- Institut Universitaire de Cardiologie et de Pneumologie de Québec, Université Laval, QC, Canada
Lung dendritic cells (DCs) are divided into two major populations, which include CD103+XCR1+ cDC1s and CD11b+Sirpα+ cDC2s. The maintenance of their relative proportions is dynamic and lung inflammation, such as caused by exposure to lipopolysaccharide (LPS), a component of the outer membrane of Gram-negative bacteria, can have a significant impact on the local cDC signature. Alterations in the lung cDC signature could modify the capacity of the immune system to respond to various pathogens. We consequently aimed to assess the impact of the Gram-negative bacteria Pseudomonas aeruginosa on lung cDC1 and cDC2 populations, and to identify the mechanisms leading to alterations in cDC populations. We observed that exposure to P. aeruginosa decreased the proportions of CD103+XCR1+ cDC1s, while increasing that of CD11b+ DCs. We identified two potential mechanisms involved in this modulation of lung cDC populations. First, we observed an increase in bone marrow pre-DC IRF4 expression suggesting a higher propensity of pre-DCs to differentiate towards the cDC2 lineage. This observation was combined with a reduced capacity of lung XCR1+ DC1s to express CD103. In vitro, we demonstrated that GM-CSF-induced CD103 expression on cDCs depends on GM-CSF receptor internalization and RUNX1 activity. Furthermore, we observed that cDCs stimulation with LPS or P. aeruginosa reduced the proportions of intracellular GM-CSF receptor and decreased RUNX1 mRNA expression. Altogether, these results suggest that alterations in GM-CSF receptor intracellular localization and RUNX1 signaling could be involved in the reduced CD103 expression on cDC1 in response to P. aeruginosa. To verify whether the capacity of cDCs to express CD103 following P. aeruginosa exposure impacts the immune response, WT and Cd103-/- mice were exposed to P. aeruginosa. Lack of CD103 expression led to an increase in the number of neutrophils in the airways, suggesting that lack of CD103 expression on cDC1s could favor the innate immune response to this bacterium.
Introduction
Conventional dendritic cells (cDCs) play an important role in both innate and adaptive immune responses. In the lungs, cDCs are critical sentinel cells that capture, process and present antigens to activate naive T cells in lymph nodes. In addition, cDCs are involved in the innate immune response via cytokine and chemokine production (Macri et al., 2018). Lung conventional DCs comprise a variety of subsets that are typically subdivided into two sub-populations named cDC1s and cDC2s (Guilliams et al., 2014; Guilliams et al., 2016). cDC1s express the surface marker XCR1, and the expression of IRF8 and BATF3 transcription factors is required for their development. Additionally, they can express the alpha-E integrin CD103 in non-lymphoid organs like the lung, while they express CD8α in lymphoid tissues. cDC2s are characterized by IRF4, Sirpα and CD11b expression (Crozat et al., 2011; Guilliams et al., 2014; Gurka et al., 2015).
The majority of cDC development occurs in the bone marrow and requires the presence of the FMS-like tyrosine kinase 3 ligand (FLT3L) cytokine (Ginhoux et al., 2009). During this process, the commitment of cDC precursors to the cDC1 or cDC2 lineage happens relatively early in cDC development (Grajales-Reyes et al., 2015; Schlitzer et al., 2015). cDC precursors then leave the bone marrow at the pre-DC stage and migrate through the bloodstream to various organs such as the lung (Liu et al., 2009). Pre-DCs committed to the cDC1 lineage do not express CD103 (Brassard et al., 2019). The exact mechanisms by which cDC1s acquire CD103 expression upon their entrance in the lung remain unclear, but in vivo and in vitro studies suggest that exposure to GM-CSF, present in the lung, is a potent inducer of cDC CD103 expression (King et al., 2010; Greter et al., 2012; Mayer et al., 2014). To date, there is no information concerning transcription factors involved in cDC CD103 expression. However, the RUNX family of transcription factors is involved in the induction of CD103 expression in T cells (Grueter et al., 2005). The only reported ligand of αE integrin (CD103) is E-cadherin, which is expressed by epithelial cells (Corps et al., 2001). While the role of CD103 expression on cDC1s remains unclear, reports demonstrate that T cells CD103 expression facilitates lymphocyte localization and induces intracellular signaling (Pauls et al., 2001; Franciszkiewicz et al., 2013).
The two cDC subpopulations have distinct and often opposite functions (Schlitzer et al., 2015; Guilliams et al., 2016). cDC1s are particularly important for IL-12 production, antigen cross-presentation to CD8 T cells and CD4 T cell polarization into TH1 (Hildner et al., 2008; Mashayekhi et al., 2011; Martínez-López et al., 2015). The specific function of cDC2s is more controversial, but some studies suggested that they are important for TH2 polarization (Gao et al., 2013; Plantinga et al., 2013). To date, there is no consensus regarding the roles of cDC1s or cDC2s in the efficacy of antibacterial immune responses. Nevertheless, some interesting data suggest that, as observed in several other types of immune responses, cDC1s and cDC2s elicit distinctive functions in the fight against bacteria. Indeed, in most bacterial infections, polarization of naive T cells into TH1 improves bacterial clearance and lung function, which suggests a beneficial role for cDC1s (Moser et al., 1997; Moser et al., 2002). In accordance, in a mouse model of lung infection with the Gram-negative bacteria Chlamydia muridarum, CD103+ cDC1s induced a stronger TH1 polarization compared to cDC2s, and CD103+ cDC1 injection improved bacterial clearance (Shekhar et al., 2018). It should be noted, however, that cDC1s are usually involved in infections to intracellular bacteria, and that their role in response to Pseudomonas aeruginosa is not well-described. cDC2s, on the other hand, could be important for the innate immune response. Indeed, in response to LPS lung exposure, lung CD11b+ DCs produce more KC (CXCL1) and MIP–2 (CXCL2), two chemokines involved in neutrophil recruitment, compared to CD103+ cDC1s (Beaty et al., 2007).
These distinctive roles for cDC1s and cDC2s in the response to bacteria suggest that the tight balance between cDC lung subsets is important to support effective local immune responses. Recently, we demonstrated that lung exposure to lipopolysaccharide (LPS), which induces a strong local and peripheral inflammatory response, modulates the proportions of cDC populations by decreasing the percentage of CD103+ cDC1s and increasing CD11b+ DC proportions (Brassard et al., 2019). Since LPS is one of multiple bacterial components that may influence cDC populations and with the potential crucial role of cDC1s in the fight against bacterial infections in the lung, the impact of whole bacteria on the lung cDC signature remained an important unanswered question. We therefore set out to analyze the influence of an acute exposure to the Gram–negative bacteria P. aeruginosa on the local lung cDC signature. P. aeruginosa is ubiquitously found in nature and causes opportunistic acute and chronic infections in immunocompromised patients, such as those suffering of cystic fibrosis (Green et al., 1974; Lyczak et al., 2000; Moradali et al., 2017). We observed that P. aeruginosa modulated the proportions of lung cDC1 and cDC2 populations in favor of cDC2s, which was in part explained by a higher propensity of bone marrow cDC precursors to differentiate towards the cDC2 lineage, and by an incapacity of lung cDC1s to fully express CD103 in response to GM-CSF. The latter was linked to reduced GM–CSF receptor internal localization, following exposure to P. aeruginosa and LPS, and alterations in RUNX1 expression, which regulate CD103 expression. Finally, we report that the lack of CD103 expression on cDCs leads to an exacerbated airways neutrophilia, supporting the idea that the absence of CD103 expression on cDC1s promotes the lung innate response to P. aeruginosa. We therefore shed a light on a possible mechanism demonstrating that the blockade of cDC CD103 expression by Gram-negative bacteria is a crucial step in promoting the initial innate immune response to this potentially infectious agent in the lung.
Material and Methods
Mice
Cd103−/− (B6.129S2(C)-Itgaetm1Cmp/J) and wild-type (WT) mice were purchased from Jackson Laboratories and kept in a specific pathogen-free animal unit (Centre de recherche de l’Institut Universitaire de Cardiologie et de Pneumologie de Québec, Laval University, Québec, QC, Canada) for the duration of the experiments. Cd103−/− and WT mice were not co-housed during the duration of experiments. Experiments were approved by local ethics committees and followed Canadian animal care guidelines.
Intranasal Instillation With P. aeruginosa and LPS
Non-mucoid P. aeruginosa, strain Boston 41501 (ATCC #27853, Manassas, VA, USA) was incubated overnight in tryptic soy broth (TSB) (Wisent, St-Bruno, QC, CA) at 37°C in a rotating shaker and 1 ml of the suspension was re-incubated in new TSB media for 2 h. Bacteria were washed and diluted in saline, and the desired concentration was adjusted by spectrophotometry according to a reference curve. Bacterial concentration was systematically verified by quantitative culture of the inoculum. Age- and sex-matched WT and Cd103-/- mice received a 50 μL intranasal (i.n.) instillation of 5 x 105 or 5 x 106 colony forming units (CFU) of P. aeruginosa or 350 ng of LPS (Sigma-Aldrich, St. Louis, MO USA). Mice were euthanized at 2, 6 or 18 h following LPS or P. aeruginosa exposure. Bronchoalveolar lavages (BAL) were obtained via three injections/aspirations of 1 mL of saline, in mice euthanized at 2 or 6 h post i.n. Total BAL cells of LPS-treated mice were counted and differential counts were determined on Giemsa stained cytospins (HemaStain Set, Thermo Fisher Scientific, Waltham, MI, USA). The BAL composition of P. aeruginosa-exposed mice was analyzed by flow cytometry and neutrophils were identified as auto-fluorescence-, CD45+, Ly-6G+ and CD11b+ and macrophages were identified as auto-fluorescence+, CD45+, CD11c+ and Siglec-F+. For flow cytometry analysis, the lung, spleen, femur and tibia were collected in phosphate buffered saline (PBS) 18 h after lung i.n. instillation with P. aeruginosa.
Leukocyte Isolation
Lung leukocytes were obtained by the digestion of lung tissue with 200 U/ml collagenase IV (Sigma-Aldrich) for 45 min at 37°C. Digested lungs and spleens were pressed through a 70-μm cell strainer. Bone marrow cells were isolated by flushing the cells from tibias and femurs using a 25 Gauge needle with PBS. Red blood cells were lysed with ammonium chloride and cDC or cDC precursors were analyzed by flow cytometry.
FLT3L-BMDCs
Bone marrow cells were isolated as described in the leukocyte isolation section. Cells were cultured at 1.5 x 106 cells/ml for 7 days in RPMI 1640 media (Wisent) supplemented with 10% FBS (Wisent), 50 µM β-mercaptoethanol, antibiotic-antimycotic (Wisent) and 100 ng/ml FMS-like tyrosine kinase 3 ligand (FLT3L) (peprotech, Rocky Hill, NJ, USA, catalog no. 250-31L). On day 7, BMDCs were harvested for stimulation.
Spleen-Isolated cDCs
To expand cDC populations in vivo, WT mice were subcutaneously injected in the lower back with 5 x 105 FLT3L-producing B16 melanoma cells, previously grown in DMEM media (Wisent) supplemented with 10% FBS. When the tumor reached 1 cm diameter, mice were euthanized and the spleen collected. Spleen leukocytes were isolated as described in leukocyte isolation section. cDCs were purified by negative selection using the EasySep Mouse pan-DC Enrichment Kit (StemCell Technologies, Vancouver, BC, Canada).
DCs In Vitro Stimulation
106 cells/ml of splenic or FLT3L-BMDCs were stimulated with 10 ng/ml Granulocyte-macrophage colony-stimulating factor (GM-CSF) (Peprotech, catalog no. 315-03), 10 ng/ml LPS (Sigma-Aldrich) or P. aeruginosa at a ratio of 1 cDC: 1 P. aeruginosa for spleen-isolated cDC or 10 DCs: 1 P. aeruginosa for FLT3L-BMDCs in RPMI 1640 supplemented with 10% FBS and 50 µM β-mercaptoethanol for 12, 18 or 48 h. For some experiments, FLT3L-BMDCs were pre-treated with 40, 80 or 120 μM of the dynamin inhibitor Dynasore (Sigma-Aldrich) or with 10, 25 or 50 μM of CBFβ-Runx1 Inhibitor II (Sigma-Aldrich). Following stimulation, CD103, GM-CSFRα and RUNX1 protein or mRNA expression were analyzed by flow cytometry and qRT-PCR respectively.
Flow Cytometry
BAL leukocytes, tissue-isolated leukocytes or in vitro-stimulated cDCs were stained with TruStain FcX anti-mouse CD16/32 antibody (BioLegend, San Diego, CA, USA) and CD45-APC-Cy7, CD103-PE, CD11b-PeCy7, CD11c-BV711, I-A/I-E (MHC II)-Pacific Blue, I-A/I-E (MHC II)-PERCP, CD172a (Sirpα)-APC-Cy7, CD19-biotin, CD90.2-biotin, IRF4-PE, Ly-6G-PE, XCR1-APC, CD8α-APC-Cy7, Lineage antibody cocktail-Pacific Blue, CD135 (FLT3)-biotin, Streptavidin-PERCP (BioLegend), NK1.1-biotin (ablab, Vancouver, BC, CA), CD11c-APC, Siglec-F-BV711 (BD Biosciences, San Jose, USA), IRF8-APC (Miltenyi Biotec, Bergisch Gladbach, Allemagne) and Streptavidin-Pe-Cy7 (eBioscience, Thermo Fisher Scientific), GM–CSFRα-APC (R&D system, Minneapolis, MN, USA). Total, neutrophils and macrophages BAL number were determined using precision count beads (BioLegend). Intracellular staining was performed using the True-Nuclear™ Transcription Factor Buffer Set (BioLegend) according to the manufacturer’s instructions. Cells were analyzed using a BD LSR Fortessa cytometer (BD Biosciences) and FlowJo software V10 (BD, Franklin Lakes, NJ, USA). Mean fluorescence intensity (MFI) data were analyzed as Δ MFI, which corresponds to the MFI of the antigen-positive population minus the MFI of the fluorescence minus one (FMO) control of this population.
Real-Time PCR Analysis
1.5 x 106 FLT3L-BMDCs were stimulated with GM-CSF, LPS or P. aeruginosa for 12 h and RNA was isolated using RNAspin Mini Kit (GE Healthcare Life Sciences, Chicago, USA) and reverse transcribed with an iScript cDNA Synthesis Kit (Bio-rad, Mississauga, Ontario, CA). Real-time PCR analysis was performed for CD103 (Itgae), GM-CSFRα (Csf2ra) and RUNX1 using the Rotor-Gene 6000TM (Qiagen, Valencia, CA, USA) in Sso Advanced Universal SYBR Green Supermix (Bio-rad). The following primers (IDT, Coralville, USA) were used: Itgae (forward: 5’-AGGTCATAGATACGGTCAGGT-3’, reverse: 5’-GGTTAGATTTCAATGGCGATGG-3’), GM-CSFRα (forward: 5’-CCTCACCATCCATCGCA-3’, reverse: 5’-GAAGCAGTAGCGTGGAGAAG -3’), RUNX1 (forward: 5’-GTAGCGAGATTCAACGACCTC-3’, reverse: 5’-TCTATGGTAGGTGGCAACTTG-3’). Expression was normalized to the Gnb and Rplp0 mRNA expression validated for stability of expression in this model.
Enumeration of Colony Forming Unit in BAL and Lung
Lungs were homogenized in 1 ml of saline using the Polytron Tissue Homogenizer (Kinematica, Luzern, Switzerland). Homogenates and BALs were subjected to 10-fold serial dilutions in saline and cultured in tryptic soy agar (Wisent) at 37°C and CFUs were counted 18 to 24 h later.
Statistics
Data are presented as mean ± SEM. Graphpad Prism version 8 (San Diego, CA, USA) was used to analyze all data. Statistical analysis for multiple comparisons was performed using an ANOVA table followed by Tukey’s multiple comparison tests. Non-multiple comparisons were analyzed using paired or unpaired T-tests. Statistical significance was determined at p < 0.05.
Results
Lung Exposure to the Gram-Negative Bacteria Pseudomonas aeruginosa Leads to a Major Modulation of DC Populations
We first aimed to verify whether lung exposure to whole Gram-negative bacteria influences the CD103+ cDC1 and cDC2 population ratios in the lung. To test this, lung cDC populations were analyzed 18 h following i.n. exposure with P. aeruginosa. Lung cDCs were identified as auto-fluorescence-, CD19-, CD90.2-, NK1.1-, MHC IIHi and CD11c+. CD103+ cDC1 characterization was based on CD103 and XCR1 expression, whereas CD11b and Sirpα markers were used to identify cDC2s (Figure 1A and Supplementary Figure 1 for full gating strategy). We first observed that P. aeruginosa induced an important increase in lung total cells, which was accompanied by a two-fold increase in cDC numbers (Figure 1B). We also report a decrease in CD103+XCR1+ cDC1 proportions and, in return, an increase in CD11b+Sirpα+ cDC2 proportions (Figure 1C). These results indicate that, as observed previously with LPS (Brassard et al., 2019), P. aeruginosa modulates lung cDC populations in favor of the DC2/monocyte-derived DC population.
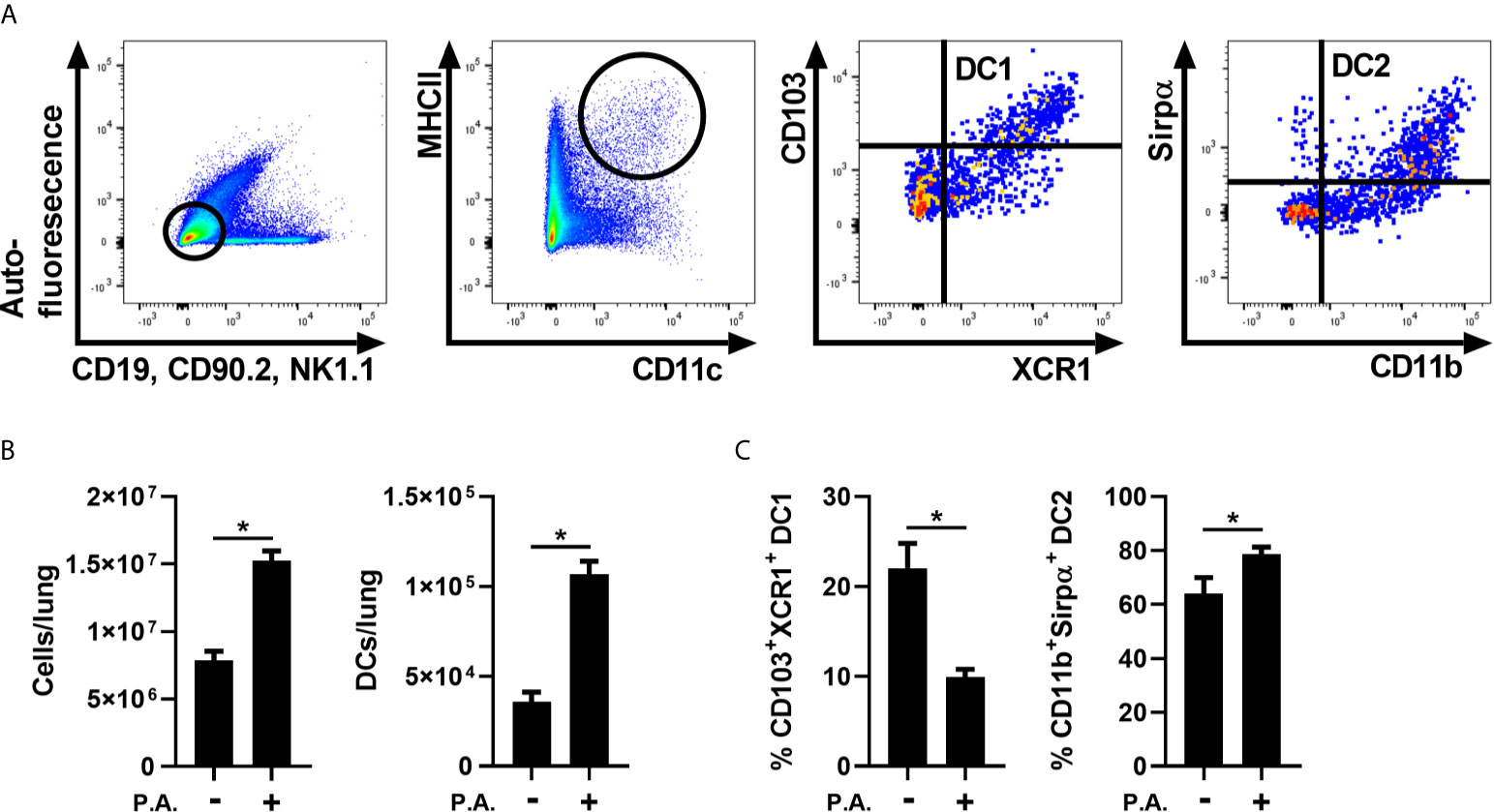
Figure 1 Lung exposure to P. aeruginosa altered the proportions of cDC1s and cDC2s in favor of cDC2s. WT mice were exposed to a single i.n. instillation of 5 x 105 CFU of P. aeruginosa (P.A.) and mice were euthanized 18 h later. Lung cDC populations were analyzed by flow cytometry. (A) Sequential gating strategy used to identify total cDCs (auto-fluorescence-, CD19-, CD90.2-, NK1.1-, MHC IIHi and CD11c+), cDC1 (CD103+XCR1+) and cDC2 (CD11b+Sirpα+). (B) Number of total lung cells and cDCs (C) Percentage of CD103+XCR1+ cDC1 and CD11b+Sirpα+ cDC2 in lung cDCs. Data are presented as mean ± SEM; n = 8-9 mice per group combined from two separate experiments *p < 0.05 using unpaired t-test.
Lung Exposure to Pseudomonas aeruginosa Influences Bone Marrow cDC Precursors
The commitment to the cDC1 or cDC2 lineage is defined before the pre-DCs stage, and can be influenced by peripheral inflammation (Schlitzer et al., 2015; Grajales-Reyes et al., 2015; Meyer et al., 2018; Beshara et al., 2018; Brassard et al., 2019). Thus, we hypothesized that following i.n. instillation with P. aeruginosa, a shift towards the cDC2 fate could support the accumulation of cDC2s in the lung. cDC1 and cDC2-committed precursors both express IRF8 initially, but the further commitment to the cDC2 lineage results in a decrease in IRF8 expression in time. In contrast, only cDC2-committed precursors express IRF4 at the later stage of development (Sichien et al., 2016). To determine whether lung exposure to P. aeruginosa alters the cDC1 vs cDC2 commitment, the expression of these two transcription factors was analyzed in bone marrow pre-DCs (Guilliams et al., 2014; Grajales-Reyes et al., 2015). Pre-DCs were identified as lineage-, MHC II-, CD11c+, Sirpα-/lo, CD135 (FLT3)+ (Figure 2A and Supplementary Figure 2) (Schlitzer et al., 2015; Grajales-Reyes et al., 2015). We report that the percentage of pre–DCs from total bone marrow cells and lineage- cells was significantly decreased in mice exposed to P. aeruginosa (Figure 2B). However, this was accompanied by an increase in IRF4 expression following lung instillation with P. aeruginosa (Figure 2C). This result suggests a propensity of pre-DCs to differentiate towards the cDC2 lineage and a strong exodus of pre-DCs in response to P. aeruginosa.
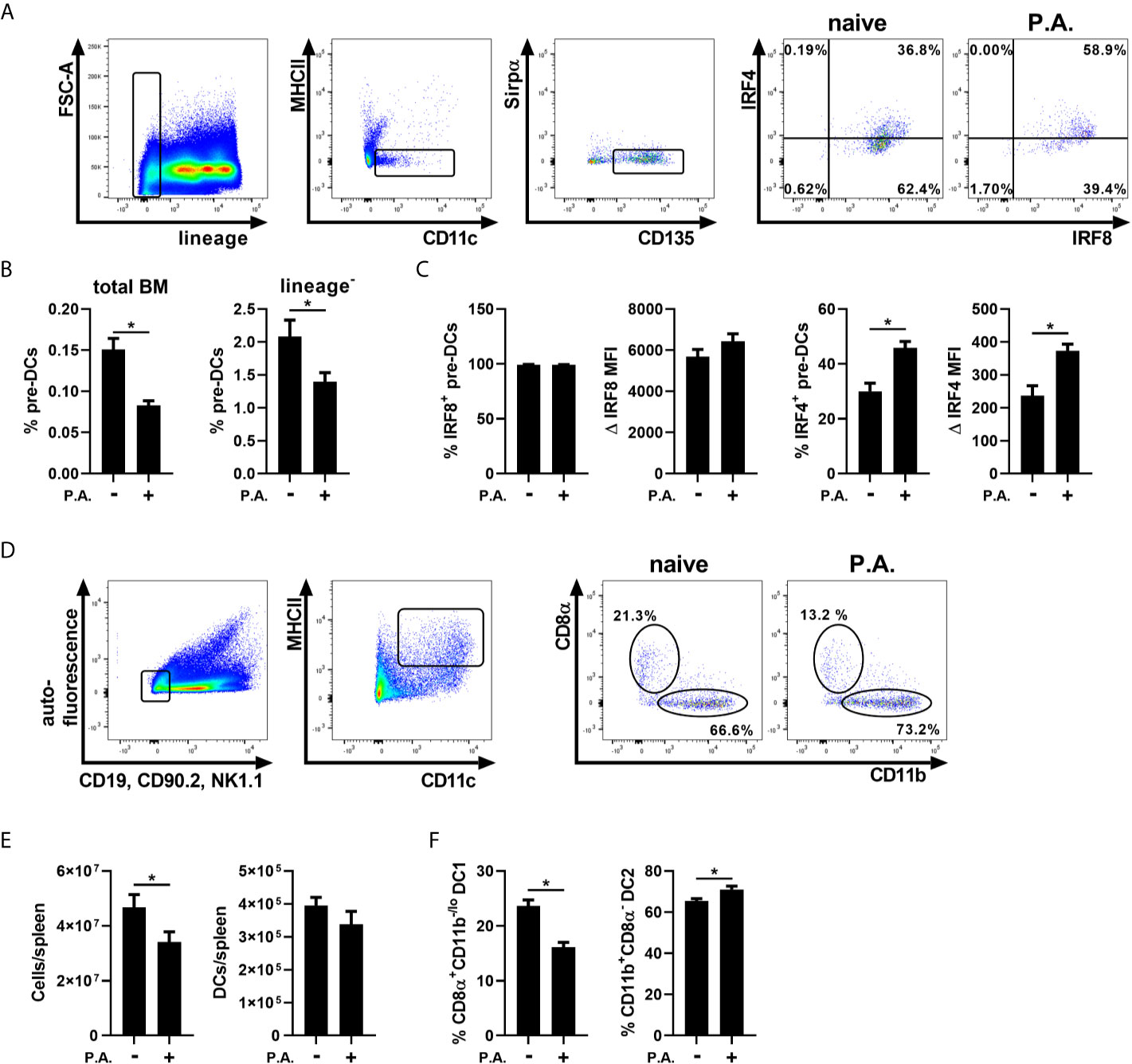
Figure 2 Intranasal instillation with P. aeruginosa induced a systemic effect on bone marrow cDC precursors and spleen cDC populations. WT mice were euthanized 18 h following i.n. instillation of 5 x 105 CFU of P. aeruginosa (P.A.) and bone marrow and spleen cells were analyzed by flow cytometry. (A) Bone marrow pre-DCs sequential gating strategy (lineage-, MHC II-, CD11c+, Sirpαlo/-, CD135+) and representative flow cytometry profile of IRF4 and IRF8 expression in pre-DCs from naive and P. aeruginosa treated mice. (B) Percentage of bone marrow pre-DCs in total bone marrow cells and lineage- cells. (C) Bone marrow pre-DCs: percentage and Δ MFI of IRF8 and IRF4. (D) Spleen cDCs sequential gating strategy (auto-fluorescence-, CD19-, CD90.2-, NK1.1-, MHC IIHi and CD11c+) and representative flow cytometry profile of CD8α and CD11b expression on cDCs from naive and P. aeruginosa treated mice. (E) Total spleen cells and spleen cDC number. (F) Percentage of CD8α+CD11b- cDC1 and CD11b+CD8α- cDC2 from spleen total cDCs. Data are presented as mean ± SEM; n = 8-10 mice per group combined from two separate experiments except for panel (C), IRF4; n = 5 representative of three separate experiments. *p < 0.05 using unpaired t-test.
To verify whether this impact was lung-specific, we tested cDC subsets in tissues that are not in direct contact with P. aeruginosa, such as the spleen. Total splenic cDCs were identified with the same gating strategy used in the lung, but, as spleen is a lymphoid organ, the CD8α surface marker was evaluated on cDC1s (Edelson et al., 2010; Guilliams et al., 2014). Thus, CD8α+CD11b- cDCs were characterized as cDC1s, while CD11b+CD8α- cDCs were classified as cDC2s (Figure 2D, Supplementary Figure 3) (Guilliams et al., 2014; Tavernier et al., 2015; Guilliams et al., 2016). Total splenic cells were decreased following i.n. instillation with P. aeruginosa, while cDC number remained unchanged (Figure 2E). The splenic proportion of CD8α+CD11b- cDC1s was decreased and in contrast the CD11b+CD8α- cDC2 proportion was increased, suggesting a systemic impact of P. aeruginosa on cDC signatures in various tissues (Figure 2F).
Pseudomonas aeruginosa Interferes With the Capacity of cDCs to Express CD103
We previously demonstrated that LPS and inflammatory factors directly abrogate the induction of CD103 expression on cDC1s (Brassard et al., 2019). To verify whether exposure to whole bacteria also influences CD103 expression on cDC1s, the proportion of XCR1+ cDC1s expressing CD103 was analyzed 18 h following i.n. instillation with P. aeruginosa. We observed a significant decrease in the percentage of CD103+ cDCs within the cDC1 population in response to bacterial exposure (Figure 3A).
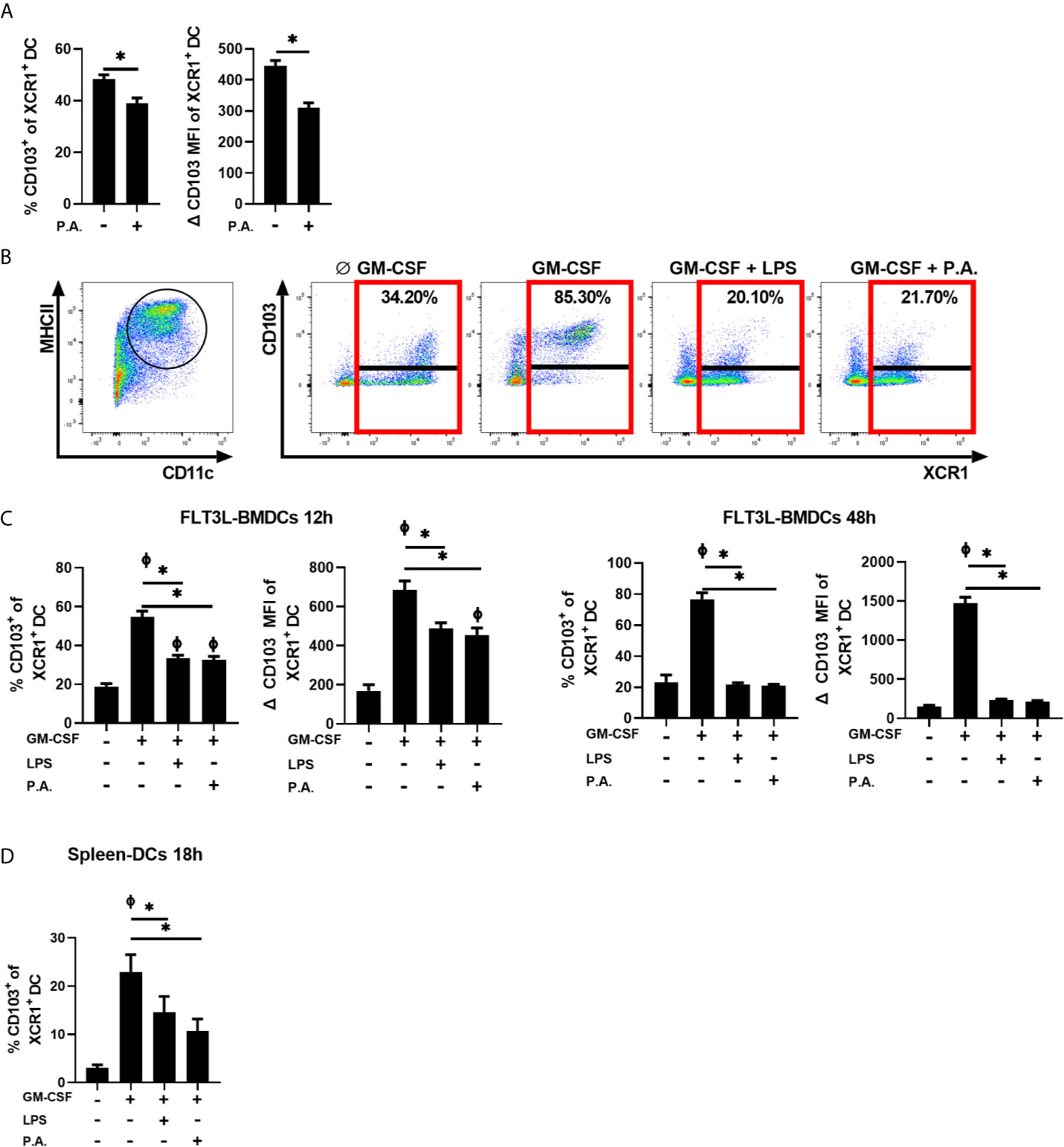
Figure 3 P. aeruginosa stimulation prevents GM-CSF-induced CD103 expression on cDCs. (A) Mice were euthanized 18 h after a single i.n. instillation of 5 x 105 CFU of P. aeruginosa (P.A.) and the percentage of CD103+ cells and CD103 MFI in XCR1+ cDC1 gated lung cells were analyzed by flow cytometry. (B, C) FLT3L-BMDCs or (D) spleen-isolated cDCs were stimulated with GM-CSF ± LPS or P. aeruginosa (P.A.) for 12 h, 18 h or 48 h and CD103 and XCR1 expression were analyzed by flow cytometry. (B) Gating strategy used to identify MHC IIHiCD11c+ cDCs and representative flow cytometry profile of CD103 expression on XCR1+ FLT3L-BMDCs stimulated for 48 h. (C, D) Percentage of CD103+ cells and CD103 MFI from previously gated XCR1+ cDCs. Data are presented as mean ± SEM; (A % CD103) n = 10-11 pooled from two separate experiments, (A CD103 MFI) n = 5 representative of three separate experiments. (C, D % CD103) n = 5-6 samples of cDCs per group pooled from two separate experiments. (C, D CD103 MFI) n = 3 representative of two separate experiments. * = p < 0.05 compared between conditions stimulated with GM-CSF. Φ = p < 0.05 compared to unstimulated condition. P-values were analyzed using unpaired t-test (A) or paired-one-way ANOVA (C, D).
Furthermore, we verified whether P. aeruginosa directly interferes with the capacity of cDC1s to express CD103 in response to GM-CSF using FLT3L-derived bone marrow DCs (BMDCs) (Gating strategy, Figure 3B and Supplementary Figure 4). Without any stimulation, the percentage of XCR1+ BMDCs expressing CD103 was low, with a mean of 18% positive cells. As expected, a 12 h stimulation with GM-CSF increased CD103 expression on cDC1s to approximately 55% (Figure 3C). However, the presence of P. aeruginosa during the GM-CSF stimulation prevented the maximal induction of CD103 (Figure 3C) to a level similar to that of LPS. This incapacity of XCR1+ BMDCs to fully express CD103 worsened in time, as CD103 expression on XCR1+ cDCs was almost entirely abrogated in response to LPS and P. aeruginosa at 48 h (Figures 3B, C). Although few freshly isolated splenic cDC1s express CD103, its expression can be induced on splenic cDCs by GM-CSF stimulation (Sathe et al., 2011; Brassard et al., 2019). We report that the induction of CD103 expression by GM-CSF is also reduced on spleen–isolated cDCs following exposure to P. aeruginosa, and to a level that is similar to LPS exposure (Figure 3D). Therefore, the blockade of CD103 expression on cDCs by these stimuli seems to be independent of the method used to generate cDCs. Of note, neither LPS nor P. aeruginosa altered cDCs viability (data not shown). These results also suggested that the presence of P. aeruginosa in the lung can directly influence the capacity of newly-recruited cDC1s to express CD103 in response to local GM–CSF.
Pseudomonas aeruginosa Influences the Localization of the GM-CSF Receptor
The exact mechanisms by which GM-CSF induces CD103 expression remain unknown, but binding of GM-CSF to its receptor (GM-CSFR) leads to a signaling cascade that is mediated in part by GM-CSFR internalization (Broughton et al., 2012; Zsiros et al., 2019). To first test whether GM-CSFR internalization is indeed involved in GM-CSF-induced cDC CD103 expression, FLT3L–BMDCs were pre-treated with Dynasore, a dynamin inhibitor that blocks internalization of receptor–ligand complex, prior to GM-CSF stimulations (Macia et al., 2006; Zsiros et al., 2019). Pre-treatment with Dynasore blocked CD103 expression on FLT3L-BMDCs suggesting that GM-CSFR internalization is required for its expression on cDCs (Figure 4A).
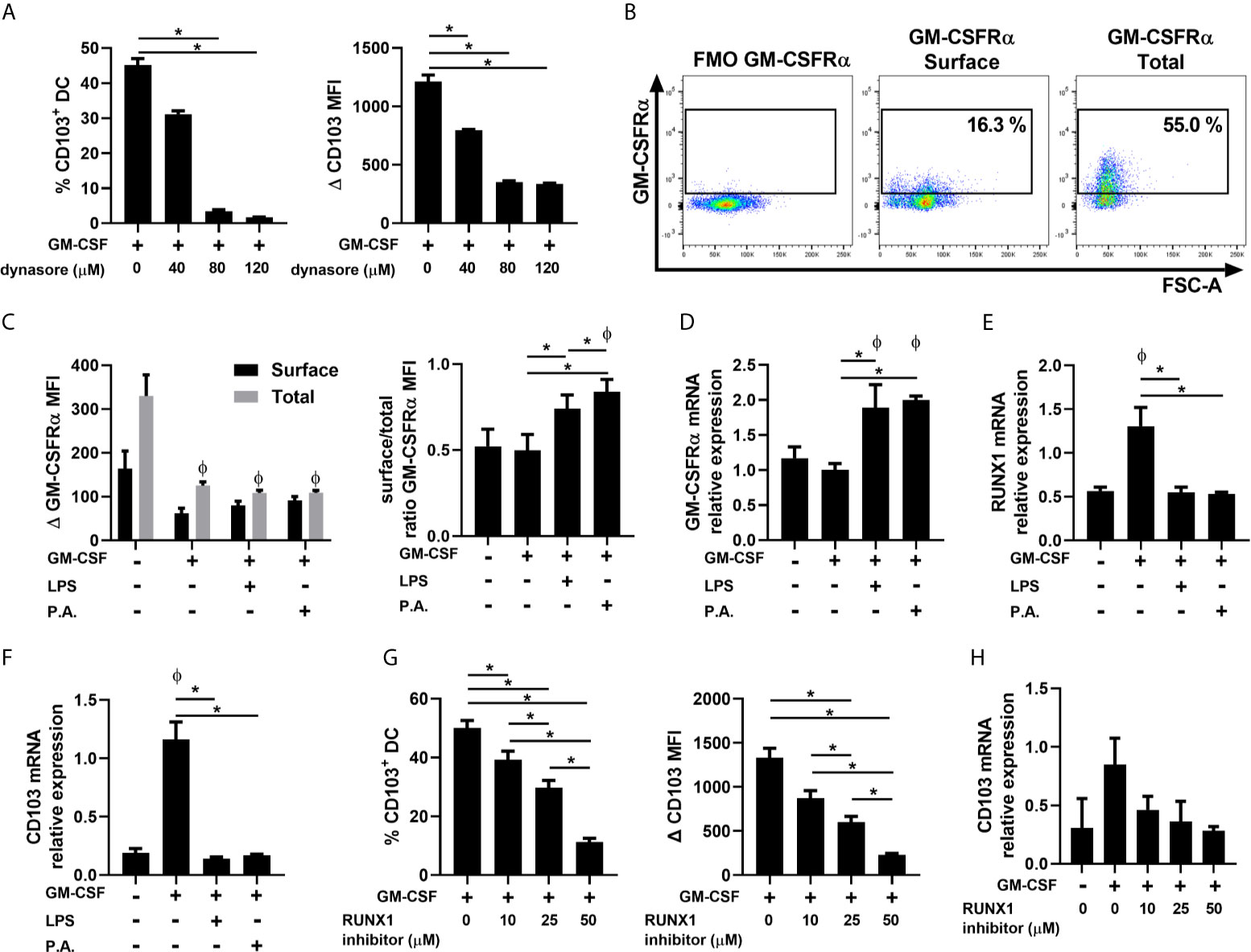
Figure 4 P. aeruginosa influences GM-CSFR localization on BMDCs. FLT3L–BMDCs were stimulated for (A–G) 48 h or (B–F, H) 12 h with GM-CSF ± (A) Dynasore, (B–F) GM-CSF ± LPS or P. aeruginosa (P.A.) or (G, H) a RUNX1 inhibitor. (A–G) Percentage of CD103+ and Δ CD103 MFI on cDCs. (B) Representative flow cytometry profile of surface and total GM–CSFRα expression on FLT3L-BMDCs. (C) Surface (extracellular) and total (extracellular + intracellular) Δ MFI (first panel) of GM-CSFRα, and ratio of surface to total expression of GM-CSFRα (second panel). (D) mRNA relative expression of GM-CSFRα (Csf2ra). (E) mRNA relative expression of RUNX1. (F, H) mRNA relative expression of CD103 (Itgae). Data are presented as mean ± SEM; (A) n = 3 representative of two separate experiments. (C–H) n = 4-6 samples of cDCs per group pooled from two separate experiments. *p < 0.05 compared between conditions stimulated with GM–CSF. Φ = p < 0.05 compared to unstimulated condition. P-values were analyzed using paired-one-way and two-way ANOVA.
To better understand whether GM-CSFR internalization is impacted by LPS or P. aeruginosa, extracellular and intracellular protein levels of the GM-CSFRα subunit were analyzed (Figures 4B, C). The combination of LPS or P. aeruginosa with GM-CSF did not alter total GM-CSFR expression (Figure 4C). However, when they were combined with GM-CSF, a higher ratio of surface to total GM-CSFRα expression was noted, indicating a reduced intracellular localization of the GM–CSFRα subunit (Figure 4C). This was not due to alterations in new receptor synthesis, as csf2ra (GM–CSFRα) mRNA expression was significantly increased in BMDCs exposed to P. aeruginosa (Figure 4D).
Additionally, RUNX1 mRNA expression, a member of the RUNX transcription factor family, was increased following GM-CSF stimulation, and abrogated in the presence of LPS or P. aeruginosa (Figure 4E). Following the same pattern as RUNX1, CD103 mRNA synthesis was also altered in the presence of LPS or P. aeruginosa (Figure 4F). To confirm the involvement of RUNX1 in the induction of CD103 expression in cDCs, FLT3L-BMDCs were pre-treated with a RUNX1 inhibitor prior to GM-CSF stimulation. We observed that the suppression of the transactivation activity of RUNX1 and its cofactor CBF in BMDCs leads to a reduction in both CD103 protein and mRNA synthesis, linking RUNX1 to CD103 expression in cDCs (Figures 4G, H). Therefore, our results suggest that the presence of P. aeruginosa impacts CD103 expression on cDC1s by interfering with the intracellular localization of the GM-CSFR, and by preventing RUNX1 mRNA expression.
Lack of CD103 Expression Enhanced the Lung Innate Immune Response to Pseudomonas aeruginosa
Lung exposure to P. aeruginosa resulted in an increase in XCR1+ cDC1s that lack CD103 expression. To determine the impact of an incapacity to express CD103 by cDCs on the immune response, we sought to verify whether the absence of CD103 expression on cDC1s modulates the early innate immune response to LPS and P. aeruginosa. LPS or P. aeruginosa were injected intranasally into WT and Cd103-/- mice and cells from the bronchoalveolar lavage (BAL) were analyzed 2 h (LPS) and 6 h (P. aeruginosa) later. These times were chosen since CD103 is still expressed on cDCs at that time following LPS exposure (Brassard et al., 2019), and because the increase in total cells in response to P. aeruginosa is slower than following LPS administration (data not shown). Of note, although CD103 is also present on T cells, they do not play a role in the rapid innate immune response to these agents (Andrew et al., 1996; Bernatchez et al., 2017). Following the i.n. administration of LPS, total cells and neutrophil numbers were increased in the BAL of Cd103-/- mice compared to WT mice (Figure 5A). This was also observed in Cd103-/- mice exposed to P. aeruginosa compared to WT (Figure 5B). These results suggest that the absence of CD103 expression facilitates neutrophil recruitment in response to Gram–negative bacteria.
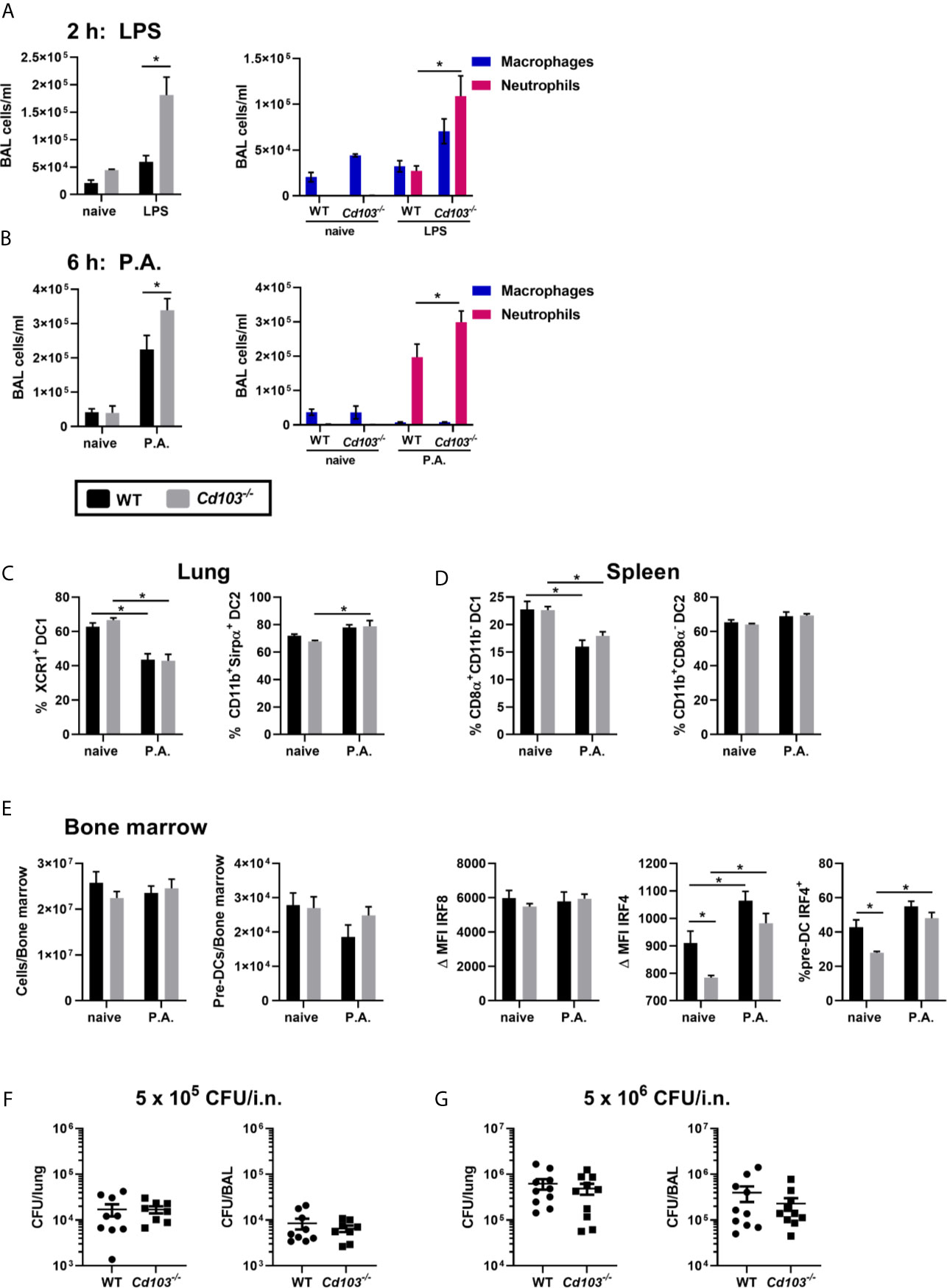
Figure 5 Lack of CD103 expression favors the recruitment of bronchoalveolar neutrophils. (A, B) Total number (first panel), macrophages and neutrophils (second panel) of bronchoalveolar lavage (BAL) cells were compared between WT and Cd103-/- mice (A) 2 h following LPS i.n. instillation or (B) 6 h following i.n. instillation of 5 x 105 CFU of P. aeruginosa (P.A.). (C–E) Lung, spleen and bone marrow cells were analyzed by flow cytometry 18 h after i.n. instillation with 5 x 105 CFU of P. aeruginosa. (C) Percentage of XCR1+ cDC1s and percentage of CD11b+Sirpα+ cDC2s in lung. (D) Percentage of CD8α+CD11b+ cDC1s and percentage of CD11b+CD8α- cDC2s in spleen. (E) Total cell number, pre-DC number, ΔIRF8 MFI, ΔIRF4 MFI and percentage of IRF4+ pre-DCs in bone marrow. (F, G) P. aeruginosa CFU number present in the lung and BAL 6 h after i.n. instillation of (F) 5 x 105 CFU or (G) 5 x 106 CFU of P. aeruginosa. Data are presented as mean ± SEM; (A) n = 2-5 (naive-P.A.) representative of two independent experiments. (B) n = 3-10 (naive-P.A.) pooled from two separate experiments. (C–E) (total and pre-DC number) (F, G) n = 9-10 pooled from two separate experiments. (E) (IRF4-IRF8) n = 5 representative of two independent experiments. *p < 0.05 using two-way ANOVA.
We then assessed whether this was caused by a modulation in cDC or cDC precursors populations. The lung and spleen total cell numbers, cDC numbers, (data not shown) and the percentage of cDC1s and cDC2s were similar between WT and Cd103-/- mice in naive and P. aeruginosa exposed mice (Figures 5C, D). Moreover, bone marrow total cells, pre–DC numbers and IRF8 MFI were also similar between strains (Figure 5E). The IRF4 MFI and the percentage of IRF4+ pre-DCs were significantly lower in Cd103-/- naive mice compared to WT naive mice, but similar in both strains following the exposure to P. aeruginosa (Figure 5E). Therefore, the higher recruitment of lung neutrophils in Cd103-/- mice is not caused by a difference in number or proportions in cDC or pre-DC populations.
To determine whether this affected bacterial clearance, we first studied the optimal time to study P. aeruginosa clearance. Almost all bacteria were cleared at 12 h following i.n. instillation of P. aeruginosa (data not shown), thus the 6 h timepoint was selected to compare CFU number in WT and Cd103-/- mice. We report similar numbers of P. aeruginosa CFU counts in the BAL and lung homogenates of the two mouse strains, (Figure 5F) and this was independent of the concentration used (Figure 5G). This likely indicates that the bacterial clearance kinetics of this model/P. aeruginosa strain may be too quick to verify whether the increase in neutrophils observed in the absence of cDC CD103 expression leads to better bacterial clearance.
Discussion
DCs take part in the induction of innate and adaptive immune responses, and the efficacy of these responses is influenced by the nature of local cDC subsets (Macri et al., 2018). Until now, few studies focused on the influence of bacterial infection on cDC populations. In this report, we demonstrated that lung exposure to live P. aeruginosa bacteria decreases the proportions of CD103+ cDC1s in favor of CD11b+ cDC2s/monocyte-derived DCs. We determined that this was in part modulated by modifications in bone marrow pre-DC populations and an altered CD103 expression on XCR1+ cDC1s (Figure 6). Furthermore, we demonstrated that the absence of CD103 expression increases neutrophils recruitment in the lung in response to LPS and P. aeruginosa.
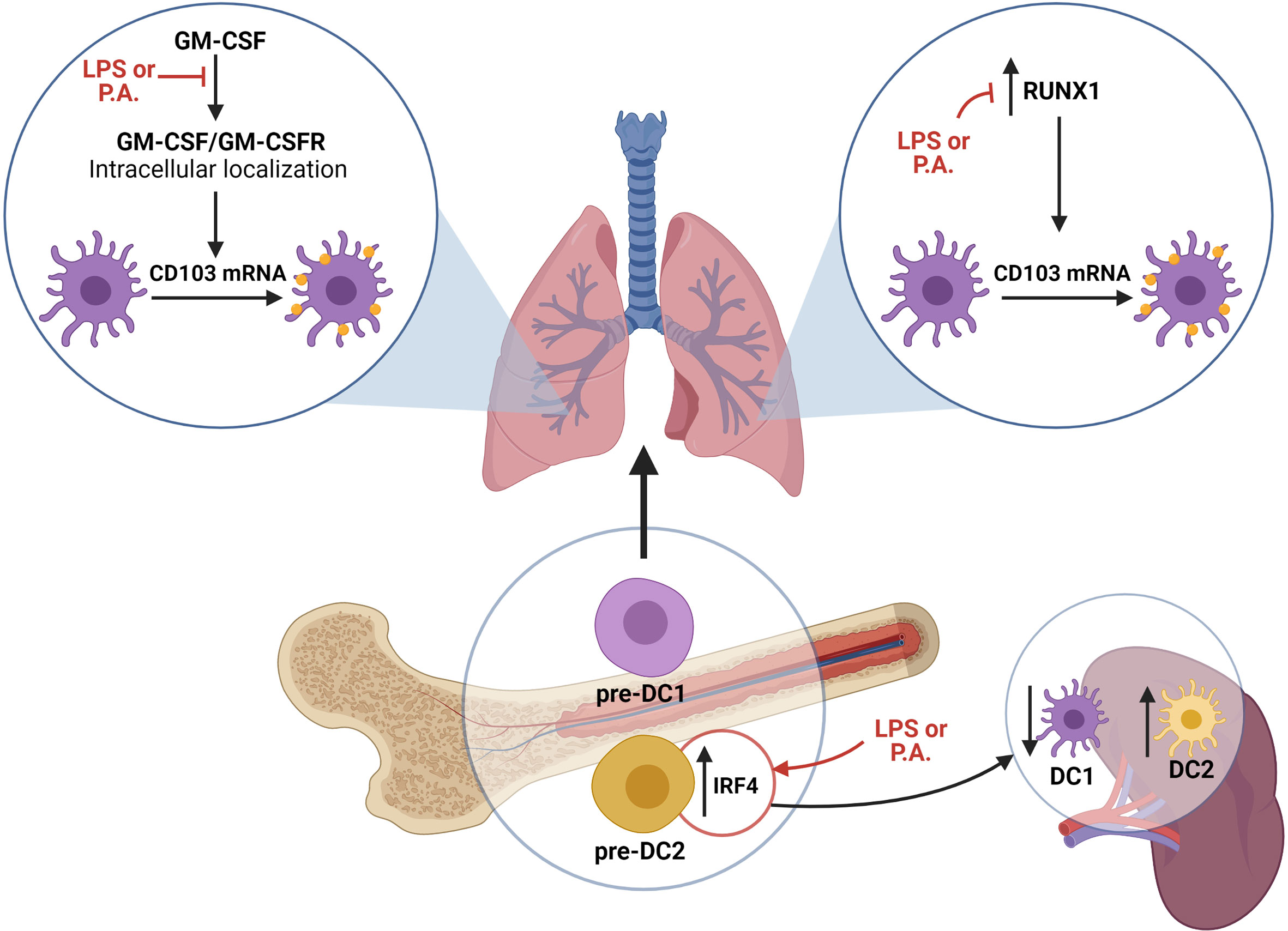
Figure 6 Schematic representation of proposed mechanisms supporting the modulation of lung cDC populations in response to P. aeruginosa. LPS and P. aeruginosa directly impact the capacity of cDCs to internally localize the GM-CSFR, which is a crucial step in GM-CSF signaling leading to CD103 mRNA synthesis and CD103 expression on cDC1s. Additionally, LPS and P. aeruginosa interfere with RUNX1 expression, whose activity is needed for GM-CSF-dependent CD103 expression. Finally, exposure to LPS and P. aeruginosa alters the pre-DC transcriptional signature, favoring cDC2 precursor differentiation. Altogether, the exposure to LPS and P. aeruginosa impacts the capacity of cDCs to express CD103, as well as the cDC precursor signature. Figure 6 was Created with BioRender.com.
First, we realize that our gating strategy to identify cDC2s does not discard monocyte-derived DCs, so this population must be considered as potentially upregulated in response to P. aeruginosa. Independently of this, the altered proportions of cDC populations are not restricted to lung exposure to LPS or P. aeruginosa. Indeed, similar results were reported in a mouse model of lung infection with Chlamydia muridarum, an LPS+ bacteria (Shekhar et al., 2018; Brassard et al., 2019). Interestingly, in the C. muridarum model, a decrease in CD103+ cDC proportions was observed up to 7 days post-infection, suggesting that this phenomenon is not limited to acute immune responses (Shekhar et al., 2018). This is also supported by similar alterations observed by our group in a 21-day mouse model of chronic allergic inflammation to Saccharopolyspora rectivirgula antigen (Bernatchez et al., 2017).
Our results suggest that lung exposure to P. aeruginosa altered bone marrow cDC precursors towards pre-DCs that are committed to the cDC2 lineage, which likely contributes to the accumulation of lung cDC2s. A modification of bone marrow pre-DCs towards the cDC1 or cDC2 lineage was observed in other immunological contexts including cancer, viral infections and lung exposure to LPS (Beshara et al., 2018; Meyer et al., 2018 Brassard et al., 2019), suggesting that this mechanism is conserved across various diseases and types of inflammatory responses. We previously observed that lung exposure to LPS leads to a decrease in IRF8 expression in pre-DCs, whereas an increase of IRF4 expression is reported here in response to P. aeruginosa (Brassard et al., 2019). Some studies suggest a sequential order between IRF8 and IRF4 expression in which the decrease in IRF8 expression precedes the increase in IRF4 expression in pre-DCs committed to the cDC2 lineage (Bajaña et al., 2016; Sichien et al., 2016). Therefore, the differences reported in both studies could be explained by a difference in the timing of induction for these transcription factors in these models. Despite this difference, both results report an imbalance towards the cDC2 lineage differentiation.
Our results also suggest that splenic cDC populations are affected by lung P. aeruginosa exposure. As the cDC turnover in the spleen is fast, even at steady state, an absence of total cDC number increase (such as observed here) does not indicate an absence of new cDC recruitment (Kamath et al., 2002), and the altered cDC populations is likely a reflection of a systemic impact of P. aeruginosa. Thus, our results suggest that bone marrow pre-DCs are biased towards the cDC2 lineage, which in turns influences the subsets of cDC precursors recruited in the spleen, resulting in a modulation of splenic cDC1 and cDC2 proportions (Figure 6). As pre-DCs are precursors for most tissue cDCs, this modification in bone marrow pre–DCs could also influence cDC populations in other organs, and ultimately impact the efficacy of immune responses in case of a systemic infection (Ginhoux et al., 2009). We are aware that other mechanisms besides alterations in cDC precursors could be involved in explaining the differences in the cDC population signature in our models. As our gating strategy also included mo-DCs as CD11b+, this population could contribute to the imbalance reported here (Guilliams et al., 2016). Also, a higher migratory rate of cDC1s from the lung to the draining lymph nodes could take part in the observed decrease percentage of this population following P. aeruginosa exposure (Ho et al., 2011; Nakano et al., 2013).
Supported by previously-published results (Brassard et al., 2019) and present data, we propose that under homeostatic conditions, newly-recruited lung pre-DCs acquire CD103 expression in response to GM-CSF stimulation through their final differentiation into cDCs. However, in the context of Gram-negative bacterial exposure, the interaction with the bacteria or LPS prevents CD103 mRNA synthesis resulting in lower GM-CSF-induced CD103 expression on cDC1s. Importantly, our results do not demonstrate that LPS nor P. aeruginosa reduce the expression of CD103 once it has been expressed, but rather block the induction upon contact with GM-CSF. Furthermore, our observations suggest that a reduced internal localization of the GM-CSFR could be one of the mechanisms contributing to the reduction in CD103 expression. Our results linking the GM-CSFR internalization to CD103 expression were obtained using Dynasore a GTPase inhibitor that inhibits dynamin activity, which prevents endocytosis. Although this inhibitor is frequently used to block GM-CSFR internalization (Katz et al., 2016; Zsiros et al., 2019), Dynasore could also exert other effects. For instance, the presence of Dynasore could also block the internalization of other receptors, such as TLR4, express by cDCs (Kagan et al., 2008). However, as our assays with Dynasore were performed using only GM-CSF stimulation, it is very likely that in this case, the inhibition of CD103 expression was linked to a reduced blockade of GM-CSFR internalization. The exact steps linking the activation of GM-CSFR signaling to the induction of CD103 mRNA expression on cDCs remained unknown. For the first time, we demonstrate that RUNX1 expression is crucial for CD103 expression in cDCs. Additionally, we show that LPS and P. aeruginosa alter RUNX1 expression, likely contributing to reduced CD103 expression in response to these stimuli. Of course, other cellular signaling pathways besides GM-CSF are involved in regulating CD103 expression on cDCs. For example, TGFβ and retinoic acid also reportedly induced CD103 expression (Iliev et al., 2009; Roe et al., 2017; Roe et al., 2020). Whether LPS and P. aeruginosa alter CD103 expression in response to these stimuli remains unknown, but definitely of interest.
Despite a wealth of knowledge on CD103 modulation on cDCs in response to various stimuli (Nakano et al., 2013; Bernatchez et al., 2017; Brassard et al., 2019), the physiological function of this phenomenon on the host immune response remained undefined. We propose here that the absence of CD103 expression supports the intensity of the initial host innate immune response. Anecdotally, in a model of skin bacterial infection, cDC1s were crucial for neutrophils recruitment to the site of infection via the secretion of VEGF-α (Janela et al., 2019), which suggested that cDC1s influence neutrophil migration. CD103 signaling in cDC1s could be involved in promoting homeostasis by restraining the production of chemokines or pro-inflammatory cytokines. In contrast, during bacterial infections, the absence of CD103 signaling on cDC1s could increase their capacity to secrete neutrophils-attracting chemokines or induce neutrophil migration via an indirect effect on surrounding cells. All in all, our study suggests that the reduced CD103 expression on cDC1s may be a step in stimulating the innate immune response to Gram-negative bacteria.
The higher recruitment of lung neutrophils in Cd103-/- mice in response to P. aeruginosa was not caused by a difference in the number or proportions of cDC or pre-DC populations. However, a reduced IRF4 expression in bone marrow pre-DCs was observed in Cd103-/- naive mice, suggesting a lower propensity of cDC2 differentiation at steady state in these mice. As pre-DCs don’t express CD103, an indirect mechanism must be involved to explain this (Brassard et al., 2019). Also, we cannot exclude the possibility that, as WT and Cd103-/- strains were not co-housed in our experiments, differences in microbiota could be involved in impacting IRF4 expression.
Neutrophils are crucial to eradicate P. aeruginosa infection (Koh et al., 2009). The fact that the increased neutrophil count in Cd103-/- mice did not in turn change bacterial clearance indicates that the number of neutrophils in WT mice was sufficient to quickly clear bacteria. This is also supported by the fact that most of this mucoid strain of P. aeruginosa was cleared from the lung past 6 h (data not shown) in WT mice. Furthermore, several other studies observed that a higher number of lung neutrophils do not necessarily lead to a better P. aeruginosa clearance (Ballinger et al., 2006; Sen-Kilic et al., 2019). As CD103 expression could restrain chemokine production by cDC1s, mice in which cDC1s constitutively express CD103 would be a great tool to address the importance of cDC-specific CD103 expression on neutrophil recruitment and bacterial clearance with WT mice. Based on current knowledge, it is hard to determine whether the alterations of cDC populations induced by P. aeruginosa promote or impair host immune responses. First, the combination of the important lung recruitment of cDC2s, known to secrete large amount of MIP-2 and KC neutrophil-attractant chemokines, and the possible higher capacity of cDC1s to recruits neutrophils in absence of CD103 expression suggest a positive impact on immune response. On the other hand, current knowledge on adaptive immune responses against bacterial infection endorse the idea that cDC1s support the efficacy of the adaptive immune response compared to cDC2s. To date, no studies have established a specific role for cDC1s or cDC2s in innate or adaptive immune responses to P. aeruginosa, but this report takes a first important step towards better understanding the modulation of local cDC populations in the context of Gram-negative bacterial clearance.
An important conclusion rising from this data and our previously published research is that several factors such as the presence of LPS, TNF or P. aeruginosa can prevent the induction of CD103 expression on cDC1 (Brassard et al., 2019). Currently, in the lung, CD103 remains one of the main markers used to identify cDC1s (Ng et al., 2018; Shekhar et al., 2018; Monaghan et al., 2020). However, our results suggest that CD103 is not an ideal marker for this population in context of lung inflammation as its expression is highly modulated. Furthermore, in a few other particular immunological contexts, some lung CD11b+ DCs can also express CD103, which could lead to misinterpretations if used as a specific marker of cDC1s (Shane et al., 2018; Tweedle and Deepe, 2018). Finally, another disadvantage is that CD103 is not a marker of human lung cDC1s (Guilliams et al., 2014; Amon et al., 2020). Thus, the ideal marker to properly identify cDC1s should be constitutively expressed by cDC1s independently of their differentiation stage or activation status, and similarly expressed by human cDC1s.
In summary, we demonstrated that i.n. exposure to the Gram-negative bacteria P. aeruginosa alters the proportions of CD103+ cDC1 and CD11b+ cDC2 populations in favor of cDC2s, via the modulation of bone marrow cDC precursors and an impact on CD103 mRNA production by cDC1s. Furthermore, we demonstrated that the absence of CD103 expression favors the recruitment of lung neutrophils, suggesting that this phenomenon could be an important step in the early innate immune response to P. aeruginosa.
Data Availability Statement
The raw data supporting the conclusions of this article will be made available by the authors, without undue reservation.
Ethics Statement
The animal study was reviewed and approved by Comité de protection des animaux de l’Université Laval.
Author Contributions
JB and M-RB conceived the study. JB, JR, M-JB, and EB performed experiments. JB and M-RB drafted and revised the paper. AML and M-JB critically revised the manuscript. MV and CD contributed to the final revision and supported microbiology experiments. M-RB supervised the study. All authors contributed to the article and approved the submitted version.
Funding
This work was supported by the Fonds sur les Maladies Respiratoires Bégin/Lavoie de l’Université Laval and by the Fondation de l’Institut Universitaire de Cardiologie et de Pneumologie de l’Université Laval.
Conflict of Interest
The authors declare that the research was conducted in the absence of any commercial or financial relationships that could be construed as a potential conflict of interest.
Acknowledgments
The authors would like to thank the CRIUCPQ animal unit as well as the CRIUCPQ animal unit personnel for their precious collaboration and support.
Supplementary Material
The Supplementary Material for this article can be found online at: https://www.frontiersin.org/articles/10.3389/fcimb.2021.617481/full#supplementary-material
Abbreviations
BAL, Bronchoalveolar lavages; BMDC, Bone marrow derived dendritic cell; CFU, Colony forming unit; cDC, Conventional dendritic cell; FLT3L, FMS-like tyrosine kinase 3 ligand; FLT3L-BMDC, BMDC differentiated with FLT3L; FMO, Fluorescence minus one; GMߛCSF, Granulocyte-macrophage colony-stimulating factor; GM-CSFR, GM-CSF receptor; GM-CSFRα, GM-CSFR subunit α; GM-CSFRβ, GM-CSFR subunit β; i.n., Intranasal; LPS, Lipopolysaccharide; MFI, Mean fluorescence intensity; PBS, Phosphate buffered saline; TSB, Tryptic soy broth; WT, wild-type.
References
Amon, L., Lehmann, C. H. K., Heger, L., Heidkamp, G. F., Dudziak, D. (2020). The Ontogenetic Path of Human Dendritic Cells. Mol. Immunol. 120, 122–129. doi: 10.1016/j.molimm.2020.02.010
Andrew, D. P., Rott, L. S., Kilshaw, P. J., Butcher, E. C. (1996). Distribution of Alpha 4 Beta 7 and Alpha E Beta 7 Integrins on Thymocytes, Intestinal Epithelial Lymphocytes and Peripheral Lymphocytes. Eur. J. Immunol. 26, 897–905. doi: 10.1002/eji.1830260427
Bajaña, S., Turner, S., Paul, J., Ainsua-Enrich, E., Kovats, S. (2016). IRF4 and IRF8 Act in CD11c+ Cells To Regulate Terminal Differentiation of Lung Tissue Dendritic Cells. J. Immunol. 196, 1666–1677. doi: 10.4049/jimmunol.1501870
Ballinger, M. N., Paine, R., 3rd, Serezani, C. H., Aronoff, D. M., Choi, E. S., Standiford, T. J., et al. (2006). Role of Granulocyte Macrophage Colony-Stimulating Factor During Gram-Negative Lung Infection With Pseudomonas Aeruginosa. Am. J. Respir. Cell Mol. Biol. 34, 766–774. doi: 10.1165/rcmb.2005-0246OC
Beaty, S. R., Rose, C. E., Jr., Sung, S. S. (2007). Diverse and Potent Chemokine Production by Lung CD11bhigh Dendritic Cells in Homeostasis and in Allergic Lung Inflammation. J. Immunol. 178, 1882–1895. doi: 10.4049/jimmunol.178.3.1882
Bernatchez, E., Langlois, A., Brassard, J., Flamand, N., Marsolais, D., Blanchet, M. R. (2017). Hypersensitivity Pneumonitis Onset and Severity is Regulated by CD103 Dendritic Cell Expression. PloS One 12, e0179678. doi: 10.1371/journal.pone.0179678
Beshara, R., Sencio, V., Soulard, D., Barthélémy, A., Fontaine, J., Pinteau, T., et al. (2018). Alteration of Flt3-Ligand-dependent De Novo Generation of Conventional Dendritic Cells During Influenza Infection Contributes to Respiratory Bacterial Superinfection. PloS Pathog. 14, e1007360. doi: 10.1371/journal.ppat.1007360
Brassard, J., Maheux, C., Langlois, A., Bernatchez, E., Marsolais, D., Flamand, N., et al. (2019). Lipopolysaccharide Impacts Murine CD103(+) DC Differentiation, Altering the Lung DC Population Balance. Eur. J. Immunol. 49, 638–652. doi: 10.1002/eji.201847910
Broughton, S. E., Dhagat, U., Hercus, T. R., Nero, T. L., Grimbaldeston, M. A., Bonder, C. S., et al. (2012). The GM-CSF/IL-3/IL-5 Cytokine Receptor Family: From Ligand Recognition to Initiation of Signaling. Immunol. Rev. 250, 277–302. doi: 10.1111/j.1600-065X.2012.01164.x
Corps, E., Carter, C., Karecla, P., Ahrens, T., Evans, P., Kilshaw, P. (2001). Recognition of E-cadherin by Integrin Alpha(E)Beta(7): Requirement for Cadherin Dimerization and Implications for Cadherin and Integrin Function. J. Biol. Chem. 276, 30862–30870. doi: 10.1074/jbc.M101712200
Crozat, K., Tamoutounour, S., Vu Manh, T. P., Fossum, E., Luche, H., Ardouin, L., et al. (2011). Cutting Edge: Expression of XCR1 Defines Mouse Lymphoid-Tissue Resident and Migratory Dendritic Cells of the CD8α+ Type. J. Immunol. 187, 4411–4415. doi: 10.4049/jimmunol.1101717
Edelson, B. T., Kc, W., Juang, R., Kohyama, M., Benoit, L. A., Klekotka, P. A., et al. (2010). Peripheral CD103+ Dendritic Cells Form a Unified Subset Developmentally Related to CD8alpha+ Conventional Dendritic Cells. J. Exp. Med. 207, 823–836. doi: 10.1084/jem.20091627
Franciszkiewicz, K., Le Floc’h, A., Boutet, M., Vergnon, I., Schmitt, A., Mami-Chouaib, F. (2013). CD103 or LFA-1 Engagement at the Immune Synapse Between Cytotoxic T Cells and Tumor Cells Promotes Maturation and Regulates T-cell Effector Functions. Cancer Res. 73, 617–628. doi: 10.1158/0008-5472.Can-12-2569
Gao, Y., Nish, S. A., Jiang, R., Hou, L., Licona-Limón, P., Weinstein, J. S., et al. (2013). Control of T Helper 2 Responses by Transcription Factor IRF4-dependent Dendritic Cells. Immunity 39, 722–732. doi: 10.1016/j.immuni.2013.08.028
Ginhoux, F., Liu, K., Helft, J., Bogunovic, M., Greter, M., Hashimoto, D., et al. (2009). The Origin and Development of Nonlymphoid Tissue CD103+ Dcs. J. Exp. Med. 206, 3115–3130. doi: 10.1084/jem.20091756
Grajales-Reyes, G. E., Iwata, A., Albring, J., Wu, X., Tussiwand, R., Kc, W., et al. (2015). Batf3 Maintains Autoactivation of Irf8 for Commitment of a CD8α(+) Conventional DC Clonogenic Progenitor. Nat. Immunol. 16, 708–717. doi: 10.1038/ni.3197
Green, S. K., Schroth, M. N., Cho, J. J., Kominos, S. K., Vitanza-jack, V. B. (1974). Agricultural Plants and Soil as a Reservoir for Pseudomonas Aeruginosa. Appl. Microbiol. 28, 987–991. doi: 10.1128/am.28.6.987-991.1974
Greter, M., Helft, J., Chow, A., Hashimoto, D., Mortha, A., Agudo-Cantero, J., et al. (2012). Gm-CSF Controls Nonlymphoid Tissue Dendritic Cell Homeostasis But is Dispensable for the Differentiation of Inflammatory Dendritic Cells. Immunity 36, 1031–1046. doi: 10.1016/j.immuni.2012.03.027
Grueter, B., Petter, M., Egawa, T., Laule-Kilian, K., Aldrian, C. J., Wuerch, A., et al. (2005). Runx3 Regulates Integrin Alpha E/CD103 and CD4 Expression During Development of CD4-/CD8+ T Cells. J. Immunol. 175, 1694–1705. doi: 10.4049/jimmunol.175.3.1694
Guilliams, M., Dutertre, C. A., Scott, C. L., McGovern, N., Sichien, D., Chakarov, S., et al. (2016). Unsupervised High-Dimensional Analysis Aligns Dendritic Cells Across Tissues and Species. Immunity 45, 669–684. doi: 10.1016/j.immuni.2016.08.015
Guilliams, M., Ginhoux, F., Jakubzick, C., Naik, S. H., Onai, N., Schraml, B. U., et al. (2014). Dendritic Cells, Monocytes and Macrophages: A Unified Nomenclature Based on Ontogeny. Nat. Rev. Immunol. 14, 571–578. doi: 10.1038/nri3712
Gurka, S., Hartung, E., Becker, M., Kroczek, R. A. (2015). Mouse Conventional Dendritic Cells can be Universally Classified Based on the Mutually Exclusive Expression of XCR1 and Sirpα. Front. Immunol. 6, 35. doi: 10.3389/fimmu.2015.00035
Hildner, K., Edelson, B. T., Purtha, W. E., Diamond, M., Matsushita, H., Kohyama, M., et al. (2008). Batf3 Deficiency Reveals a Critical Role for CD8alpha+ Dendritic Cells in Cytotoxic T Cell Immunity. Science 322, 1097–1100. doi: 10.1126/science.1164206
Ho, A. W., Prabhu, N., Betts, R. J., Ge, M. Q., Dai, X., Hutchinson, P. E., et al. (2011). Lung CD103+ Dendritic Cells Efficiently Transport Influenza Virus to the Lymph Node and Load Viral Antigen Onto MHC Class I for Presentation to CD8 T Cells. J. Immunol. 187, 6011–6021. doi: 10.4049/jimmunol.1100987
Iliev, I. D., Spadoni, I., Mileti, E., Matteoli, G., Sonzogni, A., Sampietro, G. M., et al. (2009). Human Intestinal Epithelial Cells Promote the Differentiation of Tolerogenic Dendritic Cells. Gut 58, 1481–1489. doi: 10.1136/gut.2008.175166
Janela, B., Patel, A. A., Lau, M. C., Goh, C. C., Msallam, R., Kong, W. T., et al. (2019). A Subset of Type I Conventional Dendritic Cells Controls Cutaneous Bacterial Infections Through Vegfα-Mediated Recruitment of Neutrophils. Immunity 50, 1069–1083.e8. doi: 10.1016/j.immuni.2019.03.001
Kagan, J. C., Su, T., Horng, T., Chow, A., Akira, S., Medzhitov, R. (2008). TRAM Couples Endocytosis of Toll-like Receptor 4 to the Induction of Interferon-Beta. Nat. Immunol. 9, 361–368. doi: 10.1038/ni1569
Kamath, A. T., Henri, S., Battye, F., Tough, D. F., Shortman, K. (2002). Developmental Kinetics and Lifespan of Dendritic Cells in Mouse Lymphoid Organs. Blood 100, 1734–1741. doi: 10.1182/blood.V100.5.1734.h81702001734_1734_1741
Katz, S., Zsiros, V., Dóczi, N., Szabó, A., Biczó, Á., Kiss, A. L. (2016). Gm-CSF and GM-CSF Receptor Have Regulatory Role in Transforming Rat Mesenteric Mesothelial Cells Into Macrophage-Like Cells. Inflammation Res. 65, 827–836. doi: 10.1007/s00011-016-0967-5
King, I. L., Kroenke, M. A., Segal, B. M. (2010). GM-CSF-Dependent, CD103+ Dermal Dendritic Cells Play a Critical Role in Th Effector Cell Differentiation After Subcutaneous Immunization. J. Exp. Med. 207, 953–961. doi: 10.1084/jem.20091844
Koh, A. Y., Priebe, G. P., Ray, C., Van Rooijen, N., Pier, G. B. (2009). Inescapable Need for Neutrophils as Mediators of Cellular Innate Immunity to Acute Pseudomonas Aeruginosa Pneumonia. Infect. Immun. 77, 5300–5310. doi: 10.1128/iai.00501-09
Liu, K., Victora, G. D., Schwickert, T. A., Guermonprez, P., Meredith, M. M., Yao, K., et al. (2009). In Vivo Analysis of Dendritic Cell Development and Homeostasis. Science 324, 392–397. doi: 10.1126/science.1170540
Lyczak, J. B., Cannon, C. L., Pier, G. B. (2000). Establishment of Pseudomonas Aeruginosa Infection: Lessons From a Versatile Opportunist. Microbes Infect. 2, 1051–1060. doi: 10.1016/s1286-4579(00)01259-4
Macia, E., Ehrlich, M., Massol, R., Boucrot, E., Brunner, C., Kirchhausen, T. (2006). Dynasore, a Cell-Permeable Inhibitor of Dynamin. Dev. Cell 10, 839–850. doi: 10.1016/j.devcel.2006.04.002
Macri, C., Pang, E. S., Patton, T., O’Keeffe, M. (2018). Dendritic Cell Subsets. Semin. Cell Dev. Biol. 84, 11–21. doi: 10.1016/j.semcdb.2017.12.009
Martínez-López, M., Iborra, S., Conde-Garrosa, R., Sancho, D. (2015). Batf3-Dependent CD103+ Dendritic Cells are Major Producers of IL-12 That Drive Local Th1 Immunity Against Leishmania Major Infection in Mice. Eur. J. Immunol. 45, 119–129. doi: 10.1002/eji.201444651
Mashayekhi, M., Sandau, M. M., Dunay, I. R., Frickel, E. M., Khan, A., Goldszmid, R. S., et al. (2011). Cd8α(+) Dendritic Cells are the Critical Source of interleukin-12 That Controls Acute Infection by Toxoplasma Gondii Tachyzoites. Immunity 35, 249–259. doi: 10.1016/j.immuni.2011.08.008
Mayer, C. T., Ghorbani, P., Nandan, A., Dudek, M., Arnold-Schrauf, C., Hesse, C., et al. (2014). Selective and Efficient Generation of Functional Batf3-Dependent CD103+ Dendritic Cells From Mouse Bone Marrow. Blood 124, 3081–3091. doi: 10.1182/blood-2013-12-545772
Meyer, M. A., Baer, J. M., Knolhoff, B. L., Nywening, T. M., Panni, R. Z., Su, X., et al. (2018). Breast and Pancreatic Cancer Interrupt IRF8-Dependent Dendritic Cell Development to Overcome Immune Surveillance. Nat. Commun. 9, 1250. doi: 10.1038/s41467-018-03600-6
Monaghan, K. L., Farris, B. Y., Zheng, W., Wan, E. C. K. (2020). Characterization of Immune Cells and Proinflammatory Mediators in the Pulmonary Environment. J. Vis. Exp. doi: 10.3791/61359
Moradali, M. F., Ghods, S., Rehm, B. H. (2017). Pseudomonas Aeruginosa Lifestyle: A Paradigm for Adaptation, Survival, and Persistence. Front. Cell Infect. Microbiol. 7, 39. doi: 10.3389/fcimb.2017.00039
Moser, C., Jensen, P. O., Kobayashi, O., Hougen, H. P., Song, Z., Rygaard, J., et al. (2002). Improved Outcome of Chronic Pseudomonas Aeruginosa Lung Infection is Associated With Induction of a Th1-dominated Cytokine Response. Clin. Exp. Immunol. 127, 206–213. doi: 10.1046/j.1365-2249.2002.01731.x
Moser, C., Johansen, H. K., Song, Z., Hougen, H. P., Rygaard, J., Høiby, N. (1997). Chronic Pseudomonas Aeruginosa Lung Infection is More Severe in Th2 Responding BALB/c Mice Compared to Th1 Responding C3H/HeN Mice. Apmis 105, 838–842. doi: 10.1111/j.1699-0463.1997.tb05092.x
Nakano, H., Burgents, J. E., Nakano, K., Whitehead, G. S., Cheong, C., Bortner, C. D., et al. (2013). Migratory Properties of Pulmonary Dendritic Cells are Determined by Their Developmental Lineage. Mucosal Immunol. 6, 678–691. doi: 10.1038/mi.2012.106
Ng, S. L., Teo, Y. J., Setiagani, Y. A., Karjalainen, K., Ruedl, C. (2018). Type 1 Conventional Cd103(+) Dendritic Cells Control Effector Cd8(+) T Cell Migration, Survival, and Memory Responses During Influenza Infection. Front. Immunol. 9, 3043. doi: 10.3389/fimmu.2018.03043
Pauls, K., Schön, M., Kubitza, R. C., Homey, B., Wiesenborn, A., Lehmann, P., et al. (2001). Role of Integrin alphaE(CD103)beta7 for Tissue-Specific Epidermal Localization of CD8+ T Lymphocytes. J. Invest. Dermatol. 117, 569–575. doi: 10.1046/j.0022-202x.2001.01481.x
Plantinga, M., Guilliams, M., Vanheerswynghels, M., Deswarte, K., Branco-Madeira, F., Toussaint, W., et al. (2013). Conventional and Monocyte-Derived CD11b(+) Dendritic Cells Initiate and Maintain T Helper 2 Cell-Mediated Immunity to House Dust Mite Allergen. Immunity 38, 322–335. doi: 10.1016/j.immuni.2012.10.016
Roe, M. M., Hashimi, M., Swain, S., Woo, K. M., Bimczok, D. (2020). P38 MAPK Signaling Mediates Retinoic Acid-Induced CD103 Expression in Human Dendritic Cells. Immunology 161, 230–244. doi: 10.1111/imm.13246
Roe, M. M., Swain, S., Sebrell, T. A., Sewell, M. A., Collins, M. M., Perrino, B. A., et al. (2017). Differential Regulation of CD103 (αe Integrin) Expression in Human Dendritic Cells by Retinoic Acid and Toll-like Receptor Ligands. J. Leukoc. Biol. 101, 1169–1180. doi: 10.1189/jlb.1MA0316-131R
Sathe, P., Pooley, J., Vremec, D., Mintern, J., Jin, J. O., Wu, L., et al. (2011). The Acquisition of Antigen Cross-Presentation Function by Newly Formed Dendritic Cells. J. Immunol. 186, 5184–5192. doi: 10.4049/jimmunol.1002683
Schlitzer, A., McGovern, N., Ginhoux, F. (2015). Dendritic Cells and Monocyte-Derived Cells: Two Complementary and Integrated Functional Systems. Semin. Cell Dev. Biol. 41, 9–22. doi: 10.1016/j.semcdb.2015.03.011
Schlitzer, A., Sivakamasundari, V., Chen, J., Sumatoh, H. R., Schreuder, J., Lum, J., et al. (2015). Identification of cDC1- and Cdc2-Committed DC Progenitors Reveals Early Lineage Priming at the Common DC Progenitor Stage in the Bone Marrow. Nat. Immunol. 16, 718–728. doi: 10.1038/ni.3200
Sen-Kilic, E., Blackwood, C. B., Boehm, D. T., Witt, W. T., Malkowski, A. C., Bevere, J. R., et al. (2019). Intranasal Peptide-Based Fpva-KLH Conjugate Vaccine Protects Mice From Pseudomonas Aeruginosa Acute Murine Pneumonia. Front. Immunol. 10, 2497. doi: 10.3389/fimmu.2019.02497
Shane, H. L., Reagin, K. L., Klonowski, K. D. (2018). The Respiratory Environment Diverts the Development of Antiviral Memory Cd8 T Cells. J. Immunol. 200, 3752–3761. doi: 10.4049/jimmunol.1701268
Shekhar, S., Peng, Y., Wang, S., Yang, X. (2018). CD103+ Lung Dendritic Cells (Ldcs) Induce Stronger Th1/Th17 Immunity to a Bacterial Lung Infection Than CD11b(hi) Ldcs. Cell Mol. Immunol. 15, 377–387. doi: 10.1038/cmi.2016.68
Sichien, D., Scott, C. L., Martens, L., Vanderkerken, M., Van Gassen, S., Plantinga, M., et al. (2016). Irf8 Transcription Factor Controls Survival and Function of Terminally Differentiated Conventional and Plasmacytoid Dendritic Cells, Respectively. Immunity 45, 626–640. doi: 10.1016/j.immuni.2016.08.013
Tavernier, S. J., Osorio, F., Janssens, S., Lambrecht, B. N. (2015). Isolation of Splenic Dendritic Cells Using Fluorescence-activated Cell Sorting. Bio Protoc. 5. doi: 10.21769/bioprotoc.1415
Tweedle, J. L., Deepe, G. S., Jr. (2018). Tumor Necrosis Factor Alpha Antagonism Reveals a Gut/Lung Axis That Amplifies Regulatory T Cells in a Pulmonary Fungal Infection. Infect. Immun. 86, e00109–18. doi: 10.1128/iai.00109-18
Keywords: dendritic cell (DC), Pseudomonas aeruginosa, Gram-negative bacteria, lung inflammation, lipopolysaccharide, CD103, granulocyte-macrophage colony-stimulating factor
Citation: Brassard J, Roy J, Lemay A-M, Beaulieu M-J, Bernatchez E, Veillette M, Duchaine C and Blanchet M-R (2021) Exposure to the Gram-Negative Bacteria Pseudomonas aeruginosa Influences the Lung Dendritic Cell Population Signature by Interfering With CD103 Expression. Front. Cell. Infect. Microbiol. 11:617481. doi: 10.3389/fcimb.2021.617481
Received: 14 October 2020; Accepted: 15 June 2021;
Published: 06 July 2021.
Edited by:
Chang H. Kim, University of Michigan, United StatesReviewed by:
Even Fossum, Oslo University Hospital, NorwayAllan Mowat, University of Glasgow, United Kingdom
Susan Kovats, Oklahoma Medical Research Foundation, Oklahoma Medical Research Foundation
Copyright © 2021 Brassard, Roy, Lemay, Beaulieu, Bernatchez, Veillette, Duchaine and Blanchet. This is an open-access article distributed under the terms of the Creative Commons Attribution License (CC BY). The use, distribution or reproduction in other forums is permitted, provided the original author(s) and the copyright owner(s) are credited and that the original publication in this journal is cited, in accordance with accepted academic practice. No use, distribution or reproduction is permitted which does not comply with these terms.
*Correspondence: Marie-Renée Blanchet, TWFyaWUtUmVuZWUuQmxhbmNoZXRAY3JpdWNwcS51bGF2YWwuY2E=