COVID-19: Lung-Centric Immunothrombosis
- 1College of Medicine, Alfaisal University, Riyadh, Saudi Arabia
- 2Department of Medicine, King Faisal Specialist Hospital and Research Centre (KFSHRC), Riyadh, Saudi Arabia
The respiratory tract is the major site of infection by SARS-CoV-2, the virus causing COVID-19. The pulmonary infection can lead to acute respiratory distress syndrome (ARDS) and ultimately, death. An excessive innate immune response plays a major role in the development of ARDS in COVID-19 patients. In this scenario, activation of lung epithelia and resident macrophages by the virus results in local cytokine production and recruitment of neutrophils. Activated neutrophils extrude a web of DNA-based cytoplasmic material containing antimicrobials referred to as neutrophil extracellular traps (NETs). While NETs are a defensive strategy against invading microbes, they can also serve as a nidus for accumulation of activated platelets and coagulation factors, forming thrombi. This immunothrombosis can result in occlusion of blood vessels leading to ischemic damage. Herein we address evidence in favor of a lung-centric immunothrombosis and suggest a lung-centric therapeutic approach to the ARDS of COVID-19.
Introduction
SARS-CoV-2, the coronavirus responsible for COVID-19, initially infects the nasal, bronchial and alveolar epithelial cells (Hoffmann et al., 2020; Milewska et al., 2020; Zhu et al., 2020). Resident immune cells such as macrophages and dendritic cells are also infected, albeit to a lesser extent (Carsana et al., 2020; Schaefer et al., 2020; Yang et al., 2020). The SARS-CoV-2 pulmonary tropism is manifested in the clinical features of COVID-19 (e.g., cough, dyspnea). The clinical course of SARS-CoV-2 infection is subdivided into three outcomes. Most patients will be asymptomatic or display minor respiratory symptoms and recover without hospitalization. Roughly 10-20% of affected individuals will advance to pneumonia/hypoxia and require hospitalization, but will eventually recover (Chowdhury et al., 2021; Morris et al., 2021). Only a small fraction (5 - 10%) of COVID-19 patients will progress to acute respiratory distress syndrome (ARDS) and require aggressive treatment in intensive care units (e.g., mechanical ventilation) (Chowdhury et al., 2021; Morris et al., 2021). Some of these patients will eventually succumb to the disease with the major cause of death being respiratory failure (Ackermann et al., 2020; Phua et al., 2020; Tay et al., 2020). Autopsy findings indicate that the lungs bear the greatest pathologic burden, characterized by diffuse alveolar damage, inflammatory cell infiltrates and thrombosis (Ackermann et al., 2020; Lax et al., 2020; Liu et al., 2020; Deshmukh et al., 2021). Of note, organs remote from the initial site of infection such as the heart and kidneys are not spared. Histopathologic features in autopsy specimens of these remote organs include the presence of microvascular thrombi adjacent to regions of necrosis (Ackermann et al., 2020; Rapkiewicz et al., 2020; Tang et al., 2020). Thus, in severe COVID-19 there is evidence indicative of an hypercoagulative state and multiorgan dysfunction.
A dysregulated immune response to SARS-CoV-2 infection is believed to play a major role in the pathogenesis of COVID-19. Specifically, there is an impaired antiviral response in conjunction with an excessive inflammatory response (Blanco-Melo et al., 2020; Hadjadj et al., 2020). Lymphopenia is common and may be coupled to markers of T cell exhaustion in the circulation and decreased numbers in lymphoid tissues (Diao et al., 2020; Giamarellos-Bourboulis et al., 2020; Lee et al., 2020; Liu et al., 2020; Lucas et al., 2020; Zhao et al., 2020; Zheng et al., 2020; Ronit et al., 2021). An inadequate lymphocyte-mediated antiviral response would protract the viral infection and thereby exacerbate the inflammatory response (Lee et al., 2020; Lucas et al., 2020; Manjili et al., 2020; Ronit et al., 2021).
Lung-Centric Inflammation
Analyses of bronchoalveolar lavage fluid (BALF) of COVID-19 patients indicate a pro-inflammatory environment within the lungs (De Biasi et al., 2020; Liao et al., 2020; Pandolfi et al., 2020; Wang et al., 2020a; Ronit et al., 2021). Their BALF is enriched in pro-inflammatory chemokines and cytokines as well as activated macrophages and neutrophils. Chemokines (e.g., IL-8) dominate the BALF profile in moderate cases of COVID-19, whereas cytokines (e.g., TNF-α, IL-6) are prevalent in more severe cases (Liao et al., 2020).
A major cytokine involved in initiation and progression of the inflammatory response is IL-1β, the activation of which is dependent on the NLRP3 inflammasome (Kelley et al., 2019; Swanson et al., 2019; Zhao and Zhao, 2020). In brief, detection of viral material (e.g., PAMPs) by alveolar macrophages activates the NFκB transcription pathway, resulting in the generation of nascent pro-IL-1β as well as components of the NLRP3 inflammasome (Figure 1). Subsequently, the inflammasome is assembled and serves as a platform for caspase-mediated cleavage of pro-IL-1β to the mature form. IL-1β lacks a signal sequence and is retained in the cytoplasm until an exit portal is created in the plasma membrane. To this end, gasdermins, also activated by the caspases, enter the plasma membrane and oligomerize to form pores (Broz et al., 2020). IL-1β and other pro-inflammatory material (e.g., DAMPs) exit via these gasdermin pores. Collectively, the released mediators amplify the local inflammatory response via feed-forward mechanisms, including cytokine-induced cytokine release and recruitment of additional innate immune cells (e.g., neutrophils).
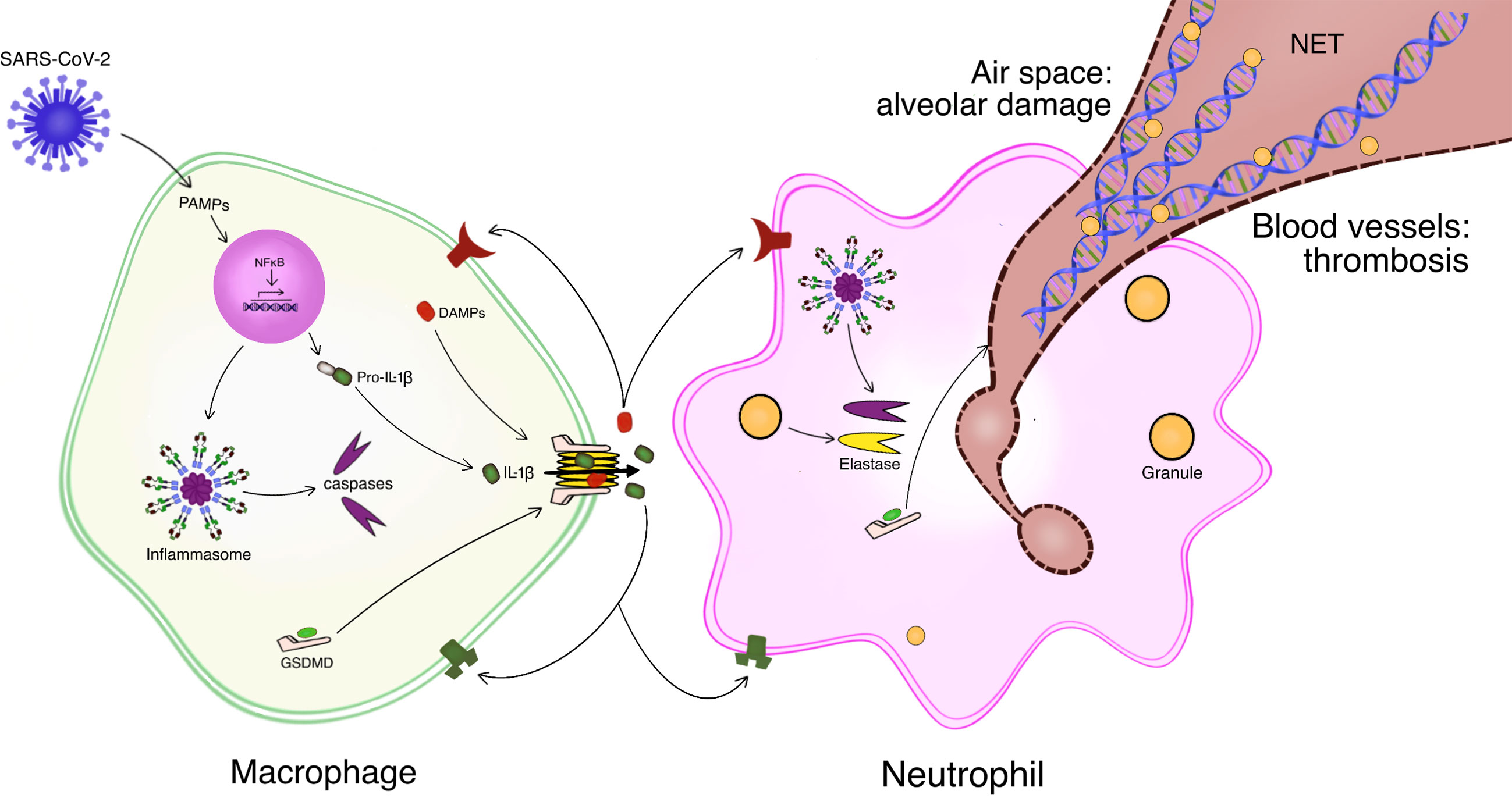
Figure 1 Schematic of lung-centric inflammation and immunothrombosis in response to SARS-CoV-2 infection. Resident alveolar macrophages mount an inflammatory response to infection of the lungs by SARS-CoV-2. Macrophages detect viral material e.g., pathogen-associated molecular patterns (PAMPs). PAMPs activate the NFκB pathway which generates pro-IL-1β and components of the inflammasome. Assembly and functional activation of the inflammasome results in caspase-mediated cleavage of pro-IL-1β to the mature IL-1β. Caspase cleavage of gasdermin D (GSDMD) allows it to enter the plasma membrane and oligomerize, forming pores. IL-1β as well as other inflammatory mediators, such as damage-associated molecular patterns (DAMPs) exit the macrophage through the GSDMD pores. The inflammatory response is amplified by feed-forward mechanisms and recruitment of additional leukocytes, e.g., neutrophils. Activated neutrophils can extrude neutrophil extracellular traps (NETs), a meshwork of decondensed DNA decorated with granule-derived proteases and antimicrobials. Inflammasome-derived caspase as well as granule-derived elastase activate GSDMD to form the pores for NET release. NETs formed in the alveolar space can induce lung injury while NETs generated within blood vessels sequester platelets and coagulation factors to promote thrombogenesis. Modified from (Tall and Westerterp, 2019).
The NFκB pathway and the NLRP3 inflammasome appear to be operative in COVID-19 patients (Lee et al., 2020; Hariharan et al., 2021) and may contribute to lethality (Lara et al., 2020). SARS-CoV-2 infects human monocytes and activates the NLRP3 inflammasome, resulting in gasdermin-mediated pyroptosis (Ferreira et al., 2021). Active caspases and cytokines are present in the circulation of these patients, with higher levels noted in severe cases (Rodrigues et al., 2021). Further, inflammasome components are detected in autopsy specimens of lung tissue, primarily in macrophages and to a lesser extent in alveolar epithelia (Rodrigues et al., 2021; Toldo et al., 2021).
Lung-Centric “Cytokine Storm”
The cytokines and chemokines generated within the lungs in response to infection can spill into the general circulation, a convenient sampling reservoir for both clinical and experimental purposes. Based on elevated circulating levels of cytokines, the term “cytokine storm” has been used as a descriptor of the hyperinflammatory response involved in the pathogenesis of COVID-19 (Barnes et al., 2020; Castelli et al., 2020; Fajgenbaum and June, 2020; Mehta et al., 2020; Merad and Martin, 2020; Tay et al., 2020). This descriptor has been challenged on the grounds that the measured blood levels of inflammatory cytokines in COVID-19 patients are orders of magnitude less than levels reported in other cases of ARDS such as sepsis or influenza (Kox et al., 2020; Mudd et al., 2020; Sinha et al., 2020). The issue is further confounded by a lack of a precise definition for the term “cytokine storm” (Fajgenbaum and June, 2020). A reasonable approach to avoid this rather semantic issue is to consider the local pulmonary storm as being more severe than the systemic storm (Mudd et al., 2020; Wang et al., 2020a). Based on this premise, data mining of the literature for ARDS studies in which measures of cytokine levels in BALF and blood are provided in the same patients yielded the results presented in Tables 1 and 2.
Table 1 presents data from ARDS of pulmonary origin, while Table 2 presents data from extrapulmonary causes. Of note, none of the studies specifically addressed a potential lung-to-systemic cytokine gradient. Further, the sample sizes are rather small, particularly for the two COVID-19 cases in Table 1. Finally, no attempt is made to establish statistical differences. Despite these limitations, there is a notable trend for higher levels of inflammatory cytokines in BALF than plasma of ARDS patients in which the inciting event was of pulmonary origin (Table 1). This lung-to-systemic gradient is not as evident in ARDS of non-pulmonary origin (Table 2). Analogous results are obtained in experimental models of lung inflammation and injury. When the inciting factor is a direct insult to the lungs, the BALF levels of cytokines exceed their blood levels (Table 3). Of note, dismantling of NETs within the airspace (intratracheal DNase), reduces LPS-induced alveolar damage. This maneuver decreases the cytokine levels in both compartments, while maintaining a BALF-to-lung cytokine gradient (Table 3). By contrast, when lung inflammation/injury is a result of an indirect insult (e.g., peritonitis), a BALF-to-blood cytokine gradient is not evident (Table 4). Collectively, a BALF-to-blood cytokine gradient is noted in ARDS and animal models when the inciting event is of lung origin. Thus, despite the limited data from COVID-19 cases, it seems likely that a lung-centric cytokine storm may characterize the ARDS of COVID-19.
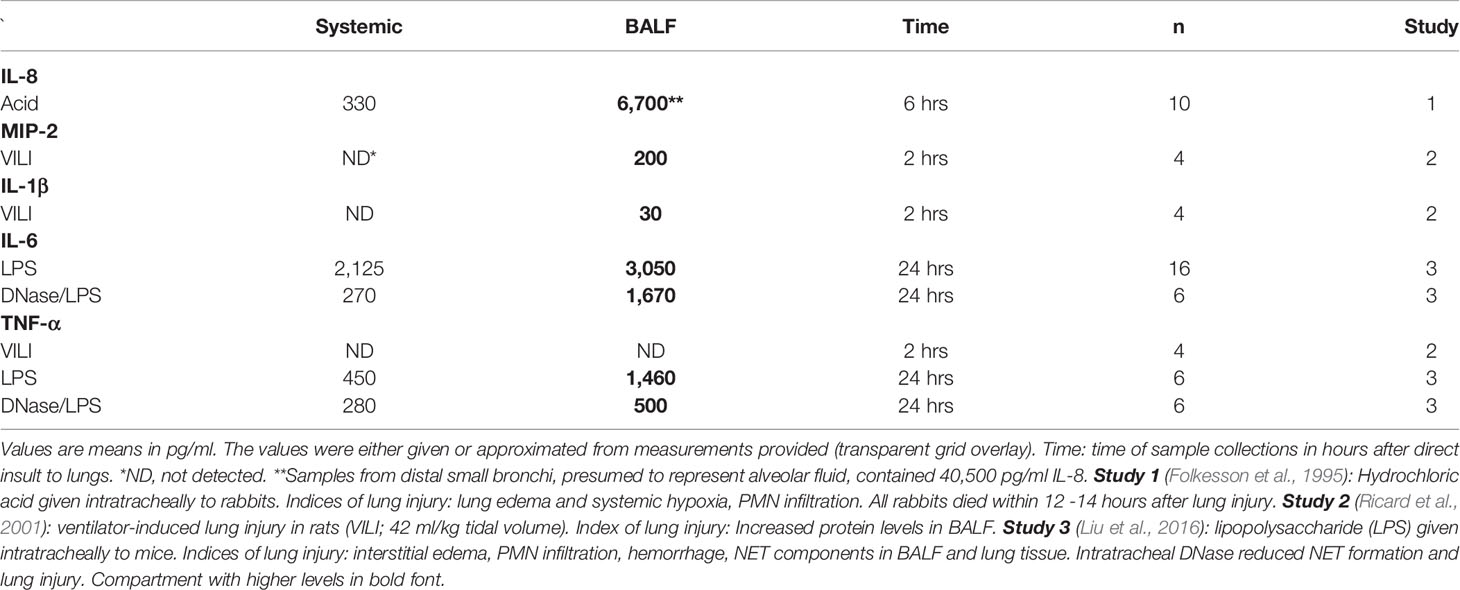
Table 3 Systemic and bronchoalveolar lavage fluid (BALF) cytokines in animal models of direct lung injury.
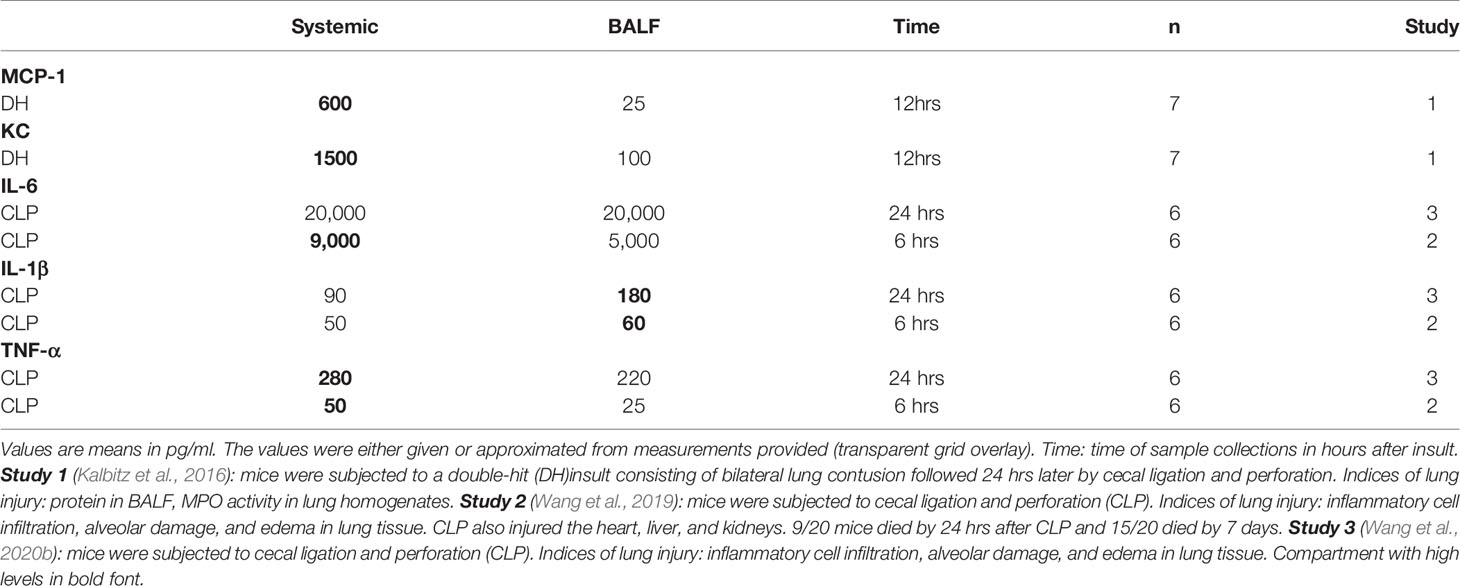
Table 4 Systemic and bronchoalveolar lavage fluid (BALF) cytokines in animal models of indirect lung injury.
Inherent in experimental models of lung inflammation/injury is a defined time interval from insult to assessment of endpoints. Chemokines (e.g., IL-8, MIP-2) are detected as early as 2 – 6 hours after direct injury to the lungs, exceeding plasma levels by at least 20-fold (Table 3). Further, the acid-induced lung injury (pulmonary edema and impaired oxygenation) is associated with the presence of neutrophils in the BALF (Folkesson et al., 1995). Both neutrophil recruitment and lung injury are prevented by therapeutic (post-acid) blockade of IL-8. These observations are consistent with the following scenario. Acid stimulates lung epithelium and/or macrophages to generate IL-8, which attracts neutrophils to the lungs, where they are activated and cause injury (Folkesson et al., 1995).
There are a few issues of relevance to COVID-19 that warrant attention. When BALF and plasma samples are obtained early after admission to ICU, a lung-to-blood gradient for the chemokines, IL-8 is detected (Table 1); correspondingly, the BALF also contains activated monocytes and neutrophils (Ronit et al., 2021). In the same samples, no such gradient was detected for the pro-inflammatory cytokines, TNF-α or IL-6 (Ronit et al., 2021). However, in a longitudinal study of one patient with protracted COVID-19 (over 3 weeks), a 4-fold BALF to blood gradient for IL-6 was attained just days before death (Table 1). Of note, in pleural effusion samples obtained concurrently, the level of IL-6 was 20-fold greater than in plasma (Wang et al., 2020a). Further, assuming a progression in disease severity over time, additional insight is gained by comparisons of moderate to severe cases of COVID-19. Chemokines (e.g., IL-8) dominate the BALF profile in moderate cases, whereas pro-inflammatory cytokines (e.g., TNF-α, IL-6) are prevalent in more severe cases (Liao et al., 2020). The BALF of moderate COVID-19 patients is enriched with macrophages, while BALF of severe cases is enriched with neutrophils, with the neutrophil count being directly related to the levels of IL-8 (Pandolfi et al., 2020). Thus, the SARS-CoV-2 infection of the lungs appears to follow the expected trajectory of an inflammatory response (Folkesson et al., 1995).
Lung-Centric Immunothrombosis
The recruitment and activation of neutrophils can result in the formation of neutrophil extracellular traps, or NETs (Figure 1). NETs are an extruded web of decondensed chromatin DNA decorated with granule-derived proteases and antimicrobials (Papayannopoulos, 2018; Boeltz et al., 2019). The formation of GSDMD pores in neutrophil membranes facilitates the release of NETs to the extracellular space (Tall and Westerterp, 2019; Chen et al., 2020). In lung tissues of fatal COVID-19 cases, NETs have been detected in close association with damaged alveoli (Middleton et al., 2020; Radermecker et al., 2020; Veras et al., 2020). Further, complexes of NETs and platelets, as well as thrombi, have been noted in the lung microvasculature (Iba et al., 2020; Leppkes et al., 2020; Radermecker et al., 2020). Collectively, these findings are consistent with immunothrombosis, a pathway linking innate immunity with thrombosis (Gaertner and Massberg, 2016; Iba et al., 2020; Nakazawa and Ishizu, 2020; Loo et al., 2021). The homeostatic function of this pathway is to limit pathogen spread.
As a caveat, the formation of thrombi may occlude the affected microvasculature and result in ischemic injury (Ackermann et al., 2020). An IL-1β/NET/coagulation pathway has been invoked in thrombogenesis in a cohort of acute coronary syndrome patients with high circulating CRP (Liberale et al., 2019). The lungs are particularly susceptible to immunothrombosis, given the readily available pool of neutrophils (Granton et al., 2018) and platelets (Lefrançais et al., 2017). In this scenario, a viral-induced inflammation promotes the formation of NETs by activated neutrophils. The NETs serve as a scaffold for sequestering activated platelets and components of the coagulation cascade (Mcdonald et al., 2017), setting the stage for the generation of thrombi. Occlusive thrombi within the pulmonary vasculature have been noted in fatal COVID-19 cases (Ackermann et al., 2020; Leppkes et al., 2020; Middleton et al., 2020; Radermecker et al., 2020; Rapkiewicz et al., 2020). Of note, anticoagulants (e.g., heparinoids) are advocated to alleviate the hypercoagulation state of COVID-19 (Bikdeli et al., 2020; Connors and Levy, 2020; Leentjens et al., 2021). However, given the potential for bleeding, specific guidelines for thromboprophylaxis in these patients await the outcome of ongoing clinical trials (Leentjens et al., 2021).
Circulating DNases can degrade the DNA backbone of NETs and may serve as an endogenous regulatory mechanism to limit immunothrombosis (Jiménez-Alcázar et al., 2017; Mcdonald et al., 2017). In murine models of sepsis, dismantling of NETs by DNases reduces occlusive intravascular clots in the lungs and improves survival (Jiménez-Alcázar et al., 2017; Lefrançais et al., 2018). In ARDS patients (all causes), the severity of disease and lethality is related to the ratio of plasma NETs/DNases (Lefrançais et al., 2018). A similar situation appears to exist in COVID-19 patients, circulating NETs are increased with a corresponding decrease in DNase levels (Lee et al., 2021).
In fatal cases of COVID-19 microvascular thrombi and necrotic injury in organs remote from the lungs have been noted on autopsies (Ackermann et al., 2020; Rapkiewicz et al., 2020; Tang et al., 2020). Potential mechanisms include spill-over of either the virus or host cytokines from damaged lungs into the systemic circulation. However, there is little evidence that viable SARS-CoV-2 becomes blood-borne (Andersson et al., 2020; Lamouroux et al., 2020; Vivanti et al., 2020). Further, circulating levels of proinflammatory cytokines do not reach levels considered detrimental to tissues (Kox et al., 2020; Mudd et al., 2020; Sinha et al., 2020). Whether the pulmonary NET-mediated immunothrombosis of COVID-19 can impact remote organs is not clear at present. While NETs and NET-associated thrombi have consistently been found in the lungs of fatal cases, their presence in remote organs is equivocal (Leppkes et al., 2020; Radermecker et al., 2020). Alternatively, while NET remnants (presumably due to DNase-induced turnover) occurs in COVID-19 (Leppkes et al., 2020), the initiated hypercoagulative and thrombotic milieu may be a source of NET-independent remote organ involvement (Connors and Levy, 2020). Given the paucity of information on this issue, any conclusions regarding mechanisms of remote organ injury in COVID-19 are rather speculative.
Potential Lung-Centric Therapy
The lung-centric inflammatory response prompts consideration of the potential clinical utility of bronchoscopy and BALF. BALF analyses are currently used to provide a microbiological diagnosis of COVID-19 in suspected cases, but in which nasopharyngeal swabs are negative for viral RNA (Mondoni et al., 2020; Patrucco et al., 2020). In addition, BALF analyses can also direct specific antimicrobial therapy and bronchoscopy can be used to clear the bronchial passage (Bruyneel et al., 2020). Of interest from a therapeutic perspective are the results of a murine study in which local lung inflammation and injury was induced by intratracheal LPS (Table 3). The recruited and activated PMN generated NETs within the airspace, as evidenced by NET markers in BALF (Liu et al., 2016). Unlike NETs formed in blood vessels, which are rapidly cleared (in part via DNase), NETs in the airspace are stable structures (Lefrançais et al., 2018). Exogenous intratracheal DNase reduces NETs in the BALF with a corresponding reduction in both BALF and systemic cytokines (Table 3). Of relevance to the ARDS of COVID-19, hypoxemic patients on ventilators were given nebulized recombinant human DNase I as part of their therapeutic regimen; no adverse effects were noted and the potential for benefit has prompted several clinical trials (Weber et al., 2020).
The possibility of directly targeting cytokines generated within the lungs of COVID-19 patients is attractive for at least two reasons. First, based on the lung-centric cytokine storm, reducing lung cytokines or their activity would also reduce corresponding circulating levels and their effects on remote organs. Second, intrapulmonary therapeutics would be less prone to off-target systemic effects. Ideally, an initial assessment of the BALF levels of cytokines in COVID-19 patients should be made as soon as possible after diagnosis. This would allow for therapeutic targeting of relevant cytokines. While early intervention would maximize patient benefit, limiting lung inflammation even in advanced cases is desirable.
The rapidly expanding repertoire of animal models of COVID-19 (Cleary et al., 2020; Muñoz-Fontela et al., 2020) should facilitate the translation of experimental findings to the clinical realm. While many of the models lack specific features noted in the human disease (e.g., lethality) (Ehaideb et al., 2020), some do have notable similarities to severe COVID-19. In this regard, transgenic mice expressing ACE2 under the cytokeratin 18 promoter (K18-hACE2) hold promise (Winkler et al., 2020; Yinda et al., 2021). These mice exhibit severe lung inflammation and dysfunction, lymphopenia, evidence of coagulopathy, remote organ involvement, and fatality. As it appears to be the case in COVID-19, viral RNA can be detected in remote organs of K18-hACE2 mice despite the absence of viremia (Yinda et al., 2021). Since murine models are readily amenable to experimental manipulation, this enigma may be resolved in future studies. Further, experiments to more directly address lung-centric immunothrombosis (e.g., NET formation, BALF to blood cytokine gradient) in these and other genetically tractable mice (Jarnagin et al., 2021) should provide a basis for lung-centric directed therapy.
Author Contributions
Conceptualization: PK and HF. Literature search: PK, HF, and SK. Data analysis and interpretation: PK and HF. Drafting the article: PK and HF. Critical revision of the article: PK, HF, SK, AY, EA-M, and KA-K. All authors contributed to the article and approved the submitted version.
Conflict of Interest
The authors declare that the research was conducted in the absence of any commercial or financial relationships that could be construed as a potential conflict of interest.
References
Ackermann, M., Verleden, S. E., Kuehnel, M., Haverich, A., Welte, T., Laenger, F., et al. (2020). Pulmonary Vascular Endothelialitis, Thrombosis, and Angiogenesis in Covid-19. N Engl. J. Med. 383, 120–128. doi: 10.1056/NEJMoa2015432
Agouridakis, P., Kyriakou, D., Alexandrakis, M. G., Prekates, A., Perisinakis, K., Karkavitsas, N., et al. (2002). The Predictive Role of Serum and Bronchoalveolar Lavage Cytokines and Adhesion Molecules for Acute Respiratory Distress Syndrome Development and Outcome. Respir. Res. 3, 25. doi: 10.1186/rr193
Andersson, M. I., Arancibia-Carcamo, C. V., Auckland, K., Baillie, J. K., Barnes, E., Beneke, T., et al. (2020). SARS-Cov-2 RNA Detected in Blood Products From Patients With COVID-19 Is Not Associated With Infectious Virus. Wellcome Open Res. 5, 181. doi: 10.12688/wellcomeopenres.16002.2
Barnes, B. J., Adrover, J. M., Baxter-Stoltzfus, A., Borczuk, A., Cools-Lartigue, J., Crawford, J. M., et al. (2020). Targeting Potential Drivers of COVID-19: Neutrophil Extracellular Traps. J. Exp. Med. 217, e20200652. doi: 10.1084/jem.20200652
Bikdeli, B., Madhavan, M. V., Jimenez, D., Chuich, T., Dreyfus, I., Driggin, E., et al. (2020). Covid-19 and Thrombotic or Thromboembolic Disease: Implications for Prevention, Antithrombotic Therapy, and Follow-Up: Jacc State-of-the-Art Review. J. Am. Coll. Cardiol. 75, 2950–2973. doi: 10.1016/j.jacc.2020.04.031
Blanco-Melo, D., Nilsson-Payant, B. E., Liu, W. C., Uhl, S., Hoagland, D., Moller, R., et al. (2020). Imbalanced Host Response to SARS-CoV-2 Drives Development of COVID-19. Cell 181, 1036–1045.e1039. doi: 10.1016/j.cell.2020.04.026
Boeltz, S., Amini, P., Anders, H. J., Andrade, F., Bilyy, R., Chatfield, S., et al. (2019). To NET or Not to NET:current Opinions and State of the Science Regarding the Formation of Neutrophil Extracellular Traps. Cell Death Differ. 26, 395–408. doi: 10.1038/s41418-018-0261-x
Bouros, D., Alexandrakis, M. G., Antoniou, K. M., Agouridakis, P., Pneumatikos, I., Anevlavis, S., et al. (2004). The Clinical Significance of Serum and Bronchoalveolar Lavage Inflammatory Cytokines in Patients at Risk for Acute Respiratory Distress Syndrome. BMC Pulm. Med. 4, 1–9. doi: 10.1186/1471-2466-4-6
Broz, P., Pelegrin, P., Shao, F. (2020). The Gasdermins, a Protein Family Executing Cell Death and Inflammation. Nat. Rev. Immunol. 20, 143–157. doi: 10.1038/s41577-019-0228-2
Bruyneel, M., Gabrovska, M., Rummens, P., Roman, A., Claus, M., Stevens, E., et al. (2020). Bronchoscopy in COVID-19 Intensive Care Unit Patients. Respirology 25, 1313–1315. doi: 10.1111/resp.13932
Carsana, L., Sonzogni, A., Nasr, A., Rossi, R. S., Pellegrinelli, A., Zerbi, P., et al. (2020). Pulmonary Post-Mortem Findings in a Series of COVID-19 Cases From Northern Italy: A Two-Centre Descriptive Study. Lancet Infect. Dis. 20, 1135–1140. doi: 10.1016/S1473-3099(20)30434-5
Castelli, V., Cimini, A., Ferri, C. (2020). Cytokine Storm in COVID-19: “When You Come Out of the Storm, You Won’t Be the Same Person Who Walked in”. Front. Immunol. 11, 2132. doi: 10.3389/fimmu.2020.02132
Chen, K. W., Demarco, B., Broz, P. (2020). Beyond Inflammasomes: Emerging Function of Gasdermins During Apoptosis and Netosis. EMBO J. 39, e103397. doi: 10.15252/embj.2019103397
Chowdhury, M. E. H., Rahman, T., Khandakar, A., Al-Madeed, S., Zughaier, S. M., Doi, S. A. R., et al. (2021). An Early Warning Tool for Predicting Mortality Risk of COVID-19 Patients Using Machine Learning. Cognit. Comput., 1–16. doi: 10.1007/s12559-020-09812-7
Cleary, S. J., Magnen, M., Looney, M. R., Page, C. P. (2020). Update on Animal Models for COVID-19 Research. Br. J. Pharmacol. 177, 5679–5681. doi: 10.1111/bph.15266
Connors, J. M., Levy, J. H. (2020). COVID-19 and Its Implications for Thrombosis and Anticoagulation. Blood 135, 2033–2040. doi: 10.1182/blood.2020006000
De Biasi, S., Meschiari, M., Gibellini, L., Bellinazzi, C., Borella, R., Fidanza, L., et al. (2020). Marked T Cell Activation, Senescence, Exhaustion and Skewing Towards TH17 in Patients With COVID-19 Pneumonia. Nat. Commun. 11, 3434. doi: 10.1038/s41467-020-17292-4
Deshmukh, V., Motwani, R., Kumar, A., Kumari, C., Raza, K. (2021). Histopathological Observations in COVID-19: A Systematic Review. J. Clin. Pathol. 74, 76–83. doi: 10.1136/jclinpath-2020-206995
Diao, B., Wang, C., Tan, Y., Chen, X., Liu, Y., Ning, L., et al. (2020). Reduction and Functional Exhaustion of T Cells in Patients With Coronavirus Disease 2019 (COVID-19). Front. Immunol. 11, 827. doi: 10.3389/fimmu.2020.00827
Ehaideb, S. N., Abdullah, M. L., Abuyassin, B., Bouchama, A. (2020). Evidence of a Wide Gap Between COVID-19 in Humans and Animal Models: A Systematic Review. Crit. Care 24, 594. doi: 10.1186/s13054-020-03304-8
Fajgenbaum, D. C., June, C. H. (2020). Cytokine Storm. New Engl. J. Med. 383, 2255–2273. doi: 10.1056/NEJMra2026131
Ferreira, A. C., Soares, V. C., De Azevedo-Quintanilha, I. G., Dias, S. D. S. G., Fintelman-Rodrigues, N., Sacramento, C. Q., et al. (2021). Sars-CoV-2 Engages Inflammasome and Pyroptosis in Human Primary Monocytes. Cell Death Discov. 7, 43. doi: 10.1038/s41420-021-00428-w
Folkesson, H. G., Matthay, M. A., Hebert, C. A., Broaddus, V. C. (1995). Acid Aspiration-Induced Lung Injury in Rabbits is Mediated by Interleukin-8-Dependent Mechanisms. J. Clin. Invest. 96, 107–116. doi: 10.1172/JCI118009
Gaertner, F., Massberg, S. (2016). Blood Coagulation in Immunothrombosis-At the Frontline of Intravascular Immunity. Semin. Immunol. 28, 561–569. doi: 10.1016/j.smim.2016.10.010
Giamarellos-Bourboulis, E. J., Netea, M. G., Rovina, N., Akinosoglou, K., Antoniadou, A., Antonakos, N., et al. (2020). Complex Immune Dysregulation in COVID-19 Patients With Severe Respiratory Failure. Cell Host Microbe. 27, 992–1000 e1003. doi: 10.1016/j.chom.2020.04.009
Granton, E., Kim, J. H., Podstawka, J., Yipp, B. G. (2018). The Lung Microvasculature Is a Functional Immune Niche. Trends Immunol. 39, 890–899. doi: 10.1016/j.it.2018.09.002
Hadjadj, J., Yatim, N., Barnabei, L., Corneau, A., Boussier, J., Smith, N., et al. (2020). Impaired Type I Interferon Activity and Inflammatory Responses in Severe COVID-19 Patients. Science 369, 718–724. doi: 10.1126/science.abc6027
Hariharan, A., Hakeem, A. R., Radhakrishnan, S., Reddy, M. S., Rela, M. (2021). The Role and Therapeutic Potential of NF-kappa-B Pathway in Severe COVID-19 Patients. Inflammopharmacology 29, 91–100. doi: 10.1007/s10787-020-00773-9
Hoffmann, M., Kleine-Weber, H., Schroeder, S., Krüger, N., Herrler, T., Erichsen, S., et al. (2020). Sars-CoV-2 Cell Entry Depends on ACE2 and TMPRSS2 and Is Blocked by a Clinically Proven Protease Inhibitor. Cell 181, 271–280. e278. doi: 10.1016/j.cell.2020.02.052
Iba, T., Levy, J. H., Levi, M., Connors, J. M., Thachil, J. (2020). Coagulopathy of Coronavirus Disease 2019. Crit. Care Med. 48, 1358–1364. doi: 10.1097/CCM.0000000000004458
Jarnagin, K., Alvarez, O., Shresta, S., Webb, D. R. (2021). Animal Models for SARS-Cov2/Covid19 Research-a Commentary. Biochem. Pharmacol. 188, 114543. doi: 10.1016/j.bcp.2021.114543
Jiménez-Alcázar, M., Rangaswamy, C., Panda, R., Bitterling, J., Simsek, Y. J., Long, A. T., et al. (2017). Host DNases Prevent Vascular Occlusion by Neutrophil Extracellular Traps. Science 358, 1202–1206. doi: 10.1126/science.aam8897
Kalbitz, M., Karbach, M., Braumueller, S., Kellermann, P., Gebhard, F., Huber-Lang, M., et al. (2016). Role of Complement C5 in Experimental Blunt Chest Trauma-Induced Septic Acute Lung Injury (Ali). PloS One 11, e0159417. doi: 10.1371/journal.pone.0159417
Kelley, N., Jeltema, D., Duan, Y., He, Y. (2019). The NLRP3 Inflammasome: An Overview of Mechanisms of Activation and Regulation. Int. J. Mol. Sci. 20, 3328. doi: 10.3390/ijms20133328
Kox, M., Waalders, N. J. B., Kooistra, E. J., Gerretsen, J., Pickkers, P. (2020). Cytokine Levels in Critically Ill Patients With COVID-19 and Other Conditions. Jama 324, 1565–1567. doi: 10.1001/jama.2020.17052
Lamouroux, A., Attie-Bitach, T., Martinovic, J., Leruez-Ville, M., Ville, Y. (2020). Evidence for and Against Vertical Transmission for Severe Acute Respiratory Syndrome Coronavirus 2. Am. J. Obstet. Gynecol. 223, e1–91. doi: 10.1016/j.ajog.2020.04.039
Lara, P. C., Macias-Verde, D., Burgos-Burgos, J. (2020). Age-Induced NLRP3 Inflammasome Over-activation Increases Lethality of SARS-CoV-2 Pneumonia in Elderly Patients. Aging Dis. 11, 756–762. doi: 10.14336/AD.2020.0601
Lax, S. F., Skok, K., Zechner, P., Kessler, H. H., Kaufmann, N., Koelblinger, C., et al. (2020). Pulmonary Arterial Thrombosis in COVID-19 With Fatal Outcome: Results From a Prospective, Single-Center, Clinicopathologic Case Series. Ann. Intern. Med. 173, 350–361. doi: 10.7326/M20-2566
Lee, S., Channappanavar, R., Kanneganti, T. D. (2020). Coronaviruses: Innate Immunity, Inflammasome Activation, Inflammatory Cell Death, and Cytokines. Trends Immunol. 41, 1083–1099. doi: 10.1016/j.it.2020.10.005
Lee, Y. L., Chen, W., Chen, L. Y., Chen, C. H., Lin, Y. C., Liang, S. J., et al. (2010). Systemic and Bronchoalveolar Cytokines as Predictors of in-Hospital Mortality in Severe Community-Acquired Pneumonia. J. Crit. Care 25, 176. e177–176. e113. doi: 10.1016/j.jcrc.2009.05.002
Leentjens, J., van Haaps, T. F., Wessels, P. F., Schutgens, R. E., Middeldorp, S. (2021). COVID-19-Associated Coagulopathy and Antithrombotic Agents—Lessons After 1 Year. Lancet Haematol. S2352-3026 (21), 00105–8. doi: 10.1016/S2352-3026(21)00105-8
Lee, Y. Y., Park, H. H., Park, W., Kim, H., Jang, J. G., Hong, K. S., et al. (2021). Long-Acting Nanoparticulate DNase-1 for Effective Suppression of SARS-CoV-2-Mediated Neutrophil Activities and Cytokine Storm. Biomaterials 267, 120389. doi: 10.1016/j.biomaterials.2020.120389
Lefrançais, E., Mallavia, B., Zhuo, H., Calfee, C. S., Looney, M. R. (2018). Maladaptive Role of Neutrophil Extracellular Traps in Pathogen-Induced Lung Injury. JCI Insight 3, e98178. doi: 10.1172/jci.insight.98178
Lefrançais, E., Ortiz-Muñoz, G., Caudrillier, A., Mallavia, B., Liu, F., Sayah, D. M., et al. (2017). The Lung Is a Site of Platelet Biogenesis and a Reservoir for Haematopoietic Progenitors. Nature 544, 105–109. doi: 10.1038/nature21706
Leppkes, M., Knopf, J., Naschberger, E., Lindemann, A., Singh, J., Herrmann, I., et al. (2020). Vascular Occlusion by Neutrophil Extracellular Traps in COVID-19. EBioMedicine 58, 102925. doi: 10.1016/j.ebiom.2020.102925
Liao, M., Liu, Y., Yuan, J., Wen, Y., Xu, G., Zhao, J., et al. (2020). Single-Cell Landscape of Bronchoalveolar Immune Cells in Patients With COVID-19. Nat. Med. 26, 842–844. doi: 10.1038/s41591-020-0901-9
Liberale, L., Holy, E. W., Akhmedov, A., Bonetti, N. R., Nietlispach, F., Matter, C. M., et al. (2019). Interleukin-1β Mediates Arterial Thrombus Formation via NET-Associated Tissue Factor. J. Clin. Med. 8, 2072. doi: 10.3390/jcm8122072
Liu, Q., Shi, Y., Cai, J., Duan, Y., Wang, R., Zhang, H., et al. (2020). Pathological Changes in the Lungs and Lymphatic Organs of 12 COVID-19 Autopsy Cases. Natl. Sci. Rev. 7, 1868–1878. doi: 10.1093/nsr/nwaa247
Liu, S., Su, X., Pan, P., Zhang, L., Hu, Y., Tan, H., et al. (2016). Neutrophil Extracellular Traps Are Indirectly Triggered by Lipopolysaccharide and Contribute to Acute Lung Injury. Sci. Rep. 6, 37252. doi: 10.1038/srep37252
Loo, J., Spittle, D. A., Newnham, M. (2021). Covid-19, Immunothrombosis and Venous Thromboembolism: Biological Mechanisms. Thorax. horaxjnl-2020-216243. doi: 10.1136/thoraxjnl-2020-216243
Lucas, C., Wong, P., Klein, J., Castro, T. B., Silva, J., Sundaram, M., et al. (2020). Longitudinal Analyses Reveal Immunological Misfiring in Severe COVID-19. Nature 584, 463–469. doi: 10.1038/s41586-020-2588-y
Manjili, R. H., Zarei, M., Habibi, M., Manjili, M. H. (2020). COVID-19 as an Acute Inflammatory Disease. J. Immunol. 205, 12–19. doi: 10.4049/jimmunol.2000413
Mcdonald, B., Davis, R. P., Kim, S. J., Tse, M., Esmon, C. T., Kolaczkowska, E., et al. (2017). Platelets and Neutrophil Extracellular Traps Collaborate to Promote Intravascular Coagulation During Sepsis in Mice. Blood 129, 1357–1367. doi: 10.1182/blood-2016-09-741298
Meduri, G. U., Headley, S., Tolley, E., Shelby, M., Stentz, F., Postlethwaite, A. (1995). Plasma and BAL Cytokine Response to Corticosteroid Rescue Treatment in Late ARDS. Chest 108, 1315–1325. doi: 10.1378/chest.108.5.1315
Mehta, P., Mcauley, D. F., Brown, M., Sanchez, E., Tattersall, R. S., Manson, J. J., et al. (2020). COVID-19: Consider Cytokine Storm Syndromes and Immunosuppression. Lancet 395, 1033–1034. doi: 10.1016/S0140-6736(20)30628-0
Merad, M., Martin, J. C. (2020). Pathological Inflammation in Patients With COVID-19: A Key Role for Monocytes and Macrophages. Nat. Rev. Immunol. 20 (6), 355–362. doi: 10.1038/s41577-020-0331-4
Middleton, E. A., He, X. Y., Denorme, F., Campbell, R. A., Ng, D., Salvatore, S. P., et al. (2020). Neutrophil Extracellular Traps Contribute to Immunothrombosis in COVID-19 Acute Respiratory Distress Syndrome. Blood 136, 1169–1179. doi: 10.1182/blood.2020007008
Milewska, A., Kula-Pacurar, A., Wadas, J., Suder, A., Szczepanski, A., Dabrowska, A., et al. (2020). Replication of Severe Acute Respiratory Syndrome Coronavirus 2 in Human Respiratory Epithelium. J. Virol. 94, e00957-20. doi: 10.1128/JVI.00957-20
Mondoni, M., Sferrazza Papa, G. F., Rinaldo, R., Faverio, P., Marruchella, A., D’arcangelo, F., et al. (2020). Utility and Safety of Bronchoscopy During the SARS-CoV-2 Outbreak in Italy: A Retrospective, Multicentre Study. Eur. Respir. J. 56, 2002767. doi: 10.1183/13993003.02767-2020
Morris, G., Bortolasci, C. C., Puri, B. K., Olive, L., Marx, W., O’neil, A., et al. (2021). Preventing the Development of Severe COVID-19 by Modifying Immunothrombosis. Life Sci. 264, 118617. doi: 10.1016/j.lfs.2020.118617
Mudd, P. A., Crawford, J. C., Turner, J. S., Souquette, A., Reynolds, D., Bender, D., et al. (2020). Distinct Inflammatory Profiles Distinguish COVID-19 From Influenza With Limited Contributions From Cytokine Storm. Sci. Adv. 6, eabe3024. doi: 10.1126/sciadv.abe3024
Muñoz-Fontela, C., Dowling, W. E., Funnell, S. G. P., Gsell, P. S., Riveros-Balta, A. X., Albrecht, R. A., et al. (2020). Animal Models for COVID-19. Nature 586, 509–515. doi: 10.1038/s41586-020-2787-6
Nakazawa, D., Ishizu, A. (2020). Immunothrombosis in Severe COVID-19. EBioMedicine 59, 102942. doi: 10.1016/j.ebiom.2020.102942
Osaki, Y., Maehara, Y., Sato, M., Hoshino, A., Yamamoto, K., Nagao, T., et al. (2010). Analysis of Cytokine/Chemokine Levels in Bronchoalveolar Lavage Fluids From Patients With Acute Respiratory Distress Syndrome. J. Jpn. Soc. Intensive Care Med. 17, 179–184. doi: 10.3918/jsicm.17.179
Pandolfi, L., Fossali, T., Frangipane, V., Bozzini, S., Morosini, M., D’amato, M., et al. (2020). Broncho-Alveolar Inflammation in COVID-19 Patients: A Correlation With Clinical Outcome. BMC Pulm. Med. 20, 301. doi: 10.1186/s12890-020-01343-z
Papayannopoulos, V. (2018). Neutrophil Extracellular Traps in Immunity and Disease. Nat. Rev. Immunol. 18, 134–147. doi: 10.1038/nri.2017.105
Patrucco, F., Albera, C., Bellocchia, M., Foci, V., Gavelli, F., Castello, L. M., et al. (2020). SARS-Cov-2 Detection on Bronchoalveolar Lavage: An Italian Multicenter Experience. Respiration 99, 970–978. doi: 10.1159/000511964
Phua, J., Weng, L., Ling, L., Egi, M., Lim, C. M., Divatia, J. V., et al. (2020). Intensive Care Management of Coronavirus Disease 2019 (COVID-19): Challenges and Recommendations. Lancet Respir. Med. 8, 506–517. doi: 10.1016/S2213-2600(20)30161-2
Radermecker, C., Detrembleur, N., Guiot, J., Cavalier, E., Henket, M., D’emal, C., et al. (2020). Neutrophil Extracellular Traps Infiltrate the Lung Airway, Interstitial, and Vascular Compartments in Severe COVID-19. J. Exp. Med. 217, e20201012. doi: 10.1084/jem.20201012
Rapkiewicz, A. V., Mai, X., Carsons, S. E., Pittaluga, S., Kleiner, D. E., Berger, J. S., et al. (2020). Megakaryocytes and Platelet-Fibrin Thrombi Characterize Multi-Organ Thrombosis at Autopsy in COVID-19: A Case Series. EClinicalMedicine 24, 100434. doi: 10.1016/j.eclinm.2020.100434
Ricard, J. D., Dreyfuss, D., Saumon, G. (2001). Production of Inflammatory Cytokines in Ventilator-Induced Lung Injury: A Reappraisal. Am. J. Respir. Crit. Care Med. 163, 1176–1180. doi: 10.1164/ajrccm.163.5.2006053
Rodrigues, T. S., De Sa, K. S. G., Ishimoto, A. Y., Becerra, A., Oliveira, S., Almeida, L., et al. (2021). Inflammasomes Are Activated in Response to SARS-CoV-2 Infection and Are Associated With COVID-19 Severity in Patients. J. Exp. Med. 218, e20201707. doi: 10.1084/jem.20201707
Ronit, A., Berg, R. M. G., Bay, J. T., Haugaard, A. K., Ahlstrom, M. G., Burgdorf, K. S., et al. (2021). Compartmental Immunophenotyping in COVID-19 Ards: A Case Series. J. Allergy Clin. Immunol. 147, 81–91. doi: 10.1016/j.jaci.2020.09.009
Schaefer, I. M., Padera, R. F., Solomon, I. H., Kanjilal, S., Hammer, M. M., Hornick, J. L., et al. (2020). In Situ Detection of SARS-CoV-2 in Lungs and Airways of Patients With COVID-19. Mod. Pathol. 33, 2104–2114. doi: 10.1038/s41379-020-0595-z
Schutte, H., Lohmeyer, J., Rosseau, S., Ziegler, S., Siebert, C., Kielisch, H., et al. (1996). Bronchoalveolar and Systemic Cytokine Profiles in Patients With ARDS, Severe Pneumonia and Cardiogenic Pulmonary Oedema. Eur. Respir. J. 9, 1858–1867. doi: 10.1183/09031936.96.09091858
Sinha, P., Matthay, M. A., Calfee, C. S. (2020). Is a “Cytokine Storm” Relevant to COVID-19? JAMA Internal Med. 180, 1152–1154. doi: 10.1001/jamainternmed.2020.3313
Swanson, K. V., Deng, M., Ting, J. P. (2019). The NLRP3 Inflammasome: Molecular Activation and Regulation to Therapeutics. Nat. Rev. Immunol. 19, 477–489. doi: 10.1038/s41577-019-0165-0
Tall, A. R., Westerterp, M. (2019). Inflammasomes, Neutrophil Extracellular Traps, and Cholesterol. J. Lipid Res. 60, 721–727. doi: 10.1194/jlr.S091280
Tang, D., Comish, P., Kang, R. (2020). The Hallmarks of COVID-19 Disease. PloS Pathog. 16, e1008536. doi: 10.1371/journal.ppat.1008536
Tay, M. Z., Poh, C. M., Renia, L., Macary, P. A., Ng, L. F. P. (2020). The Trinity of COVID-19: Immunity, Inflammation and Intervention. Nat. Rev. Immunol. 20, 363–374. doi: 10.1038/s41577-020-0311-8
Toldo, S., Bussani, R., Nuzzi, V., Bonaventura, A., Mauro, A. G., Cannata, A., et al. (2021). Inflammasome Formation in the Lungs of Patients With Fatal COVID-19. Inflammation Res. 70, 7–10. doi: 10.1007/s00011-020-01413-2
Veras, F. P., Pontelli, M. C., Silva, C. M., Toller-Kawahisa, J. E., De Lima, M., Nascimento, D. C., et al. (2020). Sars-CoV-2-Triggered Neutrophil Extracellular Traps Mediate COVID-19 Pathology. J. Exp. Med. 217, e20201129. doi: 10.1084/jem.20201129
Vivanti, A. J., Vauloup-Fellous, C., Prevot, S., Zupan, V., Suffee, C., Do Cao, J., et al. (2020). Transplacental Transmission of SARS-CoV-2 Infection. Nat. Commun. 11, 3572. doi: 10.1038/s41467-020-17436-6
Wang, Y. M., Ji, R., Chen, W. W., Huang, S. W., Zheng, Y. J., Yang, Z. T., et al. (2019). Paclitaxel Alleviated Sepsis-Induced Acute Lung Injury by Activating MUC1 and Suppressing TLR-4/NF-κb Pathway. Drug Des. Devel. Ther. 13, 3391–3404. doi: 10.2147/DDDT.S222296
Wang, C., Kang, K., Gao, Y., Ye, M., Lan, X., Li, X., et al. (2020a). Cytokine Levels in the Body Fluids of a Patient With COVID-19 and Acute Respiratory Distress Syndrome: A Case Report. Ann. Intern. Med. 173, 499–501. doi: 10.7326/L20-0354
Wang, Y. M., Qi, X., Gong, F. C., Chen, Y., Yang, Z. T., Mao, E. Q., et al. (2020b). Protective and Predictive Role of Mucin1 in Sepsis-Induced ALI/ARDS. Int. Immunopharmacol. 83, 106438. doi: 10.1016/j.intimp.2020.106438
Weber, A. G., Chau, A. S., Egeblad, M., Barnes, B. J., Janowitz, T. (2020). Nebulized In-Line Endotracheal Dornase Alfa and Albuterol Administered to Mechanically Ventilated COVID-19 Patients: A Case Series. Mol. Med. 26, 91. doi: 10.1186/s10020-020-00215-w
Winkler, E. S., Bailey, A. L., Kafai, N. M., Nair, S., Mccune, B. T., Yu, J., et al. (2020). Sars-CoV-2 Infection of Human ACE2-Transgenic Mice Causes Severe Lung Inflammation and Impaired Function. Nat. Immunol. 21, 1327–1335. doi: 10.1038/s41590-020-0778-2
Yang, D., Chu, H., Hou, Y., Chai, Y., Shuai, H., Lee, A. C.-Y., et al. (2020). Attenuated Interferon and Proinflammatory Response in SARS-CoV-2–Infected Human Dendritic Cells Is Associated With Viral Antagonism of STAT1 Phosphorylation. J. Infect. Dis. 222, 734–745. doi: 10.1093/infdis/jiaa356
Yinda, C. K., Port, J. R., Bushmaker, T., Offei Owusu, I., Purushotham, J. N., Avanzato, V. A., et al. (2021). K18-hACE2 Mice Develop Respiratory Disease Resembling Severe COVID-19. PloS Pathog. 17, e1009195. doi: 10.1371/journal.ppat.1009195
Zhao, Q., Meng, M., Kumar, R., Wu, Y., Huang, J., Deng, Y., et al. (2020). Lymphopenia Is Associated With Severe Coronavirus Disease 2019 (COVID-19) Infections: A Systemic Review and Meta-Analysis. Int. J. Infect. Dis. 96, 131–135. doi: 10.1016/j.ijid.2020.04.086
Zhao, C., Zhao, W. (2020). Nlrp3 Inflammasome—A Key Player in Antiviral Responses. Front. Immunol. 11, 211. doi: 10.3389/fimmu.2020.00211
Zheng, H.-Y., Zhang, M., Yang, C.-X., Zhang, N., Wang, X.-C., Yang, X.-P., et al. (2020). Elevated Exhaustion Levels and Reduced Functional Diversity of T Cells in Peripheral Blood May Predict Severe Progression in COVID-19 Patients. Cell. Mol. Immunol. 17, 541–543. doi: 10.1038/s41423-020-0401-3
Keywords: acute respiratory distress syndrome (ARDS), coronavirus, COVID-19, cytokine storm, NET, SARS-CoV-2
Citation: Kvietys PR, Fakhoury HMA, Kadan S, Yaqinuddin A, Al-Mutairy E and Al-Kattan K (2021) COVID-19: Lung-Centric Immunothrombosis. Front. Cell. Infect. Microbiol. 11:679878. doi: 10.3389/fcimb.2021.679878
Received: 12 March 2021; Accepted: 31 May 2021;
Published: 11 June 2021.
Edited by:
Janet S. Lee, University of Pittsburgh, United StatesReviewed by:
William Bain, University of Pittsburgh, United StatesMark Looney, University of California, San Francisco, United States
Samithamby Jey Jeyaseelan, Louisiana State University, United States
Copyright © 2021 Kvietys, Fakhoury, Kadan, Yaqinuddin, Al-Mutairy and Al-Kattan. This is an open-access article distributed under the terms of the Creative Commons Attribution License (CC BY). The use, distribution or reproduction in other forums is permitted, provided the original author(s) and the copyright owner(s) are credited and that the original publication in this journal is cited, in accordance with accepted academic practice. No use, distribution or reproduction is permitted which does not comply with these terms.
*Correspondence: Hana. M. A. Fakhoury, hana.fakhoury@gmail.com