- 1Department of Pulmonary and Critical Care Medicine, Chinese PLA General Hospital, Beijing, China
- 2Laser Institute, Qilu University of Technology (Shandong Academy of Sciences), Qingdao, China
- 3State Key Laboratory of Precision Measurement Technology and Instrument, Department of Precision Instruments, Tsinghua University, Beijing, China
- 4Department of Respiratory and Critical Care Medicine, Beijing Chao-Yang Hospital, Capital Medical University, Beijing, China
Staphylococcus aureus (S. aureus) is one of the most common clinical pathogenic bacteria with strong pathogenicity and usually leads to various suppurative infections with high fatality. Traditional bacterial culture for the detection of S. aureus is prone to diagnosis and antimicrobial treatment delays because of its long-time consumption and low sensitivity. In this study, we successfully developed a quantum dots immunofluorescence biosensor for S. aureus detection. The biosensor combined the advantages of biosensors with the high specificity of antigen-antibody immune interactions and the high sensitivity and stability of quantum dots fluorescence. The results demonstrated that the biosensor possessed high specificity and high sensitivity for S. aureus detection. The detection limit of S. aureus reached 1 × 104 CFU/ml or even 1 × 103 CFU/ml, and moreover, the fluorescence intensity had a significant positive linear correlation relationship with the logarithm of the S. aureus concentration in the range of 103–107 CFU/ml (correlation coefficient R2 = 0.9731, P = 0.011). A specificity experiment showed that this biosensor could effectively distinguish S. aureus (1 × 104 CFU/ml and above) from other common pathogenic (non-S. aureus) bacteria in nosocomial infections, such as Klebsiella pneumoniae, Pseudomonas aeruginosa, Acinetobacter baumannii and Escherichia coli. Additionally, the whole detection procedure spent only 2 h. In addition, the biosensor in this study may not be affected by the interference of the biofilm or other secretions since the clinical biological specimens are need to be fully liquefied to digest and dissolve viscous secretions such as biofilms before the detection procedure of the biosensor in this study. In conclusion, the biosensor could meet the need for rapid and accurate S. aureus detection for clinical application.
Introduction
Staphylococcus aureus (S. aureus) is one of the most common pathogenic bacteria in nosocomial infections and accounted for 9.0% among all clinical isolates of strains in China reported by the Antimicrobial Surveillance Network (CHINET) in 2018 (Hu et al., 2018). Due to its production of various toxins and invasive enzymes, S. aureus usually leads to various suppurative infections, such as scabies, carbuncles, traumatic infections, pneumonia and sepsis, and even septic shock with high mortality (Gries et al., 2020; Karer et al., 2020). The traditional detection method for S. aureus, bacterial culture is prone to diagnosis and antimicrobial treatment delays because of its long-time consumption and low sensitivity (Stürenburg, 2009; Tenover et al., 2019). Therefore, rapid and accurate S. aureus detection is of great significance for anti-infection efforts to reduce its fatality.
Recently, many new detection methods, including molecular biology and immunological methods, have been applied to S. aureus detection (Tenover et al., 2019; Andersson et al., 2020). Among them, polymerase chain reaction (PCR) related molecular biology technology has high sensitivity and specificity (McIver et al., 2020), but its widespread clinical application is limited because a very small amount of contamination can easily lead to false positives (Renevey and Thur, 2018). However, immunofluorescence technology based on antigen-antibody specificity can ensure the high specificity required by the clinic (Nishiyama et al., 2020). Simultaneously, increasingly advanced fluorescence labeling technology and fluorescence detection technology have continuously increased the high sensitivity of immunofluorescence technology.
The development of fiber optic biosensor has received much attention. These biosensors with an optical fiber probe as the light carrier and the fluorescence detector have advantages to realize the compactness, miniaturization and portable of the instrument (Wolfbeis, 2000). They were widely applied in medical science (Ma et al., 2020), biological engineering (Yu et al., 2019), food industry (Chaudhari et al., 2020), and environmental monitoring (Zhou et al., 2021). We developed an optical fiber probe-based immunofluorescence biosensor that combined the advantages of antigen-antibody specificity, fluorescence labeling and transmission of fluorescence signals along the optical fiber. The surface of the probe of the biosensor was immobilized with Escherichia coli-specific antibodies, and the detection sensitivity for E. coli reached 103 CFU/ml (CFU, colony forming units, an estimate of viable bacterial numbers usually existing in the form of colony), with high specificity (Liu et al., 2014; Zhang et al., 2014). However, the common fluorescence labelling techniques applied in their biosensor still have disadvantages, such as short fluorescence lifetime and spectral overlap affecting detection accuracy (Pu and Liu, 2009). In recent years, quantum dots have been increasingly used as fluorescence markers in labelling immunoassays (Song et al., 2017; Hao et al., 2020).
Quantum dots (QDs) is a new class of artificial luminescent fluorophores with the diameter of about 2–10 nm. QDs has obvious optical advantages that could facilitate the brighter emission and the more accurate detection of fluorescence signal, such as high fluorescence emission, long-life time with high photostability and large Stocks’ shifts (Wu et al., 2012; Abdelhamid and Wu, 2013). Additionally, the surface of QDs can be flexibly modified according to the needs of the application purpose and have been widely applied in various biological science researches. For example, Hani Nasser Abdelhamid and Hui-Fen Wu had reported a selective biosensing of S. aureus using QDs modified with Chitosan (Abdelhamid and Wu, 2017). Liu et al. (2011) had detected ferritin in human serum based on avidin–biotin system using the avidin-conjugated QDs. The results of these studies above had showed the optical advantages of quantum dots in biological detection.
Therefore, based on the abovementioned biosensor we developed and combined with the advantages of quantum dots fluorescence labeling technology, the purpose of this study is to achieve a fast, accurate sensing for S. aureus detection with high specificity and sensitivity.
Methods
Experimental Principle
The structural schematic diagram of the fiber optic biosensor is shown in Figure 1A. A semiconductor laser (Shanghai Fiblaser Technology Co., Ltd., Shanghai, China) with wavelength of 532 nm and a modulation frequency of 1 kHz was selected as the excitation light source, because the excitation wavelength of QDs is ≤565 nm. The laser was linked to one input of an optical fiber coupler (2 × 2, couple ratio: 20:80) through its pigtail fiber. The combination tapered fiber probe was connected to the 20% end of the coupler using the fluorescence optical fiber connector. The input laser light was transmitted to the fiber probe to excite QDs that bound with antibody specifically captured on the fiber probe surface (Figure 1B). The fluorescence signal with 605 nm emission wavelength of QDs was also collected by the fiber probe, and sent back to the other input end of the fiber coupler. The fluorescence signal was filtered through a long-pass filter (FEL0600, Thorlabs, USA) with a cut-on wavelength of 600 nm in order to remove the excitation light (532 nm). Then, the fluorescence signal was injected into an avalanche photo-diode (APD, LCSA500-01, Laser Components, Germany) for photo-electronic conversion, and detection in data acquisition (DAQ) system. To enhance signal resolution, digital signals from DAQ system were further processed by a correlation detection system based on phase-locked filtering algorithm, which was performed with LabVIEW (Liu et al., 2014). Finally, the fluorescence signal was displayed on the computer screen. The fiber optic immunofluorescence biosensor prototype was shown in Figure 1C.
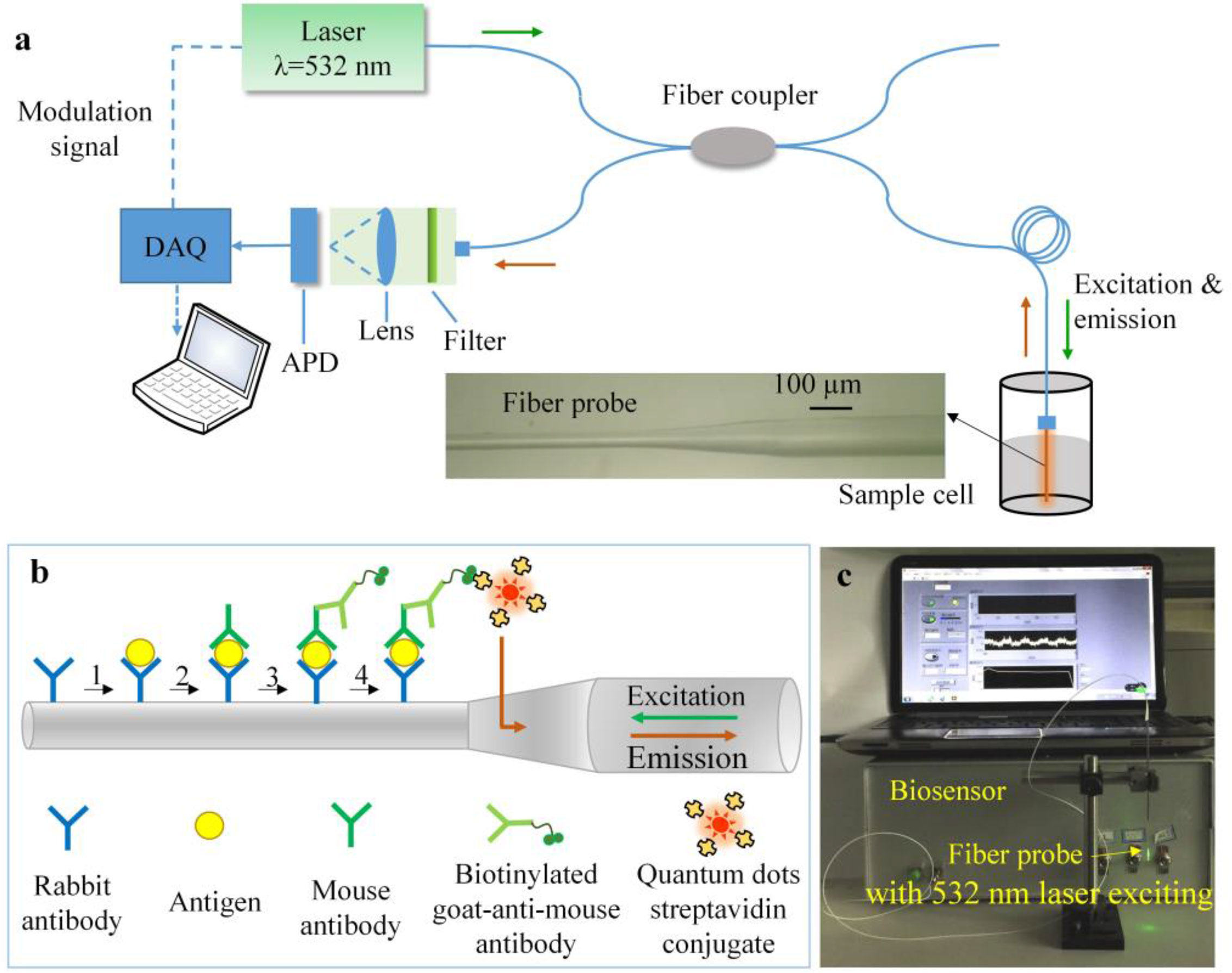
Figure 1 Principle of the fiber optic biosensor. (A) Schematic diagram of the fiber optic biosensor. A 532 nm laser was employed as excitation light that transmitted into the fiber probe through the fiber coupler. The fluorescence signal was collected and propagated back through the fiber coupler, filter, lens, avalanche photo-diode (APD), data acquisition (DAQ) system, and at last displayed on the computer. (B) The principle to identify S. aureus using the fiber probe. The rabbit polyclonal anti-S. aureus antibody immobilized on the probe surface was used to capture as many S. aureus as possible in order to improve the sensitivity. When the S. aureus strains were captured, the mouse monoclonal anti-S. aureus antibody specifically binds to the captured S. aureus again to form a double-antibody sandwich structure. The choice on the two antibodies could ensure that the probe has both high sensitivity and high specificity in capturing and detecting S. aureus. The biotin labeled goat-anti-mouse IgG antibody was bound to detecting antibody, and then avidin-labeled quantum dots (QDs) bound with the biotin and emitted fluorescence signal with the 532 nm laser excitation, the emitted fluorescence signal was collected at 605 nm wavelength. Moreover, the signal cascade amplification of the biotin–avidin–QDs system was used to enhance the fluorescence signal and improve the sensitivity. (C) Prototype of the fiber optic biosensor. All the components were encapsulated in the box except the fiber probe and sample cell. The fiber probe was fixed by a fluorescence connector to the fiber coupler by the fiber adapter in the front-panel of the box.
In this study, the immune sandwich principle of antibody–antigen specific capture of S. aureus was used to improve the sensitivity and specificity of S. aureus detection. We had selected two different kind of antibodies, among them, the rabbit polyclonal anti-S. aureus antibody (ab20920) immobilized on the probe surface was used to capture as many S. aureus as possible in order to improve the sensitivity of the biosensor. When the S. aureus strains were captured, the mouse monoclonal anti-S. aureus antibody (ab37644) specifically binds to the captured S. aureus again to form a double-antibody sandwich structure. Therefore, the choice on the two antibodies could ensure that the probe has both high sensitivity and high specificity in capturing and detecting S. aureus. As shown in Figure 1B, the activated fiber probe immobilized with rabbit polyclonal antibody to S. aureus (ab20920) defined as capture antibody was firstly used to capture S. aureus. Secondly, the fiber probe bound with bacteria was incubated in the mouse monoclonal antibody (ab37644), which was specific for S. aureus and defined as detecting antibody to bind to S. aureus. Thirdly, the biotin labelled goat-anti-mouse IgG antibody (GaMIgG) was introduced to bind to the mouse monoclonal antibody (ab37644). Finally, avidin-labelled QDs (605 nm emission wavelength) was employed for fluorescence labelling by the specific binding between biotin and avidin. Moreover, the signal cascade amplification principle of the biotin–avidin–QDs system was used to enhance the fluorescence signal and improve the sensitivity and stability of S. aureus detection.
Experimental Materials
The fiber optic immunofluorescence biosensor was developed by the Department of Precision Instruments, Tsinghua University. The fiber probe was made of a step-index multimode silicon optical fiber (NA = 0.22, 105 μm core/125 μm cladding, Beijing Scitlion Technology Co., Ltd., Beijing, China), and the composition of the silicon is mainly Silicon dioxide (SiO2). The rabbit polyclonal anti-S. aureus antibody (ab20920) and mouse monoclonal anti-S. aureus antibody (ab37644) was purchased from Abcam (USA). Biotin labeled goat-anti-mouse IgG antibody (GaMIgG) and avidin-labeled QDs (QDs-605, its emission wavelength is 605 nm and its excitation wavelength of the laser in this study is 532 nm) were purchased from Wuhan Jiayuan Quantum Dots Co., Ltd., (Wuhan, China). The QDs labeled to avidin applied in this study is a kind of silicon (Si) nano quantum dot. Sodium borohydride (NaBH4) was purchased from Shanghai McLean Biochemical Technology Company, and Trypticase Soy Broth bacterial culture medium (TSB) was purchased from Beijing Aokexing Biotechnology Company. Bovine serum albumin (BSA, P1621), phosphate buffer (1×PBS, B1203), Phosphate Tween buffer (1×PBST, A1007), and other non-special reagents were purchased from Beijing Pulilai Gene Technology Corporation in China.
All experimental bacterial strains were derived from the clinical microbiological laboratory of our hospital (Chinese PLA General Hospital), including the S. aureus standard strain (strain number ATCC 29213), Pseudomonas aeruginosa (ATCC 27853), Acinetobacter baumannii (ATCC 19606), E. coli (ATCC 25922), Staphylococcus epidermidis (clinically isolated from a patient), Klebsiella pneumoniae (clinically isolated from a patient) and five clinical S. aureus strains isolated from sputum specimen of patients with chronic obstructive pulmonary disease complicated with pulmonary infection, the five clinical S. aureus strains were respectively named as SA-1, SA-2, SA-3, SA-4 and SA-5. Among the five clinical S. aureus strains, the two S. aureus strains (SA-4 and SA-5) were from patients with severe lung infection, and both the two S. aureus strains were sensitive to vancomycin according to the antimicrobial susceptibility results. The two patients were also treated with vancomycin for anti-infective treatment, but the clinical effect was evaluated to be ineffective after excluding the influence of other clinical factors. It was speculated that the two S. aureus strains might have produced the biofilm in the infection in body, resulting in ineffective antimicrobial treatment.
Bacterial Preparation
Bacterial culture was performed in a biosafety cabinet in the clinical microbiological laboratory. The stored S. aureus standard strain (ATCC 29213 strain) was cultured on solid medium for 24 h by streaking to grow single colonies. A single colony was picked and inoculated in 20 ml of TSB liquid medium for 16 h to the logarithmic growth phase of the bacteria. The bacteria in the logarithmic growth phase were centrifuged at 5,000 revolutions per minute (rpm) for 5 min, and the supernatant was aspirated. The remaining bacteria were washed twice with PBS, and the bacterial concentration was determined using a haemocytometer. This method is to take a certain volume of cell suspension of the sample and place it in the counting chamber of a hemocytometer, and then observe and count by microscope. Since the volume of the counting chamber is constant, the number and the concentration of bacteria in the sample can be calculated according to the number of bacteria in the counting chamber of the hemocytometer. This method is simple and easy to implement, and the result can be obtained immediately. This method is not only suitable for cell and bacterial counting, but also for fungi and fungal spores. The concentration of the bacterial suspension was adjusted to 1 × 108 CFU/ml with PBS and then serially diluted 10-fold six times for subsequent experiments, producing 108, 107, 106, 105, 104, and 103 CFU/ml concentrations.
Clinical isolates of the five S. aureus strains mentioned above were derived from sputum specimen of chronic obstructive pulmonary disease (AECOPD) patients by bacterial culture, and identified by automatic microorganism identification instrument (VITEK 2). Subsequently, the clinical isolated strains and other strains used for this study were also cultured in the same way as the S. aureus standard strain (ATCC 29213).
Preparation of the Antibody-Immobilized Optical Fiber Probes of the Biosensor
A taper-and-cylinder combination optical fiber was prepared as the fiber probe. It was fabricated with a simple static tube etching method with 40% hydrofluoric acid (HF) as the etchant (Liu et al., 2014; Zhang et al., 2014). The fiber probe (inset in Figure 1A) consisted of a tapered section and a sensing region, whose length were approximately 0.03 and 1 cm, respectively. The diameter of tapered section was etched from 125 μm to about 45 μm within the length of 300 μm, and the diameter of sensing region was maintained about 45 μm for matching the V number of fiber (Anderson et al., 1994).
As shown in Supplemental Material Figure S1, the fiber probe was immersed in a piranha solution to remove the residual HF. And then, it was activated by hydroxylation, silanization, glutaraldehyde cross-linking, and covalent conjugation of the rabbit polyclonal anti-S. aureus antibody (ab20920, 20 × 10−3 g/ml in Tris–HCl buffer, which is the optimal concentration proved in the followed exploratory research) to capture the S. aureus specifically. After antibody immobilization, the fiber probe was immersed in NaBH4 solution to reduce the interference of background fluorescence caused by aldehyde group (Clancy and Cauller, 1998). And the remaining aldehyde groups and non-specific binding cites of the fiber probe were blocked by BSA solution. The activated fiber probes were stored at 4°C for future use.
The Exploratory Research on the Optimal Concentration of the Antibody Used to Immobilize Onto the Probe Surface
As the same as the rabbit polyclonal anti-S. aureus antibody (ab20920) immobilized on the probe surface, the biotin-labeled goat anti-mouse IgG antibody used in this study was also a polyclonal antibody and it was labeled with biotin. Therefore, it was used as an analog to explore the optimal concentration of the rabbit polyclonal antibody (ab20920) used to immobilize onto the probe surface. The schematic diagram of experimental principle and the results were exhibited in Supplemental Material Figure S2. The processes of the research were as followed.
The biotin-labeled goat anti-mouse IgG antibody was diluted with Tris–HCl buffer to different antibody concentrations (2, 5, 10, 20, 50, 100 × 10−3 g/ml) as shown in the Supplemental Material Figure S2 and the Tris–HCl buffer was as the control group without antibody. Firstly, the probe was incubated in the biotin-labeled goat anti-mouse IgG antibody in various concentrations mention in the Supplemental Material Figure S2b at 37°C overnight to allow the aldehyde groups on the probe to covalently bind amino groups of the antibody and made the antibody immobilized onto the probe surface. Secondly, after antibody immobilization, the fiber probe was immersed in NaBH4 solution to reduce the interference of background fluorescence caused by aldehyde group. And the remaining aldehyde groups and non-specific binding cites of the fiber probe were blocked by BSA solution. Thirdly, the probes were immersed in a biotin-labeled goat anti-mouse IgG antibody dilution solution (1:100 dilution) for 30 min at 37°C. Fourthly, the probes were washed with PBST and were immersed in the avidin-labeled quantum dots solution (QDs-605, 1:100 dilution) for 30 min at 37°C. Finally, the probes were washed with PBST for three times and then were connected to the biosensor and turn on the laser. After running 200 s, the fluorescence signal was collected and displayed on the computer. As proven in the exploratory experiment of the fluorescence signal-time response trace mentioned below, the effective response interval was defined from 50 s to 200 s and the average values of the fluorescence signal of the effective time interval calculated by the computer could be the optimal detection values. Each of the above experimental groups was repeated three times and their optimal detection values of the fluorescence signal were collected for statistical analysis of the differences between each group.
As shown in Supplemental Material Figure S2, the results had confirmed that the 20 × 10−3 g/ml was the optimal concentration of the antibody used to immobilize onto the probe surface.
The SEM and EDX Images of the S. aureus Captured on the Optical Fiber Probes
The SEM images of the S. aureus captured on the optical fiber probes were performed to evaluate the capture capacity of the prepared antibody-immobilized optical fiber probes for S. aureus. There were six groups in the experiment. Among them, the five experimental groups (five groups) were the prepared S. aureus standard strain (ATCC 29213) with a concentration gradient of 108–104 CFU/ml. In addition, the control group (one group) was the undiluted S. aureus suspension (1 × 108 CFU/ml) and the non-antibody-immobilized optical fiber probe, which was used to detect whether the non-antibody-immobilized optical fiber probe could capture non-S. aureus strains. Each probe was immersed in the 1 ml S. aureus suspension of the various concentrations for 30 min at 37°C, and then the probe was removed and washed three times with 1 ml of PBST to remove non-specific adsorption. All the probes were imaged by the scanning electron microscope (SEM, Hitachi S-5500). In addition, during the process of the SEM imaging of the probe surface, we also analyzed the energy spectrums of the SEM image of S. aureus captured on the surface of the fiber probe. Energy spectrums were recorded by the Energy dispersive X-ray spectrometry (EDX) combined with SEM (Hitachi S-5500). The principle of the EDX is as follow.
The research purpose of the SEM and EDX images is to evaluate whether there were captured bacteria and to exclude some non-bacterial false light spots in the SEM images using EDX. In all SEM images of various groups, there may be areas where bacteria are captured and blank areas where bacteria are not captured. The result exhibited in Figure 2 was just used to prove that the EDX technology during SEM image analysis can ensure that SEM image analysis can effectively identify bacteria captured on the probe.
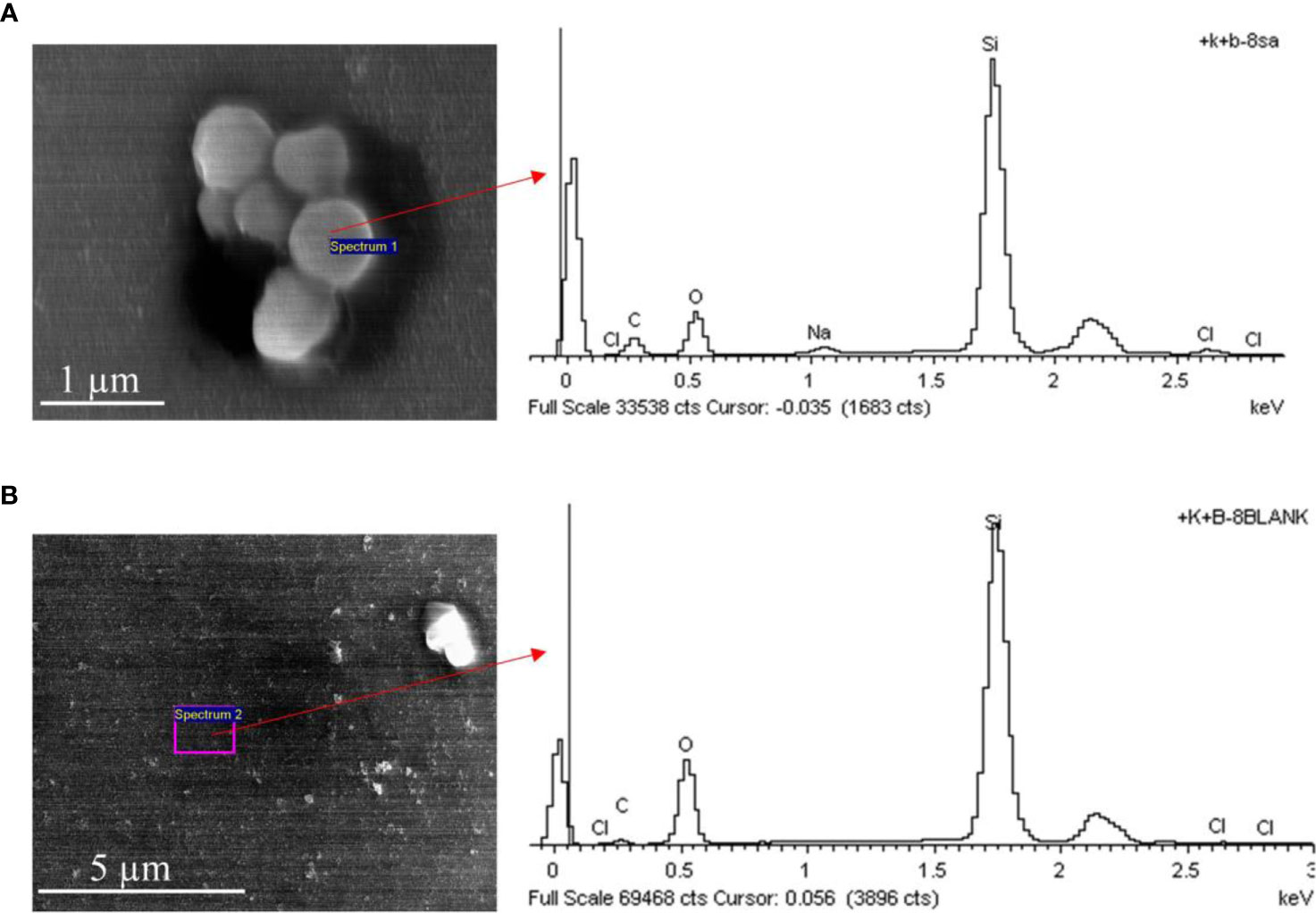
Figure 2 The SEM and EDX images of S. aureus and blank area on the surface of the fiber probe. (A) S. aureus captured on the fiber probe on the surface of the fiber probe. (B) The blank area of the fiber probe without S. aureus. The composition of the silicon optical fiber probe is mainly Silicon dioxide (SiO2), and the surface of the probe is rich in Si atoms and O atoms. While the S. aureus and other bacteria are organisms with carbohydrate as the main component, which is rich in Carbon (C) atoms and Sodium (Na) atoms. Therefore, the EDX image showed that the peak of the relative content (atomic percentage, Atomic %) of the C atoms and Na atoms were higher in the area where S. aureus were captured (A) than that in the blank area (B). However, since the background of the SME imaging is the silicon optical fiber probe, the percentages of Si atoms and O atoms are still dominant, regardless of the presence or absence of S. aureus and other bacteria. By this way, the presence or absence of S. aureus and other bacteria on the surface of the probe can be distinguished. SEM, scanning electron microscope; EDX, energy dispersive x-ray spectrometry.
The Detection Process of the Sample by the Biosensor and the Fluorescence Signal-Time Response Trace
Using the S. aureus standard strains, we exploratory studied the fluorescence signal responses corresponding to bacterial concentrations and the fluorescence signal-time response trace of the biosensor in the detection process. The exploratory experiment only included two samples. One sample was the S. aureus standard strains at the 1 × 107 CFU/ml concentration, the other sample was PBS control without any bacteria (the concentration of the S. aureus was 0). The detection process of the samples was as follows.
Firstly, two prepared antibody-immobilized optical fiber probes were immersed in the two samples solutions above for 30 min at 37°C for S. aureus capture. Secondly, both the probes were washed three times with PBST to remove non-specific adsorption, and then the probes were immersed in the mouse monoclonal anti-S. aureus antibody dilution solution (ab37644, 100 × 10−3 g/ml) for 30 min at 37°C for formatting the double antibody sandwich structure. Thirdly, after washed with PBST, the probes were immersed in a biotin-labeled goat anti-mouse IgG antibody dilution solution (1:100 dilution) for 30 min at 37°C. Fourthly, after washed with PBST, the probes were immersed in an avidin-labeled quantum dots solution (QDs-605, 1:100 dilution) for 30 min at 37°C. Finally, the probes were washed with PBST and were connected to the biosensor. Then, turn on the laser with the 532 nm wavelength excitation light. The emitted fluorescence signal was collected at 605 nm wavelength for 200 seconds (200 s) or longer and the fluorescence signal-time response trace was displayed on the computer.
According to the signal-time response trace, the effective response interval could be defined from 50 to 200 s and the average values of the fluorescence signal of the effective time interval calculated by the computer could be the optimal detection values applied in this study.
Biosensor Sensitivity Experiment
The sensitivity experiment was performed to evaluate the detection limit of S. aureus provided by the optical fiber probe-based fluorescence biosensor. There were seven groups in the experiment: the control group was PBS, and the other six experimental groups were the prepared S. aureus suspensions with the concentration gradients of 108–103 CFU/ml. The detection process of all the samples by the biosensor was as the same as the detection process in exploratory experiment of the fluorescence signal-time response trace mentioned above. And each of the above experimental groups was repeated three times and their optimal detection values of the fluorescence signal were collected for statistical analysis of the differences between each group.
Biosensor Specificity Experiment
To evaluate the specificity of the biosensor, five most common clinical pathogenic non-S. aureus strains were employed in the experiment. The bacterial strains were S. epidermidis, K. pneumoniae, P. aeruginosa, A. baumannii and E. coli. All the strains were cultured to the logarithmic growth phase, and the bacterial suspension concentrations were all adjusted to 1 × 107 CFU/ml with PBS. The experiment consisted of eight groups, including the five experimental groups (the five non-S. aureus strains mentioned above), two positive control groups (1 × 104 CFU/ml and 1 × 107 CFU/ml S. aureus suspensions) and 1 blank control group (PBS). The detection process of all the samples by the biosensor was as the same as the detection process in exploratory experiment of the fluorescence signal-time response trace mentioned above. And each of the above experimental groups was repeated three times and their optimal detection values of the fluorescence signal were collected for statistical analysis of the differences between each group.
Clinical Application of the Biosensor
To verify the sensitivity (detection limit) of the biosensor for clinically isolated S. aureus strains, five S. aureus strains (named SA-1 to SA-5) isolated from different patients in our hospital were detected by the biosensor. Each of the 5 S. aureus strain suspensions above was adjusted to two concentrations (1 × 103 CFU/ml and 1 × 104 CFU/ml). A S. aureus-free PBS solution was used as a negative control group. Thus, a total of 11 groups were in the experiment. The detection process of all the samples by the biosensor was as the same as the detection process in exploratory experiment of the fluorescence signal-time response trace mentioned above. And each of the above experimental groups was repeated three times and their optimal detection values of the fluorescence signal were collected for statistical analysis of the differences between each group.
Statistical Analysis
SPSS 19.0 software (IBM Corp., Armonk, NY, USA) was used for statistical analysis of the experimental data in this study. All data are tested for normal distribution and homogeneity of variance. All data conforming to the normal distribution were analyzed by two independent sample t-tests for statistical differences between groups. On the contrary, the rank sum test is used to analyze the statistical differences between groups for non-normally distributed data. For the linear regression relationship between the fluorescence intensity and the concentration gradient of S. aureus, the linear regression equation was established and the correlation coefficient (r) was calculated. All statistical analyses were performed using two-sided tests, and P <0.05 was considered to be statistically significant.
Results
The SEM and EDX Images of S. aureus Captured by the Antibody-Immobilized Optical Fiber Probes
As exhibited in Figure 3, the SEM images of the optical fiber probes capturing S. aureus show that the higher the concentration of the S. aureus suspension is, the more S. aureus can be captured by the probes. However, with the increase of the S. aureus suspension concentration (from 1 × 104 to 1 × 108 CFU/ml), the capture amounts of the S. aureus strain on the surface of the probes gradually increased in S. aureus suspensions at various concentrations.
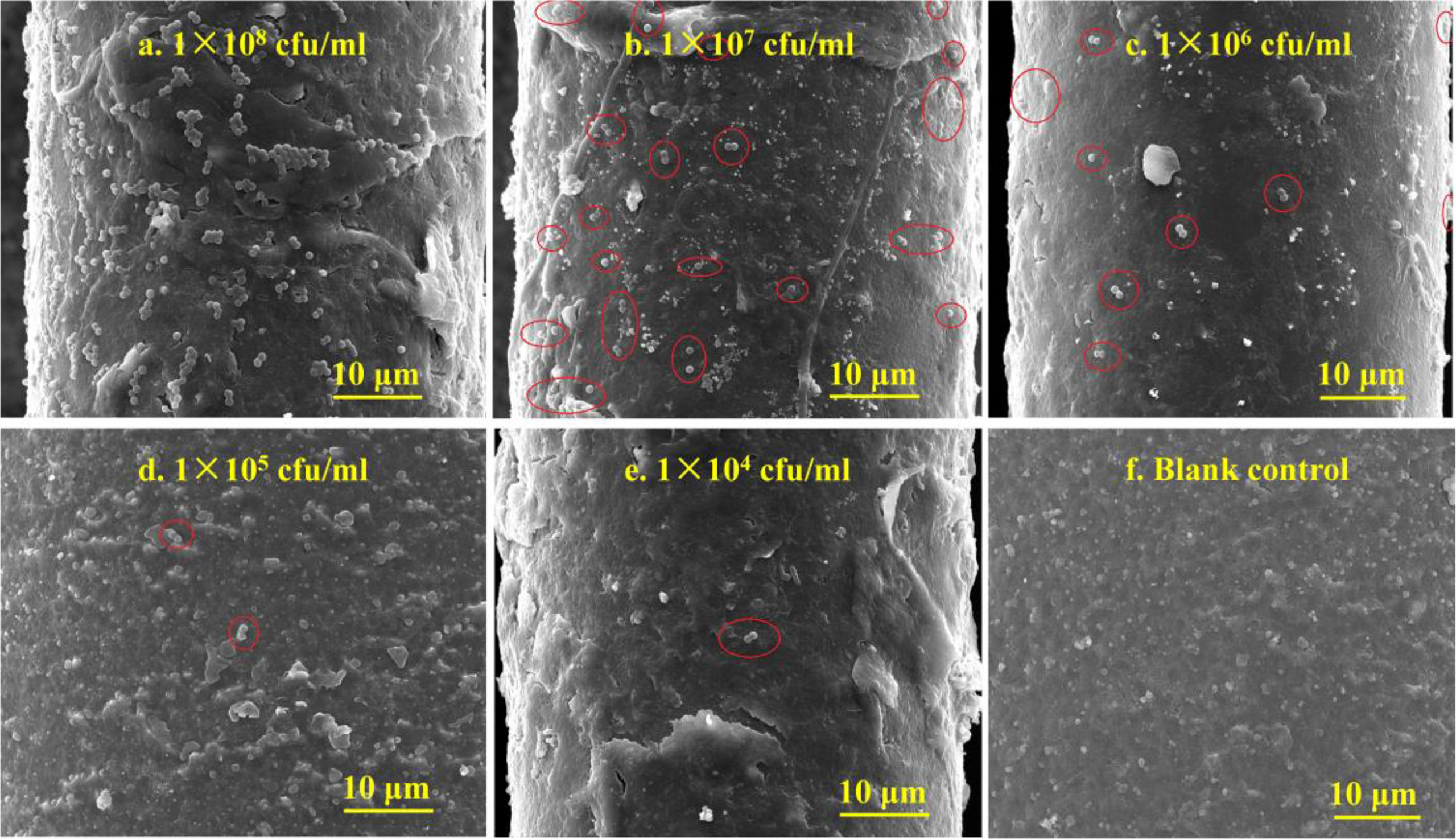
Figure 3 The SEM images of the S. aureus captured by the antibody-immobilized optical fiber probes. Figures (A–E) show the captured images of bacterial concentration from 1 × 108 to 1 × 104 CFU/ml respectively, while Figure (F) shows the blank control group without bacterial capture. The probes could capture more S. aureus with the increase of the concentration gradients of the S. aureus suspension.
In addition, the energy spectrums were recorded by the energy dispersive X-ray spectrometry (EDX) combined with SEM images. The comparation of the SEM and EDX image of between S. aureus and the blank area on the surface of the fiber probe were shown in Figure 2. The precise EDX analysis is shown in Supplemental Material Table S1. And the results suggested that the S. aureus strain contain Carbon (C) atoms and Sodium (Na) atoms, and the mass percentage (Weight %) and atomic percentage (Atomic %) of carbon (C) and sodium (Na) were higher than the blank area of the fiber probe. By this way, the presence or absence of S. aureus and other bacteria on the surface of the probe can be distinguished.
The Fluorescence Signal-Time Response Trace and the Optimal Detection Values of the Biosensor
The fluorescence signal-time response trace during a complete test cycle (200 s) was shown in Figure 4. The time response curve shows that the signal reached the maximum value instantly after the laser turning on and then decreased back gradually. This was caused by photo-bleaching (Liu et al., 2014; Zhang et al., 2014). After decreasing back, the fluorescence signal gradually tended to be stable. The burr phenomenon on the curve may be the electrical signal interference during the instrument operation. The results of the trace showed that the effective response interval could be defined from 50 to 200 s and the average values of the fluorescence signal of the effective time interval calculated by the computer could be the optimal detection values applied in this study. In addition, the results also showed that the signal response of the S. aureus at the 107 CFU/ml concentration was significantly higher than that of 0 CFU/ml (PBS control without any bacteria) and the biosensor might be used to distinguish S. aureus at different concentrations.
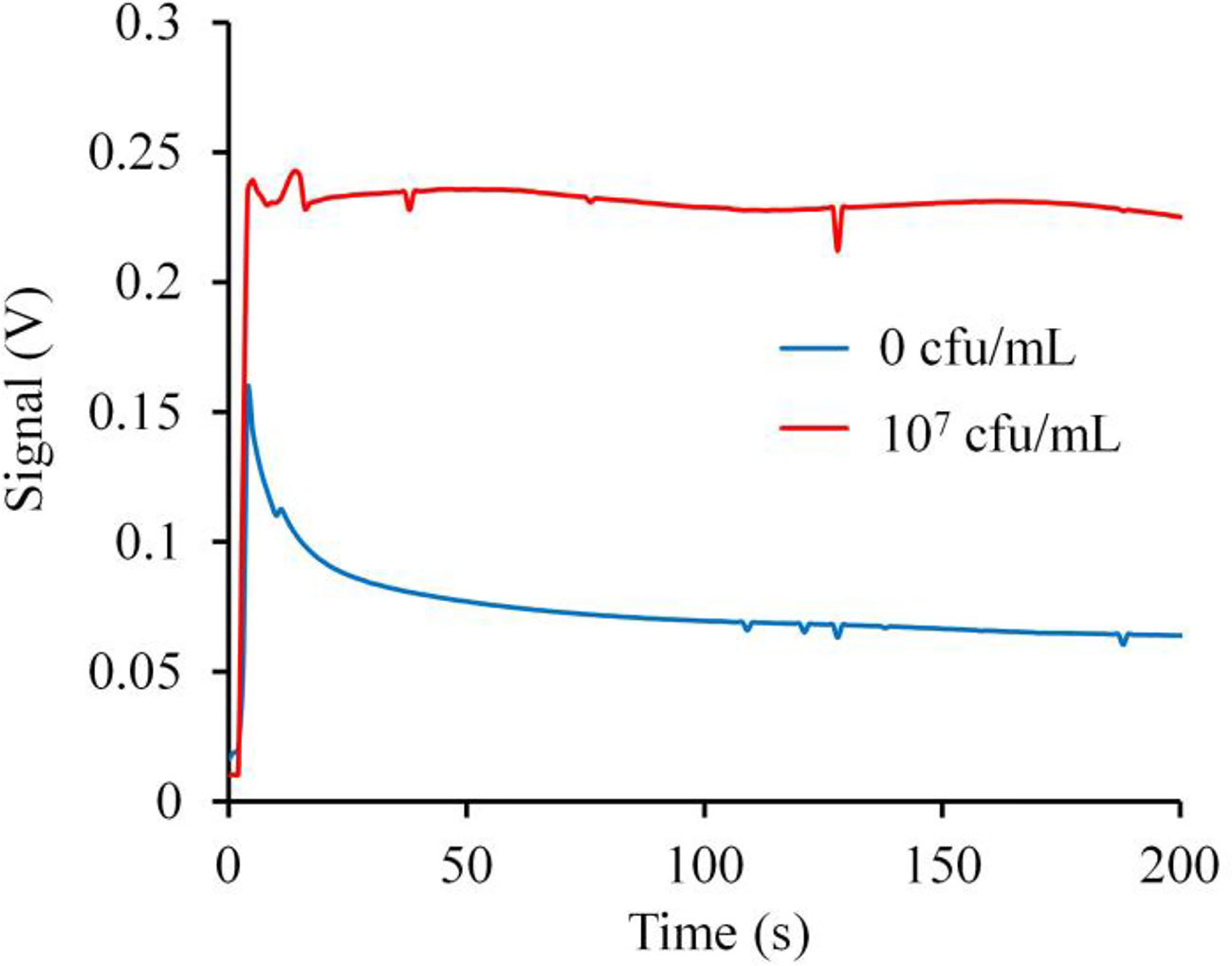
Figure 4 The fluorescence signal-time response trace and the optimal detection values applied in this study. The time response curve shows that the signal reached the maximum value instantly after the laser turning on and then decreased back gradually. After decreasing back, the fluorescence signal gradually tended to be stable. The trace showed that the effective response interval could be defined from 50 to 200 s and the average values of the fluorescence signal of the effective time interval calculated by the computer could be the optimal detection values applied in this study. In addition, the results also showed that the signal response of the S. aureus at the 107 CFU/ml concentration was significantly higher than that of 0 CFU/mL and the biosensor might be used to distinguish S. aureus at different concentrations.
Biosensor Sensitivity Results
The signal corresponding to fluorescence intensity and significant difference between the S. aureus concentration groups are shown in Figures 5A, B. Signal-time response trace during a complete test cycle (200 s) with the S. aureus concentration gradients from 0 to 1 × 108 CFU/ml is shown in Figure 5A. The histogram in Figure 5B shows the average signal measured in triplicate at different concentrations of S. aureus. Compared with the signal of the blank control group, the fluorescence intensity of the 1 × 103 CFU/ml concentration group was higher but not significantly (P >0.05). However, the fluorescence intensity of all concentrations ≥1 × 104 CFU/ml was significantly higher than that of the blank control (P <0.05). Moreover, in the concentration range of 1 × 104 CFU/ml to 1 × 107 CFU/ml, the fluorescence intensity gradually increased with increasing S. aureus concentration, and there was a dose-effect relationship. Surprisingly, the fluorescence intensity of the 1 × 108 CFU/ml group was lower than that of the 1 × 107 CFU/ml experimental group.
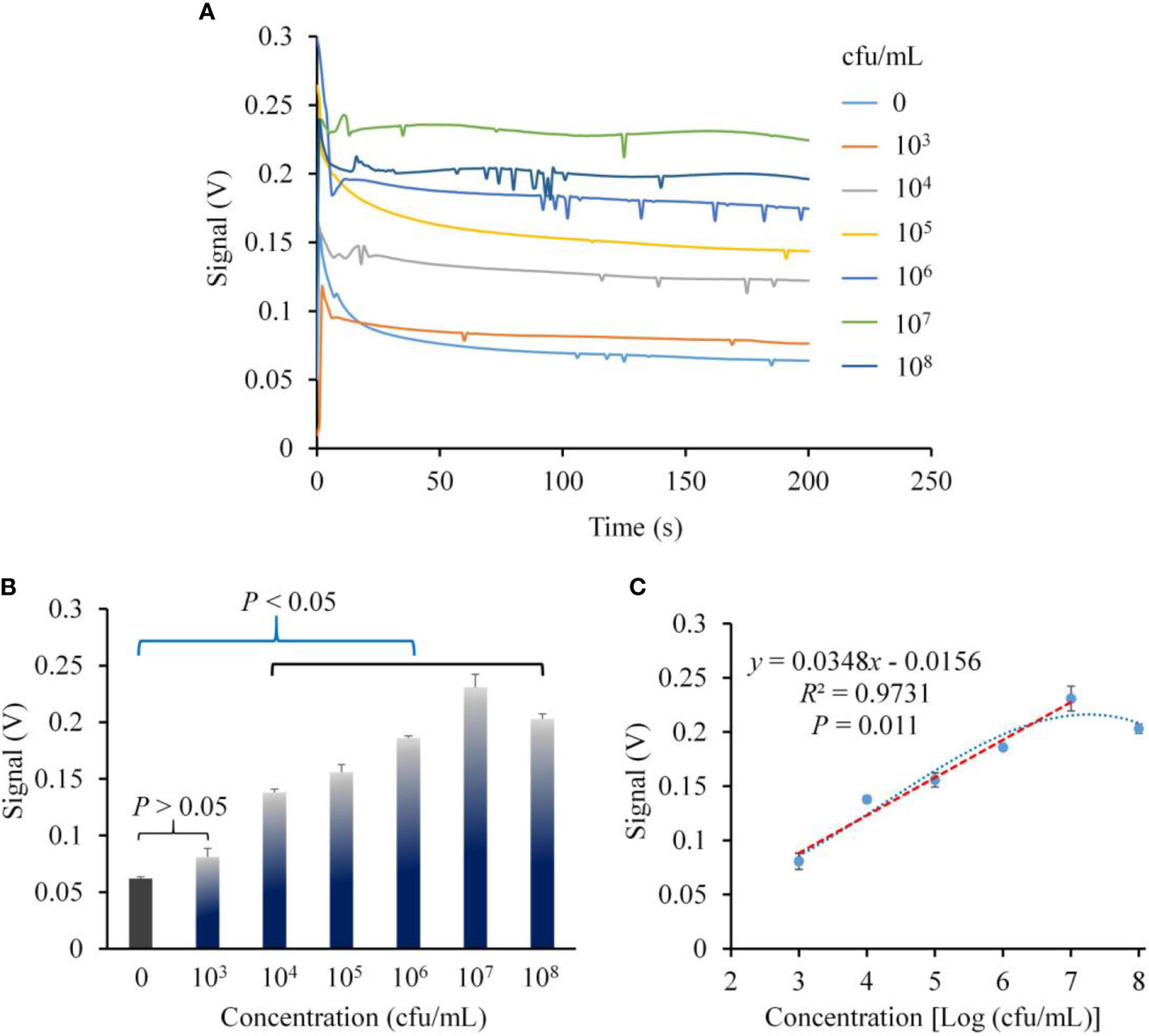
Figure 5 The sensitivity of the biosensor for the detection of S. aureus. (A) Signal-time response trace during a complete test cycle (200 seconds, 200 s) with the bacterial concentration gradients from 0 to 108 CFU/ml, and the signals value at the 200 s were applied to the statistical analysis in (B) and (C, B) The optimal signal detection values of the fluorescence intensity of S. aureus at various concentration gradients and their statistical analysis showed that the sensitivity was 104 CFU/ml. (C) The linear regression curve of signal versus the logarithm of the S. aureus concentration gradients. There was a significant positive linear correlation relationship with the fluorescence intensity in the range of 103–107 CFU/ml concentration (correlation coefficient R2 = 0.9731, P = 0.011). Error bars in (B, C) are based on standard deviations (n = 3).
To evaluate the linear correlation between fluorescence intensity and S. aureus concentration, a linear regression curve of fluorescence intensity and S. aureus concentrations was established (Figure 5C). The results showed that the logarithm of the S. aureus concentration had a significant positive linear correlation relationship with the fluorescence intensity in the range of 103–107 CFU/ml (correlation coefficient R2 = 0.9731, P = 0.011). The linear regression equation is y = 0.0348x − 0.0156. Therefore, this biosensor could achieve quantitative detection of S. aureus. Correspondingly, the approximate concentration of S. aureus in the samples to be tested might be estimated by the fluorescence intensity.
Biosensor Specificity Experiment
The fluorescence intensity and the significant difference between S. aureus and five non-S. aureus strains are shown in Figure 6. Signal-time response trace during a complete test cycle (200 s) with the different kinds of pathogenic bacteria are shown in Figure 6A. Histogram in Figure 6B suggests that the fluorescence intensities of all non-S. aureus groups were not significantly different from that of the blank control group (P >0.05), but all were significantly lower than those of both S. aureus groups (1 × 104 CFU/ml and 1 × 105 CFU/ml, P <0.05).
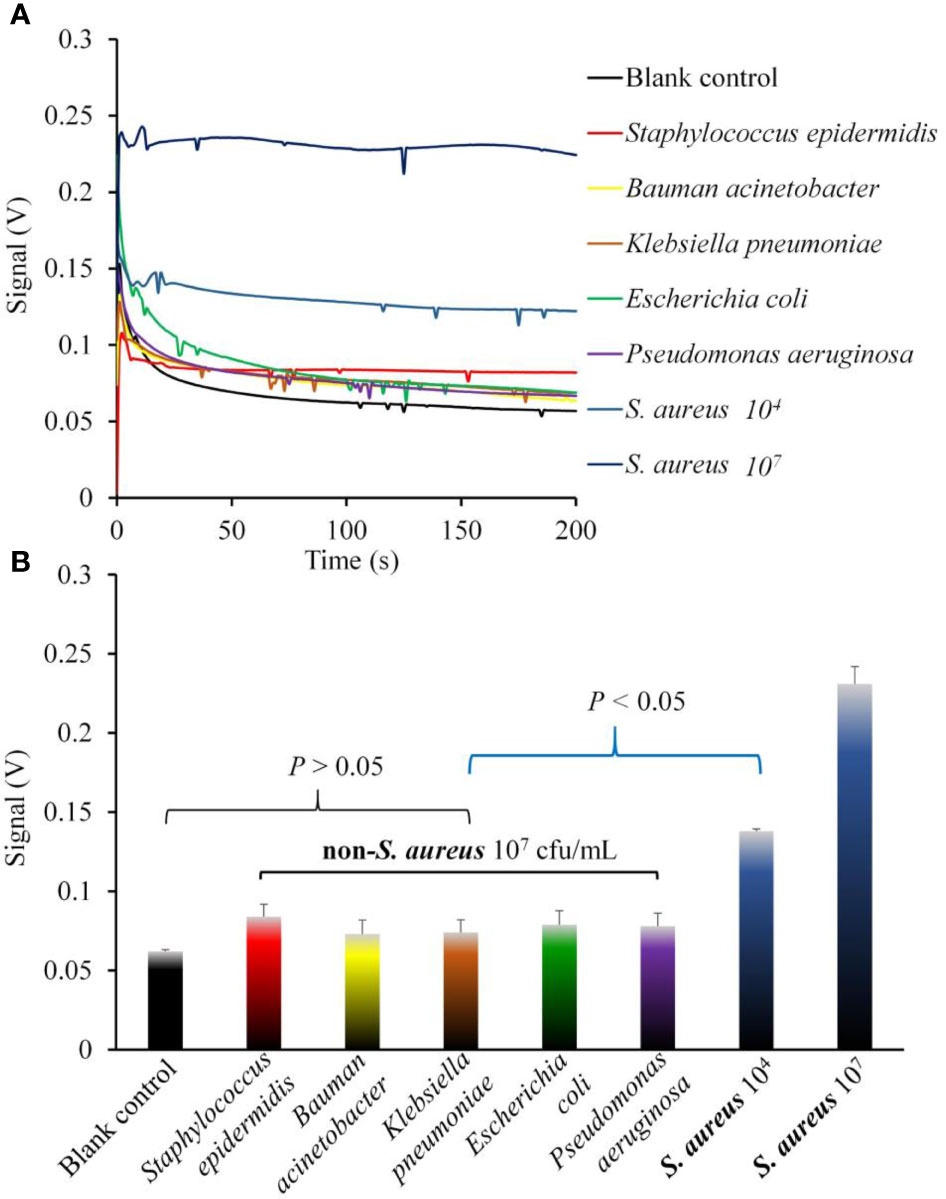
Figure 6 The specificity of the biosensor for the detection of S. aureus compared with non-S. aureus. (A) Signal-time response trace during a complete test cycle (200 s) with the different kinds of pathogenic bacteria. (B) The optimal signal detection values of the fluorescence intensity of S. aureus and five non-S. aureus strains. The statistical analysis suggested that the signal values of all non-S. aureus groups were not significantly different from that of the blank control group (P >0.05), but all of them were significantly lower than that of both the two S. aureus groups (104 and 107 CFU/ml, P <0.05). Error bars in (B) are based on standard deviations (n = 3).
Detection Results of Clinically Isolated S. aureus Strains
The results of the biosensor sensitivities for five clinically isolated S. aureus strains are shown in Figure 7. The results showed that compared with that of the blank control group (PBS), the fluorescence intensity of the five S. aureus groups (1 × 104 CFU/ml) was significantly higher (P <0.05). However, although the fluorescence intensity of the five S. aureus groups (1 × 103 CFU/ml) was higher than that of the blank control group, there were no significant differences between three (3/5, 60%) of the S. aureus strains (SA-2, SA-4, SA-5) and the blank control (P >0.05).
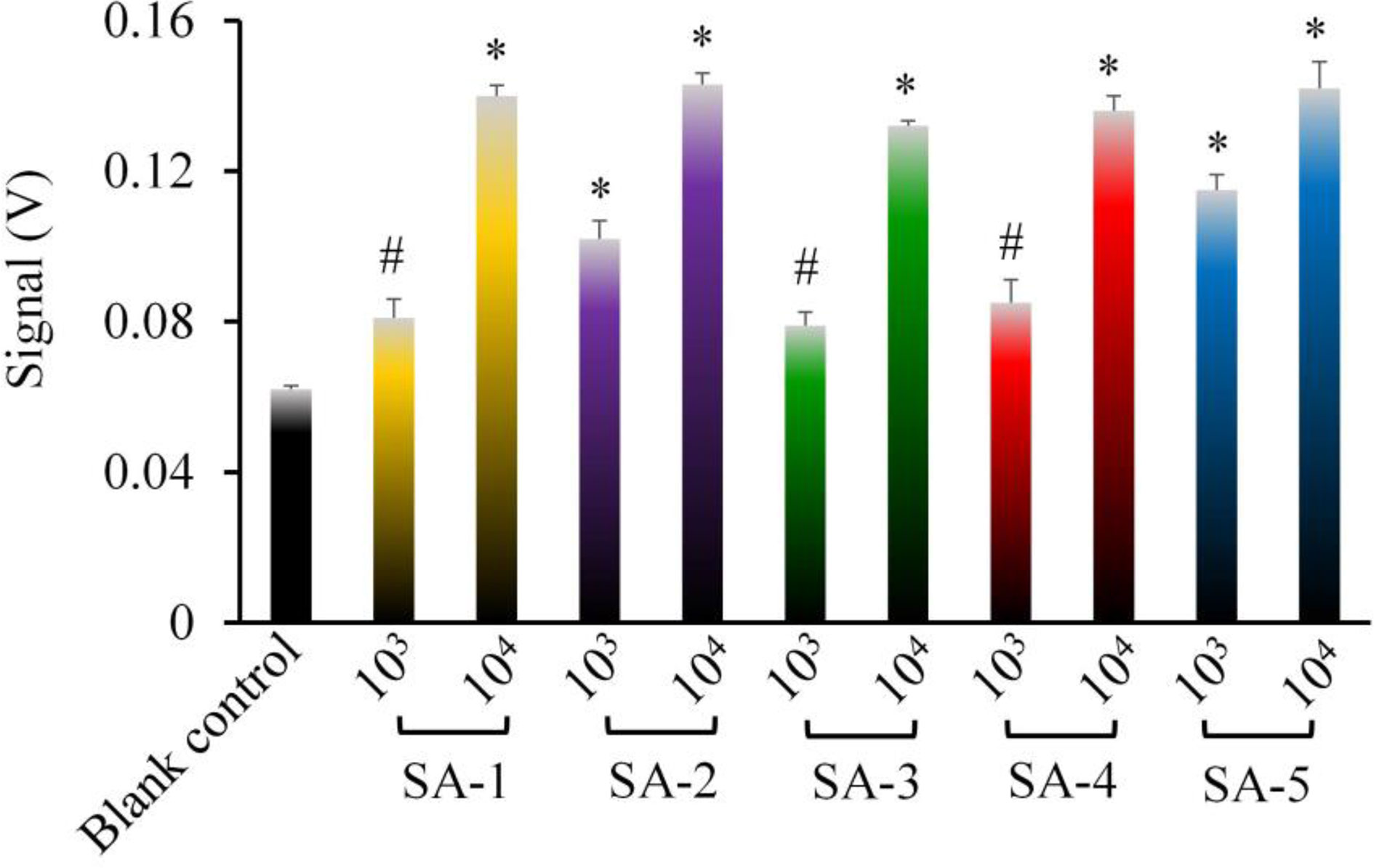
Figure 7 The sensitivity of the biosensor for the detection of the five clinically isolated S. aureus strains. The results showed that the sensitivity could reach 103 CFU/ml in the detection of some clinical S. aureus strains. * represented P <0.05 and # represented P >0.05 comparing with the blank control group (PBS). SA-1 to SA-5 represented five S. aureus strains isolated from different patients. Error bars are based on standard deviations (n = 3).
Discussion
S. aureus is a common gram-positive pathogenic bacterium with strong pathogenicity, which greatly threatens the health and even life of S. aureus-infected patients (Briaud et al., 2020; Gao et al., 2020). Early rapid diagnosis of S. aureus is valuable for the timely use of effective antimicrobial therapy to improve prognosis. Previous studies have reported many methods for the rapid detection of S. aureus, such as PCR, protein markers, and immunofluorescence, but each method has advantages and disadvantages, as mentioned above, and has not been widely used in clinical practice (Wu et al., 2013; Hirvonen, 2014; Gill et al., 2019).
Biosensors are a multidisciplinary technology that integrates biology with other disciplines, such as physics and chemistry. Since the first oxygen biosensor appeared in 1962, biosensor operation has become simpler, smarter, faster and more accurate due to the advancement of biosensor-related technology. Therefore, there are increasing application studies in clinical and food aetiology detection (Rubab et al., 2018; Cui et al., 2020). In this study, we constructed a new set of quantum dots immunofluorescence biosensors that combined the advantages of biosensors with the high specificity of antigen-antibody immune interactions and the high sensitivity and stability of quantum dots fluorescence. Our research suggested that this biosensor has high specificity and sensitivity and takes only 2 h to produce results, so it can achieve rapid and accurate S. aureus detection.
Among all the research results, SEM data showed that the antibody-immobilized optical fiber probe we prepared could specifically capture S. aureus, and more S. aureus were captured as the concentration of S. aureus increased. Additionally, the sensor sensitivity experiment also revealed that the fluorescence intensity detected by the biosensor increased with the increase of the S. aureus concentration, and there was a dose-response relationship. Thus, the concentration of S. aureus could be roughly estimated by the fluorescence intensity. Additionally, compared with the signal of the S. aureus-free control, when the concentration of S. aureus was 1 × 104 CFU/ml or greater, the quantum dots fluorescence intensity increased significantly. However, the fluorescence intensity of the 1 × 103 CFU/ml group was not significantly different from that of the control group. This result indicated that the biosensor detection sensitivity for S. aureus reached 1 × 104 CFU/ml.
However, we also found that the fluorescence intensity of the 1 × 108 CFU/ml group was not greater than that of the 1 × 107 CFU/ml group. This result was consistent with that of the optical fiber probe capture rate experiment. The capture rate experiment showed that the capture rate of the 1 × 108 CFU/ml group was the lowest although the 1 × 108 CFU/ml group had captured the greatest number of S. aureus. The reason we inferred that the quantity of antibody-immobilized to the optical fiber probe was limited, and the number of S. aureus in the 1 × 108 CFU/ml group exceeded the maximum capture capacity of the probe. In addition, excessive S. aureus may increase non-specific adsorption with poor stability, competitively reducing the capture of specifically bound S. aureus with good stability.
S. aureus used in the sensitivity experiments was a S. aureus standard laboratory strain (ATCC 29213 strain). However, when this biosensor was applied to the detection of five clinically isolated S. aureus strains, we found that the fluorescence intensities of two strains (1 × 103 CFU/ml) were significantly higher than that of the blank control (P <0.05), while those of the remaining three strains (1 × 103 CFU/ml) were not significantly higher than that of the control (P >0.05). The difference in sensitivity of this biosensor between the S. aureus standard strain and the clinically isolated S. aureus strains may be one of the shortcomings of this biosensor. The specific reason might be related to the complexity of the clinically isolated S. aureus strains, such as bacterial activity, gene polymorphism, sources of specimen, and clinical medications. Therefore, we concluded that the detection limit of S. aureus reached 1 × 104 CFU/ml or even 1 × 103 CFU/ml in the detection of some clinical strains, and moreover, the fluorescence intensity had a significant positive linear correlation relationship with the logarithm of the S. aureus concentration in the range of 103–107 CFU/ml (correlation coefficient R2 = 0.9731, P = 0.011).
The biosensor specificity experiment showed that the fluorescence intensities of the five most common pathogenic bacteria other than S. aureus, such as E. coli and K. pneumoniae, in nosocomial infections were not significantly different from that of the blank control. However, it was satisfactory that they were all significantly less than those of the two S. aureus concentration groups (1 × 104 CFU/ml and 1 × 107 CFU/ml) (P <0.05). Therefore, this biosensor could effectively distinguish S. aureus (1 × 104 CFU/ml and above) from other common pathogenic bacteria (non-S. aureus) in nosocomial infections. The specificity of the biosensor was high enough for S. aureus detection.
We believe that the high sensitivity and high specificity of the new biosensor in the detection of S. aureus could attribute to the principle used to identify S. aureus in our study. This experimental principle was taken into account the dual needs of high sensitivity and high specificity at the same time. For the specificity, we adopt a double-antibody sandwich structure to capture the S. aureus and the high specificity could be guaranteed based on the principle of antigen-antibody specific binding. While the various ultra-sensitive biosensors (Li et al., 2018; Andersson et al., 2020; Chen et al., 2020) based on the molecular detection technology of S. aureus-related genes may lead to excessively high false positive rate. In particular, even a small amount of contamination in clinical samples can lead to false positives. In addition, for high sensitivity, on the basis of ensuring high specificity, we also adopted two experimental principles to improve the sensitivity of the biosensor. First, we had selected two different kind of antibodies. Among them, the rabbit polyclonal anti-S. aureus antibody (ab20920) immobilized on the probe surface was used to capture as many S. aureus as possible in order to improve the sensitivity of the biosensor. The other antibody was the mouse monoclonal anti-S. aureus antibody (ab37644) and it could ensure the specificity. Second. We used quantum dots (QDs) with obvious optical advantages as the fluorescence signal and combined the signal cascade amplification principle of the biotin–avidin system to promote the sensitivity of the biosensor, instead of other common fluorescence materials (Bhardwaj et al., 2017; Loi and Antelmann, 2020) or just using quantum dots without biotin-avidin system (Shi et al., 2015). Therefore, through the above-mentioned experimental principles, it is ensured that the biosensor has both high sensitivity and high specificity.
In addition, as mentioned in the method about sensitivity experiment, it was speculated that the two S. aureus strains (SA-4 and SA-5) might have produced the biofilm in the infection in body, resulting in ineffective antimicrobial treatment, while many previous literatures about the drug resistance related to the S. aureus biofilm had been reported (Bui et al., 2017; Grassi et al., 2017; Kavanagh et al., 2018). Bacterial biofilm (BF) is a membrane-like viscous secretion formed by bacteria (Seethalakshmi et al., 2020). Generally, biofilm is produced under microenvironmental conditions that are not suitable for bacterial growth, such as nutritional deficiency, antibacterial drugs, and immune attacks, and the biofilm can protect bacteria from the attack. In the body, the antibacterial drugs cannot reach the minimum inhibitory concentration in the bacterial biofilm, which reduces the bactericidal activity and therapeutic effect of sensitive antibacterial drugs. But there are no good drugs or solvents that can be used to dissolve and liquefy the biofilm in the body to improve the therapeutic effect of antimicrobial drugs. However, in vitro, before the bacterial culture and other detection operations of specimens with viscous clinical secretions (such as sputum specimens and skin pus), various digestive juices (such as Sputasol and trypsin digestion solution) can and need to be used to liquefy the specimen, so that the bacterial biofilm and other secretions in the specimen can be digested and decomposed to form a uniform bacterial suspension. Then, the bacterial suspension can be correctly sampled for bacterial culture or other detection operations. While the insufficient liquefaction of the specimens might result in false negative results of bacterial culture due to uneven distribution of pathogenic bacteria in the specimen and incorrect sampling for bacterial culture. Just as the same as the bacterial culture, the clinical biological specimens also need to be fully liquefied to digest and dissolve viscous secretions such as biofilms before the detection procedure of the biosensor in this study. For this reason, the biosensor in this study might not be affected by the interference of biofilms or other secretions and showed good detection results in sensitivity experiment and the clinical application on the detection of S. aureus.
However, although the biosensor we constructed have significant advantages, there were also three limitations to be improved. First, our biosensor cannot yet distinguish between methicillin-resistant S. aureus (MRSA) and methicillin-sensitive S. aureus (MSSA) because antigen-antibody reaction is not specific enough to distinguish between the two, while it is valuable to distinguish MRSA from MSSA because of the need to choose different antibiotics for antibacterial treatment (Guo et al., 2020). However, we believe that this limitation of the biosensor will be resolved in the future with the advancement of related specific antigen–antibody interaction knowledge. Second, we have also applied this biosensor in the detection of more clinical real samples from clinical patients. But in this process, we discovered an important problem as follow. The gold standard for detection of S. aureus in real samples is bacterial culture, and in these real samples with positive culture of S. aureus, the detection rate of this biosensor was almost 100%. However, the positive rate of bacterial culture is relatively low in clinical real samples, and for some bacterial culture-negative samples (maybe false negative), the detection results of the biosensor were still positive. For this reason, these positive results could not accurately determine whether they are the real S. aureus strain infection or just the false positive results. In these cases, the sensitivity and specificity of the biosensor in real samples cannot be accurately judged. However, with the increase in our ongoing application research of clinical real samples, the value of the sensitivity of the biosensor to detect S. aureus can get to a much more accurate result, which may not be the current 100%. Similarly, the experimental result of specificity will be more accurate.
In conclusion, we successfully constructed a set of quantum dots immunofluorescence biosensors for S. aureus detection. The biosensor possessed high specificity and high sensitivity for the S. aureus detection. The concentration of S. aureus can reach 1 × 104 CFU/mL or even 1 × 103 CFU/mL and still be detected, and the detection takes only 2 h. Therefore, the sensor can meet the need for rapid and accurate S. aureus detection for clinical application.
Data Availability Statement
The raw data supporting the conclusions of this article will be made available by the authors, without undue reservation.
Author Contributions
JC and MZ helped to perform the experiment and drafted the manuscript. YingL and ZL completed most of the experiment, especially the detection of strains by the sensor and the imaging of the scanning electron microscope. YuanL and YangL helped the statistical analysis of experimental data and revised the manuscript. YanqL and LY participated in the preparation of the optical fiber probe of the sensor in the experiment. LC and CY designed and provided the fund to support this experiment. All authors contributed to the article and approved the submitted version.
Funding
This study was supported by the Chinese Military Medical Innovation Project (Grant number 16CXZ041). On behalf of my co-authors, we declare that there was no conflict of interest exited in this study.
Conflict of Interest
The authors declare that the research was conducted in the absence of any commercial or financial relationships that could be construed as a potential conflict of interest.
Supplementary Material
The Supplementary Material for this article can be found online at: https://www.frontiersin.org/articles/10.3389/fcimb.2021.665241/full#supplementary-material
References
Abdelhamid, H. N., Wu, H. F. (2013). Probing the Interactions of Chitosan Capped CdS Quantum Dots With Pathogenic Bacteria and Their Biosensing Application. J. Mater. Chem. B. 1, 6094–6106. doi: 10.1039/c3tb21020k
Abdelhamid, H. N., Wu, H. F. (2017). Selective Biosensing of Staphylococcus Aureus Using Chitosan Quantum Dots. Spectrochim. Acta A. Mol. Biomol. Spectrosc. 5, 50–56. doi: 10.1016/j.saa.2017.06.047
Anderson, G. P., Golden, J. P., Ligler, F. S. (1994). An Evanescent Wave Biosensor–Part I: Fluorescent Signal Acquisition From Step-Etched Fiber Optic Probes. IEEE Trans. BioMed. Eng. 41, 578–584. doi: 10.1109/10.293245
Andersson, T., Bläckberg, A., Lood, R., Ertürk Bergdahl, G. (2020). Development of a Molecular Imprinting-Based Surface Plasmon Resonance Biosensor for Rapid and Sensitive Detection of Staphylococcus Aureus Alpha Hemolysin From Human Serum. Front. Cell Infect. Microbiol. 10, 571578. doi: 10.3389/fcimb.2020.571578
Bhardwaj, N., Bhardwaj, S. K., Mehta, J., Kim, K., Deep, A. (2017). Mof-Bacteriophage Biosensor for Highly Sensitive and Specific Detection of Staphylococcus Aureus. ACS Appl. Mater. Interfaces 9, 33589–33598. doi: 10.1021/acsami.7b07818
Briaud, P., Bastien, S., Camus, L., Boyadjian, M., Reix, P., Mainguy, C., et al. (2020). Impact of Coexistence Phenotype Between Staphylococcus Aureus and Pseudomonas Aeruginosa Isolates on Clinical Outcomes Among Cystic Fibrosis Patients. Front. Cell Infect. Microbiol. 10, 266. doi: 10.3389/fcimb.2020.00266
Bui, L. M. G., Conlon, B. P., Kidd, S. P. (2017). Antibiotic Tolerance and the Alternative Lifestyles of Staphylococcus Aureus. Essays Biochem. 61, 71–79. doi: 10.1042/EBC20160061
Chaudhari, P. P., Chau, L. K., Tseng, Y. T., Huang, C. J., Chen, Y. L. (2020). A Fiber Optic Nanoplasmonic Biosensor for the Sensitive Detection of Ampicillin and its Analogs. Mikrochim. Acta 187, 396. doi: 10.1007/s00604-020-04381-w
Chen, X., Ma, K., Yi, X., Xiong, L., Wang, Y., Li, S. (2020). The Rapid and Visual Detection of Methicillin-Susceptible and Methicillin-Resistant Staphylococcus Aureus Using Multiplex Loop-Mediated Isothermal Amplification Linked to a Nanoparticle-Based Lateral Flow Biosensor. Antimicrob. Resist. Infect. Control 9, 111. doi: 10.1186/s13756-020-00774-x
Clancy, B., Cauller, L. J. (1998). Reduction of Background Autofluorescence in Brain Sections Following Immersion in Sodium Borohydride. J. Neurosci. Methods 83, 97–102. doi: 10.1016/s0165-0270(98)00066-1
Cui, F., Zhou, Z., Zhou, H. S. (2020). Molecularly Imprinted Polymers and Surface Imprinted Polymers Based Electrochemical Biosensor for Infectious Diseases. Sensors (Basel) 20, 996. doi: 10.3390/s20040996
Gao, S., Liu, G., Li, J., Chen, J., Li, L., Li, Z., et al. (2020). Antimicrobial Activity of Lemongrass Essential Oil (Cymbopogon Flexuosus) and Its Active Component Citral Against Dual-Species Biofilms of Staphylococcus Aureus and Candida Species. Front. Cell Infect. Microbiol. 10, 603858. doi: 10.3389/fcimb.2020.603858
Gill, A., Singh, S., Thapliyal, N., Karpoormath, R. (2019). Nanomaterial-Based Optical and Electrochemical Techniques for Detection of Methicillin-Resistant Staphylococcus Aureus: A Review. Mikrochim. Acta 186, 114. doi: 10.1007/s00604-018-3186-7
Grassi, L., Maisetta, G., Esin, S., Batoni, G. (2017). Combination Strategies to Enhance the Efficacy of Antimicrobial Peptides Against Bacterial Biofilms. Front. Microbiol. 8:2409. doi: 10.3389/fmicb.2017.02409
Gries, C. M., Rivas, Z., Chen, J., Lo, D. D. (2020). Intravital Multiphoton Examination of Implant-Associated Staphylococcus Aureus Biofilm Infection. Front. Cell Infect. Microbiol. 10:574092. doi: 10.3389/fcimb.2020.574092
Guo, Y., Song, G., Sun, M., Wang, J., Wang, Y. (2020). Prevalence and Therapies of Antibiotic-Resistance in Staphylococcus Aureus. Front. Cell Infect. Microbiol. 10, 107. doi: 10.3389/fcimb.2020.00107
Hao, L., Xue, L., Huang, F., Cai, G., Qi, W., Zhang, M., et al. (2020). A Microfluidic Biosensor Based on Magnetic Nanoparticle Separation, Quantum Dots Labeling and MnO2 Nanoflower Amplification for Rapid and Sensitive Detection of Salmonella Typhimurium. Micromachines (Basel) 11, 281. doi: 10.3390/mi11030281
Hirvonen, J. J. (2014). The Use of Molecular Methods for the Detection and Identification of Methicillin-Resistant Staphylococcus Aureus. Biomark Med. 8, 1115–1125. doi: 10.2217/bmm.14.60
Hu, F., Zhu, D., Wang, F., Wang, M. (2018). Current Status and Trends of Antibacterial Resistance in China. Clin. Infect. Dis. 67, S128–S134. doi: 10.1093/cid/ciy657
Karer, M., Kussmann, M., Ratzinger, F., Obermueller, M., Reischer, V., Winkler, H., et al. (2020). Different Types of Coagulase are Associated With 28-Day Mortality in Patients With Staphylococcus Aureus Bloodstream Infections. Front. Cell Infect. Microbiol. 10, 236. doi: 10.3389/fcimb.2020.00236
Kavanagh, N., Ryan, E. J., Widaa, A., Sexton, G., Fennell, J., O’Rourke, S., et al. (2018). Staphylococcal Osteomyelitis: Disease Progression, Treatment Challenges, and Future Directions. Clin. Microbiol. Rev. 31, e00084–e00017. doi: 10.1128/CMR.00084-17
Liu, P., Na, N., Liu, T., Huang, L., He, D., Hua, W., et al. (2011). Ultrasensitive Detection of Ferritin in Human Serum by Western Blotting Based on Quantum Dots-Labeled Avidin-Biotin System. Proteomics 11, 3510–3517. doi: 10.1002/pmic.201000742
Liu, T., Yong, Z., Zhang, Z., Zhang, P., Li, J., Yang, R., et al. (2014). A Fiber Optic Biosensor for Specific Identification of Dead Escherichia Coli O157:H7. Sensors Actuators B. Chem. 196, 161–167. doi: 10.1016/j.snb.2014.02.003
Li, Q., Zhou, D., Pan, J., Liu, Z., Chen, J. (2018). Ultrasensitive and Simple Fluorescence Biosensor for Detection of the Meca Gene of Staphylococcus Aureus by Using an Exonuclease III-Assisted Cascade Signal Amplification Strategy. Analyst 143, 5670–5675. doi: 10.1039/c8an01805g
Loi, V. V., Antelmann, H. (2020). Method for Measurement of Bacillithiol Redox Potential Changes Using the Brx-roGFP2 Redox Biosensor in Staphylococcus Aureus. MethodsX 7, 100900. doi: 10.1016/j.mex.2020.100900
Ma, Z., Jiang, M., Zhu, Q., Luo, Y., Chen, D. (2020). A Porous Hollow Fiber Sensor for Detection of Cellular Hydrogen Peroxide Release Based on Cell-in-Lumen Configuration. Sensors Actuators B. Chem. 321, 128516. doi: 10.1016/j.snb.2020.128516
McIver, C. J., De Silva, R., Er, N., Pratama, R., Mukerjee, C., Stevens, R., et al. (2020). A Simple and Expedient PCR Format for Rapid Molecular Screening of Methicillin-Resistant Staphylococcus Aureus in Amies Gel Swabs. Pathology 52, 466–472. doi: 10.1016/j.pathol.2020.02.005
Nishiyama, K., Takeda, Y., Maeki, M., Ishida, A., Tani, H., Shigemura, K., et al. (2020). Rapid Detection of Anti-H5 Avian Influenza Virus Antibody by Fluorescence Polarization Immunoassay Using a Portable Fluorescence Polarization Analyzer. Sensors Actuators B. Chem. 316, 128160. doi: 10.1016/j.snb.2020.128160
Pu, K. Y., Liu, B. (2009). Optimizing the Cationic Conjugated Polymer-Sensitized Fluorescent Signal of Dye Labeled Oligonucleotide for Biosensor Applications. Biosens. Bioelectron. 24, 1067–1073. doi: 10.1016/j.bios.2008.07.029
Renevey, N., Thur, B. (2018). Outbreak Investigation: How to Prevent Laboratory Contamination During High-Throughput Testing. Rev. Off. Int. Epizoot. 37, 843–856. doi: 10.20506/rst.37.3.2891
Rubab, M., Shahbaz, H. M., Olaimat, A. N., Oh, D. H. (2018). Biosensors for Rapid and Sensitive Detection of Staphylococcus Aureus in Food. Biosens Bioelectron 105, 49–57. doi: 10.1016/j.bios.2018.01.023
Seethalakshmi, P. S., Rajeev, R., Kiran, G. S., Selvin, J. (2020). Promising Treatment Strategies to Combat Staphylococcus Aureus Biofilm Infections: An Updated Review. Biofouling 36, 1159–1181. doi: 10.1080/08927014.2020.1857743
Shi, J., Chan, C., Pang, Y., Ye, W., Tian, F., Lyu, J., et al. (2015). A Fluorescence Resonance Energy Transfer (FRET) Biosensor Based on Graphene Quantum Dots (Gqds) and Gold Nanoparticles (AuNPs) for the Detection of Meca Gene Sequence of Staphylococcus Aureus. Biosens Bioelectron 67, 595–600. doi: 10.1016/j.bios.2014.09.059
Song, D., Qu, X., Liu, Y., Li, L., Yin, D., Li, J., et al. (2017). A Rapid Detection Method of Brucella With Quantum Dots and Magnetic Beads Conjugated With Different Polyclonal Antibodies. Nanoscale Res. Lett. 12, 179. doi: 10.1186/s11671-017-1941-z
Stürenburg, E. (2009). Rapid Detection of Methicillin-Resistant Staphylococcus Aureus Directly From Clinical Samples: Methods, Effectiveness and Cost Considerations. Ger Med. Sci. 7, Doc06. doi: 10.3205/000065
Tenover, F. C., Tickler, I. A., Le, V. M., Dewell, S., Mendes, R. E., Goering, R. V. (2019). Updating Molecular Diagnostics for Detecting Methicillin-Susceptible and Methicillin-Resistant Staphylococcus Aureus Isolates in Blood Culture Bottles. J. Clin. Microbiol. 57, e01195-19. doi: 10.1128/JCM.01195-19
Wolfbeis, O. S. (2000). Fiber-Optic Chemical Sensors and Biosensors. Anal. Chem. 72, 81R–89R. doi: 10.1021/a1000013k
Wu, H. F., Gopal, J., Abdelhamid, H. N., Hasan, N. (2012). Quantum Dot Applications Endowing Novelty to Analytical Proteomics. Proteomics 12, 2949–2961. doi: 10.1002/pmic.201200295
Wu, Q., Li, Y., Wang, M., Wu, Z. G., Huang, B. H. (2013). Rapid Identification of Staphylococcus Aureus Directly From Positive Blood Culture Media Using Quantum Dots as Fluorescence Probes. APMIS 121, 348–352. doi: 10.1111/apm.12005
Yu, S., Ding, L., Lin, H., Wu, W., Huang, J. (2019). A Novel Optical Fiber Glucose Biosensor Based on Carbon Quantum Dots-Glucose Oxidase/Cellulose Acetate Complex Sensitive Film. Biosens Bioelectron 146:111760. doi: 10.1016/j.bios.2019.111760
Zhang, Z., Hua, F., Liu, T., Zhao, Y., Li, J., Yang, R., et al. (2014). A Double-Taper Optical Fiber-Based Radiation Wave Other Than Evanescent Wave in All-Fiber Immunofluorescence Biosensor for Quantitative Detection of Escherichia Coli O157:H7. PloS One 9, e95429. doi: 10.1371/journal.pone.0095429
Zhou, Y., Wang, H., Song, D., Li, Z., Han, S., Long, F., et al. (2021). Simple, Rapid, and Sensitive on-Site Detection of Hg 2+ in Water Samples Through Combining Portable Evanescent Wave Optofluidic Biosensor and Fluorescence Resonance Energy Transfer Principle. Anal. Chim. Acta 1155, 338351. doi: 10.1016/j.aca.2021.338351
Keywords: Staphylococcus aureus, biosensor, quantum dots, sensitivity, specificity
Citation: Cui J, Zhou M, Li Y, Liang Z, Li Y, Yu L, Liu Y, Liang Y, Chen L and Yang C (2021) A New Optical Fiber Probe-Based Quantum Dots Immunofluorescence Biosensors in the Detection of Staphylococcus aureus. Front. Cell. Infect. Microbiol. 11:665241. doi: 10.3389/fcimb.2021.665241
Received: 07 February 2021; Accepted: 10 May 2021;
Published: 31 May 2021.
Edited by:
Mingkai Li, Fourth Military Medical University, ChinaReviewed by:
Hani Nasser Abdelhamid, Assiut University, EgyptFengjun Sun, Third Military Medical University, China
Copyright © 2021 Cui, Zhou, Li, Liang, Li, Yu, Liu, Liang, Chen and Yang. This is an open-access article distributed under the terms of the Creative Commons Attribution License (CC BY). The use, distribution or reproduction in other forums is permitted, provided the original author(s) and the copyright owner(s) are credited and that the original publication in this journal is cited, in accordance with accepted academic practice. No use, distribution or reproduction is permitted which does not comply with these terms.
*Correspondence: Liangan Chen, Y2hlbmxpYW5nYW4zMDFAMTYzLmNvbQ==; Changxi Yang, Y3h5YW5nQHRzaW5naHVhLmVkdS5jbg==
†These authors have contributed equally to this work