- 1Laboratory of Signal Transduction and Plant Resistance, UNESCO-Regional Centre for Biotechnology (RCB), NCR Biotech Science Cluster, Faridabad, India
- 2Kalinga Institute of Industrial Technology (KIIT) University, Bhubaneswar, India
- 3Division of Plant Sciences, C. S. Bond Life Sciences Center and Interdisciplinary Plant Group, University of Missouri, Columbia, MO, United States
Steady-state SUMOylome of a plant is adjusted locally during developmental transitions and more globally during stress exposures. We recently reported that basal immunity in Arabidopsis thaliana against Pseudomonas syringae pv tomato strain DC3000 (PstDC3000) is associated with strong enhancements in the net SUMOylome. Transcriptional upregulations of SUMO conjugases, suppression of protease, and increased SUMO translations accounted for this enhanced SUMOylation. Antagonistic roles of SUMO1/2 and SUMO3 isoforms further fine-tuned the SUMOylome adjustments, thus impacting defense amplitudes and immune outcomes. Loss of function of SUPPRESSOR OF rps4-RLD1 (SRFR1), a previously reported negative regulator of basal defenses, also caused constitutive increments in global SUMO-conjugates through similar modes. These suggest that SRFR1 plays a pivotal role in maintenance of SUMOylation homeostasis and its dynamic changes during immune elicitations. Here, we demonstrate that SRFR1 degradation kinetically precedes and likely provides the salicylic acid (SA) elevations necessary for the SUMOylome increments in basal defenses. We show that SRFR1 not only is a SUMOylation substrate but also interacts in planta with both SUMO1 and SUMO3. In sum1 or sum3 mutants, SRFR1 stabilities are reduced albeit by different modes. Whereas a srfr1 sum1 combination is lethal, the srfr1 sum3 plants retain developmental defects and enhanced immunity of the srfr1 parent. Together with increasing evidence of SUMOs self-regulating biochemical efficiencies of SUMOylation-machinery, we present their impositions on SRFR1 expression that in turn counter-modulates the SUMOylome. Overall, our investigations reveal multifaceted dynamics of regulated SUMOylome changes via SRFR1 in defense-developmental balance.
Introduction
In higher eukaryotes, pivotal roles of post-translational modifications (PTMs) balance protein expressions, activities, interacting partners, localization, and proteostasis (Walsh et al., 2005; Millar et al., 2019). With structural similarities to ubiquitin, the SMALL UBIQUITIN-LIKE MODIFIERs (SUMOs) covalently attach to lysine (K) residues of substrates through a cascade of enzymatic reactions biochemically similar to ubiquitination cycles and termed as SUMOylation (Geiss-Friedlander and Melchior, 2007; Flotho and Melchior, 2013; Zhao, 2018). The partially conserved motif, ψ–K–X–D/E (ψ = hydrophobic amino acid, X = any amino acid, and D/E = aspartate/glutamate), most often harbors the SUMOylated lysine residue. SUMOs can also facilitate non-covalent protein–protein associations requiring the essential presence of hydrophobic core-containing SUMO-interaction motifs (SIMs), K–X3–5–[V/I]–[I/L]–X3–[D/E/Q/N]–[D/E]2, in the cognate recipient (Hannich et al., 2005). Computational studies identify SUMOylation-annotated candidates as strategic central relay players of protein–protein interaction webs, and with noted predominance of DNA-modification enzymes and transcription factors (TFs), transcriptional processes are especially modulated (Duan and Walther, 2015).
Maintenance of SUMOylome homeostasis necessitates tight coordination of SUMO-conjugation/deconjugation cycles (Kurepa et al., 2003; Morrell and Sadanandom, 2019). SUMOylation requires the availability of processed SUMOs, with exposed diglycine (GG) residues at their C-terminus. SUMO proteases generate these conjugation-proficient SUMOs from precursors that contain extended C-terminus residues. The mature SUMOs through an ATP-dependent AMP–SUMO intermediate formation is linked via thiol–ester bond to the catalytic cysteine of SUMO E1 ACTIVATING ENZYME (SAE), a heterodimer of two subunits SAE1 and SAE2. SUMO E2 CONJUGATING ENZYME (SCE) then acquires the SUMO moiety from SAE via trans-esterification. Although SCE is capable of direct SUMOylation, its binding to SUMO E3 ligases, which also simultaneously interact with targeted substrates, augments the process and imparts specificity (Gareau and Lima, 2010). SUMOs are also self-SUMOylated at internal lysines forming poly-SUMO chains. These are catalyzed by SUMO E4 ligases that belong to the PIAL (protein inhibitor of activated stat-like) class of proteins (Tomanov et al., 2014). Some SUMO proteases remove SUMOs from SUMOylated substrates or disintegrate polySUMO chains, thus recycling free SUMOs (Chosed et al., 2006; Colby et al., 2006; Morrell and Sadanandom, 2019).
Insights from studies on the model plant Arabidopsis thaliana have provided vital clues into the complexity of SUMOylation and its genetic link to diverse cellular processes. Arabidopsis SUMO-machineries are mostly encoded by multiple genes showing tissue/stage-dependent, stress-inducible expressions and interaction/modification specificities with substrates. Although A. thaliana genome encodes eight SUMO isoforms, only four (SUM1, -2, -3, and -5) are expressed (van den Burg et al., 2010). SUMO1 and -2 proteins share considerable sequence identity (94%) and, in several instances, are functionally redundant or additive and surprisingly in some responses contrasting (Saracco et al., 2007; van den Burg et al., 2010; Castano-Miquel et al., 2011; Ingole et al., 2021b). SUMO3 and -5 proteins are more diverged with less than 50% identity to SUMO1. Simultaneous loss of SUM1 and SUM2 is embryonic lethal, indicating that plants require at least one functional copy of either of these isoforms. In plants, SUMO1/2 predominantly occur as free non-conjugated forms, which upon exposure to heat, peroxide, or ethanol stress are rapidly utilized for SUMOylating target proteins (Kurepa et al., 2003; van den Burg et al., 2010). The Arabidopsis sum3 mutant is viable with mild late-flowering phenotype (van den Burg et al., 2010). SUMO3, unlike SUMO1/2, cannot form poly-SUMO chains in vitro, is barely detected in plant extracts, and shows little or no change to heat shock treatments (Kurepa et al., 2003; Chosed et al., 2006; Colby et al., 2006; Budhiraja et al., 2009; van den Burg et al., 2010). SUMO1/2 but not SUMO3-modified targets are efficiently deconjugated by ULPs (Chosed et al., 2006; Colby et al., 2006). Lastly, SUMO1 and SUMO3 reciprocally influence each other’s conjugation efficiencies in vitro, implying functional cooperativity (Ingole et al., 2021b). HIGH PLOIDY 2/METHYL METHANE SULFONATE 21 (HPY2/MMS21) and SAP and MIZ 1 (SIZ1) remain the two well-characterized SUMO E3 ligases in Arabidopsis. Multiple Arabidopsis SUMO proteases have been identified till date and include EARLY IN SHORT DAYS 4 (ESD4), its closest homologs ELS1/2 (ESD4-LIKE SUMO PROTEASE1/2), OVERLY TOLERANT TO SALT 1/2 (OTS1/2), SUMO-PROTEASES RELATED TO FERTILITY 1/2 (SPF1/2), and DE-SUMOYLATING ISOPEPTIDASES (DeSIs), among others (Kurepa et al., 2003; Murtas et al., 2003; Chosed et al., 2006; Colby et al., 2006; Kong et al., 2017; Orosa et al., 2018). However, only a fraction of these remain functionally characterized (Morrell and Sadanandom, 2019; Srivastava et al., 2021).
In broadly understood layers of plant defenses, conserved molecular signatures or pathogen-associated molecular patterns (PAMPs) present on microbes are sensed by extracellular transmembrane pattern-recognition receptors (PRRs) to transduce downstream induction of defense-associated genes (Jones and Dangl, 2006; Bentham et al., 2020). This route of immune signaling comprise the PAMP-triggered immunity (PTI). Intracellular perception of a pathogen attack is performed by strategically deployed resistance (R) proteins that directly or indirectly sense manipulations by the invader-secreted effectors. Defense responses elicited downstream of these perceptions are termed as effector-triggered immunity (ETI) and include heightened production of defensive hormone salicylic acid (SA), and prolonged and aggravated expression of PTI-responsive markers such as PATHOGENESIS-RELATED PROTEINS (PRs) and SA-biosynthesis SALICYLIC ACID-DEFICIENT 2/ISOCHORISMATE SYNTHASE 1 (SID2/ICS1) gene, among others. Basal and ETI mediated by the Toll–interleukin1 (TNL)-type of R proteins require ENHANCED DISEASE SUSCEPTIBILITY 1 (EDS1) to potentiate SA-based defenses (Aarts et al., 1998; Falk et al., 1999).
Disturbances in SUMO pathway affect immune responses often with developmental costs to the host (Lee et al., 2007; van den Burg et al., 2010; Bailey et al., 2016; Orosa et al., 2018; Verma et al., 2018). Studies overall suggest that SUMO1/2 suppresses whereas SUMO3 potentiates immunity primarily through modulation of SA-signaling networks (van den Burg et al., 2010; Saleh et al., 2015; Ingole et al., 2021b). However, adjustments of a host SUMOylome in response to pathogen attack or in SUMOylation-perturbed mutants present a more complicated involvement not only of SUMO isoforms but also of SUMO-machineries. For example, globally reduced SUMOylome in siz1-2 or enhanced SUMO1/2-conjugates in esd4-2 or ots1 ots2 both lead to elevated SA levels with constitutive activation of defenses (Villajuana-Bonequi et al., 2014; Bailey et al., 2016). ESD4 and SIZ1 are SUMOylated and non-covalently bind SUMOs, implying that their activities are self-regulated by SUMOylation (Miller et al., 2010; Mazur et al., 2017). Our studies recently identified both positive and negative immune regulators as differentially SUMOylated candidates upon a pathogen attack (Ingole et al., 2021a). Further, we also revealed that SUMO-conjugation efficiencies are affected by the crosstalk between SUMO isoforms independent of their covalent-modification activities. Taken together, how a plant maintains SUMOylome homeostasis, performs response-appropriate modifications on substrates, and prevents fitness costs remains uncharacterized.
We recently showed that in the autoimmune mutant srfr1-4 constitutive and during PstDC3000 infections on wild-type plants progressive, increments of SUMO1/2 conjugations were observed, respectively (Ingole et al., 2021a). This implies a genetic link of SRFR1 to SUMOylome maintenance and responsive adjustments. Here, we reveal that SRFR1 potentiates PstDC3000-induced SUMOylome changes, undergoing transient instability that kinetically precedes SA accumulations and global SUMOylome enhancements. With known link in suppressing SID2/ICS1 expressions, SRFR1 reduction thus likely provides the SA stimulus previously shown to enhance SUMO-conjugations (Bailey et al., 2016). We deduce here that in addition to being a SUMOylation candidate, SUMOylome perturbation also reciprocally affects SRFR1 expressions at both transcriptional and post-transcriptional levels. Further, enhanced basal defenses or SUMO1/2-conjugation increments are negligibly SUM3-dependent in srfr1-4 plants. Overall, our investigations here present SRFR1 role in SUMOylome homeostasis and perturbations providing fine-tuning of immune amplitudes.
Materials and Methods
Plant Materials and Growth Conditions
Mutants of A. thaliana used here, namely, sum1-1, sum3-1, sum1-1 sum3-1, srfr1-4, sid2-1, srfr1-4 eds1-2, srfr1-4 snc1-11, and esd4-2, have been described earlier (van den Burg et al., 2010; Bhattacharjee et al., 2011; Villajuana-Bonequi et al., 2014; Ingole et al., 2021b). Plants were propagated under short day (SD; 8-h light; 16-h dark) conditions at 22°C (or 24°C for PstDC3000 infection-based assays) with 70% relative humidity in controlled growth chambers with a light intensity of 100 μmol photons m–2 s–1. To generate double mutants srfr1-4 sid2-1, srfr1-4 sum3-1, or HA-SRFR1 expressing plants in SUMOylation-disturbed (sum1-1, sum3-1, esd4-2, or esd4-2 sum3-1) mutants, indicated plants discussed in the respective results sections were crossed. From the segregating F2 or F3 populations, the desired genotype was identified and propagated for experiments. To generate plants co-expressing EDS1-YFP and His-H89R-SUM1, the parental EDS1-YFP (Garcia et al., 2010) and His-H89R-SUM1 (Miller et al., 2010) plants were crossed. F1 plants were verified for the presence of both transgenes before analysis. Primers for genotyping are listed in Supplementary Table 1.
Salicylic Acid Measurements
Free and total SA (SA + glucose-conjugated, SAG) measurements were performed according to Defraia et al. (2008) using the Acinetobacter sp. ADPWH_lux biosensor system. Briefly, 100 mg of frozen tissue was homogenized in 250 μl of acetate buffer (0.1 M, pH 5.6) and clarified by centrifugation at 12,000 rpm for 15 min to remove cell debris. A 100 μl of the supernatant was kept for measuring free SA, while a similar volume was treated with 6 U of β-glucosidase (Sigma-Aldrich, St. Louis, MO, United States) for 90 min at 37°C for total SA determination. A 20 μl aliquot of plant extract was mixed with 50 μl of Acinetobacter suspension (grown to OD600 = 0.4) along with 60 μl of fresh Luria–Bertani (LB) broth. Standard curve was generated with sid2-1 tissue extracts spiked with known amounts of SA (Sigma-Aldrich, United States). After incubation for 1 h at 37°C, luminescence was measured using a Luminometer (POLARStar Omega, BMG Labtech, Ortenberg, Germany). At least three biological replicates were used for each measurement, and data were reported as mean ± SD.
Total RNA Extraction and Quantitative Real-Time PCR
Total RNA extraction from plant tissues was performed using RNAiso Plus reagent (Takara, Maebashi, Japan), DNase-treated (TURBOTM DNA-free kit, Thermo Fisher Scientific, Wilmington, DE, United States), and then reverse transcribed (iScriptTM cDNA Synthesis Kit, Bio-Rad, Hercules, CA, United States) according to the manufacturer’s instructions. All qPCR primers used here are listed in Supplementary Table 1. Real-time PCRs were performed with HOT FIREPol EvaGreen qPCR Mix Plus (ROX) (Solis BioDyne, Tartu, Estonia) as suggested by the manufacturer and on a QuantStudio 6 Flex machine (Applied Biosystems, Foster City, CA, United States). Expressions were normalized to the endogenous control MON1 (At2g28390) levels and calculated according to the (PCR efficiency)–ΔΔ Ct formula (Kim et al., 2010). Each experiment was repeated at least twice with three biological and technical replicates.
Protein Extraction, Immunoprecipitation, and Immunoblotting
Sample processing for anti-SUMO1/2 immunoblots is described in Ingole et al. (2021a). In brief, tissues snap frozen and stored in –80°C were homogenized in protein extraction buffer (PEB) [50 mM of Tris–HCl pH 8.0, 8 M of urea, 50 mM of NaCl, 1% w/v NP-40, 0.5% w/v sodium deoxycholate, 0.1% w/v sodium dodecyl sulfate (SDS) and 1 mM of EDTA, and 20 mM of N-ethylmaleimide (NEM)] containing freshly added 1 × plant protease inhibitors (Sigma-Aldrich, United States) and 2% w/v polyvinylpolypyrrolidone (PVPP). For anti-HA, anti-EDS1, anti-GFP, or anti-PR1/2 immunoblots, tissues were ground with 6 M of urea. Clarified homogenates of extracts were mixed with loading dye, boiled, resolved in SDS–polyacrylamide gel electrophoresis (SDS-PAGE), and transferred to polyvinylidene difluoride (PVDF) membrane via wet transfer. After blocking with 5% w/v non-fat skimmed milk powder in 1 × Tris-buffered saline (TBST; containing 0.1% w/v Tween® 20), membrane was incubated overnight with indicated primary antibodies [anti-SUMO1 (Abcam, Cambridge MA, United States), anti-PR1/2 (Agrisera, Vännäs, Sweden), anti-EDS1 (custom generated against full-length Arabidopsis EDS1 from BioBharati LifeScience, Kolkata, India), anti-HA (Sigma, United States), or anti-GFP (BioBharati LifeScience, India)] in 1 × TBST. Membranes were washed thrice the next day with TBST, incubated at room temperature (RT) with secondary antibodies conjugated with horseradish peroxidase (HRP) (Santa Cruz Biotech, Dallas, TX, United States), and developed with ECL Prime western blotting kit (GE Healthcare, Chicago, IL, United States). Images were acquired with ImageQuant LAS 4000 (GE Healthcare, United States). The membranes were stained with Ponceau S and imaged to demonstrate comparable loading.
SUMO1/2-conjugate enrichments from plants expressing EDS1-YFP with or without His-H89R-SUM1 were performed as earlier (Ingole et al., 2021a).
For immunoprecipitation assays between HA-SRFR1 and Myc-EDS1 or HA-PAD4 and Myc-EDS1, agro-infiltrated Nicotiana benthamiana leaves were used 48 h post-infiltration. Tissues were ground in chilled radioimmunoprecipitation assay (RIPA) buffer [5 mM of Tris–HCl pH 7.5, 150 mM of NaCl, 10 mM of MgCl2, 1 mM of EDTA, 1% NP-40, 1 mM of sodium deoxycholate, and 1 × protease inhibitor cocktail (Sigma-Aldrich, United States)] and clarified by centrifugation. Supernatant was precleared with IgG agarose beads (Sigma-Aldrich, United States) rotated at 4°C for 1 h. The mix was then centrifuged to remove non-specific proteins bound on the IgG agarose beads, and supernatant was added to anti-HA-conjugated beads (Sigma, United States). After tumbling 3 h at 4°C, the suspension was centrifuged, and agarose-bead pellet washed three times with RIPA buffer. Beads were then resuspended in loading dye and used for indicated immunoblots.
For MG132 treatments, the proteasome inhibitor at a dose of 100 μM in 10 mM of MgCl2, 10 mM of 2-(N-morpholino)ethanesulfonic acid (MES) was infiltrated into plant tissues 12 h before harvesting.
Densitometric quantifications of protein bands in immunoblots were performed with the ImageJ software.
Construction of Clones for Escherichia coli SUMOylation Assay
SUM1 cDNA sequences in pCDFDuet TM1 vector were mutagenized via overlapping oligos at position 91 to incorporate arginine (R) replacing the threonine (T) residue. This resulted in SUMO1 T91R, which imparts smaller SUMOylation footprint of RGG on the modified lysine. For generating FLAG-SRFR1, cDNA sequence was directionally cloned as a NotI restriction fragment at the identical site of pFLAG-TEV vector (BioBharati LifeScience, India). For generating T7-EDS1, the cDNA sequence was cloned as a SalI–XhoI fragment into the pET28a(+) vector (Novagen, Madison, WI, United States). Details of primers used here are listed in Supplementary Table 1.
The SUMOylation assay was performed according to Okada et al. (2009). Briefly, BL21 (DE3) cells containing pCDFDuet TM1-SUMO1/2/3 GG form together with SCE1, or pCDFDuet TM1-SUMO1/2/3 AA form co-cloned with SCE1, and pACYCDuet TM1-SAE2 and SAE1 were co-transformed with either pFLAG-SRFR1 or pT7-EDS1 plasmid. Heterologous protein expression was induced with 0.5 mM of IPTG overnight at 28°C. The cell pellet harvested the next day was resuspended in phosphate-buffered saline (0.137 M of NaCl, 0.0027 M of KCl, 0.01 M of Na2HPO4, and 0.0018 M of KH2PO4, pH 7.4), lysed by boiling in 1 × loading dye, and then used for immunoblots with anti-FLAG (Sigma, United States) or anti-T7 antibodies (Merck Millipore, Kenilworth, NJ, United States).
Sample Preparation and Liquid Chromatography–Tandem MS Analysis for Determination of SUMOylation Sites
Processing for in-gel trypsin digestion and MS analysis was according to Ingole et al. (2021a). Briefly, proteins from in vitro SUMOylation reaction were separated on 6% SDS-PAGE, stained with Coomassie brilliant blue (CBB R-250) solution, and destained; and gel slices were excised as 1-mm3 pieces with sterile surgical blade. After processing as earlier, trypsin digestion (Promega, Madison, WI, United States) was performed; samples were desalted with C18 tips (Thermo Fisher Scientific, United States) according to manufacturer’s instructions and dried in a speed-vac centrifuge. Tandem MS (MS/MS) analysis was done using TripleTOF® 5600+ (AB SCIEX, Redwood City, CA, United States) mass spectrometer instrument. Raw MS data files were searched for peptide sequences (MASCOT software, Matrix Science, Boston, MA, United States) against the A. thaliana protein database1.
Construction of Bimolecular Fluorescence Complementation, GFP-Tagged SUM1 GG/AA Vectors, and in planta Assays
Bimolecular fluorescence complementation (BiFC) vectors for SRFR1, EDS1, SUMO1 GG/AA, and SUMO3 GG/AA have been described earlier (Bhattacharjee et al., 2011; Ingole et al., 2021b). Clones were introduced into Agrobacterium tumefaciens GV3101 strain via electroporation. For in planta interaction assays, Agrobacterium strains harboring the indicated BiFC vectors were cultured overnight in LB broth, centrifuged, and resuspended in 10 mM of MgCl2, 10 mM of MES containing 150 mM of acetosyringone. After induction for 3–4 h, indicated combinations were mixed at equal bacterial density and infiltrated in fully expanded leaves of 4-week-old N. benthamiana. At 48 h post-infection (hpi), tissue sections from the infiltrated area were excised and imaged under a 40 × oil objective in SP8 Leica confocal microscope (Leica microsystems, Wetzlar, Germany) using the fluorescein isothiocyanate (FITC) filter (488-nm argon laser).
Construction of HA-SRFR1K325R, HA-SRFR1K427R, HA-SRFR1K325R + K427R, GFP-SUMO1 GG/AA Forms, or SUMO3, Myc-EDS1WT, or Myc-EDS1K478R Expression Vectors and Generation of Transgenic Plants
The construction of HA-SRFR1 expressing native promoter-driven HA-epitope tagged genomic clone of SRFR1 has been described earlier (Kim et al., 2010). With the use of this vector as a backbone, the indicated lysine residues were converted to arginine using overlapping primers (listed in Supplementary Table 1). pDONR201 clone of SUMO1 GG or AA, SUMO3-GG described earlier (Ingole et al., 2021b), was subcloned via Gateway reaction into pSITE-2CA binary vector that carries an N-terminal GFP tag (Chakrabarty et al., 2007). The above clones were confirmed by sequencing and then electroporated into Agrobacterium GV3101 strain. Transformants obtained were cultured; and srfr1-4 or sum3-1, as appropriate, was transformed via floral-dip method (Clough and Bent, 1998). Transgenic plants were selected on kanamycin (for HA-SRFR1 or GFP-SUMO3 transformations) containing plant growth media and then propagated to T2 and T3 generations and genotyped. Simultaneously, expression of GFP-SUMO3 was also determined via anti-GFP immunoblots. GFP-SUM3 Line#1 was crossed to HA-SRFR1 expressing plants and F2/F3 population genotyped for sum3-1, srfr1-4, mutations, and HA-SRFR1 or GFP-SUM3 transgene homozygosity. The plants were then used for further analysis, as indicated.
For co-expression assays with HA-SRFR1, Agrobacterium strains with GFP alone (pSITE-2CA) or GFP-SUM1 GG or AA forms (in pSITE-2CA backbone) was co-infiltrated as above. Total protein from infiltrated tissues was isolated at 48 hpi and used for immunoblot with anti-HA antibodies.
Construction of Myc-EDS1WT binary has been described earlier (Bhattacharjee et al., 2011). With the use of the pDONR201-EDS1WT, the lysine 478 residue was mutated to arginine (EDS1K478R) by overlap PCR. This was then subcloned into pBA-Myc binary vector via Gateway-based cloning. Plant transformations with eds1-2 were done as earlier. Independent transgenic lines were selected on 1/2 MS + Basta (10 μg/ml) plates and grown to F3 for assays. Expression of recombinant protein was checked with anti-Myc immunoblots. Primers for various cloning are listed in Supplementary Table 1.
In planta Qualitative and Quantitative Bacterial Growth Assay
Bacterial growth assays were performed according to standardized protocol in Kim et al. (2010). Briefly, fully expanded leaves of 3- to 4-week-old SD-grown plants were infiltrated with a needleless syringe at a bacterial density of 106 cfu/ml (half-leaf infiltrated for qualitative disease symptom assay) or 5 × 104 cfu/ml (full leaf infiltrated for quantitative bacterial growth assays) with virulent PstDC3000 or avirulent PstDC3000 (avrRps4) strains. For disease symptom determinations, infiltrated leaves were detached 4 days post-infiltration (dpi) and imaged. For quantitative measurements on bacterial growth, leaf disks of defined area were harvested at 0 and 3 dpi, macerated in 10 mM of MgCl2, and plated with serial dilution on appropriate antibiotic containing media plates. Anti-SUMO1/2 immunoblot on Col-0, sum1-1, sum2-1, and sum3-1 post-PstDC3000 challenge (106 cfu/ml bacterial inoculum) was performed at 24 hpi.
Results
SRFR1 Instability Precedes Global SUMO1/2ylation and Salicylic Acid Elevations During Basal Defenses
Recently, we demonstrated that SRFR1 regulates Arabidopsis SUMOylome maintenance (Ingole et al., 2021a). The srfr1-4 plants contained elevated levels of SUMO1/2-conjugates and displayed upregulated SUMO conjugation-promoting enzymes (SCE1, SAE1/2, SIZ1, and HPY2) and downregulated SUMO-protease (ESD4 and ELS1) expressions with preferential recruitment of SUM1 transcripts on polysomes. Though SUMOylome increments were also observed when wild-type (Col-0) plants were challenged with PstDC3000, SRFR1 functions were not directly attributed to these responses. To determine this, HA-SRFR1/srfr1-4 plants (referred hereafter as HA-SRFR1) expressing HA-epitope tagged SRFR1 protein from its native promoter were used. These plants were shown earlier to complement the growth and defense defects of srfr1-4 (Kim et al., 2010). HA-SRFR1 plants were challenged with PstDC3000, and tissue extracts from progressive time points were immuno-probed with anti-HA antibodies. Decrease in SRFR1 protein levels was noted as early at 1 hpi and persisted moderately at 3 and 6 hpi, after which gradual restoration to near wild-type levels was noted by 24 hpi (Figure 1A). Transcripts of SRFR1 showed progressive upregulation increasing from 1 to 6 hpi (∼2- to 2.5-fold) and restored to endogenous levels by 24 hpi (Figure 1B). Immunoblot with anti-SUMO1/2 antibodies showed gradual increments in SUMO1/2-conjugations from 1 to 24 hpi (Figure 1C). Bailey et al. (2016) demonstrated that SA application enhances SUMO1/2-conjugations in Col-0. With previously known functions in suppressing SA-based defenses, to determine whether transient instability of SRFR1 preceded SA increase to cause SUMOylome changes, we measured free SA and its glucose-conjugate (SAG) levels in the above extracts. Significant increase in free and total (SA + SAG) levels (∼4- and ∼8-fold, respectively) was first noted at 6 hpi, and by 24 hpi, drastic elevations were observed (∼15- and ∼55-fold, respectively) (Figure 1D). This moderately correlated to the upregulation in SID2/ICS1 expressions (Figure 1E). Taken together, these data suggest that transient decrease in SRFR1 protein is linked to SUMOylome perturbation via SA-signaling routes.
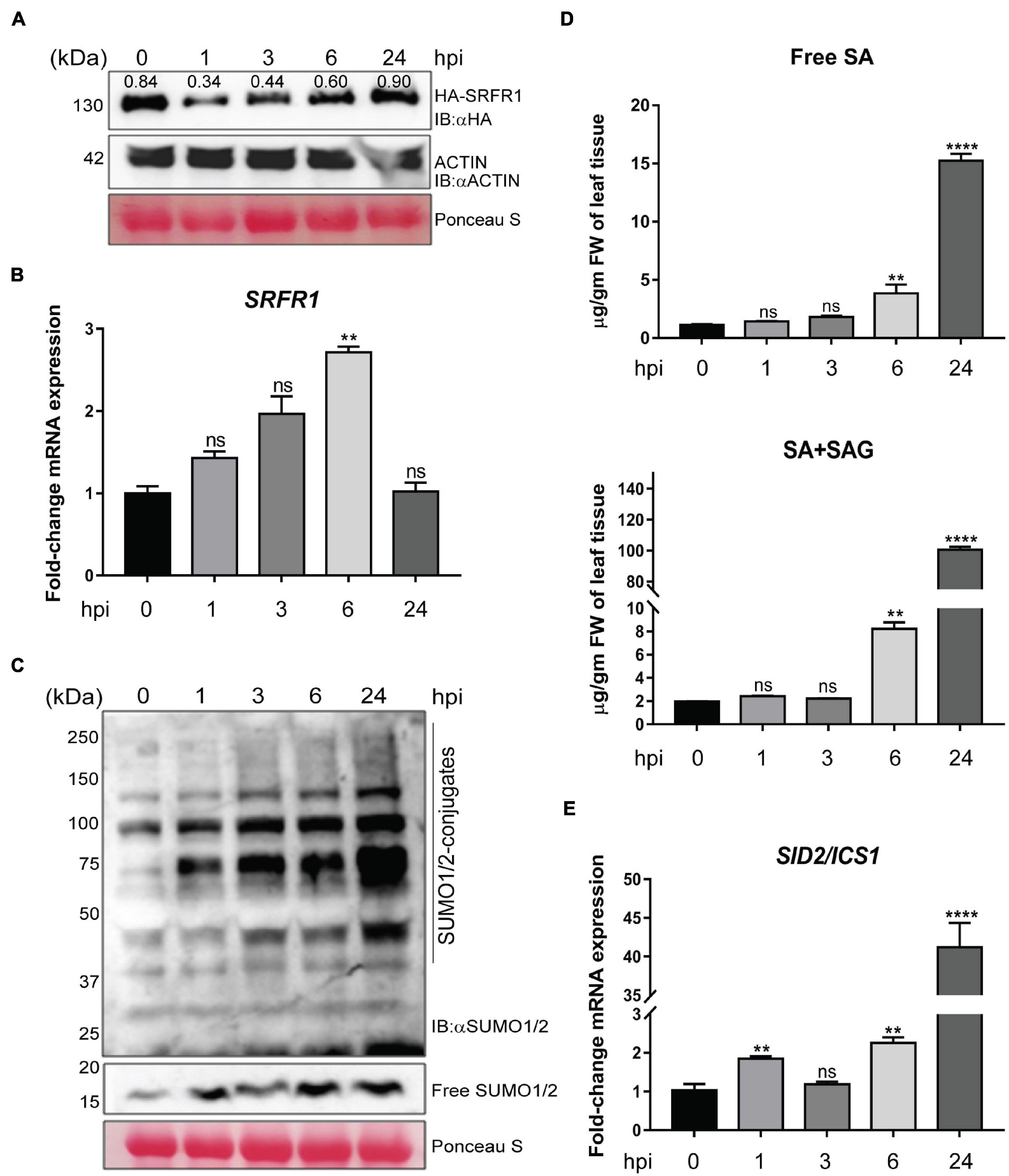
Figure 1. SRFR1 protein instability promotes salicylic acid (SA) and SUMOylome elevations in basal defenses. At progressive time points (hpi, hours post-infection) upon PstDC3000 challenge (A) immunoblot of HA-SRFR1, (B) expression levels of SRFR1 transcripts, (C) SUMO1/2-conjugates and free SUMO1/2 levels, (D) free SA and total SA + SAG levels, and (E) expression of SID2/ICS1. Blots were probed with anti-HA or anti-SUMO1/2 antibodies, as indicated. Ponceau S-stained membrane or anti-actin immunoblot shows comparable protein loading across samples. Numbers above the HA-SRFR1 protein bands in panel (A) are densitometric quantification values relative to actin expression levels in the same extracts. Transcript abundance is relative to internal control MON1 gene expressions and presented as fold-change relative to uninfected (0 hpi) samples (n = 3). Statistical significance is by pairwise comparison with 0-hpi sample with Student’s t-test (**p < 0.01, ****p < 0.0001, and ns = not significant).
To directly correlate SA increase due to SID2/ICS1 upregulation as the causal factor of SUMOylome enhancements in srfr1-4, we generated srfr1-4 sid2-1 plants by genetic crossing. Unlike srfr1-4 eds1-2 that are developmentally similar to Col-0, srfr1-4 sid2-1 plants retained stunted stature-like srfr1-4 (Figure 2A). Thus, growth deficiencies in srfr1-4 are SID2/ICS1-independent. Total (SA + SAG) and free SA levels in srfr1-4 sid2-1 resembled those of Col-0 (Supplementary Figure 1A). To determine global SUMOylome profile in srfr1-4 sid2-1, anti-SUMO1/2 immunoblots were performed. We also included srfr1-4 snc1-11 plants in this analysis. The snc1-11 is a knockout mutation in SNC1, the R gene responsible for growth abnormalities in srfr1-4 (Kim et al., 2010). The srfr1-4 snc1-11 plants developmentally resemble Col-0 but retain intermediate upregulation of defense-associated markers and enhanced resistance than srfr1-4 (Kim et al., 2010). We noted that sid2-1 or snc1-11 mutation completely abolished SUMO1/2-conjugate or free SUMO1/2 enhancements of srfr1-4 to Col-0 levels (Figure 2B and Supplementary Figure 1B). Further, elevated free SA and total SA were also restored to Col-0 levels in the srfr1-4 eds1-2 or srfr1-4 snc-11 plants (Supplementary Figure 1A). Additionally, increased expressions of SUMOylation-promoting genes shown earlier (SIZ1, SCE1, or SUM3) and PR1 transcripts were considerably downregulated to Col-0 levels in srfr1-4 sid2-1 plants (Ingole et al., 2021a; Figures 2C,D and Supplementary Figure 1C). Expression of SUM1 remained unaltered in all plant genotypes. These results implied that SA upregulation via heightened expression of SID2/ICS1 was mainly responsible for SUMOylome enhancements in srfr1-4 in SNC1- and EDS1-dependent manner.
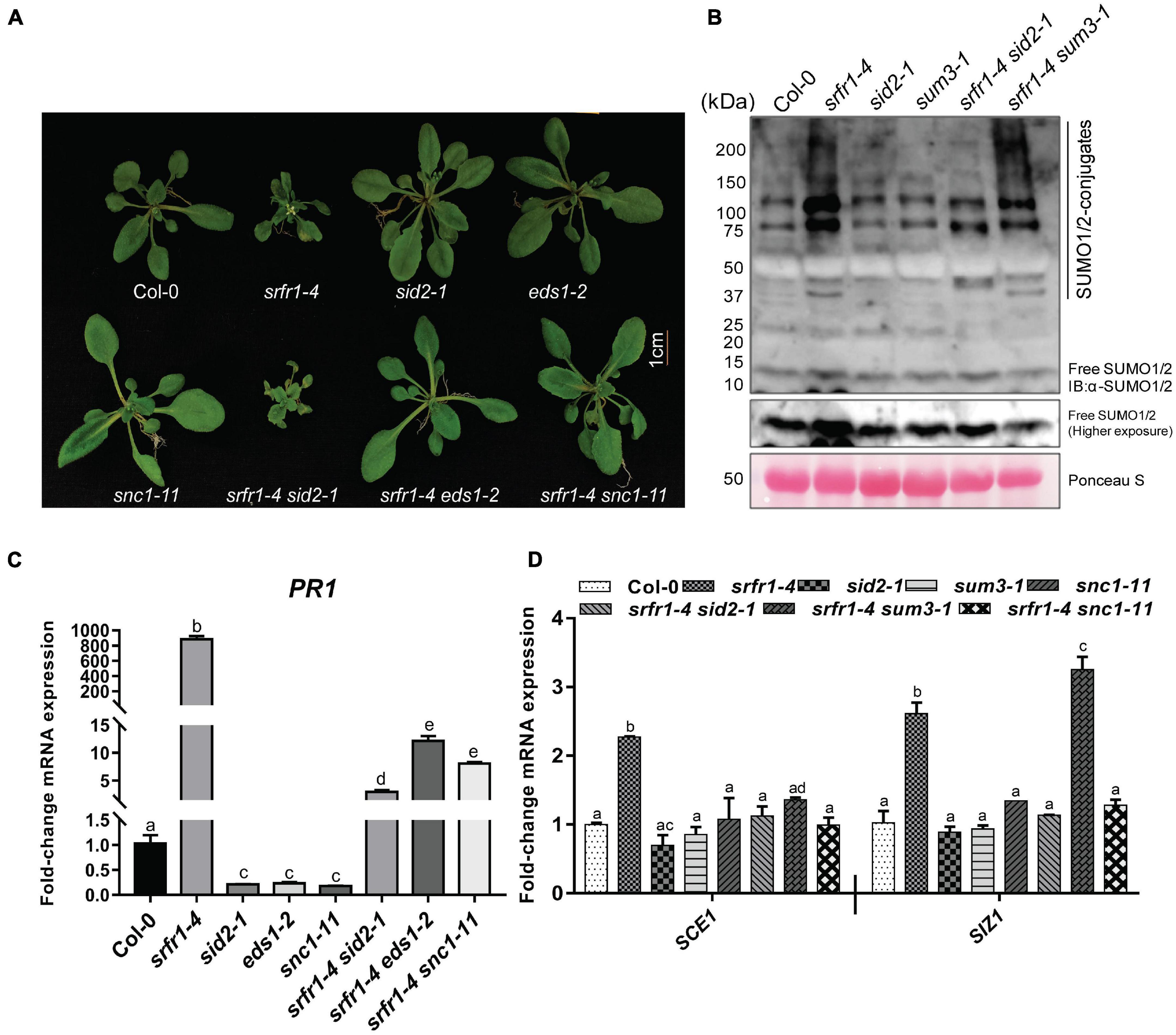
Figure 2. SUMOylome enhancements but not growth defects in srfr1-4 are SID2/ICS1-dependent. (A) Growth phenotypes of 4-week-old Col-0, srfr1-4, srfr1-4 sid2-1, srfr1-4 eds1-2, srfr1-4 snc1-11, sid2-1, eds1-2, and snc1-11 plants. Scale bar = 1 cm. (B) Immunoblot of SUMO1/2-conjugates and free SUMO1/2 in indicated plant genotypes. Protein extracts were probed with anti-SUMO1/2 antibodies. Ponceau S staining is indicative of loading controls. Transcript abundance of (C) PR1, (D) SCE1, or SIZ1, is relative to control MON1 expression in the indicated genotypes (n = 3). Values are mean ± SD and shown as fold-change relative to Col-0 levels. Different letters indicate statistical differences according to post hoc Tukey’s test (p < 0.05).
SRFR1 Is a SUMOylation Candidate
SRFR1 harbors several intrinsically disordered regions (IDRs) especially between its multiple TPRs and the C-terminal domain (Gassmann and Bhattacharjee, 2012). Protein with IDR features often has various interacting partners adjusted according to the cellular signaling needs (Haynes et al., 2006; Liu et al., 2009). Most often, IDRs are also enriched for motifs that attract PTM changes (Gao and Xu, 2012; Narasumani and Harrison, 2018). In SRFR1, two sites that match canonical SUMOylation motifs (LK325EE and LK427QE) are predicted by the SUMOsp2.0 tool (Ren et al., 2009; Figure 3A). Plus, at least two each of high-scoring SIMs and non-consensus SUMOylation sites are also present in SRFR1. To test whether SRFR1 is SUMOylated by the Arabidopsis SUMO isoforms, we utilized the Escherichia coli SUMOylation reconstitution system, with minor modifications (Okada et al., 2009). The N-terminal 91st residue N-terminal to GG in SUMO1 was modified from threonine to arginine (T91R) to facilitate smaller SUMO footprints on targets and improved identification via MS. SUMO3 contains a relatively proximal arginine at the 88th position to the diglycine G92G93 ends. MYB30, a previously known target tested by Okada et al. (2009), was SUMOylated by SUMO1 T91R variant (Supplementary Figure 2). SRFR1 cDNA sequences were co-expressed with a FLAG-epitope tag (FLAG-SRFR1) in the presence of SUMO1 T91R, SUMO2, or SUMO3 SUMOylation-competent (containing diglycine, GG) or SUMOylation-deficient (diglycine replaced by dialanine, AA) isoforms. Induced E. coli extracts probed with anti-FLAG antibodies showed a slower migrating band than FLAG-SRFR1 in the presence of only GG, but not AA forms of the co-expressed SUMOs (Figure 3B). This band was more intense when SUMO3 was co-expressed than with SUMO1/2. These results suggested that SRFR1 is a potential SUMOylation candidate of multiple SUMO isoforms.
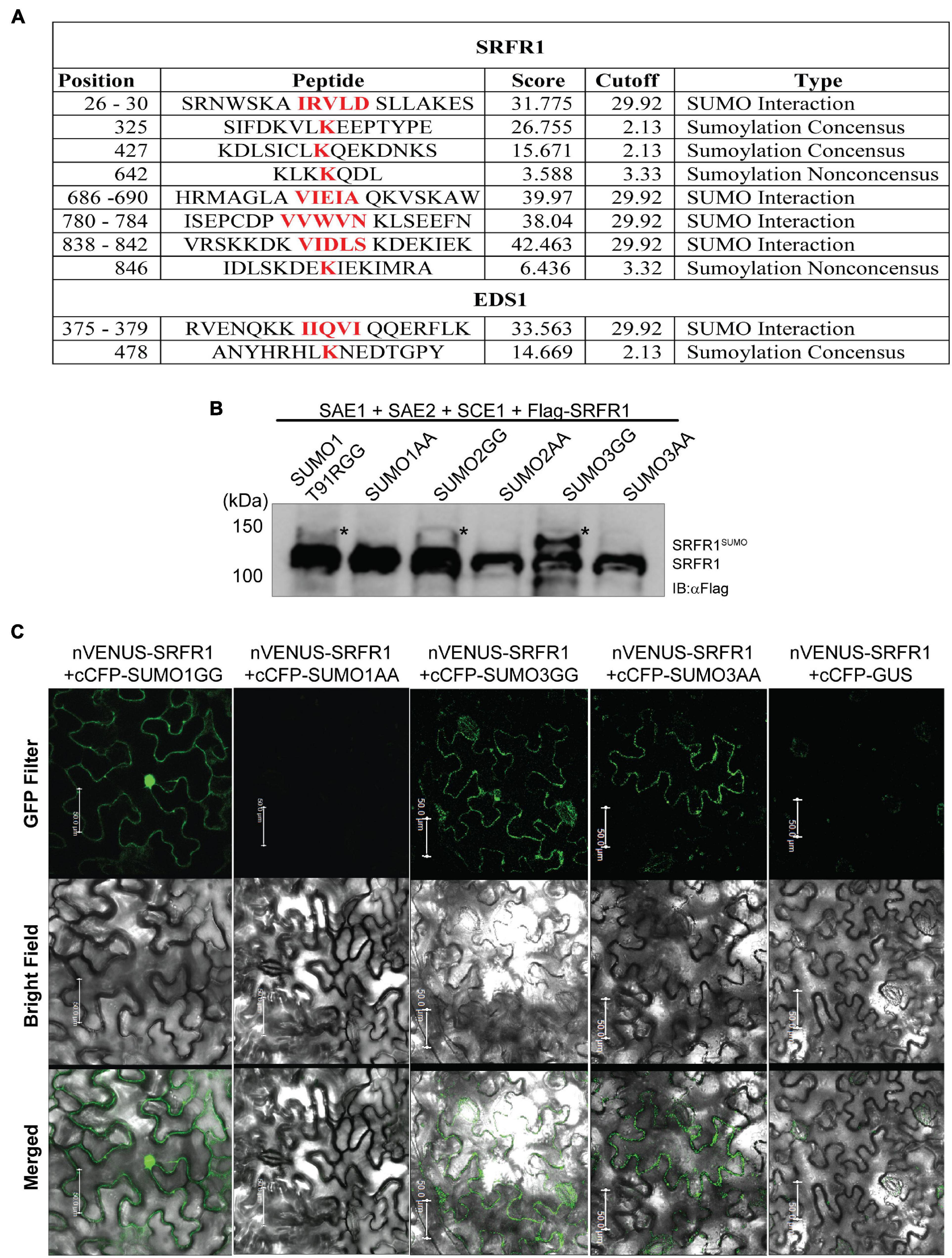
Figure 3. SRFR1 is a SUMOylation and SUMO-binding candidate. (A) Prediction of putative SUMOylation and SIM motifs in SRFR1 and EDS1. In silico prediction was performed using SUMOsp2.0 tool. (B) SRFR1 is SUMOylated by SUMO1 T91R, SUMO2, or SUMO3 GG forms in Escherichia coli SUMOylation reconstitution system. Immunoblot was probed with anti-FLAG antibodies to detect FLAG-SRFR1. Migration position of molecular weight standards (in kDa), SUMOylated or non-SUMOylated SRFR1 (SRFR1SUMO or SRFR1, respectively), is indicated. (C) Bimolecular fluorescence complementation (BiFC) panels of co-expressed nVenus-SRFR1 with cCFP-clones of SUMO1 GG/AA, SUMO3 GG/AA, or negative control GUS (β-glucuronidase). Images were acquired using a confocal microscope with GFP or bright-field filters. Merged images of YFP with bright field are shown. Scale bar = 50 μm.
We subjected the slower migrating SRFR1 protein bands to trypsin digests and liquid chromatography (LC)–MS/MS analysis. SUMO1, but not SUMO2 or SUMO3, footprints on selective lysine residues of SRFR1 were detected in the identified peptides (Supplementary Figure 3). Remarkably, K325 in the predicted LK325EE SUMOylation motif was identified with SUMO1-modifications. In addition, SRFR1 K229 was also covalently modified by SUMO1, though conserved features of a SUMOylation motif were absent for this lysine (CK229PC). These results demonstrated SRFR1 as a SUMOylation candidate, at least in the E. coli system. To test the functional relevance of in silico predicted SRFR1 SUMOylation sites, we generated native promoter-driven HA-SRFR1 expressing transgenic plants containing K325R, K427R, or K325R + K427R mutations (named as HA-SRFR1K325R, HA-SRFR1K427R, or HA-SRFR1K325R + K427R, accordingly), in the srfr1-4 background. Functional complementation of the expressed SRFR1 variant is expected to abolish growth defects of srfr1-4. Transgenic plants expressing HA-SRFR1K325R, HA-SRFR1K427R, or HA-SRFR1K325R + K427R versions of SRFR1 restored wild-type growth in srfr1-4 (Supplementary Figure 4). These results implied that R substitutions of at least these K residues do not affect SRFR1 function. With one additional lysine residue (K229) identified in SRFR1 as a potential SUMO-acceptor, similar mutational and complementation studies are needed to decipher its functional relevance. Detection in planta of SUMO1-modifications on K721 of TPR1, which is not a predicted SUMOylation site, encourages possibility of a non-conventional motif in SUMO-influences on SRFR1 (Niu et al., 2019).
To evaluate non-covalent binding of SUMOs to SRFR1, we utilized the BiFC assays. N. benthamiana leaves transiently expressing SRFR1 showed restoration of YFP signal with SUMO1 or SUMO3 co-expressed as GG (SUMOylation-competent) forms (Figure 3C). When SUMOs were expressed as AA (SUMOylation-deficient) forms, only SUMO3 but not SUMO1 bound SRFR1. As controls, neither SRFR1 nor SUMOs interacted with the negative control beta-glucuronidase (GUS) protein or the corresponding empty vector (Figure 3C and Supplementary Figure 5). These results suggest that SRFR1 is a likely SUMOylation target as well as SUMO-binding protein.
EDS1 K478, a Predicted SUMOylated Residue, Is Essential for Interaction With SRFR1
To test for possible functional relevance of SRFR1 SIMs, we utilized its previously known interaction with EDS1 (Bhattacharjee et al., 2011). As a negative immune regulator, SRFR1 likely sequesters EDS1 from activation of defenses. A high-scoring LK478NE matching the consensus SUMOylation motif is identified in the EDS1 protein sequence (Figure 3A). EDS1 K478 is located in the EP domain and is bracketed by residues R475 and D481 that form salt bridges with the corresponding loop residues in its partner SAG101 (Wagner et al., 2013). When tested in the E. coli–SUMOylation system, EDS1 was not SUMOylated by any of the SUMO isoforms (Supplementary Figure 6). Interestingly, in BiFC assays, co-expression of EDS1 and SUMO1 GG but not SUMO1 AA or SUMO3 GG/AA allowed reconstitution of YFP fluorescence, hinting that SUMO1 is a direct modifier of EDS1 (Figure 4A). To test the relevance of EDS1 K478 in plant defenses, we generated binary vectors that express CaMV 35S promoter-driven Myc-epitope tagged wild-type (Myc-EDS1WT) or K478R (lysine replace by arginine; Myc-EDS1K478R) versions of EDS1 cDNA. The binary vectors were then used to generate transgenic plants in the EDS1-null (eds1-2) background (Cui et al., 2017). Transgenic plants obtained were then tested in qualitative disease assays with the virulent PstDC3000 or avirulent PstDC3000 (avrRps4) strains. While Myc-EDS1WT expression in eds1-2 reinstated the basal defenses causing considerably lower chlorotic symptoms, Myc-EDS1K478R remained similar to hyper-susceptible and collapsed eds1-2 leaves indicative of enhanced bacterial accumulations (Figure 4B). To the avirulent PstDC3000 (avrRps4) challenges, Myc-EDS1K478R/eds1-2 remained as hyper-symptomatic as eds1-2, while Myc-EDS1WT/eds1-2 displayed Col-0-like resistance. Comparable Myc-EDS1WT and Myc-EDS1K478R protein expressions were noted between the transgenic plants (Figure 4C). Overall, these results indicated that EDS1K478R is functionally deficient in supporting defenses. To test whether interactions with SRFR1 are affected for EDS1K478R, we co-expressed Myc-EDS1 (wild type or K478R) with HA-SRFR1 in N. benthamiana leaves. Immuno-enrichment of HA-SRFR1 detected Myc-EDS1WT but not Myc-EDS1K478R, indicating that EDS1 K478 is important for SRFR1 interaction (Figure 4D). Interestingly, PAD4, a known partner of EDS1 (Feys et al., 2001), when transiently co-expressed (as HA-PAD4) in N. benthamiana leaves showed comparable interaction with either Myc-EDS1WT or Myc-EDS1K478R, implying that EDS1 K478 is not essential for their association (Figure 4E).
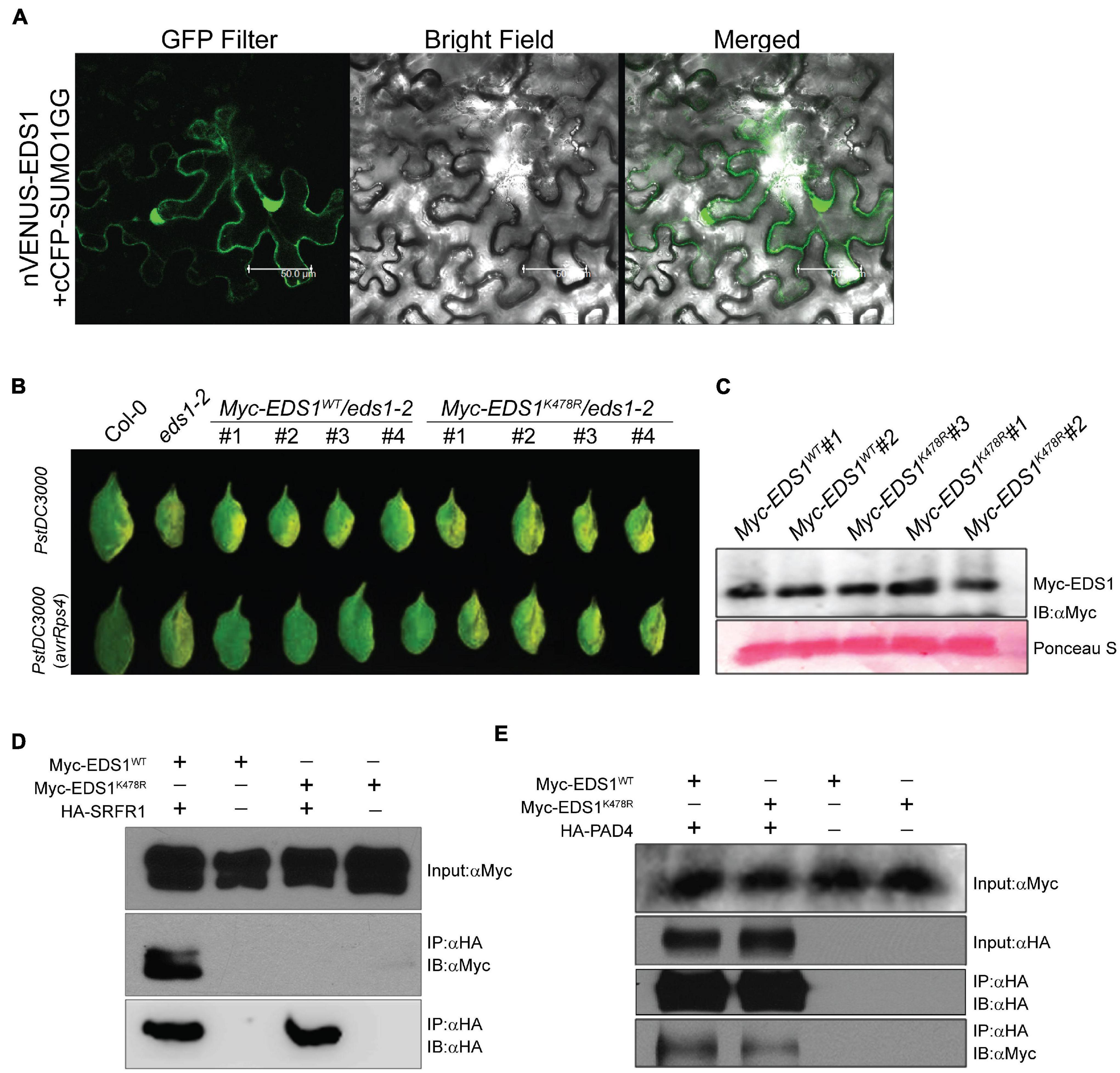
Figure 4. EDS1K478R does not interact with SRFR1 and is functionally deficient in supporting defenses. (A) EDS1 interaction with SUMO1 in bimolecular fluorescence complementation (BiFC) assays. YFP reconstitution was detected with a confocal microscope equipped with a GFP filter. Also shown are merge of GFP filter and bright-field images. Scale bar = 50 μm. (B) Disease symptoms on independent transgenic plants expressing Myc-EDS1WT or Myc-EDS1K478R in eds1-2 background infected with virulent PstDC3000 (top panel) or avirulent PstDC300 (avrRps4) (bottom panel) strains. (C) Expression levels of Myc-EDS1WT and Myc-EDS1K478R in independent transgenic lines. Total protein extracts were immunoblotted with anti-Myc antibodies. (D) Immuno-enrichment of HA-SRFR1 co-elutes Myc-EDS1WT but not Myc-EDS1K478R. Co-expression was performed in Nicotiana benthamiana leaves, and anti-HA immuno-enrichments were probed with anti-HA or anti-Myc antibodies, as indicated. (E) Both Myc-EDS1WT and Myc-EDS1K478R interact similarly with HA-PAD4. PAD4 was pulled down with anti-HA antibodies and probed for Myc-EDS1 or Myc-EDS1K478R presence.
To detect for SUMOylated EDS1 in planta, we obtained F1 plants that co-expressed EDS1-YFP and His-H89R-SUM1. The crossed parents expressed EDS1-YFP or His-H89R-SUM1 that functionally complement the loss of respective endogenous EDS1 or SUM1 (Garcia et al., 2010; Miller et al., 2010). Expression of His6-SUM1-H89R facilitates improved affinity-based enrichment of SUMO1-SUMOylated proteins. EDS1-YFP (control) or EDS1-YFP/His-H89R-SUM1 plants were sprayed with SA to mimic defense responses and then enriched for His-SUMO1-conjugated proteins with Ni2+-affinity chromatography, as earlier (Ingole et al., 2021a). Eluates showed enrichment of SUMO1-conjugates and when probed with anti-GFP antibodies identified a protein band at expected migration positions for SUMOylated EDS1-YFP (EDS1SUMO1) only in EDS1-YFP/His6-SUM1-H89R but not EDS1-YFP samples (Supplementary Figure 7). Input extracts from both samples displayed similar levels of EDS1-YFP expression. Because of their low amounts, we were unable to perform MS analysis on the hypothesized EDS1SUMO1 protein bands. In a parallel approach, we transiently co-expressed Myc-EDS1WT or Myc-EDS1K478R with GFP-SUMO1 or His-StrepII-SUM3g (Ingole et al., 2021b) in N. benthamiana leaves and probed whether SUMO1/3 co-expression caused reduced migration of EDS1 indicative of its SUMOylation. Immunoblot, however, showed similar migration of Myc-EDS1WT across all SUMO combinations (Supplementary Figure 8). Thus, despite our attempts, reasonable doubt whether in planta EDS1 is SUMOylated (on K478) persists, and further investigations to elucidate this are therefore warranted.
sum1-1 or sum3-1 Affect Endogenous Levels and Dynamics of SRFR1 During Basal Defenses
With indications of SRFR1 as a potential SUMOylation and SIM-harboring candidate, we tested whether enhanced or compromised defenses, respectively, in sum1-1 or sum3-1 (Ingole et al., 2021b), are the result of changes in SRFR1 levels. Toward this, we generated HA-SRFR1/sum1-1 plants by crossing sum1-1 to the HA-SRFR1 line. Plants with homozygous sum1-1 mutation displayed reduced HA-SRFR1 protein in comparison with the HA-SRFR1 parent (Figure 5A). To determine whether the lower protein levels were due to transcriptional or post-transcriptional effects, we measured SRFR1 transcript abundance. In sum1-1 plants, SRFR1 transcripts displayed only a slight reduction (∼0.8-fold), suggesting that reduced SRFR1 protein is mostly due to post-transcriptional effects (Figure 5B). Addition of the 26S proteasome inhibitor MG132 slightly improved HA-SRFR1 levels in HA-SRFR1/sum1-1 plants. Furthermore, GFP-SUMO1 GG or AA variant co-expressed with HA-SRFR1 in N. benthamiana leaves caused an increase in HA-SRFR1 protein abundance in comparison with the GFP alone control (Figure 5C). Taken together, our results imply that SUM1 role as a negative immune regulator may be via maintaining the steady-state levels of SRFR1.
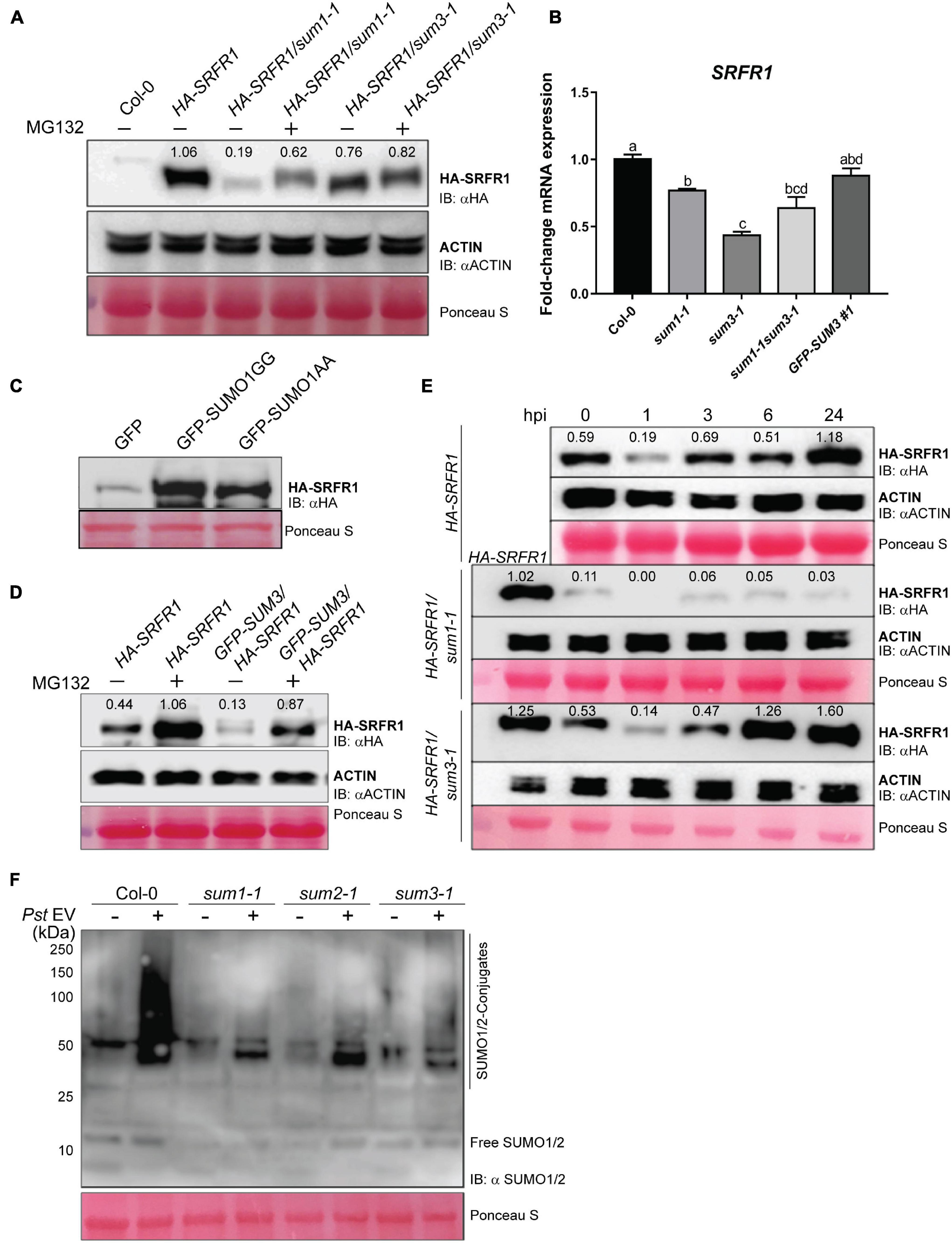
Figure 5. Steady-state levels of SRFR1 are lower in sum1-1 or sum3-1 plants. (A) HA-SRFR1 protein detection in Col-0 (negative control), wild-type (HA-SRFR1), HA-SRFR1/sum1-1, and HA-SRFR1/sum3-1 plants. (B) Relative abundance of SRFR1 transcripts in Col-0, sum1-1, sum3-1, sum1-1 sum3-1, or GFP-SUM3 overexpressing plants. MON1 expressions were used as the internal control, and values are mean ± SD (n = 3). Statistical significance is according to post hoc Tukey’s test (p < 0.05) and represented by different alphabets. HA-SRFR1 protein levels with overexpressed (C) GFP alone, or GFP-SUMO1 GG or AA forms. (D) GFP-SUMO3. (E) Changes in HA-SRFR1 protein at 0, 1, 3, 6, and 24 hpi PstDC3000 (Pst EV) infection (hpi) in wild-type (HA-SRFR1), HA-SRFR1/sum1-1, or HA-SRFR1/sum3-1 plants. (F) Accumulation of SUMO1/2-conjugates in Col-0, sum1-1, sum2-1, or sum3-1 at 24 hpi. Immunoblots were probed with anti-HA or anti-SUMO1 antibodies as mentioned. Ponceau S staining show protein loadings. Migration position of molecular weight standards (in kDa) is indicated. MG132 treatments, where indicated, was performed at 12 h prior to respective analysis. Numbers above the HA-SRFR1 bands are densitometric quantification values relative to actin expression levels in the same extracts.
Increased SA levels in sum1-1 result in upregulated SUM3 expression (van den Burg et al., 2010; Ingole et al., 2021b). To test whether reduced SRFR1 in sum1-1 is a consequence of elevated SUM3 expression, we generated HA-SRFR1/sum3-1 plants. From the segregating population, plants expressing HA-SRFR1 in sum3-1 background showed significantly lower (∼2-fold) SRFR1 transcript and protein levels than Col-0 (Figures 5A,B). Addition of MG132 did not improve HA-SRFR1 levels in sum3-1, implying that SUM3 regulates SRFR1 transcription (Figure 5A). Since we did not have the HA-SRFR1/sum1-1 sum3-1 plants to investigate their cumulative effect on SRFR1 protein, we checked SRFR1 transcript abundance in the sum1-1 sum3-1 plants that we reported recently (Ingole et al., 2021b). SRFR1 transcripts in sum1-1 sum3-1 were intermediate between sum1-1 and sum3-1 levels, suggesting interplay among the SUMO isoforms on its steady-state expressions (Figure 5B). SUM3 overexpression enhances basal defenses in Arabidopsis (van den Burg et al., 2010). With SUM3 role in SRFR1 transcriptions indicated from our results, we generated HA-SRFR1 plants that overexpressed GFP-tagged SUMO3 (GFP-SUM3). These plants (GFP-SUM3/HA-SRFR1 Lines#1 and #2) were homozygous for the sum3-1 mutation. Anti-GFP immunoblot detected overexpressed GFP-SUMO3 in extracts from the transgenic lines (Supplementary Figure 9). We continued with Line#1 for further assays. Although downregulated SRFR1 transcripts noted in sum3-1 restored to Col-0 levels in the GFP-SUM3 transgenic line, HA-SRFR1 protein were lower than the parental HA-SRFR1 (Figures 5B–D). Unlike what was noted for HA-SRFR1/sum3-1, MG132 treatment stabilized HA-SRFR1 protein in the GFP-SUM3 overexpressing plants, suggesting that as in sum1-1, the proteasome pathway was responsible for lower SRFR1 accumulations. Together with known SUM3 overexpression enhancing basal defenses, our results connect these responses again to consequences of reduced SRFR1 levels (van den Burg et al., 2010).
To further substantiate this, we challenged HA-SRFR1/sum1-1 or HA-SRFR1/sum3-1 plants with virulent PstDC3000 and evaluated SRFR1 protein changes (Figure 5E). Interestingly, lack of SUM1 delayed SRFR1 restoration, and even at 24 hpi, HA-SRFR1 levels were barely detectable. Contrastingly, HA-SRFR1 recovery was rapid in sum3-1 reaching native levels by 6 hpi. Accumulation of SUMO1/2-conjugates with PstDC3000 challenge was dramatically lower in sum3-1 and in agreement with our earlier observations (Ingole et al., 2021b; Figure 5F). Together, these data demonstrated intricate interplay between SUMO isoforms in basal defenses with SUM1 essential for SRFR1 restitution at post-transcriptional level and SUM3 modulating transcriptional efficiency as a part of feedback loop mechanism.
Upregulated SUM3 Maintains SRFR1 Protein Levels in Autoimmune esd4-2 Plants
The SUMO-protease ESD4 mutant (esd4-2) has increased SUMO1/2-conjugates, elevated SA, and enhanced basal defenses (Villajuana-Bonequi et al., 2014). We showed earlier that ESD4 transcriptions are downregulated during PstDC3000 infections (Ingole et al., 2021a). Therefore, with overall similarities to basal defense patterns, we investigated the fate of SRFR1 in esd4-2. SRFR1 transcripts were significantly higher (∼2-fold) likely due to higher SUM3 expressions in esd4-2 (Figure 6A; Villajuana-Bonequi et al., 2014). To assess protein levels, we generated HA-SRFR1/esd4-2 plants by crossing HA-SRFR1/sum3-1 to esd4-2. In F2 plants with loss of ESD4 but wild-type SUM3, SRFR1 protein levels were unchanged (Figure 6B). This contrast between SRFR1 transcriptional upregulations versus its unchanged protein levels in esd4-2 plants once again indicated complex regulations on SRFR1 expressions by SUMOylome perturbations.
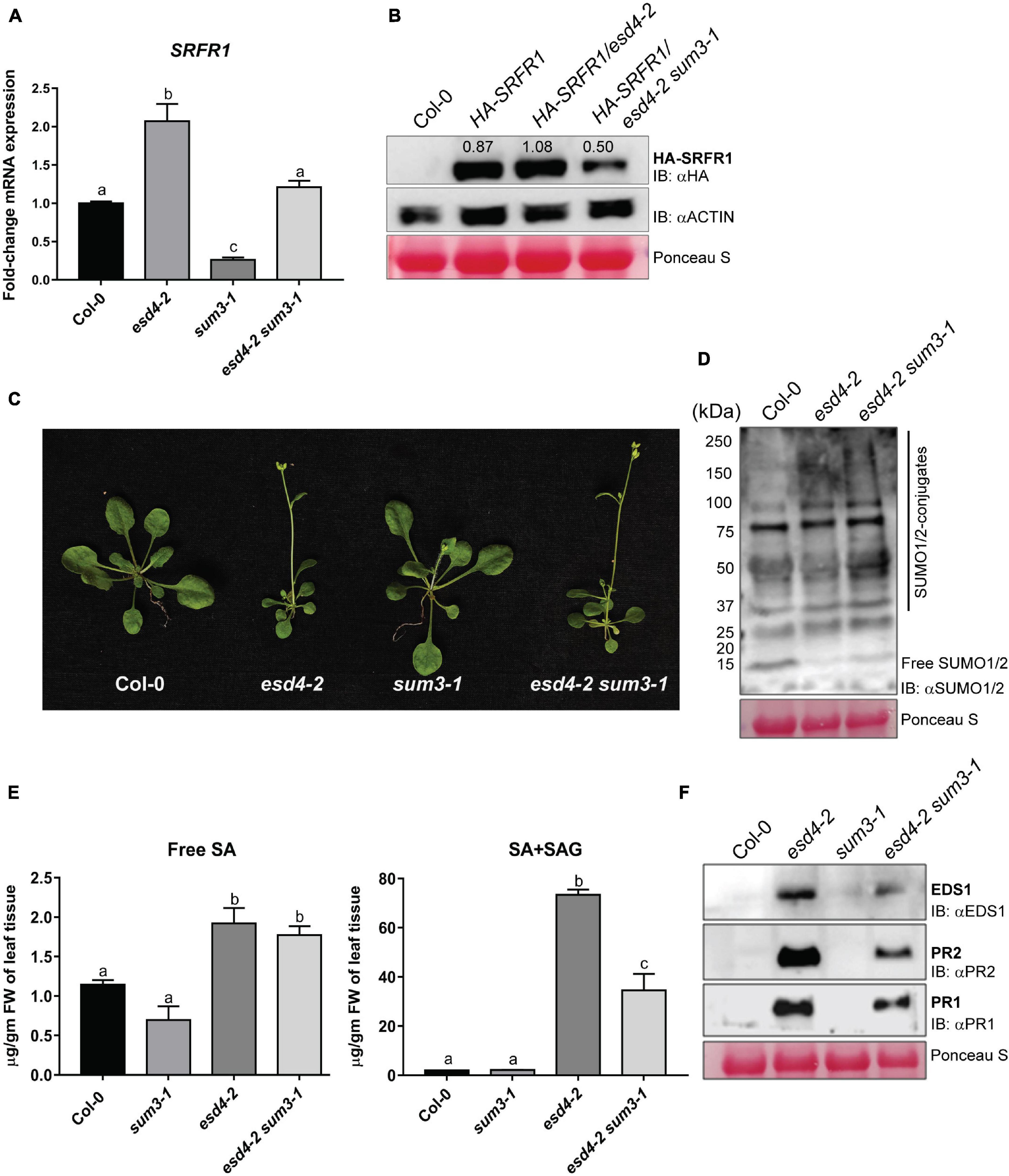
Figure 6. SUM3 is partially responsible for increased defenses but not growth phenotype of esd4-2. (A) SRFR1 transcript abundance in Col-0, esd4-2, sum3-1, and esd4-2 sum3-1 plants. Expressions were compared with MON1 levels and presented as fold-change relative to Col-0 (n = 3). Statistical significance is by post hoc Tukey’s test (p < 0.05) and shown by different letters. (B) HA-SRFR1 protein in wild-type (HA-SRFR1), HA-SRFR1/esd4-2, and HA-SRFR1/esd4-2 sum3-1 background. Col-0 is the negative control for the immunoblot. (C) esd4-2 sum3-1 plants resemble esd4-2 growth deficiencies. Images are representative of 4-week-old plants of indicated genotypes. (D) Global SUMOylome enhancements in esd4-2 and esd4-2 sum3-1 plants compared with Col-0. (E) Free and total SA levels, and (F) accumulation of EDS1, PR2, or PR1 proteins in esd4-2, sum3-1, and esd4-2 sum3-1 plants compared with Col-0. Protein blots were probed with anti-HA, anti-SUMO1/2, anti-EDS1, anti-PR1, or anti-PR2 antibodies, as indicated. Migration positions of SUMO1/2-conjugates, free SUMO1/2, or molecular weight standards (in kDa) are shown. Similar protein loadings are shown by Ponceau S staining. Numbers above the HA-SRFR1 bands in panel (B) are densitometric quantification values relative to actin expression levels in the same extracts.
To directly determine whether SUM3 contributed to this process, from the above cross, we identified HA-SRFR1/esd4-2 sum3-1 plants in the F2 and F3 populations. These plants were genetically similar to esd4-2 sum3-1 double mutant. Growth deficiencies including early bolting and enhanced global SUMO1/2-conjugates in esd4-2 were not affected by SUM3 loss (Figures 6C,D). Levels of free SUMO1/2, however, showed lower levels than in Col-0, suggesting that ESD4 SUMO-protease functions are essential for maintaining these pools of SUMOs. sum3-1 reduced total but not free SA levels and also lowered the protein or transcript abundance of several positive defense-associated players (EDS1, PR1, or PR2) that were elevated in the esd4-2 background (Figures 6E,F and Supplementary Figure 10A). Introducing sum3-1 in esd4-2 reduced SRFR1 transcripts to Col-0 levels, whereas protein abundance was lower than that in HA-SRFR1 or HA-SRFR1/esd4-2 plants (Figures 6A,B). We accord this to transcriptional promotion of SUM3 on SRFR1 observed earlier. Overall, these data reiterated that relative levels of SUMO1/3 modulate SRFR1 expression.
Several TFs are SUMOylation targets (Miller et al., 2010). SUMOylome disturbances cause expression differences of several SUMOylation-associated genes (Ingole et al., 2021b). To evaluate this in esd4-2 combination mutants, we performed quantitative real-time PCRs (qRT-PCRs). Expressions especially for SIZ1 and HPY2, the two E3 ligases, were higher and SUM3-independent in esd4-2 (Supplementary Figure 10C). SUM1 transcripts remain unaffected in all plants tested (Supplementary Figure 10B). Taken together, these data reflected consequences of deficient ESD4 activity rather than increased SUMOylation efficiencies by upregulated SIZ1/HPY2 or due to SUM3 involvement in their expressions as the primary cause of elevated SUMO1/2-conjugates in esd4-2 plants.
Enhanced Basal Defenses in srfr1-4 Are Partially SUM3-Dependent
Our results here and earlier suggested mutual expression influences between SRFR1 and SUMOylation-associated genes (Ingole et al., 2021a). To evaluate this interplay in the context of enhanced immunity in srfr1-4, we attempted to generate srfr1-4 sum1-1 or srfr1-4 sum3-1 double mutants. Considering SUM1 role as a negative immune regulator and SUM3 as a positive immune regulator, we tested whether pathogenesis outcomes of srfr1-4 are altered. From the F2/F3 segregating population of sum1-1 crossed with HA-SRFR1 plants used earlier, we screened for srfr1-4 sum1-1 double mutants. Even after extensive screening, srfr1-4 sum1-1 plants were not obtained, suggesting their embryonic lethality. It remains a possibility that developmental consequences that occur in srfr1-4 are compounded by the loss of SUM1. Observed growth and immune enhancements that we reported recently in sum1-1 support our speculation (Ingole et al., 2021b).
We identified srfr1-4 sum3-1 in the segregating populations of sum3-1 crossed with HA-SRFR1. As observed for srfr1-4 sid2-1 plants, sum3-1 mutation does not restore growth defects of srfr1-4 (Figure 7A). Enhanced SUMO1/2-conjugates were also unaffected by introducing sum3-1 in srfr1-4 (Figures 2B–D). Heightened expression of SCE1 but not SIZ1 in srfr1-4 was abolished in srfr1-4 sum3-1 plants, meaning that mis-regulations of some SUMOylation-associated genes in srfr1-4 are SUM3-dependent. To compare defense responses, these plants were challenged with virulent PstDC3000 or avirulent PstDC3000 (avrRps4) strains. Surprisingly, unlike deficient immunity in sum3-1 plants (Ingole et al., 2021b), bacterial accumulation for both strains in srfr1-4 sum3-1 was lower than in Col-0 but higher than in srfr1-4 (Figure 7B). Thus, srfr1-4 mutation was epistatic to sum3-1. Free SA elevations were abolished, whereas total SA levels though are reduced than srfr1-4 and remained significantly (∼20-fold) higher in srfr1-4 sum3-1 to Col-0 (Figure 7C). Relative expression levels of PR1, PR2, and SID2/ICS1 transcripts were also higher than those of Col-0 in srfr1-4 sum3-1 but lower than in srfr1-4 plants (Figure 7D). Similarly, protein levels of PR1, PR2, or EDS1 were also elevated than Col-0 but lower than srfr1-4 in the double mutant (Figure 7E). These results indicated that upregulated defenses in srfr1-4 only partially involved SUM3 contributions. Together, our data suggested that SRFR1 dynamics primarily modulated defense-associated SUMOylome changes and immune amplitudes in partial SUM3-dependent and SA-dependent manner. Overall, with our investigations, here we reveal an intricate molecular crosstalk between SRFR1 role in SUMOylome homeostasis/adjustments during defense and counter-repercussions on SRFR1 expressions by the SUMOylation changes overall to modulate immune amplitudes.
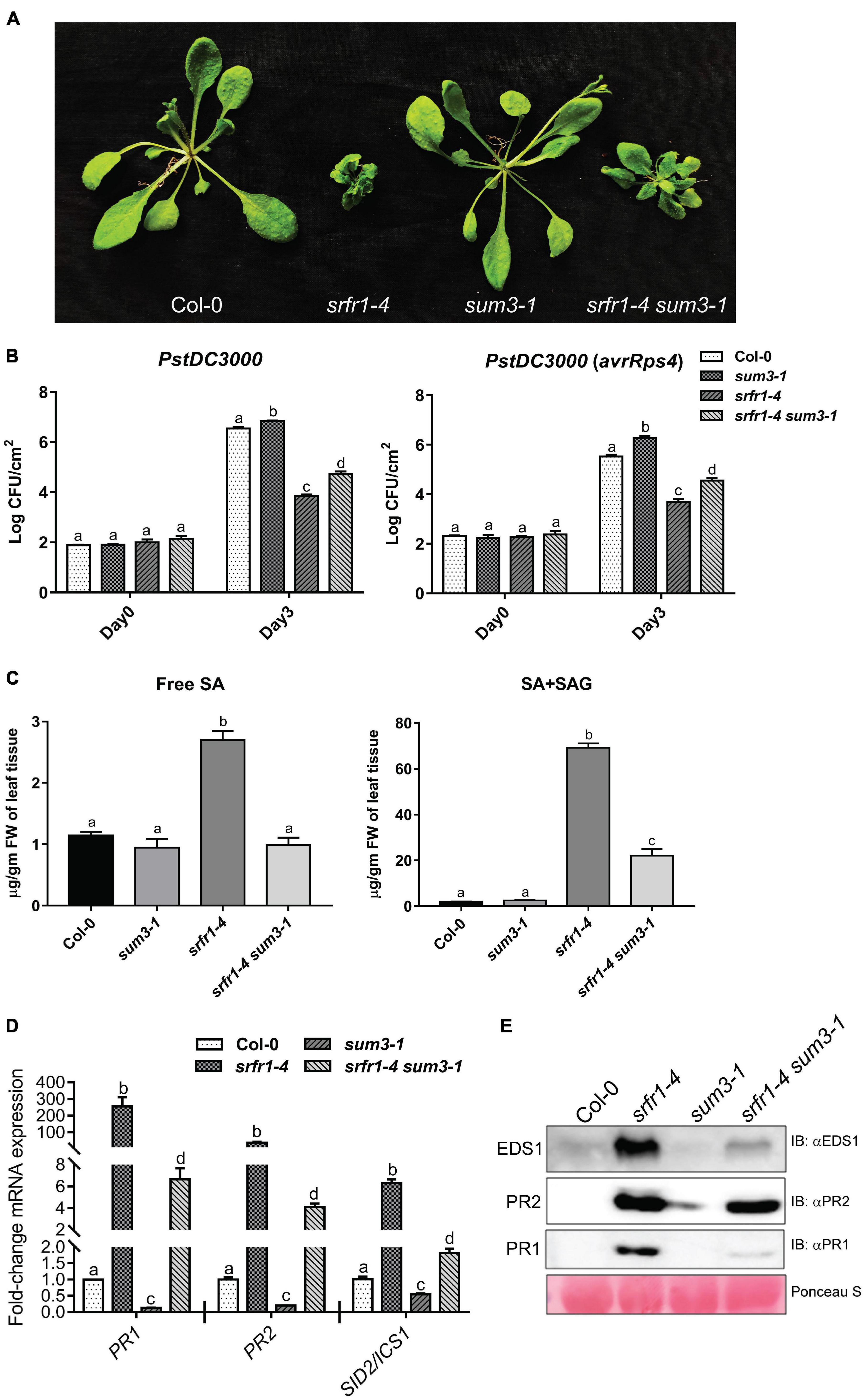
Figure 7. Enhanced defenses in srfr1-4 are partially SUM3-dependent. (A) Growth phenotypes of 3- to 4-week-old plants of indicated genotypes. (B) Bacterial growth measurements with virulent PstDC3000 or avirulent PstDC3000 (avrRps4) strains; (C) free and total SA levels; (D) transcript abundance of PR1, PR2, or SID2/ICS1; and (E) protein levels of EDS1, PR1, or PR2 in Col-0, srfr1-4, sum3-1, or srfr1-4 sum3-1 plants. Growth of bacterial strains at day 0 or day 3 post-challenge is shown. Statistical significance in transcript expression levels is via post hoc Tukey’s test (p < 0.05) and marked by different letters (n = 3).
Discussion
Pleiotropic phenotypes in the context of host SUMOylome alterations have remained restricted to studies in mutants of SUMOylation pathway genes (Lee et al., 2007; van den Burg et al., 2010; Villajuana-Bonequi et al., 2014; Bailey et al., 2016). We first demonstrated the role of a negative immune regulator SRFR1 in maintenance of host SUMOylome homeostasis (Ingole et al., 2021a). That loss of SRFR1 caused upregulated SUMOylation-promoting and downregulated SUMO protease expressions, a consequence mirrored in basal defenses, genetically placed SRFR1 as a transcriptional modulator of the host SUMOylome (Ingole et al., 2021a). Also, with our demonstration that SUM1 transcripts are preferentially loaded onto polysomes in srfr1-4 plants, we further expanded SRFR1 involvement also at the post-transcriptional level. Here, using srfr1-4 sid2-1 or srfr1-4 snc1-11 plants, we reaffirmed that similar to srfr1-4 eds1-2, elevated SA through SID2/ICS1, and SNC1 upregulated expressions in srfr1-4 are the principal cause of increased SUMO1/2-conjugates (Ingole et al., 2021a). Taken in the context of SUMO1/2-conjugation increase observed upon SA treatment, SRFR1 suppression of SID2/ICS1 expressions likely maintains global SUMOylome and mis-priming of immunity (Kim et al., 2010; Bhattacharjee et al., 2011; Bailey et al., 2016; Ingole et al., 2021a).
SA targets SUMO proteases OTS1/2 for proteasome-mediated degradation, thus enhancing SUMO1/2-conjugate levels (Bailey et al., 2016). We show here that SRFR1 undergoes transient instability when basal defenses are induced upon PstDC3000 exposure. The reduction in SRFR1 correlates with subsequent increase in SA levels and SUMO1/2-conjugate enhancements. Considering SA degrades OTS1/2, SRFR1 changes via downstream SA increase may drive the condemned fates of OTS1/2, presenting another example of its host SUMOylome adjustment role at the post-transcriptional level (Bailey et al., 2016). Recuperation of SRFR1 levels at latter time points of basal defense elicitation perhaps is indicative of fine-tuning of immune amplitudes, preventing overshoots or pleiotropic consequences like in srfr1-4 or in the SUMOylation-perturbed mutants such as siz1-2 or esd4-2 (Lee et al., 2007; Kim et al., 2010; Villajuana-Bonequi et al., 2014).
SUMOylome changes in turn regulate SRFR1 expressions. Firstly, in sum1-1 plants, unchanged SRFR1 transcripts but reduced protein levels are stabilized by the proteasome inhibitor MG132. Secondly, increased SRFR1 protein accumulation is detected by transient overexpression of SUM1 in N. benthamiana leaves. Thirdly, SRFR1 restoration is deficient in sum1-1 plants when challenged with PstDC3000. Last but not the least, gradual restoration of SRFR1 protein levels parallels progressive increase in SUMO1/2-conjugates during PstDC3000 challenges. Overall, these results suggest that SUM1 affects SRFR1 at a post-transcriptional level, possibly regulating protein turnover. Unlike sum1-1, reduced SRFR1 protein in sum3-1 matches its lower transcript levels. Taking into consideration our recent data that SUM3 potentiates SUMO1/2-conjugation efficiencies and in sum3-1 plants endogenous as well as defense-induced increase in SUMO1/2-conjugates are deficient, lower SRFR1 levels in this mutant likely also incorporate additive effects from this intersection (Ingole et al., 2021b). Thus, sum3-1 partially mimics sum1-1 consequences in destabilizing SRFR1. This notion is supported by our observation that MG132 treatment improves SRFR1 protein stability to a modest extent in sum3-1. Immune responses, however, remain deficient in sum3-1 likely because of functional inadequacies in the SA-signaling sector through NPR1, a known exclusive SUMO3-substrate (Saleh et al., 2015).
SUM3 expression is induced transiently at early time points of SA application (van den Burg et al., 2010). Increased SA levels and concomitant upregulation of SRFR1 transcripts during basal defenses noted in our data imply transcriptional contributions of SUM3 as we suggested above. However, SRFR1 instability also noted at same time points leads us to speculate that SUMO3, antagonistic to SUMO1, may negatively affect SRFR1 protein accumulation upon SUMOylation. Our data that SRFR1 is unstable in SUM3 overexpressing transgenic lines are in accordance with this hypothesis. Presence of multiple predicted SUMOylation motifs and in vitro SUMOylation by SUMO3 more prominent than for SUMO1, indeed, present SRFR1 as a candidate whose functions/stabilities may in turn be affected by host SUMOylome/SUMO isoform ratio changes. Stimulus-driven SUMO isoform switches that affect the stability of a substrate have been widely documented in animal systems (Meulmeester et al., 2008; Zhu et al., 2008, 2009). The mammalian GTPase activating protein RanGAP1, although is equally modified by SUMO1/2/3 in vitro, conjugation in vivo to SUMO1, but not SUMO2, imparts more stability from isopeptidases (Zhu et al., 2009). Similarly, HDAC1 is targeted for degradation upon SUMOylation by SUMO1, but not SUMO2 (Citro et al., 2013). Reduced SRFR1 levels in sum1-1 plants can therefore be also attributed to increased SUM3 expressions (Ingole et al., 2021a). However, that the same is not noted for esd4-2 plants with elevated SUM3 levels indicates that ESD4 functions are necessary for this process. Indeed, involvement of a SUMO-protease has been reported recently for stimulus-dependent SUMO paralog switching and its impact on the substrate stability (Fasci et al., 2015).
Bioinformatics predictions of SIMs in SRFR1 taken together with non-covalent binding of multiple SUMO isoforms also present another mode by which a change in SUMO homeostasis may impact SRFR1 activities. Our observation that SRFR1 interaction with EDS1K478R, a speculated SUMOylation-deficient version, is abolished indicates biological implication of SRFR1 SIMs in interaction with positive defense regulators. The placement of a predicted SIMs (I39LDIC43) in the first TPR motif of SRFR1 (residues 39–72, TPRpred, toolkit.tuebingen.mpg.de), a well-known platform for protein–protein interaction, introduces encouraging direction to pursue further. Precise mutagenesis of this and other predicted SIMs followed by interaction analysis with EDS1 or other known interactors (such as RPS4/6, or SNC1) is needed to be tested in vivo (Kim et al., 2010; Bhattacharjee et al., 2011). With our assays, here, we are, however, unable to convincingly demonstrate EDS1 as an in vivo SUMOylation candidate. Since only a minor pool of EDS1 interacts with SRFR1, in planta identification of SUMOylated EDS1 may prove challenging (Bhattacharjee et al., 2011). SNC1 or TCP8/14/15 also contains multiple predicted SUMOylation motifs. Although SUMOylated SNC1 or TCPs has been detected in vivo or in vitro, respectively, their functional relevance remains untested (Gou et al., 2017; Mazur et al., 2017). Consolidated SUMO1/3 SUMOylome changes have strong potential to interfere with SRFR1 properties at multiple levels and modulate immune signaling. A mechanistic implication into this is presented in the schematic (Figure 8).
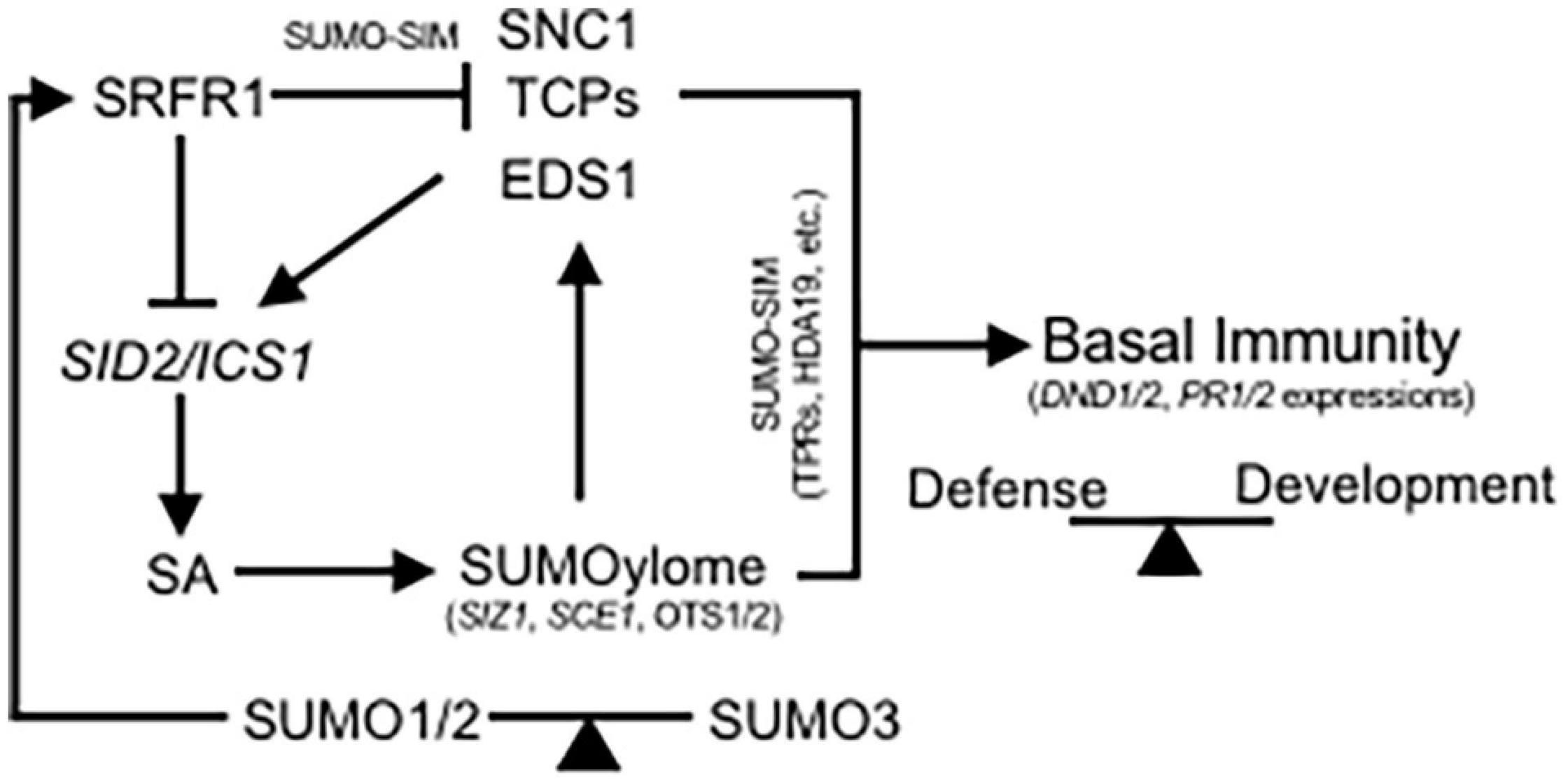
Figure 8. Simplified schematic representation of crosstalk between SRFR1 and SUMOylome maintenance in defense-developmental balance. Under homeostasis, SRFR1 prevents SUMOylome perturbations via its suppression of SID2/ICS1 expression that drive SA responses. This mode of negative regulation incorporates transcriptional suppression of SUMO-conjugases (such as SIZ1 and SCE1, among others), SUM3, and protein stabilities of SUMO proteases OTS1/2. Basal SUMOylome adjustments in turn reciprocates on SRFR1 function at multiple levels. As SUMOylation and/or SUMO-binding candidates, intermolecular SUMO-SIM-type associations of SRFR1 with SNC1, TCPs, and EDS1 prevent mis-primed immune activations. Similar nature of association occurring between these immune players and their downstream targets (TPRs and HDA19) regulates expression of defense-responsive genes such as DND1/2 and PR1/2, among others. SUMO1/2 or SUMO3 isoforms with their respective roles as negative or positive immune modulators balance the association stoichiometry through SRFR1 expression/stability. During a pathogen threat, transient reduction in SRFR1 initiates SUMOylome perturbations and activates defense players to execute defense signaling. A feedback signaling loop also initiated restoration of SRFR1 levels to avoid development penalties of constitutive immunity.
In summary, our results here provide a unique molecular basis into SRFR1 role in the SUMO-immune balances. With regulatory influences on expression of several SUMOylation-associated genes countered by SRFR1’s own stability determined by SUMO isoform crosstalk, we highlight its mediation of immune response amplitudes in plants (Ingole et al., 2021a). In addition to positive and negative immune regulators that are directly affected, SUMOylation efficiencies are also modulated by SUMO changes. This self-regulation is evident from the observation that SIZ1, SCE1, and ESD4 are themselves substrates for SUMOylation, and depending on the SUMO isoform they associate with, their localization or specificities are affected (Miller et al., 2010; Mazur et al., 2017). For example, SUMO1 bound to SCE1 SIM localizes the ternary complex (SUMO-SCE1-SIZ1) to nuclear bodies, whereas SUMO3 binding partitions it as nucleocytoplasmic. Overall, defense amplitudes therefore rely on strict harmony between SUMOylome modulators such as SRFR1, (de)SUMOylation efficiencies, localization of SUMOylation-machineries, and selection of substrates (Ingole et al., 2021a). Comprehensive elucidation of these events requires not only qualitative (i.e., which SUMO isoform-modified) but also quantitative (ratio of SUMOylated versus non-SUMOylated) evaluation of a host SUMOylome adaptation during immunity. While SUMO1/2-modified protein list is ever-increasing, SUMO3-targets remain grossly underrepresented. Development of an efficient SUMO3-enrichment system, on the similar theme to Miller et al. (2010), is therefore a necessity. Equally important is the characterization of protein–protein interactome changes that are defined by the SUMO-SIM nature. Lastly, functional intersection among SUMO-machineries, especially the SUMO-ligases/proteases, recently identified DeSIs (de-SUMOylating isopeptidases) (Orosa et al., 2018), STUbLs (SUMO-targeted ubiquitin ligases; Elrouby et al., 2013), and PIALs (E4-type SUMO ligases) (Tomanov et al., 2014), and their cognate substrates are warranted to decipher the net impact on immune signaling. Toward this endeavor, we present SRFR1 role in SUMOylome regulations and the srfr1-4 mutant as a promising system to pursue these investigations.
Data Availability Statement
The datasets presented in this study can be found in online repositories. The names of the repository/repositories and accession number(s) can be found below: PRIDE Archive, accession no: PXD026117.
Author Contributions
SB and WG conceived the research. MK, KI, SR, and SB designed the research. SR generated T91R SUMO1 clone. MK and KI generated other clones and plants used here and performed the experiments. MK, KI, and SB analyzed the data. MK, WG, and SB wrote the manuscript. All authors contributed to the article and approved the submitted version.
Funding
Funding sources are DST-SERB (Grant No: EMR/2016/001899) and DBT (Grant No. BT/PR23666/AGIII/103/1039/2018) awarded to SB, and US National Science Foundation grant IOS-1456181 awarded to WG.
Conflict of Interest
The authors declare that the research was conducted in the absence of any commercial or financial relationships that could be construed as a potential conflict of interest.
Publisher’s Note
All claims expressed in this article are solely those of the authors and do not necessarily represent those of their affiliated organizations, or those of the publisher, the editors and the reviewers. Any product that may be evaluated in this article, or claim that may be made by its manufacturer, is not guaranteed or endorsed by the publisher.
Acknowledgments
MK, KI, SR, and SB are deeply thankful to Regional Centre for Biotechnology (RCB), Faridabad, for providing financial support and central instrumental facilities. We acknowledge Katsunori Tanaka (Kwansei Gakuin University, Japan) for providing the E. coli SUMOylation system. Nirpendra Singh and his team at Advanced Technology Platform Centre (ATPC), Faridabad, India, provided support in LC-MS/MS analysis.
Supplementary Material
The Supplementary Material for this article can be found online at: https://www.frontiersin.org/articles/10.3389/fcell.2021.680760/full#supplementary-material
Supplementary Figure 1 | snc1-11 abolishes enhanced SUMO1/2-conjugates and restores elevated SA to Col-0 levels in srfr1-4.
Supplementary Figure 2 | MYB30 is SUMOylated in the E. coli SUMOylation reconstitution system by the T91R variant of SUMO1.
Supplementary Figure 3 | A consensus-type and a non-conventional lysine residue in SRFR1 is SUMOylated by SUMO1 in the E. coli SUMOylation reconstitution system.
Supplementary Figure 4 | Growth defects of srfr1-4 is abolished by the expression of HA-SRFR1K325R, HA-SRFR1K427R, or HA-SRFR1K325R+K427R variant.
Supplementary Figure 5 | EDS1 does not interact with SUMO1 AA or SUMO3 GG/AA.
Supplementary Figure 6 | EDS1 SUMOylation is not detected in the E. coli SUMOylation reconstitution system.
Supplementary Figure 7 | Enrichment of SUMO1-conjugates from SA-treated plants detect possible SUMOylated EDS1.
Supplementary Figure 8 | Over-expression of SUMO1 or SUMO3 in planta does not detect SUMOylated EDS1.
Supplementary Figure 9 | GFP-SUM3 overexpressing plants show detectable levels of GFP-SUMO3 proteins.
Supplementary Figure 10 | PR1, PR2, but not SIZ1 or HPY2 upregulation in esd4-2 plants is SUM3-dependent.
Supplementary Table 1 | List of oligonucleotide primers.
Footnotes
References
Aarts, N., Metz, M., Holub, E., Staskawicz, B. J., Daniels, M. J., and Parker, J. E. (1998). Different requirements for EDS1 and NDR1 by disease resistance genes define at least two R gene-mediated signaling pathways in Arabidopsis. Proc. Natl. Acad. Sci. U.S.A. 95, 10306–10311. doi: 10.1073/pnas.95.17.10306
Bailey, M., Srivastava, A., Conti, L., Nelis, S., Zhang, C., Florance, H., et al. (2016). Stability of small ubiquitin-like modifier (SUMO) proteases overly tolerant to SALT1 and -2 modulates salicylic acid signalling and SUMO1/2 conjugation in Arabidopsis thaliana. J. Exp. Bot. 67, 353–363. doi: 10.1093/jxb/erv468
Bentham, A. R., De La Concepcion, J. C., Mukhi, N., Zdrzalek, R., Draeger, M., Gorenkin, D., et al. (2020). A molecular roadmap to the plant immune system. J. Biol. Chem. 295, 14916–14935. doi: 10.1074/jbc.rev120.010852
Bhattacharjee, S., Halane, M. K., Kim, S. H., and Gassmann, W. (2011). Pathogen effectors target Arabidopsis EDS1 and alter its interactions with immune regulators. Science 334, 1405–1408. doi: 10.1126/science.1211592
Budhiraja, R., Hermkes, R., Muller, S., Schmidt, J., Colby, T., Panigrahi, K., et al. (2009). Substrates related to chromatin and to RNA-dependent processes are modified by Arabidopsis SUMO isoforms that differ in a conserved residue with influence on desumoylation. Plant Physiol. 149, 1529–1540. doi: 10.1104/pp.108.135053
Castano-Miquel, L., Segui, J., and Lois, L. M. (2011). Distinctive properties of Arabidopsis SUMO paralogues support the in vivo predominant role of AtSUMO1/2 isoforms. Biochem. J. 436, 581–590. doi: 10.1042/bj20101446
Chakrabarty, R., Banerjee, R., Chung, S. M., Farman, M., Citovsky, V., Hogenhout, S. A., et al. (2007). PSITE vectors for stable integration or transient expression of autofluorescent protein fusions in plants: probing Nicotiana benthamiana-virus interactions. Mol. Plant Microbe Interact. 20, 740–750. doi: 10.1094/mpmi-20-7-0740
Chosed, R., Mukherjee, S., Lois, L. M., and Orth, K. (2006). Evolution of a signalling system that incorporates both redundancy and diversity: Arabidopsis SUMOylation. Biochem. J. 398, 521–529. doi: 10.1042/bj20060426
Citro, S., Jaffray, E., Hay, R. T., Seiser, C., and Chiocca, S. (2013). A role for paralog-specific sumoylation in histone deacetylase 1 stability. J. Mol. Cell Biol. 5, 416–427.
Clough, S. J., and Bent, A. F. (1998). Floral dip: a simplified method for Agrobacterium-mediated transformation of Arabidopsis thaliana. Plant J. 16, 735–743. doi: 10.1046/j.1365-313x.1998.00343.x
Colby, T., Matthai, A., Boeckelmann, A., and Stuible, H. P. (2006). SUMO-conjugating and SUMO-deconjugating enzymes from Arabidopsis. Plant Physiol. 142, 318–332. doi: 10.1104/pp.106.085415
Cui, H., Gobbato, E., Kracher, B., Qiu, J., Bautor, J., and Parker, J. E. (2017). A core function of EDS1 with PAD4 is to protect the salicylic acid defense sector in Arabidopsis immunity. New Phytol. 213, 1802–1817. doi: 10.1111/nph.14302
Defraia, C. T., Schmelz, E. A., and Mou, Z. (2008). A rapid biosensor-based method for quantification of free and glucose-conjugated salicylic acid. Plant Methods 4:28.
Duan, G., and Walther, D. (2015). The roles of post-translational modifications in the context of protein interaction networks. PLoS Comput. Biol. 11:e1004049. doi: 10.1371/journal.pcbi.1004049
Elrouby, N., Bonequi, M. V., Porri, A., and Coupland, G. (2013). Identification of Arabidopsis SUMO-interacting proteins that regulate chromatin activity and developmental transitions. Proc. Natl. Acad. Sci. U.S.A. 110, 19956–19961. doi: 10.1073/pnas.1319985110
Falk, A., Feys, B. J., Frost, L. N., Jones, J. D., Daniels, M. J., and Parker, J. E. (1999). EDS1, an essential component of R gene-mediated disease resistance in Arabidopsis has homology to eukaryotic lipases. Proc. Natl. Acad. Sci. U.S.A. 96, 3292–3297. doi: 10.1073/pnas.96.6.3292
Fasci, D., Anania, V. G., Lill, J. R., and Salvesen, G. S. (2015). SUMO deconjugation is required for arsenic-triggered ubiquitylation of PML. Sci. Signal. 8:ra56. doi: 10.1126/scisignal.aaa3929
Feys, B. J., Moisan, L. J., Newman, M. A., and Parker, J. E. (2001). Direct interaction between the Arabidopsis disease resistance signaling proteins, EDS1 and PAD4. EMBO J. 20, 5400–5411. doi: 10.1093/emboj/20.19.5400
Flotho, A., and Melchior, F. (2013). Sumoylation: a regulatory protein modification in health and disease. Annu. Rev. Biochem. 82, 357–385. doi: 10.1146/annurev-biochem-061909-093311
Gao, J., and Xu, D. (2012). Correlation between posttranslational modification and intrinsic disorder in protein. Pac. Symp. Biocomput. 2012, 94–103. doi: 10.1142/9789814366496_0010
Garcia, A. V., Blanvillain-Baufume, S., Huibers, R. P., Wiermer, M., Li, G., Gobbato, E., et al. (2010). Balanced nuclear and cytoplasmic activities of EDS1 are required for a complete plant innate immune response. PLoS Pathog. 6:e1000970. doi: 10.1371/journal.ppat.1000970
Gareau, J. R., and Lima, C. D. (2010). The SUMO pathway: emerging mechanisms that shape specificity, conjugation and recognition. Nat. Rev. Mol. Cell Biol. 11, 861–871. doi: 10.1038/nrm3011
Gassmann, W., and Bhattacharjee, S. (2012). Effector-triggered immunity signaling: from gene-for-gene pathways to protein-protein interaction networks. Mol. Plant Microbe Interact. 25, 862–868. doi: 10.1094/MPMI-01-12-0024-IA
Geiss-Friedlander, R., and Melchior, F. (2007). Concepts in sumoylation: a decade on. Nat. Rev. Mol. Cell Biol. 8, 947–956. doi: 10.1038/nrm2293
Gou, M., Huang, Q., Qian, W., Zhang, Z., Jia, Z., and Hua, J. (2017). Sumoylation E3 ligase SIZ1 modulates plant immunity partly through the immune receptor gene SNC1 in Arabidopsis. Mol. Plant Microbe Interact. 30, 334–342. doi: 10.1094/MPMI-02-17-0041-R
Hannich, J. T., Lewis, A., Kroetz, M. B., Li, S. J., Heide, H., Emili, A., et al. (2005). Defining the SUMO-modified proteome by multiple approaches in Saccharomyces cerevisiae. J. Biol. Chem. 280, 4102–4110. doi: 10.1074/jbc.M413209200
Haynes, C., Oldfield, C. J., Ji, F., Klitgord, N., Cusick, M. E., Radivojac, P., et al. (2006). Intrinsic disorder is a common feature of hub proteins from four eukaryotic interactomes. PLoS Comput. Biol. 2:e100. doi: 10.1371/journal.pcbi.0020100
Ingole, K. D., Kasera, M., Van Den Burg, H. A., and Bhattacharjee, S. (2021b). Antagonism between SUMO1/2 and SUMO3 regulates SUMO conjugate levels and fine-tunes immunity. J. Exp. Bot. erab296. doi: 10.1093/jxb/erab296
Ingole, K. D., Dahale, S. K., and Bhattacharjee, S. (2021a). Proteomic analysis of SUMO1-SUMOylome changes during defense elicitation in Arabidopsis. J. Proteomics 232:104054. doi: 10.1016/j.jprot.2020.104054
Kim, S. H., Gao, F., Bhattacharjee, S., Adiasor, J. A., Nam, J. C., and Gassmann, W. (2010). The Arabidopsis resistance-like gene SNC1 is activated by mutations in SRFR1 and contributes to resistance to the bacterial effector AvrRps4. PLoS Pathog. 6:e1001172. doi: 10.1371/journal.ppat.1001172
Kong, X., Luo, X., Qu, G. P., Liu, P., and Jin, J. B. (2017). Arabidopsis SUMO protease ASP1 positively regulates flowering time partially through regulating FLC stability. J. Integr. Plant Biol. 59, 15–29. doi: 10.1111/jipb.12509
Kurepa, J., Walker, J. M., Smalle, J., Gosink, M. M., Davis, S. J., Durham, T. L., et al. (2003). The small ubiquitin-like modifier (SUMO) protein modification system in Arabidopsis. Accumulation of SUMO1 and -2 conjugates is increased by stress. J. Biol. Chem. 278, 6862–6872. doi: 10.1074/jbc.M209694200
Lee, J., Nam, J., Park, H. C., Na, G., Miura, K., Jin, J. B., et al. (2007). Salicylic acid-mediated innate immunity in Arabidopsis is regulated by SIZ1 SUMO E3 ligase. Plant J. 49, 79–90. doi: 10.1111/j.1365-313X.2006.02947.x
Liu, J., Faeder, J. R., and Camacho, C. J. (2009). Toward a quantitative theory of intrinsically disordered proteins and their function. Proc. Natl. Acad. Sci. U.S.A. 106, 19819–19823. doi: 10.1073/pnas.0907710106
Mazur, M. J., Spears, B. J., Djajasaputra, A., Van Der Gragt, M., Vlachakis, G., Beerens, B., et al. (2017). Arabidopsis TCP transcription factors interact with the SUMO conjugating machinery in nuclear foci. Front. Plant Sci. 8:2043. doi: 10.3389/fpls.2017.02043
Meulmeester, E., Kunze, M., Hsiao, H. H., Urlaub, H., and Melchior, F. (2008). Mechanism and consequences for paralog-specific sumoylation of ubiquitin-specific protease 25. Mol. Cell 30, 610–619. doi: 10.1016/j.molcel.2008.03.021
Millar, A. H., Heazlewood, J. L., Giglione, C., Holdsworth, M. J., Bachmair, A., and Schulze, W. X. (2019). The scope, functions, and dynamics of posttranslational protein modifications. Annu. Rev. Plant Biol. 70, 119–151. doi: 10.1146/annurev-arplant-050718-100211
Miller, M. J., Barrett-Wilt, G. A., Hua, Z., and Vierstra, R. D. (2010). Proteomic analyses identify a diverse array of nuclear processes affected by small ubiquitin-like modifier conjugation in Arabidopsis. Proc. Natl. Acad. Sci. U.S.A. 107, 16512–16517. doi: 10.1073/pnas.1004181107
Morrell, R., and Sadanandom, A. (2019). Dealing with stress: a review of plant SUMO proteases. Front. Plant Sci. 10:1122. doi: 10.3389/fpls.2019.01122
Murtas, G., Reeves, P. H., Fu, Y. F., Bancroft, I., Dean, C., and Coupland, G. (2003). A nuclear protease required for flowering-time regulation in Arabidopsis reduces the abundance of small ubiquitin-related modifier conjugates. Plant Cell 15, 2308–2319. doi: 10.1105/tpc.015487
Narasumani, M., and Harrison, P. M. (2018). Discerning evolutionary trends in post-translational modification and the effect of intrinsic disorder: analysis of methylation, acetylation and ubiquitination sites in human proteins. PLoS Comput. Biol. 14:e1006349. doi: 10.1371/journal.pcbi.1006349
Niu, D., Lin, X. L., Kong, X., Qu, G. P., Cai, B., Lee, J., et al. (2019). SIZ1-mediated SUMOylation of TPR1 suppresses plant immunity in Arabidopsis. Mol. Plant 12, 215–228. doi: 10.1016/j.molp.2018.12.002
Okada, S., Nagabuchi, M., Takamura, Y., Nakagawa, T., Shinmyozu, K., Nakayama, J., et al. (2009). Reconstitution of Arabidopsis thaliana SUMO pathways in E. coli: functional evaluation of SUMO machinery proteins and mapping of SUMOylation sites by mass spectrometry. Plant Cell Physiol. 50, 1049–1061. doi: 10.1093/pcp/pcp056
Orosa, B., Yates, G., Verma, V., Srivastava, A. K., Srivastava, M., Campanaro, A., et al. (2018). SUMO conjugation to the pattern recognition receptor FLS2 triggers intracellular signalling in plant innate immunity. Nat. Commun. 9:5185. doi: 10.1038/s41467-018-07696-8
Ren, J., Gao, X., Jin, C., Zhu, M., Wang, X., Shaw, A., et al. (2009). Systematic study of protein sumoylation: development of a site-specific predictor of SUMOsp 2.0. Proteomics 9, 3409–3412. doi: 10.1002/pmic.200800646
Saleh, A., Withers, J., Mohan, R., Marques, J., Gu, Y., Yan, S., et al. (2015). Posttranslational modifications of the master transcriptional regulator NPR1 enable dynamic but tight control of plant immune responses. Cell Host Microbe 18, 169–182. doi: 10.1016/j.chom.2015.07.005
Saracco, S. A., Miller, M. J., Kurepa, J., and Vierstra, R. D. (2007). Genetic analysis of SUMOylation in Arabidopsis: conjugation of SUMO1 and SUMO2 to nuclear proteins is essential. Plant Physiol. 145, 119–134. doi: 10.1104/pp.107.102285
Srivastava, M., Sadanandom, A., and Srivastava, A. K. (2021). Towards understanding the multifaceted role of SUMOylation in plant growth and development. Physiol. Plant. 171, 77–85. doi: 10.1111/ppl.13204
Tomanov, K., Zeschmann, A., Hermkes, R., Eifler, K., Ziba, I., Grieco, M., et al. (2014). Arabidopsis PIAL1 and 2 promote SUMO chain formation as E4-type SUMO ligases and are involved in stress responses and sulfur metabolism. Plant Cell 26, 4547–4560. doi: 10.1105/tpc.114.131300
van den Burg, H. A., Kini, R. K., Schuurink, R. C., and Takken, F. L. (2010). Arabidopsis small ubiquitin-like modifier paralogs have distinct functions in development and defense. Plant Cell 22, 1998–2016. doi: 10.1105/tpc.109.070961
Verma, V., Croley, F., and Sadanandom, A. (2018). Fifty shades of SUMO: its role in immunity and at the fulcrum of the growth-defence balance. Mol. Plant Pathol. 19, 1537–1544. doi: 10.1111/mpp.12625
Villajuana-Bonequi, M., Elrouby, N., Nordstrom, K., Griebel, T., Bachmair, A., and Coupland, G. (2014). Elevated salicylic acid levels conferred by increased expression of isochorismate synthase 1 contribute to hyperaccumulation of SUMO1 conjugates in the Arabidopsis mutant early in short days 4. Plant J. 79, 206–219. doi: 10.1111/tpj.12549
Wagner, S., Stuttmann, J., Rietz, S., Guerois, R., Brunstein, E., Bautor, J., et al. (2013). Structural basis for signaling by exclusive EDS1 heteromeric complexes with SAG101 or PAD4 in plant innate immunity. Cell Host Microbe 14, 619–630. doi: 10.1016/j.chom.2013.11.006
Walsh, C. T., Garneau-Tsodikova, S., and Gatto, G. J. Jr. (2005). Protein posttranslational modifications: the chemistry of proteome diversifications. Angew. Chem. Int. Ed. Engl. 44, 7342–7372. doi: 10.1002/anie.200501023
Zhao, X. (2018). SUMO-mediated regulation of nuclear functions and signaling processes. Mol. Cell 71, 409–418. doi: 10.1016/j.molcel.2018.07.027
Zhu, J., Zhu, S., Guzzo, C. M., Ellis, N. A., Sung, K. S., Choi, C. Y., et al. (2008). Small ubiquitin-related modifier (SUMO) binding determines substrate recognition and paralog-selective SUMO modification. J. Biol. Chem. 283, 29405–29415. doi: 10.1074/jbc.M803632200
Keywords: SUMOylation, SUMO isoforms, SRFR1, basal defenses, PstDC3000
Citation: Kasera M, Ingole KD, Rampuria S, Walia Y, Gassmann W and Bhattacharjee S (2021) Global SUMOylome Adjustments in Basal Defenses of Arabidopsis thaliana Involve Complex Interplay Between SMALL-UBIQUITIN LIKE MODIFIERs and the Negative Immune Regulator SUPPRESSOR OF rps4-RLD1. Front. Cell Dev. Biol. 9:680760. doi: 10.3389/fcell.2021.680760
Received: 15 March 2021; Accepted: 03 September 2021;
Published: 30 September 2021.
Edited by:
Girish S. Ratnaparkhi, Indian Institute of Science Education and Research, Pune, IndiaReviewed by:
Beatriz Orosa, University of Edinburgh, United KingdomEswarayya Ramireddy, Indian Institute of Science Education and Research, Tirupati, India
Copyright © 2021 Kasera, Ingole, Rampuria, Walia, Gassmann and Bhattacharjee. This is an open-access article distributed under the terms of the Creative Commons Attribution License (CC BY). The use, distribution or reproduction in other forums is permitted, provided the original author(s) and the copyright owner(s) are credited and that the original publication in this journal is cited, in accordance with accepted academic practice. No use, distribution or reproduction is permitted which does not comply with these terms.
*Correspondence: Saikat Bhattacharjee, c2Fpa2F0QHJjYi5yZXMuaW4=