Trypanosoma cruzi-Infected Human Macrophages Shed Proinflammatory Extracellular Vesicles That Enhance Host-Cell Invasion via Toll-Like Receptor 2
- 1Departamento de Ciências Farmacêuticas, Universidade Federal de São Paulo (UNIFESP), São Paulo, Brazil
- 2Instituto René Rachou/FIOCRUZ – MG, Belo Horizonte, Brazil
- 3Border Biomedical Research Center, Department of Biological Sciences, University of Texas at El Paso (UTEP), El Paso, TX, United States
- 4Department of Biomolecular Sciences, Weizmann Institute of Science, Rehovot, Israel
Extracellular vesicles (EVs) shed by trypomastigote forms of Trypanosoma cruzi have the ability to interact with host tissues, increase invasion, and modulate the host innate response. In this study, EVs shed from T. cruzi or T.cruzi-infected macrophages were investigated as immunomodulatory agents during the initial steps of infection. Initially, by scanning electron microscopy and nanoparticle tracking analysis, we determined that T. cruzi-infected macrophages release higher numbers of EVs (50–300 nm) as compared to non-infected cells. Using Toll-like-receptor 2 (TLR2)-transfected CHO cells, we observed that pre-incubation of these host cells with parasite-derived EVs led to an increase in the percentage of infected cells. In addition, EVs from parasite or T.cruzi-infected macrophages or not were able to elicit translocation of NF-κB by interacting with TLR2, and as a consequence, to alter the EVs the gene expression of proinflammatory cytokines (TNF-α, IL-6, and IL-1β), and STAT-1 and STAT-3 signaling pathways. By proteomic analysis, we observed highly significant changes in the protein composition between non-infected and infected host cell-derived EVs. Thus, we observed the potential of EVs derived from T. cruzi during infection to maintain the inflammatory response in the host.
Introduction
Research interest in extracellular vesicles (EVs) and their involvement in cell-cell, cell-pathogen (parasites, viruses, bacteria, and fungi) or pathogen-pathogen communication and modulation processes in infectious and inflammatory diseases has continuously grown in recent years (Campos et al., 2010). The study of EVs has mainly focused on the types of membrane vesicles secreted into the extracellular compartment isolated from different cells, tissues, and biofluids in healthy and pathological conditions. EVs may be divided into exosomes, microvesicles, and apoptotic bodies (Théry et al., 2018; Mathieu et al., 2019; Witwer and Théry, 2019). These types of EVs have different origins, markers, and sizes (Théry et al., 2002; Tkach and Théry, 2016; van Niel et al., 2018; Mathieu et al., 2019; Witwer and Théry, 2019). EVs are heterogeneous membranous particles from 20 nm to 5 μm, differing in their biogenesis, molecular composition, biodistribution, and function (Campos et al., 2010; Lässer et al., 2011; Street et al., 2012; Madison et al., 2014). EVs are secreted by either prokaryotic or eukaryotic cells, thus extending their phenotype (Campos et al., 2010; Torrecilhas et al., 2012).
EVs from parasitic protozoa have been demonstrated as important virulence factors (Gonçalves et al., 1991; Trocoli Torrecilhas et al., 2009; Torrecilhas et al., 2012; Marcilla et al., 2014; Nogueira et al., 2015). They function as cell-to-cell effectors in the host-parasite interaction and manipulation of the host immune system (Aline et al., 2004; Bhatnagar et al., 2007; Pope and Lässer, 2013; Regev-Rudzki et al., 2013; Marcilla et al., 2014; Coakley et al., 2015; Ribeiro et al., 2018). EVs isolated from parasitic protozoa contain a wide variety of molecules, including proteins, glycoconjugates, lipids, RNAs, non-transcribed RNAs, and microRNAs (Torrecilhas et al., 2012). In infection-derived inflammatory processes, EVs can induce release of cytokines and nitric oxide. EVs may promote an inflammatory/immunosuppressive response in the host environment, thus affecting the subsequent immunopathological events, resulting in pathogen escape and invasion of the host cells (Silverman and Reiner, 2011; Nogueira et al., 2015). In infective host cell-derived T. cruzi trypomastigotes, α-galactosyl (α-Gal)-enriched EVs strongly trigger proinflammatory responses in murine macrophages via Toll-like receptor (TLR2)-dependent pathway, and the proteomic analysis of these EVs revealed the presence of several members of the TS/gp85 superfamily (Nogueira et al., 2015; Ribeiro et al., 2018). In Leishmania spp., it has been shown that promastigote-derived EVs contain virulence factors such as GP63 and lipophosphoglycan (LPG), and several molecules involved in the pathogenesis of Leishmania (Silverman et al., 2010; Atayde et al., 2015; Barbosa et al., 2018), suggesting that these EVs can contribute in the leishmaniasis infection and disease progression. Moreover, EVs isolated from Plasmodium-infected red blood cells (iRBC) transfer genetic material to the host cell and induce parasite gametocytogenesis, demonstrating a mechanism of interaction and communication with host cells as well as between parasites. In malaria, EVs can also mediate cellular communication processes, delivering virulence factors in the circulation and inflammatory infiltrate (Regev-Rudzki et al., 2013). It is known that inflammatory responses occur during T. cruzi infection and that this can be reproduced by the parasite EVs (Nogueira et al., 2015). Likewise, Dr Ramirez's group showed that metacyclic-trypomastigotes induce the release of TGF-β coated plasma membrane vesicles from blood macrophages and lymphocytes, which could further increase parasite infectivity (Cestari et al., 2012; Wyllie and Ramirez, 2017; Gavinho et al., 2018; Rossi et al., 2019). However, it is unknown whether EVs released by T. cruzi-infected macrophages or EVs isolated from parasite modulate hotst inflammatory responses and cell invasion.
The invasion of host cells is a key process in T. cruzi infection and it has been found that parasite surface molecules play an important role for parasite attachment and entry in the parasitophorous vacuole (Andrews, 2002). Cell-derived trypomastigotes induce lysosome mobilization, cytoskeleton rearrangements, membrane repair and elevation of Ca2+ and cAMP concentrations within the host cell (Tardieux et al., 1992, 1994; Burleigh and Andrews, 1998; Andrews, 2002). In fact, these processes are modulated by parasite EVs that were found to contain the major surface glycoconjugates of the parasite (Ribeiro et al., 2018).
Therefore, in this work, we evaluated whether EV shed by T. cruzi infected macrophage modulate inflammatory responses and if EVs from cell-derived trypomastigotes could also modify the cell invasion and the signaling mechanisms involved in this process.
Materials and Methods
Ethics Statement
All experimental procedures used in this work were approved by the Ethics Committee on Animal Use (CEUA) of the Federal University of São Paulo, protocol 1073090614.
Cell Lines and Parasite Infection
Tissue culture-derived trypomastigotes (TCT) of the T. cruzi Y strain were collected from the culture supernatants after 5 days after infection of LLCMK2 epithelial cells (monkey kidney epithelial cell line, ATCC© CCL-7™, VA, USA). Cells were maintained in low-glucose DMEM supplemented with 10% fetal bovine serum (FBS) (Invitrogen, CA, USA) at 37°C in a humidified 5% CO2 atmosphere. THP-1 (human peripheral blood monocyte cell line, ATCC© TIB-202™, VA, USA) cells were cultured in RPMI-1640 medium supplemented with 10% FBS and maintained at 37°C in a humidified 5% CO2 atmosphere. Cells were tested for Mycoplasma contamination by using the polymerase chain reaction (PCR) methodology (Uphoff and Drexler, 2002). The THP-1 monocytes were induced to differentiate into macrophages by the addition of phorbol myristate acetate (50 ng/mL) (Calbiochem, CA, USA) for 24 h in serum-free RPMI 1640. To determine the number of infected cells and the number of intracellular parasites, cells were cultured on circular glass coverslips and subsequently stained with Giemsa.
Isolation and Characterization of EVs
EVs from trypomastigotes (EV-TY) were obtained by incubation of parasites for 2 h in DMEM containing 2% glucose at 37°C and 5% CO2 and purified by size-exclusion chromatography (SEC), as previously described (Ribeiro et al., 2018). To obtain EVs from THP-1 infected and non-infected macrophages (respectively EV-THP-1inf and EV-THP1) were incubated with T. cruzi trypomastigotes at a 1:10 ratio (1 mL) for 4 h at 37°C/5% CO2 in RPMI 1640 containing 10% ultracentrifuged FBS to remove serum EVs. After infection, the THP-1 macrophages were washed three times with PBS, and then maintained in the same fresh medium for the production of EVs. Controls included THP-1 cells without T. cruzi infection. EVs were then recovered at the indicated times by differential ultracentrifugation of culture supernatants. Briefly, the supernatants were submitted to 10 min at 500 × g, followed by 10 min at 3,000 × g and 15 min at 8,000 × g to remove cells and cellular debris. EVs were then pelleted at 100,000 × g for 1 h using a T-890 fixed angle rotor (Thermo Fisher Scientific, MA, USA) as previously described in MISEV guideline (Théry et al., 2018).
The obtained EVs were characterized by NTA analysis to determine concentration and size using NanoSight NS300 (Malvern Instruments, Worcestershire, UK) equipped with a 405 nm laser and coupled to a CCD camera. Data were analyzed using NTA software (version 2.3). Each sample diluted (1:10) in PBS was analyzed in triplicate; and loaded into the instrument for 30 s at 20 frames per second with the camera level set to 14.
Scanning Electron Microscopy (SEM)
THP-1 cells infected or not with T. cruzi, and THP-1 cells treated with EV-TY were fixed in a 2.5% glutaraldehyde solution as reported elsewhere (Nogueira et al., 2015). The cells were post fixed with osmium tetroxide, treated with tannic acid, and dehydrated with ethanol. Samples were observed in a Field Emission FEI Quanta 250 FEG scanning electron microscope (FEI, OR, USA).
Immunoblotting
EVs samples containing 10 μg of protein, quantified by Micro BCA Assay kit (Thermo Fisher Scientific, MA, USA), were resolved by 12% SDS-PAGE and transferred to nitrocellulose membranes using standard procedures. The presence of exosome proteins and parasite surface proteins was evaluated by incubating membranes with primary antibodies against CD63, CD9, MHC II (Thermo Fisher Scientific, MA, USA) and the serum of a rabbit immunized with the extract of T.cruzi TCT membranes (Schenkman et al., 1991). Detection was achieved using horseradish peroxidase-conjugated anti-mouse or anti-rabbit (KPL Antibodies Seracare, MA, USA) secondary Abs and visualized with Pierce ECL Western Blotting Substrate (Thermo Fisher Scientific, MA, USA).
CHO Cell Lines
The CHO reporter cell lines (CHO/CD14, CHO/CD14/TLR-2, and CHO/CD14/TLR-4) were generated as described (Lien et al., 1999). These reporter cell lines contain a human CD25 gene reporter under the control of the E-selectin promoter, which contains an NF-κB-binding site (Delude et al., 1998; Lien et al., 1999 and Campos et al., 2001). All CHO reporter cell lines were grown in Ham's F-12 medium containing 10% FBS, 10 μg/ml ciprofloxacin, and 400 units/ml hygromycin B. Cells were plated at a concentration of 105/well in 24-well tissue-culture dishes. Cells were incubated with EV-THP-1, EV-THP-1 infected (EV-THP-1 inf) and EV-TY. Medium alone, 100 ng/ml LPS (from Escherichia coli serotype 055: B5; Sigma-Aldrich, MI, USA), and UV-killed Staphylococcus aureus at a ratio of S. aureus/cell of 500:1 (American Type Culture Collection 12.692), were used as controls. After 24 h of stimulation, cells were stained with PE-labeled anti-CD25 (mouse MAb to human CD25, PE conjugate; Caltag Laboratories, CA, USA). The cells were examined by flow cytometry (BD Biosciences, NJ, USA), and analyses were performed using Cell Quest software (BD Biosciences, NJ, USA) (Campos et al., 2001).
Infection Assays
TLR2- or TLR4-transfected CHO/CD14 cells were grown on 13 mm diameter glass coverslips in a 24-well dish and washed twice with DMEM/F12 medium (with antibiotics). Cells were incubated with culture supernatant or EV-TY (105 and 106particles/well) for 30 min, at 37°C, in RPMI-10% FBS. These cells were infected with TCTs (1:10, host cell: parasite ratio), for 1 h at 37°C, and then washed twice with RPMI without FBS. Non-adherent parasites were removed by addition of Lymphoprep (Axis-Shield, Norton, MA) to the cell layers, followed by two washes with PBS. The cells were incubated with RPMI-10% FBS for 18 h, at 37°C, or immediately fixed with methanol and stained with Hoechst fluorescent dye (Molecular Probes, Invitrogen Co., Carlsbad, CA). All experiments were performed in triplicate, and 24 photos of each replicate were made using a digital video-imaging fluorescent inverted microscope (Nikon), enabling the counting of infected and non-infected cells.
Gene Expression
To evaluate the gene expression, quantitative reverse transcriptase polymerase chain reaction (qRT-PCR) was used in THP-1 cells untreated as control, or THP-1 incubated with EV-THP-1 inf, EV-TY (108 particles/mL), or infected with trypomastigotes (TY). Total RNA was obtained from macrophages by using TRIzol reagent (Life Technologies, CA, USA). RNA samples were subjected to DNase treatment and cDNA synthesis with the RevertAid First Strand cDNA Synthesis kit (Thermo Fisher Scientific, MA, USA). RNA samples were quantified by UV absorption in a spectrophotometer (Nanodrop 2000c, Thermo Fisher) followed by electrophoresis in 1.5% agarose gels to assess RNA integrity. RNA samples with high quality and integrity were subjected to DNase treatment (RQ1 RNase-free DNase; Promega, Madison, WI, United States) and cDNA synthesis with the RevertAid First Strand cDNA Synthesis kit (Thermo Fisher Scientific, MA, USA). Specific primers for TLR2, TLR4, IL1β, IL6, TNF-α, STAT1, and STAT3 genes were used to analyze gene expression using RT-qPCR; all sequences were from PrimerBank (https://pga.mgh.harvard.edu/primerbank/) (Supplementary Table 1). The expression levels were normalized to those of the GAPDH and Actin-Beta (ACTB) reference genes using the 2−ΔΔCt cycle threshold method (Schmittgen and Livak, 2008). Differences in the relative expression levels of target genes were determined by com comparison between noninfected cells (as reference samples) and the cells infected or treated with EVs. The gene expression from the reference sample was adjusted to be equal to 1·00. All qRT-PCR procedures were developed following the MIQE guidelines (Bustin et al., 2009).
LC-MS/MS and Bioinformatic Analysis
EVs (~200 μg of protein) from uninfected and infected macrophages (EV-THP-1 and EV-THP-1inf) were concentrated in a Speedvac. For LC-MS/MS, the filter-aided sample preparation (FASP) method was used, following the manufacturer's protocol (Expedeon, San Diego, CA). The samples were reduced by adding 10 mM DTT for 30 min at room temperature (RT) and centrifuging in a spin filter at 14,000× g for 15 min at rt. After washing twice with 8 M urea in 50 mM Tris-HCl buffer, samples were washed again 2× with the urea/Tris-HCl buffer and 3× with 50 mM ammonium bicarbonate. Then, samples were digested with trypsin (Sigma-Aldrich, St. Louis, MO) was performed, as described by Ribeiro et al. (2018). Peptides were eluted from the filter using 0.1% formic acid and subjected to high-resolution LC-MS/MS analysis in a QE Plus Orbitrap (Thermo Fisher Scientific) equipped with a Nanospray Flex Ion Source (Thermo Fisher Scientific). Using a Dionex UltiMate 3000 RSLCnano UHPLC system (Thermo Fisher Scientific), peptides were separated with a PicoFrit column (75-μm ID × OD 360 μm, 25-cm length, New Objective, Woburn, MA) packed in-house with reversed-phase Aqua C18 porous silica (5 μm, 125 Å, Phenomenex). The column was equilibrated before sample injection at a flow rate of 0.5 μL/min with 95% solvent A (100% H2O, 0.1% formic acid) and 5% solvent B (90% acetonitrile, 0.1% formic acid). Samples were then injected onto the C18 column, and the same equilibration phase was run for 10 min. Elution of the peptides was performed using a linear gradient of solvent B up to 35% for 85 min, followed by a 5-min increase to 95%, where the plateau was maintained for 9 min. The column was then re-equilibrated with 5% solvent B for 10 min before injection of the next sample. An automated 2-h run was programmed into the Xcalibur software (Thermo Fisher Scientific), and each sample was analyzed in technical duplicates. Full scan spectra were collected from the 400–1,600 m/z range. Peptides were not excluded based on charge state, and 1 microscan for both full and MS/MS scans were acquired.
The spectra were searched using Proteome Discoverer (PD) 2.1.1.21 (Thermo Fisher Scientific) and filtered via Percolator with an estimated false-discovery rate (FDR) of 1 against sequences from T. cruzi, human, bovine, human keratin, and porcine trypsin. Parameters for the database search were as follows: 2 and 1 Da for peptide and fragment mass tolerance, respectively, cysteine carbamidomethylation and methionine oxidation as fixed and variable modifications, respectively. The dataset was further processed through Scaffold Q + 4.8.2 (Proteome Software, Portland, OR), merged, and the probability of their peptide assignments and protein identifications was assessed. Files were exported from Scaffold 4.8.2 for further analysis in Scaffold PerSPECtives (Proteome Software). Only peptides with ≥95% probability were accepted. The criteria for human protein identification included detection of at least two uniquely identified peptides and a probability score of ≥99%. The results were further filtered with the following peptide thresholds: Fisher's exact test (p-value) ≤0.001; fold change ≥50% and ≤50%. Gene ontology (GO) annotation was carried out using FunRich (version 3.1.3; http://www.funrich.org/) with the NCBI database (downloaded on 28 March 2019).
Statistics
All statistical tests were performed using GraphPad Prism version 6 (GraphPad Software, CA, USA). The Mann-Whitney U-test was used to determine differences between two groups. Analysis of variance (ANOVA) followed by Tukey's post-test was carried out to compare multiple groups. The differences were considered significant when p was < 0.05.
Results
Increased Numbers of EVs Are Released From Macrophages Infected With T. cruzi
Scanning electron microscopy (SEM) micrographs of THP-1-derived macrophages uninfected (EV-THP-1), infected (EV-THP-1 inf), or only treated with EVs from trypomastigotes (EV-TY) showed the presence of round-shaped vesicles with the expected size for exosomes (50–100 nm) and ectosomes (100–200 nm) on the surface of their plasma membrane (Figure 1). Compared to uninfected macrophages and those incubated with EV-TY, infected macrophages released a larger number of vesicles. EVs were isolated from the supernatants of THP-1 macrophages through a series of ultracentrifugation steps. The NTA showed a size distribution of 50–200 nm for all EVs (Supplementary Figure 1). The concentration of vesicles was also measured in the same experiment, revealing an increase in vesicle release by infected THP-1 macrophages (EV-THP-inf) (Figure 2A). In the immunoblotting analysis, the exosomal marker proteins CD63 and CD9 and the surface membrane protein MHC II were detected in EV-THP-1 and EV-THP-1 inf samples (Figure 2B). The presence of parasite surface proteins was only found in the EV-TY group (Figure 2B). The increase in EVs released from infected macrophages is related to presence of parasite from 24 to 72 h. (Figure 3A). However, the EVs release did not appear to increase when the number of parasites augment in infected cells (Figure 3B). After that, cells start to lyse and new trypomastigotes are released in the extracellular milieu.
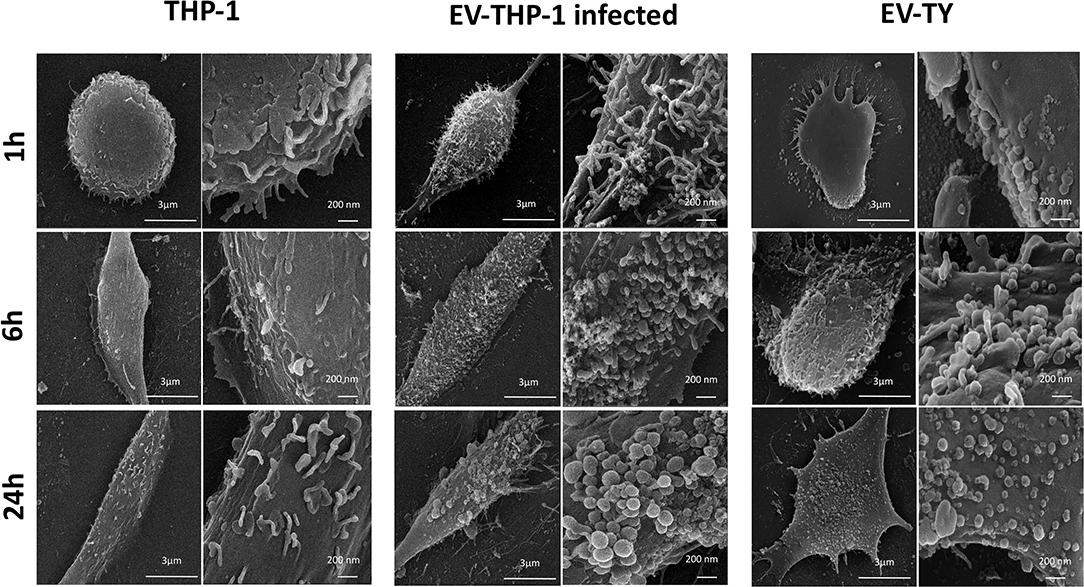
Figure 1. Scanning electron microscopy (SEM) micrographs of cultures of uninfected human macrophages (EV-THP-1), macrophages infected with Y-strain trypomastigotes (EV-THP-1 infected), and macrophages treated with EVs isolated from Y-strain trypomastigotes (EV-TY) for 1, 6, and 24 h. Cells were fixed with glutaraldehyde, and the slides were sent to the Electron Microscopy Center of UNIFESP. The protocol is described in the Materials and Methods section of Nogueira et al. (2015) (8). Images were obtained from macrophages grown on circular glass coverslips and infected with T. cruzi (MOI = 10:1) or not, as well as macrophages incubated with Y-strain trypomastigote EVs alone (107 particles/cell).
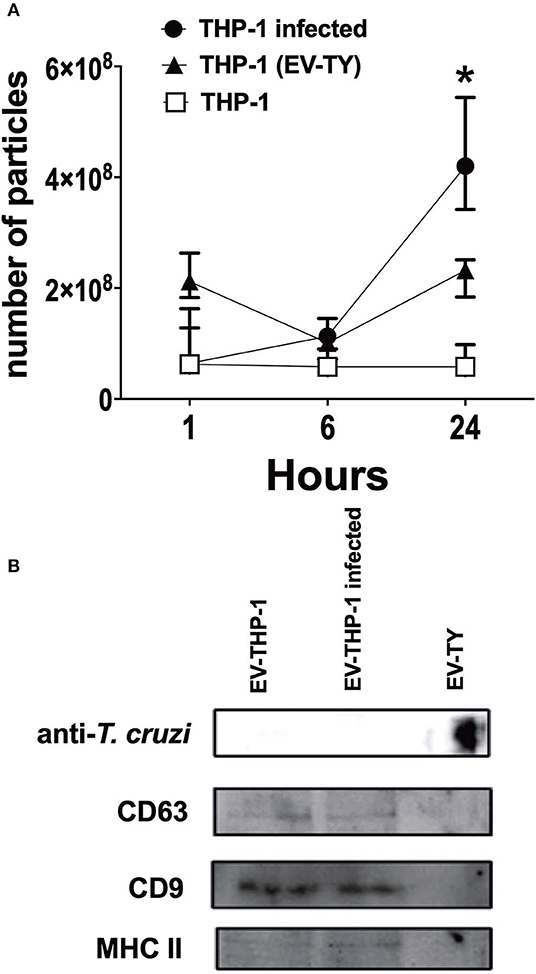
Figure 2. Characterization of EVs isolated from infected macrophages. Kinetics of released of EVs by human THP1 macrophages infected with TCT (EV-THP-1inf) or uninfected (EV-THP-1), or after the addition of T. cruzi EVs (EV-TY). Supernatants of macrophages were collected after 1, 6, and 24 h and then EVs isolated by differential ultracentrifugation as described in Methods. (A) Particle concentration as measured by NTA. (B) Immunoblotting EVs isolated from EV-THP-1inf, EV-THP-1 after 24 h, or EV-TY strain revealed with anti-CD63, anti-CD9, anti-MHC class II and anti-T. cruzi polyclonal antibody. Points represent the median of the triplicates, and the dispersion denotes the range. Data are representative of three independent experiments. *Indicates p < 0.05 to the respective time of EV-THP-1.
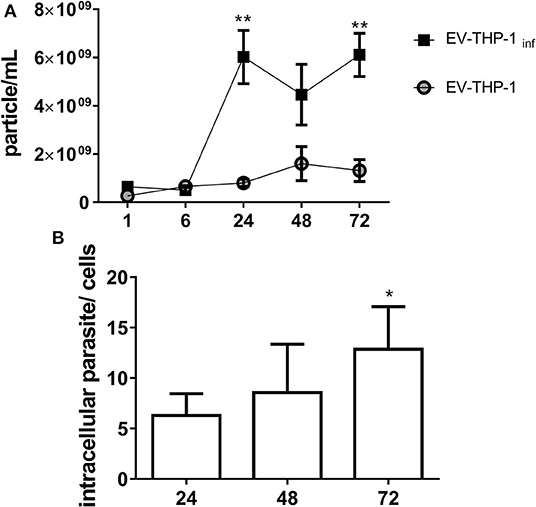
Figure 3. EVs released from THP-1 macrophages during T. cruzi infection. (A) NTA analysis showing particles/mL EVs released by cells from 1 to 72 h after infection by TCT as described in Methods. *p < 0.01, **p ≤ 0.001. (B) Number of intracellular parasites in macrophages at the indicated times as detected by Giemsa staining. The number of intracellular parasites was determined by microscopic examination in 20 fields and at least 200 cells were counted in triplicate samples. The number of intracellular parasites per each cell are shown in the bars with median with range of the triplicates.
The Interaction of EVs With TLR2 Is Critical for the Induction of NF-κB Activation and Increase the Susceptibility of Target Cells to T. cruzi Infection
Next, we evaluated the importance of the interaction of EVs from T. cruzi trypomastigotes with TLRs for the interaction and internalization of the parasite. The CHO reporter cell line contained an inducible NF-κB-dependent promoter driving the surface expression of CD25 (Campos et al., 2001). CHO cells were incubated with different concentrations of (EV-TY) and with EV-THP-1inf or EV-THP-1. The expression of CD25, which indicates activation of NF-κB, was measured by flow cytometry. These EVs did not increase the induction of CD25 expression by CHO/CD14 or CHO/CD14/TLR4 cells (Figure 4). Previous studies using wild-type, TLR2- or TLR4-knockout murine macrophages from C57BL/6 mice (WT, TLR2−/−, or TLR4−/− C57BL/6) showed that TLR2 but not in TLR4 was required for production of nitric oxide (NO) or TNF-α following stimulation with EV-TY and activate MAPKs (ERK 1/2, p38 and JNK) (Nogueira et al., 2015). The data presented in Figure 4 confirmed these previous results showing that the expression of CD25 through the activation of NF-κB was induced in CHO/CD14/TLR2, but not CHO/CD14/TLR4, exposed to EV-TY. Importantly, EV-THP-1 and EV-THP-1inf were also found to quantitatively promote the expression of CD25 in CHO/CD14/TLR2 cells, but not TLR4 (Figure 4), indicating that both EVs derived from T. cruzi (EV-TY) and macrophages interact with TLR2, but not TLR4, activating NF-κB. This result also suggested that induction by macrophage EVs was not due to the presence of parasite molecules, as when we used the same number of particles of non-infected macrophages, the induction was similar. The presence of tGPI-mucins and glycoinositolphospholipids (GIPLs) in the EV-TY was likely responsible for the induction of potent inflammatory responses in macrophages (Almeida et al., 2000; Trocoli Torrecilhas et al., 2009).
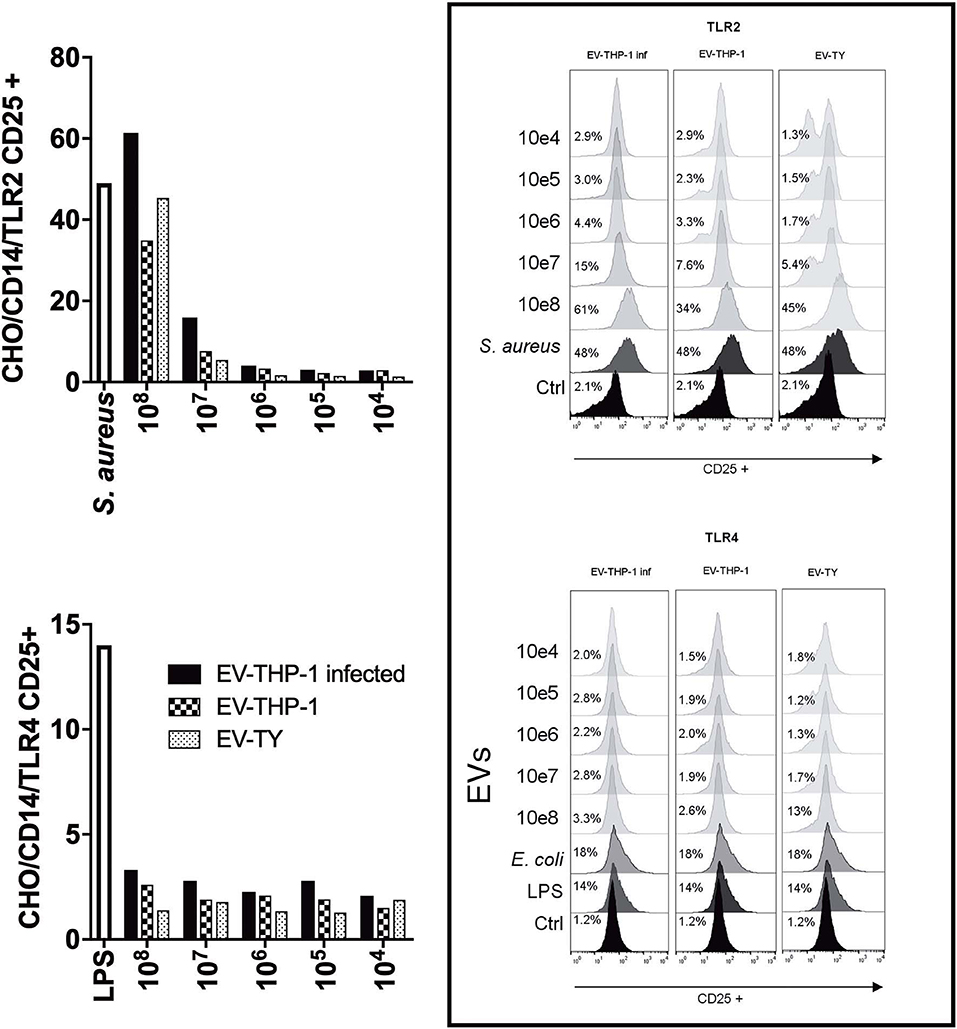
Figure 4. NF-κB translocation assay using CHO reporter cells transfected with TLR2 or TLR4. Flow cytometry of CD25 expression in CHO cells expressing TLR2 or TLR4 and stimulated with EVs derived from infected or non-infected macrophages or with EVs released by the parasite. Fluorescence was analyzed 24 h after stimulation with EVs on a flow cytometer (FACS) (FACScan BD). Bars represent the percentages of positive cells. Data are representative of two independent experiments.
The participation of TLR2 in the host-cell infection by T. cruzi was then investigated. To test this hypothesis, infection experiments were performed in CHO/CD14 cells, transfected or not with TLR2 or TLR4. Upon prior exposure to culture supernatants of trypomastigotes or EVs, cells transfected with TLR2, but not TLR4- or non-transfected cells were increase the susceptibility of target cells to T. cruzi infection. A remarkable (up to 6–7-fold) increase in percentage of infected cells was observed in TLR2-transfected CHO/CD14 cells exposed either to total shedding or EV-TY (Figure 5).
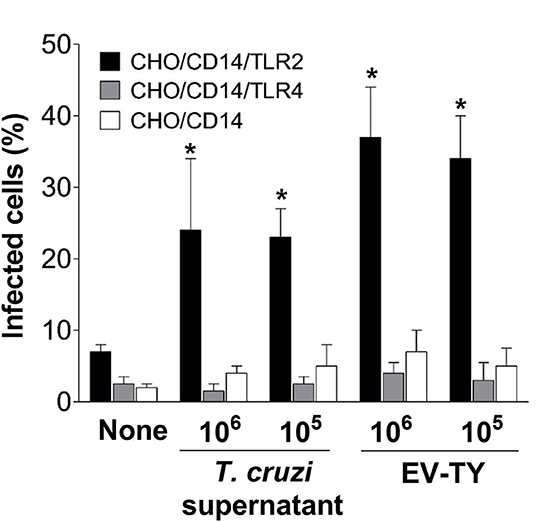
Figure 5. Invasion of TLR2- and TLR4-transfected CHO/CD14 cells with trypomastigotes in the presence or absence of trypomastigotes culture supernatant and EVs isolated from the T. cruzi Y strain. Macrophages were preincubated with EVs for 30 min at 37°C in RPMI medium without FCS. Cells were washed with PBS (3×) and Lymphoprep (1×) and then infected with trypomastigotes at a 1:10 ratio (host cell:parasite) for 2 h at 37°C. Cells were then washed (3×) with PBS to remove non-adherent parasites and incubated in RPMI medium with 10% FCS for 24 h at 37°C. Cells were fixed with 100% methanol. Then, the cells were stained with 4′,6-diamidino-2-phenylindole (DAPI). The number of infected cells was estimated using an inverted Nikon fluorescence microscope. *p < 0.01.
EVs Induce the Differential Expression of TLR2, TLR4, IL-1β, IL-6, TNF-α and STAT1/STAT3
TLRs are involved in the recognition of T. cruzi following the activation of host cells to produce TNF-α, IL-12, and NO (Campos et al., 2004; Koga et al., 2006). Macrophages showed differences in TLR gene expression upon stimulation with EVs and T. cruzi infection. We analyzed the gene expression of TLR2, TLR4 by RT-qPCR in macrophages untreated and treated with EV-TY, EV-THP-1control, and EV-THP-1 inf. The gene expression analysis in macrophages treated with EV-THP-1inf and EV-TY revealed alterations in TLR2 and TLR4 (Figure 6).
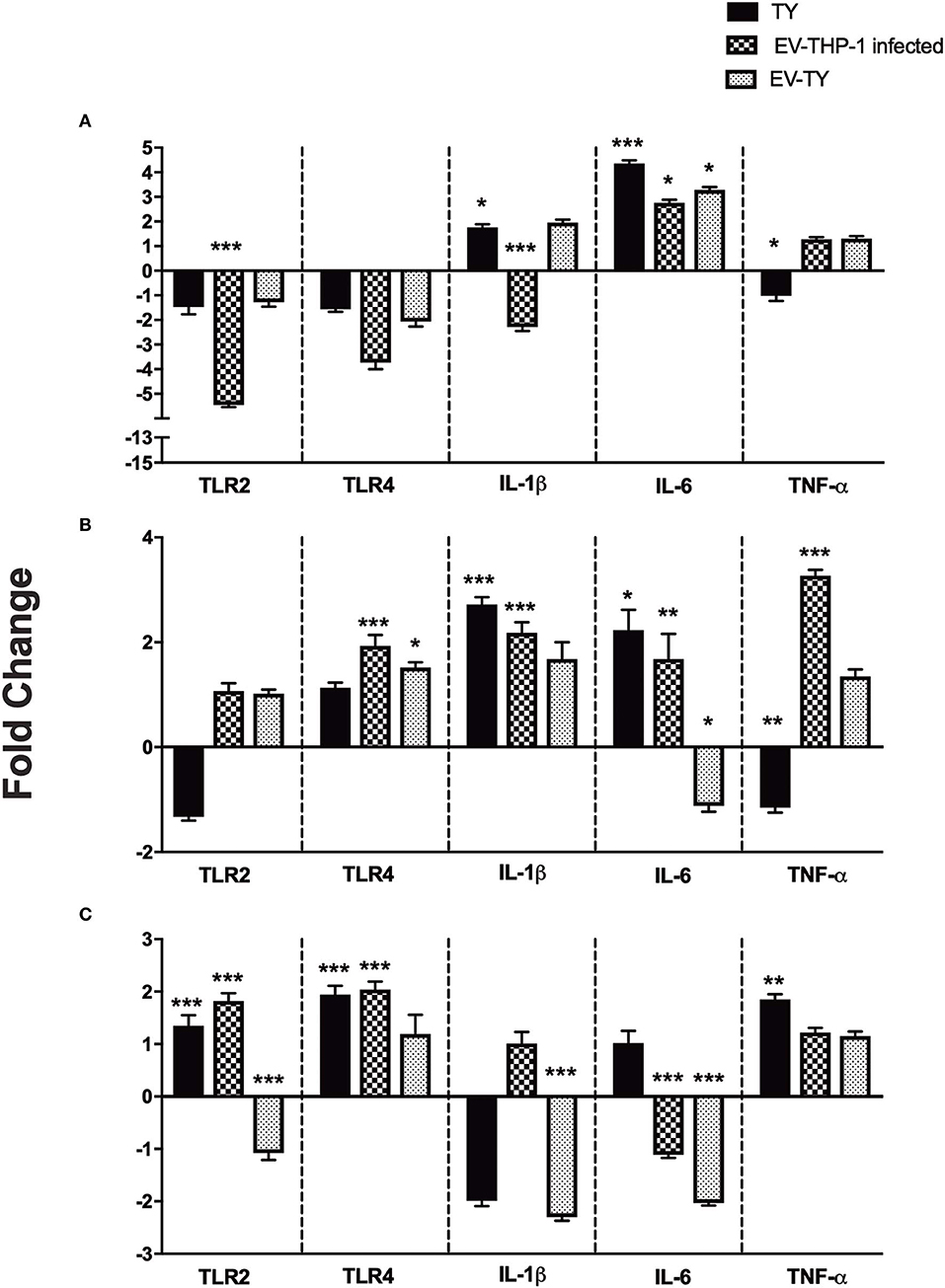
Figure 6. Gene expression levels of TLR2, TLR4, IL-1β, IL-6, and TNF-α proinflammatory cytokines in differentiated THP-1 cells. Relative mRNA expression of TLRs and cytokines were measured after 1 (A), 6 (B), and 24 h (C) of macrophages infected with 5 × 107/mL trypomastigotes (TY), or macrophages treated with EV-THP1 inf, EV-TY (both EVs at 108 particles/mL). After these treatments, RNA was extracted, and the expression of TLR2, TLR4 and specific cytokines was determined by RT-qPCR. Bars represent the mean of the triplicates, and the dispersion denotes the standard deviation subtracted to THP-1 macrophages maintained in medium. Data are representative of two independent experiments. Statistical analysis with ANOVA, compared to the respective control; *p < 0.05; **p < 0.01; and ***p < 0.001.
TLR2 expression is essential for proinflammatory cytokine production (Campos and Gazzinelli, 2004). RT-qPCR analysis was performed to assess the changes in gene expression of cytokines and transcription factors. Macrophages, therefore, were incubated with EVs (EV-THP-1 control, EV-THP-1 inf and EV-TY) and also infected with T. cruzi. Evaluation of the cytokine gene expression profile revealed an increase in IL-1β levels after 6 h in both T. cruzi-infected cells and in those exposed to EV-THP-1inf (Figure 6). There was also an increase in the transcript levels of IL-1β after 1 h of T. cruzi infection. After 24 h of T. cruzi infection or stimulation with EV-THP-1 inf, no change in IL-1β transcript levels was observed, whereas a marked reduction was seen in cells that were treated with EV-TY or EV-THP-1. After 1 and 6 h of T. cruzi infection, the cells showed elevated transcript levels of IL-6. The same result was observed after 1 and 6 h of treatment with EV-TY or EV-THP-1 inf. However, 24 h after the other treatments, there was a reduction in IL-6 gene expression, except for T. cruzi infection. In the TNF-α gene expression analysis, elevation of the transcript level was observed after 1 and 6 h of treatment with EV-THP-1inf and after 6 h of treatment with EV-THP-1. Only T. cruzi infection induced the gene expression of TNF-α at 24 h.
Signal transduction through cytokine receptor-binding occurs via the JAK/STAT pathway signaling. We observed the gene expression of STAT1 and STAT3 in the macrophages stimulated with the different EVs. STAT1 and STAT3 gene expression increased only in response to T. cruzi infection (Figure 7). Stimulation with the different EVs reduced the gene expression of STAT3 at 1 and 6 h (Figure 7).
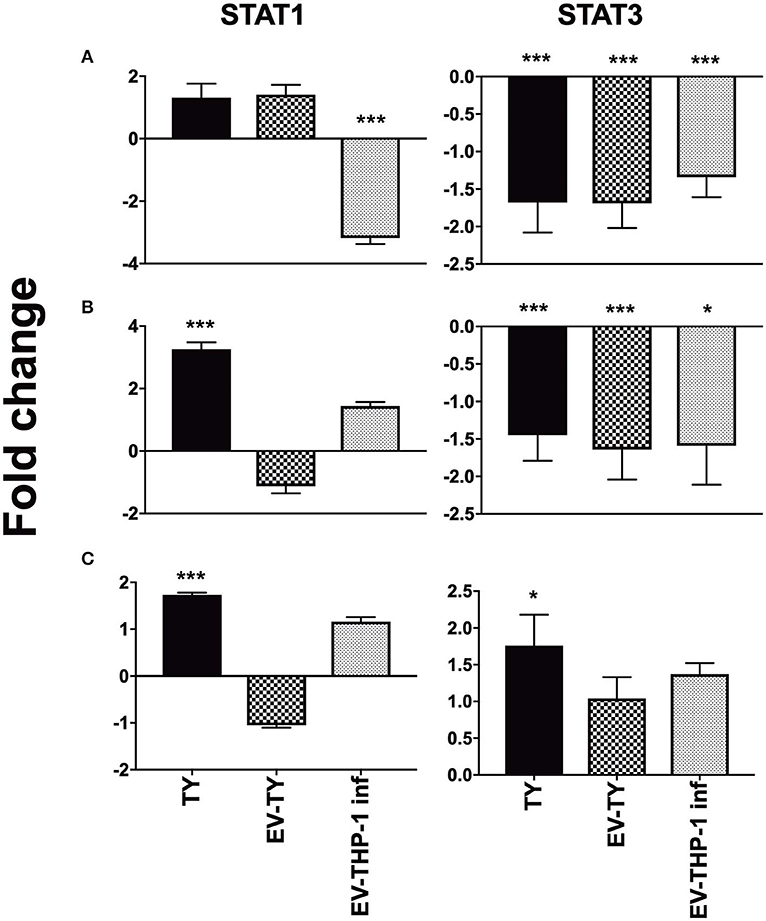
Figure 7. Gene expression of STAT1 and STAT3 in differentiated THP-1 cells. Relative mRNA expression levels of STAT1 and STAT3 transcription factors in macrophages after 1 (A), 6 (B), and 24 h (C) of macrophages infected with 5 × 107/mL trypomastigotes (TY), or macrophages treated with EV-THP1 inf, EV-TY (both EVs at 108 particles/mL). After these treatments, RNA was extracted, and the expression of TLR2, TLR4 and specific cytokines was determined by RT-qPCR. Bars represent the mean of the triplicates, and the dispersion denotes the standard deviation subtracted to THP-1 macrophages maintained in medium. Data are representative of two independent experiments. Statistical analysis with ANOVA, compared to the respective control; *p < 0.05; **p < 0.01; ***p < 0.001.
EVs Released by Infected Macrophages Have Distinct Contents and Parasite Proteins
We performed mass spectrometry-based proteomic analysis (LC-MS/MS) to determine the content of the EVs purified from uninfected (EV-THP-1) and infected macrophages (EV-THP-1 inf). A total of 123 proteins were found in THP-1-EVs and 89 were found in EV-THP-1 inf, from a total of 154 proteins in both samples (Figure 8A). Using FunRich (version 3.1.3), we compared the list of identified proteins to previously published EV data in the Exocarta database (www.exocarta.org) and found that the majority of the proteins had been previously observed to be present in EVs from different sources (Figure 8B). T. cruzi-infected host-cell derived EVs carry proteins from the parasite, as previously described (Ramirez et al., 2017). We also looked for T. cruzi proteins in EV-THP-1 inf. We found 6 proteins (i.e., HSP60, tryparedoxin peroxidase, trans-sialidase Group II, flagellar calcium-binding protein, surface protein TolT and paraflagellar rod protein 2) (Supplementary Table 1). We also compared the levels of abundance of the 58 common proteins by calculating their fold change. The abundance of 20 proteins increased and 38 decreased after infection with T. cruzi (Supplementary Table 2). Most of the proteins found in EV-THP-1 and EV-THP-1 inf are involved in binding, immunological and metabolic processes based on GO term annotations (Figure 8C). Moreover, the majority of the proteins in EV-THP-1 and EV-THP-1 inf were localized in exosomes (Figure 8D). Noteworthy, there was a higher percentage of proteins with catalytic activity in EV-THP-1 inf, particularly enzymes such as trans-sialidase (Figure 8E). It is possible that some of these proteins from T. cruzi were not directly associated with the EVs obtained from infected macrophages and were released in the culture supernatant of the infected cells concomitantly with EV-THP-1 inf production. Furthermore, although unlike, the presence of remaining proteins from the parasites used to infected cells cannot be fully excluded.
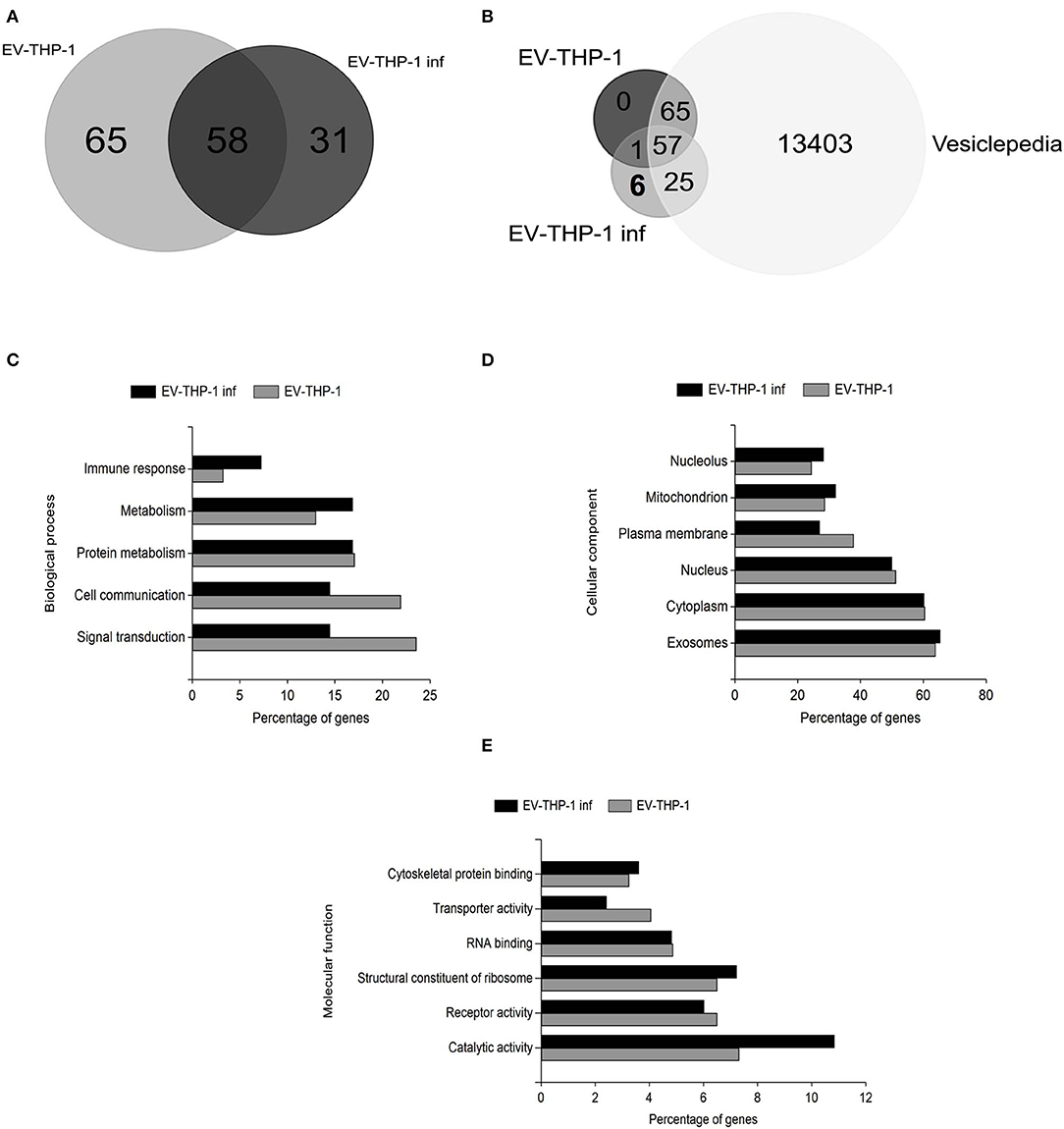
Figure 8. Proteomic and gene ontology analysis of EVs derived from infected or non-infected macrophages. Common and unique proteins identified by LC-MS/MS in EVs from infected or non-infected macrophages (A). Comparison of identified proteins by LC-MS/MS in EVs from infected or non-infected macrophages, T. cruzi and Exocarta database proteins (Vesiclepedia) (B). GO graphs of the proteins identified in EV-THP-1 and EV-THP-1inf indicating changes in biological process and molecular functions (C–E).
Discussion
Macrophages and other mononuclear cells are the host's first line of defense to combat infection of intracellular parasites, such as T. cruzi. The release of EVs by T. cruzi promotes the activation of murine macrophages (Nogueira et al., 2015). Here, we demonstrate that EVs derived from macrophages infected with T. cruzi also modulate the activation of other human THP-1 macrophages to maintain the inflammatory response in the course of infection.
The recognition and response to pathogenic organisms by the immune system is essential for infection control. The expression of TLRs is critical for the response during the early stages of infection for the activation of the immune response. A large number of pathogens express ligands for different TLRs. T. cruzi trypomastigote-derived mucin-like glycoproteins (tGPI-mucins) are strong proinflammatory molecules and agonists for the TLR2-TLR6 heterodimer (Campos et al., 2001). T. cruzi also express other glycoconjugates such as GIPLs, which activate TLR4 (Almeida et al., 2000; Oliveira et al., 2004).
In recent years, there has been a growing number of studies showing the capacity of EVs to modulate the immune response, especially in the relationship between parasite and host (Campos et al., 2010; Marcilla et al., 2014). Different strains of T. cruzi have been shown to release EVs and promote the activation of macrophages via TLR2 (Nogueira et al., 2015). EVs isolated from macrophages infected with intracellular pathogens (Mycobacterium bovis BCG and M. tuberculosis) induce a TLR/MyD88-dependent proinflammatory response in naive macrophages (Bhatnagar et al., 2007). In this work, we observed that EVs derived from T. cruzi-infected THP-1 human macrophages were able to induce the translocation of NF-κB to the nucleus by interacting with TLR2. EVs released by uninfected THP-1 cells also activated the cells via TLR2. However, the amount of EVs produced by the infected cells was 10-fold higher than that produced by the uninfected cells, showing that the amount of EVs is the prime factor for activation. In addition, uninfected macrophages produce EVs when there is tissue damage, which leads to the activation of these cells or dendritic cells and results in the secretion of TNF-α and other inflammatory mediators through the activation of the p38 MAPK pathway and NF-κB (Thomas and Salter, 2010). Damage-associated molecular pattern molecules (DAMPs) could also be related to the mechanism of action of the EVs described herein. Among the DAMPs, we found heat shock proteins, hyaluronic acid, β-defensin 3 and HMGB1, which are recognized by TLR2 (Scheibner et al., 2006; Funderburg et al., 2007; Curtin et al., 2009).
Internalization and phagocytosis by phagocytic cells such as macrophages are crucial processes for initiation of the killing of intracellular pathogens. T. cruzi can invade different mammalian cell types (Yoshida, 2006). It has been demonstrated that metacyclic trypomastigotes can invade cells in a Ca2+-dependent manner by exocytosis and the recruitment of host lysosomes to the plasma membrane (Tardieux et al., 1994; Rodríguez et al., 1995). Another mechanism for T. cruzi entry in cells independent of lysosome recruitment has been described to occur through the activation of PI3K (Woolsey et al., 2003). Here we observed that macrophages infected with T. cruzi, as evaluated by SEM, showed an increase in the number of EVs budding from the plasma membrane after the 24-h culture period. This increase was also confirmed by NTA. The release of EVs can be regulated by changes in intracellular calcium levels. When cells were treated with calcium ionophore (A23187), an increase in the release of EVs was found (Savina et al., 2003). T. cruzi infection increases the Ca2+ concentration in macrophages (Wilkowsky et al., 1996). Additionally, activated cells release a greater number of particles than non-activated cells (Théry et al., 2009). Thus, the increased release of EVs by infected macrophages is related to an increase in intracellular calcium after T. cruzi infection and macrophage activation. The size range of the EVs isolated herein (50–200 nm) corroborates that described in the literature (Raposo and Stoorvogel, 2013; Wang et al., 2015; Hui et al., 2018). EVs can further enhance the parasite's ability to invade the host cells through TLR2 signaling. We observed an increase in the number of infected cells when they expressed TLR2 and were incubated with parasite-derived EVs (EV-TY). EVs from T. cruzi participate in invasion through a mechanism independent of lysosome recruitment. The parasite invasion is associated with activation of PI3K (Wilkowsky et al., 2001). TLR2 activates PI3K during T. cruzi infection, leading to Rab5 activation, which is essential for phagosome formation (Maganto-Garcia et al., 2008). The release of EVs by infected macrophages is related to increased parasite replication, which was observed in macrophages infected with T. cruzi herein.
Similarly, in mice infected with Mycobacterium bovis, an increase in the release of plasma EVs is also related to an increase in the bacterial load (Singh et al., 2012).
We performed proteomic analysis to compare the content of the EVs released from uninfected THP-1 cells and THP-1 cells infected with T. cruzi. According to the Exocarta database (www.exocarta.org), all the proteins found in the EVs have previously been identified in different types of cells and body fluids. The infection changed the composition of the EVs from the host cells; 65 proteins were unique to the EV-THP-1 cells, 31 were unique to the EV-THP-1 inf, and the expression of 58 proteins was altered in both EVs (20 proteins increase and 38 decrease).
The EVs isolated from macrophage supernatants showed the presence of the MHC II surface marker and the exosomal markers CD9 and CD63. No T. cruzi antigens could be detected by western blotting in EV-THP-1 and the presence of plasma membrane glycoproteins expressing α-Gal epitopes (i.e., TcMUCII mucins or tGPI-mucins) of the parasite in the EVs of macrophages infected by T. cruzi were not identified by total proteomic analysis. In this analysis only six parasite proteins were found in EVs from macrophages infected with T. cruzi i.e., HSP60, tryparedoxin peroxidase, trans-sialidase Group II, flagellar calcium-binding protein, surface protein TolT and paraflagellar rod protein 2 (Lobo et al., 2019). These parasite proteins are involved in the recognition of the parasite by host cells, invasion, the adhesion of host cells, metabolism and the induction of the host immune response (Godsel et al., 1995; Quanquin et al., 1999; Mattos et al., 2014; Urményi et al., 2014; Girard et al., 2018).
Previous studies of EVs derived from macrophages infected with intracellular microorganisms, such as mycobacteria and Leishmania, also identified proteins of these intracellular organisms that can be carried by EVs (Giri et al., 2010; Hassani and Olivier, 2013).
A variation in cytokine expression in macrophages stimulated with EVs was observed by RT-qPCR. An increase in IL-1β expression was evidenced in cells stimulated with TY and EV-THP-1inf at 6 and 24 h. IL-1β is related to the resistance of the host to infection through stimulation of the IL-1 receptor and activation of the adapter protein MyD88, leading to the production of NO in macrophages (Lima-Junior et al., 2013). Thus, increased IL-1β production may be important in infection control. In addition, immune activation through TLR2 and NF-κB receptors by macrophages has been found to rapidly elevate IL-1β levels (Petersen et al., 2005). Macrophages stimulated with EV-THP-1 and EV-THP-1 inf as well as those infected with T. cruzi displayed increased TNF-α expression. TNF-α is a proinflammatory cytokine produced by macrophages and lymphocytes and is important for the control of T. cruzi infection. The production of TNF-α by macrophages when stimulated with INF-γ is associated with resistance to infection in mice (Silva et al., 1995). Increased IL-6 production was seen only at 6 h in response to treatment with the EVs of infected macrophages and parasites. Interleukin-6 is a proinflammatory cytokine produced primarily by T cells, dendritic cells and macrophages. It has been reported that IL-6 is not required for a strong Th1 response (Moskowitz et al., 1997) and that in the presence of TGF-β, IL-1β, and IL-23 cause the differentiation of Th0 lymphocytes into Th17 lymphocytes (Acosta-Rodriguez et al., 2007; Zhou et al., 2007).
The cytokine expression profile observed after stimulation with EVs released by infected cells demonstrates the modulation of the proinflammatory conditions caused by the release of these EVs. This cytokine expression profile also occurs in response to EVs from Leishmania amazonensis-infected macrophages, which promote the production of the inflammatory cytokines IL-12, IL-1β, and TNF-α (Cronemberger-Andrade et al., 2014). The production of TNF-α, IL-1β and IL-6 by macrophages is associated with the M1 type phenotype. M1 macrophages are responsible for polarizing the Th1 immune response, producing IL-12 and maintaining a low production level of IL-10. The main stimuli that favor the activation and polarization in M1 macrophages are LPS and IFN-γ (Mantovani et al., 2004). It has recently been demonstrated that plasma-isolated EVs from chronic Chagas disease patients promote increased regulation of genes related to the proinflammatory response in macrophages (Chowdhury et al., 2017). In addition, the composition of the EVs isolated from the plasma of mice and patients revealed them to be of cardiac, lymphocyte, and macrophage origin (Chowdhury et al., 2017) thus demonstrating the importance of the potential modulation and activation of macrophages in the chronic phase of Chagas disease.
Signal transduction through IFN-γ receptors is mediated by the phosphorylation of JAK and STAT1. The activation of STAT1 is important for the production of a proinflammatory response. In STAT1 knockout mice, an increase in mortality was found due to increased numbers of parasites found in the blood and tissues (Kulkarni et al., 2015). We did not observe regulation by this mechanism after stimulation with the different EVs. STAT3 phosphorylation induces transcription of the gene encoding SOCS-3 (He et al., 2003). In macrophages infected with M. tuberculosis, NF-κB activation was found to be inhibited, and SOCS-3 expression was found to be induced as a mechanism of inflammatory response evasion (Nair et al., 2011; Hillmer et al., 2016). The production of IL-10 leads to increased expression of SOCS-3 and STAT3 in T. cruzi-infected cardiomyocytes due to the inactivation of NF-κB and ERK/MAPK (Hovsepian et al., 2013). In the analysis of STAT3 expression, we observed a reduction in expression at 1 and 6 h and an increase only at 24 h after T. cruzi infection. The EVs released by infected or non-infected macrophages, as well as the parasite-derived EVs, modulated the response of macrophages favoring the maintenance of a proinflammatory response. It was previously shown that T. cruzi GIPLs were not able to activate STAT1 or STAT3 (Stahl et al., 2013). However, in that study the authors did not evaluate tGPI-mucins, which have much stronger proinflammatory than GIPLs (Almeida et al., 2000; Campos et al., 2001). Therefore, we conclude that tGPI-mucins (or TcMUCII mucins), which are present in trypomastigote-derived EVs (Ribeiro et al., 2018), and/or other molecules expressed on the surface of the parasite and the EVs (EV-TY) there from -TY may be involved in the activation of these transcription factors.
In summary, our results indicate that signaling through EVs during T. cruzi infection is essential in host-parasite interactions. After infection, increased amounts of EVs are released from infected macrophages that interact with TLR2 and stimulate the translocation of NF-κB. As a result of this interaction and activation, proinflammatory cytokines (TNF-α, IL-6, and IL-1β) are produced and maintain the inflammatory response generated by T. cruzi infection. In addition, human macrophage-derived EVs carry parasite proteins, which could be the reason for their increased ability to induce inflammatory responses.
Data Availability Statement
All datasets generated for this study are included in the article/Supplementary Material.
Ethics Statement
All experimental procedures used in this work were approved by the Ethics Committee on Animal Use (CEUA) of the Federal University of São Paulo, protocol 1073090614.
Author Contributions
AC-A, PX, IA, YO-B, NR-R, and AT conceived and designed the experiments. AC-A, PX, YO-B, and AT performed most experiments. NP and MC assisted in NF-κB translocation assay. IA, CE, and BG performed the proteomic analysis. AC-A, PX, YO-B, and AT wrote the manuscript. AC-A, PX, RS, YO-B, IA, NR-R, and AT contributed to final manuscript. All the authors reviewed the manuscript.
Funding
This work was supported by the FAPESP (2016-01917-3) and doctoral fellowship from CNPq and CAPES. IA was partially supported by the grant No. 2G12MD007592 from the National Institute of General Medical Sciences (NIGMS). We are grateful to the Biomolecule Analysis Core Facility (BACF) at UTEP/BBRC, funded by NIGMS grant No. 2G12MD007592, for the access to the LC-MS instrument.
Conflict of Interest
The authors declare that the research was conducted in the absence of any commercial or financial relationships that could be construed as a potential conflict of interest.
Acknowledgments
We thank all colleagues from Laboratório de Imunologia Celular & Bioquímica de fungos e protozoários (LICBfp), Departamento de Ciências Farmacêuticas, UNIFESP who provided helpful technical advice and expertise that greatly assisted the research.
Supplementary Material
The Supplementary Material for this article can be found online at: https://www.frontiersin.org/articles/10.3389/fcimb.2020.00099/full#supplementary-material
Supplementary Figure 1. Size distribution of EVs from macrophages. Kinetics of released EVs isolated from THP-1 cells (differentiated to macrophages) infected or uninfected with T. cruzi and or treated with T. cruzi EVs. Supernatants from infected or not macrophages and from macrophages incubated with T. cruzi EVs were collected after 1, 6, and 24 h and then isolated by ultracentrifugation.
Supplementary Table 1. Proteins identified by LC-MS/MS in EVs derived from uninfected (THP-1) and infected (THP-1 infected) macrophages.
Supplementary Table 2. Table containing sequences of the primers selected for the gene expression analysis of human macrophages stimulated with EVs derived from infected or non-infected macrophages and infected with T. cruzi strain Y trypomastigotes.
References
Acosta-Rodriguez, E. V., Napolitani, G., Lanzavecchia, A., and Sallusto, F. (2007). Interleukins 1β and 6 but not transforming growth factor-β are essential for the differentiation of interleukin 17-producing human T helper cells. Nat. Immunol. 8, 942–949. doi: 10.1038/ni1496
Aline, F., Bout, D., Amigorena, S., Roingeard, P., and Dimier-Poisson, I. (2004). Toxoplasma gondii antigen-pulsed-dendritic cell-derived exosomes induce a protective immune response against T. gondii infection. Infect. Immun. 72, 4127–4137. doi: 10.1128/IAI.72.7.4127-4137.2004
Almeida, I. C., Camargo, M. M., Procópio, D. O., Silva, L. S., Mehlert, A., Travassos, L. R., et al. (2000). Highly purified glycosylphosphatidylinositols from Trypanosoma cruzi are potent proinflammatory agents. EMBO J. 19, 1476–1485. doi: 10.1093/emboj/19.7.1476
Andrews, N. W. (2002). Lysosomes and the plasma membrane. J. Cell Biol. 158, 389–394. doi: 10.1083/jcb.200205110
Atayde, V. D., Aslan, H., Townsend, S., Hassani, K., Kamhawi, S., and Olivier, M. (2015). Exosome secretion by the parasitic protozoan Leishmania within the sand fly midgut. Cell Rep. 13, 957–967. doi: 10.1016/j.celrep.2015.09.058
Barbosa, F. M. C., Dupin, T. V., Toledo, M. D. S., Reis, N. F. D. C., Ribeiro, K., Cronemberger-Andrade, A., et al. (2018). Extracellular vesicles released by Leishmania (Leishmania) amazonensis promote disease progression and induce the production of different cytokines in macrophages and B-1 cells. Front. Microbiol. 9:3056. doi: 10.3389/fmicb.2018.03056
Bhatnagar, S., Shinagawa, K., Castellino, F. J., and Schorey, J. S. (2007). Exosomes released from macrophages infected with intracellular pathogens stimulate a proinflammatory response in vitro and in vivo. Blood 110, 3234–3244. doi: 10.1182/blood-2007-03-079152
Burleigh, B. A., and Andrews, N. W. (1998). Signaling and host cell invasion by Trypanosoma cruzi. Curr. Opin. Microbiol. 1, 461–465. doi: 10.1016/S1369-5274(98)80066-0
Bustin, S. A., Benes, V., Garson, J. A., Hellemans, J., Huggett, J., Kubista, M., et al. (2009). The MIQE guidelines: minimum information for publication of quantitative Real-Time PCR experiments. Clin. Chem. 55, 611–622. doi: 10.1373/clinchem.2008.112797
Campos, F. M. F., Franklin, B. S., Teixeira-Carvalho, A., Filho, A. L. S., de Paula, S. C. O., Fontes, C. J., et al. (2010). Augmented plasma microparticles during acute Plasmodium vivax infection. Malar. J. 9:327. doi: 10.1186/1475-2875-9-327
Campos, M. A., Almeida, I. C., Takeuchi, O., Akira, S., Valente, E. P., Procópio, D. O., et al. (2001). Activation of Toll-like receptor-2 by glycosylphosphatidylinositol anchors from a protozoan parasite. J. Immunol. 167, 416–423. doi: 10.4049/jimmunol.167.1.416
Campos, M. A., Closel, M., Valente, E. P., Cardoso, J. E., Akira, S., Alvarez-Leite, J. I., et al. (2004). Impaired production of proinflammatory cytokines and host resistance to acute infection with Trypanosoma cruzi in mice lacking functional myeloid differentiation factor 88. J. Immunol. 172, 1711–1718. doi: 10.4049/jimmunol.172.3.1711
Campos, M. A., and Gazzinelli, R. T. (2004). Trypanosoma cruzi and its components as exogenous mediators of inflammation recognized through Toll-like receptors. Mediators Inflamm. 13, 139–143. doi: 10.1080/09511920410001713565
Cestari, I., Ansa-Addo, E., Deolindo, P., Inal, J. M., and Ramirez, M. I. (2012). Trypanosoma cruzi immune evasion mediated by host cell-derived microvesicles. J. Immunol. 4, 1942–1952. doi: 10.4049/jimmunol.1102053
Chowdhury, I. H., Koo, S.-J., Gupta, S., Liang, L. Y., Bahar, B., Silla, L., et al. (2017). Gene expression profiling and functional characterization of macrophages in response to circulatory microparticles produced during Trypanosoma cruzi infection and chagas disease. J. Innate Immun. 9, 203–216. doi: 10.1159/000451055
Coakley, G., Maizels, R. M., and Buck, A. H. (2015). Exosomes and other extracellular vesicles: the new communicators in parasite infections. Trends Parasitol. 31, 477–489. doi: 10.1016/j.pt.2015.06.009
Cronemberger-Andrade, A., Aragão-França, L., de Araujo, C. F., Rocha, V. J., da Cruz Borges-Silva, M., Pereira Figueira, C., et al. (2014). Extracellular vesicles from Leishmania-infected macrophages confer an anti-infection cytokine-production profile to naïve macrophages. PLoS Negl. Trop. Dis. 8:e3161. doi: 10.1371/journal.pntd.0003161
Curtin, J. F., Liu, N., Candolfi, M., Xiong, W., Assi, H., Yagiz, K., et al. (2009). HMGB1 mediates endogenous TLR2 activation and brain tumor regression. PLoS Med. 6:e10. doi: 10.1371/journal.pmed.1000010
Delude, R. L., Yoshimura, A., Ingalls, R. R., and Golenbock, D. T. (1998). Construction of a lipopolysaccharide reporter cell line and its use in identifying mutants defective in endotoxin, but not TNF-α, signal transduction. J. Immunol. 161, 3001–3009.
Funderburg, N., Lederman, M. M., Feng, Z., Drage, M. G., Jadlowsky, J., Harding, C. V., et al. (2007). Human -defensin-3 activates professional antigen-presenting cells via Toll-like receptors 1 and 2. Proc. Natl. Acad. Sci. U.S.A. 104, 18631–18635. doi: 10.1073/pnas.0702130104
Gavinho, B., Rossi, I. V., Evans-Osses, I., Inal, J., and Ramirez, M. I. (2018). A new landscape of host-protozoa interactions involving the extracellular vesicles world. Parasitology 12, 1521–1530. doi: 10.1017/S0031182018001105
Girard, M. C., Acevedo, G. R., López, L., Ossowski, M. S., Piñeyro, M. D., Grosso, J. P., et al. (2018). Evaluation of the immune response against Trypanosoma cruzi cytosolic tryparedoxin peroxidase in human natural infection. Immunology 155, 367–378. doi: 10.1111/imm.12979
Giri, P. K., Kruh, N. A., Dobos, K. M., and Schorey, J. S. (2010). Proteomic analysis identifies highly antigenic proteins in exosomes from M. tuberculosis-infected and culture filtrate protein-treated macrophages. Proteomics 10, 3190–3202. doi: 10.1002/pmic.200900840
Godsel, L. M., Tibbetts, R. S., Olson, C. L., Chaudoir, B. M., and Engman, D. M. (1995). Utility of recombinant flagellar calcium-binding protein for serodiagnosis of Trypanosoma cruzi infection. J. Clin. Microbiol. 33, 2082–2085.
Gonçalves, M. F., Umezawa, E. S., Katzin, A. M., de Souza, W., Alves, M. J., Zingales, B., et al. (1991). Trypanosoma cruzi: shedding of surface antigens as membrane vesicles. Exp. Parasitol. 72, 43–53. doi: 10.1016/0014-4894(91)90119-H
Hassani, K., and Olivier, M. (2013). Immunomodulatory impact of leishmania-induced macrophage exosomes: a comparative proteomic and functional analysis. PLoS Negl. Trop. Dis. 7:e2185. doi: 10.1371/journal.pntd.0002185
He, B., You, L., Uematsu, K., Matsangou, M., Xu, Z., He, M., et al. (2003). Cloning and characterization of a functional promoter of the human SOCS-3 gene. Biochem. Biophys. Res. Commun. 301, 386–391. doi: 10.1016/S0006-291X(02)03071-1
Hillmer, E. J., Zhang, H., Li, H. S., and Watowich, S. S. (2016). STAT3 signaling in immunity. Cytokine Growth Factor Rev. 31, 1–15. doi: 10.1016/j.cytogfr.2016.05.001
Hovsepian, E., Penas, F., Siffo, S., Mirkin, G. A., and Goren, N. B. (2013). IL-10 inhibits the NF-κB and ERK/MAPK-mediated production of pro-inflammatory mediators by up-regulation of SOCS-3 in Trypanosoma cruzi-infected cardiomyocytes. PLoS ONE 8:e79445. doi: 10.1371/journal.pone.0079445
Hui, W. W., Hercik, K., Belsare, S., Alugubelly, N., Clapp, B., Rinaldi, C., et al. (2018). Salmonella enterica Serovar Typhimurium alters the extracellular proteome of macrophages and leads to the production of proinflammatory exosomes. Infect. Immun. 86:e00386–e00317 doi: 10.1128/IAI.00386-17
Koga, R., Hamano, S., Kuwata, H., Atarashi, K., Ogawa, M., Hisaeda, H., et al. (2006). TLR-dependent induction of IFN- β mediates host defense against Trypanosoma cruzi. J. Immunol. 177, 7059–7066. doi: 10.4049/jimmunol.177.10.7059
Kulkarni, M. M., Varikuti, S., Terrazas, C., Kimble, J. L., Satoskar, A. R., and McGwire, B. S. (2015). Signal transducer and activator of transcription 1 (STAT-1) plays a critical role in control of Trypanosoma cruzi infection. Immunology 145, 225–231. doi: 10.1111/imm.12438
Lässer, C., Alikhani, V. S., Ekström, K., Eldh, M., Paredes, P. T., Bossios, A., et al. (2011). Human saliva, plasma and breast milk exosomes contain RNA: uptake by macrophages. J. Transl. Med. 9:9. doi: 10.1186/1479-5876-9-9
Lien, E., Sellati, T. J., Yoshimura, A., Flo, T. H., Rawadi, G., Finberg, R. W., et al. (1999). Toll-like receptor 2 functions as a pattern recognition receptor for diverse bacterial products. J. Biol. Chem. 274, 33419–33425. doi: 10.1074/jbc.274.47.33419
Lima-Junior, D. S., Costa, D. L., Carregaro, V., Cunha, L. D., Silva, A. L. N., Mineo, T. W. P., et al. (2013). Inflammasome-derived IL-1β production induces nitric oxide-mediated resistance to Leishmania. Nat. Med. 19, 909–915. doi: 10.1038/nm.3221
Lobo, M., Balouz, V., Melli, L., Carlevaro, G., Cortina, M. E., Cámara, M., et al. (2019). Molecular and antigenic characterization of Trypanosoma cruzi TolT proteins. PLoS Negl. Trop. Dis. 13:e0007245. doi: 10.1371/journal.pntd.0007245
Madison, M. N., Roller, R. J., and Okeoma, C. M. (2014). Human semen contains exosomes with potent anti-HIV-1 activity. Retrovirology 11:102. doi: 10.1186/s12977-014-0102-z
Maganto-Garcia, E., Punzon, C., Terhorst, C., and Fresno, M. (2008). Rab5 activation by Toll-like receptor 2 is required for Trypanosoma cruzi internalization and replication in macrophages. Traffic 9, 1299–1315. doi: 10.1111/j.1600-0854.2008.00760.x
Mantovani, A., Sica, A., Sozzani, S., Allavena, P., Vecchi, A., and Locati, M. (2004). The chemokine system in diverse forms of macrophage activation and polarization. Trends Immunol. 25, 677–686. doi: 10.1016/j.it.2004.09.015
Marcilla, A., Martin-Jaular, L., Trelis, M., de Menezes-Neto, A., Osuna, A., Bernal, D., et al. (2014). Extracellular vesicles in parasitic diseases. J. Extracell. Vesicles 3:25040. doi: 10.3402/jev.v3.25040
Mathieu, M., Martin-Jaular, L., Lavieu, G., and Théry, C. (2019). Specificities of secretion and uptake of exosomes and other extracellular vesicles for cell-to-cell communication. Nat. Cell Biol. 1, 9–17. doi: 10.1038/s41556-018-0250-9
Mattos, E. C., Tonelli, R. R., Colli, W., and Alves, M. J. M. (2014). The Gp85 surface glycoproteins from Trypanosoma cruzi. Subcell. Biochem. 74, 151–180. doi: 10.1007/978-94-007-7305-9_7
Moskowitz, N. H., Brown, D. R., and Reiner, S. L. (1997). Efficient immunity against Leishmania major in the absence of interleukin-6. Infect. Immun. 65, 2448–2450. doi: 10.1128/IAI.65.6.2448-2450.1997
Nair, S., Pandey, A. D., and Mukhopadhyay, S. (2011). The PPE18 protein of mycobacterium tuberculosis Inhibits NF- B/rel-Mediated proinflammatory cytokine production by upregulating and phosphorylating suppressor of cytokine signaling 3 protein. J. Immunol. 186, 5413–5424. doi: 10.4049/jimmunol.1000773
Nogueira, P. M., Ribeiro, K., Silveira, A. C. O., Campos, J. H., Martins-Filho, O. A., Bela, S. R., et al. (2015). Vesicles from different Trypanosoma cruzi strains trigger differential innate and chronic immune responses. J. Extracell. Vesicles 4:28734. doi: 10.3402/jev.v4.28734
Oliveira, A.-C., Peixoto, J. R., de Arruda, L. B., Campos, M. A., Gazzinelli, R. T., Golenbock, D. T., et al. (2004). Expression of functional TLR4 confers proinflammatory responsiveness to Trypanosoma cruzi glycoinositolphospholipids and higher resistance to infection with T. cruzi. J. Immunol. 173, 5688–5696. doi: 10.4049/jimmunol.173.9.5688
Petersen, C. A., Krumholz, K. A., and Burleigh, B. A. (2005). Toll-like receptor 2 regulates interleukin-1β -dependent cardiomyocyte hypertrophy triggered by Trypanosoma cruzi. Infect. Immun. 73, 6974–6980. doi: 10.1128/IAI.73.10.6974-6980.2005
Pope, S. M., and Lässer, C. (2013). Toxoplasma gondii infection of fibroblasts causes the production of exosome-like vesicles containing a unique array of mRNA and miRNA transcripts compared to serum starvation. J. Extracell. Vesicles 2:22484. doi: 10.3402/jev.v2i0.22484
Quanquin, N. M., Galaviz, C., Fouts, D. L., Wrightsman, R. A., and Manning, J. E. (1999). Immunization of mice with a TolA-like surface protein of Trypanosoma cruzi generates CD4(+) T-cell-dependent parasiticidal activity. Infect. Immun. 67, 4603–4612. doi: 10.1128/IAI.67.9.4603-4612.1999
Ramirez, M. I., Deolindo, P., de Messias-Reason, I. J., Arigi, E. A., Choi, H., Almeida, I. C., et al. (2017). Dynamic flux of microvesicles modulate parasite-host cell interaction of Trypanosoma cruzi in eukaryotic cells. Cell. Microbiol. 19:e12672. doi: 10.1111/cmi.12672
Raposo, G., and Stoorvogel, W. (2013). Extracellular vesicles: exosomes, microvesicles, and friends. J. Cell Biol. 200, 373–383. doi: 10.1083/jcb.201211138
Regev-Rudzki, N., Wilson, D. W., Carvalho, T. G., Sisquella, X., Coleman, B. M., Rug, M., et al. (2013). Cell-cell communication between malaria-infected red blood cells via exosome-like vesicles. Cell 153, 1120–1133. doi: 10.1016/j.cell.2013.04.029
Ribeiro, K. S., Vasconcellos, C. I., Soares, R. P., Mendes, M. T., Ellis, C. C., Aguilera-Flores, M., et al. (2018). Proteomic analysis reveals different composition of extracellular vesicles released by two Trypanosoma cruzi strains associated with their distinct interaction with host cells. J. Extracell. Vesicles 7:1463779. doi: 10.1080/20013078.2018.1463779
Rodríguez, A., Rioult, M. G., Ora, A., and Andrews, N. W. (1995). A trypanosome-soluble factor induces IP3 formation, intracellular Ca2+ mobilization and microfilament rearrangement in host cells. J. Cell Biol. 129, 1263–1273. doi: 10.1083/jcb.129.5.1263
Rossi, I. V., Gavinho, B., and Ramirez, M. I. (2019). Isolation and characterization of extracellular vesicles derived from Trypanosoma cruzi. Methods Mol. Biol. 89–104. doi: 10.1007/978-1-4939-9148-8_7
Savina, A., Furlán, M., Vidal, M., and Colombo, M. I. (2003). Exosome release is regulated by a Calcium-dependent mechanism in K562 Cells. J. Biol. Chem. 278, 20083–20090. doi: 10.1074/jbc.M301642200
Scheibner, K. A., Lutz, M. A., Boodoo, S., Fenton, M. J., Powell, J. D., and Horton, M. R. (2006). Hyaluronan fragments act as an endogenous danger signal by engaging TLR2. J. Immunol. 177, 1272–1281. doi: 10.4049/jimmunol.177.2.1272
Schenkman, S., Pontes de Carvalho, L., and Nussenzweig, V. (1991). Trypanosoma cruzi trans-sialidase and neuraminidase activities can be mediated by the same enzymes. J. Exp. Med. 2, 567–575. doi: 10.1084/jem.175.2.567
Schmittgen, T. D., and Livak, K. J. (2008). Analyzing real-time PCR data by the comparative CT method. Nat. Protoc. 3, 1101–1108. doi: 10.1038/nprot.2008.73
Silva, J. S., Vespa, G. N., Cardoso, M. A., Aliberti, J. C., and Cunha, F. Q. (1995). TNF-α mediates resistance to Trypanosoma cruzi infection in mice by inducing nitric oxide production in infected gamma interferon-activated macrophages. Infect. Immun. 63, 4862–4867. doi: 10.1128/IAI.63.12.4862-4867.1995
Silverman, J. M., Clos, J., De'Oliveira, C. C., Shirvani, O., Fang, Y., Wang, C., et al. (2010). An exosome-based secretion pathway is responsible for protein export from Leishmania and communication with macrophages. J. Cell Sci. 123, 842–852. doi: 10.1242/jcs.056465
Silverman, J. M., and Reiner, N. E. (2011). Exosomes and other microvesicles in infection biology: organelles with unanticipated phenotypes. Cell. Microbiol. 13, 1–9. doi: 10.1111/j.1462-5822.2010.01537.x
Singh, P. P., Smith, V. L., Karakousis, P. C., and Schorey, J. S. (2012). Exosomes isolated from mycobacteria-infected mice or cultured macrophages can recruit and activate immune cells in vitro and in vivo. J. Immunol. 189, 777–785. doi: 10.4049/jimmunol.1103638
Stahl, P., Ruppert, V., Meyer, T., Schmidt, J., Campos, M. A., Gazzinelli, R. T., et al. (2013). Trypomastigotes and amastigotes of Trypanosoma cruzi induce apoptosis and STAT3 activation in cardiomyocytes in vitro. Apoptosis 18, 653–663. doi: 10.1007/s10495-013-0822-x
Street, J. M., Barran, P. E., Mackay, C. L., Weidt, S., Balmforth, C., Walsh, T. S., et al. (2012). Identification and proteomic profiling of exosomes in human cerebrospinal fluid. J. Transl. Med. 10:5. doi: 10.1186/1479-5876-10-5
Tardieux, I., Nathanson, M. H., and Andrews, N. W. (1994). Role in host cell invasion of Trypanosoma cruzi-induced cytosolic-free Ca2+ transients. J. Exp. Med. 179, 1017–1022. doi: 10.1084/jem.179.3.1017
Tardieux, I., Webster, P., Ravesloot, J., Boron, W., Lunn, J. A., Heuser, J. E., et al. (1992). Lysosome recruitment and fusion are early events required for trypanosome invasion of mammalian cells. Cell 71, 1117–1130. doi: 10.1016/S0092-8674(05)80061-3
Théry, C., Ostrowski, M., and Segura, E. (2009). Membrane vesicles as conveyors of immune responses. Nat. Rev. Immunol. 9, 581–593. doi: 10.1038/nri2567
Théry, C., Witwer, K. W., Aikawa, E., Alcaraz, M. J., Anderson, J. D., Andriantsitohaina, R., et al. (2018). Minimal information for studies of extracellular vesicles 2018 (MISEV2018): a position statement of the international society for extracellular vesicles and update of the MISEV2014 guidelines. J. Extracell. Vesicles 7:1535750. doi: 10.1080/20013078.2018.1535750
Théry, C., Zitvogel, L., and Amigorena, S. (2002). Exosomes: composition, biogenesis and function. Nat. Rev. Immunol. 2, 569–579. doi: 10.1038/nri855
Thomas, L. M., and Salter, R. D. (2010). Activation of macrophages by P2X7-induced microvesicles from myeloid cells is mediated by phospholipids and is partially dependent on TLR4. J. Immunol. 185, 3740–3749. doi: 10.4049/jimmunol.1001231
Tkach, M., and Théry, C. (2016). Communication by extracellular vesicles: where we are and where we need to go. Cell. 6, 1226–1232. doi: 10.1016/j.cell.2016.01.043
Torrecilhas, A. C., Schumacher, R. I., Alves, M. J. M., and Colli, W. (2012). Vesicles as carriers of virulence factors in parasitic protozoan diseases. Microbes Infect. 14, 1465–1474. doi: 10.1016/j.micinf.2012.07.008
Trocoli Torrecilhas, A. C., Tonelli, R. R., Pavanelli, W. R., da Silva, J. S., Schumacher, R. I., de Souza, W., et al. (2009). Trypanosoma cruzi: parasite shed vesicles increase heart parasitism and generate an intense inflammatory response. Microbes Infect. 11, 29–39. doi: 10.1016/j.micinf.2008.10.003
Uphoff, C. C., and Drexler, H. G. (2002). Comparative PCR analysis for detection of mycoplasma infections in continuous cell lines. In Vitro Cell Dev. Biol. Anim.38, 79–85. doi: 10.1290/1071-2690(2002)038<0079:CPAFDO>2.0.CO
Urményi, T. P., Silva, R., and Rondinelli, E. (2014). The heat shock proteins of Trypanosoma cruzi. Subcell. Biochem. 74, 119–135. doi: 10.1007/978-94-007-7305-9_5
van Niel, G., D'Angelo, G., and Raposo, G. (2018). Shedding light on the cell biology of extracellular vesicles. Nat. Rev. Mol. Cell Biol. 19, 213–228. doi: 10.1038/nrm.2017.125
Wang, J., Yao, Y., Wu, J., and Li, G. (2015). Identification and analysis of exosomes secreted from macrophages extracted by different methods. Int. J. Clin. Exp. Pathol. 8, 6135–6142.
Wilkowsky, S. E., Barbieri, M. A., Stahl, P., and Isola, E. L. (2001). Trypanosoma cruzi: phosphatidylinositol 3-kinase and protein kinase B activation is associated with parasite invasion. Exp. Cell Res. 264, 211–218. doi: 10.1006/excr.2000.5123
Wilkowsky, S. E., Wainszelbaum, M. J., and Isola, E. L. D. (1996). Trypanosoma cruzi: participation of intracellular Ca2+during metacyclic trypomastigote–macrophage interaction. Biochem. Biophys. Res. Commun. 222, 386–389. doi: 10.1006/bbrc.1996.0753
Witwer, K. W., and Théry, C. (2019). Extracellular vesicles or exosomes? On primacy, precision, and popularity influencing a choice of nomenclature. J Extracell Vesicles. 1:1648167. doi: 10.1080/20013078.2019.1648167
Woolsey, A. M., Sunwoo, L., Petersen, C. A., Brachmann, S. M., Cantley, L. C., and Burleigh, B. A. (2003). Novel PI 3-kinase-dependent mechanisms of trypanosome invasion and vacuole maturation. J. Cell Sci. 116, 3611–3622. doi: 10.1242/jcs.00666
Wyllie, M. P., and Ramirez, M. I. (2017). Microvesicles released during the interaction between Trypanosoma cruzi TcI and TcII strains and host blood cells inhibit complement system and increase the infectivity of metacyclic forms of host cells in a strain-independent process. Pathog. Dis. 75. doi: 10.1093/femspd/ftx077
Yoshida, N. (2006). Molecular basis of mammalian cell invasion by Trypanosoma cruzi. An. Acad. Bras. Cienc. 78, 87–111. doi: 10.1590/S0001-37652006000100010
Keywords: Trypanosoma cruzi, toll-like receptor 2, inflammation, extracellular vesicles, macrophage
Citation: Cronemberger-Andrade A, Xander P, Soares RP, Pessoa NL, Campos MA, Ellis CC, Grajeda B, Ofir-Birin Y, Almeida IC, Regev-Rudzki N and Torrecilhas AC (2020) Trypanosoma cruzi-Infected Human Macrophages Shed Proinflammatory Extracellular Vesicles That Enhance Host-Cell Invasion via Toll-Like Receptor 2. Front. Cell. Infect. Microbiol. 10:99. doi: 10.3389/fcimb.2020.00099
Received: 06 November 2019; Accepted: 26 February 2020;
Published: 20 March 2020.
Edited by:
Noelia Lander, University of Georgia, United StatesReviewed by:
Carlos A. Buscaglia, National Council for Scientific and Technical Research (CONICET), ArgentinaCelio Geraldo Freire-de-Lima, Federal University of Rio de Janeiro, Brazil
Copyright © 2020 Cronemberger-Andrade, Xander, Soares, Pessoa, Campos, Ellis, Grajeda, Ofir-Birin, Almeida, Regev-Rudzki and Torrecilhas. This is an open-access article distributed under the terms of the Creative Commons Attribution License (CC BY). The use, distribution or reproduction in other forums is permitted, provided the original author(s) and the copyright owner(s) are credited and that the original publication in this journal is cited, in accordance with accepted academic practice. No use, distribution or reproduction is permitted which does not comply with these terms.
*Correspondence: Ana Claudia Torrecilhas, ana.torrecilhas@unifesp.br