression of either integrin subunit. Western blot analysis revealed that siRNA-mediated silencing of NRP2 resulted in a significant upregulation of ITGA5 subunit expression in four different EC lines (Figure 3A), whilst β1-integrin (ITGB1) expression remained unchanged (data not shown). Whilst endothelial ITGA5 specifically pairs with ITGB1 (Avraamides et al., 2008), ITGB1 can form heterodimers with α subunits 1–9 (with the exception of α7) (Velling et al., 1996; Hynes, 2002), suggesting α5β1-integrin behavior can be studied by examining ITGA5 discretely (e.g., ITGB1 expression profiles are sum measures of its interactions with multiple α subunits present in the cell). Therefore, we chose to subsequently focus our attentions on ITGA5 and its interplay with NRP2.
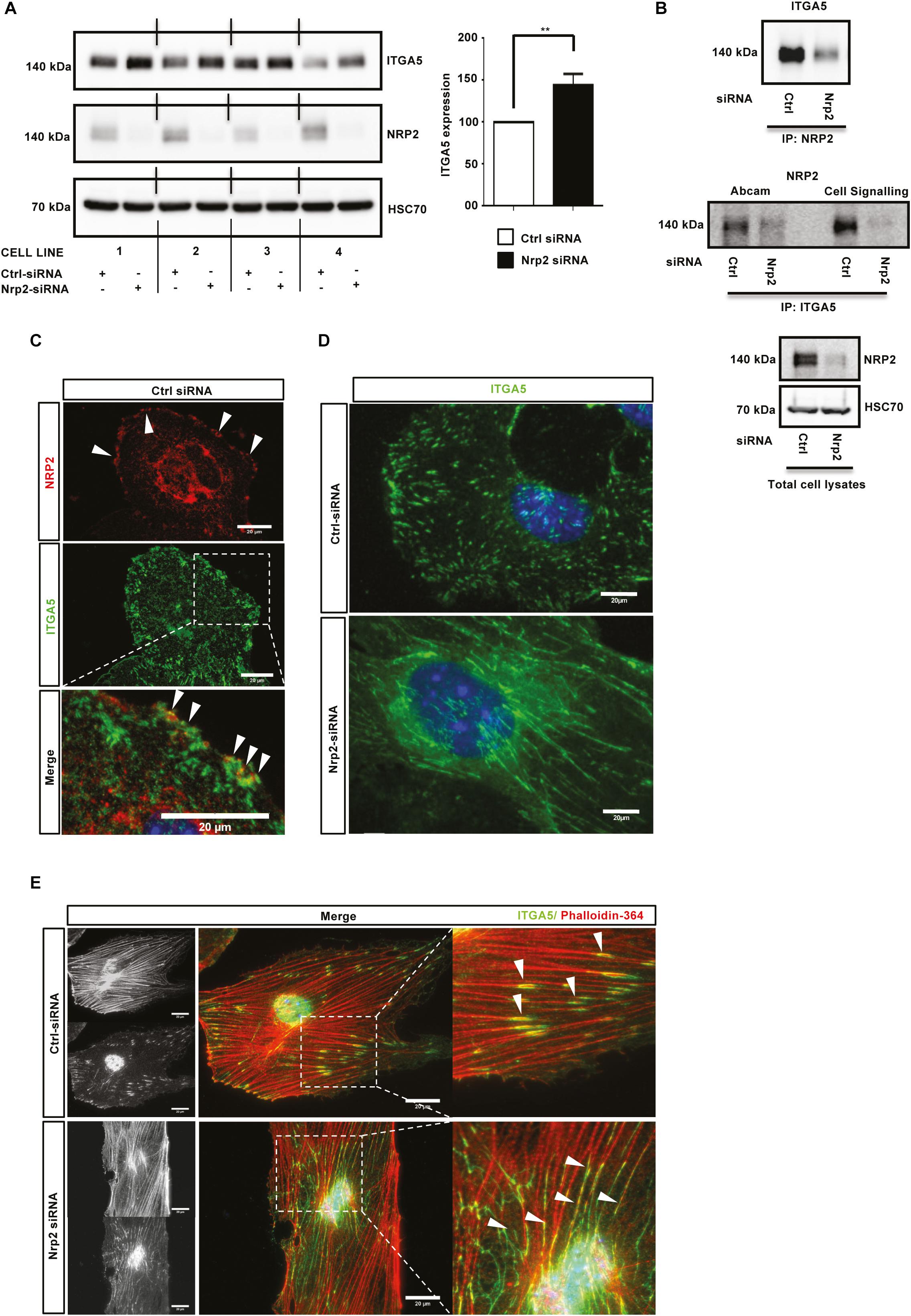
Figure 3. NRP2 regulates ITGA5 expression in ECs. (A): siRNA-transfected ECs from four different clones were seeded onto FN and incubated for 48 h. EC extracts were immunoblotted using antibodies to ITGA5, NRP2, and HSC70. Left panel shows Western blot image, right panel shows densitometric analysis of ITGA5 band intensities normalized against HSC70 and obtained using ImageJTM. **P < 0.01. (B): siRNA-transfected ECs were seeded onto FN and incubated for 48 h. Left panel: EC extracts were immunoprecipitated by incubation with protein-G Dynabeads® coupled to a NRP2 antibody. Middle panel: EC extracts were immunoprecipitated by incubation with protein-G Dynabeads® coupled to two different ITGA5 antibodies (as indicated). Immunoprecipitated complexes were subjected to Western blot analysis using antibodies against ITGA5 and NRP2, respectively. Right panel: NRP2 silencing was confirmed by subjecting the total cell lysate to Western blot analysis and incubating blots in antibodies against NRP2 and HSC70. (C): Control siRNA-transfected ECs were prepared as described in Figure 2D legend. Fixed ECs were incubated in primary antibodies against NRP2 and ITGA5. Images were captured using a Zeiss AxioImager M2 microscope at 63x magnification. Arrows indicate co-localization of NRP2 and ITGA5. (D): siRNA-transfected ECs were prepared as described in Figure 2D legend, however, ECs were allowed to adhere overnight prior to fixation. ECs were incubated in primary antibody against ITGA5. (E) siRNA-transfected ECs were prepared as described in (D), however, fixed cells were incubated in ITGA5 primary antibody and phalloidin-364. Arrows indicate localization of ITGA5 with actin filaments. Image panels shown in (C–E) are representative of n ≥ 10 cells per condition/treatment.
Studies have previously reported NRP1 to complex with ITGA5 in HUVECs (Valdembri et al., 2009) and NRP2 to complex with ITGA5 from co-cultures between HUVECs and renal cell carcinoma (Cao et al., 2013). To investigate whether a direct interaction between NRP2 and ITGA5 exists in mLMECs, lysates were subjected to a series of co-immunoprecipitation studies followed by Western blot analysis. We found both NRP2 and ITGA5 co-immunoprecipitate with each other, indicating a physical interaction between the two receptors (Figure 3B). Immuno-staining using a highly specific NRP2 antibody, reported previously not to cross-react with NRP1 (Bae et al., 2008; Goel et al., 2012), also showed a strong co-localization between NRP2 and ITGA5 close to the cell membrane (Figure 3C). Given the significant upregulation of ITGA5 subunit expression following NRP2 depletion, and evidence to suggest a physical interaction between NRP2 and ITGA5, we next examined whether NRP2 depletion elicited an effect on ITGA5 localization within our immortalized cells. Following treatment with either control or NRP2 siRNA, ECs were seeded overnight on a FN matrix and subsequently fixed to visualize endogenous ITGA5 expression. Compared to control siRNA treated ECs, NRP2 depleted ECs exhibited significant disruptions in ITGA5 organization: ITGA5 appeared in elongated fibrillar-like structures (Figure 3D), reminiscent of what has been described as fibrillar adhesions (Webb et al., 2002). ImageJTM analysis of these ITGA5 containing structures confirmed a significant increase in the length of ITGA5 fibrils in NRP2 siRNA treated ECs suggestive of a disruption in ITGA5 trafficking (Supplementary Figure S3A). We see this NRP2-dependent ITGA5 phenotype when using multiple NRP2 specific siRNAs (Supplementary Figure S3B) and in primary ECs (Supplementary Figures S3C,D). It is becoming increasingly clear that microtubule-actin highways co-ordinately regulate a range of intracellular trafficking mechanisms, including integrin transport (Goley and Welch, 2006; Kaksonen et al., 2006; Valdembri et al., 2009; Zech et al., 2011; Duleh and Welch, 2012). Co-immunostaining for both phalloidin and ITGA5 revealed that whilst ITGA5 localized to the ends of actin filaments in control treated ECs, at what we assume to be FAs, in NRP2 depleted cells, the elongated ITGA5 fibrils share a strong co-localization along the actin filaments themselves (Figure 3E). We believe this to support a mechanism of actin-dependent ITGA5 trafficking, whereby loss of NRP2 impedes the transport of ITGA5 to FAs at the leading edge of the cell.
ITGA5 Trafficking Is Dependent on NRP2 in ECs
Whilst a role for NRP2 in trafficking ITGA5 is novel, NRP1 has been shown previously to promote endocytosis of active α5β1 integrin through a Rab5 pathway (Valdembri et al., 2009). In order to take an unbiased approach to elucidating candidate trafficking proteins NRP2 may associate with to regulate ITGA5 localization in ECs, we used label-free quantitative (LFQ) mass spectrometry. Mass spectrometry was performed on two WT EC lines, transfected either with control or NRP2 siRNA, and immunoprecipitated for NRP2. siRNA-mediated depletion of NRP2 was confirmed for both cell lines. This analysis revealed proteins immunoprecipitating with NRP2 at a significantly increased fold-change compared to proteins analyzed from a NRP2 knockdown cell-lysate. Shown are protein hits detected in both cell lines, including both ITGA5 and ITGB1. A number of endocytic trafficking proteins were also detected as candidate binding partners of NRP2, including clathrin, caveolin-1, lamtor1, scamp1, and annexin-A1 (Figure 4A). The full list of identified co-immunoprecipitated proteins is shown in Supplementary Table S1.
α5β1 integrin is known to be internalized both by clathrin and caveolae-mediated endocytosis (Shi and Sottile, 2008; Margadant et al., 2011). In order to validate a potential mechanism by which NRP2 regulates ITGA5 internalization via its contact with clathrin and caveolin, we performed coimmunoprecipitation assays to prove a physical interaction. NRP2 coimmunoprecipitated with both clathrin heavy chain-1 and caveolin-1 (Figure 4B), supporting the mass spectrometry results. We subsequently conducted cell surface biotinylation assays to examine ITGA5 internalization directly, in ECs treated either with control siRNA or NRP2 siRNA. We observed no change in the rate of ITGA5 internalization in NRP2 depleted ECs (Figure 4C), suggesting that NRP2 does not regulate internalization of total ITGA5 levels. We subsequently considered whether NRP2 regulates ITGA5 recycling back to the membrane.
Integrin recycling is mediated by Rab GTPase proteins (Roberts et al., 2001; Paul et al., 2015), specifically, α5β1 integrin follows long-loop recycling via a Rab11 dependent mechanism (Laukaitis et al., 2001; Caswell et al., 2007). NRP2 coimmunoprecipitated with Rab11 in both directions (Figure 4D). However, NRP2 did not co-immunoprecipitate with NRP1 in our mass spec studies (see Supplementary Table S1), suggesting these two structurally related proteins regulate ITGA5 trafficking through distinct pathways. As our mass spectrometry analysis had identified candidate interactions between NRP2 and other trafficking and recycling molecules, such as Rab11Fip5, an adaptor for Rab11 recycling vesicles and previously reported to co-immunoprecipitate with ITGA5, we performed biotin recycling assays. In these assays, the biotin-labeled cell surface proteins were allowed to internalize before stripping off any remaining surface biotin. Cells were then incubated over 20 min to stimulate the recycling process. In contrast to our internalization assays, NRP2 silencing significantly attenuated the rate of total ITGA5 recycling back to the membrane compared to ECs treated with control siRNA (Figure 4E). We therefore present a potential mechanism whereby NRP2 promotes mLMEC migration and adhesion to FN by regulating actin dependent ITGA5 recycling back to the cell membrane (Figure 5).
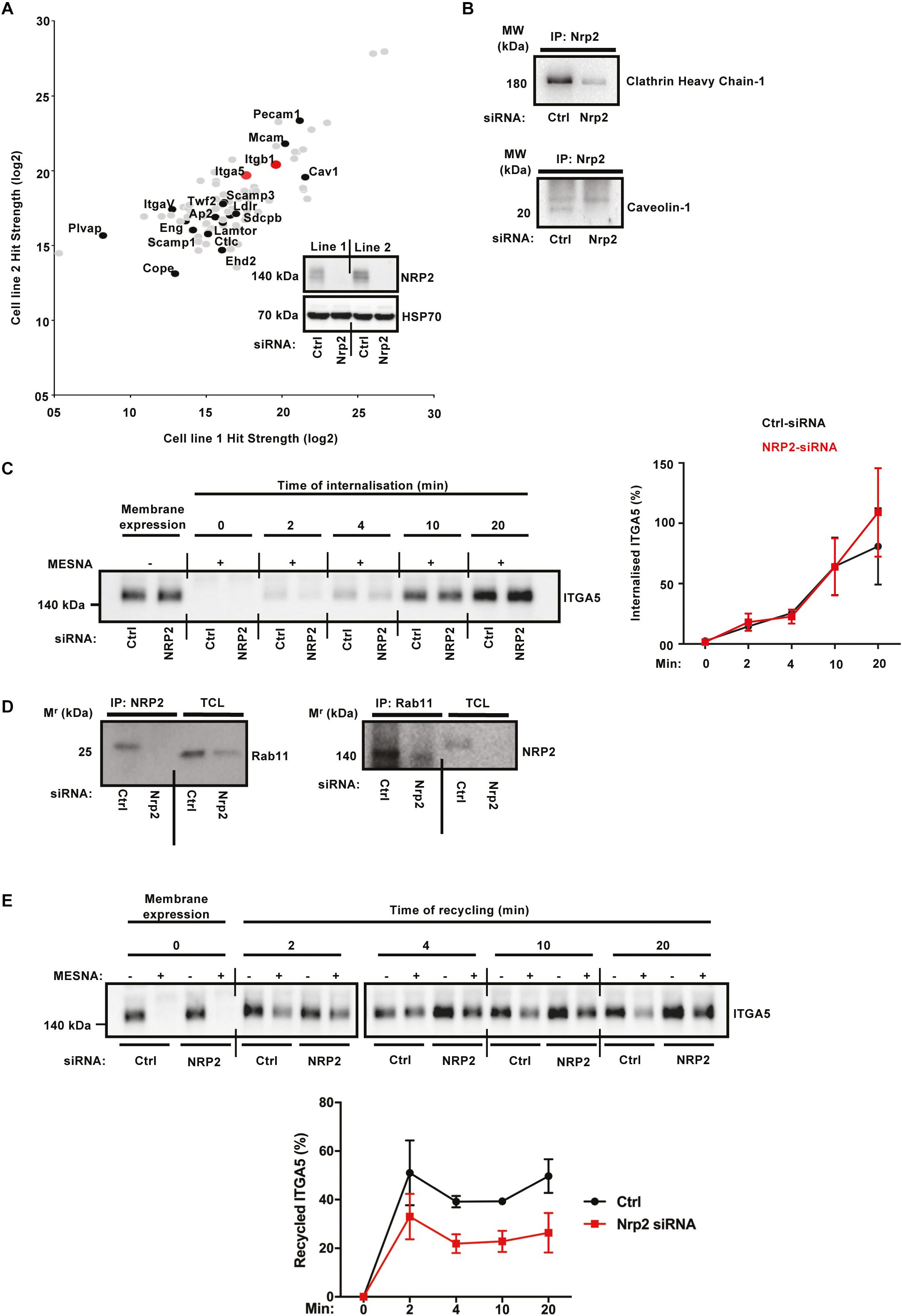
Figure 4. ITGA5 trafficking in ECs is NRP2 dependent. (A): Label-free quantitative mass spectrometry peptide hits identified using MaxQuant software from the Andromeda peptide database. Panel depicts peptide hits detected at a higher fold-change in two control siRNA treated EC lines than those detected from two NRP2 siRNA treated EC lines. NRP2 silencing was confirmed by subjecting EC extracts to Western blot analysis. (B): siRNA-transfected ECs were seeded onto FN and incubated for 48 h. EC extracts were immunoprecipitated by incubation with protein-G Dynabeads® coupled to a NRP2 antibody. Immunoprecipitated complexes were subjected to Western blot analysis using antibodies against clathrin heavy chain-1 (top) and caveolin-1 (bottom). (C): siRNA-transfected ECs were seeded onto FN and incubated for 48 h. ECs were subsequently starved in serum-free media, before being placed on ice. EC cell surface proteins were labeled with 0.3 mg/ml biotin. ECs were then incubated for the indicated timepoints at 37°C, 5% CO2. A sample of ECs were maintained at 4°C as (±Mesna) controls. Following incubation at 37°C, ECs were placed on ice and incubated with 100 mM Mesna. EC lysates were then immunoprecipitated with protein-G Dynabeads® coupled to an anti-biotin antibody. Immunoprecipitated biotin-labeled proteins were separated by SDS-PAGE and subjected to Western bolt analysis. The level of internalized ITGA5 at each time of incubation was normalized to the (-) Mesna control. Left panel shows Western blot image, right panel shows the mean densitometric analysis obtained using ImageJTM. Also shown is confirmation of NRP2 silencing. N = 3 independent experiments. (D): Co-immunoprecipitation study as described in (B), immunoprecipitated complexes were subjected to Western blot analysis using anti-Rab11 and anti-NRP2 antibodies, respectively. (E): siRNA-transfected ECs were prepared as described in (C), after biotin surface labeling, ECs were incubated in serum free media for 20 min at 37°C to allow for internalization. A sample of ECs were maintained at 4°C for use as positive/negative controls. The remaining ECs were then placed on ice, and any un-internalized biotin-labeled proteins stripped off using 100 mM Mesna. The internalized protein fraction was allowed to recycle by incubating the ECs for the indicated timepoints at 37°C. ECs were then returned to ice and incubated in 100 mM Mesna. No Mesna treatment dishes at each timepoint were used as comparative controls. All subsequent stages were performed in the same manner as described in (C), the level of the recycled ITGA5 was determined by normalizing the amount of ITGA5 quantified from dishes treated with Mesna to the total ITGA5 on the membranes of the Mesna-untreated cells in the same period of incubation. N = 3 independent experiments.
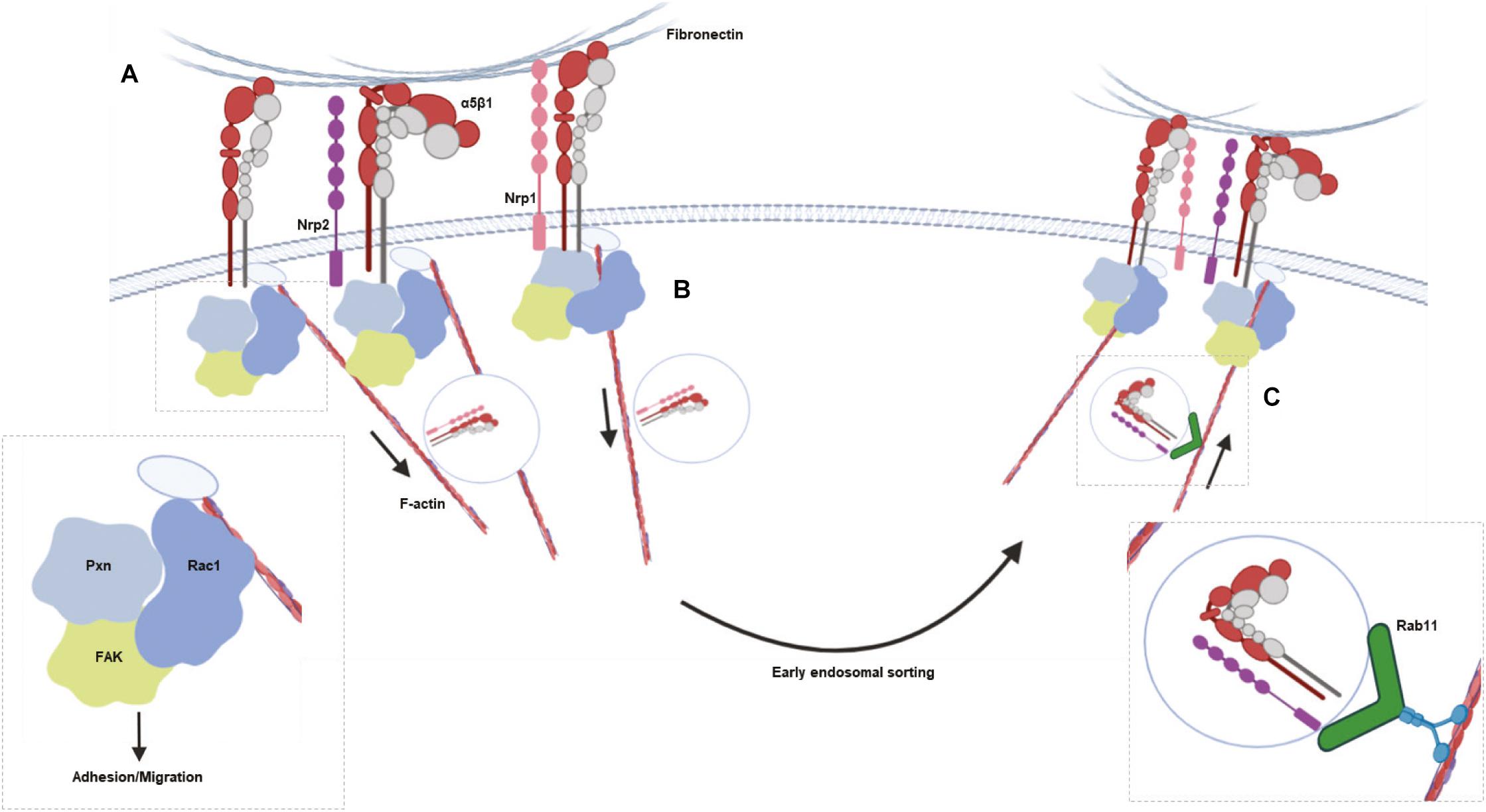
Figure 5. Schematic summarizing the major results. (A), NRP2 and ITGA5 share an interaction at the cell membrane, promoting EC adhesion and migration on FN matrices by regulating Rac1 activation. (B), NRP1 is known to selectively stimulate internalization of active α5β1 integrin from fibrillar adhesions along F-actin microfilaments to early endosomes in a Rab5 dependent manner. (C) NRP2 then regulates Rab11 dependent recycling of total ITGA5 back to adhesion sites along the actin cytoskeleton.
Discussion
NRP2 is known to regulate ECM adhesion and migration in various cell lines, including human ECs (Favier et al., 2006), immune cells (Curreli et al., 2007) and cancer cells (Kawakami et al., 2002; Fukahi et al., 2004; Caunt et al., 2008; Dallas et al., 2008; Cao et al., 2013). In the latter, it has been described as a biomarker for poor prognosis because its expression correlates with increased migratory and invasive behavior (Kawakami et al., 2002; Caunt et al., 2008; Dallas et al., 2008; Cao et al., 2013). In many of these examples, NRP2 promotes cell adhesion and migration by exerting influence over VEGF-mediated pro-angiogenic pathways and inducing integrin engagement. ITGA5 and FN are known to be upregulated in ECs during angiogenesis (Kim et al., 2002; Goodman and Picard, 2012), and are key players in formation of neo-vasculature (Brooks et al., 1994; Kim et al., 2000). The data we present here support a model whereby NRP2 promotes EC adhesion and migration on FN by regulating actin-dependent ITGA5 recycling. Importantly, the means by which it does so appears to be mechanistically distinct from that of NRP1, which is known to regulate internalization of active ITGA5 via a rab5-dependent pathway (Valdembri et al., 2009).
We previously demonstrated an ITGB3-dependent role for NRP1 in mediating EC migration and FA turnover on FN. EC migration on FN becomes NRP1 dependent when ITGB3 levels are perturbed (Ellison et al., 2015). Like NRP1, NRP2 expression is upregulated in ITGB3 depleted cells (Figure 1A). Given this observation, and the structural and domain homologies between NRP1 and NRP2 (Zachary, 2014), we sought to investigate whether NRP2 shared a similar regulatory interplay with ITGB3. We demonstrated by siRNA knockdown that EC migration depends on NRP2, but this is independent of ITGB3 expression levels (Figure 1C).
Like NRP1, NRP2 also regulates a number of integrin-dependent cellular processes on FN. Upon NRP2 knockdown, EC adhesion is reduced (Figure 2A), FA dynamics are altered (Figures 2C,D), and Rac1 activation is impaired (Figure 2E). Rac1 was also identified from our mass spectrometry analysis as a candidate binding partner of NRP2, which supports these findings. Integrins provide the structural link that allows for: (1) adhesion to the ECM; (2) anchorage of actin stress fibers to the membrane; (3) the generation of force that is required for migration (Laukaitis et al., 2001; Le Boeuf et al., 2004; Buskermolen et al., 2018). Both αvβ3- and α5β1-integrins are FN receptors in ECs (Brooks et al., 1994; Kim et al., 2000), but given the lack of any ITGB3 input into the NRP2 processes we were examining, and the upregulation of ITGA5 expression upon NRP2 depletion (Figure 3A), we focused our attention on this integrin subunit. Cao et al. (2013) demonstrated NRP2 to interact with ITGA5 in co-cultures between HUVECs and renal cell carcinoma to promote FN-mediated adhesion. To our knowledge, though, we are the first to show evidence of a direct interaction between NRP2 and ITGA5 in microvascular ECs (Figures 3B,C). Furthermore, our findings demonstrate that NRP2 modulates ITGA5 function by regulating its subcellular trafficking along the actin cytoskeleton. Importantly, the disrupted ITGA5 phenotype we see following NRP2 depletion in our immortalized ECs is also present in primary ECs. Along with our previous studies demonstrating they express a canonical adhesome (Atkinson et al., 2018), this rebuts the concept that immortalized ECs are an inappropriate model for studying angiogenic processes.
NRP2’s role in trafficking ITGA5 in ECs also appears to be distinct from that of NRP1. It has been reported that NRP1 regulates active α5β1 integrin endocytosis via GIPC1 (Valdembri et al., 2009), however, interactions between GIPC and NRP2 were not detected from either mass spectrometry analysis or co-immunoprecipitation studies (data not shown). Although we did not observe any changes in internalization of total ITGA5 upon NRP2 depletion (Figure 4C), we were unable to examine trafficking of active α5β1 in our murine cells. In fact, we observed physical interactions between NRP2 and both clathrin and caveolin by immunoprecipitation (Figure 4B) suggesting NRP2 at least has the potential to regulate the endocytosis of active α5β1 integrin. Following internalization by clathrin or caveolin-dependent mechanisms, α5β1 integrin has been shown to undergo long-loop recycling back to the membrane within Rab11 positive vesicles (Laukaitis et al., 2001; Caswell et al., 2007). To our knowledge neither NRP1 or NRP2 have been reported to regulate ITGA5 recycling in microvascular ECs. Not only do we show NRP2 depletion slows total ITGA5 recycling (Figure 4D), we also show NRP2 co-immunoprecipitates with Rab11 in our ECs (Figure 4E).
Finally, the elevated total cellular levels of ITGA5 that occur when NRP2 is knocked-down, particularly in light of no changes at the cell surface (data not shown), suggest other points in the ITGA5 life cycle are governed by NRP2. Given NRP2’s interactions with clathrin and caveolin, both known to play a role in endosomal trafficking to lysosomes (Sachse et al., 2002; Shi and Sottile, 2008; Margadant et al., 2011), we speculate a role for the molecule in regulating ITGA5 degradation. We support this hypothesis by showing NRP2 also immunoprecipitates with both Lamtor1 and Scamp1 (Figure 4A), proteins previously shown to regulate lysosomal trafficking (Cai et al., 2011; Malek et al., 2012).
In close, we propose a novel mechanism by which NRP2, independently of its role as a coreceptor for VEGF-A, promotes Rac1-mediated EC adhesion and migration to FN matrices by regulating recycling of ITGA5. Importantly, we provide evidence to suggest that NRP2 acts in a mechanistically distinct manner to NRP1. Finally, whilst we have not yet shown any phenotypic interactions between NRP2 and ITGB3, we cannot rule out a role for ITGB3 in regulating NRP2 trafficking. Like NRP1, NRP2 levels are significantly elevated when ITGB3 expression is reduced (Figure 1A). Our findings allude to a complex interplay between FN-binding integrins and neuropilins which regulate EC migration.
Materials and Methods
Animal Generation
All experiments were performed in accordance with United Kingdom home office regulations and the European Legal Framework for the Protection of Animals used for Scientific Purposes (European Directive 86/609/EEC), prior to the start of this project. Transgenic mice expressing a knockout for the β3-integrin allele (KO-Itgb3) were generated by substituting a 1.4 kb HindIII fragment of the β3 gene including exons I and II with a 1.7 kb construct containing a Pgk-neomycin (neo)-resistance cassette (Supplementary Figure S1G). The PCR analysis was carried out using the following oligonucleotide primers as previously described by Hodivala-Dilke et al. (1999). Forward primer 1: 5′-CTTAGACACCTGCTACGGGC-3′ Reverse primer 2: 5′-CACGAGACTAGTGAGACGTG-3′.
Cell Isolation, Immortalization and Cell Culture
Primary mouse lung microvascular endothelial cells (mLMECs) were isolated from adult mice bred on a mixed C57BL6/129 background. Primary ECs were twice positively selected for their expression of intracellular adhesion molecule-2 (ICAM-2) by magnetic activated cell sorting (MACS) as previously described by Reynolds and Hodivala-Dilke (Reynolds and Hodivala-Dilke, 2006). ECs were immortalized using polyoma-middle-T-antigen (PyMT) retroviral transfection as previously described by Robinson et al. (2009). Immortalized mLMECs were cultured in IMMLEC media, a 1:1 mix of Ham’s F-12:DMEM medium (low glucose) supplemented with 10% FBS, 100 units/mL penicillin/streptomycin (P/S), 2 mM glutamax, 50 μg/mL heparin (Sigma). Immortalized mLMECs were cultured on 0.1% gelatin coated flasks at 37°C in a humidified incubator with 5% CO2. For experimental analyses, plates, dishes, flasks and coverslips were coated in 10 μg/ml human plasma fibronectin (FN) (Millipore) overnight at 4°C. Vascular endothelial growth factor-A (VEGF-A164: mouse equivalent of VEGF-A165) was made in-house as previously described by Krilleke et al. (2007).
siRNA Transfection
ECs were transfected with non-targeting control siRNA or mouse-specific NRP2 siRNA constructs (Dharmacon), suspended in nucleofection buffer (200 mM Hepes, 137 mM NaCl, 5 mM KCl, 6 mM D-glucose, and 7 mM Na2HPO4 in nuclease-free water) using either the Amaxa nucleofector system II (Lonza) under nucleofection program T-005 or the Amaxa 4Dnucleofector system (Lonza) under nucleofection program EO-100 according to manufacturer’s instructions. For the majority of our studies we used siGENOME mouse Nrp2 siRNA D-040423-03 (denoted as Nrp2 siRNA on figures). We confirmed key observations with siGENOME mouse Nrp2 siRNA D-040423-04 (denoted as Nrp2 siRNA#4 on figures).
Western Blot Analysis
siRNA transfected ECs were seeded into FN-coated 6-well plates at a seeding density of 5 × 105 cells/well and incubated for 48 h at 37°C in a 5% CO2 incubator. ECs were lysed in electrophoresis sample buffer (ESB) (Tris–HCL: 65 mM pH 7.4, sucrose: 60 mM, 3% SDS), and homogenized using a Tissue Lyser (Qiagen) with acid-washed glass beads (Sigma). Following protein quantification using the DC BioRad assay, 30 μg of protein from each sample was loaded onto 8% polyacrylamide gels and subjected to SDS-PAGE. Proteins were transferred to a nitrocellulose membrane (Sigma) and incubated in 5% milk powder in PBS 0.1% Tween-20 (0.1% PBST) for 1 h at room temperature followed by an overnight incubation in primary antibody diluted 1:1000 in 5% bovine serum albumin (BSA) in 0.1% PBST at 4°C. Membranes were washed 3× with 0.1% PBST and incubated in an appropriate horseradish peroxidase (HRP)-conjugated secondary antibody (Dako) diluted 1:2000 in 5% milk powder in 0.1% PBST for 2 h at room temperature. Membranes were washed again 3× with 0.1% PBST before being incubated with Pierce ECL Western Blotting Substrate solution (Thermo Scientific). Chemiluminescence was detected on a ChemiDocTM MP Imaging System darkroom (BioRad). Densitometric readings of band intensities for blots were obtained using ImageJTM. Primary antibodies (all used at 1:1000 dilution and purchased from Cell Signaling Technology, unless noted otherwise) were: anti-NRP2 (clone D39A5), anti-ITGB3 (clone 4702S), anti-HSC70 (clone B-6, Santa Cruz Biotechnology), anti-phospho VEGFR2 (Y1175) (clone 2478), anti-VEGFR2 (clone 2479), anti-NRP1 (clone 3725S), anti-phospho ERK1/2 (clone 9101), anti-ERK1/2 (clone 4695), anti-ITGA5 (clone 4705S), anti-ITGB1 (clone ab179471, Abcam), anti-clathrin heavy chain-1 (clone ab21679, Abcam), anti-caveolin-1 (clone ab18199, Abcam), anti-Rab11 (clone 3539), ERG: Ab92513, Pecam-1 (77699), prox-1 (clone ab11941, Abcam), claudin-5 (clone ab131259, Abcam), VE-cadherin (clone ab205336, Abcam).
Signaling Assays
siRNA-transfected ECs were seeded into FN-coated 6 cm cultures dishes at a density of 5 × 105 cells/well and incubated for 48 h at 37°C and 5% CO2. ECs were then PBS washed and starved for 3 h in serum free medium (OptiMEM®; Invitrogen). VEGF was then added at a final concentration of 30 ng/ml. After the desired time of VEGF-stimulation, ECs were subjected to lysing, protein quantification and protein expression analysis by Western blot.
Random Migration Assays
siRNA-transfected ECs were seeded into FN coated 24-well plates at a density of 7 × 104 cells/well 24 h post nucleofection, and allowed to adhere for 3 h at 37°C and 5% CO2. Fixed images of multiple fields/well were taken every 10 min for 15 h at 37°C and 5% CO2 using an inverted Zeiss Axiovert microscope with one-phase contrast. Random migration was quantified by manually tracking individual cells using the ImageJTM plugin mTrackJ. Random migration speed was calculated in μm/hour.
Colorimetric Adhesion Assays
siRNA-transfected ECs were seeded into 96-well plates at a density of 4 × 104 cells/well 48 h post nucleofection. 96-well plates were pre-coated with FN overnight, then blocked in 5% BSA for 1 h at room temperature. ECs were then incubated at 37°C in a 5% CO2 incubator for the indicated timepoints, in addition to a 3-h incubation control plate. Following incubation, ECs were washed 3× with PBS + 1 mM MgCl2 + 1 mM CaCl2, fixed in 4% PFA, and stained with methylene blue for 30 min at room temperature. ECs were washed in dH2O and air-dried, before the dye from stained adhered ECs was extracted by a de-stain solution (50% ethanol, 50% 0.1 M HCL). The absorbance of each well was then read at 630 nm. Data was normalized to the relative number of the total cells seeded in the 3-h incubation plate.
Focal Adhesion Turnover Assays
ECs double transfected with control or NRP2 siRNA and a GFP-tagged paxillin construct (kindly provided by Professor Maddy Parsons, Kings College, London) were seeded onto FN-coated acid-washed, oven sterilized glass coverslips in 24-well plates at a seeding density of 4 × 104 cells/well. 48 h post nucleofection, coverslips were PBS washed, fixed in a Ludin chamber (Life Imaging Services GmbH), and live imaged in OptiMEM® phenol-red free medium supplemented with 2% FBS and P/S at 37°C and 5% CO2 using an inverted Axiovert (Carl Zeiss Ltd.) microscope in which an individual cell was captured every one minute for 30 min. Focal adhesion (FA) assembly and disassembly speeds were analyzed by manually tracking the number of selected GFP-paxillin-positive focal adhesions using the Image JTM MTrackJ plugin software.
Immunocytochemistry
siRNA-transfected ECs were seeded onto FN-coated acid-washed, oven sterilized glass coverslips in 24-well plates at a seeding density of 2.5 × 104 cells/well, and incubated at 37°C and 5% CO2. ECs were fixed at indicated timepoints in 4% paraformaldehyde (PFA) for 10 min, washed in PBS, blocked and permeabilized with 10% goat serum, PBS 0.3% triton X-100 for 1 h at room temperature. Cells were incubated in primary antibody diluted 1:100 in PBS overnight at 4°C. Primary antibodies were: anti-paxillin (clone ab32084; Abcam), anti-ITGA5 (clone ab150361; Abcam). Coverslips were PBS washed, and incubated with donkey anti-rabbit Alexa fluor-488 secondary antibody diluted 1:200 in PBS for 2 h at room temperature. F actin staining was performed by incubating cells in phalloidin-564 diluted 1:40 in PBS for 2 h at room temperature during secondary antibody incubation. Coverslips were PBS washed again, before being mounted onto slides with Prolong® Gold containing DAPI (Invitrogen). Images were captured using a Zeiss AxioImager M2 microscope (AxioCam MRm camera) at 63× magnification. FA number and size was quantified using ImageJTM software as previously described by Lambert et al. (2020). A FA size lower detection limit was set at 0.8 microns. ITGA5 length was measured using the ImageJTM software plugin simple neurite tracer.
Rac1 Pulldown
siRNA-transfected ECs were seeded onto FN at a density of 2 × 105 cells/dish and incubated for 180 min at 37°C and 5% CO2. ECs were lysed on ice with MLB (Sigma) (diluted to 1× with sterile water containing 10% glycerol and 1× HaltTM protease inhibitor cocktail (Thermo Scientific). EC lysates were cleared by centrifugation. EC extracts were immunoprecipitated by incubation with 10 μg Rac1 assay reagent (PAK-1 PBD magnetic beads, Sigma) for 45 min at 4°C with gentle agitation. Immunoprecipitated complexes were subjected to Western blot analysis. Nitrocellulose membranes were immunoblotted using 1 μg/mL of anti-Rac1, clone 23A8 (Sigma).
Co-Immunoprecipitation Assays
siRNA-transfected ECs were seeded into FN-coated 10 cm dishes at a density of 2 × 106 cells/dish, and incubated for 48 h at 37°C and 5% CO2. ECs were then lysed on ice in lysis buffer as previously described by Valdembri et al. (2009) in the presence of 1× Halt protease inhibitor cocktail (Thermo Scientific) and protein quantified using the DC BioRad assay. 100 μg protein from each sample was immunoprecipitated by incubation with protein-G Dynabeads® (Invitrogen) coupled to a rabbit anti-NRP2 antibody (clone 3366, Cell Signaling Technology) on a rotator overnight at 4°C. Immunoprecipitated complexes were then washed 3× with lysis buffer + 1× HaltTM protease inhibitor cocktail, and once in PBS, before being added to and boiled in NuPAGE sample reducing agent and sample buffer (Life Technologies) for Western blot analysis.
Co-localization Assays
siRNA-transfected ECs were prepared the same as for immuno-cytochemistry. Cells were incubated in primary antibody diluted 1:50 in PBS overnight at 4°C. Primary antibodies were: anti-NRP2 (clone sc-13117, Santa Cruz Biotechnology), and anti-ITGA5 (clone ab150361; Abcam). Coverslips were PBS washed, and incubated with both donkey anti-rabbit Alexa fluor-488, and goat anti-mouse Alexa fluor-546 secondary antibodies diluted 1:200 in PBS for 2 h at room temperature. Coverslips were PBS washed again, before being mounted onto slides with Prolong® Gold containing DAPI (Invitrogen). Images were captured using a Zeiss AxioImager M2 microscope (AxioCam MRm camera) at 63× magnification.
Mass Spectrometry Analysis
NRP2 co-immunoprecipitation samples were sent to Fingerprints Proteomics Facility (Dundee University, United Kingdom), which carried out LFQ mass spectrometry and peptide identification using the MaxQuant software based on the Andromeda peptide database as described by Schiller et al. (2011). Figure 4A depicts peptide hits detected at a higher fold-change in two control siRNA treated EC lines than those detected from two NRP2 siRNA treated EC lines.
Internalization and Recycling Assays
Internalization
siRNA-transfected ECs were seeded into FN-coated 10 cm dishes at a density of 2 × 106 cells/dish, and incubated for 48 h at 37°C and 5% CO2. ECs were then starved in serum-free OptiMEM® for 3 h at 37°C in a 5% CO2 incubator, before being placed on ice for 5 min, then washed twice with Soerensen buffer (SBS) pH 7.8 (14.7 mM KH2PO4, 2 mM Na2HPO4, and 120 mM Sorbitol pH 7.8) as previously described by Remacle et al. (2003). EC cell surface proteins were labeled with 0.3 mg/ml biotin (Thermo Scientific) in SBS for 30 min at 4°C. Unreacted biotin was quenched with 100 mM glycine for 10 min at 4°C. ECs were then incubated in pre-warmed serum-free OptiMEM® for the indicated time points at 37°C in a 5% CO2 incubator. A sample of ECs were maintained at 4°C for use as positive/negative (±Mesna) controls. Following incubation, dishes were immediately placed on ice, washed twice with SBS pH 8.2, and incubated with 100 mM Mesna (Sigma) for 75 min at 4°C (with the exception of Mesna control plates, which were lysed in lysis buffer (25mM Tris–HCl, pH 7.4, 100 mM NaCl, 2mM MgCl2, 1mM Na3VO4, 0.5 mM EGTA, 1% Triton X-100, 5% glycerol, and protease inhibitors), and placed on ice). Following Mesna incubation, excess Mesna was quenched with 100mM iodoacetamide (Sigma) for 10 min at 4°C, then ECs were washed twice with SBS pH 8.2 and lysed. Lysates were cleared by centrifugation at 12,000 g for 20 min at 4°C. Supernatant proteins were then quantified using the DC BioRad assay, and subsequently immunoprecipitated with Dynabeads coupled to mouse anti-biotin antibody overnight at 4°C. Immunoprecipitated biotin-labeled proteins were separated by SDS-PAGE and subjected to Western bolt analysis. The level of internalized ITGA5 at each time of incubation was normalized to the (− Mesna) control.
Recycling
After surface labeling, ECs were incubated in pre-warmed serum free OptiMEM® for 20 min at 37°C to allow internalization. A sample of ECs were maintained at 4°C for use as positive/negative controls. The remaining dishes were subsequently placed on ice, washed twice with SBS pH 8.2, and any un-internalized biotin-labeled proteins were stripped off using 100 mM Mesna in Tris buffer for 75 min at 4°C. The internalized fraction of proteins was then allowed to recycle to the membrane by incubating the ECs for the indicated time points in serum-free OptiMEM® at 37°C. Following the indicated times of incubation, dishes were placed on ice, washed twice with SBS pH 8.2, and subjected to Mesna incubation for 75 min at 4°C. No Mesna treatment dishes at each timepoint were used as controls. All subsequent stages were performed in the same manner as the internalization assay. The level of the recycled ITGA5 was determined by normalizing the amount of ITGA5 quantified from dishes treated with Mesna, to the total ITGA5 on the membranes of the Mesna-untreated cells in the same period of incubation.
Statistical Analysis
The graphic illustrations and analyses to determine statistical significance were generated using GraphPad Prism 6.0 software and Student’s t-tests, respectively. Bar charts show mean values and the standard error of the mean (±SEM). Asterisks indicate the statistical significance of P-values: P > 0.05 = ns (not significant), ∗P < 0.05, ∗∗P < 0.01, ∗∗∗P < 0.001, and ****P < 0.0001.
Data Availability Statement
The raw data supporting the conclusions of this article will be made available by the authors, without undue reservation, to any qualified researcher.
Ethics Statement
The animal study was reviewed and approved by University of East Anglia Animal Welfare and Ethical Review.
Author Contributions
AA, CB, SA, RJ, and JL performed data acquisition, formal analyses, data visualisation, and participated in review and editing of the manuscript. SR performed investigation conceptualisation, data acquisition, formal analyses, data visualisation, participated in review and editing of the manuscript, acquired resources and funding, and performed supervision of the study.
Conflict of Interest
The authors declare that the research was conducted in the absence of any commercial or financial relationships that could be construed as a potential conflict of interest.
Funding
This work was supported by funding from: the UKRI Biotechnology and Biological Sciences Research Council Norwich Research Park Biosciences Doctoral Training Partnership (Grant numbers BB/M011216/1, BB/J014524/1, BB/M011216/1); BHF (Grant number PG/15/25/31369); The Ministry of Education, Saudi Arabia. Additionally, we thank Norfolk Fundraisers, Mrs Margaret Doggett, and the Colin Wright Fund for their kind support and fundraising over the years. Robinson is also partially funded by the BBSRC Institute Strategic Programme Gut Microbes and Health BB/R012490/1 and its constituent project (BBS/E/F/000PR10355). This manuscript has been released as a pre-print at BioRxiv (Alghamdi et al., 2020).
Supplementary Material
The Supplementary Material for this article can be found online at: https://www.frontiersin.org/articles/10.3389/fcell.2020.00395/full#supplementary-material
FIGURE S1 | (A): PyMT transfected mLMEC pellets from nine different immortalized EC lines were subjected to DNA extraction and PCR-based genotyping at the ITGB3 locus. Shown is an agarose gel of P1/P3 and P1/P2 primer products from all EC lines. Lines 1–5 show only a P1/P3 product and are therefore wild-type (WT) for the ITGB3 locus, whilst lines 6–9 show both a P1/P3 product, and a P1/P2 product, and are therefore heterozygous (β3HET) for the ITGB3 locus. (B): Western blot analysis of VE-Cadherin and ITGB3 expression in the same clones shown in (A). HSC70 was used as a loading control. Because the antibody used for detection of ITGB3 recognizes a non-specific band at approximately (135kDa), a β3-knockout (NULL) lysate was included as a control (cell line #10). (C): Left panel shows the densitometric analysis of mean ITGB3 band intensities normalized against HSC70 obtained using ImageJTM [from the Western blot shown in (B)]. ∗∗P < 0.01. (D): The EC identity of three immortalized EC lines was re-confirmed by Western blot analysis, immunoblotting against additional EC markers Pecam-1, Endomucin, ERG and Claudin-5, alongside a GAPDH loading control and a lymphatic marker Prox-1. (E): ECs were transfected either with control siRNA or one of four different NRP2-specific siRNAs (01–04) and incubated for 48 h. EC extracts were then subjected to Western blot analysis using antibodies against NRP2, NRP1 and HSC70. Except where noted (Supplementary Figure S3), NRP2 siRNA #03 was used for all subsequent experiments to silence NRP2 expression. (F): siRNA-transfected ECs were incubated for the indicated timepoints before being lysed and subjected to Western blot analysis using antibodies against NRP2 and HSC70. Asterisks indicate statistical significance from unpaired two-tailed t-tests. (G): Schematic diagram of the ITGB3 (WT) and (HET) loci, showing where PCR genotyping primers align. P1 and P3 amplify a wild-type product of 446-bp, whilst P1 and P2 amplify a knockout product of 538-bp.
FIGURE S2 | (A): siRNA-transfected ECs were seeded onto FN and incubated for 30 h. ECs were then re-seeded onto FN-coated coverslips, and allowed to adhere for 4 h in serum-free media. Media was then replaced with 10 μM BrdU in complete culture medium, and the cells incubated for 12 h at 37°C. ECs were then fixed with 4% PFA, before hydrolyzing the DNA with 1 M HCL. ECs were permeabilized and blocked with Dako® Protein Block Serum-Free. ECs were then incubated with anti-BrdU at 4°C overnight in a humidified chamber, followed by incubation in secondary antibody. Coverslips were subsequently mounted and the number of cells in S-phase (proliferating cells) was determined by dividing the number of the BrdU-labeled cells by the number of DAPI-labeled cells. n = 19 independent fields of view, containing on average 50 cells per field, per condition. (B): Adhesion assay performed as described in Figure 2A, however, ECs were transfected with either control or NRP2 siRNA#04. Bars show mean number of adhered cells calculated from absorbance readings from 40 wells per condition, per timepoint, normalized to a 3-h incubation control plate, N = 1. (C): Accompanying analysis to Figure 2D. The cell area (microns2) was measured using ImageJTM. Quantification performed on mean data from n ≥ 25 ECs over N = 3 independent experiments, nsd = not significantly different from unpaired two-tailed t-test. (D): siRNA-transfected ECs were prepared as described in Figure 2D legend, measuring mean FA number, FA size and FA distribution, however, ECs were transfected with either control or NRP2 siRNA#04. Quantification performed on mean data from n = 15 ECs. Asterisks indicate statistical significance from an unpaired two-tailed t-test.
FIGURE S3 | (A): Accompanying analysis to Figure 3D. siRNA-transfected ECs were prepared for immunocytochemistry as described in Figure 2C. Fixed ECs were incubated in primary antibodies against ITGA5 overnight at 4°C. ITGA5 length was measured using the ImageJTM software plugin simple neurite tracer. n = 490 cells per condition, ****P (0.0001). Asterisks indicate statistical significance from unpaired two-tailed t-tests. (B): siRNA-transfected ECs from three different immortalized EC lines were prepared as described in Figure 3D legend, however, ECs were transfected with either control (top) or NRP2 siRNA#04 (bottom). Panels show representative images from N = 3 independent lines, n ≥ 10 cells per line. (C): Western blot analysis of cell lysates from both primary EC clones alongside a lysate from a known fibroblast control cell line. EC extracts were immunoblotted using antibodies against known EC markers Endomucin, ERG and Claudin-5, alongside a GAPDH loading control. (D): Primary ECs were transfected with either ctrl or NRP2 siRNA and prepared for immunostaining as described in Figure 3E. Panels show representative images from n = 10 cells per condition from two independent primary EC lines.
TABLE S1 | NRP2-immunoprecipitating label-free quantitative (LFQ) mass spectrometry list.
References
Alghamdi, A. A., Benwell, C., Atkinson, S. J., Lambert, J., and Robinson, S. D. (2020). NRP2 as an emerging angiogenic player; promoting endothelial cell adhesion and migration by regulating recycling of α5 integrin. BioRxiv [Preprint] doi: 10.1101/744763
Atkinson, S. J., Gontarczyk, A. M., Alghamdi, A. A., Ellison, T. S., Johnson, R. T., Fowler, W. J., et al. (2018). The beta3-integrin endothelial adhesome regulates microtubule-dependent cell migration. EMBO Rep. 19:e44578. doi: 10.15252/embr.201744578
Avraamides, C. J., Garmy-Susini, B., and Varner, J. A. (2008). Integrins in angiogenesis and lymphangiogenesis. Nat. Rev. Cancer 8, 604–617. doi: 10.1038/nrc2353
Bachelder, R. E., Crago, A., Chung, J., Wendt, M. A., Shaw, L. M., Robinson, G., et al. (2001). Vascular endothelial growth factor is an autocrine survival factor for neuropilin-expressing breast carcinoma cells. Cancer Res. 61, 5736–5740.
Bae, D., Lu, S., Taglienti, C. A., and Mercurio, A. M. (2008). Metabolic stress induces the lysosomal degradation of neuropilin-1 but not neuropilin-2. J. Biol. Chem. 283, 28074–28080. doi: 10.1074/jbc.M804203200
Bielenberg, D. R., Hida, Y., Shimizu, A., Kaipainen, A., Kreuter, M., Kim, C. C., et al. (2004). Semaphorin 3F, a chemorepulsant for endothelial cells, induces a poorly vascularized, encapsulated, nonmetastatic tumor phenotype. J. Clin. Invest. 114, 1260–1271. doi: 10.1172/jci21378
Bouvree, K., Brunet, I., Del Toro, R., Gordon, E., Prahst, C., Cristofaro, B., et al. (2012). Semaphorin3A, neuropilin-1, and plexinA1 are required for lymphatic valve formation. Circ. Res. 111, 437–445. doi: 10.1161/CIRCRESAHA.112.269316
Brooks, P. C., Clark, R. A., and Cheresh, D. A. (1994). Requirement of vascular integrin alpha v beta 3 for angiogenesis. Science 264, 569–571. doi: 10.1126/science.7512751
Buskermolen, A. B. C., Kurniawan, N. A., and Bouten, C. V. C. (2018). An automated quantitative analysis of cell, nucleus and focal adhesion morphology. PLoS One 13:e0195201. doi: 10.1371/journal.pone.0195201
Cai, Y., Jia, T., Lam, S. K., Ding, Y., Gao, C., San, M. W., et al. (2011). Multiple cytosolic and transmembrane determinants are required for the trafficking of SCAMP1 via an ER-Golgi-TGN-PM pathway. Plant J. 65, 882–896. doi: 10.1111/j.1365-313X.2010.04469.x
Cao, Y., Hoeppner, L. H., Bach, S., E, G., Guo, Y., Wang, E., et al. (2013). Neuropilin-2 promotes extravasation and metastasis by interacting with endothelial alpha5 integrin. Cancer Res. 73, 4579–4590. doi: 10.1158/0008-5472.CAN-13-0529
Caswell, P. T., Spence, H. J., Parsons, M., White, D. P., Clark, K., Cheng, K. W., et al. (2007). Rab25 associates with alpha5beta1 integrin to promote invasive migration in 3D microenvironments. Dev. Cell 13, 496–510. doi: 10.1016/j.devcel.2007.08.012
Caunt, M., Mak, J., Liang, W. C., Stawicki, S., Pan, Q., Tong, R. K., et al. (2008). Blocking neuropilin-2 function inhibits tumor cell metastasis. Cancer Cell 13, 331–342. doi: 10.1016/j.ccr.2008.01.029
Curreli, S., Arany, Z., Gerardy-Schahn, R., Mann, D., and Stamatos, N. M. (2007). Polysialylated neuropilin-2 is expressed on the surface of human dendritic cells and modulates dendritic cell-T lymphocyte interactions. J. Biol. Chem. 282, 30346–30356. doi: 10.1074/jbc.m702965200
Dallas, N. A., Gray, M. J., Xia, L., Fan, F., Van Buren, G. II, Gaur, P., et al. (2008). Neuropilin-2-mediated tumor growth and angiogenesis in pancreatic adenocarcinoma. Clin. Cancer Res. 14, 8052–8060. doi: 10.1158/1078-0432.CCR-08-1520
De Pascalis, C., and Etienne-Manneville, S. (2017). Single and collective cell migration: the mechanics of adhesions. Mol. Biol. Cell 28, 1833–1846. doi: 10.1091/mbc.E17-03-0134
Duleh, S. N., and Welch, M. D. (2012). Regulation of integrin trafficking, cell adhesion, and cell migration by WASH and the Arp2/3 complex. Cytoskeleton 69, 1047–1058. doi: 10.1002/cm.21069
Ellison, T. S., Atkinson, S. J., Steri, V., Kirkup, B. M., Preedy, M. E., Johnson, R. T., et al. (2015). Suppression of beta3-integrin in mice triggers a neuropilin-1-dependent change in focal adhesion remodelling that can be targeted to block pathological angiogenesis. Dis. Model. Mech. 8, 1105–1119. doi: 10.1242/dmm.019927
Fakhari, M., Pullirsch, D., Abraham, D., Paya, K., Hofbauer, R., Holzfeind, P., et al. (2002). Selective upregulation of vascular endothelial growth factor receptors neuropilin-1 and -2 in human neuroblastoma. Cancer 94, 258–263. doi: 10.1002/cncr.10177
Favier, B., Alam, A., Barron, P., Bonnin, J., Laboudie, P., Fons, P., et al. (2006). Neuropilin-2 interacts with VEGFR-2 and VEGFR-3 and promotes human endothelial cell survival and migration. Blood 108, 1243–1250. doi: 10.1182/blood-2005-11-4447
Fukahi, K., Fukasawa, M., Neufeld, G., Itakura, J., and Korc, M. (2004). Aberrant expression of neuropilin-1 and -2 in human pancreatic cancer cells. Clin. Cancer Res. 10, 581–590. doi: 10.1158/1078-0432.ccr-0930-03
Fukasawa, M., Matsushita, A., and Korc, M. (2007). Neuropilin-1 interacts with integrin beta1 and modulates pancreatic cancer cell growth, survival and invasion. Cancer Biol. Ther. 6, 1173–1180.
Goel, H. L., Pursell, B., Standley, C., Fogarty, K., and Mercurio, A. M. (2012). Neuropilin-2 regulates alpha6beta1 integrin in the formation of focal adhesions and signaling. J. Cell Sci. 125, 497–506. doi: 10.1242/jcs.094433
Goley, E. D., and Welch, M. D. (2006). The ARP2/3 complex: an actin nucleator comes of age. Nat. Rev. Mol. Cell Biol. 7, 713–726. doi: 10.1038/nrm2026
Goodman, S. L., and Picard, M. (2012). Integrins as therapeutic targets. Trends Pharmacol. Sci. 33, 405–412. doi: 10.1016/j.tips.2012.04.002
Herzog, Y., Kalcheim, C., Kahane, N., Reshef, R., and Neufeld, G. (2001). Differential expression of neuropilin-1 and neuropilin-2 in arteries and veins. Mech. Dev. 109, 115–119. doi: 10.1016/s0925-4773(01)00518-4
Hodivala-Dilke, K. M., Mchugh, K. P., Tsakiris, D. A., Rayburn, H., Crowley, D., Ullman-Cullere, M., et al. (1999). Beta3-integrin-deficient mice are a model for Glanzmann thrombasthenia showing placental defects and reduced survival. J. Clin. Invest. 103, 229–238. doi: 10.1172/jci5487
Ikuno, T., Masumoto, H., Yamamizu, K., Yoshioka, M., Minakata, K., Ikeda, T., et al. (2017). Efficient and robust differentiation of endothelial cells from human induced pluripotent stem cells via lineage control with VEGF and cyclic AMP. PLoS One 12:e0173271. doi: 10.1371/journal.pone.0173271
Jurisic, G., Maby-El Hajjami, H., Karaman, S., Ochsenbein, A. M., Alitalo, A., Siddiqui, S. S., et al. (2012). An unexpected role of semaphorin3a-neuropilin-1 signaling in lymphatic vessel maturation and valve formation. Circ. Res. 111, 426–436. doi: 10.1161/CIRCRESAHA.112.269399
Kaksonen, M., Toret, C. P., and Drubin, D. G. (2006). Harnessing actin dynamics for clathrin-mediated endocytosis. Nat. Rev. Mol. Cell Biol. 7, 404–414. doi: 10.1038/nrm1940
Kawakami, T., Tokunaga, T., Hatanaka, H., Kijima, H., Yamazaki, H., Abe, Y., et al. (2002). Neuropilin 1 and neuropilin 2 co-expression is significantly correlated with increased vascularity and poor prognosis in nonsmall cell lung carcinoma. Cancer 95, 2196–2201. doi: 10.1002/cncr.10936
Kim, S., Bakre, M., Yin, H., and Varner, J. A. (2002). Inhibition of endothelial cell survival and angiogenesis by protein kinase A. J. Clin. Invest. 110, 933–941. doi: 10.1172/jci0214268
Kim, S., Bell, K., Mousa, S. A., and Varner, J. A. (2000). Regulation of angiogenesis in vivo by ligation of integrin alpha5beta1 with the central cell-binding domain of fibronectin. Am. J. Pathol. 156, 1345–1362. doi: 10.1016/s0002-9440(10)65005-5
Krilleke, D., Deerkenez, A., Schubert, W., Giri, I., Robinson, G. S., Ng, Y. S., et al. (2007). Molecular mapping and functional characterization of the VEGF164 heparin-binding domain. J. Biol. Chem. 282, 280450–280456.
Lambert, J., Makin, K., Akbareian, S., Johnson, R. T., Alghamdi, A. A., Robinson, S. D., et al. (2020). ADAMTS-1 and Syndecan-4 intersect in the regulation of cell migration and angiogenesis. J. Cell Sci. 133:jcs235762. doi: 10.1242/jcs.235762
Lantuejoul, S., Constantin, B., Drabkin, H., Brambilla, C., Roche, J., and Brambilla, E. (2003). Expression of VEGF, semaphorin SEMA3F, and their common receptors neuropilins NP1 and NP2 in preinvasive bronchial lesions, lung tumours, and cell lines. J. Pathol. 200, 336–347. doi: 10.1002/path.1367
Laukaitis, C. M., Webb, D. J., Donais, K., and Horwitz, A. F. (2001). Differential dynamics of alpha 5 integrin, paxillin, and alpha-actinin during formation and disassembly of adhesions in migrating cells. J. Cell Biol. 153, 1427–1440. doi: 10.1083/jcb.153.7.1427
Le Boeuf, F., Houle, F., and Huot, J. (2004). Regulation of vascular endothelial growth factor receptor 2-mediated phosphorylation of focal adhesion kinase by heat shock protein 90 and Src kinase activities. J. Biol. Chem. 279, 39175–39185. doi: 10.1074/jbc.m405493200
Malek, M., Guillaumot, P., Huber, A. L., Lebeau, J., Petrilli, V., Kfoury, A., et al. (2012). LAMTOR1 depletion induces p53-dependent apoptosis via aberrant lysosomal activation. Cell Death Dis. 3:e300. doi: 10.1038/cddis.2012.39
Margadant, C., Monsuur, H. N., Norman, J. C., and Sonnenberg, A. (2011). Mechanisms of integrin activation and trafficking. Curr. Opin. Cell Biol. 23, 607–614. doi: 10.1016/j.ceb.2011.08.005
Mucka, P., Levonyak, N., Geretti, E., Zwaans, B. M. M., Li, X., Adini, I., et al. (2016). Inflammation and lymphedema are exacerbated and prolonged by neuropilin 2 deficiency. Am. J. Pathol. 186, 2803–2812. doi: 10.1016/j.ajpath.2016.07.022
Nobes, C. D., and Hall, A. (1995). Rho, rac, and cdc42 GTPases regulate the assembly of multimolecular focal complexes associated with actin stress fibers, lamellipodia, and filopodia. Cell 81, 53–62. doi: 10.1016/0092-8674(95)90370-4
Paul, N. R., Jacquemet, G., and Caswell, P. T. (2015). Endocytic trafficking of integrins in cell migration. Curr. Biol. 25, R1092–R1105. doi: 10.1016/j.cub.2015.09.049
Pellet-Many, C., Frankel, P., Jia, H., and Zachary, I. (2008). Neuropilins: structure, function and role in disease. Biochem. J. 411, 211–226. doi: 10.1042/bj20071639
Prahst, C., Heroult, M., Lanahan, A. A., Uziel, N., Kessler, O., Shraga-Heled, N., et al. (2008). Neuropilin-1-VEGFR-2 complexing requires the PDZ-binding domain of neuropilin-1. J. Biol. Chem. 283, 25110–25114. doi: 10.1074/jbc.C800137200
Price, L. S., Leng, J., Schwartz, M. A., and Bokoch, G. M. (1998). Activation of Rac and Cdc42 by integrins mediates cell spreading. Mol. Biol. Cell 9, 1863–1871. doi: 10.1091/mbc.9.7.1863
Remacle, A., Murphy, G., and Roghi, C. (2003). Membrane type I-matrix metalloproteinase (MT1-MMP) is internalised by two different pathways and is recycled to the cell surface. J. Cell Sci. 116, 3905–3916. doi: 10.1242/jcs.00710
Reynolds, L. E., and Hodivala-Dilke, K. M. (2006). Primary mouse endothelial cell culture for assays of angiogenesis. Methods Mol. Med. 120, 503–509.
Ridley, A. J. (2011). Life at the leading edge. Cell 145, 1012–1022. doi: 10.1016/j.cell.2011.06.010
Ridley, A. J., Schwartz, M. A., Burridge, K., Firtel, R. A., Ginsberg, M. H., Borisy, G., et al. (2003). Cell migration: integrating signals from front to back. Science 302, 1704–1709. doi: 10.1126/science.1092053
Roberts, M., Barry, S., Woods, A., Van Der Sluijs, P., and Norman, J. (2001). PDGF-regulated rab4-dependent recycling of alphavbeta3 integrin from early endosomes is necessary for cell adhesion and spreading. Curr. Biol. 11, 1392–1402. doi: 10.1016/s0960-9822(01)00442-0
Robinson, S. D., Reynolds, L. E., Kostourou, V., Reynolds, A. R., Da Silva, R. G., Tavora, B., et al. (2009). Alphav beta3 integrin limits the contribution of neuropilin-1 to vascular endothelial growth factor-induced angiogenesis. J. Biol. Chem. 284, 33966–33981. doi: 10.1074/jbc.M109.030700
Robinson, S. D., Reynolds, L. E., Wyder, L., Hicklin, D. J., and Hodivala-Dilke, K. M. (2004). Beta3-integrin regulates vascular endothelial growth factor-A-dependent permeability. Arterioscler. Thromb. Vasc. Biol. 24, 2108–2114. doi: 10.1161/01.atv.0000143857.27408.de
Sachse, M., Urbe, S., Oorschot, V., Strous, G. J., and Klumperman, J. (2002). Bilayered clathrin coats on endosomal vacuoles are involved in protein sorting toward lysosomes. Mol. Biol. Cell 13, 1313–1328. doi: 10.1091/mbc.01-10-0525
Schiller, H. B., Friedel, C. C., Boulegue, C., and Fassler, R. (2011). Quantitative proteomics of the integrin adhesome show a myosin II-dependent recruitment of LIM domain proteins. EMBO Rep. 12, 259–266. doi: 10.1038/embor.2011.5
Schiller, H. B., Hermann, M. R., Polleux, J., Vignaud, T., Zanivan, S., Friedel, C. C., et al. (2013). beta1- and alphav-class integrins cooperate to regulate myosin II during rigidity sensing of fibronectin-based microenvironments. Nat. Cell Biol. 15, 625–636. doi: 10.1038/ncb2747
Shi, F., and Sottile, J. (2008). Caveolin-1-dependent beta1 integrin endocytosis is a critical regulator of fibronectin turnover. J. Cell Sci. 121, 2360–2371. doi: 10.1242/jcs.014977
Sivarapatna, A., Ghaedi, M., Le, A. V., Mendez, J. J., Qyang, Y., and Niklason, L. E. (2015). Arterial specification of endothelial cells derived from human induced pluripotent stem cells in a biomimetic flow bioreactor. Biomaterials 53, 621–633. doi: 10.1016/j.biomaterials.2015.02.121
Small, J. V., Rottner, K., Kaverina, I., and Anderson, K. I. (1998). Assembling an actin cytoskeleton for cell attachment and movement. Biochim. Biophys. Acta 1404, 271–281. doi: 10.1016/s0167-4889(98)00080-9
Soker, S., Takashima, S., Miao, H. Q., Neufeld, G., and Klagsbrun, M. (1998). Neuropilin-1 is expressed by endothelial and tumor cells as an isoform-specific receptor for vascular endothelial growth factor. Cell 92, 735–745. doi: 10.1016/s0092-8674(00)81402-6
Suzuki-Inoue, K., Yatomi, Y., Asazuma, N., Kainoh, M., Tanaka, T., Satoh, K., et al. (2001). Rac, a small guanosine triphosphate-binding protein, and p21-activated kinase are activated during platelet spreading on collagen-coated surfaces: roles of integrin alpha(2)beta(1). Blood 98, 3708–3716. doi: 10.1182/blood.v98.13.3708
Tomizawa, Y., Sekido, Y., Kondo, M., Gao, B., Yokota, J., Roche, J., et al. (2001). Inhibition of lung cancer cell growth and induction of apoptosis after reexpression of 3p21.3 candidate tumor suppressor gene SEMA3B. Proc. Natl. Acad. Sci. U.S.A. 98, 13954–13959. doi: 10.1073/pnas.231490898
Valdembri, D., Caswell, P. T., Anderson, K. I., Schwarz, J. P., Konig, I., Astanina, E., et al. (2009). Neuropilin-1/GIPC1 signaling regulates alpha5beta1 integrin traffic and function in endothelial cells. PLoS Biol. 7:e25. doi: 10.1371/journal.pbio.1000025
Valdembri, D., and Serini, G. (2012). Regulation of adhesion site dynamics by integrin traffic. Curr. Opin. Cell Biol. 24, 582–591. doi: 10.1016/j.ceb.2012.08.004
Vales, A., Kondo, R., Aichberger, K. J., Mayerhofer, M., Kainz, B., Sperr, W. R., et al. (2007). Myeloid leukemias express a broad spectrum of VEGF receptors including neuropilin-1 (NRP1) and NRP2. Leuk. Lymphoma 48, 1997–2007. doi: 10.1080/10428190701534424
Velling, T., Collo, G., Sorokin, L., Durbeej, M., Zhang, H., and Gullberg, D. (1996). Distinct alpha 7A beta 1 and alpha 7B beta 1 integrin expression patterns during mouse development: alpha 7A is restricted to skeletal muscle but alpha 7B is expressed in striated muscle, vasculature, and nervous system. Dev. Dyn. 207, 355–371. doi: 10.1002/(sici)1097-0177(199612)207:4<355::aid-aja1>3.0.co;2-g
Webb, D. J., Parsons, J. T., and Horwitz, A. F. (2002). Adhesion assembly, disassembly and turnover in migrating cells – over and over and over again. Nat. Cell Biol. 4, E97–E100.
Yuan, L., Moyon, D., Pardanaud, L., Breant, C., Karkkainen, M. J., Alitalo, K., et al. (2002). Abnormal lymphatic vessel development in neuropilin 2 mutant mice. Development 129, 4797–4806.
Zachary, I. (2014). Neuropilins: role in signalling, angiogenesis and disease. Chem. Immunol. Allergy 99, 37–70. doi: 10.1159/000354169
Keywords: Neuropilins, endothelium, integrins, protein trafficking, cell migration
Citation: Alghamdi AAA, Benwell CJ, Atkinson SJ, Lambert J, Johnson RT and Robinson SD (2020) NRP2 as an Emerging Angiogenic Player; Promoting Endothelial Cell Adhesion and Migration by Regulating Recycling of α5 Integrin. Front. Cell Dev. Biol. 8:395. doi: 10.3389/fcell.2020.00395
Received: 07 February 2020; Accepted: 29 April 2020;
Published: 26 May 2020.
Edited by:
Stephan Huveneers, Amsterdam University Medical Center (UMC), NetherlandsReviewed by:
Eloi Montanez, University of Barcelona, SpainJaap Diederik Van Buul, University of Amsterdam, Netherlands
Copyright © 2020 Alghamdi, Benwell, Atkinson, Lambert, Johnson and Robinson. This is an open-access article distributed under the terms of the Creative Commons Attribution License (CC BY). The use, distribution or reproduction in other forums is permitted, provided the original author(s) and the copyright owner(s) are credited and that the original publication in this journal is cited, in accordance with accepted academic practice. No use, distribution or reproduction is permitted which does not comply with these terms.
*Correspondence: Stephen D. Robinson, stephen.robinson@quadram.ac.uk
†These authors have contributed equally to this work
‡Present address: Abdullah A. A. Alghamdi, Department of Biology, Faculty of Science, Albaha University, Albaha, Saudi Arabia Samuel J. Atkinson, Cancer Research UK Beatson Institute, Glasgow, United Kingdom Jordi Lambert, Division of Cardiovascular Medicine, University of Cambridge, Cambridge Biomedical Campus, Cambridge, United Kingdom Robert T. Johnson, School of Pharmacy, University of East Anglia, Norwich Research Park, Norwich, United Kingdom