- 1Laboratory of Veterinary Public Health, Joint Faculty of Veterinary Medicine, Yamaguchi University, Yamaguchi, Japan
- 2Department of Pathology and Parasitology, Patuakhali Science and Technology University, Barisal, Bangladesh
- 3Department of Veterinary Science, National Institute of Infectious Diseases, Tokyo, Japan
Francisella tularensis, the causative agent of tularemia, is transmitted by arthropod vectors within mammalian hosts. The detailed mechanisms contributing to growth and survival of Francisella within arthropod remain poorly understood. To identify novel factors supporting growth and survival of Francisella within arthropods, a transposon mutant library of F. tularensis subsp. novicida (F. novicida) was screened using an F. novicida–silkworm infection model. Among 750 transposon mutants screened, the mltA-encoding membrane-bound lytic murein transglycosylase A (MltA) was identified as a novel growth factor of F. novicida in silkworms. Silkworms infection with an mltA deletion mutant (ΔmltA) resulted in a reduction in the number of bacteria and prolonged survival. The ΔmltA strain exhibited limited intracellular growth and cytotoxicity in BmN4 silkworm ovary cells. Moreover, the ΔmltA strain induced higher expression of the antimicrobial peptide in silkworms compared to the wild-type strain. These results suggest that F. novicida MltA contributes to the survival of F. novicida in silkworms via immune suppression-related mechanisms. Intracellular growth of the ΔmltA strain was also reduced in human monocyte THP-1 cells. These results also suggest the contribution of MltA to pathogenicity in humans and utility of the F. novicida–silkworm infection model to explore Francisella infection.
Introduction
Francisella tularensis is a facultative intracellular pathogen and the causative agent of tularemia (Ellis et al., 2002). It shows high infectivity via aerosol transmission; as few as 10 F. tularensis bacterial cells can cause disease in humans (McLendon et al., 2006). As such, F. tularensis may be deployed as a potential biological weapon and is currently classified as a category A bioterrorism agent by the US Centers for Disease Control and Prevention (CDC) (Dennis et al., 2001). Among the different subspecies, only F. tularensis subsp. tularensis (Type A) and F. tularensis subsp. holarctica (Type B) are highly pathogenic to humans (Maurin, 2015). F. tularensis subsp. novicida, referred to here as F. novicida, shares significant homology with F. tularensis subsp. tularensis, as it is virulent in mice but nonpathogenic to immunocompetent humans. As such, F. novicida serves as a surrogate organism for experimental studies (Kingry and Petersen, 2014). Although F. tularensis has been identified in a wide range of organisms, including mammals, birds, amphibians, and invertebrates (Keim et al., 2007), the disease tularemia predominantly occurs in rodents, voles, hares, rabbits, and humans (Carvalho et al., 2014). Interactions between F. tularensis and arthropod hosts play major roles in supporting its life cycle (Petersen et al., 2009). The pathogen is transmitted between animals including humans by arthropod vectors such as deer flies, horse flies, ticks, and mosquitoes (Carvalho et al., 2014). The routes of infection to humans are varied, e.g. inhalation, contact with infection animals or contaminated tissues (Ellis et al., 2002). Arthropods vectors are also considered as one of main infection routes of F. tularensis (Carvalho et al., 2014). However, the detailed mechanisms of the growth and survival of F. tularensis in arthropod vectors remain poorly understood.
Mammalian infection models are typically used for the identification and evaluation of virulence factors; however, large-scale experiments using mammals raise the issue of ethics and feasibility. To address this issue, we have developed a non-mammalian infection model using the silkworm, Bombyx mori. As silkworms have been domestically cultivated for the past 5000 years to produce silk, there are many lineages, which are well-defined and maintained, and tools for genetic engineering of these organisms are also readily available. Silkworms survive at 37°C; this is the optimal temperature for humans and one that supports the growth of most pathogens (Kaito et al., 2011). The body size of silkworm larva (3–85 mm) and the period of the larvae (25 days) facilitate injection of pathogens and experimental repetitions. Therefore, B. mori larvae have been used as infection models for experiments focused on a large variety of agents, such as bacteria, viruses, and fungi (Kaito et al., 2002; Jiang et al., 2016; Kaito, 2016; Uchida et al., 2016). Recently, non-mammalian infection models, including the wax moth (Galleria mellonella), fruit fly (Drosophila melanogaster), cockroach (Blaptica dubia), and zebrafish (Danio rerio), have all been employed as models to promote understanding of host–pathogen interactions associated with Francisella (Aperis et al., 2007; Vonkavaara et al., 2008; Vojtech et al., 2009; Ahlund et al., 2010; Moule et al., 2010; Eklund et al., 2017). Compared with these infection models, the silkworm model has advantages with respect to body size, breeding period, breeding equipment, temperature, cost, and availability of genetic information. We have developed both symbiotic and pathogenic silkworm infection models. Infection with F. tularensis subsp. holarctica LVS (LVS), the bacteria fail to proliferate and are maintained in the silkworm in a symbiotic relationship (Suzuki et al., 2016). By contrast, F. novicida proliferates in silkworms and promotes a lethal infection; these results underscore the pathogenic properties of F. novicida in silkworm (Saha et al., 2017).
We herein carried out a large-scale screening of a F. novicida transposon mutant library using the F. novicida–silkworm infection model. Membrane-bound lytic murein transglycosylase A (MltA) was identified as a novel factor, which supports growth and survival of Francisella in arthropod species.
Materials and Methods
Bacterial Strains and Culture Conditions
F. novicida U112 was obtained from the Pathogenic Microorganism Genetic Resource Stock Center (Gifu University, Japan). F. novicida was cultured aerobically at 37°C in a chemically defined medium (CDM) (Nagle et al., 1960), brain heart infusion broth (Becton, Dickinson and Company, Franklin Lakes, NJ) supplemented with cysteine (BHIc) (Mc Gann et al., 2010), or BHIc containing 1.5% agar (Wako Laboratory Chemicals, Osaka, Japan). All experiments were conducted in compliance with the institutional biosecurity guidelines and were approved by Yamaguchi University.
Cell Culture
Human monocytic THP-1 cells were grown in Roswell Park Memorial Institute (RPMI) 1640 medium (Sigma-Aldrich, St. Louis, MO) supplemented with 10% heat-inactivated fetal bovine serum at 37°C in an atmosphere containing 5% CO2. Silkworm ovary-derived BmN4 cells were grown in Sf-900 III SFM medium (Thermo Fisher, Waltham, MA) at 27°C.
Silkworms
Fourth instar B. mori larvae (Hu/Yo × Tukuba/Ne) were obtained from Ehime-Sanshu (Ehime, Japan). The larvae were raised by feeding them with Silkmate 2M (Nosan Corporation, Kanagawa, Japan) at room temperature (25°C).
Plasmid Construction, Transformation, and Transfection
Supplementary Table 1 lists the primer sets and templates used to construct the plasmids used in this study. Polymerase chain reaction (PCR) was performed using KOD-Plus-Neo polymerase (Toyobo, Osaka, Japan), and ligation was performed using an In-Fusion HD Cloning Kit (Takara Bio, Shiga, Japan). Plasmids were used to transform F. novicida via electroporation. Specifically, bacterial cells were suspended 0.5 M sucrose with 2 μg of plasmid DNA and were electroporated using a Bio-Rad micropulser (Bio-Rad, Hercules, CA) at 3.0 kV, 10 µF, and 600 Ω with 0.2 cm cuvette; transformants were precultured in CDM medium overnight. To select the transformed bacteria, the pre-incubated bacteria were cultured on BHIc agar plates containing 30 μg/ml kanamycin or 2.5 μg/ml chloramphenicol.
Construction of a Transposon Mutant Library
The transposon mutant library was constructed using the Ez-Tn5 transposon system (Epicentre, Madison, WI) as previously reported (Nakamura et al., 2019). Briefly, the multiple cloning site of pMOD3 was opened by digestion with Hind III and EcoRI, and the kanamycin resistance cassette of pKEK1140 (Rodriguez et al., 2008) was ligated into these sites to generate pMOD3-FtKm. The transposon moiety of pMOD3-FtKm was amplified by PCR, purified, mixed with transposase according to the instruction manual, and then used to transform F. novicida via electroporation. Transformed bacteria were cultured on BHIc plates containing 30 μg/ml kanamycin.
Sequence Analysis of Transposon Mutants
pMOD3 harbors the E. coli R6Kγ origin of replication. The genomes of F. novicida transposon mutants were purified using a PureLink Genomic DNA Mini Kit (Thermo Fisher) and digested with a combination of restriction enzymes, such as XhoI, BglII, EcoRI, SalI, NotI, and BamHI. The ends of the digested DNAs were then blunted using a DNA Blunting Kit (Takara Bio) and ligated using Ligation High Ver. 2 (Toyobo). The ligated DNA was used to transform One Shot PIR1 Chemically Competent E. coli (Thermo Fisher). The transformed E. coli were selected for kanamycin resistance, and the plasmid DNAs were purified. Sequence analysis was performed using the primer described in the instruction manual for the Ez-Tn5 transposon system.
Construction of F. novicida Mutants
A deletion mutant of dotU (ΔdotU) was previously constructed (Shimizu et al., 2019) through group II intron insertion using a TargeTron Gene Knockout System (Sigma-Aldrich), which was modified for Francisella species (Rodriguez et al., 2008). A deletion mutant of mltA (ΔmltA) was generated via homologous recombination using the Francisella suicide vector pFRSU (Shimizu et al., 2019). The upstream and downstream regions of mltA (1.5 kbp each) were cloned into the BamHI site of pFRSU to generate pFRSU-mltA. The pFRSU-mltA vector (2 μg) was used to transform F. novicida; transformants were selected on BHIc plates containing 30 μg/ml kanamycin. Isolated bacteria were cultured in BHIc without antibiotics overnight and then plated on BHIc plates containing 5% sucrose. The deletion of the mltA gene was confirmed via PCR.
Green Fluorescent Protein (GFP)- and MltA-Expressing F. novicida Strains
A GFP-expressing plasmid pOM5-GFP was constructed according to published procedures (Shimizu et al., 2019). The F. novicida chromosomal mltA gene with its native promoter region (300 bp upstream) was cloned into pOM5 to generate pOM5-MltA. pOM5-MltA and pOM5-GFP were used to transform the wild-type or ΔmltA strains of F. novicida via electroporation.
Silkworm Infection Assay
Hemocoels of day 2 fifth instar larvae were inoculated with 50 μl of bacterial suspension in BHIc medium at 5 × 106 colony-forming unit (CFU)/larva using a 1 ml syringe equipped with a 30-gauge needle (Terumo Corp., Tokyo, Japan). After inoculation, the silkworms were incubated at room temperature with free access to food. The mortality rate of the infected silkworms was assessed daily for 9 days. The bacterial load was evaluated at the same time each day for 4 days after infection. To obtain bacterial counts (CFU/larva) from whole silkworm bodies, the infected larvae were placed in disposable 15 ml centrifuge tubes and homogenized in 3 ml phosphate-buffered saline (PBS) using Biomasher SP (Funakoshi Co., Ltd, Tokyo, Japan) followed by centrifugation at 300 × g for 30 s. Appropriate dilutions of the suspension samples were spread onto BHIc agar plates to facilitate colony counts.
Intracellular Growth Assay
THP-1 cells (4 × 105 cells/well) were incubated in a 48-well tissue culture plate with 200 nM phorbol myristate acetate (PMA) for 48 h. Then, F. novicida strains were added at a multiplicity of infection (MOI) = 1. Next, plates were centrifuged for 10 min at 300 × g and incubated for 1 h at 37°C. The cells were washed three times with RPMI 1640 medium, and extracellular bacteria were killed with gentamicin at 50 μg/ml for 1 h. The cells were then incubated in fresh medium at 37°C for the times indicated. To measure intracellular growth, the cells were washed with PBS and then lysed with 0.1% Triton X-100 in CDM. The number of CFUs was determined on BHIc agar plates via plating serial dilutions of cultures. Similar methods were used to determine growth in BmN4 cells (3 × 105 cells/well), which were incubated in a 48-well tissue culture plate overnight at 27°C in Sf-900 III SFM medium.
Fluorescence Microscopy
THP-1 cells (4 × 105 cells/well) were incubated with 200 nM PMA for 48 h on 12 mm glass coverslips in 24-well tissue culture plates. GFP-expressing F. novicida strains were introduced at MOI = 1. Plates were centrifuged for 10 min at 300 × g and incubated for 1 h at 37°C. The cells were washed three times with RPMI 1640 medium, and extracellular bacteria were eliminated with gentamicin at 50 μg/ml for 1 h. The cells were then incubated in fresh medium at 37°C for the times indicated. Cells were fixed with 4% paraformaldehyde at room temperature for 30 min. A FluoView FV100 confocal laser scanning microscope (Olympus, Tokyo, Japan) was used to obtain images of the cells. Similarly, BmN4 cells (3 × 105 cells/well) were grown on 12 mm glass coverslips in Sf-900 III SFM in 24-well tissue culture plates and were incubated overnight at 27°C; a similar procedure was performed. To examine the hemocytes, the hemocoels of day 2 fifth instar larvae were inoculated with 50 μl of bacterial suspension at 5 × 106 CFU/larva. At indicated times after inoculation, hemolymph was collected via an incision in the caudal horn and observed using a FluoView FV100 confocal laser scanning microscope.
Trypan Blue Exclusion Assay
BmN4 cells (3 × 105 cells/well) were incubated in a 24-well tissue culture plate overnight and were then infected with F. novicida strains at MOI = 1. The plates were then centrifuged for 10 min at 300 × g and incubated for 1 h. Subsequently, the cells were washed three times with Sf-900 III SFM medium, and extracellular bacteria were eliminated with gentamicin at 50 μg/ml for 1 h. Cells were provided with fresh medium and grown for 48 h at 27°C. Cells were then resuspended in an equivalent volume of 0.5% trypan blue solution (Nacalai Tesque, Kyoto, Japan) to facilitate counting of live (trypan blue negative) or dead (trypan blue positive) cells using a hemocytometer. Cell death was determined as the percentage of dead (blue) cells in the grid square.
Lactate Dehydrogenase (LDH) Release Assay
THP-1 cells (4 × 105 cells/well) were incubated in a 48-well tissue culture plate with 200 nM PMA for 48 h. Cells were infected with F. novicida strains at MOI = 0.01. The plates were centrifuged for 10 min at 300 × g and incubated for 1 h and then washed three times with RPMI 1640 medium, and extracellular bacteria were eliminated with gentamicin (50 μg/ml for 1 h). Cells were then incubated in fresh medium at 37°C for 48 h. Release of LDH into culture medium was measured using an LDH Cytotoxicity Detection Kit (Takara Bio).
Immunoblotting
Hemocoels of day 2 fifth instar larvae were inoculated with 50 μl of bacterial suspension at OD595 = 0.05 in BHIc medium using a 1 ml syringe equipped with a 30-gauge needle. After 6, 12, and 24 h, the hemolymph was collected from the caudal horn. The proteins in 0.5 μl of hemolymph were separated by SDS-PAGE on a 4%–12% Bis-Tris Gel (Thermo Fisher) and were transferred onto polyvinylidene difluoride membranes (Millipore, Billerica, MA, USA). The membranes were incubated overnight with anti-cecropin B antibody (1:1,000; ab27571; Abcam, Cambridge, UK) at 4°C. The membrane was then incubated with horseradish peroxidase-conjugated secondary antibody (0.01 μg/ml) at room temperature and was developed using an enhanced chemiluminescence detection system (GE Healthcare Life Science, Little Chalfont, UK).
Statistical Analysis
Multiple comparisons using the Tukey–Kramer test were used to evaluate the significance of differences between groups. The Kaplan–Meier method was used to generate survival curves, and the log-rank test was used to evaluate the significance of differences compared with the wild type strain; P < 0.05 indicates a significant difference.
Results
Identification of Genes Required for the Growth of F. novicida in Arthropods
We previously demonstrated that F. novicida can replicate in silkworms and ultimately promote death (Saha et al., 2017). To identify novel factors that support growth of F. novicida in arthropods, silkworms were infected with a transposon mutant library previously developed (Nakamura et al., 2019); strains with reduced pathogenicity for silkworms were identified. The screening was performed by infecting three silkworms with each transposon mutant of F. novicida. Among 750 characterized transposon mutants, we identified 26 mutants that failed to kill at least one of the three silkworms; these were identified as strains with reduced pathogenicity. To confirm the reduced pathogenicity of these mutants, 10 silkworms were infected with each of these 26 transposon mutants. A ΔdotU strain, lacking a gene encoding part of the type VI secretion system, was used as a negative control (Suzuki et al., 2016). Among the 26 mutants, we identified 8 mutants that permitted more than 80% survival; these were identified as strains with minimal pathogenicity in the silkworm infection model (Figure 1). To identify the genes responsible for the pathogenicity, transposon insertion sites of the mutant strains were evaluated; seven unique genes were identified (Table 1). In this study, we focused on the characteristics and pathogenicity associated with mltA (FTN_1286), the gene encoding hypothetical membrane-bound lytic transglycosylase MltA.
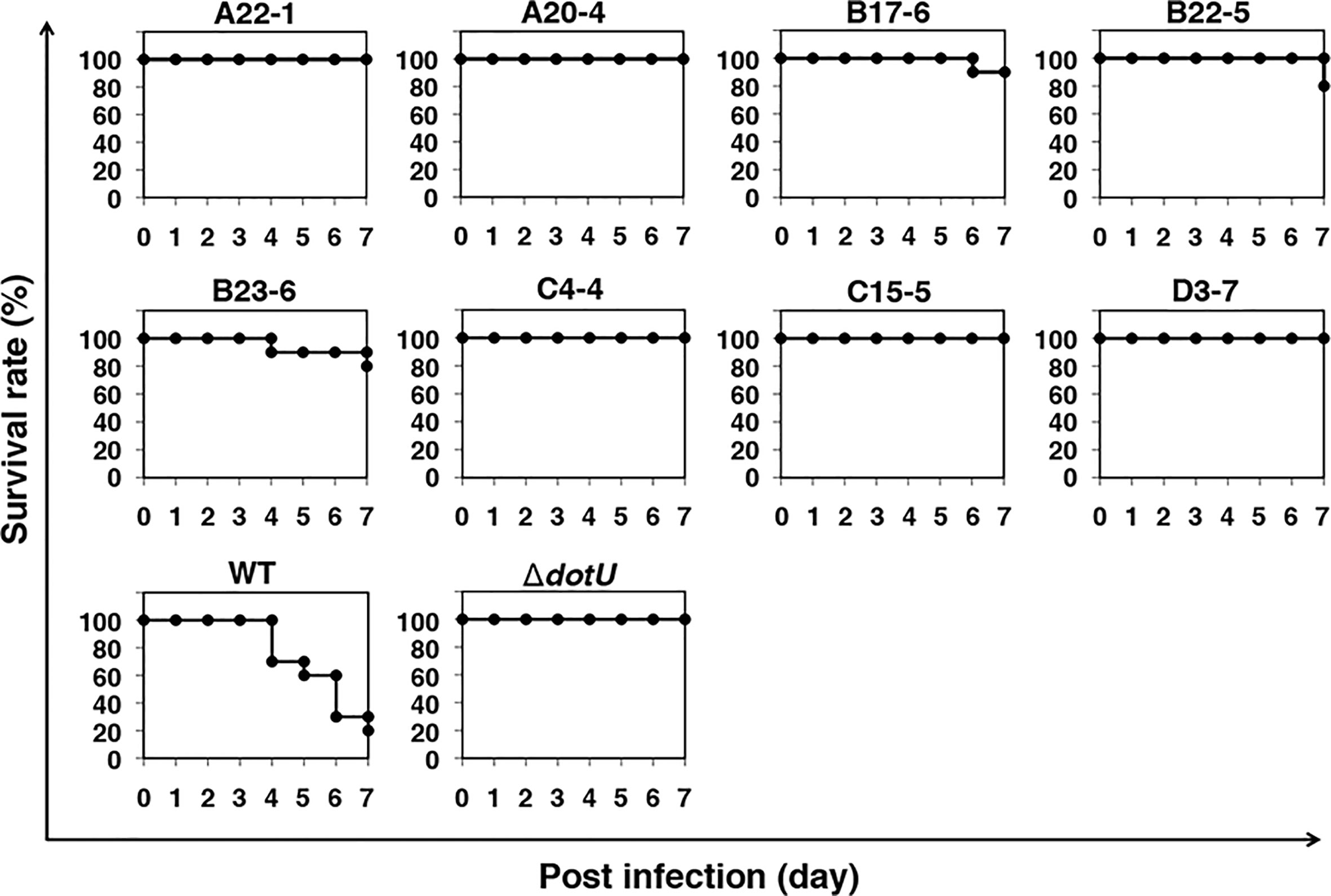
Figure 1 Screening of a transposon mutant library using a silkworm infection model. Each group of silkworms (n = 10) was infected with either the wild-type (WT), negative control (ΔdotU), or transposon mutant strains of F. novicida at 5 × 106 CFU/larva. Survival was determined at each time point as indicated.
Characteristics of an mltA Deletion Mutant
To analyze the effect of MltA in Francisella infection, a ΔmltA strain of F. novicida was constructed via homologous recombination. The known functions of MltA are related to degradation of cell wall peptidoglycan (PG); cell morphology and growth rates of the mutant strains were evaluated. The ΔmltA cells all maintained the characteristic rod shape in the early-exponential phase of liquid culture and were otherwise indistinguishable from the wild-type strain (Figure 2A). The growth rates of ΔmltA strain and of a complemented (ΔmltA/mltA) strain in liquid culture medium were slightly slower than those of the wild-type strain and of the transposon mutant at mid-exponential phase; there were no significant differences at late- exponential phase (Figure 2B).
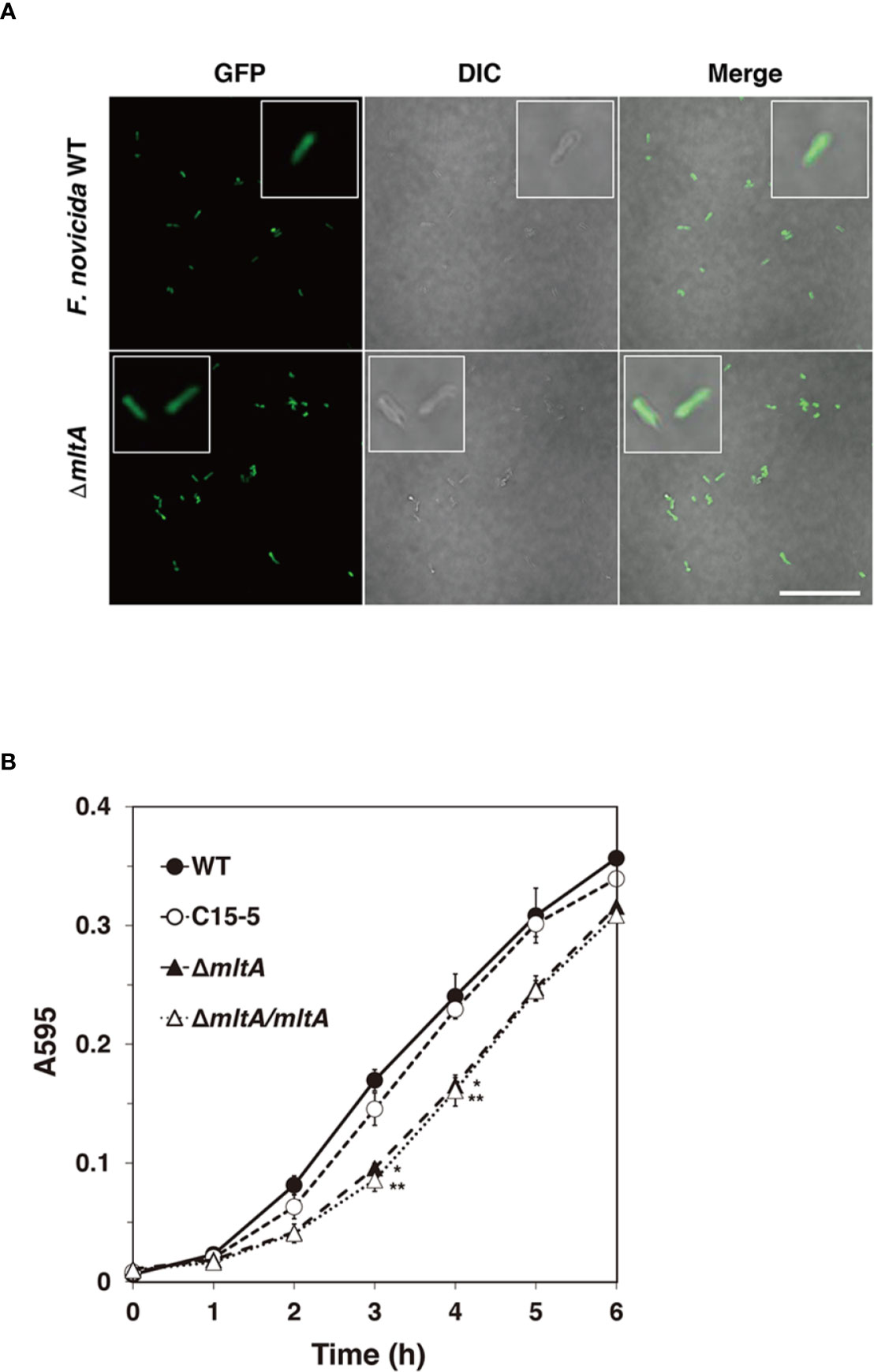
Figure 2 Characteristics of the mltA deletion mutant. (A) The green fluorescent protein (GFP)-expressing wild-type (WT) and ΔmltA strains of F. novicida at early-exponential phase in BHIc medium. High-magnification images are as shown in the box frame. Scale bar = 50 µm. (B) The wild-type (WT), transposon mutant (C15-5), ΔmltA, and the complemented (ΔmltA/mltA) strains of F. novicida were cultured in BHIc medium. Absorbance was measured at 595 nm. The data represent the averages and standard error of three identical experiments. Differences from the wild-type strain were determined via multiple comparison methods and are indicated by asterisks; **P < 0.01, *P < 0.05.
Role of MltA in Promoting Multiplication and Virulence of F. novicida in Silkworm
To explore the role of mltA in promoting virulence, silkworms were infected with the ΔmltA strain. All silkworms infected with the wild-type strain were dead within 7 days; by contrast, 80%–90% of the silkworms infected with the ΔmltA or transposon mutant strains remained viable at 9 days after infection (Figure 3A). Complementation (ΔmltA/mltA) restored virulence; survival of silkworms infected with this strain was comparable to that of the wild-type strain (Figure 3A). The number of bacterial cells in whole silkworms was also determined. As expected, the wild-type and complemented strains increased in number through 1 to 2 days post infection in whole silkworms; no growth of the ΔmltA or transposon mutant strains was detected (Figure 3B). A similar tendency was observed when analyzing the silkworm hemolymph. Specifically, when silkworms were infected with a GFP-expressing wild-type F. novicida, the bacterial population detected in the hemolymph increased at 24 to 48 h post infection (Figure 3C). This was not observed in response to infection with GFP-expressing ΔmltA strain (Figure 3C). Taken together, these results indicate that MltA is important for growth and virulence of F. novicida in silkworms.
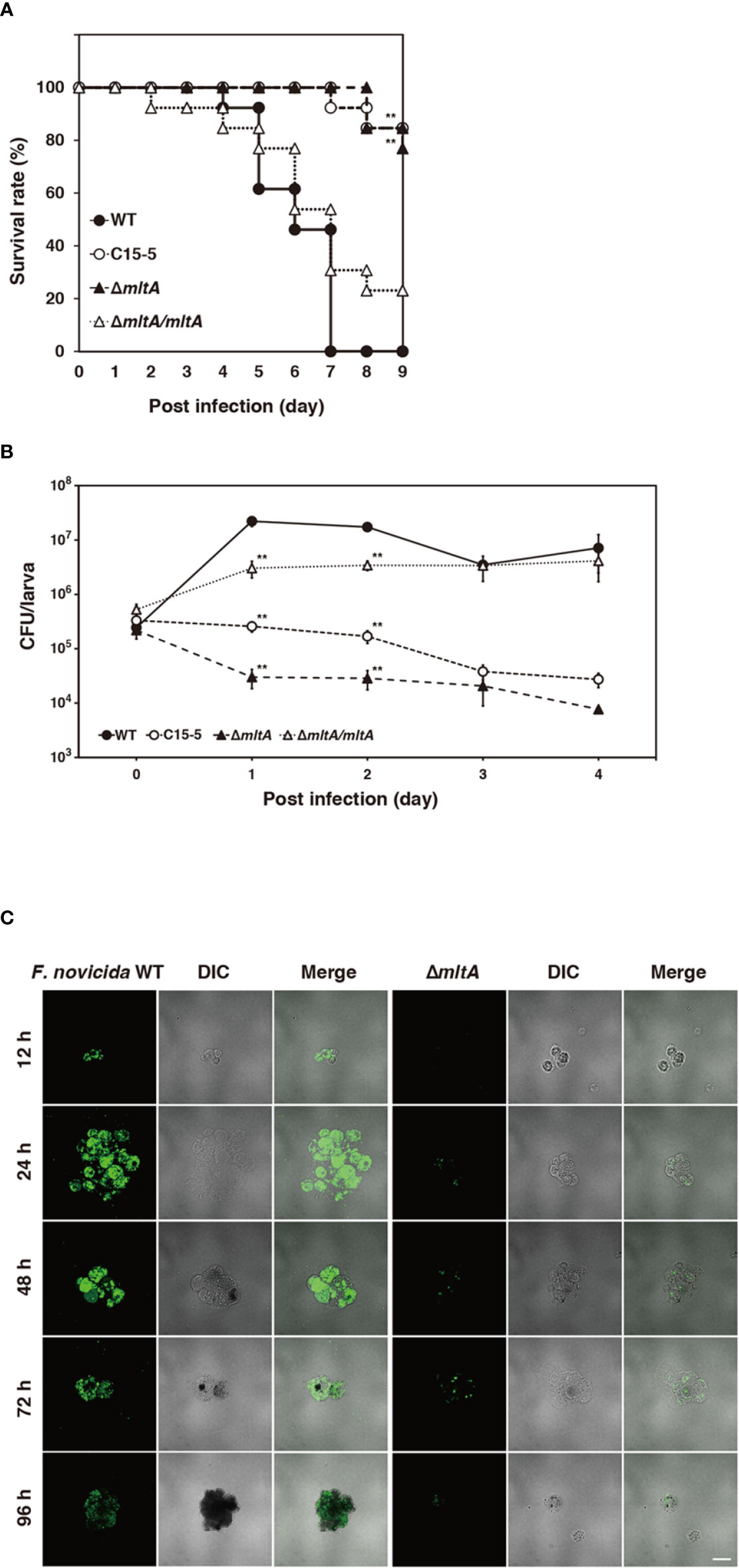
Figure 3 Role of MltA in multiplication and virulence of F. novicida in the silkworm infection model. (A) Each group of silkworms (n = 13) was infected with the wild-type (WT), transposon mutant (C15-5), ΔmltA, or the complemented (ΔmltA/mltA) strains of F. novicida at 5 × 106 CFU/larva. Survival was evaluated at the time points; differences from the wild-type strain were evaluated via log-rank test; **P < 0.01. (B) Each group of silkworms (n = 15) was infected with the wild-type (WT), transposon mutant (C15-5), ΔmltA, and the complemented (ΔmltA/mltA) strains of F. novicida at 5 × 106 CFU/larva. Bacterial numbers in silkworms were determined at 0 (immediately after infection), 1, 2, 3, 4 days post infection. These data represent the averages and standard error of bacterial numbers in three different silkworms. Differences from the wild-type strain were determined via multiple comparisons and are indicated by asterisks; **P < 0.01. (C) Silkworms were infected with the green fluorescent protein (GFP)-expressing wild-type (WT) or ΔmltA strains of F. novicida. Hemolymph was harvested at time points indicated and observed by confocal laser scanning microscopy. Scale bar = 20 µm.
Effect of MltA on Intracellular Growth and Cytotoxicity of F. novicida in BmN4 Cells
To confirm the role of MltA in promoting intracellular growth of F. novicida in silkworm cells, F. novicida strains were used to infect to cultures of the silkworm ovary BmN4 cell line (Grace, 1967). The wild-type strain grew intracellularly in BmN4 cells from 2 to 48 h post infection; by contrast, the ΔmltA and transposon mutant strains showed comparatively limited growth under these conditions. The complemented strain restored the capacity for intracellular growth (Figures 4A, B). To investigate a role for MltA in promoting cytotoxicity, BmN4 cells were infected with F. novicida strains and cell death was evaluated by the trypan blue exclusion assay. Among our results, the ΔmltA strain was significantly less cytotoxic than either the wild-type or complemented strains (Figure 4C). These results suggest that MltA is necessary for intracellular growth and is a critical factor underlying the cytotoxicity of F. novicida within silkworm cells.
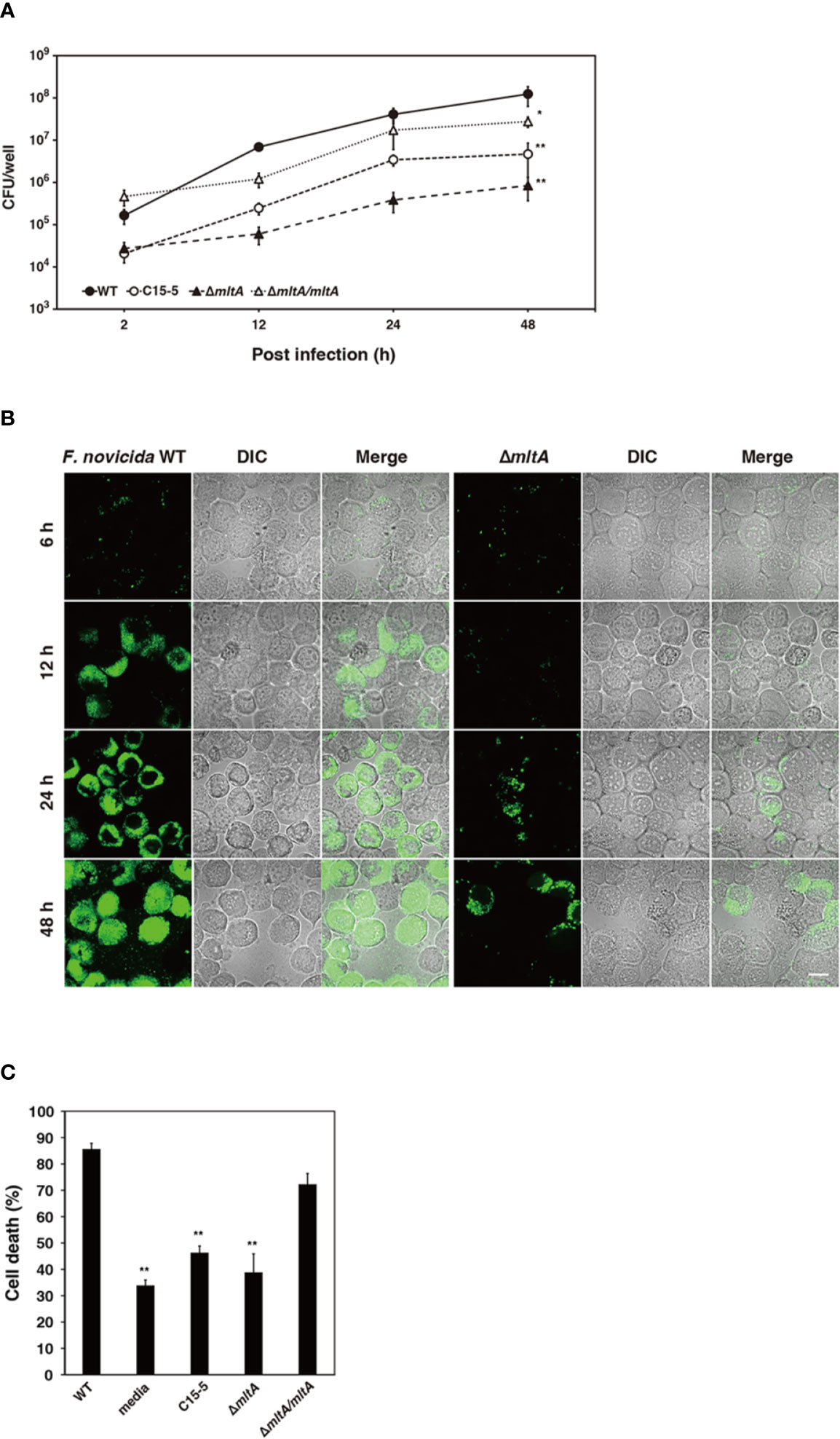
Figure 4 Impact of MltA on intracellular growth and cytotoxicity of F. novicida in BmN4 cells. (A) BmN4 cells were infected with the wild-type (WT), transposon mutant (C15-5), ΔmltA, or the complemented (ΔmltA/mltA) strains of F. novicida at MOI = 1 followed by treatment with 50 µg/ml gentamicin for 1 h. The cells were disrupted with 0.1% Triton X-100 and plated on BHIc agar at the time points indicated. (B) BmN4 cells were infected with the green fluorescent protein (GFP)-expressing wild-type (WT) or ΔmltA strains of F. novicida at MOI = 1 and treated 50 µg/ml gentamicin for 1 h. The cells were fixed and evaluated by confocal laser scanning microscopy at indicated time points. Scale bar = 20 µm. (C) BmN4 cells were infected with the wild-type (WT), transposon mutant (C15-5), ΔmltA, and the complemented (ΔmltA/mltA) strains of F. novicida at MOI = 1 and treated 50 µg/ml gentamicin for 1 h and incubated for 48 h. Cell survival was evaluated by trypan blue exclusion assay. (A, C) The data represent the averages and standard error of three identical experiments. Differences from the wild-type strain were determined via multiple comparisons and are indicated by asterisks; **P < 0.01, *P < 0.05.
Immune Suppression of Silkworm Promoted by MltA
F. tularensis suppresses host immune systems of both mammals and arthropods (Suzuki et al., 2016; Putzova et al., 2017). One of the main immune responses of arthropods when confronted with pathogens is the production of antimicrobial peptides (AMPs) (Chen and Lu, 2018). To explore the impact of MltA on the immune responses in silkworms, we examined expression levels of the major AMP, cecropin B, by immunoblotting. We found that the induction of cecropin B expression was accelerated in response to infection with the ΔmltA strain. The ΔmltA-induced expression of cecropin B was detected as early as 6 h post infection, and no response was observed in response to infection with the wild-type strain at this time point. Cecropin B was detected at 12 h post infection with both the wild-type and ΔmltA strains, although expression was stronger in the ΔmltA-infected silkworms. At 24 h post infection, expression levels were nearly indistinguishable (Figure 5). These results suggest that F. novicida promotes host immune suppression at the early stages of infection via an MltA-dependent mechanism.

Figure 5 MltA-mediated host immune suppression in the silkworm infection model. Hemolymph plasma samples from silkworms infected with the wild-type (WT) or the ΔmltA strains of F. novicida were collected at post-infection time points as indicated. Cecropin B expression was determined by immunoblotting. Representative data from three independent experiments are as shown. Full blots are included in Supplementary Figure 1.
Impact of MltA on the Pathogenicity of F. novicida in Human Macrophage Cell Line
Intracellular growth of Francisella in macrophages is closely related to its pathogenicity in humans (Ozanic et al., 2015). To determine whether mltA plays an important role in human virulence, cells of the human monocyte cell line, THP-1, were infected with the wild-type and mutant strains of F. novicida. The wild-type strain multiplied intracellularly within THP-1 cells at 2 to 48 h post infection (Figures 6A, B). The ΔmltA and transposon mutant strains failed to grow in THP-1 cell culture; their numbers decreased during this time period (Figures 6A, B). Complementation restored the capacity for intracellular growth during 2 to 24 h post infection. To explore the role of MltA in promoting cytotoxicity in human macrophages, THP-1 cells were infected with F. novicida strains and cytotoxicity was measured using the LDH release assay. Infection with the ΔmltA and transposon mutant strains showed less cytotoxicity than that with the wild-type and complemented strains (Figure 6C). These results suggest that MltA is essential for intracellular growth of F. novicida in human macrophages.
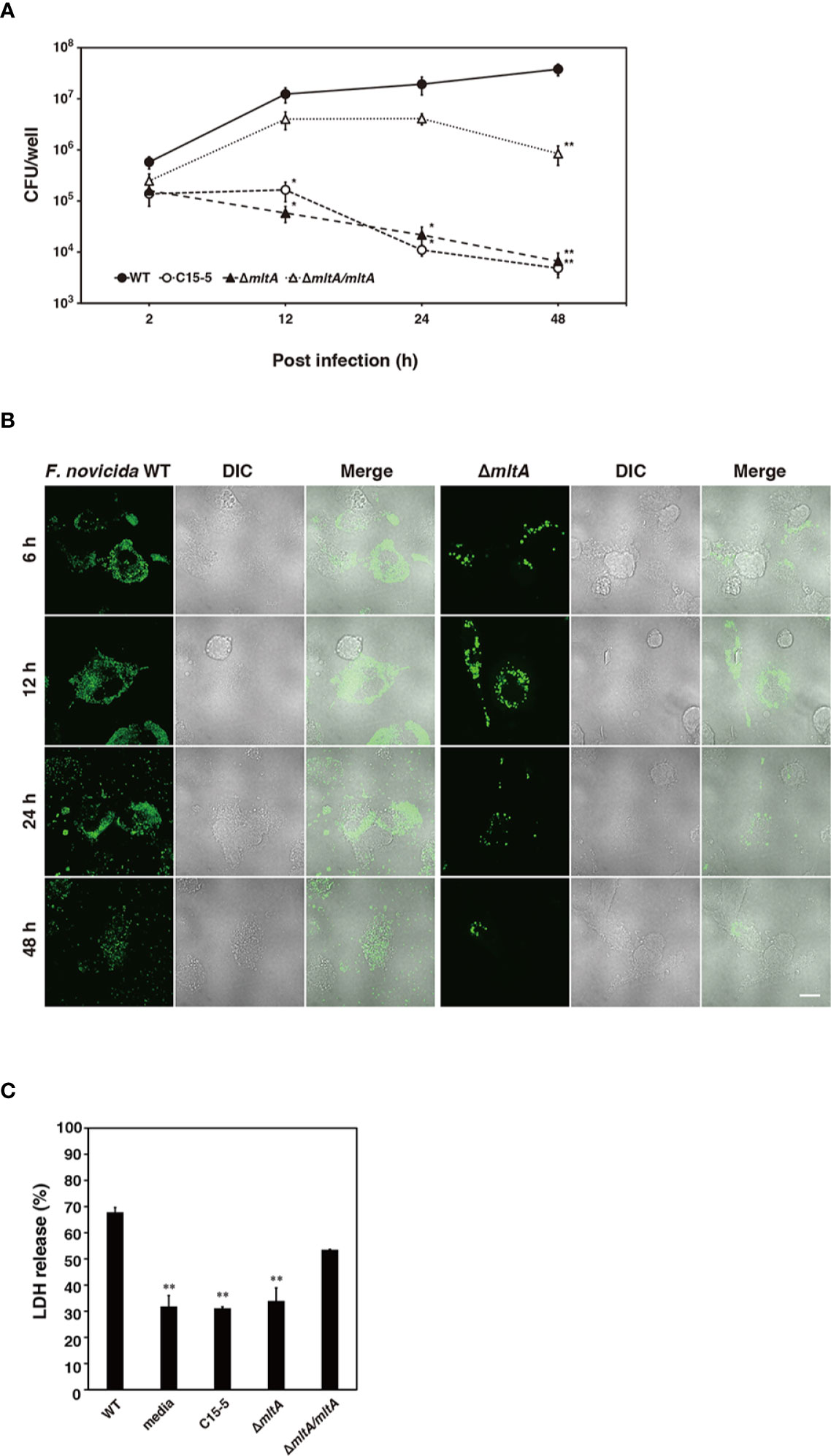
Figure 6 Impact of MltA on pathogenicity of F. novicida in a human macrophage cell line. (A) THP-1 cells were infected with the wild-type (WT), transposon mutant (C15-5), ΔmltA, or the complemented (ΔmltA/mltA) strains of F. novicida at multiplicity of infection (MOI) = 1 and treated 50 µg/ml gentamicin for 1 h. Cells were disrupted with 0.1% Triton X-100 and plated on BHIc agar at the times indicated. (B) THP-1 cells were infected with the green fluorescent protein (GFP)-expressing wild-type (WT) or ΔmltA strains of F. novicida at MOI = 1 and treated 50 µg/ml gentamicin for 1 h. Cells were fixed and evaluated by confocal laser scanning microscopy at the time points as indicated. Scale bar = 20 µm. (C) THP-1 cells were infected with the wild-type (WT), transposon mutant (C15-5), ΔmltA, or the complemented (ΔmltA/mltA) strains of F. novicida at MOI = 0.01 and treated 50 µg/ml gentamicin for 1 h. Cells were incubated for 48 h and cytotoxicity following infection was measured using the lactate dehydrogenase (LDH) assay. (A, C) The data represent the averages and standard error of three identical experiments. Differences from the wild type strain were evaluated via multiple comparisons and are indicated by asterisks; **P < 0.01, *P < 0.05.
Discussion
Francisella is transmitted to mammalian hosts by arthropod vectors (Petersen et al., 2009); as such, a careful analysis of the unique growth mechanisms in arthropods is important for our understanding of the full life cycle of Francisella. In this study, we identified a novel factor, which promotes growth and survival of F. novicida in arthropods using the silkworm infection model. We previously developed two distinct silkworm infection models. The first uses LVS. In this infection model, LVS was maintained and the infected silkworms survived, resulting in symbiotic relationship (Suzuki et al., 2016). The second features F. novicida; F. novicida proliferates within the silkworms and ultimately promotes their death (Saha et al., 2017). As bacterial symbiosis and growth within arthropods are considered to be related processes in the Francisella life cycle, symbiotic factors that predominate in LVS infection and growth factors associated with F. novicida infection may ultimately cooperate to promote survival of Francisella in arthropods. We identified herein critical factors underlying bacterial growth in the F. novicida–silkworm infection model; specifically, we identified seven distinct genes that were critical for F. novicida growth in silkworms by screening a transposon mutant library.
To date, arthropod infection models, such as wax moth (G. mellonella) and fruit fly (D. melanogaster), have been used to explore features of the Francisella–arthropod interaction (Aperis et al., 2007; Vonkavaara et al., 2008; Ahlund et al., 2010; Moule et al., 2010). Several of the aforementioned seven genes (iglB, slt) identified based on screening using the silkworm infection model include those previously identified in G. mellonella or D. melanogaster infection models (Ahlund et al., 2010; Vonkavaara et al., 2013). Several of these genes have already been reported to encode pathogenic factors related to Francisella infection in humans. Despite the previous screenings, MltA encoded by mltA had not been reported until now as a pathogenic factor in human infection or a bacterial growth factor in arthropods. Although many reports point to bacterial-derived lytic transglycosylases (LTs) as closely associated with pathogenicity (Koraimann, 2003), information about the function of LTs associated with Francisella infection in mammals or arthropods is limited (Bachert et al., 2019; Nakamura et al., 2019). Given these previous findings, we proceeded on the assumption that mltA might be involved in proliferation of F. novicida in arthropods and that this function might be revealed in our silkworm infection model.
To analyze functions of MltA in F. novicida, we generated a ΔmltA strain. Infection with the wild-type strain killed silkworms, while that with the ΔmltA or associated transposon mutant strains permitted longer-term survival. Intracellular growth of the ΔmltA and transposon mutant strains was reduced in lymphocyte and in the silkworm-derived cell line BmN4 when compared with growth of the wild-type and complemented strains. These results indicate that MltA is a critical factor and is required to support intracellular growth in silkworms. In addition, bacterial numbers of ΔmltA and transposon mutant strains in BmN4 cells at early stage of infection (2 h post infection) tended to be decreased compared with that of the wild-type and complemented strains, indicating that MltA of F. novicida may affect the invasion ability to silkworm cells.
LTs are known to be involved in cell division and membrane stability of other bacteria (Koraimann, 2003); as such, we considered the possibility that the ΔmltA and/or the transposon mutant strains might exhibit growth defects in the culture medium. Bobie et al. (Adu-Bobie et al., 2004) reported that a ΔmltA strain of Neisseria meningitidis exhibited delayed growth in vitro. In our study, we found that the ΔmltA strain showed similar morphology to the wild-type strain but grew at slightly decreased rates in liquid culture medium. Interestingly, the transposon mutant exhibited no growth delay, and mltA complementation failed to restore the growth rate. These data suggest that growth delay may be a structural result associated with the gene deletion. For example, the deletion of the mltA gene may have an impact on the expression of neighboring genes. Of note, although growth of ΔmltA strain was only slightly delayed in liquid culture, its growth was significantly inhibited in silkworms and in cultures of BmN4 and THP-1 cells. Likewise, while growth of the transposon mutant was similar to that of the wild-type strain in liquid culture, its growth in silkworms, BmN4 cells, and THP-1 cells was markedly reduced. Moreover, despite the slightly delayed growth of the complemented strain when evaluated in liquid culture, complementation restored the capacity for growth in silkworms, BmN4 cells, and THP-1 cells. From these results, we concluded that diminished proliferation of the ΔmltA strain in both silkworms and cell lines could not be attributed to factors promoting growth delay in liquid culture.
MltA is a membrane-bound lytic murein transglycosylase, which belongs to the LT enzyme family. LTs show same substrate specificity as the muramidases (lysozymes), and catalyze the cleavage of β-1,4-glycosidic bond between N-acetylglucosamine and N-acetylmuramic acid residues of PG, but resulted in the different formation of product with 1,6-anhydromuramyl residues (Holtje et al., 1975). LTs are lytic enzymes that target PG and promote appropriate cell division and membrane stability (Dik et al., 2017). LTs also create spaces within the PG to facilitate assembly of macromolecular complexes including flagella, pili, and secretion systems larger than the size of PG pores (Scheurwater et al., 2008). Specifically, LTs were associated with the activity of the type IV secretion system in both Brucella abortus and Helicobacter pylori (Rohde et al., 2003; Bao et al., 2017) and with the type VI secretion system of Escherichia coli (Santin and Cascales, 2017). Francisella spp. have a type VI secretion system, which plays an important role in promoting growth within arthropods; this secretion system was previously reported to be critical for intracellular growth in a mosquito cell line (Read et al., 2008). In addition, we previously showed that the type VI secretion system was directly involved in proliferation of Francisella within silkworms (Saha et al., 2017). In Acinetobacter, soluble lytic transglycosylase (SLT) is required in order to form type IV pili, which are associated with bacterial pathogenicity (Crepin et al., 2018). In F. novicida, peptidase and chitin-binding proteins are secreted from the type IV pili apparatus (Hager et al., 2006). These findings suggest that proteins secreted from type VI secretion system or type IV pili may contribute to the intracellular growth and/or to the immunosuppressive activity associated with F. novicida infection.
While we could not fully clarify the detailed mechanisms via which F. novicida MltA promotes growth and survival in silkworms, our results suggest a critical role played by its capacity to suppress host immune responses. Previous studies have reported that Francisella spp. are generally capable of suppressing host immune responses, thus enabling them to survive within the host (Jones et al., 2012). Our previous studies in silkworms showed that both F. novicida and LVS were capable of inhibiting host immune activity (Suzuki et al., 2016; Saha et al., 2017). Arthropods, including silkworms, possess Toll pathways to overcome the pathogen infection (Cheng et al., 2008; Kurata, 2014). Pathogen-associated molecular pattern molecules, including lipopolysaccharide (LPS) and PG, were captured by recognition proteins, resulting in immune responses that include expression of AMPs (Chen and Lu, 2018). Cecropin B is one of major AMPs effective against both Gram-positive and Gram-negative bacteria (Moore et al., 1996); cecropin B was shown to be effective against F. novicida in a D. melanogaster infection model (Vonkavaara et al., 2013). In this study, expression of cecropin B was suppressed during the early stages, at 6–12 h post infection with the wild-type strain. By contrast, although the bacterial number was diminished, the ΔmltA strain induced high levels of expression of cecropin B compared to that observed in response to infection with the wild-type strain. These results suggest that limited survival of the ΔmltA strain may be attributed to the fact that it does not suppress expression of AMPs in infected silkworms. MltA has been associated with PG remodeling, LPS synthesis, and the production of outer membrane vesicles (OMVs) in various bacterial species (Ferrari et al., 2006; Suvorov et al., 2008; Liu et al., 2012). In Neisseria gonorrhoeae, LTs plays an important role in escape of the bacterium from TLR recognition via control of OMVs production (Knilans et al., 2017). These findings in earlier works may suggest that MltA of Francisella modulates exposure of LPS, PG, and OMVs and thus avoids host recognition by modifying membrane structures.
In this study, MltA was identified as a growth factor in the silkworm infection model. MltA also contributed to intracellular growth and cytotoxicity in human macrophages. Among the seven genes identified as growth factors in silkworms, four genes encoding the major facilitator superfamily (MFS) transport protein, intracellular growth locus protein B, gamma-glutamyl transpeptidase, and SLT were previously identified as promoting pathogenicity in mammalian hosts (Bröms et al., 2010; Ireland et al., 2011; Balzano et al., 2018). These results suggest that many of the factors that promote growth and survival in silkworms may also be involved in intracellular proliferation in mammal cells. This finding is consistent with the suggestion made by Akimana and Kwaik (2011), specifically that the mechanisms associated with the Francisella pathogenicity may depend on systems that are common to or at least similar among arthropods and mammals. As such, we conclude that the F. novicida–silkworm infection model is useful as an infection model for mammals, including humans.
In conclusion, our research has highlighted the importance of MltA of F. novicida as a novel factor that promotes bacterial growth in silkworms and similarly contributes to pathogenicity in human cells. Because highly pathogenic F. tularensis subspecies possess mltA, it might be a potential target for the control of arthropod-mediated transmission of F. tularensis.
Data Availability Statement
The raw data supporting the conclusions of this article will be made available by the authors, without undue reservation.
Author Contributions
TN contributed to laboratory analysis, investigation, and writing original draft. TS contributed to conceptualization, laboratory analysis, investigation, and writing original draft. FI, SO, and SS contributed to laboratory analysis. AU contributed to methodology and resources. KW contributed to laboratory analysis, investigation, and validation. MW was involved in conceptualization, laboratory analysis, supervision, review, and editing. All authors contributed to the article and approved the submitted version.
Funding
This work was supported by JSPS KAKENHI Grant Number 15K08463, 17H03914, and 19K07556.
Conflict of Interest
The authors declare that the research was conducted in the absence of any commercial or financial relationships that could be construed as a potential conflict of interest.
Supplementary Material
The Supplementary Material for this article can be found online at: https://www.frontiersin.org/articles/10.3389/fcimb.2020.581864/full#supplementary-material
References
Adu-Bobie J., Lupetti P., Brunelli B., Granoff D., Norais N., Ferrari G., et al. (2004). GNA33 of Neisseria meningitidis is a lipoprotein required for cell separation, membrane architecture, and virulence. Infect. Immun. 72 (4), 1914–1919. doi: 10.1128/iai.72.4.1914-1919.2004
Ahlund M. K., Ryden P., Sjostedt A., Stoven S. (2010). Directed screen of Francisella novicida virulence determinants using Drosophila melanogaster. Infect. Immun. 78 (7), 3118–3128. doi: 10.1128/IAI.00146-10
Akimana C., Kwaik Y. A. (2011). Francisella-arthropod vector interaction and its role in patho-adaptation to infect mammals. Front. Microbiol. 2, 34. doi: 10.3389/fmicb.2011.00034
Aperis G., Fuchs B. B., Anderson C. A., Warner J. E., Calderwood S. B., Mylonakis E. (2007). Galleria mellonella as a model host to study infection by the Francisella tularensis live vaccine strain. Microbes Infect. 9 (6), 729–734. doi: 10.1016/j.micinf.2007.02.016
Bachert B. A., Biryukov S. S., Chua J., Rodriguez S. A., Toothman R. G. Jr., Cote C. K., et al. (2019). A Francisella novicida Mutant, Lacking the Soluble Lytic Transglycosylase Slt, Exhibits Defects in Both Growth and Virulence. Front. Microbiol. 10, 1343. doi: 10.3389/fmicb.2019.01343
Balzano P. M., Cunningham A. L., Grassel C., Barry E. M. (2018). Deletion of the Major Facilitator Superfamily Transporter fptB Alters Host Cell Interactions and Attenuates Virulence of Type A Francisella tularensis. Infect. Immun. 86 (3), e00832–17. doi: 10.1128/IAI.00832-17
Bao Y., Tian M., Li P., Liu J., Ding C., Yu S. (2017). Characterization of Brucella abortus mutant strain Delta22915, a potential vaccine candidate. Vet. Res. 48 (1), 17. doi: 10.1186/s13567-017-0422-9
Bröms J. E., Sjöstedt A., Lavander M. (2010). The role of the Francisella tularensis pathogenicity island in type VI secretion, intracellular survival, and modulation of host cell signaling. Front. Microbiol. 1, 136. doi: 10.3389/fmicb.2010.00136
Carvalho C. L., Lopes de Carvalho I., Ze-Ze L., Nuncio M. S., Duarte E. L. (2014). Tularaemia: a challenging zoonosis. Comp. Immunol. Microbiol. Infect. Dis. 37 (2), 85–96. doi: 10.1016/j.cimid.2014.01.002
Chen K., Lu Z. (2018). Immune responses to bacterial and fungal infections in the silkworm. Bombyx mori. Dev. Comp. Immunol. 83, 3–11. doi: 10.1016/j.dci.2017.12.024
Cheng T. C., Zhang Y. L., Liu C., Xu P. Z., Gao Z. H., Xia Q. Y., et al. (2008). Identification and analysis of Toll-related genes in the domesticated silkworm, Bombyx mori. Dev. Comp. Immunol. 32 (5), 464–475. doi: 10.1016/j.dci.2007.03.010
Crepin S., Ottosen E. N., Peters K., Smith S. N., Himpsl S. D., Vollmer W., et al. (2018). The lytic transglycosylase MltB connects membrane homeostasis and in vivo fitness of Acinetobacter baumannii. Mol. Microbiol. 109 (6), 745–762. doi: 10.1111/mmi.14000
Dennis D. T., Inglesby T. V., Henderson D. A., Bartlett J. G., Ascher M. S., Eitzen E., et al. (2001). Tularemia as a biological weapon: medical and public health management. JAMA 285 (21), 2763–2773. doi: 10.1001/jama.285.21.2763
Dik D. A., Marous D. R., Fisher J. F., Mobashery S. (2017). Lytic transglycosylases: concinnity in concision of the bacterial cell wall. Crit. Rev. Biochem. Mol. Biol. 52 (5), 503–542. doi: 10.1080/10409238.2017.1337705
Eklund B. E., Mahdi O., Huntley J. F., Collins E., Martin C., Horzempa J., et al. (2017). The orange spotted cockroach (Blaptica dubia, Serville 1839) is a permissive experimental host for Francisella tularensis. Proc. W. Va. Acad. Sci. 89 (3), 34–47.
Ellis J., Oyston P. C., Green M., Titball R. W. (2002). Tularemia. Clin. Microbiol. Rev. 15 (4), 631–646. doi: 10.1128/CMR.15.4.631-646.2002
Ferrari G., Garaguso I., Adu-Bobie J., Doro F., Taddei A. R., Biolchi A., et al. (2006). Outer membrane vesicles from group B Neisseria meningitidis delta gna33 mutant: proteomic and immunological comparison with detergent-derived outer membrane vesicles. Proteomics 6 (6), 1856–1866. doi: 10.1002/pmic.200500164
Grace T. D. (1967). Establishment of a line of cells from the silkworm Bombyx mori. Nature 216 (5115), 613. doi: 10.1038/216613a0
Hager A. J., Bolton D. L., Pelletier M. R., Brittnacher M. J., Gallagher L. A., Kaul R., et al. (2006). Type IV pili-mediated secretion modulates Francisella virulence. Mol. Microbiol. 62 (1), 227–237. doi: 10.1111/j.1365-2958.2006.05365.x
Holtje J. V., Mirelman D., Sharon N., Schwarz U. (1975). Novel type of murein transglycosylase in Escherichia coli. J. Bacteriol. 124 (3), 1067–1076. doi: 10.1128/JB.124.3.1067-1076.1975
Ireland P. M., LeButt H., Thomas R. M., Oyston P. C. (2011). A Francisella tularensis SCHU S4 mutant deficient in gamma-glutamyltransferase activity induces protective immunity: characterization of an attenuated vaccine candidate. Microbiology 157 (Pt 11), 3172–3179. doi: 10.1099/mic.0.052902-0
Jiang L., Peng Z., Guo Y., Cheng T., Guo H., Sun Q., et al. (2016). Transcriptome analysis of interactions between silkworm and cytoplasmic polyhedrosis virus. Sci. Rep. 6, 24894. doi: 10.1038/srep24894
Jones C. L., Napier B. A., Sampson T. R., Llewellyn A. C., Schroeder M. R., Weiss D. S. (2012). Subversion of host recognition and defense systems by Francisella spp. Microbiol. Mol. Biol. Rev. 76 (2), 383–404. doi: 10.1128/MMBR.05027-11
Kaito C., Akimitsu N., Watanabe H., Sekimizu K. (2002). Silkworm larvae as an animal model of bacterial infection pathogenic to humans. Microb. Pathog. 32 (4), 183–190. doi: 10.1006/mpat.2002.0494
Kaito C., Usui K., Kyuma T., Sekimizu K. (2011). Isolation of mammalian pathogenic bacteria using silkworms. Drug Discovery Ther. 5 (2), 66–70. doi: 10.5582/ddt.2011.v5.2.66
Kaito C. (2016). Understanding of bacterial virulence using the silkworm infection model. Drug Discovery Ther. 10 (1), 30–33. doi: 10.5582/ddt.2016.01020
Keim P., Johansson A., Wagner D. M. (2007). Molecular epidemiology, evolution, and ecology of Francisella. Ann. N. Y. Acad. Sci. 1105, 30–66. doi: 10.1196/annals.1409.011
Kingry L. C., Petersen J. M. (2014). Comparative review of Francisella tularensis and Francisella novicida. Front. Cell Infect. Microbiol. 4, 35. doi: 10.3389/fcimb.2014.00035
Knilans K. J., Hackett K. T., Anderson J. E., Weng C., Dillard J. P., Duncan J. A. (2017). Neisseria gonorrhoeae Lytic Transglycosylases LtgA and LtgD Reduce Host Innate Immune Signaling through TLR2 and NOD2. ACS Infect. Dis. 3 (9), 624–633. doi: 10.1021/acsinfecdis.6b00088
Koraimann G. (2003). Lytic transglycosylases in macromolecular transport systems of Gram-negative bacteria. Cell Mol. Life Sci. 60 (11), 2371–2388. doi: 10.1007/s00018-003-3056-1
Kurata S. (2014). Peptidoglycan recognition proteins in Drosophila immunity. Dev. Comp. Immunol. 42 (1), 36–41. doi: 10.1016/j.dci.2013.06.006
Liu W., Dong N., Zhang X. H. (2012). Overexpression of mltA in Edwardsiella tarda reduces resistance to antibiotics and enhances lethality in zebra fish. J. Appl. Microbiol. 112 (6), 1075–1085. doi: 10.1111/j.1365-2672.2012.05291.x
Maurin M. (2015). Francisella tularensis as a potential agent of bioterrorism? Expert Rev. Anti Infect. Ther. 13 (2), 141–144. doi: 10.1586/14787210.2015.986463
Mc Gann P., Rozak D. A., Nikolich M. P., Bowden R. A., Lindler L. E., Wolcott M. J., et al. (2010). A novel brain heart infusion broth supports the study of common Francisella tularensis serotypes. J. Microbiol. Methods 80 (2), 164–171. doi: 10.1016/j.mimet.2009.12.005
McLendon M. K., Apicella M. A., Allen L. A. (2006). Francisella tularensis: taxonomy, genetics, and Immunopathogenesis of a potential agent of biowarfare. Annu. Rev. Microbiol. 60, 167–185. doi: 10.1146/annurev.micro.60.080805.142126
Moore A. J., Beazley W. D., Bibby M. C., Devine D. A. (1996). Antimicrobial activity of cecropins. J. Antimicrob. Chemother. 37 (6), 1077–1089. doi: 10.1093/jac/37.6.1077
Moule M. G., Monack D. M., Schneider D. S. (2010). Reciprocal analysis of Francisella novicida infections of a Drosophila melanogaster model reveal host-pathogen conflicts mediated by reactive oxygen and imd-regulated innate immune response. PLoS Pathog. 6 (8), e1001065. doi: 10.1371/journal.ppat.1001065
Nagle S. C. Jr., Anderson R. E., Gary N. D. (1960). Chemically defined medium for the growth of Pasteurella tularensis. J. Bacteriol. 79, 566–571. doi: 10.1128/JB.79.4.566-571.1960
Nakamura T., Shimizu T., Uda A., Watanabe K., Watarai M. (2019). Soluble lytic transglycosylase SLT of Francisella novicida is involved in intracellular growth and immune suppression. PLoS One 14 (12), e0226778. doi: 10.1371/journal.pone.0226778
Ozanic M., Marecic V., Abu Kwaik Y., Santic M. (2015). The Divergent Intracellular Lifestyle of Francisella tularensis in Evolutionarily Distinct Host Cells. PLoS Pathog. 11 (12), e1005208. doi: 10.1371/journal.ppat.1005208
Petersen J. M., Mead P. S., Schriefer M. E. (2009). Francisella tularensis: an arthropod-borne pathogen. Vet. Res. 40 (2), 7. doi: 10.1051/vetres:2008045
Putzova D., Panda S., Hartlova A., Stulik J., Gekara N. O. (2017). Subversion of innate immune responses by Francisella involves the disruption of TRAF3 and TRAF6 signalling complexes. Cell Microbiol. 19 (11), e12769. doi: 10.1111/cmi.12769
Read A., Vogl S. J., Hueffer K., Gallagher L. A., Happ G. M. (2008). Francisella genes required for replication in mosquito cells. J. Med. Entomol. 45 (6), 1108–1116. doi: 10.1603/0022-2585(2008)45[1108:fgrfri]2.0.co;2
Rodriguez S. A., Yu J. J., Davis G., Arulanandam B. P., Klose K. E. (2008). Targeted inactivation of Francisella tularensis genes by group II introns. Appl. Environ. Microbiol. 74 (9), 2619–2626. doi: 10.1128/AEM.02905-07
Rohde M., Puls J., Buhrdorf R., Fischer W., Haas R. (2003). A novel sheathed surface organelle of the Helicobacter pylori cag type IV secretion system. Mol. Microbiol. 49 (1), 219–234. doi: 10.1046/j.1365-2958.2003.03549.x
Saha S. S., Suzuki J., Uda A., Watanabe K., Shimizu T., Watarai M. (2017). Silkworm model for Francisella novicida infection. Microb. Pathog. 113, 94–101. doi: 10.1016/j.micpath.2017.10.036
Santin Y. G., Cascales E. (2017). Domestication of a housekeeping transglycosylase for assembly of a Type VI secretion system. EMBO Rep. 18 (1), 138–149. doi: 10.15252/embr.201643206
Scheurwater E., Reid C. W., Clarke A. J. (2008). Lytic transglycosylases: bacterial space-making autolysins. Int. J. Biochem. Cell Biol. 40 (4), 586–591. doi: 10.1016/j.biocel.2007.03.018
Shimizu T., Otonari S., Suzuki J., Uda A., Watanabe K., Watarai M. (2019). Expression of Francisella pathogenicity island protein intracellular growth locus E (IglE) in mammalian cells is involved in intracellular trafficking, possibly through microtubule organizing center. Microbiologyopen 8 (4), e00684. doi: 10.1002/mbo3.684
Suvorov M., Lee M., Hesek D., Boggess B., Mobashery S. (2008). Lytic transglycosylase MltB of Escherichia coli and its role in recycling of peptidoglycan strands of bacterial cell wall. J. Am. Chem. Soc. 130 (36), 11878–11879. doi: 10.1021/ja805482b
Suzuki J., Uda A., Watanabe K., Shimizu T., Watarai M. (2016). Symbiosis with Francisella tularensis provides resistance to pathogens in the silkworm. Sci. Rep. 6, 31476. doi: 10.1038/srep31476
Uchida R., Namiguchi S., Ishijima H., Tomoda H. (2016). Therapeutic effects of three trichothecenes in the silkworm infection assay with Candida albicans. Drug Discovery Ther. 10 (1), 44–48. doi: 10.5582/ddt.2016.01013
Vojtech L. N., Sanders G. E., Conway C., Ostland V., Hansen J. D. (2009). Host immune response and acute disease in a zebrafish model of Francisella pathogenesis. Infect. Immun. 77 (2), 914–925. doi: 10.1128/IAI.01201-08
Vonkavaara M., Telepnev M. V., Ryden P., Sjostedt A., Stoven S. (2008). Drosophila melanogaster as a model for elucidating the pathogenicity of Francisella tularensis. Cell Microbiol. 10 (6), 1327–1338. doi: 10.1111/j.1462-5822.2008.01129.x
Keywords: Francisella, tularemia, silkworm, transglycosylase, infection model
Citation: Nakamura T, Shimizu T, Inagaki F, Okazaki S, Saha SS, Uda A, Watanabe K and Watarai M (2021) Identification of Membrane-Bound Lytic Murein Transglycosylase A (MltA) as a Growth Factor for Francisella novicida in a Silkworm Infection Model. Front. Cell. Infect. Microbiol. 10:581864. doi: 10.3389/fcimb.2020.581864
Received: 13 July 2020; Accepted: 04 December 2020;
Published: 22 January 2021.
Edited by:
Martin John McGavin, Western University, CanadaReviewed by:
Erin C. Garcia, University of Kentucky, United StatesAnthony J. Clarke, University of Guelph, Canada
Copyright © 2021 Nakamura, Shimizu, Inagaki, Okazaki, Saha, Uda, Watanabe and Watarai. This is an open-access article distributed under the terms of the Creative Commons Attribution License (CC BY). The use, distribution or reproduction in other forums is permitted, provided the original author(s) and the copyright owner(s) are credited and that the original publication in this journal is cited, in accordance with accepted academic practice. No use, distribution or reproduction is permitted which does not comply with these terms.
*Correspondence: Masahisa Watarai, d2F0YXJhaUB5YW1hZ3VjaGktdS5hYy5qcA==
†These authors have contributed equally to this work