- 1Department of Biomedical Sciences, Quillen College of Medicine, East Tennessee State University, Johnson City, TN, United States
- 2Center for Infectious Disease, Inflammation and Immunity, Quillen College of Medicine, East Tennessee State University, Johnson City, TN, United States
Chlamydia trachomatis infections represent the predominant cause of bacterial sexually transmitted infections. As an obligate intracellular bacterium, C. trachomatis is dependent on the host cell for survival, propagation, and transmission. Thus, factors that affect the host cell, including nutrition, cell cycle, and environmental signals, have the potential to impact chlamydial development. Previous studies have demonstrated that activation of Wnt/β-catenin signaling benefits C. trachomatis infections in fallopian tube epithelia. In cervical epithelial cells chlamydiae sequester β-catenin within the inclusion. These data indicate that chlamydiae interact with the Wnt signaling pathway in both the upper and lower female genital tract (FGT). However, hormonal activation of canonical and non-canonical Wnt signaling pathways is an essential component of cyclic remodeling in another prominent area of the FGT, the endometrium. Given this information, we hypothesized that Wnt signaling would impact chlamydial infection in endometrial epithelial cells. To investigate this hypothesis, we analyzed the effect of Wnt inhibition on chlamydial inclusion development and elementary body (EB) production in two endometrial cell lines, Ishikawa (IK) and Hec-1B, in nonpolarized cell culture and in a polarized endometrial epithelial (IK)/stromal (SHT-290) cell co-culture model. Inhibition of Wnt by the small molecule inhibitor (IWP2) significantly decreased inclusion size in IK and IK/SHT-290 cultures (p < 0.005) and chlamydial infectivity (p ≤ 0.01) in both IK and Hec-1B cells. Confocal and electron microscopy analysis of chlamydial inclusions revealed that Wnt inhibition caused chlamydiae to become aberrant in morphology. EB formation was also impaired in IK, Hec-1B and IK/SHT-290 cultures regardless of whether Wnt inhibition occurred throughout, in the middle (24 hpi) or late (36 hpi) during the development cycle. Overall, these data lead us to conclude that Wnt signaling in the endometrium is a key host pathway for the proper development of C. trachomatis.
Introduction
Chlamydia trachomatis genital infections represent the most common notifiable infectious disease in the United States with 1.5 million cases reported in 2015. In fact, C. trachomatis has been one of the most prevalent sexually transmitted pathogens in the United States for over two decades (CDC, 2015). Young women, ages 15–29, are disproportionately affected by chlamydial infections, reporting 36% higher rates of infection compared to males of the same age group (CDC, 2015). While many of these infections are clinically mild or asymptomatic, they can contribute to infertility and increase the risk of potentially life-threatening medical emergencies, such as ectopic pregnancy (Peipert, 2003).
Chlamydia trachomatis is an obligate intracellular, Gram negative bacterium that possesses a biphasic developmental cycle. Extracellularly, chlamydiae exist in a condensed, infectious form, called elementary bodies (EB). Upon transmission to a new host, EB infect genital luminal and glandular epithelial cells, where they establish a modified endocytic vesicle called an inclusion. After inclusion formation, EB decondense to form larger, metabolically active, non-infectious forms called reticulate bodies (RB). RB undergo several rounds of division within the inclusion before converting into EB, which are released from the infected cell and disseminate to new host cells (Wyrick, 2000; Elwell et al., 2016). Due to their obligate intracellular lifestyle, chlamydiae have evolved mechanisms for interaction with numerous host cell pathways to acquire the essential factors for replication. Interaction of host signaling components with inclusion membrane proteins manipulates host trafficking and signaling so that nutrients are diverted to the RB and prevent host cell death (Elwell et al., 2016). Thus, the general health and signaling environment of genital epithelial cells play a role in chlamydial development.
One group of host pathways that is known to regulate endometrial epithelial cells are the Wnt signaling pathways. At least 20 Wnt signaling ligands have been identified (van der Horst et al., 2012). Wnt signaling plays a major role in embryonic development and regulation of adult tissues (Sonderegger et al., 2010; Wang et al., 2010; van der Horst et al., 2012; Tepekoy et al., 2015). In the endometrium, Wnt signals are secreted from epithelial cells and stromal cells in response to sex hormones (Das et al., 2000; Tulac et al., 2003, 2006). In the canonical pathway, Wnt molecules bind to Frizzled (FZD) receptors on the surface of endometrial cells. Once Wnt is bound to FZD, the receptor interacts with a low density lipoprotein receptor-related protein co-receptor, LRP5/6. This interaction stimulates the recruitment and subsequent disruption of the β-catenin destruction complex, composed of adenomatous polyposis coli (APC), axin, casein kinase Iα and glycogen synthase kinase 3β (GSK-3β). Disruption of the destruction complex by Wnt signaling prevents the degradation of β-catenin that occurs in the absence of Wnt, allowing β-catenin to accumulate in the cytoplasm. β-catenin then translocates to the nucleus where it impacts transcription of genes, including genes that regulate cell survival, migration, and proliferation (Tulac et al., 2003; Wang et al., 2010). Non-canonical Wnt signaling does not elicit action through β-catenin and is less defined due to the multiple signaling pathways that can be activated through this process. The Wnt/planar cell polarity pathway activates GTPases RhoA and Rac, which activate downstream effectors including JNK. The Wnt/Ca2+ pathway stimulates the release of calcium from the endoplasmic reticulum. Additionally, non-canonical Wnt signaling can activate ERK and PI3K/AKT signaling (Sonderegger et al., 2010). Thus, cellular signaling via Wnt molecules can impact endometrial cells by altering gene transcription or through downstream activation of additional signaling pathways.
Studies have suggested that Wnt/β-catenin signaling positively influences chlamydial infection by altering epithelial cell structure and/or decreasing host cell apoptosis during infection. Prozialeck et al. demonstrated that N-cadherin/β-catenin cellular junctional complexes were disrupted in C. trachomatis-infected cervical epithelial cells and that β-catenin co-localized to the chlamydial inclusion in these cultures (Prozialeck et al., 2002). In fact, knockdown of β-catenin expression in infected host cells decreases both C. trachomatis and C. pneumoniae infectivity (Kessler et al., 2012; Flores and Zhong, 2015). Activation of Wnt signaling and co-localization of β-catenin to the inclusion has also been demonstrated in C. trachomatis-infected ex-planted fallopian tubes. Kessler et al., demonstrated that Wnt activation by chlamydial infection disrupted epithelial cell proliferation, polarity and adhesion in both infected and neighboring epithelial cells, indicating that chlamydial infection impacts uninfected cells via paracrine Wnt signals. (Kessler et al., 2012). Additionally, the C. pneumoniae protein Cpn1027 has been shown to interact with the Wnt signaling regulators and members of the β-catenin destruction complex, cytoplasmic activation/proliferation associated protein 2 (caprin 2) and GSK-3β, potentially increasing β-catenin accumulation in the infected cell and expression of β-catenin-regulated anti-apoptotic genes (Flores and Zhong, 2015).
A unique feature of epithelial cells lining the endometrium of the female genital tract (FGT) is that they are stimulated to proliferate, differentiate, and are shed in response to hormone-induced signaling events during the menstrual cycle. Interestingly, modulation of Wnt signaling pathways by sex hormones plays a role in this cyclic remodeling of the endometrium. (Sonderegger et al., 2010; Wang et al., 2010; van der Horst et al., 2012; Tepekoy et al., 2015). Given the previous observations that Wnt/β-catenin signaling impacts chlamydial infection in fallopian tubes and in cervical cells, we sought to determine if inhibiting Wnt signals in endometrial epithelial cells has a negative influence on chlamydial development.
Methods
Culture of Endometrial Epithelial Cell Lines and Chlamydia
Ishikawa (IK), Hec-1B and an endometrial stromal cell line (SHT-290) were maintained as described previously (Hall et al., 2011). Briefly, IK, Hec-1B, and SHT-290 cells were maintained in phenol-red free Dulbecco's modified Eagle's medium (DMEM; Gibco) with 1x glutamax (Gibco). IK and Hec-1B medium was supplemented with 5 or 10% FBS, respectively. SHT-290 medium was supplemented with 2% charcoal-stripped FBS (CSFBS). C. trachomatis Serovar E stocks were prepared in Hec-1B cells grown in bead culture as previously described (Guseva et al., 2007). Short tandem repeat profiling was performed by the American Type Culture Collection to authenticate the identity and/or origin of all cell lines used in this study (data not shown). All cell lines were tested for Mycoplasma by PCR and found to be free of contamination (data not shown).
Wnt Inhibitor Exposure
Ishikawa (IK) cells were cultured on Matrigel-coated filters (Corning) until they reached 75% confluency. The IK cultures were placed into wells containing SHT-290 monolayers growing on the bottom of the well so that the IK and SHT-290 monolayers were in close proximity to each other, but not touching. Co-cultures were replenished with SHT-290 medium containing DMSO or 5 uM IWP2 (Tocris) and incubated at 37°C for 48 h. At this time, the medium was removed from the cultures and an inoculum of C. trachomatis EB in 2SPG (0.2 M sucrose, 0.02 M phosphate buffer, and 5 mM l-glutamine) was overlaid on the IK monolayers. A low MOI of 0.03 IFU/cell was used for all studies so that the percentage of infected cells could be accurately determined. SHT-290 monolayers were overlaid with an equal volume of mock inoculum (2SPG) to prevent drying. The cultures were incubated for 1 h at 35°C before the inoculum was removed. The cultures were refed with the appropriate experimental medium containing DMSO or IWP2 and incubated for 48 h at 35°C before collection for various analyses. Alternatively, replicate Hec-1B and/or IK nonpolarized monolayers were exposed to IWP2 or 25 uM IWR-1 (Selleckchem) as described above or at 0, 24, or 36 h post Chlamydia infection (hpi). The chlamydia-infected, DMSO, IWP2 or IWR-1-exposed Hec-1B and/or IK monolayers were collected at 44 hpi. In duplicate experiments, samples were washed and refed with culture medium containing neither DMSO nor IWP2 at 44 hpi. These cultures were incubated for an additional 72 h at 35°C before collection. Inhibitor concentrations were chosen based upon the manufacture's and published literature in combination with preliminary dose response curves in which the viability of host cells was determined by monitoring cell growth and Live/Dead assay (Kessler et al., 2012; Lee et al., 2015).
Percent Infectivity
Infected IK or Hec-1B samples were fixed with methanol and stained for chlamydial inclusions using Pathfinder anti-chlamydia MOMP stain (BioRad). Cell nuclei were stained with DAPI (ThermoFisher). Stained coverslips and filters were mounted on glass slides. Chlamydial inclusions and nuclei were visualized on a Ziess Axiovert Discovery Microscope at 400x magnification. In each experiment, the number of inclusions and nuclei were counted in 15 random fields from triplicate samples and the average percentage of infected cells was calculated per condition.
EB Titer Analysis
Chlamydia trachomatis-infected monolayers were scraped into the culture medium and frozen at −80°C. EB released from the cells by freeze/thaw and sonication were diluted in culture medium and used to infect HeLa 229 monolayers grown on coverslips by spin infection (1 h, 1,100x g). Triplicate infected monolayers were incubated for 48 h at 35°C before methanol fixation and staining with Pathfinder anti-chlamydia MOMP stain (BioRad). Total inclusions were counted on each coverslip using a Ziess Axiovert Discovery Microscope at 200x magnification. The average number of inclusion forming units (IFU)/ml was calculated per sample and experimental condition.
Inclusion Size Measurement
Triplicate chlamydia-infected IK and Hec-1B nonpolarized monolayers grown on coverslips or polarized IK monolayers from IK/SHT-290 co-cultures were stained with Pathfinder anti-chlamydia MOMP stain (BioRad). Five images of random fields were taken of each sample at 630x magnification using a Ziess Axiovert Discovery Microscope. The area of 10–12 inclusions/sample was measured using Zen Professional Imaging Software (Ziess). The average inclusion size was determined for each experimental condition.
Live/Dead Assay
Ishikawa (IK) cultures were exposed to DMSO or IWP2 for a period of 1–4 days at 35°C. Cell death in the cultures was assessed using the Live/Dead Reduced Biohazard Viability/Cytotoxicity Kit (Molecular Probes) according to the manufacturer's instructions.
Confocal Microscopy Analysis
Infected IK monolayers from IK/SHT-290 co-cultures exposed to DMSO or IWP2 were stained for percent infectivity analysis as described above. Alternatively, IK and Hec-1B nonpolarized monolayers were stained with anti-β-catenin (Cell Signaling), and anti-chlamydial MOMP (Meridian LifeSciences) primary antibodies followed by staining with rabbit-anti-mouse Alexa Fluor 488 (Molecular Probes), rabbit-anti-goat Alexa Fluor 594 (Molecular Probes) secondary antibodies, and DAPI (ThermoFisher) stain. Confocal images of chlamydial inclusions were captured on a Leica TCS SP8 with Leica LASX software at 630x or 1,000x magnification. Imaris imaging and Leica LASX software were used to analyze the images. All images used in intensity measurements were taken at a standard exposure. Digital slices representing the center depth of 24 inclusions/condition were used to determine the intensity of β-catenin staining inside the inclusion. The intensity of β-catenin staining within a region of the host cell equivalent to the area of each inclusion was determined to assess the ratio of β-catenin staining inside the inclusion compared to the cell cytoplasm and/or nucleus.
Transmission Electron Microscopy
Infected IK monolayers from IK/SHT-290 co-cultures exposed to DMSO or IWP2 were fixed with glutaraldehyde in 0.1 M Cacodylate buffer (EM Sciences) for 24 h. The monolayers were then collected, agar enrobed, and stained with osmium tetroxide and uranyl acetate before alcohol and propylene oxide dehydration. Dehydrated samples were embedded in EPON (Polysciences, Inc Araldite 502/PolyBed 812 Kit) embedding medium (Wyrick et al., 1994). Thin sections were examined on a Tecnai 10 (FEI) transmission electron microscope operating at 60–80 kV.
Western Blot Analysis
Infected IK and SHT-290 monolayers from IK/SHT-290 co-cultures were collected in 8 M urea. The protein samples were examined by SDS-PAGE electrophoresis for Sypro Ruby (BioRad) staining and Western Blot analysis as previously described (Deka et al., 2006). Blots were probed with anti-β-catenin antibodies (Cell Signaling). Protein accumulation in each experiment was quantified from triplicate samples using a BioRad G-box and SynGene software. β-catenin accumulation was normalized to the total amount of protein detected in each sample by Sypro Ruby staining.
Statistical Analysis
All experiments contained three biological replicates and were independently repeated a minimum of three times. Values from independent experiments were averaged and presented as the mean ± the standard error of the mean (SEM). Means were compared by Analysis of Variance (ANOVA) and independent two-sample T-tests using MiniTab Version 17 Statistical Software and Microsoft Excel. Comparisons with p ≤ 0.05 were considered significantly different.
Results
Inhibition of Wnt in IK/SHT-290 Co-culture Decreases Chlamydial Infectivity and EB Production
To determine if Wnt signaling is important for chlamydial development in the endometrium, we examined the effects of a Wnt inhibitor, IWP2 on chlamydial infection in an endometrial epithelial cell (IK)/stromal (SHT-290) cell co-culture model. This physiologically relevant co-culture model incorporates paracrine regulatory signaling events that occur between endometrial stromal and epithelial cells (Arnold et al., 2001, 2002). IWP2 is a small molecule inhibitor of Wnt protein production that has the ability to inhibit both the canonical, Wnt/β-catenin signaling pathways and non-canonical Wnt signaling pathways (Chen et al., 2009). Replicate IK/SHT-290 co-cultures were prepared as described in the methods. The co-cultures were exposed to DMSO or 5 uM IWP2 48 h prior to and following C. trachomatis infection. Replicate samples were harvested at 44 hpi for chlamydial infectivity, EB titer analysis, inclusion size measurements and Western blot analysis of β-catenin accumulation. In a separate study, Live/Dead assays revealed that prolonged exposure to 5 uM IWP2 did not increase IK cell death significantly compared to the DMSO control (data not shown). Chlamydial infectivity was lowered in the presence of Wnt inhibition compared to DMSO exposure, although not to a significant degree (Figure 1A, p = 0.20). Inhibition of Wnt by IWP2 significantly decreased the production of infectious EB during IK/SHT-290 co-culture (Figure 1B, p = 0.002). Inclusion size was also significantly decreased in cultures exposed to IWP2 compared to DMSO (Figure 1C, p = 0.0002). Accumulation of β-catenin was significantly decreased in SHT-290 cells (p = 0.04), but not in IK cells (p = 0.60) from infected IK/SHT-290 co-cultures (Figure 1D, Supplemental Figure 1).
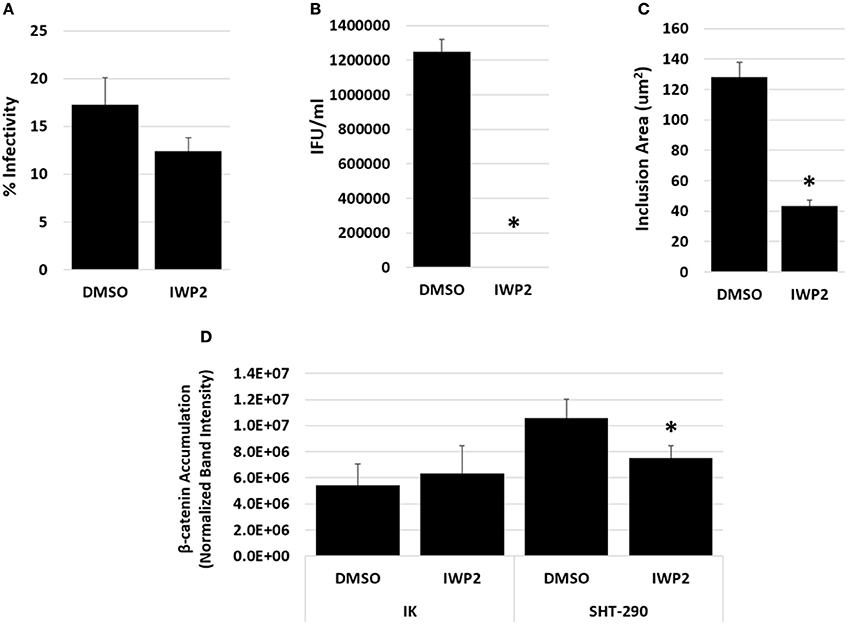
Figure 1. Impact of Wnt inhibition on chlamydial infection in IK/SHT-290 co-culture. Replicate IK/SHT-290 co-cultures were exposed to DMSO or the Wnt inhibitor, IWP2, prior to and following C. trachomatis infection. At 44 hpi, the infected IK monolayers and SHT-290 monolayers were collected and processed for (A) percent infectivity analysis, (B) EB titer assay, (C) inclusion size measurement, (D) and Western blot analysis of β-catenin accumulation. The data shown represent three independent experiments with 3 biological replicates per experiment. Comparison of means was performed using a 2-sample T-test for independent samples. P ≤ 0.05 were considered statistically significant. *Indicates a significant difference (p ≤ 0.05) from the DMSO control.
Examination of chlamydia-infected IK monolayers exposed to DMSO or IWP2 during co-culture by TEM and confocal microscopy reveal that Wnt inhibition caused inclusions to be smaller in size and contain aberrant RB (Figures 2B,D, yellow arrows) compared to DMSO-exposed controls, which had large inclusions, containing numerous RB (white arrows) and EB (green arrow) (Figures 2A,C). These data indicate that inhibition of Wnt signaling in IK/SHT-290 co-cultures inhibits chlamydial development and production of infectious progeny. Moreover, formation of aberrant RB suggests that Wnt inhibition may cause developing chlamydiae to enter into a stressed or persistent state. Given that β-catenin accumulation was decreased in SHT-290 cells but not in IK cells, these data suggest that Wnt inhibition during co-culture impacts chlamydial infection by inhibiting paracrine stromal cell effectors released in response to canonical Wnt/β-catenin activation. Alternatively, inhibition of non-canonical Wnt signaling pathways or alterations in β-catenin localization in infected IK cells may contribute to the negative effect of Wnt inhibition on chlamydial infection.
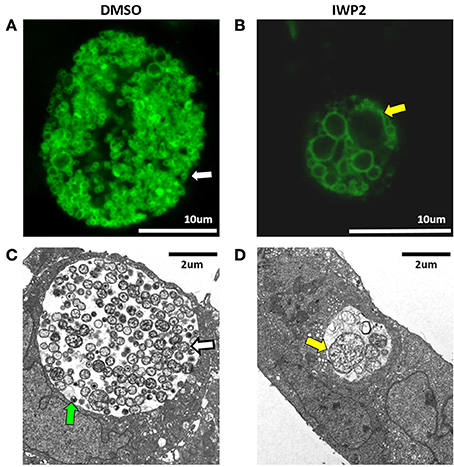
Figure 2. Confocal and transmission electron microscopy of chlamydia-infected IK cells cultivated in the presence of DMSO or IWP2 during IK/SHT-290 co-culture. Replicate IK/SHT-290 co-cultures were exposed to DMSO or the Wnt inhibitor, IWP2, prior to and following C. trachomatis infection. At 44 hpi, the infected IK monolayers were fixed, stained for chlamydia and visualized by confocal microscopy (A,B, green) or processed for TEM (C,D). Shown are representative images of chlamydial inclusions in infected IK cells exposed to DMSO (A,C) or IWP2 (B,D). Confocal images were captured at 1,000x magnification. TEM images were captured at 7,000x magnification. EB, normal RB, and aberrant RB are indicated by the green, white and yellow arrows, respectively.
Inhibition of Wnt in Nonpolarized IK and Hec-1B Cells Decreases Infectivity and EB Progeny Production
To ensure that our observed results were not limited to IWP2-exposed, chlamydia-infected IK/SHT-290 co-cultures, we repeated our studies using nonpolarized IK cells and a second endometrial cell line, Hec-1B. Nonpolarized IK or Hec-1B cell monolayers were exposed to DMSO or IWP2 48h prior to C. trachomatis infection. Replicate cultures were exposed to IWP2 at 0, 24, or 36 h post C. trachomatis infection. Samples were harvested for various analyses at 44 hpi. Data from chlamydial persistence models demonstrate that aberrant chlamydiae recover infectivity from this stressed state when the persistence inducer is removed (Hogan et al., 2004; Vanover et al., 2010). Given that we observed aberrant RB morphology during IWP2 exposure in IK/SHT-290 co-culture, we sought to determine if C. trachomatis could recover from Wnt inhibition by IWP2. Duplicate samples were washed with sterile phosphate buffered saline and refed with medium containing no diluent or inhibitor. These samples were allowed to recover for 72 h (72 h R) before collection for EB titer analysis. Compared to DMSO-exposed controls, chlamydial infectivity was significantly decreased in IK cultures when exposed to IWP2 before infection (p < 0.001). However, addition of the Wnt inhibitor at times after infection did not significantly impact chlamydial inclusion development in IK cells (Figure 3A, black bars). In Hec-1B cultures, inclusion development was decreased compared to the control when IWP2 was added before or at 0 hpi (p = 0.013) chlamydial infection; however, Wnt inhibition had no significant effect on continued inclusion development when IWP2 was added to Hec-1B cultures at 24 or 36 hpi (Figure 3A, white bars). Inhibition of Wnt significantly decreased the average size of inclusions in IK cells compared to DMSO exposure (Figure 3B, p = 0.0046). Inclusion size in Hec-1B cells was also decreased in response to Wnt inhibition, but not to a significant degree (Figure 3B, p = 0.07). EB production was suppressed in both IK (Figure 3C) and Hec-1B (Figure 3D) cultures exposed to IWP2 at 44 hpi (p < 0.0001). Inclusion size in IWP2-exposed samples was increased at 72 h R compared to 44 hpi (Supplemental Figure 2), but EB production did not recover appreciably in IK or Hec-1B cells following the removal of the Wnt inhibitor except in IK cultures exposed to IWP2 at 36 hpi (Figures 3C,D). Western blot analysis demonstrated that IWP2 exposure did not significantly alter accumulation of β-catenin in nonpolarized IK or Hec-1B cultures at 44 hpi (Supplemental Figure 3).
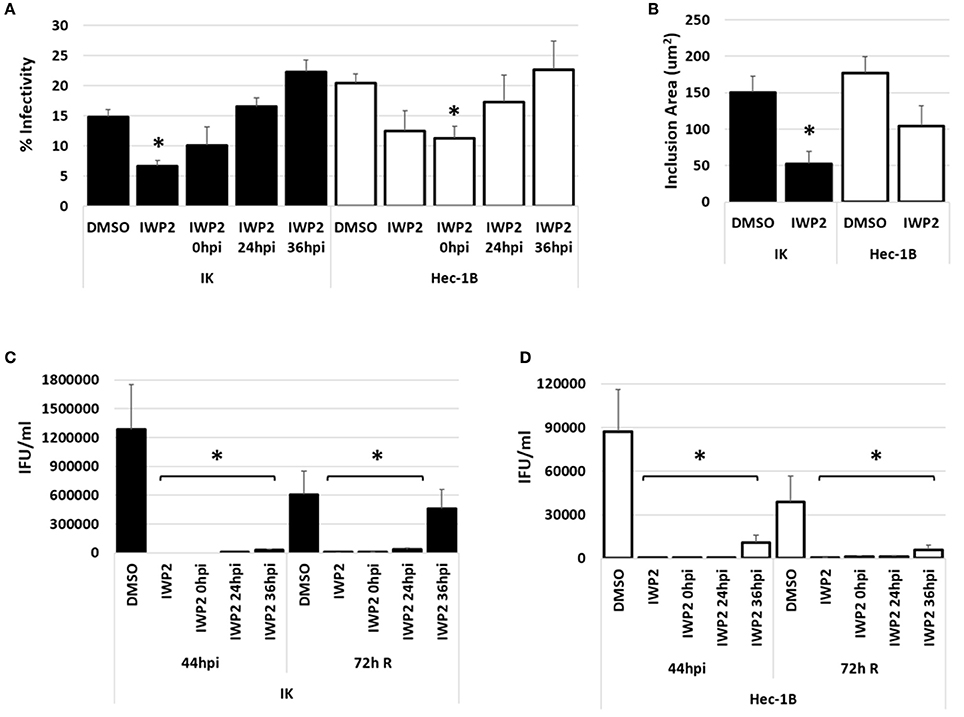
Figure 3. Impact of Wnt inhibition by IWP2 on chlamydial infection in nonpolarized endometrial epithelial cells, IK and Hec-1B. Nonpolarized IK or Hec-1B cell monolayers were exposed to DMSO or IWP2 prior to and/or after (0, 24, or 36 hpi) C. trachomatis infection. Samples were harvested for infectivity and EB titer assay at 44 hpi. Replicate samples were replenished with fresh medium at 44 hpi and collected 72h after removal of DMSO or IWP2 from the cultures (72 h R). (A) Chlamydial percent infectivity and (B) average inclusion size in IK (black bars) or Hec-1B (white bars) cultures at 44 hpi. (C,D) EB progeny production from infected IK (C) or Hec-1B (D) cultures exposed to DMSO or IWP2 at 44 hpi and 72 h R. Comparison of means was performed using ANOVA and 2-sample T-tests for independent samples. *Indicates a significant difference (p ≤ 0.05) from the DMSO control.
Confocal microscopy examination of fluorescently labeled β-catenin in DMSO or IWP2-exposed, chlamydia-infected IK or Hec-1B cultures revealed that β-catenin localized to chlamydial inclusions under both experimental conditions (Figures 4A,B). Although elevated by approximately 2 fold, the intensity of β-catenin staining observed within inclusions was not significantly different than that observed in the host cell in the DMSO-exposed controls, suggesting that while β-catenin is present in the inclusion it was not concentrated there (Figure 4C). IWP2 exposure decreased β-catenin localization in the inclusion compared to the DMSO controls in infected Hec-1B cultures (Figure 4C, white bars, p = 0.003). Overall, these data indicate that inhibition of Wnt signaling in nonpolarized endometrial epithelial cells severely inhibits chlamydial inclusion development and infectious progeny production. Importantly, the detrimental effects of Wnt inhibition on C. trachomatis was evident no matter if the inhibition occurred before infection, or during early, mid, or late infection, indicating that Wnt signaling is important at every stage of the chlamydial intracellular development cycle.
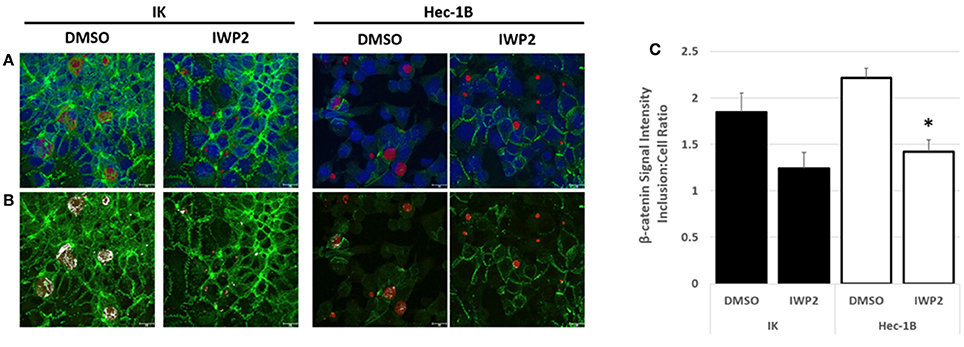
Figure 4. Confocal microscopy of chlamydia-infected IK and Hec-1B monolayers cultivated in the presence of DMSO or IWP2. Nonpolarized IK and Hec-1B cell monolayers were exposed to DMSO or IWP2 and infected with C. trachomatis. At 44 hpi the cultures were methanol fixed and subjected to immunofluorescent staining. Confocal microscopy images were captured at 630x magnification. (A) Representative images depicting fluorescently labeled β-catenin (green), chlamydial MOMP (red), and host cell nuclei (DAPI, blue). (B) Duplicates of the representative images in panel A are shown. Areas in which β-catenin and MOMP co-localized are indicated in white. (C) The average ratio of β-catenin staining intensity ±SEM measured in the center of an inclusion compared to an equal area of the host cell. *Indicate a significant difference (p ≤ 0.05) between the β-catenin staining intensity inclusion:cell ratio detected in DMSO verses IWP2-exposed samples. Black and white bars represent IK and Hec-1B samples respectively.
Inhibition of Wnt in Nonpolarized IK Cells by IWR-1 Decreases Infectivity and EB Progeny Production
To confirm that the observed effects of Wnt inhibition on chlamydial infection were not limited to IWP2 exposure, we examined the effect of another Wnt inhibitor, IWR-1, on chlamydial infection in endometrial epithelial cells. IWR-1 is a small molecule that inhibits canonical Wnt activity via stabilization of the β-catenin destruction complexes (Chen et al., 2009). Nonpolarized IK cell monolayers were exposed to DMSO or IWR-1 at 0 h post C. trachomatis infection. Samples were harvested for analysis of infectivity and EB production at 44 hpi. As shown in Figures 5A,B, IWR-1 exposure significantly decreased C. trachomatis percent infectivity (p = 0.01) and the production of infectious EB (p = 0.001) from the cultures. These data confirm the importance of Wnt/β-catenin signaling on chlamydial infection in endometrial cells.
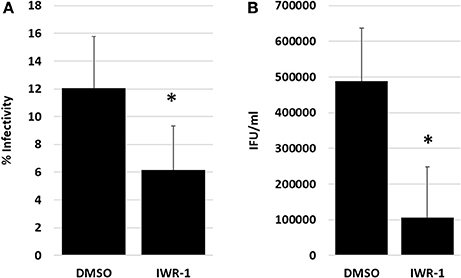
Figure 5. Impact of Wnt inhibition by IWR-1 on chlamydial infection in nonpolarized IK cells. Nonpolarized IK monolayers were exposed to DMSO or IWR-1 at 0 h post C. trachomatis infection. Samples were harvested for infectivity and EB titer assay at 44 hpi. (A) Chlamydial percent infectivity. (B) EB progeny production from infected IK. Comparison of means was performed 2-sample T-tests for independent samples. *Indicates a significant difference (p ≤ 0.05) from the DMSO control.
Discussion
Here, we have demonstrated that Wnt signaling is an essential pathway for chlamydial development in endometrial epithelial cells. Inhibition of Wnt significantly decreases chlamydial inclusion development, causing decreased percent infectivity and inclusion size. Furthermore, RB that formed during Wnt inhibition were aberrant in morphology. Inhibition of Wnt also caused a dramatic decrease in EB formation regardless of the time that Wnt was inhibited during the developmental cycle. Recovery of progeny formation following the removal of the Wnt inhibitor was only observed when the Wnt inhibitor was added late during chlamydial development. These data indicate that C. trachomatis may enter a persistent state or be killed when Wnt signals are inhibited in the host cell. Overall these data lead us to conclude that Wnt signaling is a key host pathway for the proper development of C. trachomatis in the endometrium. Our observations support previous work that indicate activation of Wnt signaling and/or β-catenin is important for chlamydial development in other areas of the FGT, including the fallopian tubes and cervical cells (Prozialeck et al., 2002; Kessler et al., 2012). Conversely, Kessler et al., found that IWP2 exposure increased chlamydial Hsp60 accumulation in infected fallopian tube tissue. The authors concluded that this result indicated increased chlamydial replication during Wnt inhibition (Kessler et al., 2012). However, continued or increased accumulation of Hsp60 has been observed in several models of chlamydial persistence or stress, including interferon-γ, viral co-infection, iron limitation, and monocyte infection (Beatty et al., 1993, 1994; Raulston, 1997; Gerard et al., 2004; Deka et al., 2006). While certainly not the only possibility, it is intriguing to speculate that IWP2 caused chlamydiae to enter a stressed state in fallopian tissues rather than increase chlamydial replication. Our observation that RB become aberrant during IWP2 exposure in endometrial cells supports this alternative conclusion. Together, data from the current study and previous studies highlight an important role for Wnt signaling in C. trachomatis infections throughout the entire tract.
Previous research has demonstrated that female sex hormones, estrogen and progesterone, can influence chlamydial development (Rank et al., 1982, 1993; Bose and Goswami, 1986; Sweet et al., 1986; Maslow et al., 1988; Wyrick et al., 1993; Guseva et al., 2003, 2007; Hall et al., 2011; Kintner et al., 2015). Using the IK/SHT-290 co-culture model of C. trachomatis infection, we have demonstrated that estrogen increases chlamydial development whereas progesterone negatively impacts C. trachomatis infection by unknown molecular mechanisms. These studies revealed the paracrine stromal cell signaling molecules released in response to hormones play a role in hormone-induced effects on chlamydial development (Hall et al., 2011; Kintner et al., 2015). Wnt signaling pathways are essential to the cyclic replenishment of the endometrium during the menstrual cycle. Following loss of the functional epithelial layer of the endometrium during menses, estrogen stimulates proliferation of epithelial cells in the basal endometrial layer. During the proliferative phase, estrogen stimulates the transcription of a variety of genes and activates cellular signaling pathways to induce cellular division and migration (Sonderegger et al., 2010; Wang et al., 2010; van der Horst et al., 2012; Tepekoy et al., 2015). Activation of both canonical and non-canonical Wnt signaling by estrogen is thought to play a central role in estrogen's proliferative and regulatory effects on endometrial epithelia (Das et al., 2000; Gunin et al., 2004; Hou et al., 2004; Wang et al., 2009; Sonderegger et al., 2010). Following the estrogen-dominant phase of the menstrual cycle, progesterone is produced during the secretory phase. Progesterone counterbalances the proliferative effects of estrogen on the endometrium to promote cellular differentiation in preparation for embryo implantation (Sonderegger et al., 2010). One mechanism employed by progesterone to inhibit the effects of estrogen on endometrial epithelial cell growth and proliferation is increasing gene expression of Wnt signaling inhibitors, dickkopf homolog 1 (DKK1), forkhead box O1 (FOXO1), and miR-152 (Wang et al., 2009; Nie et al., 2017). Thus, growth and differentiation of endometrial epithelium is directly linked to hormone-induced regulation of Wnt signaling networks.
Given that Wnt signaling is important to chlamydial development in the endometrium and that hormones naturally regulate Wnt signaling as a part of the menstrual cycle, it is intriguing to hypothesize that hormonal regulation of Wnt signaling may contribute to the impact of estrogen and progesterone on C. trachomatis infection. The demonstrated effects of Wnt/β-catenin signaling on chlamydial development could be attributed to hormonal modulation of Wnt signaling. For example, Kessler et al. proposed that Chlamydia-activated Wnt/β-catenin signaling in fallopian tube epithelia altered host cell adhesion and polarity (Kessler et al., 2012). Prozialeck et al. also demonstrated that sequestration of β-catenin to the inclusion altered host cell junctional complexes (Prozialeck et al., 2002). Estrogen signaling in the endometrium also contributes to endometrial epithelial cell junction remodeling during cellular proliferation and migration (Tulac et al., 2003; van der Horst et al., 2012). Future studies investigating the regulation of Wnt signaling pathways by steroid hormones in C. trachomatis-infected endometrial epithelial cells will further illuminate the importance of this intricate and complex system to chlamydial growth and pathogenesis.
Author Contributions
JK, CM, JW, MB, and JH contributed substantially to the design, execution, and/or data collection/analysis of the experiments within this study. JH drafted the manuscript. All authors contributed to revision and final approval of the manuscript.
Funding
This work was financially supported by an ETSU RDC Major Grant to JH, NIH/NIAID R15AI117632 to JH and NIH/NCRR: 1C06RR030651 to ETSU.
Conflict of Interest Statement
The authors declare that the research was conducted in the absence of any commercial or financial relationships that could be construed as a potential conflict of interest.
Acknowledgments
The authors are grateful for the excellent work by the Department of Biomedical Sciences Imaging Core Facility staff, particularly Rolf Fritz for sharing their technical expertise and to Dr. Robert Schoborg for his many helpful discussions about this work.
Supplementary Material
The Supplementary Material for this article can be found online at: https://www.frontiersin.org/articles/10.3389/fcimb.2017.00501/full#supplementary-material
Supplemental Figure 1. Images of representative β-catenin Western blots from IK/SHT-290 co-cultures.
Supplemental Figure 2. Example images of chlamydial inclusions at 44 hpi and 72 h R from DMSO or IWP2-exposed IK and Hec-1B cultures. Chlamydial inclusions (green) were stained with BioRad anti-MOMP Pathfinder stain. Cell nuclei (blue) were stained with DAPI. The images were captured at 400x magnification.
Supplemental Figure 3. (A) Images of representative β-catenin Western blots from chlamydia-infected, DMSO or IWP2-exposed, IK and Hec-1B cultures at 44 hpi. (B) Western blot analysis of β-catenin accumulation. β-catenin accumulation was normalized to total protein detected by Sypro Ruby staining as described in the methods. Comparison of means was performed using a 2-sample T-test for independent samples. There was no statistical difference in β-catenin accumulation between DMSO and IWP2-exposed, C. trachomatis-infected IK (p = 0.38) or Hec-1B (p = 0.92) cultures.
References
Arnold, J. T., Kaufman, D. G., Seppala, M., and Lessey, B. A. (2001). Endometrial stromal cells regulate epithelial cell growth in vitro: a new co-culture model. Hum. Reprod. 16, 836–845. doi: 10.1093/humrep/16.5.836
Arnold, J. T., Lessey, B. A., Seppala, M., and Kaufman, D. G. (2002). Effect of normal endometrial stroma on growth and differentiation in Ishikawa endometrial adenocarcinoma cells. Cancer Res. 62, 79–88.
Beatty, W. L., Byrne, G. I., and Morrison, R. P. (1993). Morphologic and antigenic characterization of interferon gamma-mediated persistent Chlamydia trachomatis infection in vitro. Proc. Natl. Acad. Sci. U.S.A. 90, 3998–4002. doi: 10.1073/pnas.90.9.3998
Beatty, W. L., Morrison, R. P., and Byrne, G. I. (1994). Persistent chlamydiae: from cell culture to a paradigm for chlamydial pathogenesis. Microbiol. Rev. 58, 686–699.
Bose, S. K., and Goswami, P. C. (1986). Enhancement of adherence and growth of Chlamydia trachomatis by estrogen treatment of HeLa cells. Infect. Immun. 53, 646–650.
CDC (2015). Sexually Transmitted Disease Surveillance 2014. Atlanta, GA: U.S. Department of Health and Human Services. Available online at: http://www.cdc.gov/std/stats14/toc.htm (Accessed Feb 3, 2016).
Chen, B., Dodge, M. E., Tang, W., Lu, J., Ma, Z., Fan, C. W., et al. (2009). Small molecule-mediated disruption of Wnt-dependent signaling in tissue regeneration and cancer. Nat. Chem. Biol. 5, 100–107. doi: 10.1038/nchembio.137
Das, S. K., Tan, J., Raja, S., Halder, J., Paria, B. C., and Dey, S. K. (2000). Estrogen targets genes involved in protein processing, calcium homeostasis, and Wnt signaling in the mouse uterus independent of estrogen Receptor-α and -β. J. Biol. Chem. 275, 28834–28842. doi: 10.1074/jbc.M003827200
Deka, S., Vanover, J., Dessus-Babus, S., Whittimore, J., Howett, M. K., Wyrick, P. B., et al. (2006). Chlamydia trachomatis enters a viable but non-cultivable (persistent) state within herpes simplex virus type 2 (HSV-2) co-infected host cells. Cell Microbiol. 8, 149–162. doi: 10.1111/j.1462-5822.2005.00608.x
Elwell, C., Mirrashidi, K., and Engel, J. (2016). Chlamydia cell biology and pathogenesis. Nat. Rev. Microbiol. 14, 385–400. doi: 10.1038/nrmicro.2016.30
Flores, R., and Zhong, G. (2015). The Chlamydia pneumoniae inclusion membrane protein Cpn1027 interacts with host cell Wnt signaling pathway regulator cytoplasmic activation/proliferation-associated protein 2 (Caprin2). PLoS ONE 10:e0127909. doi: 10.1371/journal.pone.0127909
Gerard, H. C., Whittum-Hudson, J. A., Schumacher, H. R., Hudson, A. P., Gérard, H. C., Whittum-Hudson, J. A., et al. (2004). Differential expression of three Chlamydia trachomatis hsp60-encoding genes in active vs. persistent infections. Microb Pathog 36, 35–39. doi: 10.1016/j.micpath.2003.08.005
Gunin, A. G., Emelianov, V. U., Mironkin, I. U., Morozov, M. P., and Tolmachev, A. S. (2004). Lithium treatment enhances estradiol-induced proliferation and hyperplasia formation in the uterus of mice. Eur. J. Obstet. Gynecol. Reprod. Biol. 114, 83–91. doi: 10.1016/j.ejogrb.2003.09.023
Guseva, N. V., Dessus-Babus, S., Moore, C. G., Whittimore, J. D., and Wyrick, P. B. (2007). Differences in Chlamydia trachomatis Serovar E growth rate in polarized endometrial and endocervical epithelial cells grown in three-dimensional culture. Infect. Immun. 75, 553–564. doi: 10.1128/IAI.01517-06
Guseva, N. V., Knight, S. T., Whittimore, J. D., and Wyrick, P. B. (2003). Primary cultures of female swine genital epithelial cells in vitro: a new approach for the study of hormonal modulation of Chlamydia infection. Infect. Immun. 71, 4700–4710. doi: 10.1128/IAI.71.8.4700-4710.2003
Hall, J. V., Schell, M., Dessus-Babus, S., Moore, C. G., Whittimore, J. D., Sal, M., et al. (2011). The multifaceted role of oestrogen in enhancing Chlamydia trachomatis infection in polarized human endometrial epithelial cells. Cell. Microbiol. 13, 1183–1199. doi: 10.1111/j.1462-5822.2011.01608.x
Hogan, R. J., Mathews, S. A., Mukhopadhyay, S., Summersgill, J. T., and Timms, P. (2004). Chlamydial persistence: beyond the biphasic paradigm. Infect. Immun. 72, 1843–1855. doi: 10.1128/IAI.72.4.1843-1855.2004
Hou, X., Tan, Y., Li, M., Dey, S. K., and Das, S. K. (2004). Canonical Wnt signaling is critical to estrogen-mediated uterine growth. Mol. Endocrinol. 18, 3035–3049. doi: 10.1210/me.2004-0259
Kessler, M., Zielecki, J., Thieck, O., Mollenkopf, H. J., Fotopoulou, C., and Meyer, T. F. (2012). Chlamydia trachomatis disturbs epithelial tissue homeostasis in fallopian tubes via paracrine Wnt signaling. Am. J. Pathol. 180, 186–198. doi: 10.1016/j.ajpath.2011.09.015
Kintner, J., Schoborg, R. V., Wyrick, P. B., and Hall, J. V. (2015). Progesterone antagonizes the positive influence of estrogen on C. trachomatis serovar E in an Ishikawa/ SHT-290 co-culture model. Pathog. Dis. 73:ftv015. doi: 10.1093/femspd/ftv015
Lee, S. C., Kim, O.-H., Lee, S. K., and Kim, S.-J. (2015). IWR-1 inhibits epithelial-mesenchymal transition of colorectal cancer cells through suppressing Wnt/β-catenin signaling as well as survivin expression. Oncotarget 6, 27146–27159. doi: 10.18632/oncotarget.4354
Maslow, A. S., Davis, C. H., Choong, J., and Wyrick, P. B. (1988). Estrogen enhances attachment of Chlamydia trachomatis to human endometrial epithelial cells in vitro. Am. J. Obstet. Gynecol. 159, 1006–1014. doi: 10.1016/S0002-9378(88)80189-3
Nie, L., Zhao, Y., Pan, J., Lei, Y., Liu, M., Long, Y., et al. (2017). Progesterone-Induced miR-152 inhibits the proliferation of endometrial epithelial cells by downregulating WNT-1. Reprod. Sci. 4, 1444–1453. doi: 10.1177/1933719116689595
Peipert, J. F. (2003). Clinical practice. Genital chlamydial infections. N. Engl. J. Med. 349, 2424–2430. doi: 10.1056/NEJMcp030542
Prozialeck, W. C., Fay, M. J., Lamar, P. C., Pearson, C. A., Sigar, I., and Ramsey, K. H. (2002). Chlamydia trachomatis disrupts N-cadherin-dependent cell-cell junctions and sequesters beta-catenin in human cervical epithelial cells. Infect. Immun. 70, 2605–2613. doi: 10.1128/IAI.70.5.2605-2613.2002
Rank, R. G., Sanders, M. M., and Kidd, A. T. (1993). Influence of the estrous cycle on the development of upper genital tract pathology as a result of chlamydial infection in the guinea pig model of pelvic inflammatory disease. Am. J. Pathol. 142, 1291–1296.
Rank, R. G., White, H. J., Hough, A. J. Jr., Pasley, J. N., and Barron, A. L. (1982). Effect of estradiol on chlamydial genital infection of female guinea pigs. Infect. Immun. 38, 699–705.
Raulston, J. E. (1997). Response of Chlamydia trachomatis serovar E to iron restriction in vitro and evidence for iron-regulated chlamydial proteins. Infect. Immun. 65, 4539–4547.
Sonderegger, S., Pollheimer, J., and Knöfler, M. (2010). Wnt signalling in implantation, decidualisation and placental differentiation–review. Placenta 31, 839–847. doi: 10.1016/j.placenta.2010.07.011
Sweet, R. L., Blankfort-Doyle, M., Robbie, M. O., and Schacter, J. (1986). The Occurrence of chlamydial and gonococcal salpingitis during the menstrual cycle. JAMA 255, 2062–2064. doi: 10.1001/jama.1986.03370150104037
Tepekoy, F., Akkoyunlu, G., and Demir, R. (2015). The role of Wnt signaling members in the uterus and embryo during pre-implantation and implantation. J. Assist. Reprod. Genet. 32, 337–346. doi: 10.1007/s10815-014-0409-7
Tulac, S., Nayak, N. R., Kao, L. C., Van Waes, M., Huang, J., Lobo, S., et al. (2003). Identification, characterization, and regulation of the canonical Wnt signaling pathway in human endometrium. J. Clin. Endocrinol. Metab. 88, 3860–3866. doi: 10.1210/jc.2003-030494
Tulac, S., Overgaard, M. T., Hamilton, A. E., Jumbe, N. L., Suchanek, E., and Giudice, L. C. (2006). Dickkopf-1, an inhibitor of Wnt signaling, is regulated by progesterone in human endometrial stromal cells. J. Clin. Endocrinol. Metab. 91, 1453–1461. doi: 10.1210/jc.2005-0769
van der Horst, P. H., Wang, Y., van der Zee, M., Burger, C. W., and Blok, L. J. (2012). Interaction between sex hormones and WNT/β-catenin signal transduction in endometrial physiology and disease. Mol. Cell. Endocrinol. 358, 176–184. doi: 10.1016/j.mce.2011.06.010
Vanover, J., Kintner, J., Whittimore, J., and Schoborg, R. V. (2010). Interaction of herpes simplex virus type 2 (HSV-2) glycoprotein D with the host cell surface is sufficient to induce Chlamydia trachomatis persistence. Microbiology 156, 1294–1302. doi: 10.1099/mic.0.036566-0
Wang, Y., Hanifi-Moghaddam, P., Hanekamp, E. E., Kloosterboer, H. J., Franken, P., Veldscholte, J., et al. (2009). Progesterone inhibition of Wnt/β-catenin signaling in normal endometrium and endometrial cancer. Clin. Cancer Res. 15, 5784–5793. doi: 10.1158/1078-0432.CCR-09-0814
Wang, Y., van der Zee, M., Fodde, R., and Blok, L. J. (2010). Wnt/β-catenin and sex hormone signaling in endometrial homeostasis and cancer. Oncotarget 1, 674–684. doi: 10.18632/oncotarget.201
Wyrick, P. B. (2000). Intracellular survival by Chlamydia. Cell. Microbiol. 2, 275–282. doi: 10.1046/j.1462-5822.2000.00059.x
Wyrick, P. B., Choong, J., Knight, S. T., Goyeau, D., Stuart, E. S., and MacDonald, A. B. (1994). Chlamydia trachomatis antigens on the surface of infected human endometrial epithelial cells. Immun. Inf. Dis. 4, 131–141.
Keywords: Chlamydia trachomatis, co-culture model, Wnt signaling, IWP2, hormones, endometrial epithelial cells, polarized cell culture
Citation: Kintner J, Moore CG, Whittimore JD, Butler M and Hall JV (2017) Inhibition of Wnt Signaling Pathways Impairs Chlamydia trachomatis Infection in Endometrial Epithelial Cells. Front. Cell. Infect. Microbiol. 7:501. doi: 10.3389/fcimb.2017.00501
Received: 07 August 2017; Accepted: 20 November 2017;
Published: 11 December 2017.
Edited by:
Kenneth Fields, University of Kentucky, United StatesReviewed by:
Elizabeth Ann Rucks, University of Nebraska Medical Center, United StatesEdward Shaw, Oklahoma State University, United States
Copyright © 2017 Kintner, Moore, Whittimore, Butler and Hall. This is an open-access article distributed under the terms of the Creative Commons Attribution License (CC BY). The use, distribution or reproduction in other forums is permitted, provided the original author(s) or licensor are credited and that the original publication in this journal is cited, in accordance with accepted academic practice. No use, distribution or reproduction is permitted which does not comply with these terms.
*Correspondence: Jennifer V. Hall, aGFsbGpsMUBldHN1LmVkdQ==