Molecular Basis of the Slow Growth of Mycoplasma hominis on Different Energy Sources
- 1Department of Molecular Biology and Genetics, Federal Research and Clinical Center of Physical-Chemical Medicine, Federal Medical Biological Agency Malaya Pirogovskaya 1a, Moscow, Russia
- 2Department of Systems and Synthetic Biology, Scientific Research Institute for Systems Biology and Medicine Nauchniy proezd 18, Moscow, Russia
- 3Shemyakin-Ovchinnikov Institute of Bioorganic Chemistry, Russian Academy of Sciences Miklukho-Maklaya 16/10, Moscow, Russia
Mycoplasma hominis is an opportunistic urogenital pathogen in vertebrates. It is a non-glycolytic species that produces energy via arginine degradation. Among genital mycoplasmas, M. hominis is the most commonly reported to play a role in systemic infections and can persist in the host for a long time. However, it is unclear how M. hominis proceeds under arginine limitation. The recent metabolic reconstruction of M. hominis has demonstrated its ability to catabolize deoxyribose phosphate to produce ATP. In this study, we cultivated M. hominis on two different energy sources (arginine and thymidine) and demonstrated the differences in growth rate, antibiotic sensitivity, and biofilm formation. Using label-free quantitative proteomics, we compared the proteome of M. hominis under these conditions. A total of 466 proteins were identified from M. hominis, representing approximately 85% of the predicted proteome, while the levels of 94 proteins changed significantly. As expected, we observed changes in the levels of metabolic enzymes. The energy source strongly affects the synthesis of enzymes related to RNA modifications and ribosome assembly. The translocation of lipoproteins and other membrane-associated proteins was also impaired. Our study, the first global characterization of the proteomic switching of M. hominis in arginine-deficiency media, illustrates energy source-dependent control of pathogenicity factors and can help to determine the mechanisms underlying the interaction between the growth rate and fitness of genome-reduced bacteria.
Introduction
Pathogenic bacteria can infect and persist in their hosts for a long time. They have developed different ways to cope with adverse conditions and to be invisible to the host’s defense systems. Slow growth is one such strategy (Gray et al., 2019). Spreading inside the host, bacteria encounter different host environments with different nutrient availabilities. Some bacteria adapt their metabolic activity to host environments (Koczula et al., 2017) that allows them to occupy different niches.
M. hominis is a facultative-pathogenic cell wall-less bacterium that is found as a commensal bacterium in the urogenital tract but is also associated with pelvic inflammatory disease, bacterial vaginosis, and severe complications in pregnancy (Donders et al., 2017). The metabolic capabilities of M. hominis are reduced and seem to adapt to their natural niche. M. hominis is a non-glycolytic species due to the absence of 6-phosphofructokinase (Pfk) (Pereyre et al., 2009). This bacterium produces energy through arginine or dimethylarginine degradation by four enzymes. In the first step, arginine deiminase (ArcA) hydrolyzes arginine to citrulline and ammonia or N(G),N(G)-dimethylarginine dimethylaminohydrolase (DDAH) metabolizes dimethylarginine to citrulline and dimethylamine. In the second step, ornithine carbamoyltransferase (ArcB) converts citrulline to ornithine and carbamoyl phosphate in the presence of phosphate. The final step is the synthesis of ATP by carbamate kinase (ArcC) from carbamoyl phosphate and ADP (Pereyre et al., 2009). Free N(G),N(G)-dimethyl-L-arginine, like other arginine and lysine analogs, can be isolated from human urine. The concentration of N(G),N(G)-dimethyl-L-arginine in human urine is higher than that of arginine (Kakimoto and Akazawa, 1970). Recent data suggest that M. hominis can utilize ribose-phosphate and deoxyribose-phosphate, particularly thymidine, formed by nucleoside catabolism as an energy source. Generally, thymidine phosphorylase (DeoA) reversibly phosphorylates pyrimidine nucleosides to deoxyribose-1-phosphate and pyrimidine bases. Phosphopentomutase (DeoB) converts deoxyribose-1-phosphate to deoxyribose-5-phosphate that is further converted to acetaldehyde and glyceraldehyde 3-phosphate (G3P) by deoxyribose-phosphate aldolase (DeoC). G3P can undergo sequential enzymatic reactions by glyceraldehyde-3-phosphate dehydrogenase (Gap), phosphoglycerate kinase (Pgk), phosphoglucomutase (Pgm), enolase (Eno), pyruvate kinase (Pyk), and lactate dehydrogenase (Ldh) to form lactate, generating ATP as a by-product (Pereyre et al., 2009; Fisunov et al., 2022),. M. hominis has been reported to leave its natural niche and exists in various organs and tissues (Mian et al., 2005; Henao-Martínez et al., 2012; Gagneux-Brunon et al., 2015). Thus, the question arises: what changes do occur in M. hominis under arginine and its derivatives-restriction conditions and how does this affect its physiology? We hypothesized that changes in metabolism, primarily the ability to use deoxyribose as an energy source, may be involved in the adaptation to a new niche and proliferation of M. hominis.
In this study, we cultivated M. hominis on two different energy sources (arginine and thymidine) and demonstrated the differences in growth rate, antibiotic sensitivity, and biofilm formation. Using label-free quantitative proteomics, we identified proteins that were differentially expressed under these conditions. These proteins are likely to explain the phenotypic features of M. hominis grown on arginine and thymidine and reveal the molecular mechanisms underlying slow growth as a way to adapt to a new niche and spread.
Materials and Methods
Bacterial Strains and Growth Conditions
The M. hominis H34 strain was grown on Brain Heart Infusion medium (DIFCO, USA) supplemented with 10% horse serum (Biolot, Russia), 1% yeast extract (Helicon, Russia), and penicillin (Sintez, Russia) with a final concentration of 500 units/mL with the addition of 1% arginine or 20 mM thymidine as a carbon source. The cultures were incubated at 37 °C in aerobic condition. The growth curves were monitored through total amount of genomic DNA. The R package Growthcurver was used to fit the growth curve data to the logistic equation (Sprouffske and Wagner, 2016). The value of arguments (r, the growth rate) were extracted (Supplementary Table S1). The 0.002% (w/v) phenol red (Sigma-Aldrich, USA) was added in the culture medium to indirect detect of metabolic activity. A color change of medium was monitored using the OD at 560 nm (Cummings and McCormack, 1990).
DNA Extraction
The genomic DNA was isolated from 200 μL of bacterial cell suspension. M. hominis cells were harvested by centrifugation (12000 g for 10 min, 4 °C) and lyzed with CTAB buffer [2% CTAB, 100 mM Tris–HCl (pH 8.0), 20 mM EDTA, and 1.4 M NaCl] at 60 °C for 30 min with subsequent chloroform extraction (1:1) and isopropanol precipitation (1:1) with the addition of 10% v/v 3M sodium acetate (pH 5.2) (Stewart and Via, 1993). The pallets of nucleic acids were washed with 80% ethanol and finally resuspended in 22 µl of mQ (Panreac, Spain). The amount of DNA was determined using the Qubit 2.0 fluorometer (Thermo Fisher Scientific, USA).
Quantitative Real-Time PCR
Quantitative real-time PCR was performed using dNTP, PCR buffer, Taq-polymerase (Lytech, Russia), SYBR Green I (Invitrogen, USA), and CFX96 Real-Time PCR Detection System (Bio-Rad, USA) PCR machine. Primers for tuf amplification were used (tuf_mho_F: TATTGCTACGTGGAATTGACAG; tuf_mho_R: CCTTCACGAATAGAGAACTTGG). Primers were designed using BAC-Browser (Garanina et al., 2018). Each 20-µl reaction contained 1 µL of template gDNA. Thermal cycling conditions were as follows: initial denaturation at 95 °C for 1 min, then 40-cycle amplification (94 °C for 15 s, 58 °C for 20 s, and 68 °C for 40 s) with a single fluorescence per reading. Melting curve was obtained by gradually heating the PCR mixture from 65 to 92 °C at a rate of 0.5 °C every 5 s, with continuous fluorescence scanning. All PCR experiments were carried out in three replicates. The efficiency of the PCR amplification was determined for tuf primer pair. Standard curve was plotted for five twofold serial dilutions of gDNA. The slope of the standard curve was used to calculate the PCR efficiency. For tuf primer pair, PCR efficiency was 105%. Three technical repeats were used (Supplementary Table S2, Figure S2).
Plate Assay for Antibiotic Sensitivity Test
The following 4 antibiotics were used: tetracycline (Sigma-Aldrich, USA), ofloxacin (Sigma-Aldrich, USA), gentamicin (Dalhimpharm, Russia), and chloramphenicol (Sigma-Aldrich, USA). Stock solutions (20.0 μg/μL) of the antibiotics were prepared in the appropriate diluents and filter-sterilized using a 0.2-μm ABLUO® syringe-driven filter unit (GVS Filter Technology, USA). The aliquots (1 mL) of the stock solutions were stored at −20°C until use. The antibiotic sensitivity test for M. hominis was performed by the broth microdilution method with different antibiotic concentration ranges of 0.25, 1, 2.5, 5, 10, and 50 μg/mL. The third passage culture of M. hominis H34 grown in an appropriate medium was diluted with BHI broth supplemented with arginine or thymidine, as described above. The initial cells biomass calculated as amount of genomic DNA was equal for both conditions. In summary, 198 μL of culture was dispensed into the wells of sterile flat-bottom microtiter plates with lids (Corning Inc., USA), and 2.0 μL of the appropriate amount of the antibiotic solution was added. The culture without antibiotic solution (positive), the culture supplemented with diluents (mQ or 0.5% C2H5OH) (positive), and bacteria-free media (negative) were used as controls. The plates were incubated at 37°C for 30 or 48 h (until sufficient growth of the positive control samples was achieved). After that, 200 μL of culture from each well was transferred into tubes, gDNA was isolated and qPCR was performed as described above. All experiments were performed in triplicate.
Plate Assay for Biofilm Quantification
Biofilm density was measured using a crystal violet assay (McAuliffe et al., 2006). Cells were grown in 96-well plates for 7 days. Biofilms grown in microtiter plates were rinsed in PBS to remove non-adherent cells and stained with 0.5 % crystal violet solution for 30 min. Biofilms were then washed five times in distilled water before being left to dry at room temperature for at least 30 min. Crystal violet in stained biofilms in microtiter plates was solubilized by the addition of 200 μL 100 % ethanol. Biofilm production was quantified by measuring the absorbance (560 nm) of 100 μL of the solubilized crystal violet in a microplate. At least 24 wells were analyzed for each biofilm strain.
Protein Extraction and In-Solution Digestion
Protein samples were prepared as described previously (Semashko et al., 2020). Briefly, 10 mL of log-phase growing M. hominis H34 cells were collected by centrifugation at 12,000 × g at 4 °C for 10 min and washed twice with a cold PBS buffer. The cell pellets were resuspended in 10 μL of 10% sodium deoxycholate (DCNa) and a 0.5 μL of nuclease mix (GE Healthcare, USA) was added. After incubation for 1 h at 4 °C, the samples were resuspended in 100 µL of 100 mM Tris-HCl buffer (pH 8.0) containing 0.1% DCNa, 8 M urea, and 2.5 mM EDTA. The lysates were clarified by centrifugation at 16,000 × g for 10 min at 4 °C. The supernatants were saved, and the concentration was determined using a BCA Assay Kit (Sigma-Aldrich, USA). Two hundred micrograms of total protein per sample were used for peptide extract preparation. Disulfide bonds were reduced using 5 mM tris(2-carboxyethyl)phosphine hydrochloride (TCEP) (Sigma-Aldrich, USA) for 60 min at 37°C and alkylated with 30 mM chloroacetamide (Sigma-Aldrich, USA) for 30 min at room temperature in the dark. The sample was then diluted 6-fold with 50 mM Tris-HCl (pH 8.0) with 0.01% DCNa. Trypsin Gold (Promega, USA) was added to obtain the final trypsin:protein ratio of 1:50 (w/w) and incubated at 37 °C overnight. To stop digestion and degrade the acid-labile DCNa, trifluoroacetic acid (TFA) was added to a final concentration of 0.5% (v/v), incubated at 37 °C for 45 min, and the samples were centrifuged at 14,000 × g for 10 min to remove the DCNa. The peptide extract was desalted using a Discovery DSC-18 Tube (Supelco, USA) according to the manufacturer’s protocol. Peptides were eluted with 1 mL of 75% acetonitrile in water containing 0.1% TFA, dried in an Acid-Resistant CentriVap Benchtop Vacuum concentrator (Labconco, USA), and resuspended in 3% of acetonitrile in water containing 0.1% TFA to a final concentration of 5 μg/μL.
Liquid Chromatography-Tandem Mass Spectrometry (LC-MS/MS) Analysis
Peptide products were analyzed on an Ultimate 3000 RSLC nano HPLC system connected to a QExactive Plus mass spectrometer (Thermo Fisher Scientific, USA). Samples were loaded onto a home-made trap column 20 × 0.1 mm packed with Inertsil ODS3 3 μm sorbent (GL Sciences, Japan), in loading buffer (2% ACN, 98% H2O, 0.1% TFA) at 10 μL/min flow and separated at RT in a home-packed fused-silica column 500 × 0.1 mm packed with Reprosil PUR C18AQ 1.9 (Dr. Maisch, Germany) into the emitter prepared with a P2000 laser puller (Sutter, USA) (Kovalchuk et al., 2019). Samples were eluted with a linear gradient of 80% ACN, 19.9% H2O, 0.1% FA (buffer B) in 99.9% H2O, 0.1% FA (solvent A) from 4% to 36% of solvent B in 1 h at 0.44 μL/min flow at RT. MS data were collected in DDA mode. MS1 parameters were as follows: 70 K resolution, 350 - 2000 scan range, maximum injection time 50 ms, and AGC target 3 × 106. Ions were isolated with 1.4 m/z windows and 0.2 m/z offset targeting 10 highest intensity peaks of +2 to +6 charge, 8 × 103 minimum AGC, preferred peptide match, and isotope exclusion. Dynamic exclusion was set to 40 s. MS2 fragmentation was carried out in HCD mode at 17.5 K resolution with 27% NCE. Ions were accumulated for a maximum of 45 ms with a target AGC of 1 × 105.
Proteomic Data Analysis
MS data were analyzed using the PEAKS software (https://www.bioinfor.com/) with default settings. The data were searched against the M. hominis ATCC 23114 NCBI database and were deposited in the ProteomeXchange Consortium via the PRIDE partner repository with the dataset identifier PXD018714 and project 10.6019/PXD018714 (http://dx.doi.org/10.6019/PXD018714, https://www.ebi.ac.uk/pride/archive/projects/PXD018714). Three biological replicates per each condition were used. For statistical analysis, a t-test with Benjamini–Hochberg correction was conducted. The abundance of proteins with fold change ≥ 1.5 and adjusted p-value < 0.05 were regarded as significant. Full proteomic data description is found in (Semashko et al., 2020). Significantly changed proteins for M. hominis growing on thymidine compared to the arginine-grown culture are described in Supplementary Tables S3, S4). M. hominis ATCC 23114 with complete genome assembly was used as a reference, but it can limit identification results due to possible genomic differences between strains ATCC 23114 and H34.
In Silico Analysis
The available protein annotation for M. hominis ATCC 23114 is incomplete. The InterProScan software (Jones et al., 2014) was used to predict protein family membership and the presence of functional domains and sites. The analysis was run locally for all M. hominis ATCC 23114 protein sequences and the output results are available in Supplementary Table S5. Protein localization was predicted using PSORTb v3.0.2 (https://www.psort.org/psortb/), BUSCA (http://busca.biocomp.unibo.it/) (Savojardo et al., 2018), PRED-LIPO (http://www.compgen.org/tools/PRED-LIPO) (Bagos et al., 2008), LipoP 1.0 (http://www.cbs.dtu.dk/services/LipoP/) (Juncker et al., 2003), and SecretomeP 2.0a Server (http://www.cbs.dtu.dk/services/SecretomeP/) (Bendtsen et al., 2005). Programs for the prediction of signal peptides usually operate in two modes: gram-positive and gram-negative bacteria. Class Mollicutes is a specialized clade of Gram-positive bacteria but the signal peptides of mycoplasmas show closer sequence similarity to those of the Gram-negative bacteria (Song et al., 2009). We performed a search in both modes. Only the PSORTb v3.0.2 program proposes advanced options for the analysis of signal peptides in Mollicutes Supplementary Table S6. Transmembrane helices were predicted using the TMHMM server (Krogh et al., 2001).
Results and Discussion
Mycoplasma hominis Grown on Arginine and Thymidine Supplemented Medium Differ in the Carrying Capacity and the Growth Rate
To examine how the substitution of energy sources was reflected in changes in the growth rates of M. hominis, we evaluated its growth curves via total amount of genomic DNA at several time points (Figure 1A). We used the R package Growthcurver (Sprouffske and Wagner, 2016) to fit the growth curve data using the standard logistic equation (Verhulst equation) commonly applied in ecology and evolution (Rockwood, 2015), and the value of arguments was extracted (Supplementary Table S1, Figure S1). The growth rate of M. hominis grown on thymidine was reduced as compared to that on arginine, the carrying capacity was two-fold lower. Thus, we conclude that M. hominis grown on thymidine undergoes significant energy limitation and a general metabolic slowdown.
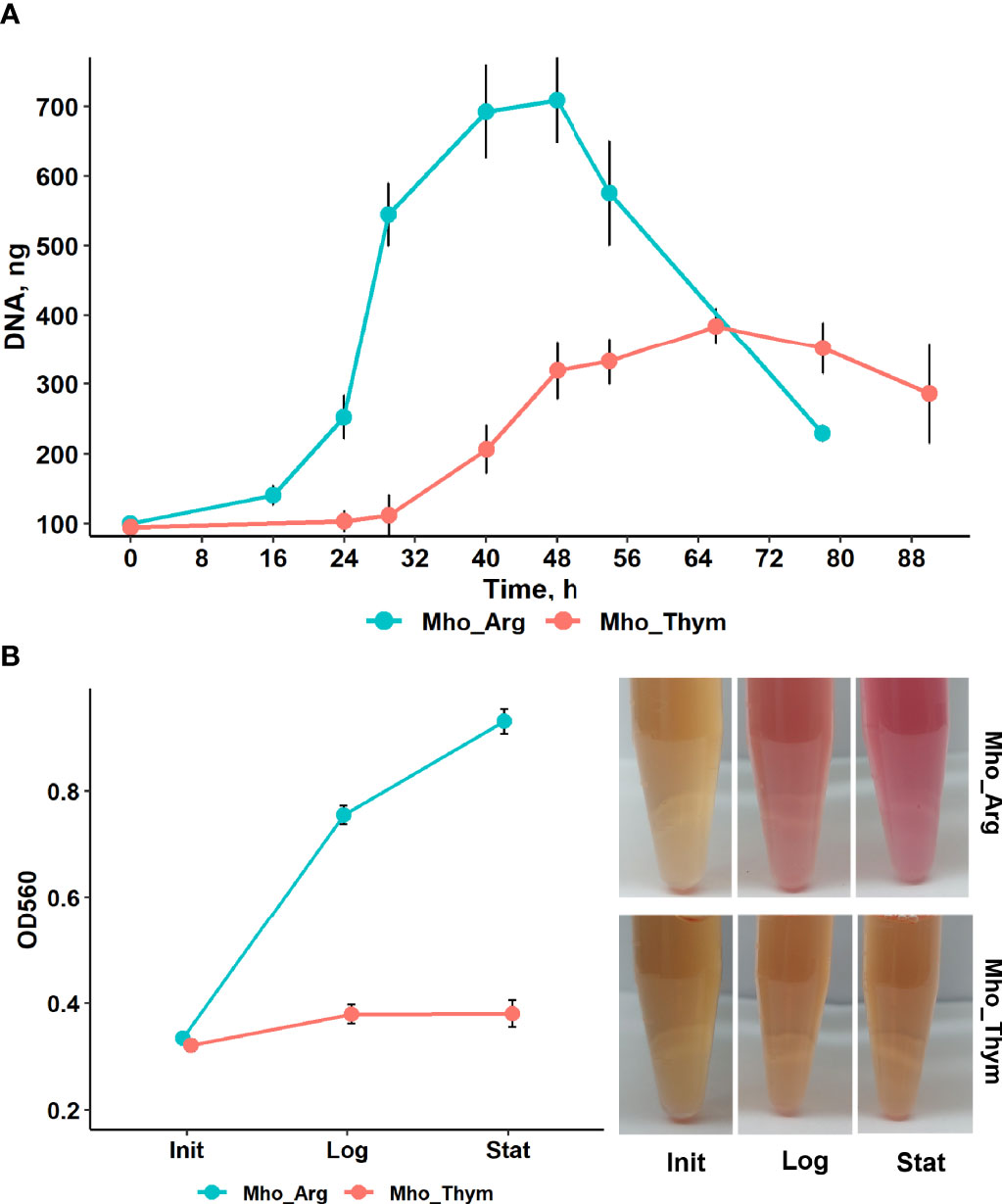
Figure 1 The growth curves of M. hominis on medium supplemented with arginine or thymidine. (A) Growth curves of M. hominis. All points are averages of triplicate experiments with standard deviation error bars (B) M. hominis was cultured in BHI media containing phenol red with arginine or thymidine. Arginine utilization and as a consequence production of ammonia was indicated by the color change of indicator to pink. The OD560 values were measured in three time point – after dilution of the third passage culture of M. hominis H34 in appropriate medium (Init), 0 h for both culture; in the mild-log phase (Log), 30 h for arginine supplemented culture, 42 h for thymidine; and in the stationary phase (Stat), 45 h and 65 h, respectively.
In our study we used Brain Heart Infusion (BHI) medium supplemented with horse serum, yeast extract and arginine or thymidine. There is some amount of arginine or arginine-containing peptides in BHI. M. hominis produces energy through arginine or dimethylarginine degradation with the production of ammonia that leads to elevation of the pH medium. To evaluate the activity of arginine deiminase pathway of M. hominis in medium supplemented with arginine or thymidine, a pH testing experiment was performed. We added pH indicator phenol red in the culture medium and measured OD at 560 nm during mycoplasma growth (Figure 1B). The value of OD560 in BHI media with arginine significantly increased as bacteria growth. No color change occurred when the medium was supplied with thymidine. This allowed us to assume that activity of arginine deiminase pathway was dramatically reduced or absent. M. hominis could be grown in BHI without addition arginine (Pereyre et al., 2009) or thymidine but it lost ability to grow to third passage (Fisunov et al., 2022). How long M. hominis can be passaged on thymidine-containing media was not determined.
The Growth Condition of M. hominis Influences Their Susceptibility to Antibiotics
We showed differences in the growth rates of M. hominis grown on different sources. For some bacteria, there is a direct correlation between growth rate and antibiotic efficiency (Lee et al., 2018). To examine this observation validity for M. hominis, we used the broth microdilution method with a wide range of antibiotics including tetracyclines (tetracycline), fluoroquinolones (ofloxacin), aminoglycosides (gentamicin), and amphenicols (chloramphenicol). Tetracyclines and fluoroquinolones are commonly used to treat M. hominis infections (Waites et al., 2005). A real-time PCR assay targeting M. hominis tuf (encodes elongation factor Tu) was performed to estimate bacteria viability. Mycoplasma under both growth conditions was equally resistant to chloramphenicol that inhibits protein biosynthesis by targeting the peptidyl transferase center on the large ribosomal subunit. In contrast, M. hominis was less susceptible to tetracycline that binds to the 16S rRNA at the 30S ribosomal subunit, and gentamicin. This effect was more pronounced for tetracycline (Figure 2). Comparation of melting curves for negative control samples and mycoplasma samples under the highest antibiotic concentration showed a peak of specific product (Tm=82°C) for mycoplasma samples and a lower temperature peak (Tm=75-77°C) for negative control samples (Supplementary Figure S3). This can be explained by the presence of dead or starved bacteria in the high-dose antibiotics treatment samples.
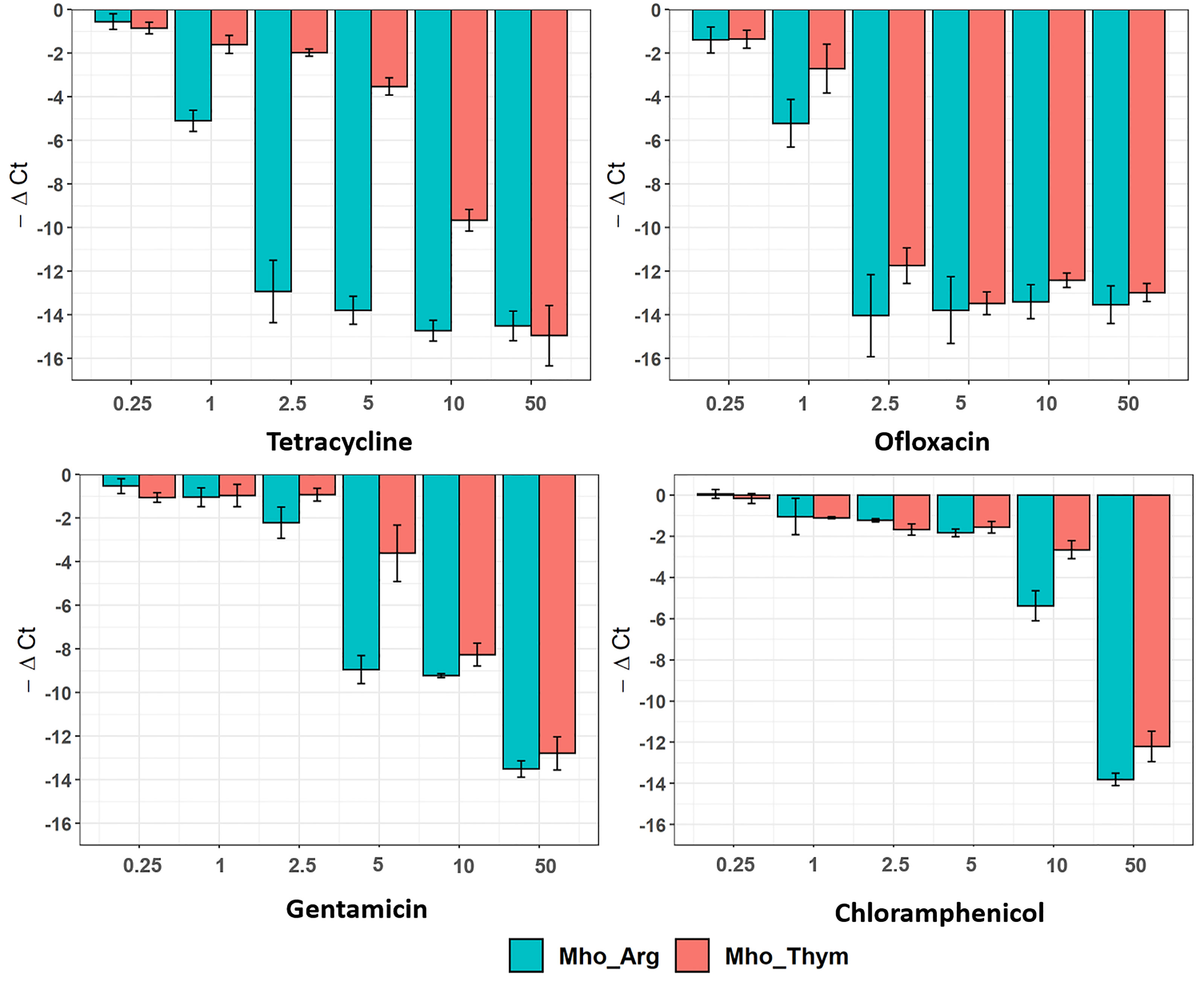
Figure 2 Susceptibility of M. hominis to antibiotics. M. hominis was cultivated on an arginine or thymidine-supplemented medium with different antibiotic concentration range: 0.25, 1, 2.5, 5, 10 and 50 μg/mL. Differences between Cq-values for antibiotic treatment samples and positive control samples without treatment are shown. All bar-plots are averages of triplicate experiments with standard deviation error bars.
All ribosome-acting antibiotics used in this study reversibly bind to ribosomes and impair different activities. Tetracycline competitively inhibits aminoacyl-tRNA binding to the A-site, chloramphenicol inhibits transpeptidase activity, and gentamicin decreases the ribosome’s decoding specificity that leads to the transfer of non-cognate aminoacyl-tRNAs to the nascent peptide chain. We hypothesize that the observed increase in tetracycline resistance during growth on thymidine may be explained as follows. The growth on thymidine leads to a drastic slowdown in the growth rate, as demonstrated above. A slower growth rate leads to slower metabolism and energy starvation. Tetracycline competes with aminoacyl-tRNAs for A-site binding. However, the binding is reversible and aminoacyl-tRNAs have a chance to enter the A-site as well, albeit at a lower rate. We hypothesize that the translation rate in M. hominis grown on thymidine is even slower (due to energy starvation) than the tetracycline-mediated slow-down at the given concentration. Thus, tetracycline-mediated slowdown is not a limiting step. For gentamycin, the error rate does not depend on the translation rate. At the same time, M. hominis already shows substantial resistance to chloramphenicol, and thus the effect of the slow-down translation rate may not be detectable on the background of the observed resistance. However, this assumption needs to be confirmed. Mutations or modifications of a drug target could also explain the observed effect. However, after transfer and passaging on medium with arginine, M. hominis grown on thymidine became sensitive again (data not shown). Other mechanisms that can ensure bacterial resistance to antibiotics are discussed in the context of proteomic changes below. A study performed on Gram-positive and Gram-negative bacteria demonstrated a direct relationship between bacterial metabolism and bactericidal antibiotic efficacy. Currently, energy metabolism is considered a novel target pathway in drug discovery (Bald et al., 2017; Lopatkin et al., 2019).
Effects of Energy Sources on Biofilm Formation Ability
The ability to form biofilms is an advantage for bacteria. Cells in biofilms have increased resistance to host defenses and resistance to stress (Costerton et al., 1999; McAuliffe et al., 2006), including antibiotics (Feng et al., 2020; Schulze et al., 2021),. In this study, we estimated the effects of the energy source on biofilm formation by M. hominis. M. hominis were grown for 7 days at 37 °C. Growth on thymidine significantly enhanced biofilm production compared to growth on arginine-supplemented medium based on the OD at 560 nm measurements of bound crystal violet (Figure 3). A previous study showed that the extent of biofilm formation is very diverse among mycoplasma species (McAuliffe et al., 2006). Our results indicate the ability of M. hominis H34 to form biofilms in vitro as measured by cell density, and that it can be stimulated by energy sources.
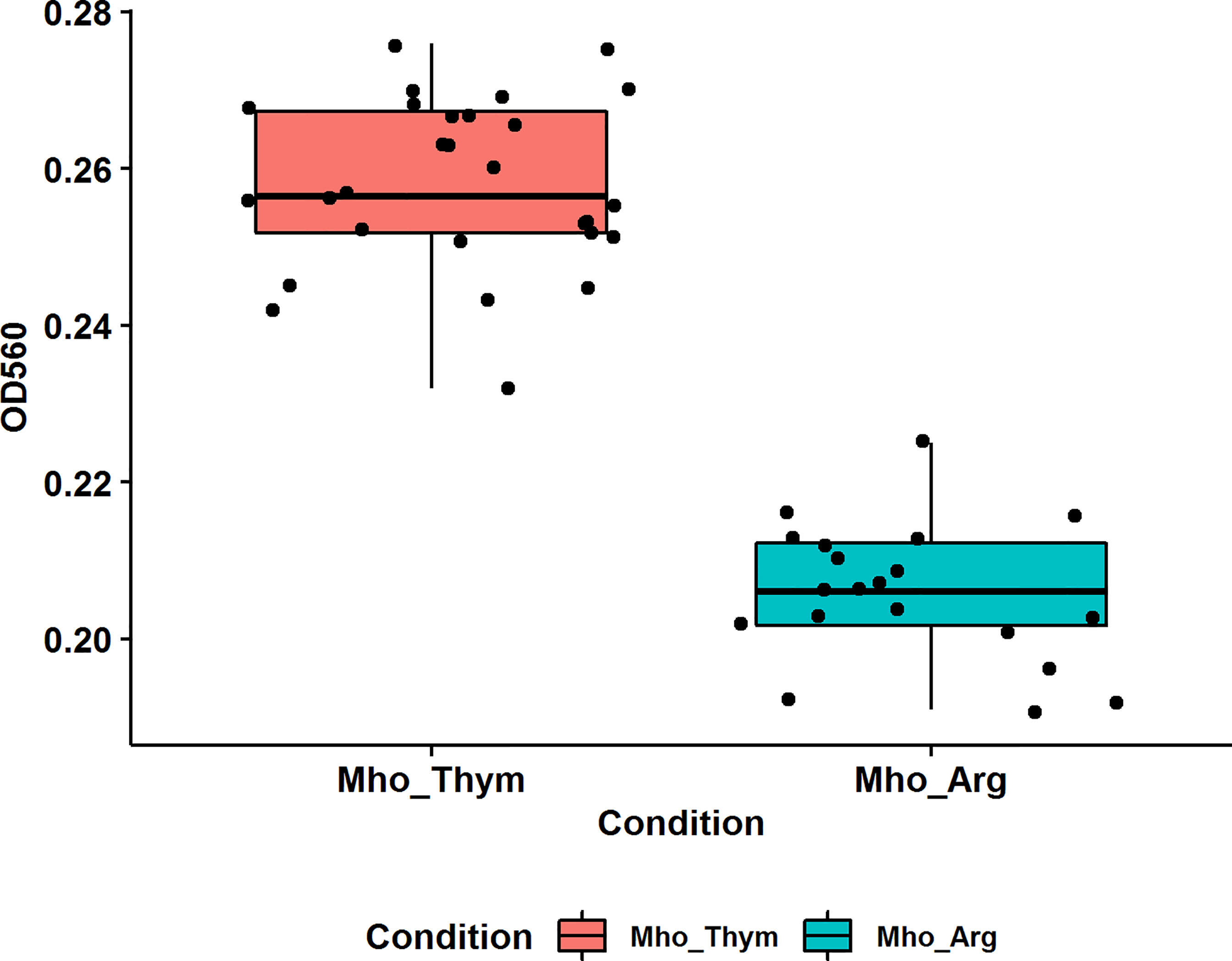
Figure 3 The effect of different carbon sources on M. hominis biofilm formation. Biofilm production was quantified by measuring the absorbance (560 nm) of crystal violet in a microplate. Groups are significantly different, pairwise comparisons using Wilcoxon rank sum test, p-value = 5e-09.
M. hominis Energy Metabolism Undergoes Rearrangement During Growth on Thymidine
It has been known that the major pathway of energy metabolism in M. hominis is arginine deamination (Pereyre et al., 2009). Previously, we performed the metabolic reconstruction of M. hominis and demonstrated that it is capable of growing on thymidine (Fisunov et al., 2022). We identified possible alternative pathways of energy metabolism: the utilization of ribose from ribonucleosides and the utilization of deoxyribose from deoxyribonucleosides. The alternative pathways start from the cleavage of nucleosides into nucleobases and phosphosugars. Phosphosugars are then processed to glyceraldehyde-3-phosphate that is utilized by the enzymes of lower glycolysis.
To study the adaptation of M. hominis to growth on thymidine, we performed quantitative proteome analysis. Cells grown on arginine were used as the control. A total of 466 proteins were identified from M. hominis, representing approximately 85% of the predicted proteome. The abundance of 23 proteins was significantly increased and 71 decreased (adjusted p-value < 0.05) during growth on thymidine compared to growth on arginine. All proteins from the arginine deiminase pathway were identified, but the differential expression of only carbamate kinase (ArcC) changed slightly (Figure 4). The abundance of enzymes involved in the utilization of nucleosides including thymidine phosphorylase (DeoA), lactate dehydrogenase (Ldh), glyceraldehyde-3-phosphate dehydrogenase (Gap), and pyruvate kinase (Pyk) was significantly increased. Thus, we conclude that M. hominis rearranges its energy metabolism toward the utilization of nucleosides.
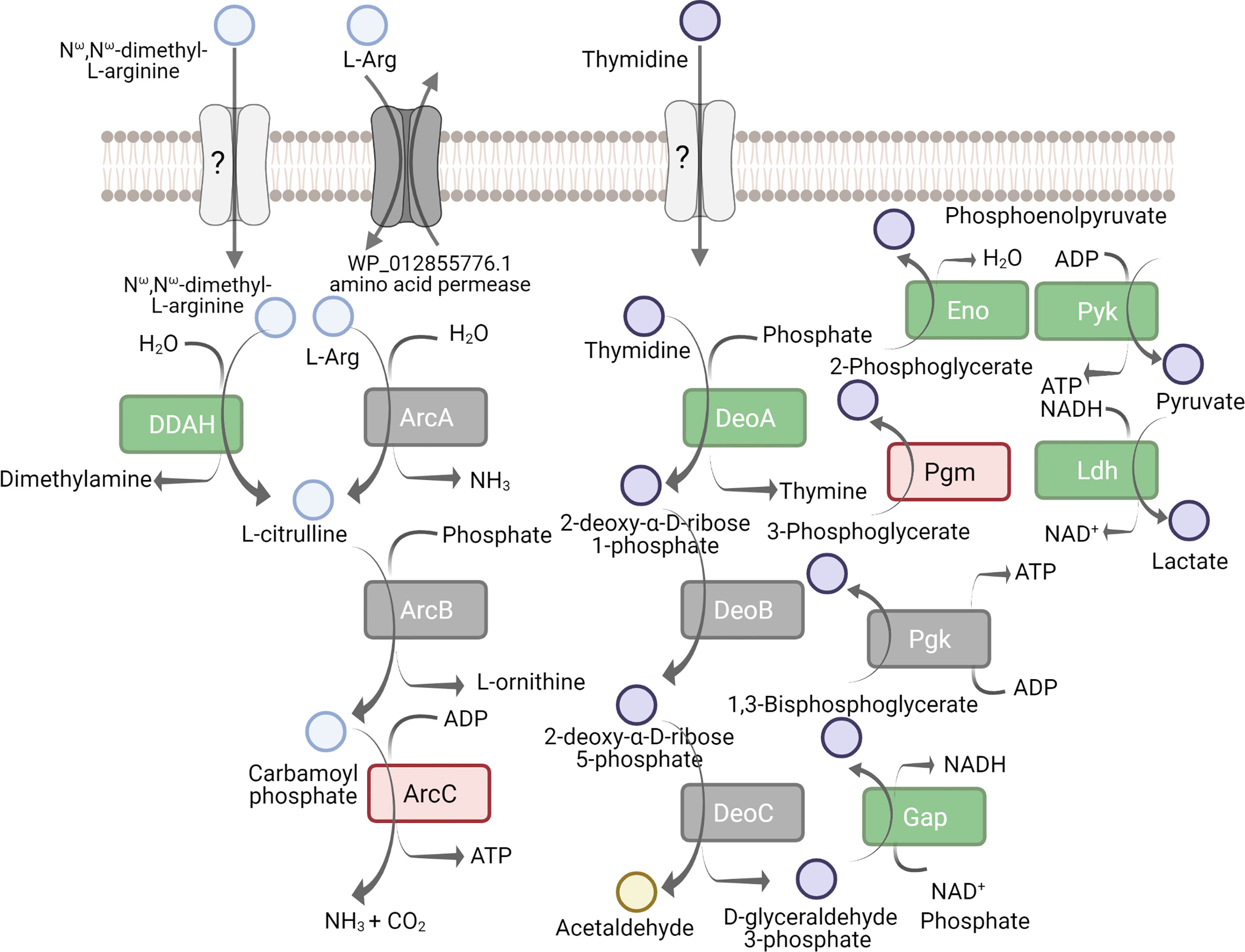
Figure 4 Proteins implicated in the arginine deiminase pathway and utilization of pyrimidine deoxynucleoside as energy source. Comparative proteomic analysis of M. hominis grown on thymidine-supplemented medium relative to arginine-supplemented medium. The down-regulated proteins are indicated red, up-regulated - in green, unchanged - in grey. Unknown proteins are in light grey. The full names of proteins are given in Supplementary Tables S3, S4.
The Energy Source Strongly Affects the Synthesis of Enzymes Related to RNA Modifications and Ribosome Assembly
The switch of the energy source to thymidine resulted in numerous changes in the translation machinery of M. hominis. The abundance of the eight proteins involved in rRNA modification decreased (Figure 5). The number of enzymes that catalyze rRNA modification varies across bacteria. Mycoplasmas feature on average 10 enzymes of this function (De Crécy-Lagard et al., 2007). The modification targets are concentrated around the A and P sites, the peptidyl transferase center, the peptide exit tunnel, and on both sides of the intersubunit bridges (Sergiev et al., 2011). Numerous studies have shown that the loss of rRNA modifications results in the alteration of the structures of the active sites (Desaulniers et al., 2008; Demirci et al., 2010), that causes slower rates and lower accuracy of translation (Baxter-Roshek et al., 2007; Liang et al., 2007), as well as impaired responses to metabolites and antibiotics (Helser et al., 1971; Doi and Arakawa, 2007).
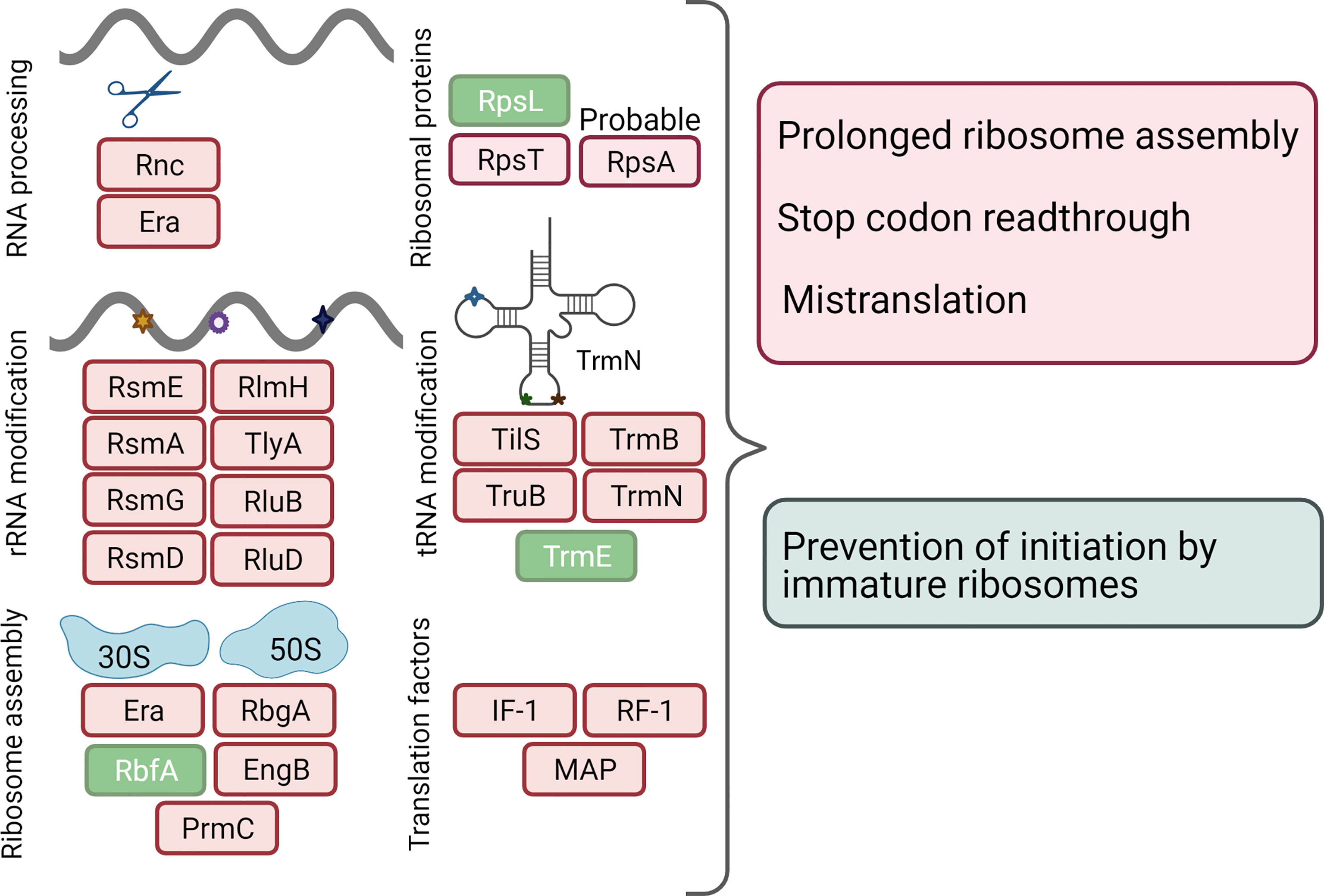
Figure 5 Response of translation apparatus to a change in the energy source. The down-regulated proteins are indicated in red, up-regulated - in green. The corresponding full names of proteins are given in Supplementary Tables S3, S4.
Among the proteins that are involved in the modification of tRNAs, four were down-regulated and only one, TrmE/MnmE (WP_012855730.1) was upregulated (Figure 5). tRNA modifications can occur in various positions. The number of modified nucleosides varies in different organisms from 2 to 30 - 50. It is believed that the modified nucleosides in and near the anticodon stem-loop (especially 34 and 37 nucleosides) can have critical roles, expanding or restricting the decoding properties of a given tRNA molecule; modifications outside the anticodon stem-loop have more structural roles (de Crécy-Lagard and Jaroch, 2021). In bacteria, the elimination of several anticodon stem-loop modifications gives rise to pleiotropic phenotypes. Moreover, recent studies have demonstrated that tRNA modifications in bacteria act as quality control signals, as in eukaryotes; the absence of some modifications leads to tRNA degradation (Kimura and Waldor, 2019). The upregulation of MnmE was surprising considering the downregulation of the majority of other modifying enzymes. MnmE is a multi-domain GTPase that is highly conserved among bacteria and eukaryotes (Armengod et al., 2012). Deletion of mnmE and its partner mnmG genes has been shown to have pleiotropic effects on growth, cell division, and virulence in a wide range of pathogenic bacteria, both gram-positive and gram-negative (Shippy and Fadl, 2014; Gao et al., 2019). However, all studies are rather phenomenological in nature; the mechanisms underlying the selective response of cells upon mnmE inactivation remain unknown.
In addition to modifications, the precursors of rRNAs and tRNAs undergo a processing stage catalyzed by endonucleases and exonucleases. We found that RNase III and the GTPase Era required for the maturation of ribosomal and other structural RNAs were downregulated by thymidine. In Escherichia coli, the rnc and era genes are transcribed from the same operon; moreover, their translation is coupled to ensure similar levels of expression (Britton et al., 1998). In the genome of M. hominis, era gene is far from rnc but its expression is apparently synchronized. A reduction in E. coli wild‐type era expression or reduced GTPase activity temporarily arrests cell growth at the two‐cell stages and delays cell division (Britton et al., 1998). The abundance of GTPases RbgA and EngB that are involved in ribosome biogenesis were also reduced during growth on thymidine. At the same time, 30S subunit-binding factor RbfA was upregulated. This protein is required for the efficient processing of the 5′- end of the 16S rRNA (Goto et al., 2011). A recent study demonstrated the unique ability of RbfA to suppress protein synthesis by immature E. coli 30S subunits, providing a quality control mechanism (Sharma and Woodson, 2020).
Growth on thymidine resulted in the downregulation of the protein modification enzyme PrmC (HemK). Among post-translational protein modification enzymes, only HemK is conserved in Mollicutes (Grosjean et al., 2014). This enzyme methylates the termination factor RF-1 (prfA) which is also downregulated during growth on thymidine. In M. hominis and E. coli, the initiation codon of hemK overlaps with the termination codon of prfA, suggesting that the synthesis of the two genes is coupled (Pierson et al., 2016). It has been shown that hemK knockouts of E. coli K12 grow very slowly and translational read-through at the UAG codon recognized by RF-1 is increased. This could be due to the prolonged pause of the ribosome at the stop codons (Nakahigashi et al., 2002). In Mollicutes (except phytoplasmas and Acholeplasma laidlawii), another release factor (RF-2) is missed; therefore, a decrease in the levels of HemK and PrfA can have a stronger effect on translation in M. hominis than in E. coli.
Finally, WP_012855634.1 is another interesting protein whose level has decreased significantly with thymidine. This is a short protein (111 amino acids) annotated as hypothetical but containing an S1 RNA-binding structural domain. In some studies, this protein is considered to be ribosomal protein S1 (RpsA) that is lost in most Mollicutes and remains in several species of the Hominis subgroup (Grosjean et al., 2014). The well-studied bacterial 30S ribosomal protein S1 from E. coli (RpsA) has six S1 domains, while WP_012855634.1 has only one (Machulin et al., 2019). In E. coli, ribosomal protein S1 endows the 30S subunit with an RNA chaperone activity that is essential for the binding and unfolding of structured mRNAs, allowing the correct positioning of the initiation codon for translation (Duval et al., 2013). It remains unclear whether WP_012855634.1 has a function similar to that of RpsA from E. coli. Interestingly, the gene encoding WP_012855634.1, located upstream of gpi (glucose-6-phosphate isomerase) and ldh (lactate dehydrogenase) genes, and the end of possible rpsA M. hominis, intersects the start of the gpi gene. In prokaryotes, functionally related genes or their regulators are often organized into operons or colocalized in space that ensures the synchronization of expression regulation (Rogozin, 2002; Llopis et al., 2010). WP_012855634.1 can likely play a role in the connection between central metabolism and post-transcriptional regulation of gene expression in M. hominis.
To summarize, the growth of M. hominis in the presence of thymidine leads to the downregulation of multiple proteins that enhance ribosome processivity or fidelity. We propose that this may lead to slower or less accurate translations in general. The latter may include pausing, stop-codon read-through, and mistranslation. These events may lead to general growth retardation. On the other hand, an increased level of chaperone ClpB rescues proteins from an aggregated state.
Non-Cytoplasmic Proteins Are Significantly Changed Under Thymidine Condition
In M. hominis grown on thymidine, we detected a reduced level of two proteins from the translocation system: signal recognition particle protein (Ffh) and signal peptidase II (LspA). Signal peptidase II (LspA) plays a crucial role in the subcellular localization and export of lipid-modified bacterial proteins (Vogeley et al., 2016). Moreover, signal peptidase I that is responsible for generating mature non-lipoproteins is not found in most Mycoplasma species (excluding avian pathogens), but some authors have observed its activity (Catrein et al., 2005). Ffh and 4.5S RNA form a signal recognition particle (SRP) that provides a translationally coupled mechanism of protein translocation (Akopian et al., 2013). Mycoplasmas are limited in the pathways of protein targeting and translocation. The change in the level of two important components of protein export prompted us to test the localization of up- and down-regulated proteins. We found membrane-associated proteins in the both groups. There were more of such proteins in the group of down-regulated proteins and the fold change value was greater. Most of the predicted membrane-associated proteins (Figure 6) do not have assigned annotations (Supplementary Tables S3, S4), but have lipobox, a conserved sequence at the C-region of the signal peptides with cysteine residue required for further modification and anchoring in the membrane (Kovacs-Simon et al., 2011) (Supplementary Table S6).
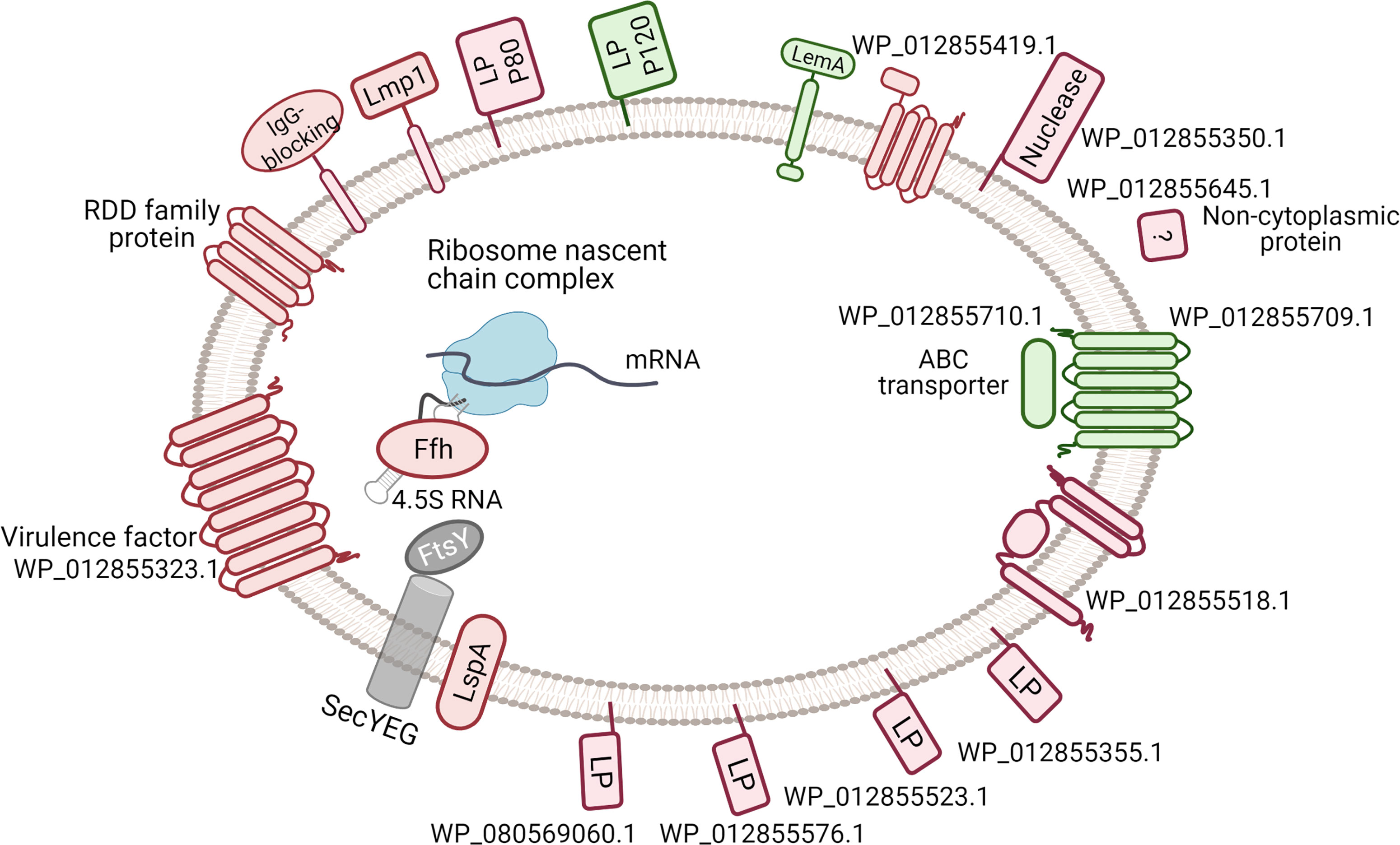
Figure 6 Difference in the membrane-associated proteins under thymidine conditions comparing with arginine conditions. The down-regulated proteins are indicated red, up-regulated - in green, unchanged - in grey. The data about signal peptides, Lipo-box, transmembrane helices and functional domains was used in protein diagramming. The SRP-mediated protein targeting is shown. LP - lipoprotein. Only two significantly changed transmembrane proteins are not shown: PotC and PepF. The corresponding full names of proteins are given in Supplementary Tables S3, S4.
In bacteria, particularly pathogens, lipoproteins play key roles in adhesion to host cells and modulate immune responses (Christodoulides et al., 2018). The lipoprotein variations provide advantages of phenotypic plasticity of mycoplasmas, allowing survival and persistence within the host (van der Woude and Bäumler, 2004). The major lipoprotein Vaa did not change in our experiments. Among membrane-associated proteins, we discovered integral proteins that can be part of the signal transduction pathway, virulence, or transport. In the up-regulated group, hypothetical protein WP_012855709.1 has a primary structure similar to the ABC transporter permease, six transmembrane helices can be predicted (Supplementary Figure S4), and its neighbor WP_012855710.1 (upregulated too) is annotated as an ABC transporter ATP-binding protein, but it is localized in the cytoplasm. The level of conserved LemA protein (WP_012855649.1) was slightly increased on thymidine. This protein has a large internal fragment and a small extracellular amino terminus (Supplementary Figure S5). The molecular function of LemA remains unknown. Among the down-regulated proteins, lipid-anchored WP_012855350.1 unique for M. hominis and two Ureaplasma species membrane protein WP_012855645.1, decreased by 11- and 6-fold, respectively, in the thymidine condition. Recently, it was shown that protein WP_012855350.1 (gene name MHO_0730) is a surface-exposed nuclease that promotes neutrophil extracellular trap escape (Cacciotto et al., 2019). There were no functional domains in the WP_012855350 protein and only two coiled coil regions were predicted using the InterProScan software (Supplementary Table S4). Eight transmembrane helices containing protein WP_012855323.1, related to the YihY/virulence factor BrkB family, were also down-regulated on thymidine and integral membrane proteins WP_012855330.1 (Lmp1), WP_012855725.1 (RDD family protein), and WP_012855518.1(hypothetical protein). Changes in proteins associated with virulence suggest the importance of an energy source for M. hominis survival in an aggressive environment and spread in the host.
In our study, we have shown that the abundance of membrane-associated proteins changes during growth on thymidine. However, the functions of most proteins remain unclear. The role of surface and membrane proteins is enormous for a pathogen that loses its cell wall. In the future, to elucidate the mechanisms of pathogenicity and host-microbe communication, it will be necessary to conduct a deep analysis of the described proteins.
We described two major groups of proteomic changes for M. hominis grown on thymidine. As we demonstrated above, such cells had different susceptibility to antibiotics, especially to tetracycline. Decreased level of proteins for specifical modification of 16S rRNA could reduce tetracycline affinity to binding site (Schedlbauer et al., 2015; Grossman, 2016). An active efflux and reduced uptake of antibiotics is another mechanism of resistance (Reygaert, 2018). A changed level of ABC-transporters like PotC and WP_012855709.1 could influence drug availability but specificity of these proteins should be verified.
Possible Modulators of Described Proteomic Effect
Under thymidine conditions, we detected an increased level of serine/threonine-protein phosphatase (WP_012855759.1), similar to PrpC (Supplementary Figure S6). In Mycoplasma pneumonia, as well as M. hominis and gram-positive bacteria, the prpC gene is downstream of prkC that encodes another serine/threonine protein kinase. Inactivation of prkC in M. pneumonia leads to a non-adherent, low-cytotoxicity phenotype. PrkC-dependent phosphorylation of large cytadherence proteins is required for the stability of these complexes (Schmidl et al., 2010). PrpC is thought to be an antagonist of PrkC (Gaidenko et al., 2002). Therefore, the decreased levels of some membrane-associated proteins can potentially be explained by a reduction in PrpC abundance. Moreover, the stationary-phase cell density of the prpC-null mutant Bacillus subtilis was significantly higher than that of the wild type (Gaidenko et al., 2002); therefore, PrpC could be a participant in cell-cell communication. It seems surprising that the abundance of many lipoproteins decreases and only a few increases in M. hominis grown on thymidine, while biofilm formation is induced under such conditions. For Mycoplasma bovis, it has been shown that different patterns of variable membrane surface lipoproteins may display different adhesion capabilities, resulting in the ability to form biofilms (McAuliffe et al., 2006; Bürki et al., 2015).
The abundance of DUF448 domain-containing protein WP_012855794.1 decreased four-fold in M. hominis grown on thymidine compared to arginine. This protein is similar to YlxR (RulR) from B. subtilis. YlxR is widely conserved in eubacteria, but its function remains elusive. Recent studies on B. subtilis showed that YlxR is a nucleoid-associated protein (Ogura and Kanesaki, 2018); it is involved in glucose-responsive metabolic changes (Ogura et al., 2019). M. hominis and some other members of class Mollicutes have lost some enzymes for glucose utilization (Pereyre et al., 2009). We suppose that YlxR is capable of performing a regulatory function in response to the energy state of the cell regardless of the availability of glucose.
Conclusion
In summary, the growth of M. hominis on thymidine-supplemented medium led to a significant difference in growth rate compared to bacteria cultivated on arginine. This slow-growth phenotype of bacteria demonstrates reduced susceptibility to some antibiotics and an increased extent of biofilm formation. Our comparative proteomic analysis of M. hominis grown on different energy sources confirmed metabolic rearrangement and allowed us to identify two major groups of differentially expressed proteins: translation-related and membrane-associated proteins, and their translocation. The first one is responsible for a slower or less accurate translation, and the second one is the variation of immunogenic proteins on the cell surface and virulence. These results may help uncover the molecular basis of the slow bacterial growth phenotype and improve the strategies for developing novel antimicrobial agents for combating mycoplasmosis. Future studies should focus on identifying the effector molecules that provide signal transduction and regulation of M. hominis switching to a slow-growth phenotype. In parallel, the elucidation of the exact function of membrane-associated proteins could cover a blind spot in the physiology and pathogenicity of genome-reduced bacteria.
Data Availability Statement
The datasets presented in this study can be found in online repositories. The names of the repository/repositories and accession number(s) can be found in the article/Supplementary Material.
Author Contributions
DE contributed to conception and design of the study, worked with nucleic acid, wrote the manuscript. TS contributed to conception and design of the study, analysed proteomic data. VL carried out cultivation of bacteria. MG performed biofilm assay. SK and RZ performed mass-spectrometry analysis. GF contributed to conception of the study, wrote section of the manuscript. OP contributed to conception of the study, prepared samples for proteomic analysis. All authors contributed to manuscript revision, read, and approved the submitted version.
Funding
This work was supported by the Russian Science Foundation (project number 19-75-10124).
Conflict of Interest
The authors declare that the research was conducted in the absence of any commercial or financial relationships that could be construed as a potential conflict of interest.
Publisher’s Note
All claims expressed in this article are solely those of the authors and do not necessarily represent those of their affiliated organizations, or those of the publisher, the editors and the reviewers. Any product that may be evaluated in this article, or claim that may be made by its manufacturer, is not guaranteed or endorsed by the publisher.
Supplementary Material
The Supplementary Material for this article can be found online at: https://www.frontiersin.org/articles/10.3389/fcimb.2022.918557/full#supplementary-material
References
Akopian, D., Shen, K., Zhang, X., Shan, S. O. (2013). Signal Recognition Particle: An Essential Protein-Targeting Machine [Internet]. Vol. 82, Annual Review of Biochemistry. Annu. Rev. Biochem. 82, 693–721. doi: 10.1146/annurev-biochem-072711-164732
Armengod, M. E., Moukadiri, I., Prado, S., Ruiz-Partida, R., Benítez-Páez, A., Villarroya, M., et al. (2012). Enzymology of tRNA Modification in the Bacterial MnmEG Pathway. Biochimie. Elsevier Vol. 94, 1510–1520. doi: 10.1016/j.biochi.2012.02.019
Bagos, P. G., Tsirigos, K. D., Liakopoulos, T. D., Hamodrakas, S. J. (2008). Prediction of Lipoprotein Signal Peptides in Gram-Positive Bacteria With a Hidden Markov Model. J. Proteome Res. 7 (12), 5082–5093. doi: 10.1021/pr800162c
Bald, D., Villellas, C., Lu, P., Koul, A. (2017). Targeting Energy Metabolism in Mycobacterium Tuberculosis , a New Paradigm in Antimycobacterial Drug Discovery. MBio 8 (2), 1–11. doi: 10.1128/mBio.00272-17
Baxter-Roshek, J. L., Petrov, A. N., Dinman, J. D. (2007). Optimization of Ribosome Structure and Function by rRNA Base Modification. PLoS One 2 (1), e174. doi: 10.1371/journal.pone.0000174
Bendtsen, J. D., Kiemer, L., Fausbøll, A., Brunak, S. (2005). Non-Classical Protein Secretion in Bacteria. BMC Microbiol. 5, 1–13. doi: 10.1186/1471-2180-5-58
Britton, R. A., Powell, B. S., Dasgupta, S., Sun, Q., Margolin, W., Lupski, J. R., et al. (1998). Cell Cycle Arrest in Era GTPase Mutants: A Potential Growth Rate-Regulated Checkpoint in Escherichia Coli. Mol. Microbiol. 27 (4), 739–750. doi: 10.1046/j.1365-2958.1998.00719.x
Bürki, S., Frey, J., Pilo, P. (2015). Virulence, Persistence and Dissemination of Mycoplasma Bovis. Vet. Microbiol. 179 (1–2), 15–22. doi: 10.1016/j.vetmic.2015.02.024
Cacciotto, C., Dessì, D., Cubeddu, T., Cocco, A. R., Pisano, A., Tore, G., et al. (2019). MHO-0730 as a Surface-Exposed Calcium-Dependent Nuclease of Mycoplasma Hominis Promoting Neutrophil Extracellular Trap Formation and Escape. J. Infect. Dis. 220 (12), 1999–2008. doi: 10.1093/infdis/jiz406
Catrein, I., Herrmann, R., Bosserhoff, A., Ruppert, T. (2005). Experimental Proof for a Signal Peptidase I Like Activity in Mycoplasma Pneumoniae, But Absence of a Gene Encoding a Conserved Bacterial Type I SPase. FEBS J. 272 (11), 2892–2900. doi: 10.1111/j.1742-4658.2005.04710.x
Christodoulides, A., Gupta, N., Yacoubian, V., Maithel, N., Parker, J., Kelesidis, T. (2018). The Role of Lipoproteins in Mycoplasma-Mediated Immunomodulation. Front. Microbiol. 9. doi: 10.3389/fmicb.2018.01682/full
Costerton, J. W., Stewart, P. S., Greenberg, E. P. (1999). Bacterial Biofilms: A Common Cause of Persistent Infections. Sci. (80- ) 284 (5418), 1318–1322. doi: 10.1126/science.284.5418.1318
Cummings, M. C., McCormack, W. M. (1990). Increase in Resistance of Mycoplasma Hominis to Tetracyclines. Antimicrob. Agents Chemother. 34 (12), 2297–2299. doi: 10.1128/AAC.34.12.2297
de Crécy-Lagard, V., Jaroch, M. (2021)Functions of Bacterial tRNA Modifications: From Ubiquity to Diversity. In: Trends in Microbiology (Elsevier Ltd). Available at: http://www.cell.com/article/S0966842X2030189X/fulltext (Accessed 2021 Apr 26).
De Crécy-Lagard, V., Marck, C., Brochier-Armanet, C., Grosjean, H. (2007). Comparative RNomics and Modomics in Mollicutes: Prediction of Gene Function and Evolutionary Implications. IUBMB Life. 59 (10), 634–658. doi: 10.1080/15216540701604632
Demirci, H., Murphy, I. V. F., Belardinelli, R., Kelley, A. C., Ramakrishnan, V., Gregory, S. T., et al. (2010). Modification of 16S Ribosomal RNA by the KsgA Methyltransferase Restructures the 30S Subunit to Optimize Ribosome Function. Rna. 16 (12), 2319–2324. doi: 10.1261/rna.2357210
Desaulniers, J. P., Chang, Y. C., Aduri, R., Abeysirigunawardena, S. C., Santalucia, J., Chow, C. S. (2008). Pseudouridines in rRNA Helix 69 Play a Role in Loop Stacking Interactions. Org Biomol Chem. 6 (21), 3892–3895. doi: 10.1039/b812731j
Doi, Y., Arakawa, Y. (2007). 16S Ribosomal RNA Methylation: Emerging Resistance Mechanism Against Aminoglycosides. Clin. Infect. Dis. 45 (1), 88–94. doi: 10.1086/518605
Donders, G. G. G., Ruban, K., Bellen, G., Petricevic, L. (2017). Mycoplasma/Ureaplasma Infection in Pregnancy: To Screen or Not to Screen. J. Perinat. Med. 45 (5), 505–515. doi: 10.1515/jpm-2016-0111
Duval, M., Korepanov, A., Fuchsbauer, O., Fechter, P., Haller, A., Fabbretti, A., et al. (2013). Escherichia Coli Ribosomal Protein S1 Unfolds Structured mRNAs Onto the Ribosome for Active Translation Initiation. PLoS Biol. 11 (12), 12–14. doi: 10.1371/journal.pbio.1001731
Feng, M., Schaff, A. C., Balish, M. F. (2020). Mycoplasma Pneumoniae Biofilms Grown In Vitro: Traits Associated With Persistence and Cytotoxicity. Microbiol. (United Kingdom). 166 (7), 629–640. doi: 10.1099/mic.0.000928
Fisunov, G. Y., Pobeguts, O. V., Ladygina, V. G., Zubov, A. I., Galyamina, M. A., Kovalchuk, S. I., et al. (2022). Thymidine Utilisation Pathway is a Novel Phenotypic Switch of Mycoplasma Hominis. J. Med. Microbiol. 71 (1), 1–17. doi: 10.1099/jmm.0.001468
Gagneux-Brunon, A., Grattard, F., Morel, J., Suy, F., Fuzellier, J. F., Verhoeven, P., et al. (2015). Mycoplasma Hominis, a Rare But True Cause of Infective Endocarditis. J. Clin. Microbiol. 53 (9), 3068–3071. doi: 10.1128/JCM.00827-15
Gaidenko, T. A., Kim, T. J., Price, C. W. (2002). The PrpC Serine-Threonine Phosphatase and PrkC Kinase Have Opposing Physiological Roles in Stationary-Phase Bacillus Subtilis Cells. J. Bacteriol. 184 (22), 6109–6114. doi: 10.1128/JB.184.22.6109-6114.2002
Gao, T., Yuan, F., Liu, Z., Liu, W., Zhou, D., Yang, K., et al. (2019). MnmE, a Central tRNA-Modifying GTPase, is Essential for the Growth, Pathogenicity, and Arginine Metabolism of Streptococcus Suis Serotype 2. Front. Cell Infect. Microbiol. 9. doi: 10.3389/fcimb.2019.00173
Garanina, I. A., Fisunov, G. Y., Govorun, V. M. (2018). BAC-BROWSER: The Tool for Visualization and Analysis of Prokaryotic Genomes. Front. Microbiol. 9 (NOV), 1–6. doi: 10.3389/fmicb.2018.02827
Goto, S., Kato, S., Kimura, T., Muto, A., Himeno, H. (2011). RsgA Releases RbfA From 30S Ribosome During a Late Stage of Ribosome Biosynthesis. EMBO J. 30 (1), 104–114. doi: 10.1038/emboj.2010.291
Gray, D. A., Dugar, G., Gamba, P., Strahl, H., Jonker, M. J., Hamoen, L. W. (2019). Extreme Slow Growth as Alternative Strategy to Survive Deep Starvation in Bacteria. Nat. Commun. 10 (1), 1–12. doi: 10.1038/s41467-019-08719-8
Grosjean, H., Breton, M., Sirand-Pugnet, P., Tardy, F., Thiaucourt, F., Citti, C., et al. (2014). Predicting the Minimal Translation Apparatus: Lessons From the Reductive Evolution of Mollicutes. PLoS Genet. 10 (5), e1004363. doi: 10.1371/journal.pgen.1004363
Grossman, T. H. (2016). Tetracycline Antibiotics and Resistance. Cold Spring Harb. Perspect. Med. 6 (4), 1–24. doi: 10.1101/cshperspect.a025387
Helser, T. L., Davies, J. E., Dahlberg, J. E. (1971). Change in Methylation of 16S Ribosomal RNA Associated With Mutation to Kasugamycin Resistance in Escherichia Coli. Nat. New Biol. 233 (35), 12–14. doi: 10.1038/newbio233012a0
Henao-Martínez, A. F., Young, H., Nardi-Korver, J. J. L., Burman, W. (2012). Mycoplasma Hominis Brain Abscess Presenting After a Head Trauma: A Case Report. J. Med. Case Rep. 6, 2–4. doi: 10.1186/1752-1947-6-253
Jones, P., Binns, D., Chang, H. Y., Fraser, M., Li, W., McAnulla, C., et al. (2014). InterProScan 5: Genome-Scale Protein Function Classification. Bioinformatics. 30 (9), 1236–1240. doi: 10.1093/bioinformatics/btu031
Juncker, A. S., Willenbrock, H., von Heijne, G., Brunak, S., Nielsen, H., Krogh, A. (2003). Prediction of Lipoprotein Signal Peptides in Gram-Negative Bacteria. Protein Sci. 12 (8), 1652–1662. doi: 10.1110/ps.0303703
Kakimoto, Y., Akazawa, S. (1970). Isolation and Identification of N-G,N-G- and N-G,N’-G-Dimethyl-Arginine, N-Epsilon-Mono-, Di-, and Trimethyllysine, and Glucosylgalactosyl- and Galactosyl-Delta-Hydroxylysine From Human Urine. J. Biol. Chem. 245 (21), 5751–5758. doi: 10.1016/S0021-9258(18)62716-4
Kimura, S., Waldor, M. K. (2019). The RNA Degradosome Promotes tRNA Quality Control Through Clearance of Hypomodified tRNA. Proc. Natl. Acad. Sci. U.S.A. 116 (4), 1394–1403. doi: 10.1073/pnas.1814130116
Koczula, A., Jarek, M., Visscher, C., Valentin-Weigand, P., Goethe, R., Willenborg, J. (2017). Transcriptomic Analysis Reveals Selective Metabolic Adaptation of Streptococcus Suis to Porcine Blood and Cerebrospinal Fluid. Pathogens. 6 (1), 6–13. doi: 10.3390/pathogens6010007
Kovacs-Simon, A., Titball, R. W., Michell, S. L. (2011). Lipoproteins of Bacterial Pathogens. Infect. Immun. 79 (2), 548–561. doi: 10.1128/IAI.00682-10
Kovalchuk, S. I., Jensen, O. N., Rogowska-Wrzesinska, A. (2019). FlashPack: Fast and Simple Preparation of Ultrahigh-Performance Capillary Columns for LC-Ms. Mol. Cell Proteomics. 18 (2), 383–390. doi: 10.1074/mcp.TIR118.000953
Krogh, A., Larsson, B., Von Heijne, G., Sonnhammer, E. L. L. (2001). Predicting Transmembrane Protein Topology With a Hidden Markov Model: Application to Complete Genomes. J. Mol. Biol. 305 (3), 567–580. doi: 10.1006/jmbi.2000.4315
Lee, A. J., Wang, S., Meredith, H. R., Zhuang, B., Dai, Z., You, L. (2018). Robust, Linear Correlations Between Growth Rates and β-Lactam–Mediated Lysis Rates. Proc. Natl. Acad. Sci. 115 (16), 4069–4074. doi: 10.1073/pnas.1719504115
Liang, X. H., Liu, Q., Fournier, M. J. (2007). rRNA Modifications in an Intersubunit Bridge of the Ribosome Strongly Affect Both Ribosome Biogenesis and Activity. Mol. Cell. 28 (6), 965–977. doi: 10.1016/j.molcel.2007.10.012
Llopis, P. M., Jackson, A. F., Sliusarenko, O., Surovtsev, I., Heinritz, J., Emonet, T., et al. (2010). Spatial Organization of the Flow of Genetic Information in Bacteria. Nature 466 (7302), 77–81. doi: 10.1038/nature09152
Lopatkin, A. J., Stokes, J. M., Zheng, E. J., Yang, J. H., Takahashi, M. K., You, L., et al. (2019). Bacterial Metabolic State More Accurately Predicts Antibiotic Lethality Than Growth Rate. Nat. Microbiol. 4 (12), 2109–2117. doi: 10.1038/s41564-019-0536-0
Machulin, A. V., Deryusheva, E. I., Selivanova, O. M., Galzitskaya, O. V. (2019). The Number of Domains in the Ribosomal Protein S1 as a Hallmark of the Phylogenetic Grouping of Bacteria. PLoS One 14 (8), 1–15. doi: 10.1371/journal.pone.0221370
McAuliffe, L., Ellis, R. J., Miles, K., Ayling, R. D., Nicholas, R. A. J. (2006). Biofilm Formation by Mycoplasma Species and its Role in Environmental Persistence and Survival. Microbiology. 152 (4), 913–922. doi: 10.1099/mic.0.28604-0
Mian, A. N., Farney, A. C., Mendley, S. R. (2005). Mycoplasma Hominis Septic Arthritis in a Pediatric Renal Transplant Recipient: Case Report and Review of the Literature. Am. J. Transplant. 5 (1), 183–188. doi: 10.1111/j.1600-6143.2004.00634.x
Nakahigashi, K., Kubo, N., Narita, S. I., Shimaoka, T., Goto, S., Oshima, T., et al. (2002). HemK, a Class of Protein Methyl Transferase With Similarity to DNA Methyl Transferases, Methylates Polypeptide Chain Release Factors, and hemK Knockout Induces Defects in Translational Termination. Proc. Natl. Acad. Sci. U S A. 99 (3), 1473–1478. doi: 10.1073/pnas.032488499
Ogura, M., Kanesaki, Y. (2018). Newly Identified Nucleoid-Associated-Like Protein YlxR Regulates Metabolic Gene Expression in Bacillus Subtilis. mSphere 3 (5), 1–15. doi: 10.1128/mSphere.00501-18
Ogura, M., Sato, T., Abe, K. (2019). Bacillus Subtilisylxr, Which is Involved in Glucose-Responsive Metabolic Changes, Regulates Expression of Tsadfor Protein Quality Control of Pyruvate Dehydrogenase. Front. Microbiol. 10, 1–15. doi: 10.3389/fmicb.2019.00923
Pereyre, S., Sirand-Pugnet, P., Beven, L., Charron, A., Renaudin, H., Barré, A., et al. (2009). Life on Arginine for Mycoplasma Hominis: Clues From its Minimal Genome and Comparison With Other Human Urogenital Mycoplasmas. PLVVoS Genet. 5 (10), e1000677. doi: 10.1371/journal.pgen.1000677
Pierson, W. E., Hoffer, E. D., Keedy, H. E., Simms, C. L., Dunham, C. M., Zaher, H. S. (2016). Uniformity of Peptide Release Is Maintained by Methylation of Release Factors. Cell Rep. 17 (1), 11–18. doi: 10.1016/j.celrep.2016.08.085
Reygaert, W. C. (2018). An Overview of the Antimicrobial Resistance Mechanisms of Bacteria. AIMS Microbiol. 4 (3), 482–501. doi: 10.3934/microbiol.2018.3.482
Rockwood, L. L. (2015). Introduction to Population Ecology. 2nd Edition (Wiley), 378. Available at: https://www.wiley.com/en-us/Introduction+to+Population+Ecology%2C+2nd+Edition-p-9781118947579.
Rogozin, I. B. (2002). Connected Gene Neighborhoods in Prokaryotic Genomes. Nucleic Acids Res. 30 (10), 2212–2223. doi: 10.1093/nar/30.10.2212
Savojardo, C., Martelli, P. L., Fariselli, P., Profiti, G., Casadio, R. (2018). BUSCA: An Integrative Web Server to Predict Subcellular Localization of Proteins. Nucleic Acids Res. 46 (W1), W459–W466. doi: 10.1093/nar/gky320
Schedlbauer, A., Kaminishi, T., Ochoa-Lizarralde, B., Dhimole, N., Zhou, S., López-Alonso, J. P., et al. (2015). Structural Characterization of an Alternative Mode of Tigecycline Binding to the Bacterial Ribosome. Antimicrob. Agents Chemother. 59 (5), 2849–2854. doi: 10.1128/AAC.04895-14
Schmidl, S. R., Gronau, K., Hames, C., Busse, J., Becher, D., Hecker, M., et al. (2010). The Stability of Cytadherence Proteins in Mycoplasma Pneumoniae Requires Activity of the Protein Kinase PrkC. Infect. Immun. 78 (1), 184–192. doi: 10.1128/IAI.00958-09
Schulze, A., Mitterer, F., Pombo, J. P., Schild, S. (2021). Biofilms by Bacterial Human Pathogens: Clinical Relevance - Development, Composition and Regulation - Therapeutical Strategies. Microb. Cell. 8 (2), 28–56. doi: 10.15698/mic2021.02.741
Semashko, T. A., Evsyutina, D. V., Ladygina, V. G., Zubov, A. I., Rakovskaya, I. V., Kovalchuk, S. I., et al. (2020). Data on Proteome of Mycoplasma Hominis Cultivated With Arginine or Thymidine as a Carbon Source. Data Br. 31, 106034. doi: 10.1016/j.dib.2020.106034
Sergiev, P. V., Golovina, A. Y., Prokhorova, I. V., Sergeeva, O. V., Osterman, I. A., Nesterchuk, M. V., et al. (2011). Modifications of Ribosomal RNA: From Enzymes to Function. Ribosomes., 97–110. doi: 10.1007/978-3-7091-0215-2_9
Sharma, I. M., Woodson, S. A. (2020). RbfA and IF3 Couple Ribosome Biogenesis and Translation Initiation to Increase Stress Tolerance. Nucleic Acids Res. 48 (1), 359–372. doi: 10.1093/nar/gkz1065
Shippy, D. C., Fadl, A. A. (2014). tRNA Modification Enzymes gidA and Mnme: Potential Role in Virulence of Bacterial Pathogens. Int. J. Mol. Sci. 15 (10), 18267–18280. doi: 10.3390/ijms151018267
Song, C., Kumar, A., Saleh, M. (2009). Bioinformatic Comparison of Bacterial Secretomes. Genomics Proteomics Bioinforma 7 (1–2), 37–46. doi: 10.1016/S1672-0229(08)60031-5
Sprouffske, K., Wagner, A. (2016). Growthcurver: An R Package for Obtaining Interpretable Metrics From Microbial Growth Curves. BMC Bioinf. 17 (1), 17–20. doi: 10.1186/s12859-016-1016-7
Stewart, C. N., Via, L. E. (1993). A Rapid CTAB DNA Isolation Technique Useful for RAPD Fingerprinting and Other PCR Applications. Biotechniques 14 (5), 748–750.
van der Woude, M. W., Bäumler, A. J. (2004). Phase and Antigenic Variation in Bacteria Phase and Antigenic Variation in Bacteria. Clin Microbiol Rev. 17, 3, 1073–1085. doi: 10.1128/CMR.17.3.581-611.2004
Vogeley, L., El Arnaout, T., Bailey, J., Stansfeld, P. J., Boland, C., Caffrey, M. (2016). Structural Basis of Lipoprotein Signal Peptidase II Action and Inhibition by the Antibiotic Globomycin. Sci. (80- ) 351 (6275), 876–880. doi: 10.1126/science.aad3747
Keywords: slow growth, antibiotic sensitivity, Mycoplasma hominis, proteomics, thymidine
Citation: Evsyutina DV, Semashko TA, Galyamina MA, Kovalchuk SI, Ziganshin RH, Ladygina VG, Fisunov GY and Pobeguts OV (2022) Molecular Basis of the Slow Growth of Mycoplasma hominis on Different Energy Sources. Front. Cell. Infect. Microbiol. 12:918557. doi: 10.3389/fcimb.2022.918557
Received: 12 April 2022; Accepted: 15 June 2022;
Published: 07 July 2022.
Edited by:
Marat R. Sadykov, University of Nebraska Medical Center, United StatesReviewed by:
Yuri Gogolev, Kazan Institute of Biochemistry and Biophysics (RAS), RussiaSvetlana A. Ermolaeva, N.F. Gamaleya Scientific Research Institute of Epidemiology and Microbiology (RAMS), Russia
Copyright © 2022 Evsyutina, Semashko, Galyamina, Kovalchuk, Ziganshin, Ladygina, Fisunov and Pobeguts. This is an open-access article distributed under the terms of the Creative Commons Attribution License (CC BY). The use, distribution or reproduction in other forums is permitted, provided the original author(s) and the copyright owner(s) are credited and that the original publication in this journal is cited, in accordance with accepted academic practice. No use, distribution or reproduction is permitted which does not comply with these terms.
*Correspondence: Daria V. Evsyutina, dar-evsyutina@yandex.ru