- 1DNA Replication and Genome Instability Unit, Grupo de Investigación en Biodiversidad, Zoonosis y Salud Pública (GIBCIZ), Instituto de Investigación en Salud Pública y Zoonosis-CIZ, Facultad de Ciencias Químicas, Universidad Central del Ecuador, Quito, Ecuador
- 2Departamento de Bioquímica y Biología Molecular, Universidad de Valencia, Burjassot, Spain
- 3Grupo de Química Computacional y Teórica, Universidad San Francisco de Quito, Quito, Ecuador
- 4Grupo de Bio-Quimioinformática, Carrera de Ingeniería en Biotecnología, Facultad de Ingeniería y Ciencias Aplicadas, Universidad de Las Américas, Quito, Ecuador
Leishmaniasis and trypanosomiasis are largely neglected diseases prevailing in tropical and subtropical conditions. These are an arthropod-borne zoonosis that affects humans and some animals and is caused by infection with protozoan of the genera Leishmania and Trypanosoma, respectively. These parasites present high genomic plasticity and are able to adapt themselves to adverse conditions like the attack of host cells or toxicity induced by drug exposure. Different mechanisms allow these adapting responses induced by stress, such as mutation, chromosomal rearrangements, establishment of mosaic ploidies, and gene expansion. Here we describe how a subset of genes encoding for DNA polymerases implied in repairing/translesion (TLS) synthesis are duplicated in some pathogenic species of the Trypanosomatida order and a free-living species from the Bodonida order. These enzymes are both able to repair DNA, but are also error-prone under certain situations. We discuss about the possibility that these enzymes can act as a source of genomic variation promoting adaptation in trypanosomatids.
Introduction
Trypanososoma spp. and Leishmania spp. protozoan are the etiological agents responsible of serious parasitosis. These parasites are widely distributed among five continents and represents a global health problem still unsolved (World Health Organization, 2020). Here we highlight the observation of DNA polymerases expansion, that opens a new perspective in terms of evolution/adaptation.
It is well-documented that DNA polymerases are responsible for replicating bulky genomic DNA during the S phase, but also have a role to play in repairing/bypassing DNA damage. Therefore, these enzymes play a global role in preserving genetic information and transmitting it to the next generation.
DNA polymerases presents a right-handed structure that embraces the DNA fiber, with three domains: the catalytic palm domain and the most conserved among the polymerases; the thumb domain that is involved in DNA substrate binding and the finger domain that contacts the nascent base pair (Yang and Gao, 2018). Eukaryotic DNA polymerases are classified into four families according to their sequence homology and structural similarities with previous described DNA polymerases: A (E. coli Pol I), B (E. coli Pol II), X (human Pol β) and Y (E. coli UmuC/DinB and eukaryotic RAD30) (Steitz, 1999; Burgers et al., 2001). The replicative enzymes, α-primase, pol δ and pol ε (B-family) are multisubunit enzymes. These polymerases are the ones mainly in charge of duplicating DNA during the S-phase and are highly conserved among all the eukaryotes. These polymerases are characterized by their high processivity and accuracy, with a restricted catalytic active site and are only able to accommodate canonical Watson-Crick base paring (A-T, G-C) (Hübscher et al., 2002). However, what often happens is that exogenous and/or endogenous sources induce DNA lesions, which blocks replication fork. In this situation, replicative DNA polymerases are exchanged by the so-called translesion (TLS) DNA polymerases by a tightly regulated cellular pathway (Pillaire et al., 2014; Yang and Gao, 2018).
Once switched, TLS polymerases bypasses DNA lesions, resolving the blocking situations, and they can perform this task in an error-free or error-prone mode. Structurally, TLS are also right-handed but their catalytic center is bigger and less restrictive, being able to accommodate distorted base pairs and bulky lesions and thereby present a lower accuracy (Yang and Gao, 2018). But TLS and other repairing DNA polymerases have also been involved in repairing pathways as BER (Base Excision Repair) or double strand break (DSB) repairing pathways. For more clarity see Supplementary Table 1, the main roles were summarized based on Burgers et al. (2001).
Trypanosomatids encodes also DNA polymerases from A, B, X, and Y families. These were documented in the firsts whole genome sequencing works performed in trypanosomatids (El-Sayed, 2005; Ivens et al., 2005) and annotated in TriTrypDB (Aslett et al., 2010).
DNA Polymerases Families Overview
Previously we observed that some species of Trypanosoma and Leishmania, harbors more than one copy of genes encoding for certain DNA polymerases. In order to get a general vision about DNA polymerases genes in species from different phyla, orthologs groups were obtained from the OrthoMCL database (Chen et al., 2006) and processed as described in the methodology (Supplementary Table 2). Polymerases with gene duplications in Trypanosomatids were compared with several eukaryotic species (Figure 1). Preliminary results indicate that analyzed kinetoplastids (Euglenozoa phylum) species have some non-replicative polymerases duplicated, particularly we will focus on pol β, κ, η, and mitochondrial DNA pol I. Also, some species of different phyla seems to harbor more than one copy, but contrarily to Euglenozoa, this is not happening in most of the represented species of the corresponding phylum. With the aim of carrying out an in-depth study, we carefully curated the DNA polymerases genes of representative species of the genera Leishmania and Trypanosoma (Table 1). These parasites are eukaryotes distantly related with fungi and metazoans, and display differences during the DNA replication and repair processes (Uzcanga et al., 2016; da Silva et al., 2018).
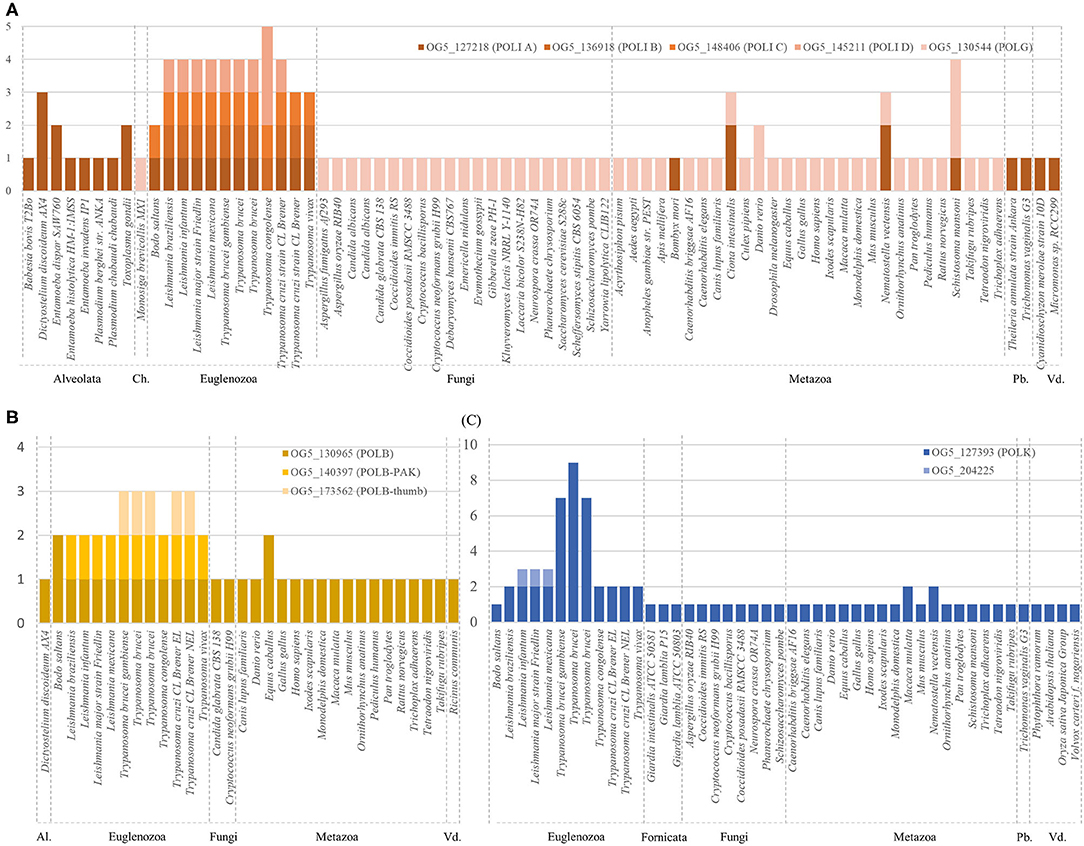
Figure 1. Representation of number of genes (y axe) of DNA polymerases orthologs groups retrieved from OrthoMCL (see Supplementary Table 2). (A) Genes related to mitochondrial DNA polymerases (POLI A-D; POLG) from the indicated orthologs groups. (B) Genes related to POLB from the indicated orthologs groups. (C) Genes related to POLK from the indicated orthologs groups. Species are grouped by phylum. ID from represented genes are available at Supplementary Material. Ch, Choanoflagellata; Pb, Parabasalids; Vd, Viridiplantae; Al, Alveolata.
Expanded DNA Polymerases Families
The A-family of DNA polymerases was described based on sequence homology to DNA pol I (E. coli) and Mus 308 (D. melanogaster) (Yousefzadeh and Wood, 2013). In metazoans three members have been described belonging to this family: Pol Theta (θ), Pol Nu (ν) and the mitochondrial Pol Gamma (γ). In trypanosomatids, Pol θ and mitochondrial-bacterial PolI related family, are present (see Table 1, Supplementary Figures 1,2).
Polymerases I (A to D) belongs to A-family and are involved in replicating kinetoplast DNA (kDNA) (Klingbeil et al., 2002; Fernández-Orgiler et al., 2016; Concepción-Acevedo et al., 2018) These genes are phylogenetically distinct from other family. A members, as bacterial DNA PolI (Harada et al., 2020) or the mitochondrial Pol γ of metazoans (Table 1).
kDNA is a complex network of covalently closed minicircles (0.5–2.5 kbp, 5,000 copies) and maxicircles (20–40 kbp, 25 copies) concatenated among them and these protists have developed a unique replication and segregation strategy (Lukeš et al., 2018). In trypanosomes four PolI-like are involved in replicating mitochondrial DNA. In T. brucei, PolIB, C, and D are essential for accomplishing the replication of kDNA, while PolIA is not; however, a role in replicating minicircles has been described for PolIB and D (Concepción-Acevedo et al., 2018). We found that B. saltans and T. cruzi CL Brener Esmeraldo-like lack the PolID, but still have PolI (A-C) coding genes. We observed in TriTrypDB that other T. cruzi strains have a copy of PolID, and we do not discard that the lack of this gene in T. cruzi CL Brener Esmeraldo-like is a consequence of incomplete sequencing or an annotation failure.
The X-family of DNA polymerases in mammals is represented by Pol β, λ, μ, and TdT. In trypanosomatids, the only member present is Pol β (Yang and Gao, 2018). However, between two and three genes can be founded in different species of trypanosomatids. In mammals, X-family polymerases are able to synthesize DNA without a template and play a main role in gap-filling repair after replication in BER, but also in immunoglobulins chain rearrangement (Tdt, Pol μ). The BER pathway is typically implicated in repairing damaged bases resulting from alkylation or oxidation (Yang and Gao, 2018). In humans, researchers have described Pol β mutations associated with poor cancer prognosis, especially after cisplatin treatment (Ray et al., 2013; Nemec et al., 2017).
In trypanosomatids, Pol β is perhaps one of the most studied TLS polymerases. While only one POLB gene has been described for Leishmania spp (Alonso et al., 2006; Khan et al., 2019), mainly located at the nucleus, in T. cruzi (de Oliveira Lopes et al., 2008; Schamber-reis et al., 2012; Rojas et al., 2018) and T. brucei (Saxowsky et al., 2003), the enzyme is mainly located in mitochondria. Interestingly, in T. brucei, two Pol β variants have been described: Pol β and Pol β-PAK (an Nt domain rich in proline-alanine-lysine, only found in these protozoans). Our analysis shows the presence of Pol β-PAK-like (similar to Pol β-PAK without the presence of PAK domain) in Leishmania species and in T. cruzi, but not in B. saltans. Additionally, we detect the presence of a Pol beta (thumb) in Trypanosoma but not in B. saltans or Leishmania genus (Supplementary Figure 3). We observed that these polymerases share a low sequence similarity (between 7–14%) with the other Pol β studied in this work. Experimental data remains to be carried out to confirm if they are real homologs. In T. cruzi has been shown that Pol β and Pol β-PAK-like have dRP lyase activity, and moreover Pol β-PAK-like is able to bypass 7,8-dihydro-8-oxoguanine (8-oxoG), strongly suggesting specialized roles in BER and TLS for kDNA oxidative protection and maintenance (de Oliveira Lopes et al., 2008; Rojas et al., 2018).
In Leishmania, the expression and activity of Pol β is regulated at the different biological parasitic stages and responds to external factors such as temperature or pH. Pol β activity is upregulated in intracellular amastigote, located inside an acidic parasitophorous vacuole. In this situation, the parasites should deal with the attack of macrophages that induces oxidative damage. According to this, it has been proposed that Pol β plays a major role in repairing oxidative DNA insults or DNA damage induced by drug exposure (Schamber-reis et al., 2012; Khan et al., 2019). The different sublocalization of Pol β among Trypanosoma and Leishmania species could be indicative of a different usage of BER at different compartments, a still unsolved question.
The Y-family translesion polymerases lack the 3′-5′ exonuclease domain and have a larger active pocket site, able to locate distorted base pairs. These properties make the translesion polymerases error-prone in certain conditions, but certain Y-family polymerases are able to replicate damaged templates with precision. Interestingly, some members of this family are amplified in trypanosomatids (Table 1). In particular, we found the amplification of Pol η and Pol κ genes.
Pol η is present in Opisthokonta- including yeast and vertebrates- and green plants. The in vivo function seems to be the TLS replication of specific DNA lesions, mostly in an accurate way. Pol η has a predominant role in accurately replicating cyclobutane pyrimidine dimer (CPD), the main UV-induced DNA lesion. Actually, humans carrying a mutation in the POLH gene develop Xeroderma Pigmentosum type V (XPV), a syndrome characterized for bringing about a predisposition to develop skin cancer through an inability to repair UV-induced damage. In addition, pol η is also able to contribute toward replicating other sorts of lesions: 7,8-dihydro-8-oxoguanine (8-oxoG), one of the most abundant oxidative DNA lesions; and G-G interstrand crosslink, generated for example by cisplatin during chemotherapy, in an error-free manner (Sale, 2013). Overall, it seems that pol η has a protective role against some kinds of lesions, also reported in T. cruzi (de Moura et al., 2009). On the other hand, these enzymes are considerably error-prone on undamaged DNA templates and, moreover, an error-prone TLS bypass seems to operate in some DNA lesions (for example, 8-nitroguanine (8-nitroG) generated by nitric oxide (NO) metabolism), inducing genomic instability and promoting carcinogenesis (Wu et al., 2006; Kawanishi et al., 2017).
POLH genes are duplicated only in the Leishmania genus (Table 1, Supplementary Figure 4), with two/three copies in most of them. Remarkably, the copies are located in tandem within the same chromosome. However, B. saltans and trypanosomes present only one copy. Based on their sequence, the genes are apparently complete and functional, except for the two copies from L. donovani that are truncated. Overall, POLH gene duplication seems to be limited to Leishmania genus.
However, POLK genes amplification is found in Trypanosoma spp. and Leishmania spp., but not in B. saltans. In mammals, Pol κ is the most faithful Y-family polymerase, but is still error-prone. But in general, Pol κ collaborates with Pol ζ to bypass DNA polycyclic aromatic hydrocarbon adducts (such as benzo[a]pyrenes, BDEP), DNA crosslinks (cisplatin), abasic sites and oxidative damage (8-oxo-G or thymine glycol) (Pillaire et al., 2014; Stern et al., 2019; Tonzi and Huang, 2019). So, the efficiency and fidelity of the replication performed depends on the nature of the lesion and the TLS polymerase recruited. Intriguingly, the expression of Pol κ is upregulated in cancerous tumors and it has also been associated with advanced cancer stages (Pillaire et al., 2014).
Pol κ has a Nt domain called N-CLASP, that confers the capacity to encircle the DNA template at the 3'end junction. Additionally, POLK genes have two ubiquitin binding zinc finger domains (UBZs) and a Rev1 interacting region (called RIR). All these domains regulate the binding of Pol κ to the replication fork. The RIR domain allows for the interaction of the TLS polymerases κ, η, and ι with the polymerase Rev1. In a previous study that identified Pol κ in trypanosomatids, the authors described the existence of at least two copies of the Pol κ gene in T. cruzi, one of them located in the mitochondria (Rajão et al., 2009). This enzyme confers protection against DNA damage and in vitro is able to bypass 8-oxoG, indicating a conserved function with respect to other eukaryotes. Also it has been described a role in the extension step on D-loops, an intermediary structure formed during homologous recombination (HR) repairing pathway (Rajão et al., 2009). Similar roles has been described in human Pol η and κ (Sebesta et al., 2013; McVey et al., 2016). However, our BLAST and HMMER searches unveil that POLK genes are amplified in trypanosomatids, with 2-3 genes in Leishmania spp. and up to seven copies in T. brucei. As well as POLH, these copies seem to be the result of a duplication process in tandem. Interestingly, only one copy appears in non-parasitic free-living kinetoplastid, B. saltans (Table 1).
In our analysis, the κ family shows low sequence conservation (similarity) between paralogs and orthologs. The exception to this observation is T. brucei gambiense DAL972 with an expansion in the copy number that is also highly conserved among them (Supplementary Figure 5). This kind of expansion is associated with positive selection to increase the beneficial activity of the duplications (Rogers et al., 2011; Andersson et al., 2015). In order to detect selection over the seven copies of T. brucei gambiense DAL972, we performed analyses in Codeml of PAML software and Fixed Effects Likelihood (FEL) of HyPhy. In both cases, sites under positive selection were detected. For Codeml, the M2 model (sites with positive selection) explains the data better than M1 model (sites with relaxation) (p = 0.001) using the likelihood-ratio test (LRT). The FEL algorithm also found at least five sites under positive selection with a p-value of 0.1. These results suggest a past and/or current adaptive pressure over these sequences.
The duplication of genes involved in DNA repairing and duplication could be a generalized process in trypanosomatids. To test this possibility, we count the number of five genes involved in DNA repairing (RAD1, BRCA2, Ligase 1, and MSH3). We found that these genes are observed in a single copy in the species analyzed here (Supplementary Table 3). The only exception was observed for T. cruzi CL Brener Non-Esmeraldo-like with two copies annotated as mismatch repair protein MSH3.
Discussion
The biological roles of repairing and TLS polymerases in trypanosomatids have been described in recent decades. Here, we want to point out the observation that some families are represented by more than one gene copy, while other polymerases (notably replicative B-family members) present a low fluctuation in number of copies. Here we focus on Pol I, Pol β, and the Y-family Pol η and Pol κ enzymes encoded for more than one allele in trypanosomatids.
Life Cycle
The first question one might ask is if in their natural environment, there is a reason why the amplification, selection, -and possible specialization- of these polymerases families have been favored. Once inoculated in the mammal host, trypanosomatids should deal with the host's immune system and macrophage attack to successfully superimpose the infection (Shio et al., 2012; Marr et al., 2014). Two important macrophage responses to the infection are: i) the production of reactive oxygen species (ROS) triggered after phagocytosis; and ii) the production of nitric oxide (NO), generating 8-oxo-G and 8-nitroG, respectively. The noticeable role of Pol η, Pol κ, and Pol β in TLS synthesis and BER repairing, respectively, raises the question of whether the amplification of POLH/K and POLB genes relies on the need to deal with ROS/NO attacks and overcome DNA lesions.
In trypanosomas Variant Surface Glycoprotein (VSG) antigenic variation is mediated by different strategies involving DNA recombination (McCulloch et al., 2015). Since Y-DNA polymerases are involved in repairing DSB (McIlwraith et al., 2005; Rajão et al., 2009), to delve if they are related in VSG switching is an interesting question.
Kinetoplast
Between three and four copies of mitochondrial PolI are present in Trypanosoma and Leishmania species. kDNA has one of the most complex structures of the eukaryotic mitochondria with a higher amount of DNA in comparison to the nucleus. Indeed, it is a unique structure that provides energy for the cell and for flagella movement. It has been described 3-4 paralogues for DNA Polymerases I in Trypanosomas and Leishmania. Indeed, Trypanosomas have 3-4 orthologs for Pol β and at least one Pol κ in T. cruzi is located at the mitochondria. In that sense, the correlation between the number of mitochondrial polymerases and the kDNA size/architecture of the kinetoplast is highly plausible. Some authors have proposed that the increased kDNA size is a neutral evolution triggered by a runaway expansion of DNA (Lukeš et al., 2018). But even if this is true, the cells still need to replicate and ensure the heritage of the uncommon kDNA. So the maintenance of four different enzymes to perform this function is a very likelihood non-neutral evolutionary process.
Genome Structure (Gene Copy Number Variation, Polysomic Mosaic)
An interesting characteristic of Leishmania is the manifestation of high genomic plasticity. Under different pressure and environmental conditions, different chromosome mosaics are stablished in a few generations, promoting genomic variability (Sterkers et al., 2012; Franssen et al., 2020). On the other hand, evidence involving translesion polymerases in promoting genomic instability and chromosome rearrangements is not fully understood (Kochenova et al., 2011; Sekimoto et al., 2015). But the question here is: are TLS polymerases involved in this process? Of course, further investigation is needed to answer this question.
Interestingly, non-B structured and difficult-to-replicate DNA regions, are present in eukaryotic genomes: Z-DNA, H-DNA triplex, cruciform, hairpins, and G- tetrads (Wickramasinghe et al., 2015; Tsao and Eckert, 2018; Stern et al., 2019). In mammals, Y-family polymerases have been implicated in replicating these particular regions (Eddy et al., 2014, 2016; Wickramasinghe et al., 2015; Quinet et al., 2018; Tsao and Eckert, 2018). Moreover, a role in DNA replication origin has been recently described (Prorok et al., 2019). Trypanosomatids genome have GC-rich sequences and highly repetitive DNA sequences, that organize themselves in no-B structures (Leeder et al., 2016; Belmonte-Reche et al., 2018; Marsico et al., 2019). G-tetrads are present at kinetoplast (Leeder et al., 2016) where a role in RNA editing has been described in the kinetoplast of T. brucei. Predictions indicates that G-tetrads are also frequent at telomeres and in less extent at some chromosomes (Belmonte-Reche et al., 2018). Another study points to an enrichment at 5'UTR in trypanosomas (Marsico et al., 2019). The role of polymerases in replicating these G-tetrads and other non-B DNA structures is an interesting issue that remains to be addressed in trypanosomatids.
Overall, different isolated strains present different polysomy and some supernumerary chromosomes, but genome-wide analyses point to the belief that multicopy genes are preferentially located in chromosomes with a normal chromosome copy number (Rogers et al., 2011; Andersson et al., 2015). Remarkably, only pol η and pol κ result from a tandem amplification in all the organism examined, while pol β and pol I genes are distributed among more than one chromosome. It has been proposed that tandem arrays of duplicated genes and/or chromosome ploidies are an alternative way of increasing gene expression, given the particular transcription control mechanism of trypanosomatids. Alternatively, gene amplification can promote specialization. It has been described that some specific gene families are expanded in the Leishmania genus (Rogers et al., 2011), many of them related to antigenic surface variation. However, to the best of our knowledge, the expansion of DNA polymerases here described is unusual (Genois et al., 2014; Uzcanga et al., 2016).
Limitations and Concluding Remarks
Kinetoplastids are eukaryotes that diverged early during the course of evolution, explaining why this class has some exclusive particularities. In recent years, scientists have launched the genome sequencing of several species from different phyla, providing lots of new information to the scientific community. Here we point out the observation that some DNA polymerases genes are amplified in species from Leishmania and Trypanosoma genera, complementing the peculiarities of these organisms.
Intriguingly, this event appears only in gene coding for some specific families, strongly pointing for a particular molecular mechanism with a different evolutionary trajectory. But this work is not a comprehensive study, and cannot provide the mechanisms or advantages that these extra copies can confer in an ecological and/or physiological context. It is plausible to think that these genes can carry out specialized roles, but to describe them further studies are needed.
An attractive question is the possibility that these enzymes can act as a source of genomic variation promoting adaptation in trypanosomatids, given their natural high genomic plasticity. Genomic instability is directly related to evolutionary adaptation (long term) or development of drug resistance (short term). While more experimental data are need to decipher the molecular roles, it would be fascinating to investigate whether and how these extra copies contribute to the high genomic plasticity of trypanosomatids, or not.
Life Science Identifiers
Life Science Identifiers (LSIDs) for ZOOBANK registered names or nomenclatural acts should be listed in the manuscript before the keywords with the following format:
urn:lsid:<Authority>:<Namespace>:<ObjectID>[:<Version>]
For more information on LSIDs please see Inclusion of Zoological Nomenclature section of the guidelines.
Author Contributions
AP, MM, and VA-J conceived and planned the study. MM and VA-J performed the bioinformatic analyses. AP and VA-J designed and represented the figures. AP wrote the manuscript. All authors reviewed it.
Funding
This research was supported by grants from the Universidad Central del Ecuador (Dirección General de Investigación y Proyectos-DGIP, project #6), SENPLADES (9175.0000.0000.377784 and 91750000.0000.375239, managed by Dirección General de Investigación y Proyectos, DGIP, from UCE), Académie de Recherche et d'Enseignement Supérieur (ARES) in Belgium and Universidad de Las Américas in Quito (project BIO.VAJ.19.06). Part of this work was developed with the support of the University of Valencia during a postdoctoral stay (AP).
Conflict of Interest
The authors declare that the research was conducted in the absence of any commercial or financial relationships that could be construed as a potential conflict of interest.
Acknowledgments
The authors express their sincere thanks to S. de la Cruz, JC. Navarro, G. Uzcanga, T. Gabaldón, M. Pamblanco, and R. Sendra for their valuable contributions and helpful comments. We thank all the staff from CIZ Institute, and the Chemical Sciences Faculty at UCE. We apologized for studies not cited due to space limitations.
Supplementary Material
The Supplementary Material for this article can be found online at: https://www.frontiersin.org/articles/10.3389/fcimb.2020.570493/full#supplementary-material
References
Alonso, A., Terrados, G., Picher, A. J., Giraldo, R., Blanco, L., and Larraga, V. (2006). An intrinsic 5 -deoxyribose-5-phosphate lyase activity in DNA polymerase beta from Leishmania infantum supports a role in DNA repair. DNA Repair (Amst). 5, 89–101. doi: 10.1016/j.dnarep.2005.08.001
Andersson, D. I., Jerlström-Hultqvist, J., and Näsvall, J. (2015). Evolution of new functions de novo and from preexisting genes. Cold Spring Harb. Perspect. Biol. 7:a017996. doi: 10.1101/cshperspect.a017996
Aslett, M., Aurrecoechea, C., Berriman, M., Brestelli, J., Brunk, B. P., Carrington, M., et al. (2010). TriTrypDB: a functional genomic resource for the Trypanosomatidae. Nucl. Acids Res. 38, D457–D462. doi: 10.1093/nar/gkp851
Belmonte-Reche, E., Martínez-García, M., Guédin, A., Zuffo, M., Arévalo-Ruiz, M., Doria, F., et al. (2018). G-quadruplex identification in the genome of protozoan parasites points to naphthalene diimide ligands as new antiparasitic agents. J. Med. Chem. 61, 1231–1240. doi: 10.1021/acs.jmedchem.7b01672
Burgers, P. M. J., Koonin, E. V., Bruford, E., Blanco, L., Burtis, K. C., Christman, M. F., et al. (2001). Eukaryotic DNA polymerases: proposal for a revised nomenclature. J. Biol. Chem. 276, 43487–43490. doi: 10.1074/jbc.R100056200
Chen, F., Mackey, A. J., Stoeckert, C. J. Jr, and Roos, D. S. (2006). OrthoMCL-DB: querying a comprehensive multi-species collection of ortholog groups. Nucl. Acids Res. 34, D363–D368. doi: 10.1093/nar/gkj123
Concepción-Acevedo, J., Miller, J. C., Boucher, M. J., and Klingbeil, M. M. (2018). Cell cycle localization dynamics of mitochondrial DNA polymerase IC in African trypanosomes. Mol. Biol. Cell 29, 2540–2552. doi: 10.1091/mbc.E18-02-0127
da Silva, M. S., Pavani, R. S., Damasceno, J. D., Marques, C. A., McCulloch, R., Tosi, L. R. O., et al. (2018). Nuclear DNA replication in trypanosomatids: there are no easy methods for solving difficult problems. Trends Parasitol. 33, 858–874. doi: 10.1016/j.pt.2017.08.002
de Moura, M. B., Fonseca Schamber Reis, B. L., Passos-Silva, D. G., Andrade Rajao, M., Macedo, A. M., Franco, G. R., et al. (2009). Cloning and characterization of DNA polymerase eta from Trypano- soma cruzi: roles for translesion bypass of oxidative damage. Environ. Andmolecularmutagenes 50, 375–386. doi: 10.1002/em.20450
de Oliveira Lopes, D, Schamber-Reis, B. L. F., Regis-da-Silva, C. G., Rajão, M. A., DaRocha, W. D., Macedo, A. M., et al. (2008). Biochemical studies with DNA polymerase β and DNA polymerase β-PAK of Trypanosoma cruzi suggest the involvement of these proteins in mitochondrial DNA maintenance. DNA Repair. 7, 1882–1892. doi: 10.1016/j.dnarep.2008.07.018
Eddy, S., Ketkar, A., Zafar, M. K., Maddukuri, L., Choi, J. Y., and Eoff, R. L. (2014). Human Rev1 polymerase disrupts G-quadruplex DNA. Nucl. Acids Res. 42, 3272–3285. doi: 10.1093/nar/gkt1314
Eddy, S., Tillman, M., Maddukuri, L., Ketkar, A., Zafar, M. K., and Eoff, R. L. (2016). Human translesion polymerase κ exhibits enhanced activity and reduced fidelity two nucleotides from G-Quadruplex DNA. Biochemistry 55, 5218–5229. doi: 10.1021/acs.biochem.6b00374
El-Sayed, N. M. (2005). Comparative genomics of trypanosomatid parasitic protozoa. Science 309, 404–409. doi: 10.1126/science.1112181
Fernández-Orgiler, A., MartínezJiménez, I., Alonso, A., Alcolea, P. J., Requena, J. M., Thomas, C., et al. (2016). A putative Leishmania DNA polymerase theta protects the parasite against oxidative damage. Nucl. Acids Res. 44, 4855–4870. doi: 10.1093/nar/gkw346
Franssen, S. U., Durrant, C., Stark, O., Moser, B., Downing, T., Imamura, H., et al. (2020). Global genome diversity of the Leishmania donovani complex. Elife 9:e51243. doi: 10.7554/eLife.51243
Genois, M.-M., Paquet, E. R., Laffitte, M.-C. N., Maity, R., Rodrigue, A., Ouellette, M., et al. (2014). DNA repair pathways in trypanosomatids: from DNA repair to drug resistance. Microbiol. Mol. Biol. Rev. 78, 40–73. doi: 10.1128/MMBR.00045-13
Harada, R., Hirakawa, Y., Yabuki, A., Kashiyama, Y., Maruyama, M., Onuma, R., et al. (2020). Inventory and evolution of mitochondrion-localized family A DNA polymerases in Euglenozoa. Pathogens 9:257. doi: 10.3390/pathogens9040257
Hübscher, U., Maga, G., and Spadari, S. (2002). Eukaryotic DNA polymerases. Annu. Rev. Biochem. 71, 133–163. doi: 10.1146/annurev.biochem.71.090501.150041
Ivens, A. C., Peacock, C. S., Worthey, E. A., Murphy, L., Aggarwal, G., Berriman, M., et al. (2005). The genome of the kinetoplastid parasite, Leishmania major. Science 309, 436–442. doi: 10.1126/science.1112680
Kawanishi, S., Ohnishi, S., Ma, N., Hiraku, Y., and Murata, M. (2017). Crosstalk between DNA damage and inflammation in the multiple steps of carcinogenesis. Int. J. Mol. Sci. 18:1808. doi: 10.3390/ijms18081808
Khan, M. I., Mishra, A., Jha, P. K., Abhishek, K., Chaba, R., Das, P., et al. (2019). DNA polymerase β of Leishmania donovani is important for infectivity and it protects the parasite against oxidative damage. Int. J. Biol. Macromol. 124, 291–303. doi: 10.1016/j.ijbiomac.2018.11.159
Klingbeil, M. M., Motyka, S. A., and Englund, P. T. (2002). Multiple mitochondrial DNA polymerases in Trypanosoma brucei. Mol. Cell 10, 175–186. doi: 10.1016/S1097-2765(02)00571-3
Kochenova, O. V., Soshkina, J. V., Stepchenkova, E. I., Inge-Vechtomov, S. G., and Shcherbakova, P. V. (2011). Participation of translesion synthesis DNA polymerases in the maintenance of chromosome integrity in yeast Saccharomyces cerevisiae. Biochemistry 76, 49–60. doi: 10.1134/S000629791101007X
Leeder, W. M., Hummel, N. F. C., and Göringer, H. U. (2016). Multiple G-quartet structures in pre-edited mRNAs suggest evolutionary driving force for RNA editing in trypanosomes. Sci. Rep. 6:29810. doi: 10.1038/srep29810
Lukeš, J., Wheeler, R., Jirsová, D., David, V., and Archibald, J. M. (2018). Massive mitochondrial DNA content in diplonemid and kinetoplastid protists. IUBMB Life 70, 1267–1274. doi: 10.1002/iub.1894
Marr, A. K., MacIsaac, J. L., Jiang, R., Airo, A. M., Kobor, M. S., and McMaster, W. R. (2014). Leishmania donovani infection causes distinct epigenetic DNA methylation changes in host macrophages. PLoS Pathog. 10:e1004419. doi: 10.1371/journal.ppat.1004419
Marsico, G., Chambers, V. S., Sahakyan, A. B., McCauley, P., Boutell, J. M., Antonio, M., et al. (2019). Whole genome experimental maps of DNA G-quadruplexes in multiple species. Nucl. Acids Res. 47, 3862–3874. doi: 10.1093/nar/gkz179
McCulloch, R., Morrison, L. J., and Hall, J. P. J. (2015). DNA recombination strategies during antigenic variation in the african trypanosome. Microbiol. Spectrum. 3:MDNA3–0016-2014. doi: 10.1128/microbiolspec.MDNA3-0016-2014
McIlwraith, M. J., Vaisman, A., Liu, Y., Fanning, E., Woodgate, R., and West, S. C. (2005). Human DNA polymerase η promotes DNA synthesis from strand invasion intermediates of homologous recombination. Mol. Cell 20, 783–792. doi: 10.1016/j.molcel.2005.10.001
McVey, M., Khodaverdian, V. Y., Meyer, D., Cerqueira, P. G., and Heyer, W.-D. (2016). Eukaryotic DNA polymerases in homologous recombination. Annu. Rev. Genet. 50, 393–421. doi: 10.1146/annurev-genet-120215-035243
Nemec, A. A., Abriola, L., Merkel, J. S., de Stanchina, E., deVeaux, M., Zelterman, D., et al. (2017). DNA polymerase beta germline variant confers cellular response to cisplatin therapy. Mol. Cancer Res. 15, 269–280. doi: 10.1158/1541-7786.MCR-16-0227-T
Pillaire, M., Bétous, R., and Hoffmann, J. (2014). Role of DNA polymerase κ in the maintenance of genomic stability. Mol. Cell. Oncol. 1:e29902. doi: 10.4161/mco.29902
Prorok, P., Artufel, M., Aze, A., Coulombe, P., Peiffer, I., Lacroix, L., et al. (2019). Involvement of G-quadruplex regions in mammalian replication origin activity. Nat. Commun. 10:3274. doi: 10.1038/s41467-019-11104-0
Quinet, A., Lerner, L. K., Martins, D. J., and Menck, C. F. M. (2018). Filling gaps in translesion DNA synthesis in human cells. Mutat. Res. Toxicol. Environ. Mutagen. 836, 127–142. doi: 10.1016/j.mrgentox.2018.02.004
Rajão, M. A., Darocha, W. D., Franco, G. R., Macedo, A. M., Pena, S. D. J., Teixeira, S. M., et al. (2009). DNA polymerase kappa from Trypanosoma cruzi localizes to the mitochondria, bypasses 8-oxoguanine lesions and performs DNA synthesis in a recombination intermediate. Mol. Microbiol. 71, 185–197. doi: 10.1111/j.1365-2958.2008.06521.x
Ramiro, T., Hankle, S. T., and Larraga, V. (2002). DNA polymerase beta mRNA determination by relative quantitative RT-PCR from Leishmania infantum intracellular amastigotes. Parasitol. Res. 88, 760–767. doi: 10.1007/s00436-002-0653-0
Ray, S., Menezes, M. R., Senejani, A., and Sweasy, B. (2013). Cellular roles of dnA polymerase beta. Yale J Biol Med. 86, 463–469.
Rogers, M. B., Hilley, J. D., Dickens, N. J., Wilkes, J., Bates, P. A., Depledge, D. P., et al. (2011). Chromosome and gene copy number variation allow major structural change between species and strains of Leishmania. Genome Res. 21, 2129–2142. doi: 10.1101/gr.122945.111
Rojas, D. A., Urbina, F., Moreira-Ramos, S., Castillo, C., Kemmerling, U., Lapier, M., et al. (2018). Endogenous overexpression of an active phosphorylated form of DNA polymerase β under oxidative stress in Trypanosoma cruzi. PLoS Negl. Trop. Dis. 12:e0006220. doi: 10.1371/journal.pntd.0006220
Sale, J. E. (2013). Translesion DNA synthesis and mutagenesis in Eukaryotes. Cold Spring Harb. Perspect. Biol. 5:a012708. doi: 10.1101/cshperspect.a012708
Saxowsky, T. T., Choudhary, G., Klingbeil, M. M., and Englund, P. T. (2003). Trypanosoma brucei has two distinct mitochondrial DNA polymerase β enzymes. J. Biol. Chem. 278, 49095–49101. doi: 10.1074/jbc.M308565200
Schamber-reis, B. L. F., Nardelli, S., Régis-silva, C. G., Carneiro, P., Gonc, P., Almeida, S., et al. (2012). DNA polymerase beta from Trypanosoma cruzi is involved in kinetoplast DNA replication and repair of oxidative lesions. Mol. Biochem. Parasitol. 183, 122–131. doi: 10.1016/j.molbiopara.2012.02.007
Sebesta, M., Burkovics, P., Juhasz, S., Zhang, S., Szabo, J. E., Lee, M. Y. W. T., et al. (2013). Role of PCNA and TLS polymerases in D-loop extension during homologous recombination in humans. DNA Repair. 12, 691–698. doi: 10.1016/j.dnarep.2013.05.001
Sekimoto, T., Oda, T., Kurashima, K., Hanaoka, F., and Yamashita, T. (2015). Both high-fidelity replicative and low-fidelity Y-family polymerases are involved in DNA rereplication. Mol. Cell. Biol. 35, 699–715. doi: 10.1128/MCB.01153-14
Shio, M. T., Hassani, K., Isnard, A., Ralph, B., Contreras, I., Gomez, M. A., et al. (2012). Host cell signalling and Leishmania mechanisms of evasion. J. Trop. Med. (2012) 2012:819512. doi: 10.1155/2012/819512
Steitz, T. A. (1999). DNA polymerases: structural diversity and common mechanisms. J. Biol. Chem. 274, 17395–17398. doi: 10.1074/jbc.274.25.17395
Sterkers, Y., Lachaud, L., Bourgeois, N., Crobu, L., Bastien, P., and Pagès, M. (2012). Novel insights into genome plasticity in Eukaryotes: mosaic aneuploidy in Leishmania. Mol. Microbiol. 86, 15–23. doi: 10.1111/j.1365-2958.2012.08185.x
Stern, H. R., Sefcikova, J., Chaparro, V. E., and Beuning, P. J. (2019). Mammalian DNA polymerase kappa activity and specificity. Molecules 24:2805. doi: 10.3390/molecules24152805
Taladriz, S., Hanke, T., Ramiro, M. J., García-Díaz, M., García De Lacoba, M., Blanco, L., et al. (2001). Nuclear DNA polymerase beta from Leishmania infantum. Cloning, molecular analysis and developmental regulation. Nucl. Acids Res. 29, 3822–3834. doi: 10.1093/nar/29.18.3822
Tonzi, P., and Huang, T. T. (2019). Role of Y-family translesion DNA polymerases in replication stress : implications for new cancer therapeutic targets. DNA Repair. 78, 20–26. doi: 10.1016/j.dnarep.2019.03.016
Tsao, W. C., and Eckert, K. A. (2018). Detours to replication: functions of specialized DNA polymerases during oncogene-induced replication stress. Int. J. Mol. Sci. 19, 1–25. doi: 10.3390/ijms19103255
Uzcanga, G., Lara, E., Gutiérrez, F., Beaty, D., Beske, T., Teran, R., et al. (2016). Nuclear DNA replication and repair in parasites of the genus Leishmania : exploiting differences to develop innovative therapeutic approaches. Crit. Rev. Microbiol. 43, 156–177. doi: 10.1080/1040841X.2016.1188758
Wickramasinghe, C. M., Arzouk, H., Frey, A., Maiter, A., and Sale, J. E. (2015). Contributions of the specialised DNA polymerases to replication of structured DNA. DNA Repair. 29, 83–90. doi: 10.1016/j.dnarep.2015.01.004
World Health Organization (2020). Ending the Neglect to Attain the Sustainable Development Goals - A Road Map for Neglected Tropical Diseases 2021–2030. 1–13. Available online at: https://www.who.int/neglected_diseases/resources/who-ucnntd-2020.01/en/ (accessed July 25, 2020).
Wu, X., Takenaka, K., Sonoda, E., Hochegger, H., Kawanishi, S., Kawamoto, T., et al. (2006). Critical roles for polymerase ζ in cellular tolerance to nitric oxide–induced DNA damage. Cancer Res. 66, 748–754. doi: 10.1158/0008-5472.CAN-05-2884
Yang, W., and Gao, Y. (2018). Translesion and repair DNA polymerases : diverse structure and mechanism. Annu. Rev. Biochem. 87, 239–261. doi: 10.1146/annurev-biochem-062917-012405
Yousefzadeh, M. J., and Wood, R. D. (2013). Mini review DNA polymerase POLQ and cellular defense against DNA damage. DNA Repair. 12, 1–9. doi: 10.1016/j.dnarep.2012.10.004
Keywords: DNA polymerases, translesion synthesis, trypanosomatids, genome stability, DNA repair, gene amplification
Citation: Poveda A, Méndez MÁ and Armijos-Jaramillo V (2020) Analysis of DNA Polymerases Reveals Specific Genes Expansion in Leishmania and Trypanosoma spp. Front. Cell. Infect. Microbiol. 10:570493. doi: 10.3389/fcimb.2020.570493
Received: 08 June 2020; Accepted: 02 September 2020;
Published: 07 October 2020.
Edited by:
Julius Lukes, Academy of Sciences of the Czechia Republic (ASCR), CzechiaReviewed by:
Richard McCulloch, University of Glasgow, United KingdomCarlos Renato Machado, Federal University of Minas Gerais, Brazil
Copyright © 2020 Poveda, Méndez and Armijos-Jaramillo. This is an open-access article distributed under the terms of the Creative Commons Attribution License (CC BY). The use, distribution or reproduction in other forums is permitted, provided the original author(s) and the copyright owner(s) are credited and that the original publication in this journal is cited, in accordance with accepted academic practice. No use, distribution or reproduction is permitted which does not comply with these terms.
*Correspondence: Ana Poveda, YXBvdmVkYUB1Y2UuZWR1LmVj; Vinicio Armijos-Jaramillo, dmluaWNpby5hcm1pam9zQHVkbGEuZWR1LmVj