Bacillus subtilis Colonization of Arabidopsis thaliana Roots Induces Multiple Biosynthetic Clusters for Antibiotic Production
- 1Department of Molecular Genetics, Weizmann Institute of Science, Rehovot, Israel
- 2Flow Cytometry Unit, Life Sciences Core Facilities, Weizmann Institute of Science, Rehovot, Israel
Beneficial and probiotic bacteria play an important role in conferring immunity of their hosts to a wide range of bacterial, viral, and fungal diseases. Bacillus subtilis is a Gram-positive bacterium that protects the plant from various pathogens due to its capacity to produce an extensive repertoire of antibiotics. At the same time, the plant microbiome is a highly competitive niche, with multiple microbial species competing for space and resources, a competition that can be determined by the antagonistic potential of each microbiome member. Therefore, regulating antibiotic production in the rhizosphere is of great importance for the elimination of pathogens and establishing beneficial host-associated communities. In this work, we used B. subtilis as a model to investigate the role of plant colonization in antibiotic production. Flow cytometry and imaging flow cytometry (IFC) analysis supported the notion that Arabidopsis thaliana specifically induced the transcription of the biosynthetic clusters for the non-ribosomal peptides surfactin, bacilysin, plipastatin, and the polyketide bacillaene. IFC was more robust in quantifying the inducing effects of A. thaliana, considering the overall heterogeneity of the population. Our results highlight IFC as a useful tool to study the effect of association with a plant host on bacterial gene expression. Furthermore, the common regulation of multiple biosynthetic clusters for antibiotic production by the plant can be translated to improve the performance and competitiveness of beneficial members of the plant microbiome.
Introduction
Rhizobacteria can promote plant growth directly by colonization of the root and exert beneficial effects on plant growth and development (Kloepper et al., 2004). These bacteria are often designated plant growth-promoting rhizobacteria (PGPR). To date, various PGPR have been isolated, including various Bacillus species, Burkholderia cepacia, and Pseudomonas fluorescens. These beneficial rhizobacteria can also confer fitness on their hosts by activating their immune system and by antagonizing plant pathogens (Berg et al., 2017; Berg and Raaijmakers, 2018; Allaband et al., 2019). In addition to the direct promotion of plant growth, PGPR enhance the efficiency of fertilizers and aid in degrading xenobiotic compounds (Adam et al., 2016; Berg et al., 2017).
Among growth-promoting strains and biocontrol agents, Bacillus subtilis and subtilis clade members, such as B. atrophaeus, B. velezensis, and B. mojavensis, are considered model organisms (Fan et al., 2017). In particular, the antimicrobial activity of B. subtilis has so far been demonstrated against bacterial, viral, and fungal soil-borne plant pathogens (Kloepper et al., 2004; Nagorska et al., 2007). This activity is mediated largely by antibiotic production: approximately 5% of the B. subtilis genome is dedicated to the synthesis of antimicrobial molecules by non-ribosomal peptide synthetases (NRPSs) or polyketide synthases (PKSs) (Stein, 2005; Ongena and Jacques, 2008; Kinsella et al., 2009; Caulier et al., 2019). In vitro and in planta studies have indicated the importance of four antibiotics for plant protection: surfactin, bacilysin, plipastatin, and bacillaene (Stein, 2005; Hou and Kolodkin-Gal, 2020; Arnaouteli et al., 2021; Ngalimat et al., 2021).
Surfactin is a small cyclic lipopeptide induced during the development of genetic competence (Magnuson et al., 1994). The machinery for surfactin synthesis is encoded within the srfAA–AB–AC–AD operon (Kluge et al., 1988). Surfactin is a powerful surfactant with antibacterial (Gonzalez et al., 2011) and antifungal properties (Falardeau et al., 2013). The expression of srfAA-AD operon is induced by the ComQXPA quorum-sensing system at the end of the exponential growth phase. In response to the competence pheromones, the phosphorylated response regulator ComA~P activates the transcription of the srf operon (Roggiani and Dubnau, 1993; Auchtung et al., 2006). A recent study reported that interaction with rice seedlings induces the expression on srfAA in B. subtilis OKB105 (Xie et al., 2015).
Bacilysin is a non-ribosomal dipeptide composed of L-alanine and amino acid L-anticapsin (Hernandez-Valdes et al., 2020), which demonstrates antibacterial activity against a wide range of pathogens (Walker and Abraham, 1970; Chen et al., 2009; Hou and Kolodkin-Gal, 2020). Its synthesis is controlled mainly by the bac operon (bacABCDE) (Inaoka et al., 2003; Rajavel et al., 2009).
Fengycin/plipastatin is a lipopeptide comprising 10 amino acid core linked to a β-hydroxy fatty acid and is synthetized by five plipastatin synthetases (ppsA, ppsB, ppsC, ppsD, and ppsE) (Tsuge et al., 2007). The ppsA-E operon is repressed by the transition state regulator AbrB during the exponential growth phase and is induced in the stationary phase. The pleiotropic regulator degQ gene increases the transcription from the plipastatin promoter (Rajavel et al., 2009).
Bacillaene and dihydrobacillaene (Butcher et al., 2007; Straight et al., 2007) are polyketides synthesized by an enzymatic complex encoded in the pks gene cluster (Butcher et al., 2007; Straight et al., 2007). The 5′ UTR of pksC was found to be an element for induction of the pks operon. The transcription of the biosynthetic clusters for bacillaene is primarily controlled by the transition phase regulators Spo0A and CodY and is also affected by DegU, ComA, and ScoC (Vargas-Bautista et al., 2014). Interestingly, we recently found that the plant host can enhance the efficiency of the killing of Serratia plymuthica by B. subtilis by inducing the synthesis of the antibiotic bacillaene (Ogran et al., 2019). These preliminary results raise the question on whether additional antibiotics that contribute to rhizocompatibility are induced by Arabidopsis thaliana to promote the colonization of preferred symbionts. However, as the transcriptional regulation of the biosynthetic clusters for antibiotics is diverse, this hypothesis needs to be evaluated experimentally.
To address this question systematically, we considered the overall effect of the plant host in regulating the transcription from four distinct promoters for B. subtilis antibiotics: surfactin, bacillaene, bacilysin, and plipastatin. As the population within B. subtilis biofilms and root associated communities is heterogeneous (Lopez et al., 2009; Beauregard et al., 2013; Tian et al., 2021), we monitored the expression in the single-cell level relying on flow cytometry and imaging flow cytometry (IFC). The latter combines the power and speed of traditional flow cytometers with the resolution of a microscope. It therefore allows for high rate complex morphometric measurements in a phenotypically defined way (Zuba-Surma et al., 2007). Our results indicated that the attachment with the root can specifically enhance antibiotic production and therefore may affect the competitiveness of root-associated bacteria compared with their free-living counterparts.
The use of IFC to study gene expression in bacteria was shown in several studies, as, for example, a study that examined the promoter activity of various genes in E. coli during the lag phase (Madar et al., 2013). However, to the best of our knowledge, this study is the first time this system has been used to study plant–bacteria interactions and the impact of such interactions on production of all four NRPs/PKS antibiotics.
Results
We wanted to investigate whether the plant can affect the production of several NRPs/PKS antibiotics and to compare between the different plant influences. Hence, we generated B. subtilis strains harboring transcriptional fusions for PsrfAA-yfp (surfactin), PpksC-gfp (bacillaene), PbacA-gfp (bacilysin), and PppsA-gfp (plipastatin) (Table 1). The native promoters of these antibiotics regulate transcription of NRPs/PKS and the rate of transcription is influenced by binding of specific transcription factors.
First, we asked whether the association of B. subtilis with the plant root impacts the expression of each of the antibiotics. Hence, using flow cytometry, we examined the expression of transcriptional reporters by comparing bacteria cultured in liquid MSgg medium with bacteria attached to plant roots. In order to select the bacteria attached to plant roots, the plant roots were washed to remove non-adherent cells. Our flow cytometry analysis showed that the percentage of cells expressing the fluorescent reporter of each operon significantly increased after root attachment in srf (p < 0.0001), pks (p < 0.0005), and bac (p = 0.03) promoters, while it was not significantly increased in pps (p = 0.579). In addition, the mean intensity of cells expressing the promoters increased significantly in pps (p < 0.0001) and pks (p < 0.0001) (Figure 1).
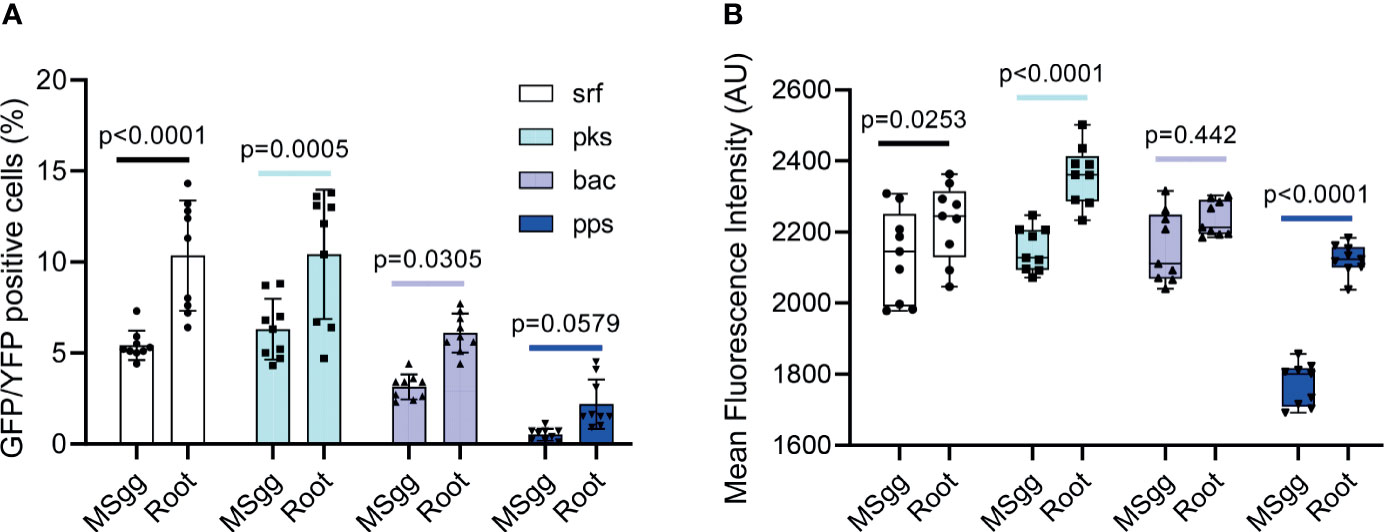
Figure 1 The indicated B. subtilis strain harboring PsrfAA-yfp (surfactin), PpksC-gfp (bacillaene), PbacA-gfp (bacilysin), and PppsA-gfp (plipastatin) reporters was analyzed by flow cytometry for (A) positively expressing fluorescent populations. Graphs represent mean ± SD. (B) The mean intensity of the fluorescent populations. Box and whisker plot shows median and interquartile range, together with the maximum and minimum values and outlier points. B. subtilis reporter strains were grown in either MSgg medium or MSgg medium in the presence of A. thaliana seedlings. Data were collected from 24 h post inoculation, and 100,000 cells were counted. Graphs represent results from three independent experiments with n = 3/experiment (total n = 9/group). Statistical analysis was performed using two-way ANOVA followed by Tukey’s multiple comparison post hoc testing. p < 0.05 was considered statistically significant.
This conventional flow cytometry allows accurate high-throughput quantification of fluorescence intensities; however, it is less accurate for bacterial analysis. Higher fluorescence levels may be interpreted as higher expression levels, but also can result from larger bacterial size or small aggregates (Branda et al., 2001; Vlamakis et al., 2008; Chai et al., 2010). Furthermore, the mean intensities measured were indicators of the entire fluorescent positive population rather than an individual bacterial cell. Therefore, to increase our resolution into the manner by which antibiotic promoters respond to the attachment of the root, we used IFC. By collecting large numbers of digital images per sample and providing a numerical representation of image-based features, the ImageStreamX Mark II combines the per-cell information content provided by standard microscopy with the statistical significance afforded by large sample sizes common to traditional flow cytometry.
This analysis indeed allowed us to exclude most of the bacterial doublets or small aggregates and calculate more accurately both the percentage of positive GFP cells and bacterial cell length and GFP intensity normalized for cell size. This detailed analysis of antibiotic reporters demonstrated that the percentage of fluorescent positive cells for all four antibiotics increased when attached to the plant roots (Figure 2). Interestingly, when attached to plant roots, percentage of cells from PsrfAA-yfp (surfactin) showed an increase of ≈2-fold, PpksC-gfp (bacillaene) showed an increase of ≈5-fold, PbacA-gfp (bacilysin) showed an increase of ≈10-fold, and PppsA-gfp (plipastatin) showed an increase of ≈3-fold. Such clear differences were not observed in the conventional flow cytometry. Furthermore, the mean fluorescent pixel intensity of the cells significantly increased following root attachment in all four antibiotic promoters (Figures 3A, B).
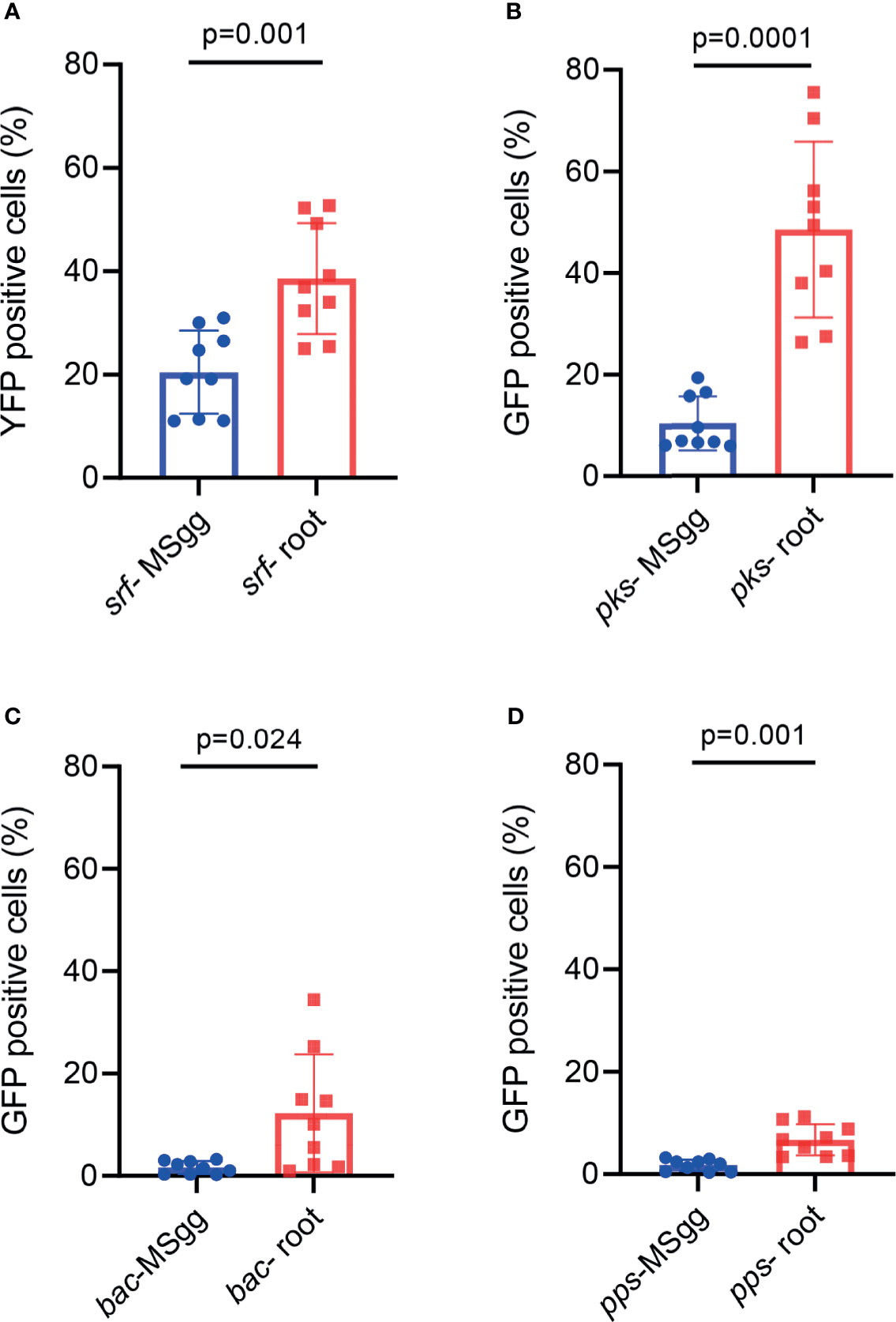
Figure 2 The indicated B. subtilis strain harboring (A) PsrfAA-yfp (surfactin), (B) PpksC-gfp (bacillaene), (C) PbacA-gfp (bacilysin), and (D) PppsA-gfp (plipastatin) reporters was analyzed by imaging flow cytometry for positively expressing fluorescent populations. B. subtilis reporter strains were grown in either MSgg medium or MSgg medium in the presence of A. thaliana seedlings. Data were collected from 24 h post inoculation, and 100,000 cells were counted. Graphs represent results from three independent experiments with n = 3/experiment (total n = 9/group). The percentage of fluorescent positive cells was measured by imaging flow cytometry and analyzed with IDEAS 6.3. Statistical analysis was performed using unpaired t-test with Welch correction. p < 0.05 was considered statistically significant. Graphs represent mean ± SD.
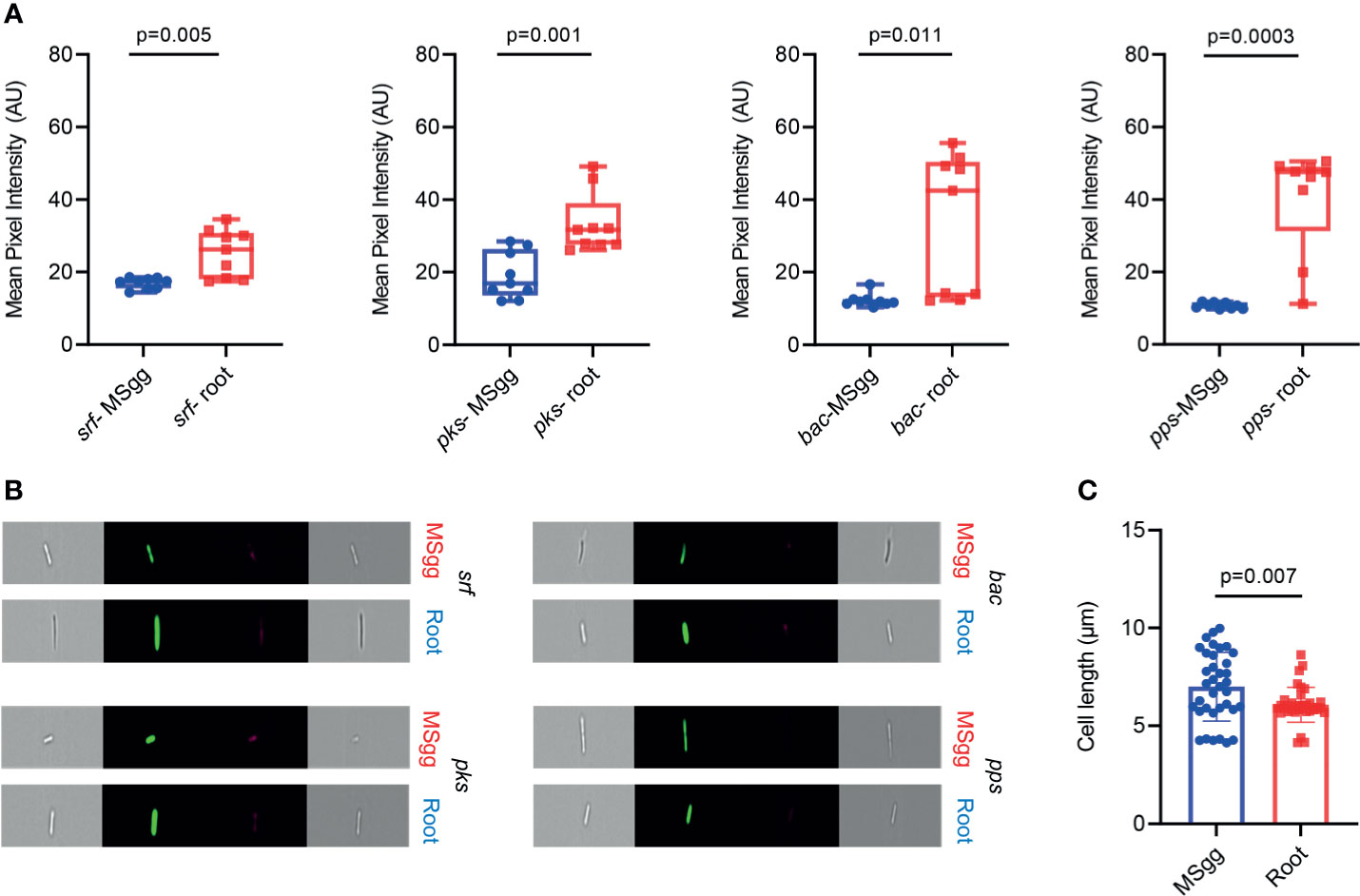
Figure 3 The indicated B. subtilis strain harboring PsrfAA-yfp (surfactin), PpksC-gfp (bacillaene), PbacA-gfp (bacilysin), and PppsA-gfp (plipastatin) reporters was analyzed by imaging flow cytometry for (A) mean pixel intensity of the fluorescent positive populations. Bacteria expressing the fluorescent reporters were cultured in the absence or presence of A. thaliana seedlings. Box and whisker plot shows median and interquartile range, together with the maximum and minimum values and outlier points. (B) Representative bright-field and fluorescent images related to expression of reporters in MSgg and on A. thaliana roots. Scale bar = 7 µm. (C) Comparison between cell length of all four reporters when grown in MSgg medium and cell length of the same reporters grown on A. thaliana roots. Graphs represent mean ± SD. Data were collected from 24 h post inoculation, and 100,000 cells were counted. The mean pixel intensity and cell length of fluorescent positive cells were measured by imaging flow cytometry and analyzed with IDEAS 6.3. Graphs represent results from three independent experiments with n = 3/experiment (total n = 9/group). Statistical analysis was performed using unpaired t-test with Welch correction. p < 0.05 was considered statistically significant.
Interestingly conventional flow cytometry could not explore differences in the mean fluorescence intensity of cells from PsrfAA-yfp (surfactin) and PbacA-gfp (bacilysin) when attached to roots (Figure 1) compared with cells grown in MSgg medium (p = 0.253 and p = 0.442, respectively). However, IFC indicated that the mean pixel intensity of the fluorescent populations of these two reporters was significantly higher (p = 0.005 and p = 0.011, respectively), showing a higher sensitivity of the method.
The quantification performed with IFC also yielded a higher percentage of fluorescence positive cells as compared with the traditional flow cytometry in all four promoters in the presence of plant root. This may be explained by the sensitivity and ability to acquire individual pixels by IFC, allowing the detection of low fluorescence intensity signals of small objects that might be within the electronic noise of conventional flow cytometers, as well as elimination of debris and aggregates. This results in better identification and separation of the bacterial population from the background noise and often provides more robust results. Overall, these results suggest that IFC is a sensitive and accurate technique to study weakly expressed genes in bacterial cells. Importantly, both flow cytometry and IFC agreed that all four antibiotics are induced by the plant.
Next, we examined if the plant and its secretions specifically regulate antibiotic production or induce all genes in B. subtilis due to non-specific effect of its growth, as the plant may affect the synthesis or stability of GFP regardless of its promoter. For this purpose, we measured the expression of transcriptional reporter of the β-lactamase PenP. β-lactamases are enzymes that account for an additional layer of defense as they hydrolyze the β-lactam ring of β-lactams, thus inactivating the antibiotic before it reaches its target, the PBPs (penicillin binding proteins) (Therrien and Levesque, 2000). The active β-lactamase of B. subtilis (Takagi et al., 1993; Bucher et al., 2019) was not induced but rather modestly decreased (p = 0.015) in the presence of the plant (Supplementary Figure S1). In addition, the expression of the core metabolic enzyme lactate dehydrogenase ldh was also unaffected by the root (Supplementary Figure S1). Therefore, the effect of the plant is not through post-translational effects on the GFP reporter, and at least two enzymes unrelated to the biosynthesis of NRP/polyketide antibiotics are not induced by the plant.
To study whether attachment to plant roots also influences bacterial cell length, we grouped all measurements into two groups, bacteria grown in MSgg medium (control) and bacteria attached to plant roots. Interestingly, when we compared the cell length among the bacteria from all the reporters attached to plant roots to that of MSgg, the data in the MSgg group showed a higher degree of dispersion (SD =1.76) as compared to the bacteria attached to the plant roots (SD = 0.90). An F-test further confirmed that the variances were significantly different between two groups (F-test, p-value = 0.0001) (Figure 3C).
To confirm that the impact of the plant root on antibiotic production could be due to a secreted factor, we monitored the expression from PsrfAA and PpksC fused to a luciferase reporter in the presence and absence of root secretions. The use of the unstable luciferase (an enzyme that degrades rapidly and therefore is not accumulated) (McLoon et al., 2011) as a reporter allows us to monitor gene expression in real time by monitoring light production in a plate reader with an illuminometer. When grown on liquid defined medium, wild-type cells expressed luciferase from srfAA and pksC promoters robustly. However, while root secretions did not alter the kinetics of the expression, they were sufficient to significantly increase the intensity of the luciferase emission (Figure 4). Therefore, the measurement of the inducing effect on the level of the population agreed with our single-cell analysis and confirmed an induction by A. thaliana and also suggested that this induction is mediated by a secreted factor that can activate the distinct promoters for bacillaene and surfactin synthesis (Table 1). Consistently, using confocal scanning laser microscopy, we could clearly confirm the expression of the pks promoter and, to some extent, surfactin on cells attached with A. thaliana roots (Figure 5). This experiment also exemplified the adherence of the bacteria to the plant root after 24 h of co-culture, which was also the time point used to quantify the expression from the four promoters of the antibiotic biosynthetic clusters by flow cytometry and IFC.
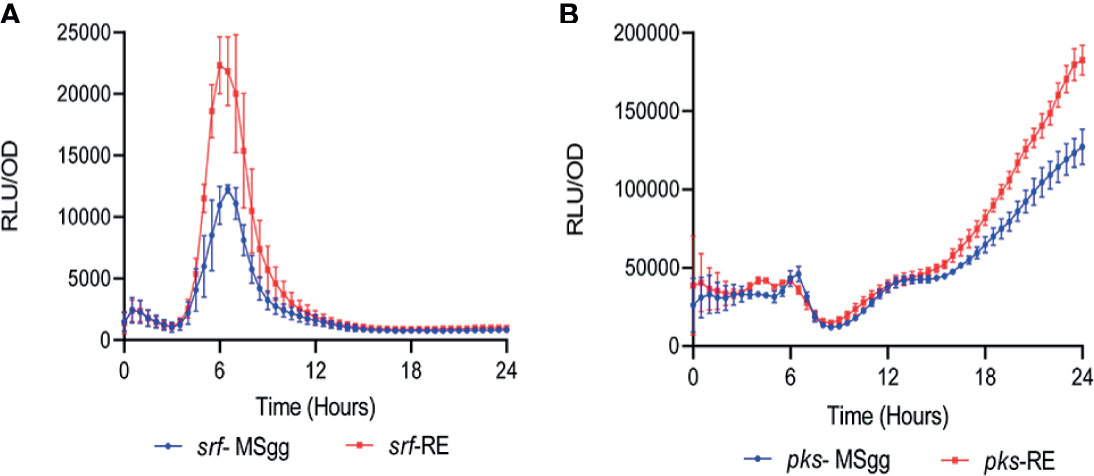
Figure 4 A. thaliana secretions increase the expression of (A) PsrfAA-lux (surfactin) and (B) PpksC-lux (bacilllane) in B. subtilis cells. B. subtilis strains expressing luciferase under the control of the srf and pks promoters were cultured in liquid MSgg medium or liquid MSgg medium supplemented with A. thaliana secretions (15% v/v). Luminescence was monitored for 24 h using a microplate reader. Graphs represent results from three independent experiments. Error bars represent ± SEM. Luciferase activity was normalized to avoid artifacts related to differential cell numbers as RLU/OD.
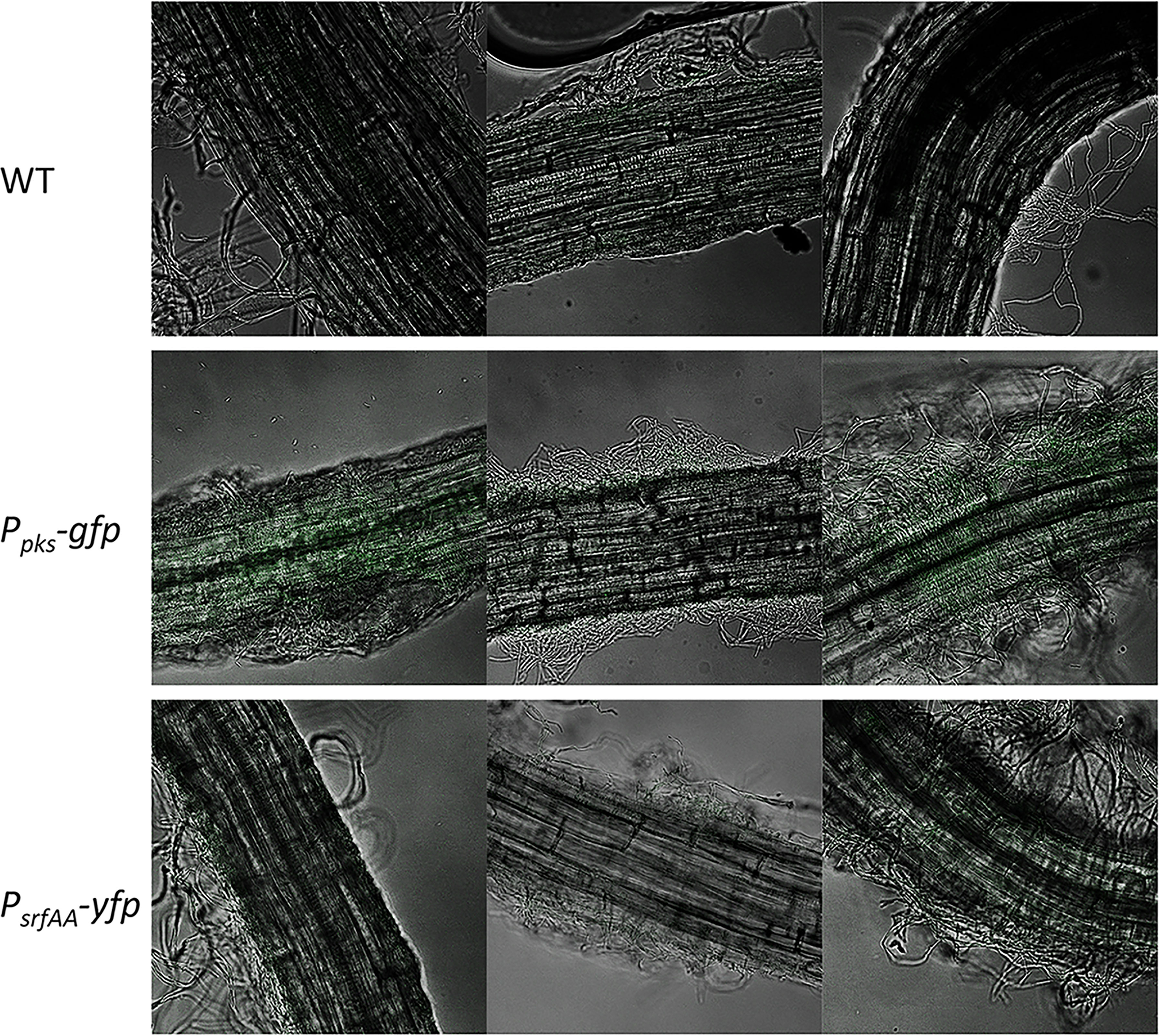
Figure 5 Visualizing the expression of PpksC-gfp (bacillaene) and PsrfAA-gfp (surfactin) on A. thaliana roots. Bacteria expressing GFP under the control of the pks and srf promoters were cultured in the presence of A. thaliana seedlings in MSgg medium. After 24 h, the bacteria colonizing the roots were photographed with a confocal microscope.
Discussion
Since B. subtilis was first described by Ferdinand Cohn in the late 1800s, it was shown to specialize in the production of secondary metabolites (Steinke et al., 2021). Many of the biosynthetic pathways for these metabolites are conserved either across the entire Bacillus genus or within specific phylogenetic clades. Surfactin, bacillaene, bacilysin, and plipastatin have essentially been observed within the subtilis clade (Aleti et al., 2015; Hou and Kolodkin-Gal, 2020). Therefore, the different environmental niches inhabited by members of the B. subtilis clade may select for conservation of metabolites with distinct (or potentially redundant) beneficial functions.
Here, we found that the production of non-ribosomal peptides and polyketides was specifically activated during symbiotic interaction with A. thaliana. Our results demonstrated that direct interactions with the root increased the expression of four different biosynthetic clusters with distinct promoters encoding for antibiotics with significance for B. subtilis competitiveness, and the secretions were sufficient to induce surfactin and bacillaene expression. The four promoters of the biosynthetic clusters differ in their sequence and regulations (Table 1). Therefore, it is not intuitive that they will be co-induced together following attachment to the root.
Indeed, various studies reported that plant metabolites induce the expression of NRPs and antibiotic production genes. We previously demonstrated that the interaction with the plant increases the capacity of B. subtilis to compete with Serattia plymuthica, and our current results further indicate that the root is an active regulator of the competitive interactions occurring on its roots (Ogran et al., 2019). An increase in bacterial competitiveness due to increased antibiotic production may be a conserved feature of rhizobacteria: plants enhance the killing efficiency of Xanthomonas citri by Paenibacillus polymyxa SC2 (Liu et al., 2021), wheat extract induces the expression of biosynthetic genes for antibiotic production in Pseudomonas genotypes (Rieusset et al., 2021), and barley induces the antifungal genes of Pseudomonas fluorescens (Jousset et al., 2011). Our methodology here offers a practical approach to study the effect of plant metabolites on heterogeneous communities, even when expressed by a small subpopulation of cells, with IFC to analyze the population at the single-cell level, and luciferase-based reporters to screen for potential activators. While flow cytometry can detect the trends efficiently, statistical significance is frequently not achieved for antibiotic producers while performing multiple comparisons. These cases include weakly expressing promoters and heterogeneous populations.
Our findings that all four biosynthetic clusters were induced by the root strongly indicate co-evolution of the regulation of biosynthetic clusters for antibiotic production. The complexity of these antibiotic–host interactions suggests that the plant host actively promotes the establishment of the most beneficial bacterial community. Our findings provide a simple example of high-order interactions that shape microbiomes; the host modulates antibiotic production in the desired bacterial colonizers, providing the colonizers a clear advantage over less beneficial potential residents.
Materials and Methods
Strains and Media
All strains used in this study are in Table 2. The strains were grown in LB broth (Difco) or MSgg medium (Branda et al., 2001) [5 mM potassium phosphate, 100 mM MOPS (pH 7), 2 mM MgCl2, 50 µM MnCl2, 50 µM FeCl3, 700 µM CaCl2, 1 µM ZnCl2, 2 µM thiamine, 0.5% glycerol, 0.5% glutamate, 50 µg/ml threonine, tryptophan, and phenylalanine) (Branda et al., 2001]. Solid LB medium contained 1.5% bacto agar (Difco).
Plant Growth Conditions
Seeds of A. thaliana Col-0 were surface-sterilized and seeded on petri dishes containing Murashige and Skoog medium (4.4 g/L), pH 5.7, supplied with 0.5% (w/v) plant agar (Duchefa) and 0.5% sucrose (Sigma-Aldrich), and then stratified at 4°C for 2 days. The seeds were further transferred to a growth chamber (MRC) at 23°C in a 12-h light/12-h dark regime.
Extraction of Plant Secretions
Plant secretions were retrieved from 14-day-old A. thaliana seedlings cultured in 6 ml of liquid MSgg of each well of a six-well microplate (Thermo Scientific). Eight seedlings were put in each well. The plant secretions were collected after 4 days, filtered with a 0.22-µm filter, and stored at 4°C for further use.
Luminescence Experiments
Luminescence reporters were grown in either MSgg medium or MSgg medium containing plant secretions. Experiments were carried using a flat bottom 96-well plate with white opaque walls (Corning). Measurements were performed every 30 min at 30°C for a period for 24 h, using a microplate reader (Synergy 2; BioTek, Winooski, VT, USA). Luciferase activity was normalized to avoid artifacts related to differential cells numbers as RLU/OD.
Confocal Microscopy
Plants cultured with bacteria were washed in PBS and mounted on a microscope slide and covered with a poly-L-Lysine 31 (Sigma)-treated coverslip. Cells were visualized and photographed using a laser scanning confocal microscope (Zeiss LSM 780) equipped with a high-resolution microscopy Axiocam camera, as required. Data were captured using Zen black software (Zeiss, Oberkochen, Germany).
Flow Cytometry
Indicated strains used in the experiments were inoculated in 1.5 ml of liquid MSgg without seedlings (control) and MSgg with 14-day-old A. thaliana seedlings in a 24-well plate (Thermo Scientific); each well contained eight seedlings. The set was incubated for 24 h in a growth chamber (MRC) at 23°C in a 12-h light/12-h dark regime. After incubation, the seedlings were removed from the liquid medium and washed in PBS for the purpose of removing non-adherent bacteria. Samples were transferred to Eppendorf tube in 500 µl of PBS and vortexed for 1 min, for the purpose of detaching the bacteria from the root. Samples were measured using a BD LSR II flow cytometer (BD Biosciences), using laser excitation of 488 nm, coupled with 505 LP and 525/50 sequential filters. For each sample, 100,000 cells were counted and samples were analyzed using Diva 8 software (BD Biosciences).
Imaging Flow Cytometry
Samples were prepared as for the flow cytometer. Data were acquired by ImageStreamX Mark II (AMNIS, part of Luminex corp., Austin Tx) using a 60× lens (NA = 0.9). Lasers used were 488 nm (200 mW) for GFP excitation and 785 nm (5 mW) for side scatter measurement. During acquisition, bacterial cells were gated according to their area (in square microns) and side scatter, which excluded the calibration beads (that run in the instrument along with the sample). For each sample, 100,000 events were collected. Data were analyzed using IDEAS 6.3 (AMNIS). Single event bacteria were selected according to their area (in square microns) and aspect ratio (width divided by the length of a best-fit ellipse). Focused events were selected by the Gradient RMS and Contrast features (measures the sharpness quality of an image by detecting large changes of pixel values in the image). Cells expressing GFP were selected using the Intensity (the sum of the background subtracted pixel values within the image) and Max Pixel values (the largest value of the background-subtracted pixels) of the GFP channel (Ch02). GFP expression was quantified using the Mean Pixel feature (the mean of the background-subtracted pixels contained in the input mask). The size of bacteria was quantified using the Length feature (measures the longest part of an object, in microns) of the bright-field image.
Statistical Analysis
All experiments were performed three separate and independent times in triplicates. Datasets were compared using a standard two-way ANOVA, followed by Tukey’s multiple comparison post hoc testing, or a pairwise comparison using unpaired t-test with Welch’s correction in order to correct for groups with significantly unequal variances. Error bars represented ± SD, unless stated otherwise. p < 0.05 was considered statistically significant.
Statistical analyses were performed with GraphPad Prism 9.0 (GraphPad Software, Inc., San Diego, CA).
Data Availability Statement
The original contributions presented in the study are included in the article/Supplementary Material. Further inquiries can be directed to the corresponding authors.
Author Contributions
HM, OG, IK-G, and ZP designed the experiments. HM, OG, and ZP performed the experiments. HM, ZP, and IK-G analyzed the data. IK-G and ZP wrote the manuscript. All authors contributed to the article and approved the submitted version.
Funding
The IK-G laboratory is supported by the Israel Science Foundation grant number 119/16 and ISF-JSPS 184/20 and Israel Ministry of Science—Tashtiot (Infrastructures)—123402 in Life Sciences and Biomedical Sciences. IK-G is supported by an internal grant from the Estate of Albert Engleman provided by the Angel–Faivovich Fund for Ecological Research and by a research grant from the Benoziyo Endowment Fund for the Advancement of Science. IK-G is a recipient of the Rowland and Sylvia Career Development Chair.
Conflict of Interest
The authors declare that the research was conducted in the absence of any commercial or financial relationships that could be construed as a potential conflict of interest.
Publisher’s Note
All claims expressed in this article are solely those of the authors and do not necessarily represent those of their affiliated organizations, or those of the publisher, the editors and the reviewers. Any product that may be evaluated in this article, or claim that may be made by its manufacturer, is not guaranteed or endorsed by the publisher.
Supplementary Material
The Supplementary Material for this article can be found online at: https://www.frontiersin.org/articles/10.3389/fcimb.2021.722778/full#supplementary-material
Supplementary Figure S1 | Indicated reporter strains for PpenP-gfp and Pldh-gfp was analyzed by flow cytometry for (A) positively expressing fluorescent populations. Graphs represent mean ± SD or (B) the mean intensity of the fluorescent populations. Box and whisker plot shows median and interquartile range, together with the maximum and minimum values and outlier points. Reporter stains were either grown in MSgg medium or in MSgg medium in presence of A. thaliana seedlings. Data were collected from 24 h post inoculation, 100,000 cells were counted. Graphs represent results from two independent experiments with n = 2/experiment (total n = 6/group). Statistical analysis was performed using Two-way ANOVA followed by Tukey’s multiple comparison post hoc testing. p < 0.05 was considered statistically significant.
References
Adam, E., Groenenboom, A. E., Kurm, V., Rajewska, M., Schmidt, R., Tyc, O., et al. (2016). Controlling the Microbiome: Microhabitat Adjustments for Successful Biocontrol Strategies in Soil and Human Gut. Front. Microbiol. 7, 1079. doi: 10.3389/fmicb.2016.01079
Aleti, G., Sessitsch, A., Brader, G. (2015). Genome Mining: Prediction of Lipopeptides and Polyketides From Bacillus and Related Firmicutes. Comput. Struct. Biotechnol. J. 13, 192–203. doi: 10.1016/j.csbj.2015.03.003
Allaband, C., McDonald, D., Vazquez-Baeza, Y., Minich, J. J., Tripathi, A., Brenner, D. A., et al. (2019). Microbiome 101: Studying, Analyzing, and Interpreting Gut Microbiome Data for Clinicians. Clin. Gastroenterol. Hepatol. 17, 218–230. doi: 10.1016/j.cgh.2018.09.017
Allmansberger, R. (1997). Temporal Regulation of sigD From Bacillus Subtilis Depends on a Minor Promoter in Front of the Gene. J. Bacteriol. 179, 6531–6535. doi: 10.1128/jb.179.20.6531-6535.1997
Arnaouteli, S., Bamford, N. C., Stanley-Wall, N. R., Kovacs, A. T. (2021). Bacillus Subtilis Biofilm Formation and Social Interactions. Nat. Rev. Microbiol. 19 (9), 600–614. doi: 10.1038/s41579-021-00540-9
Auchtung, J. M., Lee, C. A., Grossman, A. D. (2006). Modulation of the ComA-Dependent Quorum Response in Bacillus Subtilis by Multiple Rap Proteins and Phr Peptides. J. Bacteriol. 188, 5273–5285. doi: 10.1128/JB.00300-06
Beauregard, P. B., Chai, Y., Vlamakis, H., Losick, R., Kolter, R. (2013). Bacillus Subtilis Biofilm Induction by Plant Polysaccharides. Proc. Natl. Acad. Sci. U. S. A. 110, E1621–E1630. doi: 10.1073/pnas.1218984110
Berg, G., Koberl, M., Rybakova, D., Muller, H., Grosch, R., Smalla, K. (2017). Plant Microbial Diversity Is Suggested as the Key to Future Biocontrol and Health Trends. FEMS Microbiol. Ecol. 93 (5). doi: 10.1093/femsec/fix050
Berg, G., Raaijmakers, J. M. (2018). Saving Seed Microbiomes. ISME J. 12, 1167–1170. doi: 10.1038/s41396-017-0028-2
Branda, S. S., Gonzalez-Pastor, J. E., Ben-Yehuda, S., Losick, R., Kolter, R. (2001). Fruiting Body Formation by Bacillus Subtilis. Proc. Natl. Acad. Sci. U. S. A. 98, 11621–11626. doi: 10.1073/pnas.191384198
Brinsmade, S. R. (2017). CodY, a Master Integrator of Metabolism and Virulence in Gram-Positive Bacteria. Curr. Genet. 63, 417–425. doi: 10.1007/s00294-016-0656-5
Bucher, T., Keren-Paz, A., Hausser, J., Olender, T., Cytryn, E., Kolodkin-Gal, I. (2019). An Active Beta-Lactamase Is a Part of an Orchestrated Cell Wall Stress Resistance Network of Bacillus Subtilis and Related Rhizosphere Species. Environ. Microbiol. 21 (3), 1068–1085. doi: 10.1111/1462-2920.14526
Butcher, R. A., Schroeder, F. C., Fischbach, M. A., Straight, P. D., Kolter, R., Walsh, C. T., et al. (2007). The Identification of Bacillaene, the Product of the PksX Megacomplex in Bacillus Subtilis. Proc. Natl. Acad. Sci. U. S. A. 104, 1506–1509. doi: 10.1073/pnas.0610503104
Caulier, S., Nannan, C., Gillis, A., Licciardi, F., Bragard, C., Mahillon, J. (2019). Overview of the Antimicrobial Compounds Produced by Members of the Bacillus Subtilis Group. Front. Microbiol. 10, 302. doi: 10.3389/fmicb.2019.00302
Chai, Y., Norman, T., Kolter, R., Losick, R. (2010). An Epigenetic Switch Governing Daughter Cell Separation in Bacillus Subtilis. Genes Dev. 24, 754–765. doi: 10.1101/gad.1915010
Chen, X. H., Scholz, R., Borriss, M., Junge, H., Mogel, G., Kunz, S., et al. (2009). Difficidin and Bacilysin Produced by Plant-Associated Bacillus Amyloliquefaciens Are Efficient in Controlling Fire Blight Disease. J. Biotechnol. 140, 38–44. doi: 10.1016/j.jbiotec.2008.10.015
Falardeau, J., Wise, C., Novitsky, L., Avis, T. J. (2013). Ecological and Mechanistic Insights Into the Direct and Indirect Antimicrobial Properties of Bacillus Subtilis Lipopeptides on Plant Pathogens. J. Chem. Ecol. 39, 869–878. doi: 10.1007/s10886-013-0319-7
Fan, B., Blom, J., Klenk, H. P., Borriss, R. (2017). Bacillus Amyloliquefaciens, Bacillus Velezensis, and Bacillus Siamensis Form an” Operational Group B. Amyloliquefaciens” Within the B-Subtilis Species Complex. Front. Microbiol. 8, 22. doi: 10.3389/fmicb.2017.00022
Fuangthong, M., Herbig, A. F., Bsat, N., Helmann, J. D. (2002). Regulation of the Bacillus Subtilis Fur and perR Genes by PerR: Not All Members of the PerR Regulon Are Peroxide Inducible. J. Bacteriol. 184, 3276–3286. doi: 10.1128/JB.184.12.3276-3286.2002
Gonzalez, D. J., Haste, N. M., Hollands, A., Fleming, T. C., Hamby, M., Pogliano, K., et al. (2011). Microbial Competition Between Bacillus Subtilis and Staphylococcus Aureus Monitored by Imaging Mass Spectrometry. Microbiology 157, 2485–2492. doi: 10.1099/mic.0.048736-0
Haldenwang, W. G. (1995). The Sigma Factors of Bacillus Subtilis. Microbiol. Rev. 59, 1–30. doi: 10.1128/mr.59.1.1-30.1995
Hassanov, T., Karunker, I., Steinberg, N., Erez, A., Kolodkin-Gal, I. (2018). Novel Antibiofilm Chemotherapies Target Nitrogen From Glutamate and Glutamine. Sci. Rep. 8, 7097. doi: 10.1038/s41598-018-25401-z
Herbig, A. F., Helmann, J. D. (2001). Roles of Metal Ions and Hydrogen Peroxide in Modulating the Interaction of the Bacillus Subtilis PerR Peroxide Regulon Repressor With Operator DNA. Mol. Microbiol. 41, 849–859. doi: 10.1046/j.1365-2958.2001.02543.x
Hernandez-Valdes, J. A., Zhou, L., de Vries, M. P., Kuipers, O. P. (2020). Impact of Spatial Proximity on Territoriality Among Human Skin Bacteria. NPJ Biofilms Microbiomes 6 (1), 30. doi: 10.1038/s41522-020-00140-0
Hou, Q., Kolodkin-Gal, I. (2020). Harvesting the Complex Pathways of Antibiotic Production and Resistance of Soil Bacilli for Optimizing Plant Microbiome. FEMS Microbiol. Ecol. 96 (9), fiaa142. doi: 10.1093/femsec/fiaa142
Inaoka, T., Takahashi, K., Ohnishi-Kameyama, M., Yoshida, M., Ochi, K. (2003). Guanine Nucleotides Guanosine 5’-Diphosphate 3’-Diphosphate and GTP Co-Operatively Regulate the Production of an Antibiotic Bacilysin in Bacillus Subtilis. J. Biol. Chem. 278, 2169–2176. doi: 10.1074/jbc.M208722200
Jousset, A., Rochat, L., Lanoue, A., Bonkowski, M., Keel, C., Scheu, S. (2011). Plants Respond to Pathogen Infection by Enhancing the Antifungal Gene Expression of Root-Associated Bacteria. Mol. Plant Microbe Interact. 24, 352–358. doi: 10.1094/MPMI-09-10-0208
Kallio, P. T., Fagelson, J. E., Hoch, J. A., Strauch, M. A. (1991). The Transition-State Regulator Hpr of Bacillus-Subtilis Is a DNA-Binding Protein. J. Biol. Chem. 266, 13411–13417. doi: 10.1016/S0021-9258(18)98855-1
Kinsella, K., Schulthess, C. P., Morris, T. F., Stuart, J. D. (2009). Rapid Quantification of Bacillus Subtilis Antibiotics in the Rhizosphere. Soil Biol. Biochem. 41, 374–379. doi: 10.1016/j.soilbio.2008.11.019
Kloepper, J. W., Ryu, C. M., Zhang, S. (2004). Induced Systemic Resistance and Promotion of Plant Growth by Bacillus Spp. Phytopathology 94, 1259–1266. doi: 10.1094/PHYTO.2004.94.11.1259
Kluge, B., Vater, J., Salnikow, J., Eckart, K. (1988). Studies on the Biosynthesis of Surfactin, a Lipopeptide Antibiotic From Bacillus-Subtilis Atcc-21332. FEBS Lett. 231, 107–110. doi: 10.1016/0014-5793(88)80712-9
Kunst, F., Msadek, T., Bignon, J., Rapoport, G. (1994). The DegS/DegU and ComP/ComA Two-Component Systems Are Part of a Network Controlling Degradative Enzyme Synthesis and Competence in Bacillus Subtilis. Res. Microbiol. 145, 393–402. doi: 10.1016/0923-2508(94)90087-6
Liu, H., Li, Y., Ge, K., Du, B., Liu, K., Wang, C., et al. (2021). Interactional Mechanisms of Paenibacillus Polymyxa SC2 and Pepper (Capsicum Annuum L.) Suggested by Transcriptomics. BMC Microbiol. 21, 70. doi: 10.1186/s12866-021-02132-2
Lopez, D., Vlamakis, H., Kolter, R. (2009). Generation of Multiple Cell Types in Bacillus Subtilis. FEMS Microbiol. Rev. 33, 152–163. doi: 10.1111/j.1574-6976.2008.00148.x
Maan, H., Friedman, J., Kolodkin-Gal, I. (2021). Resolving the Conflict Between Antibiotic Production and Rapid Growth by Recognition of Peptidoglycan of Susceptible Competitors bioRxiv 2021.2002.2007.430110.
Madar, D., Dekel, E., Bren, A., Zimmer, A., Porat, Z., Alon, U. (2013). Promoter Activity Dynamics in the Lag Phase of Escherichia Coli. BMC Syst. Biol. 7, 136. doi: 10.1186/1752-0509-7-136
Magnuson, R., Solomon, J., Grossman, A. D. (1994). Biochemical and Genetic Characterization of a Competence Pheromone From B. Subtilis. Cell 77, 207–216. doi: 10.1016/0092-8674(94)90313-1
Mariappan, A., Makarewicz, O., Chen, X. H., Borriss, R. (2012). Two-Component Response Regulator DegU Controls the Expression of Bacilysin in Plant-Growth-Promoting Bacterium Bacillus Amyloliquefaciens FZB42. J. Mol. Microbiol. Biotechnol. 22, 114–125. doi: 10.1159/000338804
McLoon, A. L., Kolodkin-Gal, I., Rubinstein, S. M., Kolter, R., Losick, R. (2011). Spatial Regulation of Histidine Kinases Governing Biofilm Formation in Bacillus Subtilis. J. Bacteriol. 193, 679–685. doi: 10.1128/JB.01186-10
Nagorska, K., Bikowski, M., Obuchowski, M. (2007). Multicellular Behaviour and Production of a Wide Variety of Toxic Substances Support Usage of Bacillus Subtilis as a Powerful Biocontrol Agent. Acta Biochim. Pol. 54, 495–508. doi: 10.18388/abp.2007_3224
Nakano, M. M., Xia, L. A., Zuber, P. (1991). Transcription Initiation Region of the Srfa Operon, Which Is Controlled by the Comp-Coma Signal Transduction System in Bacillus-Subtilis. J. Bacteriol. 173, 5487–5493. doi: 10.1128/jb.173.17.5487-5493.1991
Ngalimat, M. S., Yahaya, R. S. R., Baharudin, M. M. A., Yaminudin, S. M., Karim, M., Ahmad, S. A., et al. (2021). A Review on the Biotechnological Applications of the Operational Group Bacillus Amyloliquefaciens. Microorganisms 9 (3), 614. doi: 10.3390/microorganisms9030614
Ogran, A., Yardeni, E. H., Keren-Paz, A., Bucher, T., Jain, R., Gilhar, O., et al. (2019). The Plant Host Induces Antibiotic Production to Select the Most Beneficial Colonizers. Appl. Environ. Microbiol 85 (13). doi: 10.1128/AEM.00512-19
Ogura, M., Asai, K. (2016). Glucose Induces ECF Sigma Factor Genes, sigX and Sigm, Independent of Cognate Anti-Sigma Factors Through Acetylation of CshA in Bacillus Subtilis. Front. Microbiol. 7, 1918. doi: 10.3389/fmicb.2016.01918
Ongena, M., Jacques, P. (2008). Bacillus Lipopeptides: Versatile Weapons for Plant Disease Biocontrol. Trends Microbiol. 16, 115–125. doi: 10.1016/j.tim.2007.12.009
Rajavel, M., Mitra, A., Gopal, B. (2009). Role of Bacillus Subtilis BacB in the Synthesis of Bacilysin. J. Biol. Chem. 284, 31882–31892. doi: 10.1074/jbc.M109.014522
Rieusset, L., Rey, M., Gerin, F., Wisniewski-Dye, F., Prigent-Combaret, C., Comte, G. (2021). A Cross-Metabolomic Approach Shows That Wheat Interferes With Fluorescent Pseudomonas Physiology Through Its Root Metabolites. Metabolites 11 (2), 84. doi: 10.3390/metabo11020084
Roggiani, M., Dubnau, D. (1993). ComA, a Phosphorylated Response Regulator Protein of Bacillus Subtilis, Binds to the Promoter Region of srfA. J. Bacteriol. 175, 3182–3187. doi: 10.1128/jb.175.10.3182-3187.1993
Saujet, L., Monot, M., Dupuy, B., Soutourina, O., Martin-Verstraete, I. (2011). The Key Sigma Factor of Transition Phase, SigH, Controls Sporulation, Metabolism, and Virulence Factor Expression in Clostridium Difficile. J. Bacteriol. 193, 3186–3196. doi: 10.1128/JB.00272-11
Stein, T. (2005). Bacillus Subtilis Antibiotics: Structures, Syntheses and Specific Functions. Mol. Microbiol. 56, 845–857. doi: 10.1111/j.1365-2958.2005.04587.x
Steinke, K., Mohite, O. S., Weber, T., Kovacs, A. T. (2021). Phylogenetic Distribution of Secondary Metabolites in the Bacillus Subtilis Species Complex. mSystems 6 (2), e00057-21. doi: 10.1128/mSystems.00057-21
Straight, P. D., Fischbach, M. A., Walsh, C. T., Rudner, D. Z., Kolter, R. (2007). A Singular Enzymatic Megacomplex From Bacillus Subtilis. Proc. Natl. Acad. Sci. U. S. A. 104, 305–310. doi: 10.1073/pnas.0609073103
Strauch, M. A., Bobay, B. G., Cavanagh, J., Yao, F., Wilson, A., Le Breton, Y. (2007). Abh and AbrB Control of Bacillus Subtilis Antimicrobial Gene Expression. J. Bacteriol. 189, 7720–7732. doi: 10.1128/JB.01081-07
Takagi, M., Ohta, T., Johki, S., Imanaka, T. (1993). Characterization of the Membrane Sensor PenJ for Beta-Lactam Antibiotics From Bacillus Licheniformis by Amino Acid Substitution. FEMS Microbiol. Lett. 110, 127–131. doi: 10.1111/j.1574-6968.1993.tb06306.x
Therrien, C., Levesque, R. C. (2000). Molecular Basis of Antibiotic Resistance and Beta-Lactamase Inhibition by Mechanism-Based Inactivators: Perspectives and Future Directions. FEMS Microbiol. Rev. 24, 251–262. doi: 10.1016/S0168-6445(99)00039-X
Tian, T., Sun, B., Shi, H., Gao, T., He, Y., Li, Y., et al. (2021). Sucrose Triggers a Novel Signaling Cascade Promoting Bacillus Subtilis Rhizosphere Colonization. ISME J. 15, 2723–2737. doi: 10.1038/s41396-021-00966-2
Tojo, S., Satomura, T., Morisaki, K., Deutscher, J., Hirooka, K., Fujita, Y. (2005). Elaborate Transcription Regulation of the Bacillus Subtilis Ilv-Leu Operon Involved in the Biosynthesis of Branched-Chain Amino Acids Through Global Regulators of CcpA, CodY and TnrA. Mol. Microbiol. 56, 1560–1573. doi: 10.1111/j.1365-2958.2005.04635.x
Tsuge, K., Matsui, K., Itaya, M. (2007). Production of the non-Ribosomal Peptide Plipastatin in Bacillus Subtilis Regulated by Three Relevant Gene Blocks Assembled in a Single Movable DNA Segment. J. Biotechnol. 129, 592–603. doi: 10.1016/j.jbiotec.2007.01.033
Vargas-Bautista, C., Rahlwes, K., Straight, P. (2014). Bacterial Competition Reveals Differential Regulation of the Pks Genes by Bacillus Subtilis. J. Bacteriol. 196, 717–728. doi: 10.1128/JB.01022-13
Vlamakis, H., Aguilar, C., Losick, R., Kolter, R. (2008). Control of Cell Fate by the Formation of an Architecturally Complex Bacterial Community. Genes Dev. 22, 945–953. doi: 10.1101/gad.1645008
Walker, J. E., Abraham, E. P. (1970). The Structure of Bacilysin and Other Products of Bacillus Subtilis. Biochem. J. 118, 563–570. doi: 10.1042/bj1180563
Wood, H. E., Devine, K. M., McConnell, D. J. (1990). Characterisation of a Repressor Gene (Xre) and a Temperature-Sensitive Allele From the Bacillus Subtilis Prophage, PBSX. Gene 96, 83–88. doi: 10.1016/0378-1119(90)90344-Q
Xie, S., Wu, H., Chen, L., Zang, H., Xie, Y., Gao, X. (2015). Transcriptome Profiling of Bacillus Subtilis OKB105 in Response to Rice Seedlings. BMC Microbiol. 15, 21. doi: 10.1186/s12866-015-0353-4
Yaseen, Y., Gancel, F., Drider, D., Bechet, M., Jacques, P. (2016). Influence of Promoters on the Production of Fengycin in Bacillus Spp. Res. Microbiol. 167, 272–281. doi: 10.1016/j.resmic.2016.01.008
Keywords: flow cytometry, imaging, antibiotics, Bacillus, plant
Citation: Maan H, Gilhar O, Porat Z and Kolodkin-Gal I (2021) Bacillus subtilis Colonization of Arabidopsis thaliana Roots Induces Multiple Biosynthetic Clusters for Antibiotic Production. Front. Cell. Infect. Microbiol. 11:722778. doi: 10.3389/fcimb.2021.722778
Received: 09 June 2021; Accepted: 16 August 2021;
Published: 03 September 2021.
Edited by:
Xihui Shen, Northwest A and F University, ChinaReviewed by:
Mikael Lenz Strube, Technical University of Denmark, DenmarkTalia Karasov, The University of Utah, United States
Copyright © 2021 Maan, Gilhar, Porat and Kolodkin-Gal. This is an open-access article distributed under the terms of the Creative Commons Attribution License (CC BY). The use, distribution or reproduction in other forums is permitted, provided the original author(s) and the copyright owner(s) are credited and that the original publication in this journal is cited, in accordance with accepted academic practice. No use, distribution or reproduction is permitted which does not comply with these terms.
*Correspondence: Ziv Porat, ziv.porat@weizmann.ac.il; Ilana Kolodkin-Gal, Ilana.kolodkin-gal@weizmann.ac.il
†These authors have contributed equally to this work