- 1Biodiversity Research Centre, Earth and Life Institute, Université catholique de Louvain (UCLouvain), Louvain-la-Neuve, Belgium
- 2Institut de Recherche sur la Biologie de l’insecte, UMR 7261, CNRS, Université de Tours, Tours, France
- 3Center for Applied Molecular Technologies, Institute of Experimental and Clinical Research, Université catholique de Louvain (UCLouvain), Woluwe-Saint-Lambert, Belgium
- 4Univ Lyon, INSA-Lyon, INRAE, BF2i, UMR203, F-69621, Villeurbanne, France
- 5Louvain Institute of Biomolecular Science and Technology (LIBST), Université catholique de Louvain (UCLouvain), Louvain-la-Neuve, Belgium
- 6Walloon Center of Industrial Biology, Université de Liège, Liège, Belgium
- 7Laboratory of Food and Environmental Microbiology, Earth and Life Institute, Université catholique de Louvain (UCLouvain), Louvain-la-Neuve, Belgium
- 8Evolutionary Biology and Ecology, Université Libre de Bruxelles, Brussels, Belgium
Mutualistic associations between insects and heritable bacterial symbionts are ubiquitous in nature. The aphid symbiont Serratia symbiotica is a valuable candidate for studying the evolution of bacterial symbiosis in insects because it includes a wide diversity of strains that reflect the diverse relationships in which bacteria can be engaged with insects, from pathogenic interactions to obligate intracellular mutualism. The recent discovery of culturable strains, which are hypothesized to resemble the ancestors of intracellular strains, provide an opportunity to study the mechanisms underlying bacterial symbiosis in its early stages. In this study, we analyzed the genomes of three of these culturable strains that are pathogenic to aphid hosts, and performed comparative genomic analyses including mutualistic host-dependent strains. All three genomes are larger than those of the host-restricted S. symbiotica strains described so far, and show significant enrichment in pseudogenes and mobile elements, suggesting that these three pathogenic strains are in the early stages of the adaptation to their host. Compared to their intracellular mutualistic relatives, the three strains harbor a greater diversity of genes coding for virulence factors and metabolic pathways, suggesting that they are likely adapted to infect new hosts and are a potential source of metabolic innovation for insects. The presence in their genomes of secondary metabolism gene clusters associated with the production of antimicrobial compounds and phytotoxins supports the hypothesis that S. symbiotia symbionts evolved from plant-associated strains and that plants may serve as intermediate hosts. Mutualistic associations between insects and bacteria are the result of independent transitions to endosymbiosis initiated by the acquisition of environmental progenitors. In this context, the genomes of free-living S. symbiotica strains provide a rare opportunity to study the inventory of genes held by bacterial associates of insects that are at the gateway to a host-dependent lifestyle.
Introduction
Many insects thriving on unbalanced diets have evolved intimate relationships with symbiotic bacteria that are maternally inherited throughout generations and can influence many aspects of their hosts’ biology (Engel and Moran, 2013; McFall-Ngai et al., 2013; Oliver et al., 2014). Certain symbionts are obligate nutritional partners for their host because they produce essential nutrients that are deficient in their diet, such as amino acids and vitamins (Douglas, 1998; Russell et al., 2017). Insects can also harbor facultative bacterial partners with various effects on the host phenotype, depending on the ecological context (Oliver et al., 2010; Lo et al., 2016). It is now well established that those symbionts, whether obligate or facultative, evolved from originally free-living bacterial lineages (Husník et al., 2011; Clayton et al., 2012; Manzano-Marín et al., 2020) and that adaptation to a host-restricted lifestyle is accompanied by a reductive evolution of their genome (van Ham et al., 2003; Burke and Moran, 2011; Nikoh et al., 2011; Sloan and Moran, 2012; Latorre and Manzano-Marín, 2017). The isolation of bacterial strains capable of living freely outside of insects but related to insect endosymbionts (including members of the genera Sodalis and Arsenophonus) suggests the presence of insect symbiont progenitors in the environment (Bressan et al., 2009; Clayton et al., 2012). In addition to this, recent experimental work on plant-sucking stinkbugs (Insecta: Hemiptera) has demonstrated that extracellular gut symbionts of mutualistic nature residing in specialized gut-associated structures arise from free-living bacteria taken up from the environment (Kikuchi et al., 2011; Hosokawa et al., 2016a; Hosokawa et al., 2016b). These findings provide tangible evidence for the existence of an environmental pool of bacteria from which new intimate partnerships between insects and bacteria can emerge and identifying potential progenitors of endosymbionts is essential to gain finer insight into the multiple evolutionary trajectories leading to bacterial mutualism.
Aphids (Hemiptera: Aphididae) have long been model systems for addressing functional diversity and evolution of bacterial mutualism in insects (Buchner, 1965; Feldhaar, 2011; Baumann et al., 2013). Almost all aphids harbor an obligate symbiont, Buchnera aphidicola, that provides essential amino acids and vitamins lacking in the plant phloem sap (Douglas, 1998; Russell et al., 2017). B. aphidicola symbionts have a highly reduced and static genome, resulting from about 150 millions of years of coevolution (van Ham et al., 2003) and isolation in dedicated insect cells called bacteriocytes (Braendle et al., 2003). In some aphid species, B. aphidicola has lost some metabolic capabilities and nutrition also relies on co-obligate partners (Manzano-Marín et al., 2016; Meseguer et al., 2017; Monnin et al., 2020). In addition to nutritional symbionts, many aphid species can be associated with heritable facultative symbionts that can have various effects on the host phenotype, such as alteration of the body color (Tsuchida et al., 2010), tolerance to heat-stress (Burke et al., 2010) or protection from natural enemies (Scarborough et al., 2005; Oliver et al., 2009). These facultative associations are often regarded as intermediate stages between the free-living and the mutualistic lifestyle (Latorre and Manzano-Marín, 2017). Indeed, compared to B. aphidicola, facultative symbionts hold moderately reduced genomes and often retain mechanisms similar to those of opportunistic pathogens to infect a wider variety of host tissues and colonize new hosts (Dale and Moran, 2006; Degnan et al., 2009).
The aphid-associated symbiont Serratia symbiotica has become a valuable model for dissecting the mechanisms underlying endosymbiosis emergence and evolution because the diversity of S. symbiotica reported to date provides a comprehensive picture of the various associations that bacteria can share with insects. Reported S. symbiotica strains are associated with various host phenotypes and hold genomes of contrasting sizes and features reflecting their lifestyle, which ranges from the free-living lifestyle to the obligate intracellular mutualism (Burke and Moran, 2011; Foray et al., 2014; Manzano-Marín and Latorre, 2016; Latorre and Manzano-Marín, 2017). For instance, strains of S. symbiotica associated with the pea aphid Acyrthosiphon pisum (subfamily Aphidinae) hold mildly-reduced genomes and are of a facultative nature as they provide protective effects against high temperatures and parasitoid wasps (Oliver et al., 2003; Burke et al., 2010; Burke and Moran, 2011) and may have some nutritional benefits (Koga et al., 2003; Nikoh et al., 2019). Other strains of S. symbiotica associated with aphid species of the subfamilies Lachninae and Chaitophorinae are involved in co-obligate associations, hold rather small genomes and compensate for the reduction of B. aphidicola’s metabolic capacity for the biosynthesis of nutrients, particularly biotin (vitamin B7), riboflavin (vitamin B2) and the amino acid tryptophan (Lamelas et al., 2011a; Lamelas et al., 2011b; Manzano-Marín and Latorre, 2014; Manzano-Marín and Latorre, 2016; Manzano-Marín et al., 2016; Manzano-Marín et al., 2017; Meseguer et al., 2017; Manzano-Marín et al., 2018; Monnin et al., 2020). Genomic analyses have indicated that this dependence of aphids on co-obligate strains has likely appeared independently on many occasions in the evolutionary history of aphids and has evolved in a very dynamic fashion, with S. symbiotica being acquired and replaced several times during the diversification of their host aphids (Manzano-Marín et al., 2017; Meseguer et al., 2017; Manzano-Marín et al., 2020). The recruitment of new bacterial partners and the repeated replacement of pre-existing intracellular symbionts is now considered as a recurrent evolutionary phenomenon that occurs in many phloem-feeding insects (Koga and Moran, 2014; Husnik and McCutcheon, 2016; Mao and Bennett, 2020).
Although there is compelling evidence that new mutualistic associations between insects and bacteria are continually forming in nature (Kikuchi et al., 2011; Clayton et al., 2012; Hosokawa et al., 2016a; Hosokawa et al., 2016b; Matsuura et al., 2018), little is known about how this occurs in aphids. This is partly due to the fact that a very limited number of free-living bacteria displaying features allowing identifying them as putative ancestors of facultative and obligate intracellular symbionts have been discovered so far. However, several S. symbiotica strains with free-living abilities have recently been discovered and they offer the rare opportunity to study the inventory of genes held by insect-associated bacteria just prior to the acquisition of an host-restricted lifestyle (Foray et al., 2014; Grigorescu et al., 2018; Renoz et al., 2020). Compared to their intracellular facultative and co-obligate relatives that are sheltered in bacteriocytes and/or sheath cells, these culturable S. symbiotica strains harbor larger genomes (Foray et al., 2014; Renoz et al., 2020) and have the propensity to rapidly invade the digestive tract of aphids (Renoz et al., 2015; Pons et al., 2019b). Once they have invaded their host’s gut, these strains cause fitness costs and become true gut pathogens, unable to establish persistent maternal transmission and transmitted primarily via the fecal-oral route (Pons et al., 2019b; Perreau et al., 2021). These strains are able to invade plants and circulate through the phloem sap before infecting aphids (Pons et al., 2019a). Interestingly, in a complementary fashion, field studies have shown that S. symbiotica can naturally reside in plants as well as in the digestive tract of various insect groups (Renoz et al., 2019; Pons et al., 2021, pre-print). All these findings suggest the existence of S. symbiotica strains that have the ability to circulate in different environmental compartments and to be acquired horizontally, and are therefore potential progenitors of stably maintained mutualistic endosymbionts. To shed light on the origin of the aphid symbiont S. symbiotica and the genomic traits that could predispose to the acquisition of a host-restricted lifestyle (i.e., exaptations in S. symbiotica for aphid symbiosis), we improved and analyzed the whole-genome sequences of three S. symbiotica strains that retain free-living abilities and isolated from different aphid strains and species, and performed comparative genomic analyses that also include the genomes of 12 host-restricted S. symbiotica strains. Our study suggests that the mutualistic strains associated with aphids have evolved from S. symbiotica strains that originally inhabited plants. The three culturable strains still hold a wide range a virulence factor that may explain their pathogenicity to aphids, but are at the same time characterized by genomic traits that predispose them to become mutualistic partners.
Materials and Methods
Genome Sequencing and Assemblies
The whole-genome sequences of three S. symbiotica strains previously published (Foray et al., 2014; Renoz et al., 2020) were improved as described below. The whole-genome sequence of the S. symbiotica strain CWBI-2.3T (hereafter SsAf2.3) isolated from the black bean aphid Aphis fabae (Foray et al., 2014) was improved using the Pacific Biosciences RS sequencing technology (KeyGene, Wageningen, Netherlands). A 20-kb library was sequenced on two single-molecule real-time (SMRT) cells. The reads were assembled using HGAP 2.1 de novo assembly pipeline. The few final gaps and long repeats found in the resulting draft genome were then covered by PCR. To validate the presence and size of the plasmids identified in silico, a plasmid profile was determined using a modified Eckhardt agarose gel electrophoresis approach (Eckhardt, 1978).
The genomes of the S. symbiotica strains 24.1 (hereafter SsAf24.1) isolated from A. fabae and Apa8A1 (hereafter SsApa8A1) isolated from the sage aphid Aphis passeriniana (Grigorescu et al., 2018) had been previously sequenced by whole-genome paired-end sequencing using Illumina on a HiSeq 4000 platform with 2 × 100 paired-end reads (Illumina, San Diego, CA, USA) and a sequencing depth >100x (Renoz et al., 2020). These data were complemented by long reads obtained using a ONT MinION sequencer. Prior to the MinION nanopore sequencing, the integrity of genomic DNA was evaluated using an Agilent 2200 TapeStation with the Genomic DNA ScreenTape (Agilent). Both samples displayed a DNA Integrity Number value ≥9.5. Libraries were then prepared from 400 ng of genomic DNA using the Rapid Barcoding Sequencing kit (SQK-RBK004) protocol (Oxford Nanopore Technologies). Briefly, the DNA molecules were cleaved by a transposase and barcoded tags were attached to the cleaved ends. The barcoded samples were then pooled and Rapid Sequencing Adapters were added to the tagged ends. The pooled libraries were sequenced into a FLO-MIN106 (R9.4.1) flow cell for a 48h run according to manufacturer’s instruction. After the run, fast5 files were basecalled on the MinIT using default settings in MinKNOWv18.12 and Guppy v3.0.3 and a High Accuracy (HAC) Flip-Flop methodology. Finally, a hybrid assembly strategy combining the data generated by the Illumina and ONT MinION sequencing approaches was performed using Unicycler v0.4.8 (Wick et al., 2017). Raw sequence reads have been deposited in the European Nucleotide Archive (studies with accession numbers: PRJEB44297 for SsAf2.3, PRJEB44257 and PRJEB44266 for SsAf24.1, and PRJEB44268 and PRJEB44273 for SsApa8A1).
Genome Annotation and Comparative Analyses
The three genomes were annotated with the Prokaryotic Genome Annotation Pipeline (PGAP) (Tatusova et al., 2016). To perform genomic comparative analyses that include the other available sequenced genomes of S. symbiotica, the datasets corresponding to their annotation were downloaded from NCBI (GenBank annotations were preferred when available) (Figure 1). The pan- and core-genomes were computed using the EDGAR software platform (Blom et al., 2016) and the protein sequences were functionally annotated using eggNOG mapper v2 (Huerta-Cepas et al., 2017). The fifteen available genomes of S. symbiotica were deposited on the MicroScope platform (Médigue et al., 2019; Vallenet et al., 2020) and their metabolic capabilities compared using MicroCyc, a collection of microbial Pathway/Genome Databases (PGDBs) based on the MetaCyc database and specifically dedicated to the analysis of microbial pathways (Caspi et al., 2020). Metabolic reconstructions generated by MicroCyc were manually verified and CDSs were considered as pseudogenes when their length was less than 80% of the one of their orthologues in the other genomes (Lerat and Ochman, 2005; Burke and Moran, 2011). AntiSMASH v5.0.0 and the MIBiG database were used to predict regions coding for complete secondary metabolites in the different genomes (Blin et al., 2019). Virulence genes were identified using the virulence factor database (VFDB, http://www.mgc.ac.cn/VFs/) (Chen et al., 2005; Liu et al., 2019): datasets of virulence genes were downloaded and BLASTp were performed (minimum 50% aa identity, 80% align. coverage). Complete secretion systems were identified with MacSyFinder (Abby et al., 2014). Insertion sequences (hereafter IS) predictions were generated with ISsaga (Varani et al., 2011). CRISPR-Cas systems were detected using CRISPRCasFinder and MacSyFinder (Abby et al., 2014; Couvin et al., 2018). Intact prophage regions were predicted with PHASTER (Arndt et al., 2016). Assemblies and annotations are available on Genbank under the following accession numbers: CP050857-CP050857 for SsAf2.3, CP072650-CP072652 for SsAf24.1 and WSPO00000000.3 for SsApa8A1.
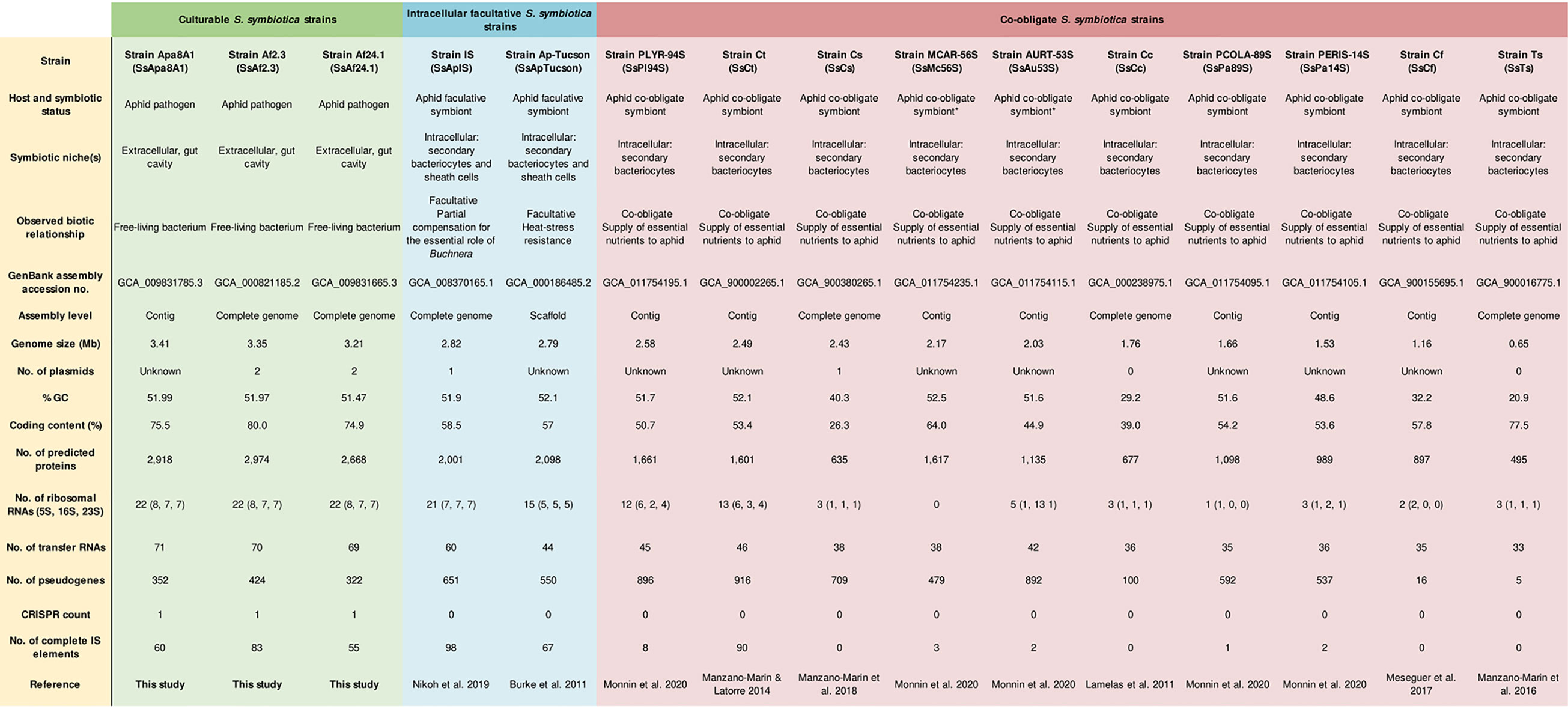
Figure 1 General genomic features of S. symbiotica strains ordered by genome size. *The nature of the relationship between aphids and strains SsMc56S and SsAu53S is currently under debate.
Molecular Phylogenetic Analyses
To infer the origin of the culturable S. symbiotica strains and to reconstruct the phylogenetic history of the symbiosis between aphids and S. symbiotica, we built a phylogenetic tree using a set of single-copy core concatenated protein sequences shared across all S. symbiotica strains and several Serratia spp. that are representative of the genus and include plant- and animal-associates (Table S1). All protein sequences of the different species and strains were analyzed with OrthoFinder (version 2.3.12) (Emms and Kelly, 2015; Emms and Kelly, 2019) to identify orthologues and orthogroups. A set of 221 single-copy genes present in each bacterial strain was then used for phylogeny estimation. The alignments of this set of genes were concatenated using FASconCAT-G (Kück and Meusemann, 2010). By initially defining each gene as a separate partition, we performed a ModelFinder analysis (Kalyaanamoorthy et al., 2017) on the concatenated alignment with IQ-TREE (version 1.6.12) (Nguyen et al., 2015); (options -m TESTMERGEONLY and -rcluster 10; set of models evaluated: mrbayes) to select the best partitioning of the alignment and the best model for each partition (-p option, allowing each partition to have its own evolution rate). A maximal likelihood analysis was then conducted on the best partitioning and modelling scheme (-p option) with IQ-TREE (MPI version). Branch support was assessed using ultrafast bootstrapping (UFBoot; (Minh et al., 2013; Hoang et al., 2018) and an approximate likelihood ratio test (SH-aLRT; (Anisimova et al., 2011).
Results and Discussion
Genome Sequencing of the Culturable S. symbiotica Strains
The sequencing of the SsAf2.3 strain using the Pacific Biosciences RS sequencing technology yielded 144,745 reads for a mean read length of 6,126 bp and resulted in a 210x average genome coverage. This allowed us to obtain an improved genome assembly version compared to the one previously published (Foray et al., 2014) in that the new version offered us the opportunity to circularize the chromosome and to identify two plasmids, namely pSsAf2.3-1 (82.6 kb, 57 CDSs) and pSsAf2.3-2 (116.7 kb, 121 CDSs). The presence and size of the plasmids identified in silico were validated by a plasmid profile generated by a modified Eckhardt agarose gel electrophoresis approach (Eckhardt, 1978) (Figure S1). The sequencing of the strains SsAf24.1 and SsApa8A1, performed using the MinION nanopore sequencing approach, generated a total of 944,030 and 686,673 reads with an average read length of 4.04 and 8.18 kb, respectively. Combining Illumina and MinION sequencing data using Unicycler improved the previously published genomes. This allowed us to circularize the chromosome of the SsAf24.1 strain and to identify to plasmids, namely pSsAf24.1-1 (104.9 kb, 106 CDSs) and pSsAf24.1-2 (54.8 kb, 47 CDSs). The SsApa8A1 genome consists of 7 contigs. Both genomes were associated with a high coverage with Illumina (>100x) and MinION data (>1000x).
General Genomic Features of Culturable S. symbiotica Strains
The culturable strains SsAf2.3, SsAf24.1 and SsApa8A1 exhibit a similar genome organization (size, %GC, coding content, number of tRNAs, etc.) (Figure 1). Their genomes have lengths comprised between 3.2 and 3.4 Mb, and contain between 2,500 and 3,000 CDSs covering about 75% of the entire genome. They are larger than those of the intracellular facultative and co-obligate S. symbiotica strains sequenced so far and contain a higher proportion of coding DNA. However, compared to genome sizes of other Serratia species, which range from 4.9 to 5.6 Mb, the ones of the three culturable strains analyzed in our work are remarkably smaller (Table S1). Compared to the genomes of the opportunistic free-living pathogen S. marcescens Db11 (hereafter Db11) and intracellular co-obligate S. symbiotica strains, the genomes of the culturable S. symbiotica strains show significant enrichment in pseudogenes and IS elements (Figure 1), suggesting, despite their free-living capabilities, an ongoing reduction of their genome and a shift from a free-living to a host-restricted lifestyle (Moran and Plague, 2004; Toft and Andersson, 2010; Latorre and Manzano-Marín, 2017). Synteny’s analyses highlight an increase of rearrangements in that the genomes of culturable S. symbiotica strains compared to free-living close relatives of the genus (Figure S2).
Among the CDSs that could be classified in at least one EGGNOG group, 95% (SsAf2.3), 96% (SsAf24.1) and 94% (SsApa8A1) were assigned to a biological function. The hierarchical clustering of COG (Clusters of Orthologous Groups of proteins) profiles reveals three main clusters among S. symbiotica strains (Figure 2). Culturable strains compose one cluster with SmDb11, characterized by an enrichment of CDSs of unknown function (category S) that are probably involved in secondary and non-essential functions. The relative abundance of CDSs associated with virulence and motility (categories U and N, respectively) is also higher in the culturable strains than in the other intracellular host-restricted strains. A second cluster is composed of co-obligate S. symbiotica strains of clade B. As previously proposed (Manzano-Marín et al., 2012), the strains from this clade are featured by a streamlined genome conserving genes involved in basic cellular functions, like protein synthesis (category J). Category M is also strongly confirmed in this second cluster, as these strains conserved a capacity to synthetize their own cell membrane despite massive erosion of their genome. Finally, facultative and co-obligate strains compose a third cluster. They show intermediate profiles compared to the other two clusters, with moderate enrichment of housekeeping genes (category J) but still a large proportion of non-essential CDSs with unknown functions. Category L is also highly conserved in this cluster, as these strains retain CDSs involved in genome replication and repair systems. These results support the hypothesis that symbiotic bacteria clustered by GOC categories tend to have similar ecological niches (Santos-Garcia et al., 2014). However, phylogenetic history seems to play an important role as the co-obligate S. symbiotica strains of clade B constitute a separate cluster from the other co-obligate strains. On the other hand, it is also possible that the monophyly of these strains is the result of a long-branch attraction (LBA) artefact (Bergsten, 2005).
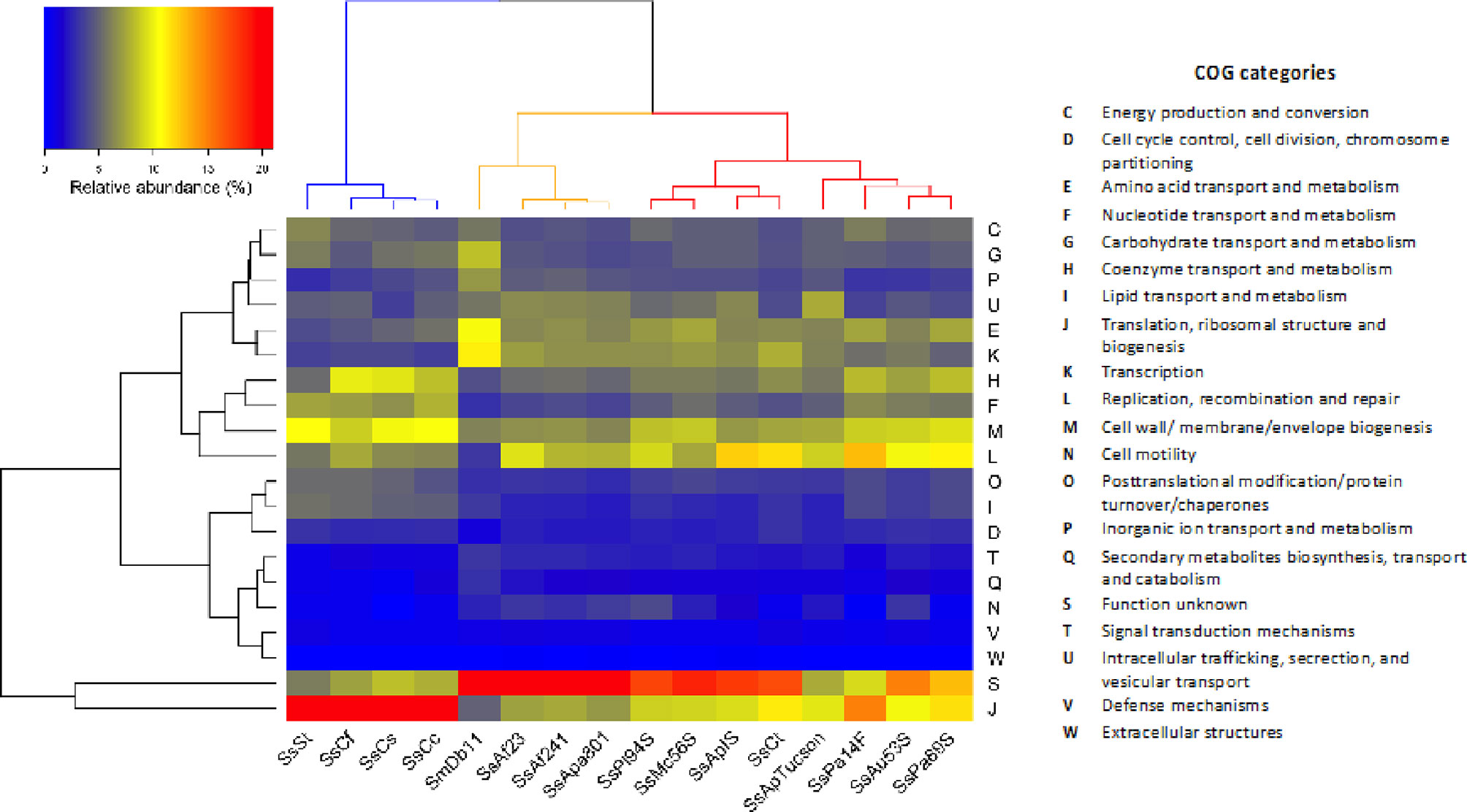
Figure 2 Functional profiles of selected S. symbiotica strains and S. marcescens Db11. Two-way hierarchical clustering heatmap showing the relative abundance of CDSs in each COG categories according to the total number of CDSs in each genome. Cladogram at the top highlights three main clusters among S. symbiotica strains: one cluster including only S. symbiotica from Clade B (left cluster); one cluster grouping all culturable strains with S. marcescens Db11 (middle cluster); and one cluster including facultative and co-obligate S. symbiotica strains (right cluster).
The genomes of the three culturable strains share most genes and pathways with a core-genome composed of 2,142 CDSs (Figure 3 and Table S2). The strain-specific genome of each strain constitutes only about 10% of the CDSs and most strain-specific genes encode CDSs with no assigned function (Table S3). The most notable differences between the strains were found in phage proteins, transposases, conjugal transfer proteins and in the proteins involved in the toxin–antitoxin (TA) systems (known to participate in plasmid stability), stress management, biofilm formation and antibiotic resistance (Van Melderen and Saavedra De Bast, 2009). Interestingly, the three genomes also differ in their respective repertoires of genes encoding proteins related to the flagellar apparatus. Indeed, the genome of SsApa8A1 contains many more flagellate-related genes than the genomes of SsAf2.3 and SsAf24.1. This point is discussed in more detail in the section devoted to the virulence factors encoded by the different S. symbiotica genomes. Overall, the genomes show few differences in the content of CDSs and little variation in pathways of considerable biological relevance, suggesting a single evolutionary origin of the three strains and a possible adaptation to similar niches: the digestive tract of the aphids and probably the phloem sap through which they can transit (Pons et al., 2019a; Pons et al., 2019b).
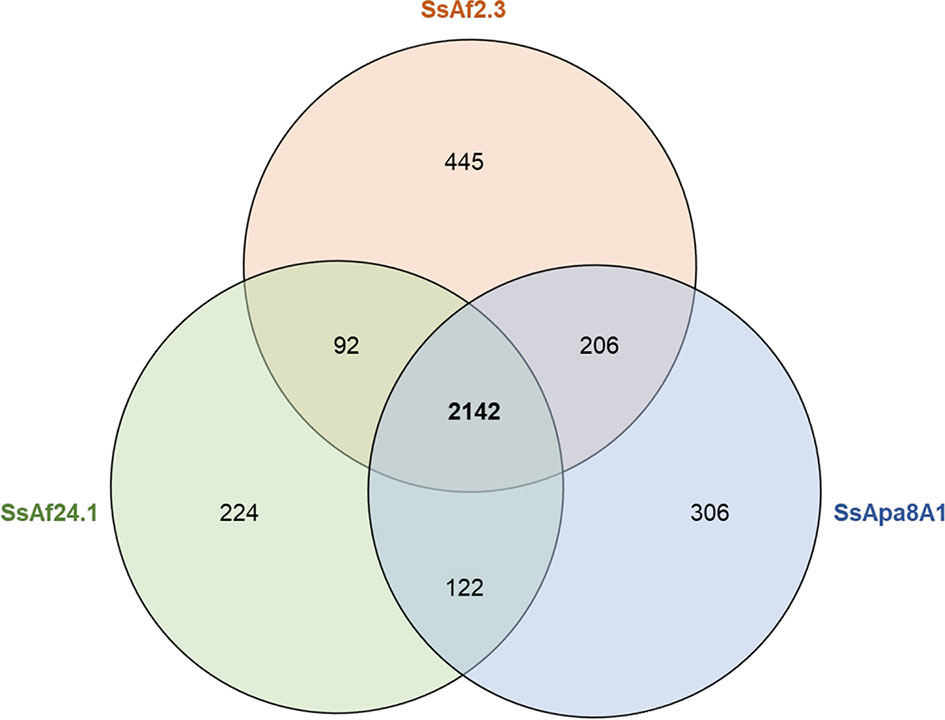
Figure 3 Venn diagram comparing the gene inventories of the three culturable S. symbiotica genomes computed by EDGAR (Blom et al., 2016) based on reciprocal best BLAST hits of the coding sequences predicted by PGAP (Tatusova et al., 2016).
Genomic Comparison Between Culturable and Intracellular Host-Restricted Strains
The core-genome defined from the fifteen S. symbiotica sequenced genomes is composed of 302 protein-coding genes, identified as housekeeping genes (Table S4). Beyond these genes, our analyses predicted that the cvpA (colicin V production accessory protein) gene is present in all the genomes (except the SsMC-56S genome for which a chromosome fragment is missing) (Figure S3 and Table S5). cvpA encodes for an inner-membrane protein primarily described as a key factor for colicin V production by members of the Enterobacteriaceae to kill competing bacteria (Fath et al., 1989; Gérard et al., 2005; Zaini et al., 2008). However, the synthesis of colicin V also requires the expression of several plasmid-borne genes (cvaA, cvaB, cvaC, and cvi) (Gérard et al., 2005) that have not been detected in the genomes we studied here. An alternative role of cvpA in the context of bacterial mutualism has never been investigated, whereas cvpA has been conserved in the genomes of many endosymbiotic bacteria, including the obligate aphid symbiont B. aphidicola (Charles et al., 2011) and the obligate tsetse fly symbiont Wigglesworthia (Prickett et al., 2006), but also symbiotic bacteria associated with deep-sea tubeworms and corals (Gardebrecht et al., 2012; Carlos et al., 2014).
Recent studies have shown that cvpA contribute to host colonization by pathogenic bacteria. The cvpA-purF locus is required for intracellular replication of uropathogenic E. coli by promoting the biosynthesis of purine nucleotides necessary for survival in an intracellular niche (Shaffer et al., 2017). However, unlike cvpA, purF was lost in S. symbiotica strains with the most eroded genomes (Figure S3 and Table S5), while it is essential for this biosynthesis of purine nucleotides. cvpA may also play a role in biofilm development (Hadjifrangiskou et al., 2012) and is required for colonization of the human intestine by enteric pathogens including Vibrio parahaemolyticus and Escherichia coli (Hubbard et al., 2016; Warr et al., 2021). It has been proposed that this membrane protein contributes to cell envelope homeostasis in response to hostile environmental conditions set up by the host to counter infection (Warr et al., 2021). In light of these recent findings, the conservation of cvpA in symbiotic bacteria, including those with an extremely small genome, may indicate that this gene helps bacterial symbionts cope with the different niches they may face, namely the gut, the hemolymph and the intracellular environment.
Origin of Aphid-S. symbiotica Symbioses and Acquisition Routes
There is increasing evidence to support the hypothesis that host-restricted symbionts are derived from free-living progenitors acquired in the environment and having been gradually and obligatorily integrated into the insect’s physiology and development (Kikuchi et al., 2007; Moya et al., 2008; Clayton et al., 2012; Hosokawa et al., 2016a; Manzano-Marín et al., 2020). The genus Serratia includes members living in a wide range of habitats including water, soil, plants, humans and invertebrates (Petersen and Tisa, 2013). Here, we first examined the evolutionary relationship between the three culturable strains with the other members of the genus and assessed the origin of relationships involving S. symbiotica by estimating a phylogenetic tree. This was based on the use of a set of single-copy core concatenated protein sequences shared between 1) all S. symbiotica strains, 2) strains representative of the diversity of the genus and reported as being associated with plants or animals, and 3) several other members of the Enterobacteriaceae. The fifteen S. symbiotica strains form a well-supported monophyletic group, sister to the groups of Serratia species described as plant and animal associates (Figure 4). However, the tree topology did not allow us to deduce the lifestyle of the last “common ancestor” of the S. symbiotica strains, i.e. whether this was associated with plants or animals, because the nature of the associated host seems to be versatile among the genus Serratia (Figure 4). Actually, Serratia ficaria, the closest relative of S. symbiotica, was originally found associated with fig trees, but the species remain poorly described and seems to be able to infect humans (Grimont et al., 1981; Anahory et al., 1998).
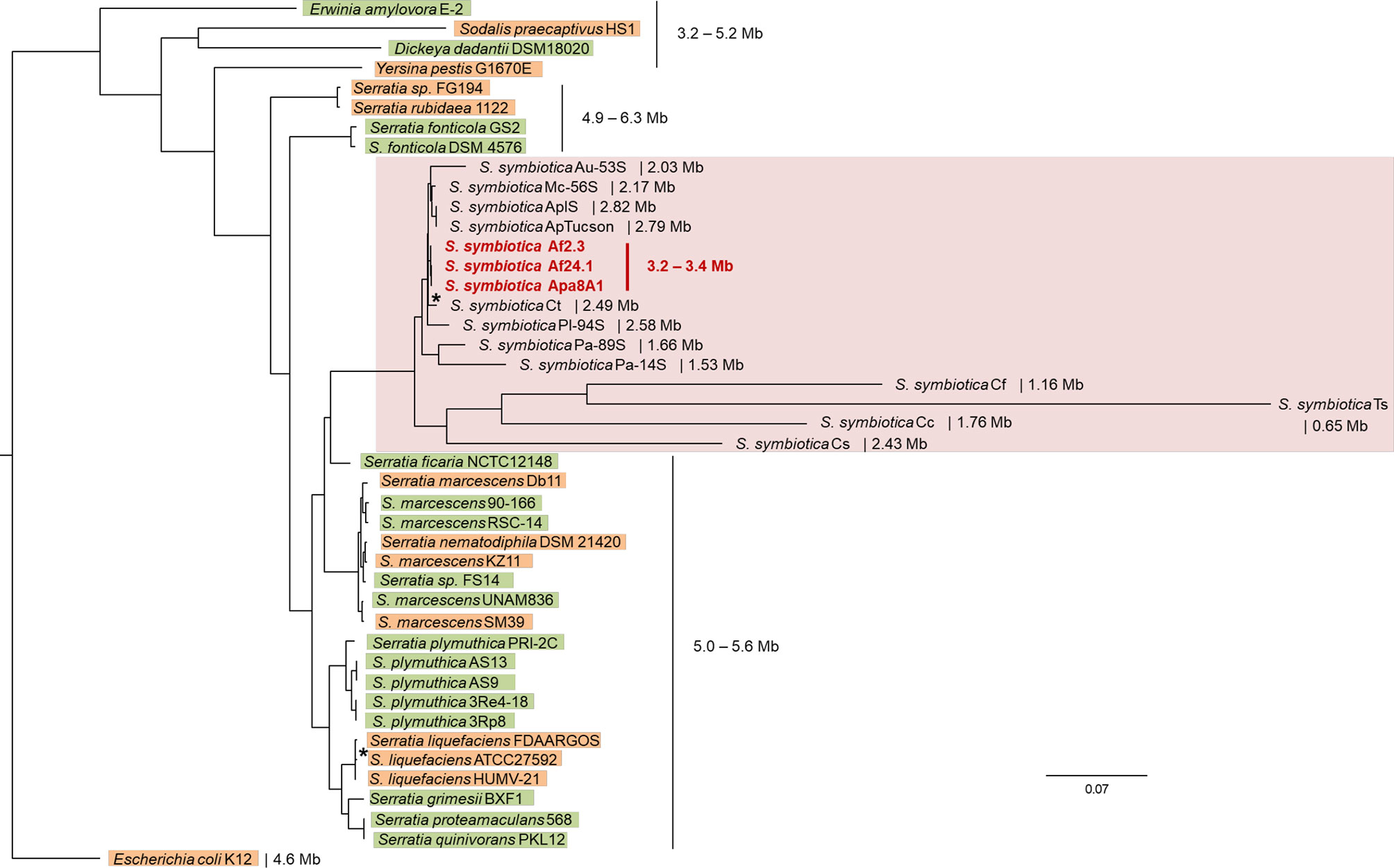
Figure 4 Phylogenetic positioning of the culturable S. symbiotica strains in the species and in the genus. The tree shown is the maximum likelihood topology inferred with IQ-TREE on 221 concatenated single-copy genes shared by all selected strains. Branch support (SH-aLRT and ultrafast bootstrap values) was > 99% for all nodes, except for two nodes associated with support values > 80% (*): (S. symbiotica Af2.3, S. symbiotica Af24.1, S. symbiotica Apa8A1 and S. symbiotica Ct) and S. liquefaciens FDAARGOS and S. liquefaciens ATCC27592). The S. symbiotica strains are highlighted in pink and the three cultured strains are denoted in bold red. Serratia members found associated with plants are highlighted in green and Serratia members found associated with animals are highlighted in orange. Partitions and associated models in a nexus file available in Supplementary Material.
To infer the origin of S. symbiotica, we therefore used a second strategy and examined the presence of gene clusters involved in the biosynthesis of secondary metabolites in the different genomes of S. symbiotica. Indeed, these gene clusters are supposed to reflect the environment that bacteria face and therefore to which they are adapted (Ziemert et al., 2014). Our analyses showed that several S. symbiotica genomes harbor gene clusters predicted to be intact and involved in the biosynthesis of secondary metabolites, particularly the genomes of the three culturable strains (Table S6). These bacteria house a gene cluster involved in the biosynthesis of massetolide A, a cyclic lipopeptide with antifungal properties that has been reported to be produced by endophytic strains of Pseudomonas and to contribute to host plant invasion (Tran et al., 2007; de Bruijn et al., 2008; Raaijmakers et al., 2010; Loper et al., 2012). Interestingly, the genome of the co-obligate strain SsCt also harbors a gene cluster involved in the biosynthesis of this lipopeptide. Similarly, the genome of strain SsAf2.3 contains a gene cluster involved in the synthesis of syringopeptin, a phytotoxin produced by members of the genus Pseudomonas and contributing to plant invasion (Ballio et al., 1991; Weeraratne et al., 2020). The genome of SsAf24.1 harbors a cluster involved in the synthesis of rhizomides, which are compounds associated with antimicrobial and cytotoxic properties and are notably produced by members of the genera Pseudomonas and Burkholderia (Wang et al., 2018; Kunakom and Eustáquio, 2019; Niem et al., 2019). The SsAf2.3 and SsApa8A1 genomes both host a gene cluster involved in the synthesis of luminmide, a cyclic pentapeptide associated with cytotoxic effects on eukaryotic cells (Li et al., 2019).
The presence in S. symbiotica genomes of gene clusters involved in the biosynthesis of certain secondary metabolites such as massetolide A and syringopeptin suggest that certain S. symbiotica members could have interacted and/or are still interacting with plants and that S. symbiotica symbionts residing in aphids may have evolved from strains that originally inhabited plants. Along the same lines, it has recently been demonstrated that SsAf2.3 can infect the roots of Vicia faba, before moving to the phloem sap and subsequently infecting the aphids that feed on it (Pons et al., 2019a). In addition, S. symbiotica has been detected from tissues of plants collected in the field (Pons et al., 2021, pre-print). In the light of these observations, we propose that plants may serve as alternative hosts and vectors for the free-living S. symbiotica strains, some of which can become intestinal associates of aphids before evolving into mutualistic symbionts. This scenario is further supported by the great versatility of members of the genus Serratia, which can be associated with both animals and plants [e.g. S. marcescens, which includes strains pathogenic to insects and plants as well as strains considered beneficial endophytes of plants (Petersen and Tisa, 2013; Eckelmann et al., 2018)]. Yet, the exact nature of the interaction between certain S. symbiotica strains and plants remains to be further studied: although it is assumed that cultivable strains may be associated with positive effects on these hosts by stimulating their growth (Pons et al., 2019a), they are unable to synthesize indole-3-acetic acid (IAA) (Figure 5), a plant hormone produced by many endophytic Serratia members (Neupane et al., 2012; Khan et al., 2017). Ongoing studies should clarify the nature of the relationships that culturable S. symbiotica strains have with plants and the effects associated with the secondary metabolites they may produce. Furthermore, a hypothesis that cannot be excluded is that these secondary metabolites are toxic to insects and contribute to the virulence of certain S. symbiotica strains. In such a context, culturable S. symbiotica strains represent fascinating models for understanding how bacteria develop cross-kingdom host jumps and exploit multiple hosts.
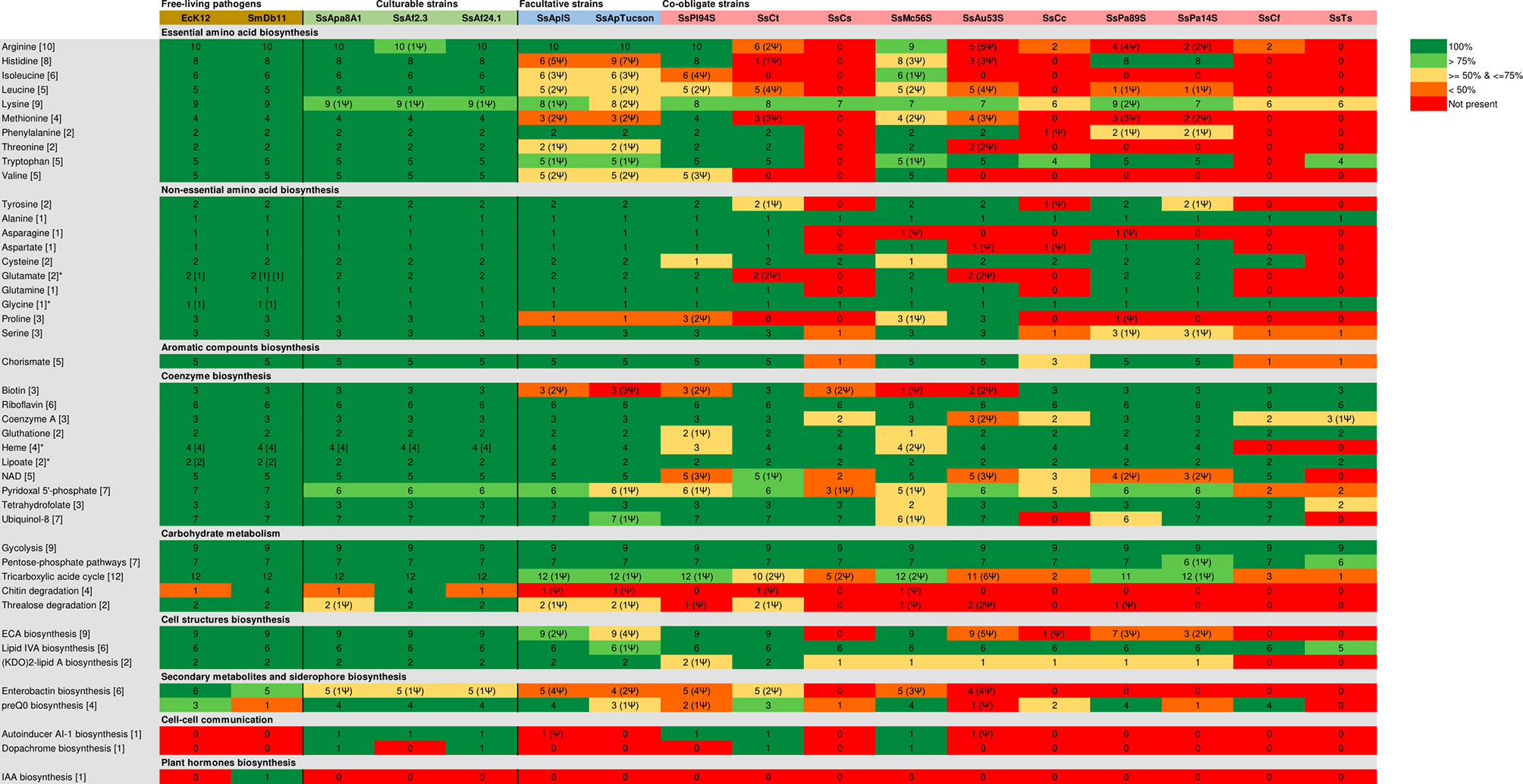
Figure 5 Comparison of metabolic genes repertories among the different S. symbiotica strains. The minimal number of genes for a metabolic pathway is shown in each of the brackets. Color represents the completeness of the MetaCyc pathways: dark green for 100%, light green for 99-75%, yellow for 74-50%, orange for 50-1% and red for 0%. Asterisk (*) denotes that the bacterium possesses a complete alternative pathway for the biosynthesis of the final product with the minimal number of genes for the alternative pathway indicated in the brackets. The numbers followed by Ψ in the round brackets indicate the number of pseudogenes found for the pathway.
Metabolic Capacities of Culturable S. symbiotica Strains
Compared to the facultative and co-obligate strains, the three culturable S. symbiotica strains display larger metabolic capabilities, that include intact pathways and then the potential to produce amino acids and vitamins. Indeed, all culturable strains are potentially capable of biosynthesizing most amino acids considered as essentials for metazoan cells, with a few exceptions (Figure 5). The argD gene is pseudogenized in all three genomes. However, the N-acetylornithine aminotransferase activity in lysine synthesis can also be achieved by the product of astC (present in the genomes of SsAf24.1 and that of SsApa8A1) and SerC (Lal et al., 2014) present in the genome of all three culturable strains, suggesting that all three strains are likely still capable to synthetize arginine and lysine.
The genomes of all three culturable strains have also intact pathways for the biosynthesis of all non-essential amino acids, as well as for many coenzymes. However, the epd gene, which is required for the erythrose-4-phosphate dehydrogenase activity in the biosynthesis of pyridoxal 5’-phosphate (the active form of vitamin B6), is absent from their genomes whereas it is present in the entomopathogens Escherichia coli K12 (EcK12) and SmDb11. However, this dehydrogenase activity may be performed by the product of gapA (Zhao et al., 1995), that is present and intact in all S. symbiotica genomes.
The metabolic pathways exhibited by S. symbiotica reflect the evolutionary trajectories of the strains and their contribution to the respective symbiotic system they are involved in. For example, the co-obligate strains associated with aphid species of the subfamilies Lachninae (SsCt, SsCs, SsCc, SsCf and SsTs) and Chaitophorinae (SsPl-94S, SsPa-89S and SsPa-14S) retained the capacity of producing biotin (B7 vitamin) and thus compensate for B. aphidicola’s inability to synthesize that coenzyme (Manzano-Marín and Latorre, 2016; Manzano-Marín et al., 2016; Manzano-Marín et al., 2017; Manzano-Marín et al., 2020) while the facultative strains SsApIS and SsApTucson associated with A. pisum have lost this ability (Figure 5). The larger metabolic potential of culturable strains for the biosynthesis of amino acids, vitamins and other coenzymes supports the hypothesis that those undergo the early stages of host adaptation and could be a source of metabolic innovations in emerging mutualistic associations.
Finally, another notable aspect concerning the metabolic capacities of three culturable S. symbiotica strains concerns their capacity to degrade chitin. SsAf2.3 is the only strain potentially capable of degrading chitin (Figure 5), the main component of the peritrophic matrix (PM) of the midgut that provides protection for the underlying epithelium from abrasion, toxins and pathogens (Hegedus et al., 2009; Merzendorfer et al., 2016). Many entomopathogenic members of the genus Serratia are capable of perforating the PM of insects via chitinases (Regev et al., 1996). However, SsAf2.3 was negative in tests for chitinase (Sabri et al., 2011).
Phages and CRISPR Arrays in the S. symbiotica Genomes
Genes that are important for host invasion and adaptation (e.g., genes encoding secretion systems and virulence factors) are often found in close proximity to mobile elements such as plasmids and phages that serve as intermediaries for the exchange of genetic material and the introduction of new functions into bacterial genomes (Clokie et al., 2011; Weldon and Oliver, 2016). In the context of the evolution of bacterial mutualism, bacteria in the early stages of host adaptation tend to harbor more phage regions than bacteria involved in the advanced stages of the process (Toft and Andersson, 2010). As expected, more intact phage regions were found in the genomes of the culturable strains than in the genomes of facultative and co-obligate strains (Table S7). In the symbiotic bacteria associated with aphids, increased attention has been paid to the lysogenic phage APSE (Acyrthosiphon pisum secondary endosymbiont), often found in the facultative symbiont Hamiltonella defensa where its presence is associated with protective effects against parasitoid wasps to the benefit of the aphid host (Oliver et al., 2009; Weldon and Oliver, 2016). This protection is based on bacteriophage-encoded toxins that hinder the development of parasitoid larvae (Oliver et al., 2012). No APSE-related regions were detected in the genomes of the three culturable strains as well as in the genomes of the intracellular strains, with the exception of SsCt where a region for which the most common phage hit was APSE-like. However, this result should be taken with caution because the DNA polymerase found in this predicted phage region is highly similar to DNA polymerases encoded by members of the genera Serratia, Yersinia, Erwinia and others.
CRISPR (clustered regularly interspaced short palindromic repeats) arrays and their associated proteins form adaptive immune systems that are present in most archaea and many bacteria and act against invading genetic elements (e.g., viruses and plasmids) (Makarova et al., 2011). CRISPR arrays were detected only in the genomes of the culturable strains SsAf2.3, SsAf24.1 and SsApa8A1, and Cas protein sequences have been found only in the SsAf24.1 genome (Table S8). These results suggest that the transition from a free-living to a host-restricted lifestyle also led to the elimination of the CRISPR defense mechanisms.
Secretion Systems Encoded by S. symbiotica Genomes
The three culturable S. symbiotica strains have pathogenic effects on infected aphids (Pons et al., 2019b; Elston et al., 2020; Perreau et al., 2021). The SsAf2.3 strain is also endocytosed into early embryos during ontogenesis and is compartmentalized into A. pisum sheath cells in a similar fashion as mutualistic strains, but ultimately fails to establish a stable association with aphids by vertical transmission (Perreau et al., 2021). This could be due to the still too high degree of pathogenicity of these strains, which hinders the establishment of stabilized associations. Secretion systems are among the many mechanisms used by pathogenic and symbiotic bacteria for host colonization and contribute to their degree of virulence: they allow bacteria to translocate effector proteins in host cells to modulate the host environment, thereby facilitating host invasion (Costa et al., 2015). In contrast to the facultative and co-obligate intracellular strains, the three culturable strains retain an intact type III secretion system (T3SS) (Table S9). These T3SSs are similar in sequence, gene content and gene orientation; and it is likely that it was acquired via horizontal gene transfer. Further, they appear to be related to the T3SS from Yersinia enterocolitica 8081 and thus belong to the SPI1 family generally associated to infection of animal hosts (Figure S4) (Abby and Rocha, 2012). The acquisition of the T3SS through horizontal gene transfer is also supported by the lower GC content of the T3SS gene cluster (44.9%) compared to the rest of the genomes (51.47-51.99%). Widely distributed among proteobacterial pathogens, T3SSs can be required during the establishment of symbiotic relationships between bacteria and eukaryotes (Göttfert et al., 2001; Dale et al., 2005), as in the case of tsetse fly invasion by the symbiont Sodalis glossinidius (Dale et al., 2001; Dale et al., 2002).
In addition, all S. symbiotica strains also exhibit several type V secretion systems (T5SSs). T5SSs are autotransporters that secrete virulence factors, often involved in cell-to-cell adhesion and biofilm formation (Leo et al., 2012). Their potential role(s) in the context of symbiotic relationships has however been poorly studied. Interestingly, SsApa8A1 is the only culturable strain that encodes for a complete flagellum: the genomes of SsAf2.3 and SsAf24.1 harbor many flagellar-coding genes, but do not have the full set of genes required for the formation of a complete flagellum (Table S9 and Figure 5). Given that the pathways for chemotaxis and motility are among the first to be altered in the transition from a free-living to a host-dependent lifestyle (Hendry et al., 2014), this suggests that compared to strains SsAf2.3 and SsAf24.1, SsAp8A1 is less advanced in the process of host-adaption and is still competent for swimming motility, which could facilitate access to new hosts or habitats. SsApa8A1 is also the only strain to harbor several T4SSs: sub-type I (T4SS-typeI) and protein secretion T4SS (pT4SSt). In addition to their ability to translocate effector proteins, T4SSs have the unique ability to mediate DNA translocation in bacterial or eukaryotic target cells (Costa et al., 2015). For example, the endosymbiont Wolbachia, but also the intracellular pathogens Coxiella burnetii and Legionella spp. use T4SSs to deliver ankyrin repeat containing proteins (ANKs) into the host cytoplasm (Segal et al., 2005; Pan et al., 2008; Nikoh et al., 2018). Intracellular symbionts of plants of the genus Rhizobium also use T4SSs to invade root cortical cells during nodulation and thus acquire an intracellular lifestyle (Deakin and Broughton, 2009). Ongoing studies should determine the role of the different macromolecular systems conserved in the genomes of culturable strains of S. symbiotica during the infection process in insect hosts, but also in plants.
The Array of Virulence Factors Encoded by the S. symbiotica Genomes
The success of host colonization by invasive bacteria, whether in the host-pathogen relationship or in mutualistic symbiosis, is largely determined by the ability of microorganisms to interact with hosts through the expression of various colonization factors (Hentschel et al., 2000; Bright and Bulgheresi, 2010). Strains SsAf2.3, SsAf24.1 and SsApa8A1 are capable of rapidly invading the digestive tract of aphids and, as shown for SsAf2.3, the embryos when it is microinjected into the hemolymph, ultimately affecting insect survival and reproduction (Renoz et al., 2015; Pons et al., 2019b; Elston et al., 2020). These observations suggest that these strains are well equipped to invade and persist in host tissues. Compared to the entomopathogenic bacterium S. marcescens Db11 (SmDb11), the genomes of the culturable S. symbiotica strains harbor a much narrower range of virulence genes. However, compared to the host-restricted S. symbiotica strains, they retain a greater diversity of virulence factors including siderophore-related proteins and T3SS effector proteins (Figure 6; see Table S10 for subject description & VFDB reference). This diversity in virulence factors may explain their pathogenicity and why they fail at this stage to establish a stable relationship by maternal transmission when infecting the aphid hemolymph (Perreau et al., 2021).
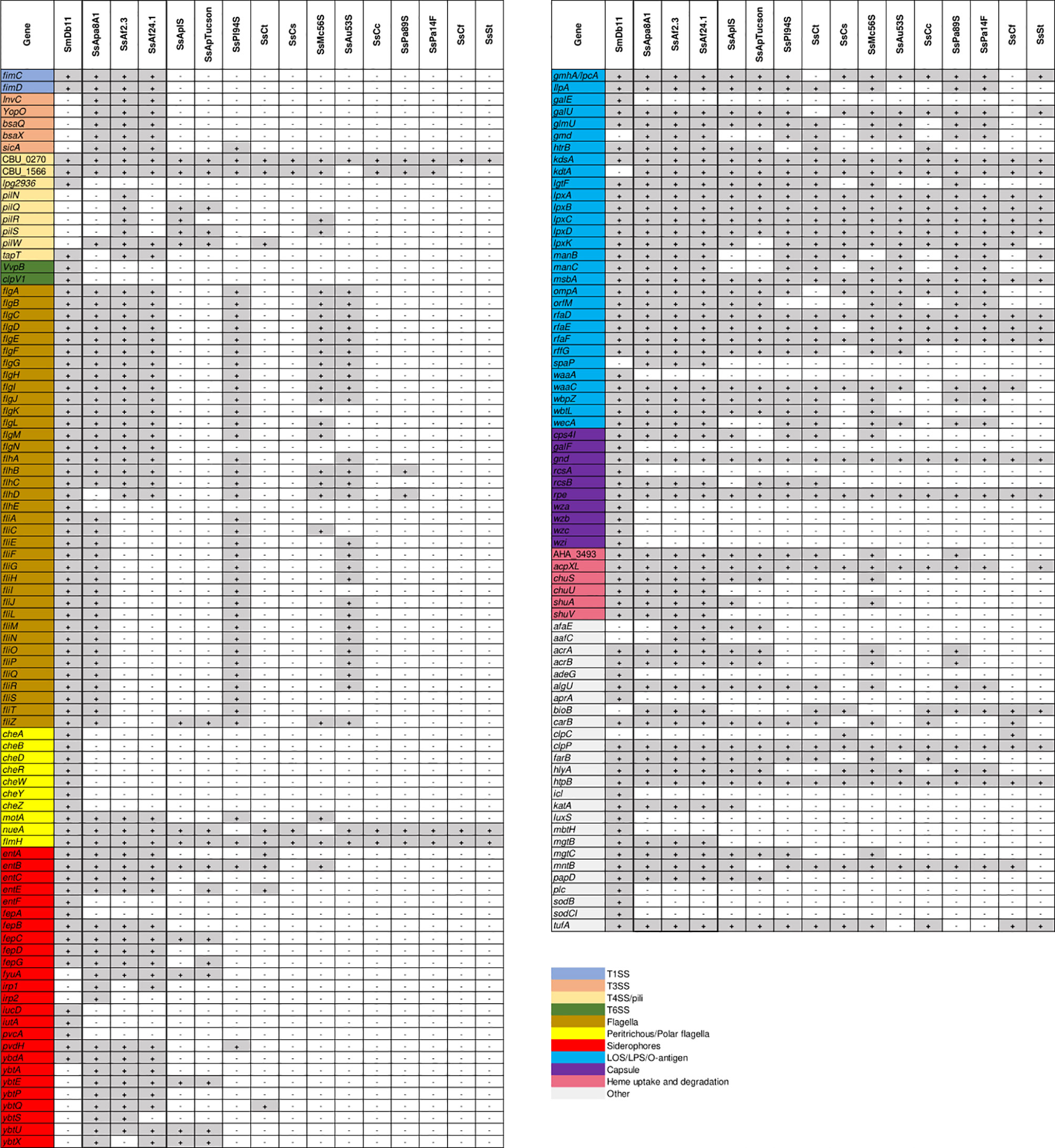
Figure 6 Presence/absence map of S. symbiotica virulence genes. Presence of a virulence gene is labeled in gray and absence in white.
Interestingly, the three culturable S. symbiotica strains share most of the potential virulence genes, suggesting that they are adapted to similar environments. Their genomes have in common the presence of many genes involved in siderophore-mediated iron transport such as yersinabactin and enterobactin. Iron uptake by siderophores promotes host colonization by many pathogens, but also by symbiotic bacteria such as S. glossinidius during the invasion of the tsetse fly (Smith et al., 2013a). The genomes of the three culturable strains also harbor many genes involved in the expression of fimbrial adhesins that may be involved in host adhesion and biofilm formation (Gerlach and Hensel, 2007). All three genomes harbor ilpA, a gene coding for an adhesin used by many gut-pathogens for host invasion (Lee et al., 2010). Several genes coding for secreted effector proteins have been detected in all three genomes, including YopO (also known as YpkA), a T3SS effector protein used by pathogenic Yersinia species to escape the host immune system by inducing actin-filament disruption during phagocytosis (Lee et al., 2015). The genes bsaQ and bsaX, also found in all three genomes, code for T3SS effector proteins that have been found essential for intracellular survival of Burkholderia pseudomallei in host cells (Muangsombut et al., 2008). Few toxin production-related genes were detected, with the exception of genes encoding the hemolytic protein hemolysin III and the pore-forming toxin Vibrio cholerae cytolysin (VCC) encoded by hlyA, both associated with a cytolytic/cytotoxic activity against wide range of eukaryotic cells (Baida and Kuzmin, 1996; Kathuria and Chattopadhyay, 2018). Intriguingly, all strains seem to have retained some ability to synthesize T4SS effectors while, with the exception of SsApa8A1, they did not retain any complete T4SS. Finally, although SsApa8A1 appears to be the only one of the three culturable strains to have retained the ability to construct a complete flagellum, the genomes SsAf2.3 and SsAf24.1 harbor many flagella-related genes. Many symbiotic bacteria have preserved flagellar basal bodies (FBBs) that are functional type III secretion systems (Moya et al., 2008). However, the presence of such systems in SsAf2.3 and SsAf24.1 remains uncertain because many genes required for the acquistion of such systems appear to be absent (e.g. fliF, fliG, fliH, fliN, and many others).
Most of the virulence genes common to the fifteen S. symbiotica genomes are involved in the biosynthesis of lipopolysaccharides (LPS) and lipooligosaccharides (LOS), the main components of the outer membrane of gram-negative bacteria that can mediate microbe-host interactions (Bright and Bulgheresi, 2010; Poole et al., 2018). The gene htpB, known for encoding a multifunctional heat-shock protein involved in the internalization of certain pathogens in host cells (Garduno et al., 2011), is also conserved in all the S. symbiotica genomes as well as clpP, a gene coding for a serine protease required for intracellular parasitism of macrophages by Listeria monocytogenes (Gaillot et al., 2000). Very little is known about the virulence factors necessary for the intracellular lifestyle of insect mutualistic symbionts. These two genes undoubtedly represent excellent candidates to tackle the genetic basis for the internalization of bacterial symbionts in host cells. How virulence factors are modulated during host invasion and internalization in host cells remains enigmatic in the context of bacterial mutualism in insects, and culturable S. symbiotica strains at the pathogen-symbiote interface, which are easier to manipulate than host-limited strains, should greatly ease the study of these mechanisms.
Conclusion and Perspective
Recently, several strains of S. symbiotica living in the guts of Aphis species have been isolated and cultivated. These strains probably evolved from plant-associates and could be, at this stage, involved in multi-host associations as has been reported for several phytopathogenic bacteria (Nadarasah and Stavrinides, 2011). Associated with pathogenic effects in aphids, these strains are remarkable because they may be a sibling group of the presumed ancestors of mutualistic S. symbiotica strains that have evolved towards a host-restricted lifestyle and thus provide a rare opportunity to decipher the genomic features of bacterial lineages before evolution towards host dependence. The three culturable strains show signs of early genome reduction but still have a gene pool that allows them to colonize new hosts, suggesting that they are intermediate stages between a free-living and a host-restricted lifestyle. With their extended metabolic capacities, they could be a source of metabolic innovations for the infected insects. A growing number of examples show that bacterial mutualisms have been established in insects through independent transitions to endosymbiosis (Clayton et al., 2012; Smith et al., 2013b; Husnik and McCutcheon, 2016; Boscaro et al., 2017). They support the hypothesis that recent events of acquisition/replacement of symbionts from a common lineage of “progenitors” from the environment may evolve into host-dependent relationships. Recent work conducted on plant-sucking stinkbugs experimentally demonstrated that the specialized obligate symbiotic bacteria associated with these insects can be replaced by less specialized free-living bacteria residing in the environment (Hosokawa et al., 2016a; Hosokawa et al., 2016b). Aphid-S. symbiotica associations offer remarkable examples of independent acquisitions of a symbiont species that have led to the emergence of mutualistic associations that may be facultative or co-obligate (Manzano-Marín and Latorre, 2016). In aphid species of the subfamilies Lachninae and Chaitophorinae, ancient co-obligate S. symbiotica strains have often been repeatedly replaced by more recently acquired strains (Manzano-Marín and Latorre, 2014; Manzano-Marín et al., 2016; Manzano-Marín et al., 2017; Monnin et al., 2020). These repeated replacements assume the existence of S. symbiotica strains available in the immediate environment of the host such its diet. Currently, little is known about how pathogenic bacteria evolve to integrate a stabilized mutualistic relationship with an insect host. The availability of culturable S. symbiotica strains at the pathogen-symbiont interface as well as their genomic sequence thus open new experimental perspectives to understand the drivers for the emergence of heritable mutualistic symbioses between bacteria and insects.
Data Availability Statement
The datasets presented in this study can be found in online repositories. The names of the repository/repositories and accession number(s) can be found in the article/Supplementary Material.
Author Contributions
FR, VF, and TH conceived and designed the research. FR, VF, JA, PB-P, BB, AG, and PM performed the research. FR, VF, JA, GL, and PM analyzed the data. FR wrote the paper. VF, JA, BB, JM, PM, J-LG, FC, and TH made manuscript revisions. All authors contributed to the article and approved the submitted version.
Funding
This study was financially supported by Grant FRFC 6886819 from the Belgian Funds for Scientific Research (F.R.S.-FNRS) and FRIA grant no. 1.E074.14). This paper is publication BRC268 of the Biodiversity Research Centre (Université catholique de Louvain). The funders had no role in study design, data collection and analysis, decision to publish, or preparation of the manuscript.
Conflict of Interest
The authors declare that the research was conducted in the absence of any commercial or financial relationships that could be construed as a potential conflict of interest.
Acknowledgments
The authors thank Hubert Charles, Diego Santos-Garcia, Sophie Abby, Chloé Peduzzi and Alain Vanderpoorten for the discussions on the study.
Supplementary Material
The Supplementary Material for this article can be found online at: https://www.frontiersin.org/articles/10.3389/fcimb.2021.660007/full#supplementary-material
References
Abby, S. S., Néron, B., Ménager, H., Touchon, M., Rocha, E. P. C. (2014). Macsyfinder: A Program to Mine Genomes for Molecular Systems With an Application to CRISPR-Cas Systems. PloS One 9, e110726. doi: 10.1371/journal.pone.0110726
Abby, S. S., Rocha, E. P. C. (2012). The Non-Flagellar Type III Secretion System Evolved From the Bacterial Flagellum and Diversified Into Host-Cell Adapted Systems. PloS Genet. 8, e1002983. doi: 10.1371/journal.pgen.1002983
Anahory, T., Darbas, H., Ongaro, O., Jean-Pierre, H., Mion, P. (1998). Serratia Ficaria: A Misidentified or Unidentified Rare Cause of Human Infections in Fig Tree Culture Zones. J. Clin. Microbiol. 36, 3266–3272. doi: 10.1128/JCM.36.11.3266-3272.1998
Anisimova, M., Gil, M., Dufayard, J.-F., Dessimoz, C., Gascuel, O. (2011). Survey of Branch Support Methods Demonstrates Accuracy, Power, and Robustness of Fast Likelihood-Based Approximation Schemes. Syst. Biol. 60, 685–699. doi: 10.1093/sysbio/syr041
Arndt, D., Grant, J. R., Marcu, A., Sajed, T., Pon, A., Liang, Y., et al. (2016). PHASTER: A Better, Faster Version of the PHAST Phage Search Tool. Nucleic Acids Res. 44, W16–W21. doi: 10.1093/nar/gkw387
Baida, G. E., Kuzmin, N. P. (1996). Mechanism of Action of Hemolysin III From Bacillus Cereus. Biochim. Biophys. Acta BBA Biomembr. 1284 (2), 122–124. doi: 10.1016/S0005-2736(96)00168-X
Ballio, A., Barra, D., Bossa, F., Collina, A., Grgurina, I., Marino, G., et al. (1991). Syringopeptins, New Phytotoxic Lipodepsipeptides of Pseudomonas Syringae Pv. Syringae. FEBS Lett. 291, 109–112. doi: 10.1016/0014-5793(91)81115-O
Baumann, P., Moran, N. A., Baumann, L. C. (2013). “Bacteriocyte-Associated Endosymbionts of Insects,” in The Prokaryotes: Prokaryotic Biology and Symbiotic Associations, Eds. Rosenberg, E., DeLong, E. F., Lory, S., Stackebrandt, E., Thompson, F. (Berlin, Heidelberg: Springer Berlin Heidelberg), 465–496. doi: 10.1007/978-3-642-30194-0_19
Bergsten, J. (2005). A Review of Long-Branch Attraction. Cladistics 21, 163–193. doi: 10.1111/j.1096-0031.2005.00059.x
Blin, K., Shaw, S., Steinke, K., Villebro, R., Ziemert, N., Lee, S. Y., et al. (2019). antiSMASH 5.0: Updates to the Secondary Metabolite Genome Mining Pipeline. Nucleic Acids Res. 47, W81–W87. doi: 10.1093/nar/gkz310
Blom, J., Kreis, J., Spänig, S., Juhre, T., Bertelli, C., Ernst, C., et al. (2016). Edgar 2.0: An Enhanced Software Platform for Comparative Gene Content Analyses. Nucleic Acids Res. 44, W22–W28. doi: 10.1093/nar/gkw255
Boscaro, V., Kolisko, M., Felletti, M., Vannini, C., Lynn, D. H., Keeling, P. J, et al. (2017). Parallel Genome Reduction in Symbionts Descended From Closely Related Free-Living Bacteria. Nat. Ecol. Evol. 1, 1160–1167. doi: 10.1038/s41559-017-0237-0
Braendle, C., Miura, T., Bickel, R., Shingleton, A. W., Kambhampati, S., Stern, D. L. (2003). Developmental Origin and Evolution of Bacteriocytes in the Aphid–Buchnera Symbiosis. PloS Biol. 1, e21. doi: 10.1371/journal.pbio.0000021
Bressan, A., Sémétey, O., Arneodo, J., Lherminier, J., Boudon-Padieu, E. (2009). Vector Transmission of a Plant-Pathogenic Bacterium in the Arsenophonus Clade Sharing Ecological Traits With Facultative Insect Endosymbionts. Phytopathology® 99, 1289–1296. doi: 10.1094/PHYTO-99-11-1289
Bright, M., Bulgheresi, S. (2010). A Complex Journey: Transmission of Microbial Symbionts. Nat. Rev. Microbiol. 8, 218–230. doi: 10.1038/nrmicro2262
Buchner, P. (1965). Endosymbiosis of Animals With Plant Microorganisms (New York: Interscience Publishers).
Burke, G., Fiehn, O., Moran, N. (2010). Effects of Facultative Symbionts and Heat Stress on the Metabolome of Pea Aphids. ISME J. 4, 242–252. doi: 10.1038/ismej.2009.114
Burke, G. R., Moran, N. A. (2011). Massive Genomic Decay in Serratia symbiotica, a Recently Evolved Symbiont of Aphids. Genome Biol. 3, 195–208. doi: 10.1093/gbe/evr002
Carlos, C., Castro, D. B. A., Ottoboni, L. M. M. (2014). Comparative Metagenomic Analysis of Coral Microbial Communities Using a Reference-Independent Approach. PloS One 9 (11), e111626. doi: 10.1371/journal.pone.0111626
Caspi, R., Billington, R., Keseler, I. M., Kothari, A., Krummenacker, M., Midford, P. E., et al. (2020). The MetaCyc Database of Metabolic Pathways and Enzymes - A 2019 Update. Nucleic Acids Res. 48, D445–D453. doi: 10.1093/nar/gkz862
Charles, H., Balmand, S., Lamelas, A., Cottret, L., Pérez-Brocal, V., Burdin, B., et al. (2011). A Genomic Reappraisal of Symbiotic Function in the Aphid/Buchnera Symbiosis: Reduced Transporter Sets and Variable Membrane Organisations. PloS One 6, e29096. doi: 10.1371/journal.pone.0029096
Chen, L., Yang, J., Yu, J., Yao, Z., Sun, L., Shen, Y., et al. (2005). VFDB: A Reference Database for Bacterial Virulence Factors. Nucleic Acids Res. 33, D325–D328. doi: 10.1093/nar/gki008
Clayton, A. L., Oakeson, K. F., Gutin, M., Pontes, A., Dunn, D. M., von Niederhausern, A. C., et al. (2012). A Novel Human-Infection-Derived Bacterium Provides Insights Into the Evolutionary Origins of Mutualistic Insect–Bacterial Symbioses. PloS Genet. 8, e1002990. doi: 10.1371/journal.pgen.1002990
Clokie, M. R. J., Millard, A. D., Letarov, A. V., Heaphy, S. (2011). Phages in Nature. Bacteriophage 1, 31–45. doi: 10.4161/bact.1.1.14942
Costa, T. R. D., Felisberto-Rodrigues, C., Meir, A., Prevost, M. S., Redzej, A., Trokter, M., et al. (2015). Secretion Systems in Gram-negative Bacteria: Structural and Mechanistic Insights. Nat. Rev. Microbiol. 13, 343–359. doi: 10.1038/nrmicro3456
Couvin, D., Bernheim, A., Toffano-Nioche, C., Touchon, M., Michalik, J., Néron, B., et al. (2018). CrisprcasFinder, an Update of CRISRFinder, Includes a Portable Version, Enhanced Performance and Integrates Search for Cas Proteins. Nucleic Acids Res. 46, W246–W251. doi: 10.1093/nar/gky425
Dale, C., Jones, T., Pontes, M. (2005). Degenerative Evolution and Functional Diversification of type-III Secretion Systems in the Insect Endosymbiont Sodalis Glossinidius. Mol. Biol. Evol. 22, 758–766. doi: 10.1093/molbev/msi061
Dale, C., Moran, N. A. (2006). Molecular Interactions Between Bacterial Symbionts and Their Hosts. Cell 126, 453–465. doi: 10.1016/j.cell.2006.07.014
Dale, C., Plague, G. R., Wang, B., Ochman, H., Moran, N. A. (2002). Type III Secretion Systems and the Evolution of Mutualistic Endosymbiosis. Proc. Natl. Acad. Sci. 99, 12397–12402. doi: 10.1073/pnas.182213299
Dale, C., Young, S. A., Haydon, D. T., Welburn, S. C. (2001). The Insect Endosymbiont Sodalis Glossinidius Utilizes a Type III Secretion System for Cell Invasion. Proc. Natl. Acad. Sci. U. S. A. 98, 1883–1888. doi: 10.1073/pnas.021450998
Deakin, W. J., Broughton, W. J. (2009). Symbiotic Use of Pathogenic Strategies: Rhizobial Protein Secretion Systems. Nat. Rev. Microbiol. 7, 312–320. doi: 10.1038/nrmicro2091
de Bruijn, I., de Kock, M. J. D., de Waard, P., van Beek, T. A., Raaijmakers, J. M. (2008). Massetolide A Biosynthesis in Pseudomonas Fluorescens. J. Bacteriol. 190, 2777–2789. doi: 10.1128/JB.01563-07
Degnan, P. H., Yu, Y., Sisneros, N., Wing, R. A., Moran, N. A. (2009). Hamiltonella defensa, Genome Evolution of Protective Bacterial Endosymbiont From Pathogenic Ancestors. Proc. Natl. Acad. Sci. 106, 9063–9068. doi: 10.1073/pnas.0900194106
Douglas, A. E. (1998). Nutritional Interactions in Insect-Microbial Symbioses: Aphids and Their Symbiotic Bacteria Buchnera. Annu. Rev. Entomol. 43, 17–37. doi: 10.1146/annurev.ento.43.1.17
Eckelmann, D., Spiteller, M., Kusari, S. (2018). Spatial-Temporal Profiling of Prodiginines and Serratamolides Produced by Endophytic Serratia marcescens Harbored in Maytenus Serrata. Sci. Rep. 8, 1–15. doi: 10.1038/s41598-018-23538-5
Eckhardt, T. (1978). A Rapid Method for the Identification of Plasmid Desoxyribonucleic Acid in Bacteria. Plasmid 1, 584–588. doi: 10.1016/0147-619X(78)90016-1
Elston, K. M., Perreau, J., Maeda, G. P., Moran, N. A., Barrick, J. E. (2020). Engineering a Culturable Serratia symbiotica Strain for Aphid Paratransgenesis. Appl. Environ. Microbiol. 87 (4), e02245-20. doi: 10.1128/AEM.02245-20
Emms, D. M., Kelly, S. (2015). OrthoFinder: Solving Fundamental Biases in Whole Genome Comparisons Dramatically Improves Orthogroup Inference Accuracy. Genome Biol. 16 (1), 1–14. doi: 10.1186/s13059-015-0721-2
Emms, D. M., Kelly, S. (2019). OrthoFinder: Phylogenetic Orthology Inference for Comparative Genomics. Genome Biol. 20, 238. doi: 10.1186/s13059-019-1832-y
Engel, P., Moran, N. A. (2013). The Gut Microbiota of Insects – Diversity in Structure and Function. FEMS Microbiol. Rev. 37, 699–735. doi: 10.1111/1574-6976.12025
Fath, M. J., Mahanty, H. K., Kolter, R. (1989). Characterization of a Purf Operon Mutation Which Affects Colicin V Production. J. Bacteriol. 171, 3158–3161. doi: 10.1128/jb.171.6.3158-3161.1989
Feldhaar, H. (2011). Bacterial Symbionts as Mediators of Ecologically Important Traits of Insect Hosts. Ecol. Entomol. 36, 533–543. doi: 10.1111/j.1365-2311.2011.01318.x
Foray, V., Grigorescu, A. S., Sabri, A., Haubruge, E., Lognay, G., Francis, F., et al. (2014). Whole-Genome Sequence of Serratia Symbiotica Strain Cwbi-2.3T, A Free-Living Symbiont of the Black Bean Aphid Aphis Fabae. Genome Announc. 2, e00767–e00714. doi: 10.1128/genomeA.00767-14
Gaillot, O., Pellegrini, E., Bregenholt, S., Nair, S., Berche, P. (2000). The ClpP Serine Protease Is Essential for the Intracellular Parasitism and Virulence of Listeria Monocytogenes. Mol. Microbiol. 35, 1286–1294. doi: 10.1046/j.1365-2958.2000.01773.x
Gardebrecht, A., Markert, S., Sievert, S. M., Felbeck, H., Thürmer, A., Albrecht, D., et al. (2012). Physiological Homogeneity Among the Endosymbionts of Riftia Pachyptila and Tevnia Jerichonana Revealed by Proteogenomics. ISME J. 6, 766–776. doi: 10.1038/ismej.2011.137
Garduño, R. A., Chong, A., Nasrallah, G. K., Allan, D. S. (2011). The Legionella Pneumophila Chaperonin – An Unusual Multifunctional Protein in Unusual Locations. Front. Microbiol. 2 (1220), 1–12. doi: 10.3389/fmicb.2011.00122
Gérard, F., Pradel, N., Wu, L.-F. (2005). Bactericidal Activity of Colicin V Is Mediated by an Inner Membrane Protein, SdaC, of Escherichia Coli. J. Bacteriol. 187, 1945–1950. doi: 10.1128/JB.187.6.1945-1950.2005
Gerlach, R. G., Hensel, M. (2007). Protein Secretion Systems and Adhesins: The Molecular Armory of Gram-Negative Pathogens. Int. J. Med. Microbiol. 297, 401–415. doi: 10.1016/j.ijmm.2007.03.017
Göttfert, M., Röthlisberger, S., Kündig, C., Beck, C., Marty, R., Hennecke, H. (2001). Potential Symbiosis-Specific Genes Uncovered by Sequencing a 410-Kilobase DNA Region of the Bradyrhizobium Japonicum Chromosome. J. Bacteriol. 183, 1405–1412. doi: 10.1128/JB.183.4.1405-1412.2001
Grigorescu, A. S., Renoz, F., Sabri, A., Foray, V., Hance, T., Thonart, P. (2018). Accessing the Hidden Microbial Diversity of Aphids: An Illustration of How Culture-Dependent Methods Can Be Used to Decipher the Insect Microbiota. Microb. Ecol. 75, 1035–1048. doi: 10.1007/s00248-017-1092-x
Grimont, P. A. D., Grimont, F., Starr, M. P. (1981). Serratia Species Isolated From Plants. Curr. Microbiol. 5, 317–322. doi: 10.1007/BF01567926
Hadjifrangiskou, M., Gu, A. P., Pinkner, J. S., Kostakioti, M., Zhang, E. W., Greene, S. E., et al. (2012). Transposon Mutagenesis Identifies Uropathogenic Escherichia Coli Biofilm Factors. J. Bacteriol. 194, 6195–6205. doi: 10.1128/JB.01012-12
Hegedus, D., Erlandson, M., Gillott, C., Toprak, U. (2009). New Insights Into Peritrophic Matrix Synthesis, Architecture, and Function. Annu. Rev. Entomol. 54, 285–302. doi: 10.1146/annurev.ento.54.110807.090559
Hendry, T. A., de Wet, J. R., Dunlap, P. V. (2014). Genomic Signatures of Obligate Host Dependence in the Luminous Bacterial Symbiont of a Vertebrate. Environ. Microbiol. 16, 2611–2622. doi: 10.1111/1462-2920.12302
Hentschel, U., Steinert, M., Hacker, J. (2000). Common Molecular Mechanisms of Symbiosis and Pathogenesis. Trends Microbiol. 8, 226–231. doi: 10.1016/S0966-842X(00)01758-3
Hoang, D. T., Chernomor, O., von Haeseler, A., Minh, B. Q., Vinh, L. S. (2018). Ufboot2: Improving the Ultrafast Bootstrap Approximation. Mol. Biol. Evol. 35, 518–522. doi: 10.1093/molbev/msx281
Hosokawa, T., Ishii, Y., Nikoh, N., Fujie, M., Satoh, N., Fukatsu, T. (2016a). Obligate Bacterial Mutualists Evolving From Environmental Bacteria in Natural Insect Populations. Nat. Microbiol. 1, 15011. doi: 10.1038/nmicrobiol.2015.11
Hosokawa, T., Matsuura, Y., Kikuchi, Y., Fukatsu, T. (2016b). Recurrent Evolution of Gut Symbiotic Bacteria in Pentatomid Stinkbugs. Zool. Lett. 2, 24. doi: 10.1186/s40851-016-0061-4
Hubbard, T. P., Chao, M. C., Abel, S., Blondel, C. J., zur Wiesch, P. A., Zhou, X., et al. (2016). Genetic Analysis of Vibrio Parahaemolyticus Intestinal Colonization. Proc. Natl. Acad. Sci. 113, 6283–6288. doi: 10.1073/pnas.1601718113
Huerta-Cepas, J., Forslund, K., Coelho, L. P., Szklarczyk, D., Jensen, L. J., von Mering, C., et al. (2017). Fast Genome-Wide Functional Annotation Through Orthology Assignment by Eggnog-Mapper. Mol. Biol. Evol. 34, 2115–2122. doi: 10.1093/molbev/msx148
Husník, F., Chrudimský, T., Hypša, V. (2011). Multiple Origins of Endosymbiosis Within the Enterobacteriaceae (γ-Proteobacteria): Convergence of Complex Phylogenetic Approaches. BMC Biol. 9, 87. doi: 10.1186/1741-7007-9-87
Husnik, F., McCutcheon, J. P. (2016). Repeated Replacement of an Intrabacterial Symbiont in the Tripartite Nested Mealybug Symbiosis. Proc. Natl. Acad. Sci. 113, E5416–E5424. doi: 10.1073/pnas.1603910113
Kalyaanamoorthy, S., Minh, B. Q., Wong, T. K. F., von Haeseler, A., Jermiin, L. S. (2017). ModelFinder: Fast Model Selection for Accurate Phylogenetic Estimates. Nat. Methods 14, 587–589. doi: 10.1038/nmeth.4285
Kathuria, R., Chattopadhyay, K. (2018). Vibrio cholerae Cytolysin: Multiple Facets of the Membrane Interaction Mechanism of a β-Barrel Pore-Forming Toxin. IUBMB Life 70, 260–266. doi: 10.1002/iub.1725
Khan, A. R., Park, G.-S., Asaf, S., Hong, S.-J., Jung, B. K., Shin, J.-H. (2017). Complete Genome Analysis of Serratia Marcescens RSC-14: A Plant Growth-Promoting Bacterium That Alleviates Cadmium Stress in Host Plants. PloS One 12, e0171534. doi: 10.1371/journal.pone.0171534
Kikuchi, Y., Hosokawa, T., Fukatsu, T. (2007). Insect-Microbe Mutualism Without Vertical Transmission: A Stinkbug Acquires a Beneficial Gut Symbiont From the Environment Every Generation. Appl. Environ. Microbiol. 73, 4308–4316. doi: 10.1128/AEM.00067-07
Kikuchi, Y., Hosokawa, T., Fukatsu, T. (2011). An Ancient But Promiscuous Host–Symbiont Association Between Burkholderia Gut Symbionts and Their Heteropteran Hosts. ISME J. 5, 446–460. doi: 10.1038/ismej.2010.150
Koga, R., Moran, N. A. (2014). Swapping Symbionts in Spittlebugs: Evolutionary Replacement of a Reduced Genome Symbiont. ISME J. 8, 1237–1246. doi: 10.1038/ismej.2013.235
Koga, R., Tsuchida, T., Fukatsu, T. (2003). Changing Partners in an Obligate Symbiosis: A Facultative Endosymbiont Can Compensate for Loss of the Essential Endosymbiont Buchnera in an Aphid. Proc. R. Soc Lond. B. Biol. Sci. 270, 2543–2550. doi: 10.1098/rspb.2003.2537
Kück, P., Meusemann, K. (2010). Fasconcat: Convenient Handling of Data Matrices. Mol. Phylogenet. Evol. 56, 1115–1118. doi: 10.1016/j.ympev.2010.04.024
Kunakom, S., Eustáquio, A. S. (2019). Burkholderia as a Source of Natural Products J. Nat. Prod. 82, 2018–2037. doi: 10.1021/acs.jnatprod.8b01068
Lal, P. B., Schneider, B. L., Vu, K., Reitzer, L. (2014). The Redundant Aminotransferases in Lysine and Arginine Synthesis and the Extent of Aminotransferase Redundancy in Escherichia Coli. Mol. Microbiol. 94, 843–856. doi: 10.1111/mmi.12801
Lamelas, A., Gosalbes, M. J., Manzano-Marín, A., Peretó, J., Moya, A., Latorre, A. (2011a). Serratia symbiotica From the Aphid Cinara Cedri: A Missing Link From Facultative to Obligate Insect Endosymbiont. PloS Genet. 7, e1002357. doi: 10.1371/journal.pgen.1002357
Lamelas, A., Gosalbes, M. J., Moya, A., Latorre, A. (2011b). New Clues About the Evolutionary History of Metabolic Losses in Bacterial Endosymbionts, Provided by the Genome of Buchnera aphidicola From the Aphid Cinara tujafilina. Appl. Environ. Microbiol. 77 (13), 4446–4454. doi: 10.1128/AEM.00141-11
Latorre, A., Manzano-Marín, A. (2017). Dissecting Genome Reduction and Trait Loss in Insect Endosymbionts. Ann. N. Y. Acad. Sci. 1389 (1), 52–75. doi: 10.1111/nyas.13222
Lee, W. L., Grimes, J. M., Robinson, R. C. (2015). Yersinia Effector YopO Uses Actin as Bait to Phosphorylate Proteins That Regulate Actin Polymerization. Nat. Struct. Mol. Biol. 22, 248–255. doi: 10.1038/nsmb.2964
Lee, K.-J., Lee, N. Y., Han, Y.-S., Kim, J., Lee, K.-H., Park, S.-J. (2010). Functional Characterization of the IlpA Protein of Vibrio vulnificus as an Adhesin and Its Role in Bacterial Pathogenesis. Infect. Immun. 78, 2408–2417. doi: 10.1128/IAI.01194-09
Leo, J. C., Grin, I., Linke, D. (2012). Type V Secretion: Mechanism(s) of Autotransport Through the Bacterial Outer Membrane. Philos. Trans. R. Soc B. Biol. Sci. 367, 1088–1101. doi: 10.1098/rstb.2011.0208
Lerat, E., Ochman, H. (2005). Recognizing the Pseudogenes in Bacterial Genomes. Nucleic Acids Res. 33, 3125–3132. doi: 10.1093/nar/gki631
Li, R., Helbig, L., Fu, J., Bian, X., Herrmann, J., Baumann, M., et al. (2019). Expressing Cytotoxic Compounds in Escherichia coli Nissle 1917 for Tumor-Targeting Therapy. Res. Microbiol. 170, 74–79. doi: 10.1016/j.resmic.2018.11.001
Liu, B., Zheng, D., Jin, Q., Chen, L., Yang, J. (2019). VFDB 2019: A Comparative Pathogenomic Platform With an Interactive Web Interface. Nucleic Acids Res. 47, D687–D692. doi: 10.1093/nar/gky1080
Lo, W.-S., Huang, Y.-Y., Kuo, C.-H. (2016). Winding Paths to Simplicity: Genome Evolution in Facultative Insect Symbionts. FEMS Microbiol. Rev. 40, 855–874. doi: 10.1093/femsre/fuw028
Loper, J. E., Hassan, K. A., Mavrodi, D. V., Ii, E. W. D., Lim, C. K., Shaffer, B. T., et al. (2012). Comparative Genomics of Plant-Associated Pseudomonas Spp.: Insights Into Diversity and Inheritance of Traits Involved in Multitrophic Interactions. PloS Genet. 8, e1002784. doi: 10.1371/journal.pgen.1002784
Makarova, K. S., Haft, D. H., Barrangou, R., Brouns, S. J. J., Charpentier, E., Horvath, P., et al. (2011). Evolution and Classification of the CRISPR–Cas Systems. Nat. Rev. Microbiol. 9, 467–477. doi: 10.1038/nrmicro2577
Manzano-Marín, A., Coeur d’acier, A., Clamens, A.-L., Orvain, C., Cruaud, C., Barbe, V., et al. (2018). A Freeloader? The Highly Eroded Yet Large Genome of the Serratia symbiotica Symbiont of Cinara Strobi. Genome Biol. Evol. 10, 2178–2189. doi: 10.1093/gbe/evy173
Manzano-Marín, A., Lamelas, A., Moya, A., Latorre, A. (2012). Comparative Genomics of Serratia Spp.: Two Paths Towards Endosymbiotic Life. PloS One 7, e47274. doi: 10.1371/journal.pone.0047274
Manzano-Marín, A., Latorre, A. (2014). Settling Down: The Genome of Serratia Symbiotica From the Aphid Cinara Tujafilina Zooms in on the Process of Accommodation to a Cooperative Intracellular Life. Genome Biol. Evol. 6, 1683–1698. doi: 10.1093/gbe/evu133
Manzano-Marín, A., Latorre, A. (2016). Snapshots of a Shrinking Partner: Genome Reduction in Serratia Symbiotica. Sci. Rep. 6 (1), 1–11. doi: 10.1038/srep32590
Manzano-Marín, A., Simon, J.-C., Latorre, A. (2016). Reinventing the Wheel and Making it Round Again: Evolutionary Convergence in Buchnera–Serratia Symbiotic Consortia Between the Distantly Related Lachninae Aphids Tuberolachnus Salignus and Cinara Cedri. Genome Biol. Evol. 8, 1440–1458. doi: 10.1093/gbe/evw085
Manzano-Marín, A., Szabó, G., Simon, J.-C., Horn, M., Latorre, A. (2017). Happens in the Best of Subfamilies: Establishment and Repeated Replacements of Co-Obligate Secondary Endosymbionts Within Lachninae Aphids. Environ. Microbiol. 19, 393–408. doi: 10.1111/1462-2920.13633
Manzano-Marín, A., D’acier, A. C., Clamens, A.-L., Orvain, C., Cruaud, C., Barbe, V., et al. (2020). Serial Horizontal Transfer of Vitamin-Biosynthetic Genes Enables the Establishment of New Nutritional Symbionts in Aphids’ Di-Symbiotic Systems. ISME J. 14, 259–273. doi: 10.1038/s41396-019-0533-6
Mao, M., Bennett, G. M. (2020). Symbiont Replacements Reset the Co-Evolutionary Relationship Between Insects and Their Heritable Bacteria. ISME J. 14, 1384–1395. doi: 10.1038/s41396-020-0616-4
Matsuura, Y., Moriyama, M., Łukasik, P., Vanderpool, D., Tanahashi, M., Meng, X.-Y., et al. (2018). Recurrent Symbiont Recruitment From Fungal Parasites in Cicadas. Proc. Natl. Acad. Sci. 115, E5970–E5979. doi: 10.1073/pnas.1803245115
McFall-Ngai, M., Hadfield, M. G., Bosch, T. C. G., Carey, H. V., Domazet-Lošo, T., Douglas, A. E., et al. (2013). Animals in a Bacterial World, a New Imperative for the Life Sciences. Proc. Natl. Acad. Sci. 110, 3229–3236. doi: 10.1073/pnas.1218525110
Médigue, C., Calteau, A., Cruveiller, S., Gachet, M., Gautreau, G., Josso, A., et al. (2019). MicroScope—An Integrated Resource for Community Expertise of Gene Functions and Comparative Analysis of Microbial Genomic and Metabolic Data. Brief. Bioinform. 20, 1071–1084. doi: 10.1093/bib/bbx113
Merzendorfer, H., Kelkenberg, M., Muthukrishnan, S. (2016). “Peritrophic Matrices,” in Extracellular Composite Matrices in Arthropods. Eds. Cohen, E., Moussian, B. (Cham: Springer International Publishing), 255–324. doi: 10.1007/978-3-319-40740-1_8
Meseguer, A. S., Manzano-Marín, A., d’Acier, A. C., Clamens, A.-L., Godefroid, M., Jousselin, E. (2017). Buchnera has Changed Flatmate But the Repeated Replacement of Co-Obligate Symbionts Is Not Associated With the Ecological Expansions of Their Aphid Hosts. Mol. Ecol. 26, 2363–2378. doi: 10.1111/mec.13910
Minh, B. Q., Nguyen, M. A. T., von Haeseler, A. (2013). Ultrafast Approximation for Phylogenetic Bootstrap. Mol. Biol. Evol. 30, 1188–1195. doi: 10.1093/molbev/mst024
Monnin, D., Jackson, R., Kiers, E. T., Bunker, M., Ellers, J., Henry, L. M. (2020). Parallel Evolution in the Integration of a Co-Obligate Aphid Symbiosis. Curr. Biol. 30 (10), 1949–1957. doi: 10.1016/j.cub.2020.03.011
Moran, N. A., Plague, G. R. (2004). Genomic Changes Following Host Restriction in Bacteria. Curr. Opin. Genet. Dev. 14, 627–633. doi: 10.1016/j.gde.2004.09.003
Moya, A., Peretó, J., Gil, R., Latorre, A. (2008). Learning How to Live Together: Genomic Insights Into Prokaryote-Animal Symbioses. Nat. Rev. Genet. 9, 218–229. doi: 10.1038/nrg2319
Muangsombut, V., Suparak, S., Pumirat, P., Damnin, S., Vattanaviboon, P., Thongboonkerd, V., et al. (2008). Inactivation of Burkholderia Pseudomallei Bsaq Results in Decreased Invasion Efficiency and Delayed Escape of Bacteria From Endocytic Vesicles. Arch. Microbiol. 190, 623–631. doi: 10.1007/s00203-008-0413-3
Nadarasah, G., Stavrinides, J. (2011). Insects as Alternative Hosts for Phytopathogenic Bacteria. FEMS Microbiol. Rev. 35, 555–575. doi: 10.1111/j.1574-6976.2011.00264.x
Neupane, S., Finlay, R. D., Alström, S., Goodwin, L., Kyrpides, N. C., Lucas, S., et al. (2012). Complete Genome Sequence of Serratia Plymuthica Strain AS12. Stand. Genom. Sci. 6, 165–173. doi: 10.4056/sigs.2705996
Nguyen, L.-T., Schmidt, H. A., von Haeseler, A., Minh, B. Q. (2015). Iq-Tree: A Fast and Effective Stochastic Algorithm for Estimating Maximum-Likelihood Phylogenies. Mol. Biol. Evol. 32, 268–274. doi: 10.1093/molbev/msu300
Niem, J., Billones-Baaijens, R., Savocchia, S., Stodart, B. (2019). Draft Genome Sequences of Endophytic Pseudomonas Spp. Isolated From Grapevine Tissue and Antagonistic to Grapevine Trunk Disease Pathogens. Microbiol. Resour. Announc 8 (26), e00345-19. doi: 10.1128/MRA.00345-19
Nikoh, N., Hosokawa, T., Oshima, K., Hattori, M., Fukatsu, T. (2011). Reductive Evolution of Bacterial Genome in Insect Gut Environment. Genome Biol. Evol. 3, 702–714. doi: 10.1093/gbe/evr064
Nikoh, N., Koga, R., Oshima, K., Hattori, M., Fukatsu, T. (2019). Genome Sequence of “Candidatus Serratia Symbiotica” Strain Is, a Facultative Bacterial Symbiont of the Pea Aphid Acyrthosiphon Pisum. Microbiol. Resour. Announc. 8, e00272–e00219. doi: 10.1128/MRA.00272-19
Nikoh, N., Tsuchida, T., Maeda, T., Yamaguchi, K., Shigenobu, S., Koga, R., et al. (2018). Genomic Insight Into Symbiosis-Induced Insect Color Change by a Facultative Bacterial Endosymbiont, “Candidatus Rickettsiella Viridis.” mBio 9 (3), e00890-18. doi: 10.1128/mBio.00890-18
Oliver, K. M., Degnan, P. H., Burke, G. R., Moran, N. A. (2010). Facultative Symbionts in Aphids and the Horizontal Transfer of Ecologically Important Traits. Annu. Rev. Entomol. 55, 247–266. doi: 10.1146/annurev-ento-112408-085305
Oliver, K. M., Degnan, P. H., Hunter, M. S., Moran, N. A. (2009). Bacteriophages Encode Factors Required for Protection in a Symbiotic Mutualism. Science 325, 992–994. doi: 10.1126/science.1174463
Oliver, K. M., Noge, K., Huang, E. M., Campos, J. M., Becerra, J. X., Hunter, M. S. (2012). Parasitic Wasp Responses to Symbiont-Based Defense in Aphids. BMC Biol. 10:11. doi: 10.1186/1741-7007-10-11
Oliver, K. M., Russell, J. A., Moran, N. A., Hunter, M. S. (2003). Facultative Bacterial Symbionts in Aphids Confer Resistance to Parasitic Wasps. Proc. Natl. Acad. Sci. 100, 1803–1807. doi: 10.1073/pnas.0335320100
Oliver, K. M., Smith, A. H., Russell, J. A. (2014). Defensive Symbiosis in the Real World – Advancing Ecological Studies of Heritable, Protective Bacteria in Aphids and Beyond. Funct. Ecol. 28, 341–355. doi: 10.1111/1365-2435.12133
Pan, X., Lührmann, A., Satoh, A., Laskowski-Arce, M. A., Roy, C. R. (2008). Ankyrin Repeat Proteins Comprise a Diverse Family of Bacterial Type IV Effectors. Science 320, 1651–1654. doi: 10.1126/science.1158160
Perreau, J., Patel, D. J., Anderson, H., Maeda, G. P., Elston, K. M., Barrick, J. E., et al. (2021). Vertical Transmission at the Pathogen-Symbiont Interface: Serratia Symbiotica and Aphids. mBio 12 (2), e00359-21. doi: 10.1128/mBio.00359-21
Petersen, L. M., Tisa, L. S. (2013). Friend or Foe? A Review of the Mechanisms That Drive Serratia Towards Diverse Lifestyles. Can. J. Microbiol. 59, 627–640. doi: 10.1139/cjm-2013-0343
Pons, I., Renoz, F., Noël, C., Hance, T. (2019a). Circulation of the Cultivable Symbiont Serratia Symbiotica in Aphids Is Mediated by Plants. Front. Microbiol. 10, 764. doi: 10.3389/fmicb.2019.00764
Pons, I., Renoz, F., Noël, C., Hance, T. (2019b). New Insights Into the Nature of Symbiotic Associations in Aphids: Infection Process, Biological Effects and Transmission Mode of Cultivable Serratia symbiotica Bacteria. Appl. Environ. Microbiol. 85 (10), e02445-18. doi: 10.1128/AEM.02445-18
Pons, I., Scieur, N., Dhondt, L., Renard, M.-E., Renoz, F., Hance, T. (2021). Ubiquity of the Symbiont Serratia symbiotica in the Aphid Natural Environment: Distribution, Diversity and Evolution at a Multitrophic Level. bioRxiv 2021, 4.18.440331. doi: 10.1101/2021.04.18.440331
Poole, J., Day, C. J., von Itzstein, M., Paton, J. C. (2018). Glycointeractions in Bacterial Pathogenesis. Nat. Rev. Microbiol. 16, 440–452. doi: 10.1038/s41579-018-0007-2
Prickett, M. D., Page, M., Douglas, A. E., Thomas, G. H. (2006). BuchneraBASE: A Post-Genomic Resource for Buchnera Sp. APS. Bioinform. Oxf. Engl. 22, 641–642. doi: 10.1093/bioinformatics/btk024
Raaijmakers, J. M., De Bruijn, I., Nybroe, O., Ongena, M. (2010). Natural Functions of Lipopeptides From Bacillus and Pseudomonas: More Than Surfactants and Antibiotics. FEMS Microbiol. Rev. 34, 1037–1062. doi: 10.1111/j.1574-6976.2010.00221.x
Regev, A., Keller, M., Strizhov, N., Sneh, B., Prudovsky, E., Chet, I., et al. (1996). Synergistic Activity of a Bacillus Thuringiensis Delta-Endotoxin and a Bacterial Endochitinase Against Spodoptera Littoralis Larvae. Appl. Environ. Microbiol. 62, 3581–3586. doi: 10.1128/aem.62.10.3581-3586.1996
Renoz, F., Ambroise, J., Bearzatto, B., Baa-Puyoulet, P., Calevro, F., Gala, J.-L., et al. (2020). Draft Genome Sequences of Two Cultivable Strains of the Bacterial Symbiont Serratia Symbiotica. Microbiol. Resour. Announc. 9 (10), e01579-19. doi: 10.1128/MRA.01579-19
Renoz, F., Noël, C., Errachid, A., Foray, V., Hance, T. (2015). Infection Dynamic of Symbiotic Bacteria in the Pea Aphid Acyrthosiphon Pisum Gut and Host Immune Response at the Early Steps in the Infection Process. PloS One 10, e0122099. doi: 10.1371/journal.pone.0122099
Renoz, F., Pons, I., Vanderpoorten, A., Bataille, G., Noël, C., Foray, V., et al. (2019). Evidence for Gut-Associated Serratia Symbiotica in Wild Aphids and Ants Provides New Perspectives on the Evolution of Bacterial Mutualism in Insects. Microb. Ecol. 78 (1), 159–169. doi: 10.1007/s00248-018-1265-2
Russell, J. A., Oliver, K. M., Hansen, A. K. (2017). Band-Aids for Buchnera and B Vitamins for All. Mol. Ecol. 26, 2199–2203. doi: 10.1111/mec.14047
Sabri, A., Leroy, P., Haubruge, E., Hance, T., Frere, I., Destain, J., et al. (2011). Isolation, Pure Culture and Characterization of Serratia symbiotica Sp. Nov., the R-Type of Secondary Endosymbiont of the Black Bean Aphid Aphis Fabae. Int. J. Syst. Evol. Microbiol. 61, 2081–2088. doi: 10.1099/ijs.0.024133-0
Santos-Garcia, D., Rollat-Farnier, P.-A., Beitia, F., Zchori-Fein, E., Vavre, F., Mouton, L., et al. (2014). The Genome of Cardinium Cbtq1 Provides Insights Into Genome Reduction, Symbiont Motility, and Its Settlement in Bemisia Tabaci. Genome Biol. Evol. 6, 1013–1030. doi: 10.1093/gbe/evu077
Scarborough, C. L., Ferrari, J., Godfray, H. C. J. (2005). Aphid Protected From Pathogen by Endosymbiont. Science 310, 1781–1781. doi: 10.1126/science.1120180
Segal, G., Feldman, M., Zusman, T. (2005). The Icm/Dot type-IV Secretion Systems of Legionella pneumophila and Coxiella Burnetii. FEMS Microbiol. Rev. 29, 65–81. doi: 10.1016/j.femsre.2004.07.001
Shaffer, C. L., Zhang, E. W., Dudley, A. G., Dixon, B. R. E. A., Guckes, K. R., Breland, E. J., et al. (2017). Purine Biosynthesis Metabolically Constrains Intracellular Survival of Uropathogenic Escherichia Coli. Infect. Immun. 85, e00471–e00416. doi: 10.1128/IAI.00471-16
Sloan, D. B., Moran, N. A. (2012). Genome Reduction and Co-Evolution Between the Primary and Secondary Bacterial Symbionts of Psyllids. Mol. Biol. Evol. 29, 3781–3792. doi: 10.1093/molbev/mss180
Smith, W. A., Oakeson, K. F., Johnson, K. P., Reed, D. L., Carter, T., Smith, K. L., et al. (2013b). Phylogenetic Analysis of Symbionts in Feather-Feeding Lice of the Genus Columbicola: Evidence for Repeated Symbiont Replacements. BMC Evol. Biol. 13, 109. doi: 10.1186/1471-2148-13-109
Smith, C. L., Weiss, B. L., Aksoy, S., Runyen-Janecky, L. J. (2013a). Characterization of the Achromobactin Iron Acquisition Operon in Sodalis Glossinidius. Appl. Environ. Microbiol. 79, 2872–2881. doi: 10.1128/AEM.03959-12
Tatusova, T., DiCuccio, M., Badretdin, A., Chetvernin, V., Nawrocki, E. P., Zaslavsky, L., et al. (2016). NCBI Prokaryotic Genome Annotation Pipeline. Nucleic Acids Res. 44, 6614–6624. doi: 10.1093/nar/gkw569
Toft, C., Andersson, S. G. E. (2010). Evolutionary Microbial Genomics: Insights Into Bacterial Host Adaptation. Nat. Rev. Genet. 11, 465–475. doi: 10.1038/nrg2798
Tran, H., Ficke, A., Asiimwe, T., Höfte, M., Raaijmakers, J. M. (2007). Role of the Cyclic Lipopeptide Massetolide A in Biological Control of Phytophthora infestans and in Colonization of Tomato Plants by Pseudomonas Fluorescens. New Phytol. 175, 731–742. doi: 10.1111/j.1469-8137.2007.02138.x
Tsuchida, T., Koga, R., Horikawa, M., Tsunoda, T., Maoka, T., Matsumoto, S., et al. (2010). Symbiotic Bacterium Modifies Aphid Body Color. Science 330, 1102–1104. doi: 10.1126/science.1195463
Vallenet, D., Calteau, A., Dubois, M., Amours, P., Bazin, A., Beuvin, M., et al. (2020). MicroScope: An Integrated Platform for the Annotation and Exploration of Microbial Gene Functions Through Genomic, Pangenomic and Metabolic Comparative Analysis. Nucleic Acids Res. 48, D579–D589. doi: 10.1093/nar/gkz926
van Ham, R. C. H. J., Kamerbeek, J., Palacios, C., Rausell, C., Abascal, F., Bastolla, U., et al. (2003). Reductive Genome Evolution in Buchnera Aphidicola. Proc. Natl. Acad. Sci. 100, 581–586. doi: 10.1073/pnas.0235981100
Van Melderen, L., Saavedra De Bast, M. (2009). Bacterial Toxin–Antitoxin Systems: More Than Selfish Entities? PloS Genet. 5 (3), e1000437. doi: 10.1371/journal.pgen.1000437
Varani, A. M., Siguier, P., Gourbeyre, E., Charneau, V., Chandler, M. (2011). Issaga Is an Ensemble of Web-Based Methods for High Throughput Identification and Semi-Automatic Annotation of Insertion Sequences in Prokaryotic Genomes. Genome Biol. 12, R30. doi: 10.1186/gb-2011-12-3-r30
Wang, X., Zhou, H., Chen, H., Jing, X., Zheng, W., Li, R., et al. (2018). Discovery of Recombinases Enables Genome Mining of Cryptic Biosynthetic Gene Clusters in Burkholderiales Species. Proc. Natl. Acad. Sci. 115, E4255–E4263. doi: 10.1073/pnas.1720941115
Warr, A. R., Giorgio, R. T., Waldor, M. K. (2021). Genetic Analysis of the Role of the Conserved Inner Membrane Protein CvpA in Enterohemorrhagic Escherichia Coli Resistance to Deoxycholate. J. Bacteriol. 203 (6), e00661-20. doi: 10.1128/JB.00661-20
Weeraratne, N., Stodart, B. J., Venturi, V., Hofte, M., Hua, G. K. H., Ongena, M., et al. (2020). Syringopeptin Contributes to the Virulence of Pseudomonas Fuscovaginae, Based on Sypa Biosynthesis Mutant Analysis. Phytopathology 110 (4), 780–789. doi: 10.1094/PHYTO-07-19-0235-R
Weldon, S. R., Oliver, K. M. (2016). “Diverse Bacteriophage Roles in an Aphid-Bacterial Defensive Mutualism,” in The Mechanistic Benefits of Microbial Symbionts Advances in Environmental Microbiology. Ed. Hurst, C. J. (Cham: Springer International Publishing), 173–206. doi: 10.1007/978-3-319-28068-4_7
Wick, R. R., Judd, L. M., Gorrie, C. L., Holt, K. E. (2017). Unicycler: Resolving Bacterial Genome Assemblies From Short and Long Sequencing Reads. PloS Comput. Biol. 13, e1005595. doi: 10.1371/journal.pcbi.1005595
Zaini, P. A., Fogaça, A. C., Lupo, F. G. N., Nakaya, H. I., Vêncio, R. Z. N., da Silva, A. M. (2008). The Iron Stimulon of Xylella Fastidiosa Includes Genes for Type IV Pilus and Colicin V-Like Bacteriocins. J. Bacteriol. 190, 2368–2378. doi: 10.1128/JB.01495-07
Zhao, G., Pease, A. J., Bharani, N., Winkler, M. E. (1995). Biochemical Characterization of gapB-Encoded Erythrose 4-Phosphate Dehydrogenase of Escherichia Coli K-12 and Its Possible Role in Pyridoxal 5’-Phosphate Biosynthesis. J. Bacteriol. 177, 2804–2812. doi: 10.1128/jb.177.10.2804-2812.1995
Keywords: aphid symbiont, bacterial mutualism, genome evolution, metabolic pathways, pathogen, secretion systems, Serratia symbiotica, virulence factors
Citation: Renoz F, Foray V, Ambroise J, Baa-Puyoulet P, Bearzatto B, Mendez GL, Grigorescu AS, Mahillon J, Mardulyn P, Gala J-L, Calevro F and Hance T (2021) At the Gate of Mutualism: Identification of Genomic Traits Predisposing to Insect-Bacterial Symbiosis in Pathogenic Strains of the Aphid Symbiont Serratia symbiotica. Front. Cell. Infect. Microbiol. 11:660007. doi: 10.3389/fcimb.2021.660007
Received: 28 January 2021; Accepted: 14 June 2021;
Published: 29 June 2021.
Edited by:
Ghassan M. Matar, American University of Beirut, LebanonReviewed by:
Alejandro Manzano Marín, University of Vienna, AustriaMira El Chaar, University of Balamand, Lebanon
Copyright © 2021 Renoz, Foray, Ambroise, Baa-Puyoulet, Bearzatto, Mendez, Grigorescu, Mahillon, Mardulyn, Gala, Calevro and Hance. This is an open-access article distributed under the terms of the Creative Commons Attribution License (CC BY). The use, distribution or reproduction in other forums is permitted, provided the original author(s) and the copyright owner(s) are credited and that the original publication in this journal is cited, in accordance with accepted academic practice. No use, distribution or reproduction is permitted which does not comply with these terms.
*Correspondence: François Renoz, ZnJhbmNvaXMucmVub3pAdWNsb3V2YWluLmJl