Molecular mechanisms and functions of pyroptosis in sepsis and sepsis-associated organ dysfunction
- Department of Pediatrics, Pediatric Intensive Care Unit (PICU), Shengjing Hospital of China Medical University, Shenyang, China
Sepsis, a life-threatening organ dysfunction caused by a dysregulated host response to infection, is a leading cause of death in intensive care units. The development of sepsis-associated organ dysfunction (SAOD) poses a threat to the survival of patients with sepsis. Unfortunately, the pathogenesis of sepsis and SAOD is complicated, multifactorial, and has not been completely clarified. Recently, numerous studies have demonstrated that pyroptosis, which is characterized by inflammasome and caspase activation and cell membrane pore formation, is involved in sepsis. Unlike apoptosis, pyroptosis is a pro-inflammatory form of programmed cell death that participates in the regulation of immunity and inflammation. Related studies have shown that in sepsis, moderate pyroptosis promotes the clearance of pathogens, whereas the excessive activation of pyroptosis leads to host immune response disorders and SAOD. Additionally, transcription factors, non-coding RNAs, epigenetic modifications and post-translational modifications can directly or indirectly regulate pyroptosis-related molecules. Pyroptosis also interacts with autophagy, apoptosis, NETosis, and necroptosis. This review summarizes the roles and regulatory mechanisms of pyroptosis in sepsis and SAOD. As our understanding of the functions of pyroptosis improves, the development of new diagnostic biomarkers and targeted therapies associated with pyroptosis to improve clinical outcomes appears promising in the future.
1. Introduction
Sepsis is a life-threatening organ dysfunction caused by a dysregulated host response to infection. It can progress to septic shock, which is accompanied by tissue and cell metabolic abnormalities (Singer et al., 2016). Epidemiological studies have found that there are approximately 18 million patients with sepsis worldwide every year with a mortality rate of 28–40%. The mortality rate of patients with septic shock is even higher (Walkey et al., 2015). Sepsis and septic shock are among the leading causes of death in patients in intensive care units worldwide, seriously threatening both life and health. Although diagnoses and treatments have improved for sepsis and septic shock, the current treatments are mainly symptomatic treatments and anti-infection therapy. Exploring the molecular mechanisms underlying sepsis is of great significance to develop novel therapeutic targets. The pathogenesis of sepsis is complicated, with inflammation and immune responses playing critical roles (Venet and Monneret, 2018; van der Poll et al., 2021). Recent studies have found that pyroptosis is involved in the regulation of inflammation and immune responses, suggesting a key role of pyroptosis in sepsis (He et al., 2021; Hsu et al., 2021; Miao et al., 2022).
As a form of pro-inflammatory programmed cell death, pyroptosis is characterized by the formation of pores on the cell membrane, cell swelling, and rupture accompanied by the release of abundant inflammatory factors and cellular contents (Cookson and Brennan, 2001; Galluzzi et al., 2018). Previous studies have shown that pyroptosis is involved in the occurrence and development of various inflammatory and infectious diseases (Liu et al., 2019; Xia et al., 2019; Zhou and Fang, 2019). Moderate pyroptosis can promote the release of pathogens by destroying infected cells, thereby recruiting immune cells to activate the host immune response, promoting the clearance of pathogens, and protecting the body against infections. However, the excessive activation of pyroptosis may adversely affect prognoses by aggravating the inflammatory response and cell or tissue damage. In recent years, an increasing number of studies have shown that pyroptosis plays an essential role in sepsis and sepsis-associated organ dysfunction (SAOD) (Chen et al., 2019; Martinez-Garcia et al., 2019).
In this review, we summarized the roles of pyroptosis in sepsis, highlighting more comprehensive molecular mechanisms regarding pyroptosis in sepsis from aspects of the potential roles of pyroptosis in septic organ dysfunctions, as well as first illustrating the factors that could influence the pyroptosis in sepsis. We also described the mechanisms that influence the onset of pyroptosis and the effects of pyroptosis on other forms of programmed cell death, providing evidence that pyroptosis plays a critical role in the process of sepsis.
2. Pyroptosis
Pyroptosis was originally known as inflammatory caspase-dependent cell death. However, with understanding of the gasdermins gene family deepens, pyroptosis is redefined as a gasdermins-mediated programmed cell death. Gasdermins, with the ability of forming pores on the membrane, are identified to be executors of pyroptosis. The family comprises six paralogous genes in humans: gasdermin A (GSDMA), gasdermin B (GSDMB), gasdermin C (GSDMC), GSDMD (GSDMD), GSDME (GSDME, DFNA5) and Pejvakin (PJVK, DFNB59). Mice carry genes GSDMA1-3, GSDMC1-4, GSDMD, GSDME and PJVK, but without GSDMD. Except for PJVK, Gasdermins comprise two domains, a N-terminal domain with pore-forming potential and a C-terminal inhibiting domain. Proteolytic cleavage between these two domains releases intramolecular inhibition and generates an active N-terminal (gasdermin-NT), allowing it to insert into the cell membrane and oligomerize to form pores that leads to pyroptosis (Liu et al., 2021). According to the Human Protein Atlas, each member of the gasdermins family exhibits distinct tissue expression (Supplementary Figure 1). Notably, during sepsis, the protein expression of GSDMA-NT, GSDMD-NT and GSDME-NT are reported to increase in sepsis-associated organ injuries, indicating that pyroptosis could play important roles in sepsis (Chen et al., 2021b; Teng et al., 2022). Depending on the inflammatory caspases involved, pyroptosis can be divided into the canonical pathway mediated by caspase-1, and the non-canonical pathway mediated by caspase-4, -5, or -11 (Kayagaki et al., 2015; Shi et al., 2015). In canonical and non-canonical pyroptosis pathway, inflammasome, a kind of macromolecular protein complex, plays a vital role as they provide a platform for the cleavage and maturation of inflammatory caspases. Notably, pyroptosis has been found to be also mediated by apoptotic caspase, granzyme, and elastase in recent years, which makes people have a more comprehensive understanding of pyroptosis (Wang et al., 2017; Sollberger et al., 2018; Zhou et al., 2020).
2.1 Canonical pyroptosis pathway
Different inflammasomes varies in their composition and the stimuli they can recognize (Sharma and Kanneganti, 2016; Xue et al., 2019). The most common inflammasome in the canonical pathway is the NLR family pyrin domain containing 3 (NLRP3) inflammasome, which is activated by recognizing multiple pathogen-associated molecular patterns (PAMPs) and damage-associated molecular patterns (DAMPs) (Figure 1) (Zhao and Zhao, 2020).
The NLRP3 inflammasome comprises three components: the sensor protein NLRP3, apoptosis-associated speck-like protein containing a CARD (ASC), and pre-caspase-1 (Lechtenberg et al., 2014; Sharma and Kanneganti, 2016). There are two steps required for the activation of NLRP3 (Vanaja et al., 2015). Under the stimulation of microbial molecules (such as toll-like receptor [TLR] ligands) or endogenous factors (such as tumor necrosis factor-α), the transcription of pyroptosis-related molecules, such as NLRP3 and pro-interleukin [IL]-1β, increases to a level where NLRP3 activation may occur, which is dependent on the activation of nuclear factor-kappa-gene binding (NF-κB). The second step is the activation and assembly of the NLRP3 inflammasome. Activated by various PAMPs and DAMPs, NLRP3 binds to ASC and recruits the caspase-1 precursor to form the NLRP3 inflammasome. The caspase-1 precursor is cleaved to form the active caspase-1 protein, which subsequently induces pyroptosis. Active caspase-1 cleaves the GSDMD protein into active GSDMD to form pores with an inner diameter of approximately 15 nm on the cell membrane. Active caspase-1 further cleaves precursors IL-1β and IL-18 to form mature IL-1β and IL-18, which are released through the pores and promote inflammation (Ruan et al., 2018; Xia et al., 2021). The pores formed by GSDMD allow ions to enter the cells, which can cause changes in osmotic pressure, cell swelling, and cell rupture accompanied by the release of cellular contents, followed by pyroptosis (Liu et al., 2016). However, the exact molecular mechanisms underlying NLRP3 inflammasome activation remain unclear. Several molecular mechanisms have been proposed, including potassium (K+) efflux, mitochondrial dysfunction, release of reactive oxygen species (ROS) and mitochondrial DNA (mtDNA), lysosome disruption, chloride (Cl−) efflux, and calcium (Ca2+) flux (Lima et al., 2013; Gong et al., 2018; Swanson et al., 2019). Notably, thioredoxin-interacting protein (TXNIP), NIMA-related kinase 7 (NEK7), pannexin-1, and the P2X7 receptor (P2X7R) have recently been shown to be essential for NLRP3 activation. TXNIP is a thioredoxin (TRX)-binding protein that has been shown to participate in several biological processes such as oxidative stress, cell proliferation, and cell apoptosis by inhibiting the function of the TRX system. Under the stimulation of ROS, TXNIP binds to NLRP3 and activates the formation of the NLRP3 inflammasome (Zhou et al., 2010). NEK7, an essential protein downstream of K+ efflux, mediates the assembly and activation of the NLRP3 inflammasome (Sharif et al., 2019). Pannexin-1 and P2X7R are associated with K+ efflux and changes in ATP levels (Kelley et al., 2019). Notably, it was recently reported that the endosomal sorting complex required for transport (ESCRT)-dependent membrane repair process could remove the GSDMD pores and inhibit pyroptosis (Ruhl et al., 2018). Besides, several studies have discovered that GSDMD pores could regulate IL-1β secretion from living cells without causing cell death, which is called cell hyperactivated state (Evavold et al., 2018; Semino et al., 2018). These results demonstrated that pyroptosis could be regulated, providing basis for targeting pyroptosis in clinical therapy.
2.2 Non-canonical pyroptosis pathway
In the non-canonical pyroptosis pathway, caspase-4/5 (human) and caspase-11 (murine) precursors are apical activators. They directly recognize lipid A of lipopolysaccharide (LPS) in the cytoplasm through the caspase activation and recruitment domain (CARD) and form an inflammasome, which leads to their activation. Active caspase-4/5/11 cleave GSDMD to cause pyroptosis (Hagar et al., 2013; Shi et al., 2014; Aglietti et al., 2016; Yi, 2017). Unlike caspase-1, caspase-11 cannot directly cleave pro-IL-1β or pro-IL-18. Instead, caspase-11 indirectly induces the maturation and release of IL-1β and IL-18 by activating the NLRP3/ASC/caspase-1 pathway (Ding and Shao, 2017). The underlying mechanism may be related to the K+ efflux induced by pannexin-1 cleaved by caspase-11 (Kayagaki et al., 2011; Ruhl and Broz, 2015; Yang et al., 2015). Additionally, caspase-4-activated GSDMD could form pores on the mitochondrial membrane and generate ROS to activate the NLRP3 inflammasome. These results suggest that there are connections between the non-canonical and canonical pathways (Figure 2).
Previous studies have shown that in the non-canonical pyroptosis pathway, gram-negative bacteria can activate the TLR4-TIR-domain-containing adapter-inducing-interferon-β (TRIF) signaling pathway, which results in the production of type I interferons and upregulation of guanylate-binding proteins (GBPs) and immune-related guanosine triphosphatase (GTPase) B10 (IRGB10). Notably, IRGB10 can target outer membrane vesicles (OMVs) carrying LPS, thereby promoting LPS release and entry into the cytoplasm (Vanaja et al., 2016; Santos et al., 2018). In addition, LPS has also been found to enter the cytoplasm by combining with high-mobility group box 1 (HMGB1) (Deng et al., 2018). Several studies have found that in addition to LPS, lipid peroxidation induced by saturated fatty acids can also induce pyroptosis by activating caspase-4/5 (Pillon et al., 2016).
2.3 Other pyroptosis pathways
In addition to inflammatory caspases, apoptotic caspases can also cleave the gasdermins family to mediate pyroptosis. For example, unlike GSDMD, GSDME cannot be cleaved by inflammatory caspase-1/4/5/11. However, under the induction of chemotherapeutic drugs, the caspase-3 protein can cleave gasdermin E (GSDME), produce GSDME-NT with a similar effect to that of GSDMD-NT, and induce pyroptosis (Wang et al., 2017; Wang et al., 2018). A study by Orning et al. further found that the activation of caspase-8 during transforming growth factor (TGF)-β-activated kinase 1 (TAK1) inhibition led to the cleavage of GSDMD and GSDME in macrophages, which resulted in pyroptosis (Orning et al., 2018; Sarhan et al., 2018). Additionally, Mandal et al. found that the caspase-8 protein can interact with the caspase-11 protein to amplify inflammatory effects and tissue damage, indicating that caspase-8 participates in pyroptosis (Mandal et al., 2018). Notably, several studies have found that there are caspase-independent gasdermins activation and pyroptosis in recent years. For example, granzyme A (GzmA) and B (GzmB) have been reported to cleave gasdermin B (GSDMB) and GSDME, respectively (Liu et al., 2020; Zhang et al., 2020b; Zhou et al., 2020). In addition, neutrophil elastase (ELANE) was reported to cleave GSDMD and thus produced a fully active NT fragment, though the cleavage site was different from that of caspase-mediated. In turn, GSDMD-NT caused protease activation and nuclear expansion in a feed-forward loop (Kambara et al., 2018; Sollberger et al., 2018). These findings provide new insights into pyroptosis (Figure 2).
3. The role of pyroptosis in sepsis and sepsis-associated organ dysfunction
3.1 The role of pyroptosis in sepsis
Sepsis is a life-threatening organ dysfunction caused by a dysregulated host response to infection, which seriously threatens life and health because of its high morbidity, mortality, and heavy disease burden. The regulation of inflammatory and immune responses contributes to the occurrence and development of sepsis (Barriere and Lowry, 1995; van der Poll and Lowry, 1995; Hotchkiss and Karl, 2003). The host response to infection is initiated when innate immune cells, particularly macrophages, recognize and bind to microbial components. Then, multiple pro-inflammatory and anti-inflammatory mediators are released by the activated macrophages. If the mediators balance each other and the initial infectious insult are overcome, homeostasis will be restored and tissue will be repaired and healed. In contrast, sepsis occurs when the release of pro-inflammatory mediators in response to an infection exceeds the boundaries of the local environment, leading to a more generalized response. The cause may include the effect of the invading microorganisms and the susceptibleness of individuals. When the immune response becomes generalized, widespread cellular injury may occur. The cellular injury, accompanied by the release of pro-inflammatory and anti-inflammatory mediators, often progresses to organ dysfunction. In addition, excess inflammation of sepsis may be followed by immunosuppression, which may aggravate sepsis and sepsis-associated organ dysfunction (Boomer et al., 2011).
Recently, numerous studies have shown that both canonical and non-canonical pyroptosis are involved in the process of sepsis. As a form of pro-inflammatory programmed cell death, pyroptosis functions as a “double-edged sword” in sepsis. A large number of studies have found that pyroptosis can result in exacerbated sepsis. For instance, Li et al. found that the Chinese medicine Babaodan (BBD) can improve the survival of septic mice by diminishing inflammatory cytokine production and improving multiple organ injury. Further experiments have demonstrated that BBD can inhibit both the NF-κB pathway and assembly of the NLRP3 complex in peritoneal macrophages, suggesting that suppressing pyroptosis may be helpful in treating sepsis (Li et al., 2022). In addition, Chen et al. reported that drug-free tea polyphenol nanoparticles (TPNs) exhibited excellent therapeutic efficacy in septic mouse models by scavenging reactive oxygen and nitrogen species and blocking pyroptosis. At the molecular level, TPNs can suppress the pore-forming function of GSDMD by blocking its oligomerization, thus inhibiting pyroptosis (Chen et al., 2022). Furthermore, Wang et al. identified an 8-hydroxyquinoline derivative named 7-[phenyl (pyridin-2-ylamino) methyl] quinolin-8-ol (8-ol, NSC84094), which specifically inhibits HMGB1-mediated caspase-11 signaling. In vivo experiments have shown that 8-ol protects mice from sepsis, suggesting that inhibiting caspase-11-dependent non-canonical pyroptosis is also effective in the treatment of sepsis (Wang et al., 2021b). However, several studies have shown that the activation of pyroptosis exerts a protective role in sepsis, especially in the early stage. For example, a prospective study carried out by Garnacho-Montero et al., which included 55 patients with sepsis and 11 critically ill non-septic patients, measured the circulating levels of IL-1β and transcriptional expression of NLRP3 at admission and on days 3 and 7. The results showed that the activation of NLRP3 was more intense in patients with sepsis than in non-septic patients, and the NLRP3 expression was significantly higher on day 7 in patients with sepsis who survived. In addition, an increase in caspase-1 protein expression was observed in survivors compared to that in non-survivors on day 0. These results indicate that sustained NLRP3 activation could be protective during the first week of sepsis (Garnacho-Montero et al., 2020). Similarly, Liu et al. demonstrated that the diminished expression of the NLRP3 inflammasome components contributes to the suppression of monocytes and macrophages in patients with septic shock (Liu et al., 2020). In addition, a study conducted by Huet et al. found that the early activation of NLRP3 by hydrogen peroxide induces the timely and efficient activation of the innate immune response and exerts a protective function (Huet et al., 2017).
In conclusion, pyroptosis participates in sepsis and plays various roles at different stages of the illness. Exploring its regulatory factors may therefore provide a basis for pyroptosis-targeted sepsis therapies.
3.2 The role of pyroptosis in sepsis-associated organ dysfunction
An increasing number of studies have explored the role and molecular mechanisms of pyroptosis in SAOD (Supplementary Table 1). In sepsis-induced myocardial depression (SIMD), which is a devastating complication of sepsis with a mortality rate of greater than 50%, Kalbitz et al. discovered that the NLRP3 and IL-1β levels were greatly increased in the left ventricular cardiomyocytes of septic mouse models induced by cecal ligation and puncture (CLP). Compared with wild-type (WT) mice, NLRP3 -/- mice showed reduced cardiovascular compromise and plasma levels of IL-1β and IL-6, indicating that NLRP3 is involved in SIMD (Kalbitz et al., 2016). Similarly, Busch et al. showed that compared with septic WT mice, septic NLRP3 knockout mice showed improved survival rates and cardiac function. At the molecular level, IL-1β matures through the activation of the NLRP3 inflammasome, which could further cause atrophy, impair contractility and relaxation, and decrease the deformation of cardiomyocytes (Busch et al., 2021). Recently, Wang et al. revealed that monocyte-derived exosomes could harbor the TXNIP-NLRP3 complex and traffic it to resident heart macrophages, subsequently promoting the maturation of IL-1β and IL-18 in macrophages and aggravating cardiovascular inflammation. In addition, they reported that a small molecule named PSSM1443 could decrease the protein levels of active caspase-1, IL-1β, and IL-18 in SIMD mice by disrupting the interaction of TXNIP-NLRP3. This indicated that inhibiting the activation of the NLRP3 inflammasome is useful for SIMD treatment (Wang et al., 2021). Furthermore, Zhang et al. discovered that carbon monoxide releasing molecule-3 (CORM-3) could improve myocardial function in septic mice by inhibiting NLRP3 inflammasome activation in cardiac fibroblasts. Experiments in vitro further showed that CORM-3 prevents the interaction between NLRP3 and ASC (Zhang et al., 2017). These results suggest that pyroptosis is a potential therapeutic candidate for clinical sepsis-induced heart dysfunction.
Regarding sepsis-induced lung injury, which is found in 50% of patients with sepsis and results in poor outcomes, Cheng et al. found that inflammatory caspases participate in endothelial pyroptosis, with human caspase-4/5 in human endothelial cells (ECs) and murine caspase-11 in mice ECs in vivo. Compared with control mice, caspase-11-/- mice showed a reduction in inflammation and lung injury after CLP for 12 h, indicating that caspase-11 is involved in the pathogenesis of sepsis-induced lung injury (Cheng et al., 2017). Numerous studies have shown that pyroptosis in alveolar macrophages also plays an important role in sepsis-induced lung injury. For instance, Liu et al. found that the Gly-Pro-Ala (GPA) peptide can significantly ameliorate lung tissue injuries, pro-inflammatory cytokine release, and inflammatory cell infiltration in CLP-induced mice. Experiments in vitro have shown that the GPA peptide can prevent alveolar macrophages from caspase-1-dependent pyroptosis by decreasing ROS levels (Liu et al., 2021). In addition, it was found that the interaction of NLRP3-NEK7 and subsequent NLRP3 inflammasome assembly and activation can be blocked by 4-[2-(1H-indol-3-yl)-1,3-thiazol-4-yl] benzene-1,2-diol (1,2-diol), which is a 4-benzene-indol derivative. Further experiments in vivo have shown that 1,2-diol can alleviate LPS-induced acute lung injury, suggesting that inhibiting pyroptosis may be a novel method of treating sepsis-induced lung injuries (Li et al., 2022).
In a rat model of sepsis-induced brain injury, Zhou et al. found that, compared with those of the sham group, the mRNA and protein expression of NLRP3 and caspase-1 and the levels of inflammatory factors were increased in the cortexes of rats in the CLP group. At the molecular level, the cortical p38 mitogen-activated protein kinase (MAPK) and extracellular signal-regulated kinase (ERK) signaling pathways were activated. Moreover, they discovered that recombinant club cell secretory protein-16 (rCC16) could alleviate pathological changes in the cortex and inhibit the p38 MAPK and ERK signaling pathways and pyroptosis. However, the detailed mechanisms by which rCC16 functions remain unclear (Zhou et al., 2019). In addition, Sun et al. found that dexmedetomidine (DEX) could protect against neuronal injury by attenuating astrocyte pyroptosis through the inhibition of the NLRP3 inflammasome pathway (Sun et al., 2019). Intriguingly, Zhao et al. reported that NLRP3-IL-1β-IL-1R1 signaling is involved in the transition from acute to chronic neuroinflammation in sepsis, which is associated with progressive neurodegeneration and not the initiation of acute neuroinflammation (Zhao et al., 2020).
Pyroptosis is also involved in sepsis-induced kidney injury (SAKI). For example, Li et al. found that the levels of pyroptosis-related proteins were upregulated in the kidney tissues of CLP mice and in LPS-treated human kidney-2 (HK-2) cells. They also discovered that macrophage migration inhibitory factor (MIF) aggravated NLRP3-mediated pyroptosis by promoting the phosphorylation of p65 in vivo and in vitro (Li et al., 2022). Similarly, Yao et al. reported that DEX could alleviate LPS-induced acute kidney injury by inhibiting NLRP3 inflammasome activation through the suppression of the TLR4/NOX4/NF-κB pathway (Yao et al., 2019).
In sepsis-induced liver injury, Li et al. found that peroxisome proliferator-activated receptor (PPAR)-γ could exert a protective role by inhibiting hepatocyte pyroptosis through the inhibition of the ROS/TXNIP/NLRP3 pathway (Li et al., 2022). Intriguingly, Pai et al. discovered that the prophylactic administration of glutamine (GLN), an abundant free amino acid in the body, could initially upregulate liver pyroptosis to eradicate pathogens 24 h after CLP yet suppress pyroptosis 72 h after CLP. The finding suggested that GLN may regulate the balance of liver pyroptosis at different stages of sepsis, which may provide a basis for pyroptosis-targeted sepsis-induced liver injury treatments (Pai et al., 2020).
Pyroptosis also participates in other SAODs, including intestinal barrier dysfunction, vascular endothelial dysfunction, and disseminated intravascular coagulation (Chen et al., 2019; Chen et al., 2021b; Yuan et al., 2021). All of these studies have demonstrated that pyroptosis plays a critical role in SAOD, indicating that pyroptosis may be a potential target for treatments.
4. Regulation of pyroptosis under sepsis
The role of pyroptosis in sepsis is related to its severity and stage. Therefore, it is of great significance to explore the factors regulating pyroptosis, which may provide a basis for targeting pyroptosis during sepsis to help in immune protection without causing excessive damage to tissues and organs. Previous studies have shown that under septic conditions, transcription factors, non-coding RNAs (ncRNAs), epigenetic modifications, and post-translational modifications (PTMs) can play vital roles in regulating pyroptosis (Figure 3).
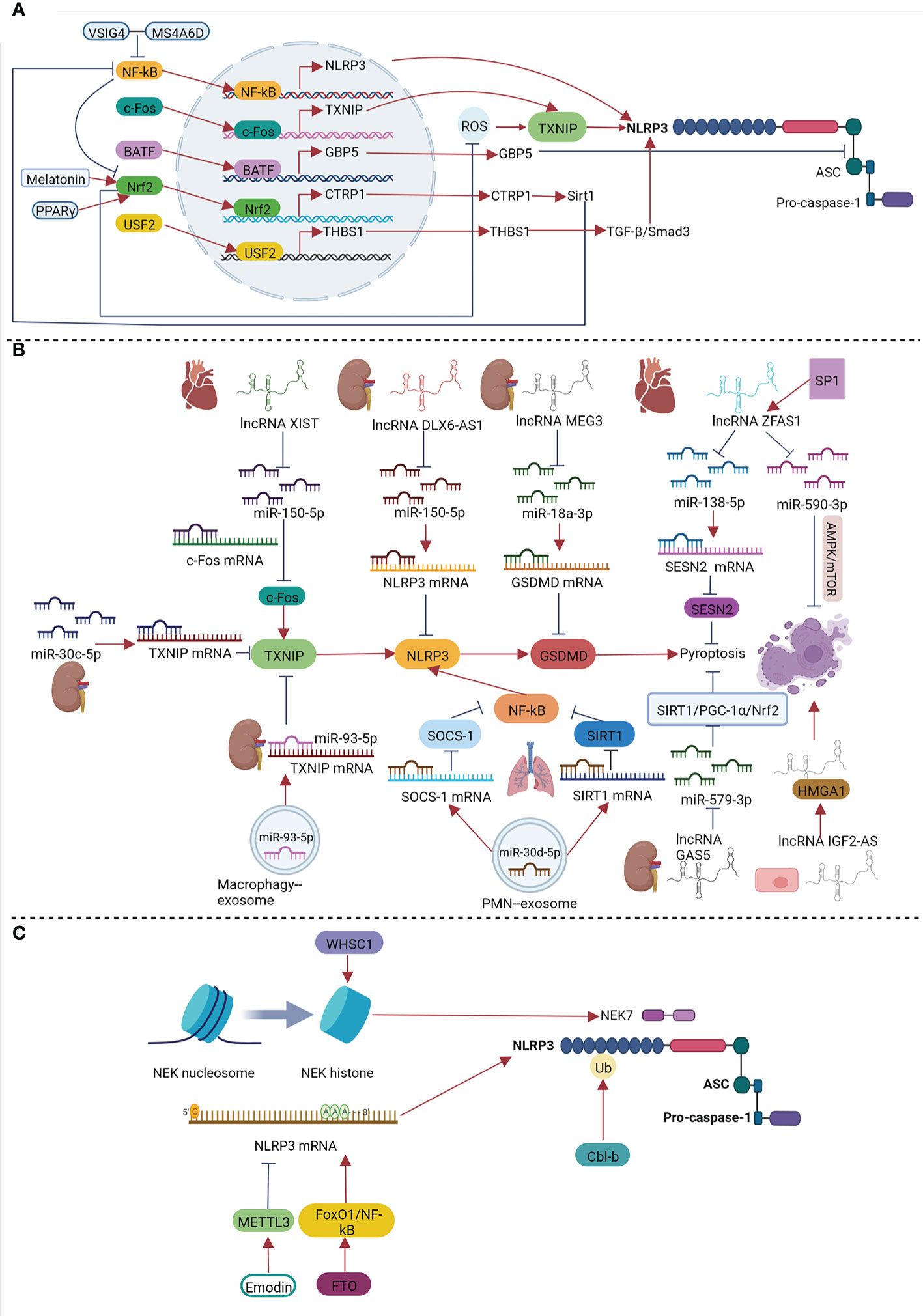
Figure 3 Regulation of pyroptosis under sepsis by transcription factors (A), non-coding RNAs (B), epigenetic modifications and PTMs (C).
4.1 Regulation of pyroptosis by transcription factors
Transcription factors are a class of proteins that can bind to specific sequences upstream of the 5’ end of genes to regulate their transcription. Various transcription factors participate in the regulation of pyroptosis in sepsis. Transcription factors can function by directly regulating the transcription of genes encoding proteins involved in pyroptosis-related pathways. For example, NF-κB is an important transcription factor that plays key roles in a variety of biological processes, such as inflammation, immunity, and cell death. As previously mentioned, NF-κB plays a role in the priming step of NLRP3 inflammasome activation, which ensures the activation and assembly of the NLRP3 inflammasome by increasing the transcriptional expression of NLRP3 and pro-IL-1β. Chen et al. found that the inhibition of p65 could significantly inhibit the decrease in cell viability in LPS-treated brain microvascular endothelial cells (BMECs) and reduce the expression of GSDMD. They also reported that p65 could directly bind to the NLRP3 promoter, indicating that p65 can directly regulate the expression of NLRP3 in LPS-induced BMECs (Chen et al., 2020). In addition, V-set and immunoglobulin domain containing 4 (VSIG4), a complement receptor of the immunoglobulin superfamily, was also found to inhibit the transcription of NLRP3 and IL-1β in macrophages in vitro and in vivo. At the molecular level, the VSIG4 protein could bind to member 6D of the membrane spanning 4-domains subfamily A (MS4A6D) and form an inhibitory signaling complex, which inhibited NF-κB (Huang et al., 2019; Shi et al., 2020). This study illustrated the essential role of NF-κB in NLRP3 inflammasome-mediated pyroptosis. Considering that NF-κB is a canonical transcription factor involved in inflammasomes and oxidative stress, we speculate that NF-κB can also modulate the second stage of NLRP3 inflammasome activation by promoting inflammation and ROS.
Transcription factors can also affect the activation and assembly of inflammasomes. The c-Fos protein, which is encoded by the Fosl2 gene, can form a transcription factor complex activator protein 1 (AP-1) with c-Jun in the nucleus. AP-1 participates in various biological processes, including inflammation and apoptosis. For example, Wang et al. found that the c-Fos protein can bind to the promoter region of TXNIP, thereby promoting pyroptosis in LPS-induced H9c2 cardiomyocytes (Wang et al., 2021a). Moreover, basic leucine zipper transcription factor ATF-like protein (BATF), a member of the AP-1 transcription factor family, participates in the regulation of the immune response (Murphy et al., 2013). Guo et al. further found that the BATF protein can promote the activation of the NLRP3 inflammasome by enhancing the transcription of guanylate-binding protein 5 (GBP5) in sepsis-induced liver injury (SALI), thus aggravating liver injury (Guo et al., 2021). GBP5 is a member of the GTPase superfamily, which has its own effect on host innate and cell-autonomous immunity (Praefcke, 2018). A previous study showed that GBP5 can promote NLRP3 inflammasome-mediated pyroptosis by facilitating the formation of the NLRP3-ASC complex (Shenoy et al., 2012).
Other transcription factors mediate pyroptosis by regulating stimulating factors or molecules (e.g., ROS). Nuclear factor erythroid 2 related factor (Nrf2) is a canonical transcription factor that regulates cellular oxidative stress responses and participates in maintaining redox homeostasis. In recent years, numerous studies have shown that Nrf2 can inhibit pyroptosis and play a regulatory role in a variety of diseases, including sepsis (Rahim et al., 2021; Li et al., 2022). Li et al. found that PPAR-γ could inhibit the ROS/TXNIP/NLRP3 signaling pathway by activating Nrf2 gene expression to inhibit hepatocyte pyroptosis, which ultimately had a protective effect against sepsis-induced liver injury (Li et al., 2022). Similarly, Rahim et al. revealed that melatonin can ameliorate sepsis-induced myocardial injury by activating the Nrf2-related pathway and inhibiting the formation of the NLRP3 inflammasome (Rahim et al., 2021). Teng et al. found that, in addition to inhibiting ROS, Nrf2 could have a protective role by upregulating C1q/tumor necrosis factor-related protein 1 (CTRP1) expression and inhibiting pyroptosis by binding to the promoter of CTRP1 in sepsis-induced myocardial injury in vivo and in vitro (Teng et al., 2022). CTRP1 is a homologue of adiponectin. Previous studies have shown that the overexpression of CTRP1 can improve the survival rate and heart function in LPS-treated mice by inhibiting myocardial inflammation, oxidative damage, and cellular apoptosis. The underlying molecular mechanism involves CTRP1 protein-activated sirtuin 1 (SIRT1) gene expression and the inhibition of the NF-κB pathway to promote Nrf2 gene expression. Based on these two studies, it is speculated that the transcription factors Nrf2 and CTRP may inhibit oxidative damage in sepsis-induced myocardial injury through positive feedback and play an important protective role. In addition to ROS, transcription factors can affect pyroptosis by regulating its associated molecules. For example, upstream stimulatory factor 2 (USF2), which is a member of the basic helix-loop-helix-leucine zipper transcription factor family, can regulate a variety of cellular processes (Sirito et al., 1992; Chi et al., 2020). In SAKI, Sun et al. found that the knockdown of USF2 led to the inhibition of the TGF-β/suppressor of mothers against decapentaplegic (Smad) 3 signaling pathway by downregulating the expression of thrombospondin1 (THBS1), thereby reducing pyroptosis and improving renal injury (Sun et al., 2022). TGF-β belongs to the TGF-β superfamily, and previous studies have shown that it is involved in the activation of the NLRP3 inflammasome (Zhang et al., 2020).
Transcription factors can therefore regulate pyroptosis in sepsis, especially SAOD, including its stimulation signals and the priming, activation, and assembly of the NLRP3 inflammasome.
4.2 Regulation of pyroptosis by non-coding RNAs
NcRNAs are a series of RNAs that do not encode proteins, including long non-coding RNAs (lncRNAs), microRNAs (miRNAs), and circular RNAs (circRNAs). They participate in and regulate various biological processes. Recent studies have shown that ncRNAs can be involved in the occurrence and development of various diseases, including sepsis, by regulating pyroptosis.
4.2.1 The role of long non-coding RNAs in pyroptosis in sepsis
LncRNAs are a class of ncRNAs that are more than 200 nucleotides in length. Related studies have suggested that lncRNAs can bind to DNA, RNA, and proteins, thus inhibiting or activating gene expression through complex biological mechanisms (Borkiewicz et al., 2021). Several studies have shown that the regulation of pyroptosis by lncRNAs is important in sepsis.
The lncRNA zinc finger antisense 1 (ZFAS1) has regulatory roles in various tumors. In 2021, An et al. found that lncRNA ZFAS1 expression was downregulated in the sera of patients with SIMD compared to that in healthy individuals. The overexpression of lncRNA ZFAS1 in vitro ameliorated the decrease in cell viability and increase in the levels of pyroptosis-associated proteins and inflammatory factors in LPS-induced H9c2 cardiomyocytes, indicating that the upregulation of lncRNA ZFAS1 expression could alleviate LPS-induced cardiomyocyte pyroptosis. Mechanistically, lncRNA ZFAS1 acts by upregulating sestrin2 (SESN2) expression through the inhibition of miR-138-5p by directly binding to it. In addition, a previous study using an LPS-induced septic rat model found that myocardial tissue damage and inflammatory cell infiltration were reduced following the overexpression of lncRNA ZFAS1, which further indicated that lncRNA ZFAS1 had a protective effect and could improve myocardial injury by inhibiting LPS-induced cardiomyocyte pyroptosis (An et al., 2021). Notably, Liu et al. came to the opposite conclusion based on a CLP-induced septic mouse model and LPS-treated mouse primary cardiomyocytes (Liu et al., 2020). They found that the expression of lncRNA ZFAS1 was upregulated in the myocardial tissues of mice in the CLP group compared with that in the control. However, inhibiting the expression of ZFAS1 could improve cardiac function, suggesting that ZFAS1 aggravates myocardial injuries in sepsis. Mechanistically, experiments in vitro showed that the upregulation in lncRNA ZFAS1 expression was caused by the activation of its transcription by the transcription factor specificity protein 1. LncRNA ZFAS1 could further act as a competing endogenous RNA (ceRNA) to inhibit the AMP-activated protein kinase (AMPK)-mammalian target of rapamycin (mTOR) pathway by suppressing miR-590-3p, thereby aggravating SIMD by inhibiting autophagy and promoting pyroptosis (Liu et al., 2020). The reason for this difference may be that the expression of lncRNA ZFAS1 changes dynamically at different stages of sepsis. Notably, there are differences between the circulatory manifestations of LPS-induced and CLP-induced septic models, as the former is hypodynamic. Thus, the expression of lncRNA ZFAS1 may be different in the heart tissues of the models. Various miRNAs may be downstream of lncRNA ZFAS1; therefore, it may also participate in other cellular processes in addition to pyroptosis. In future studies, it will be of great significance to dynamically monitor the changes in lncRNA ZFAS1 in SIMD and to explore the main downstream pathways and cellular processes regulated by lncRNA ZFAS1 at different periods. In addition to lncRNA ZFAS1, lncRNA X-inactive specific transcript (XIST) also participates in the regulatory process of pyroptosis in SIMD. For example, Wang et al. found that the expression of lncRNA XIST was upregulated in vitro and in vivo, and XIST acts as a ceRNA to inhibit miR-150-5p and promote the expression of the c-Fos gene. On one hand, c-Fos bound to the promoter of the TXNIP gene to promote TXNIP-mediated pyroptosis; on the other hand, it bound to the promoter of the XIST gene to upregulate the expression of XIST, which promoted the XIST/miR-150-5p/c-Fos axis through positive feedback. Moreover, experiments in vivo showed that after inhibiting XIST, the expression of pyroptosis-associated proteins in myocardial tissue was downregulated, and myocardial injury was alleviated (Wang et al., 2021a). These results provide new insights for the treatment of SIMD.
The regulation of pyroptosis by lncRNAs can also influence the development of SAKI. In a mouse SAKI model established using CLP and HK-2 cells induced by LPS, Ling et al. found that lncRNA growth arrest specific 5 (GAS5) could activate the SIRT1/PPAR-γ coactivator-1α/Nrf2 signaling pathway by targeting miR-579-3p and inhibiting pyroptosis. The overexpression of lncRNA GAS5 or knockdown of miR-579-3p could enhance the expression of the SIRT1 gene, improve the survival rate of mice, and relieve kidney injuries (Ling et al., 2021). In addition, the levels of lncRNAs maternally expressed 3 and distal-less homeobox 6 antisense RNA 1 were also reported to be elevated in SAKI and could promote pyroptosis in renal tubular epithelial cells induced by LPS by regulating the miR-18a-3p/GSDMD and miR-223-3p/NLRP3 signaling pathways, respectively. However, these studies lacked in vivo experiments to verify whether the inhibition of these two pathways had a protective effect in SAKI (Tan et al., 2020; Deng et al., 2021).
Additionally, pyroptosis regulated by lncRNAs also participates in the repair of vascular endothelial injury in sepsis. Liang et al. extracted and identified endothelial progenitor cells (EPCs) from the peripheral blood collected from healthy individuals and patients with sepsis. The results showed that the lncRNA insulin-like growth factor 2 antisense RNA (IGF2-AS) was highly expressed in EPCs from the septic group compared with the healthy group. Further studies showed that lncRNA IGF2-AS could bind to the high mobility group protein A1 to regulate nucleotide metabolism and promote the pyroptosis of EPCs. This study also preliminarily explored the effect of exosomal lncRNA IGF2-AS from mesenchymal stem cells (MSCs) in sepsis. The results showed that the MSC-derived exosomal lncRNA IGF2-AS could promote the pyroptosis of EPCs in patients with sepsis, providing insights for potential therapeutic targets for sepsis (Liang et al., 2022).
4.2.2 The role of miRNAs in pyroptosis in sepsis
As we know, miRNAs are a class of ncRNAs that are approximately 22–26 nucleotides in length. They regulate target gene expression at the post-transcriptional level by binding to the 3’-untranslated region (UTR) of downstream genes (Huang, 2017; Subramanian and Steer, 2019). Numerous studies have found that miRNAs participate in sepsis and SAODs (Ho et al., 2016; Ghafouri-Fard et al., 2021). For example, Sun et al. found that exosomal miR-27b derived from MSCs could inhibit sepsis in CLP-induced mice and attenuate inflammation in LPS-mediated bone marrow-derived macrophages (BMDMs). The underlying molecular mechanism was that miR-27b could inhibit JMJD3 and inactivate the NF-κB signaling pathway (Sun et al., 2021). In addition, Chen et al. reported that microRNA-23a-5p aggravated LPS-induced acute lung injury by targeting HSP20/ASK1 (Chen et al., 2021). These results suggested that miRNAs were involved in sepsis and SAODs.
Recently, miRNAs have been found to be invloved in sepsis by regulating pyroptosis (Li et al., 2021). In addition to miRNAs, which play a regulatory role in pyroptosis in sepsis as downstream lncRNAs, Li et al. revealed that miR-30c-5p expression was significantly downregulated in the kidney tissues of septic mice and HK-2 cells induced by LPS compared with control groups. They discovered that the overexpression of miR-30c-5p using a miR-30c-5p mimic could lead to the downregulation of the expression of NLRP3, caspase-1, and ASC as well as downstream cytokines such as IL-1β and IL-18 in HK-2 cells induced by LPS and ATP. This indicated that miR-30c-5p is involved in the process of SAKI through the regulation of NLRP3/caspase-1-mediated pyroptosis. Further experiments showed that the TXNIP gene was the target of miR-30c-5p, and miR-30c-5p inhibited pyroptosis by inhibiting TXNIP gene expression. Moreover, the mice that were administered kidney-specific injections of the miR-30c-5p mimic showed decreased tubular cavity expansion, vacuolar degeneration of tubular epithelial cells, and expression of inflammatory factors compared with the control group. In addition, the protein expression of TXNIP, NLRP3, caspase-1, and ASC in renal tissues was inhibited. Similarly, the kidney-specific knockdown of TXNIP protected against renal injury and significantly downregulated the expression of pyroptosis-associated proteins, further indicating that miR-30c-5p could negatively regulate NLRP3-mediated pyroptosis by inhibiting TXNIP gene expression (Li et al., 2021). Considering that the molecular mechanisms of miRNAs are relatively definite and miRNAs can not only regulate pyroptosis-associated proteins directly but also mediate the regulation of lncRNAs, miRNAs may be a valuable research topic for sepsis and SAOD.
4.2.3 The role of exosome-driven non-coding RNAs in pyroptosis in sepsis
Exosomes are extracellular vesicles secreted by various cells. They can transfer proteins and genetic material (including DNA, mRNA, and miRNA) to target cells and play a profound role in intercellular communication. In addition to the MSC-derived exosomal lncRNA IGF2-AS (Liang et al., 2022), the ncRNAs delivered by exosomes may have crucial roles in sepsis. For example, Jiao et al. found that exosomal miR-30d-5p of neutrophils (polymorphonuclear neutrophils) could activate macrophage pyroptosis and aggravate lung injury in sepsis-induced acute lung injury by activating NF-κB. The molecular mechanisms involved exosomal miR-30d-5p targeting suppressor of cytokine signaling 1 and SIRT1 genes in macrophages and subsequently activating NF-κB in part by increasing the acetylation of lysine 310 in p65 (Jiao et al., 2021). In addition, Juan et al. found that M1 macrophages aggravated the level of pyroptosis in the LPS-induced mouse kidney epithelial cell line TCMK-1, whereas M2 exerted the opposite effect. Further experiments in vitro and in vivo revealed that this opposite effect was caused by the difference in the expression of miR-93-5p carried by M1 and M2. The expression of miR-93-5p was significantly upregulated in M2 exosomes, and it could directly target TXNIP to inhibit the pyroptosis of renal tubular epithelial cells (Juan et al., 2021). In summary, the intercellular interactions mediated by exosome-derived ncRNAs may contribute to novel strategies for the treatment of sepsis.
In conclusion, the regulation of pyroptosis by ncRNAs plays a vital role in sepsis and may be a potential therapeutic target. However, there are still many gaps in this area, such as the lack of studies on circRNAs and exploring the potential roles of circRNAs and ncRNAs in other organ injuries during sepsis. These studies include those on sepsis-induced liver and brain injuries and the ncRNA regulation of caspase-1/4/5/11 and related clinical studies.
4.3 Regulation of pyroptosis by epigenetic modifications in sepsis
Epigenetic modifications are heritable changes in gene expression that do not change the nucleotide sequence of the gene. They include DNA and histone modifications. m6A methylation, which has attracted much attention in recent years, is an epigenetic modification. Studies have shown that various epigenetic modifications are closely related to the activation or inhibition of pyroptosis and ultimately affect pyroptosis-related diseases, including sepsis. For instance, Wang et al. found that emodin could upregulate the expression of methyltransferase-like 3 (METTL3) in sepsis-induced brain injury in vitro, thereby downregulating the mRNA and protein expression of NLRP3 and ultimately inhibiting pyroptosis and inflammation (Wang et al., 2022). In addition, m6A demethylase fat mass and obesity-associated proteins (FTOs) has also been found to regulate pyroptosis in sepsis. Luo et al. found that in a septic shock mouse models induced by LPS, FTO inhibition suppressed the NLRP3 inflammasome formation by inhibiting the FoxO1/NF-κB signaling pathway, thus reducing tissue damage and improving survival (Luo et al., 2021). Moreover, Liu et al. discovered that the level of the SET domain-containing histone methyltransferase Wolf-Hirschhorn syndrome candidate gene 1 (WHSC1) was elevated in LPS-induced septic lung injury, and WHSC1 knockout attenuated LPS-induced lung injury and alveolar macrophage pyroptosis. Further mechanistic studies have revealed that WHSC1 exacerbates alveolar macrophage pyroptosis in sepsis-induced ALI through the NEK7-mediated activation of the NLRP3 inflammasome (Liu et al., 2021). Previous studies have also shown that WHSC1 can regulate NEK7 via lysine dimethylation at position 36 of histone H3 (H3K36me2), thereby deteriorating head and neck squamous cell carcinoma (Saloura et al., 2015). Unfortunately, the detailed mechanisms by which WHSC1 regulates NEK7 expression in sepsis have not been explored and require further study. In conclusion, the modulation of pyroptosis by epigenetic modifications may be a new regulatory mechanism in the process of sepsis. However, in the future, many experimental studies are needed to explore the roles and mechanisms of various epigenetic modifications in regulating the expression of genes related to pyroptosis and the resulting impact on pyroptosis in sepsis.
4.4 Regulation of pyroptosis by PTMs in sepsis
PTMs refer to the chemical modifications of proteins after translation, including phosphorylation, acetylation, ubiquitination, glycosylation and succination. These play crucial roles in regulating protein activity. Previous studies have shown that various PTMs of pyroptosis-associated proteins influence NLRP3 inflammasome and pyroptosis (Song and Li, 2018; Kelley et al., 2019). For example, Humphries et al. found that dimethyl fumarate(DMF) or endogenous fumarate could modify GSDMD at critical cysteine residues to form S-(2-succinyl)-cysteine, resulting in inactivating GSDMD and inhibiting pyroptosis. In addition, they found that DMF could also succinate GSDME and inhibit GSDME-driven cell death comparably (Humphries et al., 2020). Besides, Mortimer et al. showed that the phosphorylation of NLRP3 at Ser295 (mouse NLRP3 Ser291) by protein kinase A (PKA) could inhibit NLRP3 inflammasome activation by inhibiting NLRP3 ATPase activity (Mortimer et al., 2016). However, the roles of PTMs in regulating pyroptosis during sepsis remain poorly understood. In 2020, Tang et al. found that the E3 ubiquitin ligase Casitas-B-lineage lymphoma protein-b (Cbl-b) was essential for preventing endotoxemia induced by a sub-lethal dose of LPS in a caspase-11/NLRP3-dependent manner (Tang et al., 2020). At the molecular level, the NLRP3 protein underwent both K63- and K48-linked polyubiquitination. The Cbl-b protein bound to the K63-ubiquitin chains attached to the NLRP3 leucine-rich repeat domain (LRR) via its ubiquitin-associated region (UBA) and targeted NLRP3 at K496 for K48-linked ubiquitination and proteasome-mediated degradation. The study also identified ring finger protein 125 as an additional E3 ubiquitin ligase that initiated the K63-linked ubiquitination of the NLRP3 LRR domain. In summary, the NLRP3 protein was sequentially ubiquitinated by K63- and K48-linked ubiquitination, thus preventing the NLRP3 inflammasome activation and restraining endotoxemia. Considering the profound effects of PTMs on pyroptosis-associated proteins, PTMs may be potential strategies to regulate pyroptosis and improve sepsis and should be studied in the future.
In summary, during sepsis, transcription factors, ncRNAs, epigenetic modifications, and PTMs all have regulatory effects on pyroptosis and ultimately result in the regulation of sepsis and SAOD. However, the detailed regulatory mechanisms remain unclear and require further study. In addition, most existing studies have been conducted at the cellular or animal level. Thus, further clinical studies are needed to evaluate the modulation of pyroptosis in patients with sepsis.
5. Interaction of pyroptosis with other programmed cell death pathways in sepsis
In 2018, the Nomenclature Committee on Cell Death issued the latest guidelines on the classification and nomenclature of cell death, dividing cell death into accidental cell death (ACD) and programmed cell death (PCD) (Galluzzi et al., 2018). ACD refers to the instantaneous and catastrophic death of cells exposed to severe physical, chemical, or mechanical insults. In contrast, PCD relies on dedicated molecular machinery and can occur under physiological or pathological conditions. PCD can be modulated by pharmacological or genetic interventions, which have attracted much attention in recent research on sepsis (Table 1). Numerous studies have shown that in addition to pyroptosis, PCD pathways such as autophagy, apoptosis, NETosis, necroptosis, and ferroptosis are involved in sepsis. Notably, pyroptosis and other PCD pathways are interdependent, rather than independent, in sepsis (Figure 4).
5.1 Interaction of pyroptosis and autophagy in sepsis
Autophagy is a conserved intracellular process that leads to the degradation and recycling of damaged cellular proteins and organelles and the destruction of intracellular pathogens (Mizushima and Levine, 2010; Ren et al., 2017). Autophagy contributes to the maintenance of cellular homeostasis and plays an important role in regulating immune and inflammatory responses. In sepsis-related studies, autophagy has been shown to participate in regulating NLRP3 inflammasome activation. For instance, Pu et al. reported that autophagy-related protein 7 (Atg7) is involved in inflammasome activation in sepsis induced by Pseudomonas aeruginosa. In septic Atg7fl/fl mice, IL-1β levels in the bronchoalveolar lavage fluid (BALF) and blood as well as ASC and IL-18 protein levels in the lungs were significantly elevated compared to those in the septic WT mice. In vitro, pyroptosis was increased in macrophages upon P. aeruginosa infection, as evidenced by increased caspase-1 and ASC protein levels. These results indicated that Atg7 plays an essential role in the inhibition of inflammasome activation. Mechanistically, flagellin, a conserved PAMP, may be a potential mediator of inflammasome hyperactivation in Atg7-deficient conditions during P. aeruginosa infection (Pu et al., 2017). Similarly, compared with WT bone marrow-derived macrophages (BMMs), autophagy-deficient Atg5-/-and Atg14-/- BMMs secrete elevated amounts of IL-1β and IL-18 proteins, forming ASC specks more frequently, after LPS treatment (Finethy et al., 2020). In addition, Ge et al. found that the inhibition of Beclin1 using Beclin1 small interfering RNA significantly enhanced the protein expression of NLRP3, ASC, caspase-1, and IL-1β in LPS-stimulated macrophages (Ge et al., 2019). Beclin1 is a protein required for the initiation of autophagy. Notably, there are various pathways involved in autophagy. Mitophagy is a selective autophagy that can specifically remove damaged or excess mitochondria and maintain cellular homeostasis (Wang et al., 2021b). In 2016, Kim et al. found that SESN2 could suppress sepsis by inducing mitophagy and inhibiting NLRP3 activation in macrophages. The molecular mechanism was that mitophagy could inhibit the excessive generation of mitochondrial ROS and release of mtDNA, which could activate the NLRP3 inflammasome and induce inflammation (Kim et al., 2016). Similarly, Li et al. revealed that bone marrow-derived mesenchymal stem cells (BMSCs) could restrict NLRP3 inflammasome activation by increasing mitophagy and decreasing mitochondrial ROS levels in macrophages. In C57BL/6 mice after CLP, BMSC treatment exerted beneficial effects, as evidenced by improved survival rates and multiorgan functions (Li et al., 2018). Liu et al. discovered that buformin (BF) could alleviate sepsis-induced acute lung injury by inhibiting NLRP3-mediated pyroptosis in vivo and in vitro. Further studies have found that BF inhibits pyroptosis in macrophages by enhancing autophagy via the AMPK-mTOR pathway. Notably, compared with the LPS + BF treatment, the autophagy inhibitor 3-MA upregulated the protein level of NLRP3, but not its mRNA level, suggesting that the autophagy promoted by BF most likely promoted the degradation of the NLRP3 inflammasome (Liu et al., 2022). In summary, autophagy can inhibit the activation of the NLRP3 inflammasome not only by removing endogenous NLRP3 inflammasome activators, such as ROS and DAMPs, but also by removing NLRP3 inflammasome components (Biasizzo and Kopitar-Jerala, 2020). Thus, the activation of autophagy may be a beneficial strategy for sepsis treatment. For instance, in a septic rat model, Yang et al. found that DEX could attenuate renal injury by significantly reducing the expression of NLRP3 inflammasome-mediated pyroptosis pathway proteins NLRP3, ASC, caspase-1, and cleaved caspase-1 and inflammatory factors IL-1β and IL-18. The molecular mechanism was that DEX enhanced autophagy via α2-AR/AMPK/mTOR signaling and subsequently inhibited the activation of the NLRP3 inflammasome (Yang et al., 2020). Similarly, in sepsis-induced acute lung injury, the knockdown of geranylgeranyl pyrophosphate synthase large subunit 1 was found to attenuate injury by suppressing NLRP3 inflammasome activity through the promotion of autophagy (Li et al., 2021).
Intriguingly, NLRP3 inflammasome-mediated pyroptosis regulates autophagy during sepsis. For instance, Jin et al. reported that similar levels of neutrophil recruitment to the peritoneum but improved survival were observed in NLRP3-/- mice compared with those of WT mice. Considering the critical role of neutrophils in bacterial clearance, it was speculated that there may be a difference in neutrophilic functions between WT and NLRP3-/- mice. Subsequent experiments showed that peritoneal cells (primarily neutrophils) and lung tissues from NLRP3-/- mice 24 h post-CLP displayed decreased autophagy, characterized by a reduction in Atg7 levels, inhibition of microtubule-associated protein light chain 3-II formation, and enhanced sequestosome-1 levels. The experiment also found that phagocytosis was augmented in NLRP3-/- neutrophils, which may have been associated with an increased expression of macrophage receptors with collagenous structures and mannose-binding lectins (Jin et al., 2017).
Taken together, pyroptosis and autophagy can interact and regulate the immune and inflammatory reactions in sepsis. In the future, targeting this relationship could be a possible treatment option for patients with sepsis.
5.2 Interaction of pyroptosis and apoptosis in sepsis
Although pyroptosis and apoptosis are both PCD mediated by caspase proteins, they differ in mechanisms, biological characteristics and functions. Pyroptosis is mediated by inflammatory caspase-1/4/5/11, whereas apoptosis is mediated by apoptotic caspase-2/3/6/7/8/9/10. During the process of pyroptosis, the cell nuclear condensation, pore formation on the cell membrane mediated by GSDM family, cell welling and then flatting and pyroptotic body can be observed, ultimately cell lysis and causing inflammation. However, during the process of apoptosis, chromatin condensation, nuclear fragmentation, cytoplasm shrinkage, formation of apoptotic bodies and cell membrane is intact without triggering inflammation. Nevertheless, it is worth noting that there is no strict boundary between the pathways involved in inflammatory and apoptotic caspases (Galluzzi et al., 2018). For example, in GSDMD-deficient macrophages, caspase-1 activation induces apoptosis, which is associated with the Bid/caspase-9/caspase-3 pathway (Tsuchiya et al., 2019). In addition, caspase-3 and -8 can cause pyroptosis by cleaving the gasdermin family proteins in tumor cells (Wang et al., 2017; Sarhan et al., 2018). Previous studies have demonstrated an interplay between pyroptosis and apoptosis in sepsis. For example, Sarkar et al. found that caspase-1 could regulate splenic B cell apoptosis independently of IL-1β and IL-18 (Sarkar et al., 2006). In addition, Li et al. found that stimulator of interferon genes (STING) silencing displayed obvious anti-apoptotic effects in LPS-treated neonatal rat cardiomyocytes (NRCMs). However, the upregulation of NLRP3 offset the anti-apoptotic effect of STING silencing, indicating that NLRP3 activation could trigger apoptosis in LPS-treated NRCMs (Li et al., 2019). Likewise, in sepsis-associated encephalopathy, Chen et al. showed that Maf1 protects against LPS-induced blood–brain barrier (BBB) disruption both in vitro and in vivo. At the molecular level, the Maf1 protein suppressed the NF-κB/NLRP3 inflammatory pathway by directly binding to the promoter region of NLRP3, thus reducing apoptosis and inflammation. The overexpression of NLRP3 reversed the protective effects of Maf1 against apoptosis and pyroptosis. These results demonstrated that NLRP3 is involved in promoting apoptosis in sepsis (Chen et al., 2020). Moreover, in SAKI, the inhibition of NLRP3 by siNLRP3 decreased the number of apoptotic cells, as detected using flow cytometry in LPS-treated renal tubular epithelial cells (Liu et al., 2020). In summary, these studies demonstrated that in sepsis, pyroptosis could promote apoptosis, thus aggravating inflammation and organ dysfunction. However, the detailed mechanisms, which may be associated with the caspases involved and inflammation factors produced, still need to be explored. In turn, pannexin-1 has been found to promote NLRP3 activation during apoptosis, whereas cytosolic cytochrome c released during apoptosis negatively regulates NLRP3 (Shi and Kehrl, 2016; Chen et al., 2020). Although the exact effects of apoptosis on pyroptosis in sepsis remain unclear, pyroptosis and apoptosis are closely associated with each other in that they share common regulatory factors, such as ROS and caspases.
5.3 Interaction of pyroptosis and NETosis in sepsis
Neutrophil extracellular traps (NETs) are extracellular structures composed of chromatin fibers mixed with granule-derived antimicrobial peptides and enzymes that can trap, immobilize, and kill pathogens and activate other immune cells (Burgener and Schroder, 2020). NETs are generated and released during a distinct process of cell death called NETosis, which differs from apoptosis and necrosis and plays an essential role in the innate immune response (Gupta and Kaplan, 2016; Thiam et al., 2020). Chen et al. revealed that NETs could induce macrophage (Mϕ) pyroptosis in sepsis, thereby amplifying inflammation. At the molecular level, RAGE and dynamin-dependent signaling initiates NET-released HMGB1 endocytosis. HMGB1, a DAMP molecule, triggers a cascade of molecular events, including cathepsin B release from ruptured lysosomes followed by caspase-1 activation and pyroptosis (Chen et al., 2018). Furthermore, Tian et al. discovered that citrullinated histone H3 (CitH3) could mediate sepsis-induced lung injury by activating the caspase-1-dependent pyroptosis pathway (Tian et al., 2021). As CitH3 is a key component released from cells during NETosis, it was speculated that NETosis could regulate pyroptosis. Recently, Silva et al. found that the inhibition of GSDMD with disulfiram abrogated NET formation and reduced multiple organ dysfunction and sepsis lethality. Mechanistically, the activation of the caspase-11/GSDMD pathway controls NET release by neutrophils during sepsis (Silva et al., 2021). In summary, pyroptosis and NETosis can be reciprocally promoted in sepsis and may play a crucial role in its regulation.
Necroptosis and ferroptosis may also interact with pyroptosis during sepsis. For instance, Chen et al. found that receptor-interacting serine/threonine-protein kinase 3-mediated necroptosis and GSDMD-mediated pyroptosis could collaborate to amplify inflammatory signaling and enhance tissue injury during sepsis (Chen et al., 2020a). In addition, glutathione peroxidase-4 was found to play a protective role by modulating both ferroptosis and pyroptosis in bacterial infections and polymicrobial sepsis, suggesting that they may be potential therapeutic targets for treating sepsis (Zhu et al., 2019).
In conclusion, pyroptosis and other PCD pathways interact with each other and do not occur independently. The interactions between PCD pathways regulate immune and inflammation reactions, sepsis, and SAOD. Thus, identifying the key molecules and pathways involved in their interactions may provide valuable targets for treatments.
6. Clinical significance and further prospects of pyroptosis in sepsis
Pyroptosis, a new type of PCD pathway, has been gradually recognized in recent years, and its role in various diseases has attracted increasing attention. However, the complicated molecular mechanisms of pyroptosis and its functions in the occurrence and development of different diseases remain unclear and need to be further explored. At present, existing studies exploring the influence of pyroptosis in diseases are mainly performed at the cellular and animal experimental levels. Few clinical studies have been performed, and the specific mechanisms of pyroptosis remain elusive. Therefore, further studies are warranted to reveal the mechanisms of pyroptosis and its relationship with diseases to provide effective strategies for clinical prevention and treatment. The studies discussed in this review strongly demonstrate that pyroptosis and its related pathways play critical roles in sepsis and SAOD, although many questions remain unsolved. Previous studies have shown that inhibiting pyroptosis exerts protective effects on SAOD and may be a promising approach for the clinical treatment of SAOD. However, studies focusing on targeting pyroptosis as a therapy are still limited to the experimental level. Further clinical studies should be conducted to determine the effects of pyroptosis and possibility of its clinical application. Furthermore, studies on the involvement of ncRNAs and exosomes in pyroptosis in the regulation of sepsis may provide insights for future research, as they may be potential diagnostic or therapeutic targets. Pyroptosis may provide a novel approach for the diagnosis and treatment of sepsis, although there are still many challenges to be overcome.
6.1 Pyroptosis-related proteins as indicators to evaluate the severity of sepsis and SAODs
According to the Surviving Sepsis Campaign: International Guidelines for the Management of Sepsis and Septic Shock 2021, sepsis is one of the leading causes of death in both pediatric and adult intensive care units worldwide. Moreover, the prognosis of patients with sepsis accompanied by organ dysfunction are even worse. Early diagnosis is essential for implementing treatments that prevent the progression of sepsis and reduce mortality (Evans et al., 2021). As current studies reported, the changes of pyroptosis-related proteins are detected in different organs based on septic animal models and/or in blood samples from patients with sepsis, indicating that these proteins are related with sepsis and the dysfunction of organs. Thus, it is speculated that pyroptosis-related proteins may have potential roles to help diagnose sepsis or predict the severity of sepsis and its related organ dysfunction, which may be used with other serum biomarkers at the same time. Previous studies have shown that pyroptosis-related indicators can serve as markers for diagnosing and predicting the development of sepsis. For instance, Watany et al. measured the serum levels of IL-31, IL-1β, and NLRP3 in 149 participants (38 with sepsis; 51 with systemic inflammatory response syndrome; 30 with septic shock; and 30 healthy controls) in a case-control study. The results demonstrated that the three biomarkers could be independent prognostic biomarkers. A panel of combined markers further yielded 100% sensitivity and specificity, suggesting that the combination of IL-31, IL-1β, and NLRP3 may provide a promising diagnostic and prognostic panel for sepsis (Watany et al., 2020). In addition, Cui et al. reported that the absolute number and percentage of ASC-speck+ monocytes increased on days 6 and 7 of sepsis, and patients with lower absolute numbers of ASC-speck+ monocytes on day 6 showed poor 90-day survival (Cui et al., 2020). In addition, a prospective study involving 60 trauma patients and 10 healthy controls revealed that the pyroptosis of PBMCs was a potential marker for predicting the development of sepsis after trauma (Wang et al., 2018). Furthermore, Lu et al. discovered that the 29940 G-to-C mutation within the NLRP3 3′-UTR was a gain-of-function alteration which ultimately protected patients against susceptibility to sepsis progression and poor prognosis. At the molecular level, the G-to-C mutant could bind with miR-146a, thus suppressing NLRP3 expression and downstream inflammatory cytokine production (Lu et al., 2021). These studies demonstrate that pyroptosis-related indicators may be potential biomarkers for the diagnosis and prediction of sepsis. In the future, studies should be conducted to dynamically monitor the expression level of these proteins in animal and clinical samples, in order to find the best time point to detect the proteins. Furthermore, correlation analysis should be made between the pyroptosis-related indicators and other biomarkers, so as to explore whether these indicators are useful in the diagnosis of sepsis and sepsis-associated organ dysfunction.
6.2 Pyroptosis as a therapeutic target in sepsis
Although the mortality rate of sepsis has decreased with the advancement of medical technologies, sepsis and septic shock remain major healthcare problems. Epidemiological studies have reported that sepsis and septic shock affect millions of people worldwide every year, resulting in one-third to one-sixth of all deaths (Fleischmann et al., 2016; Rhee et al., 2017; Fleischmann-Struzek et al., 2020). Furthermore, the prognosis of patients with SAOD is worse. Thus, effective therapies are critically important in the treatment of sepsis. At present, the clinical treatments of sepsis are mainly comprehensive, such as fluid resuscitation and antibiotic administration. However, given the heterogeneity of individuals, individualized and precise treatments are needed to further improve the cure rate of sepsis and prognoses of patients. Pyroptosis, which is involved in inflammation and immune regulation, provides novel insights for treating inflammation and immune-related diseases, including sepsis (Chen et al., 2021; Wang et al., 2020). For example, Chen et al. discovered that nitrosonisoldipine (NTS) protected against sepsis in mice by inhibiting both the canonical and non-canonical inflammasome pathways. Mechanistically, NTS directly inhibited the enzymatic activities of inflammatory caspase-1, -4, and -11. Furthermore, NTS was safe for intraperitoneal administration, suggesting that targeting pyroptosis may be useful for treating sepsis (Chen et al., 2021). Notably, there are many intervention targets for regulating pyroptosis, including the activation and assembly of inflammasomes and cleaving of pro-caspases and gasdermins. For instance, Fu et al. showed that the NLRP3 inhibitor MCC950 and caspase-1 inhibitor Ac-YVAD-CMK ameliorated increased hippocampal neuronal pyroptosis and reduced the levels of pro-inflammatory cytokines and rescued cognitive deficits in mice with sepsis-associated encephalopathy (Fu et al., 2019). Besides, there are several drugs reported to target GSDMD directly and block pyroptosis, including necrosulfonamide (NSA), disulfiram and DL-3-n-butylphthalide (Rathkey et al., 2018; Hu et al., 2020; Han et al., 2021). For instance, Rathkey et al. reported that NSA treatment attenuated release of inflammatory cytokine and significantly prolonged survival in a mouse model of sepsis, providing the basis for developing novel therapies for sepsis and other inflammatory diseases (Rathkey et al., 2018). Interestingly, the involvement of ncRNAs and exosomes in regulating pyroptosis in sepsis has provided novel insights for developing precise treatments. For example, Jiao et al. found that neutrophil-derived exosomal miR-30d-5p could lead to macrophage pyroptosis by activating NF-κB, ultimately aggravating sepsis-induced acute lung injury (Jiao et al., 2021). In addition, Juan et al. revealed that M1 macrophage-derived exosomal miR-93-5p could improve SAKI by inhibiting pyroptosis in renal epithelial cells by directly targeting TXNIP (Juan et al., 2021). Exosomes are a type of natural carriers that are promising therapeutic tools. NcRNAs can interact with proteins and participate in regulating various pathways, which represent a large number of potential therapeutic targets. These characteristics provide a basis for developing precise treatments. Unfortunately, at present, studies are limited to the cellular and pre-clinical levels. Furthermore, appropriate pyroptosis-associated targets and the most relevant ncRNAs and exosomes are not well understood and remain to be explored, especially in animal experiments in vivo and clinical trials.
6.3 Further prospects of pyroptosis in sepsis
Pyroptosis plays important roles in the pathogenesis, progression, and prognosis of sepsis and is a potential diagnostic and predictive marker and promising therapeutic target. However, many questions remain to be answered before its clinical application. Among them, the most important and urgent problem is the lack of a thorough and comprehensive understanding of pyroptosis in sepsis and SAOD. Considering that pyroptosis can protect or aggravate sepsis, clarifying the role of pyroptosis in a specific state may facilitate providing positive or negative interventions. In the future, it will be necessary to carry out a large number of studies to continuously monitor the changes in the expression of pyroptosis-related indicators. The trends of clinical diagnoses and prognoses should also be comprehensively analyzed to investigate the criteria for evaluating the effect of pyroptosis and guide treatments. Furthermore, the molecular mechanisms of pyroptosis are complicated. For example, in addition to the NLRP3 inflammasome, NLR family CARD domain-containing protein 4 and absent in melanoma 2 inflammasomes can also mediate the canonical pyroptosis pathway, while the members of the gasdermin family, such as GSDME, can be cleaved and activated, subsequently forming pores on cell membranes (Guo et al., 2018). Exploring the pyroptosis-associated molecules that play leading roles in different cells under various stimuli will therefore provide the basis for treating sepsis and SAOD by regulating pyroptosis. Gene editing technologies, such as knockout or knockin of proteins in vivo, may be helpful in achieving this goal. Since pyroptosis and other PCD pathways control the fate of cells together, exploring their interactions and finding potential key molecules are of great significance to ensure a better outcome in pyroptosis after intervention. In addition, studies have shown that GSDMD can form pores in the mitochondrial membrane, suggesting that pyroptosis is involved in energy and metabolic regulation. Gene knockdown and/or overexpression, combined with metabolomics and proteomics, may provide novel insights into these potential functions. Finally, most interventions for pyroptosis in existing studies have poor specificity. In addition to a few studies using specific inhibitors, such as MCC950 and Ac-YVAD-CMK, most studies have shown regulation of pyroptosis by influencing the NF-κB pathway and production of ROS. In addition, investigating the modulation of transcription factors, PTMs, ncRNAs, and epigenetics on key molecules in the pyroptosis pathways using transcriptomics, methylation modifications, and PTM omics may provide novel clues for developing targeted drugs. There is no doubt that pyroptosis is a promising therapeutic strategy for sepsis; however, further studies are warranted to confirm this possibility.
7. Conclusion and future prospects
Since the discovery of pyroptosis, its understanding has become increasingly comprehensive and profound. In recent years, many studies have shown that pyroptosis participates in various diseases, including sepsis. This review contributes to a better understanding of the role and mechanisms of pyroptosis in sepsis and SAOD. The studies described herein provide strong evidence that pyroptosis plays an important role in sepsis; however, the detailed molecular mechanisms of pyroptosis are not well understood. Future research should focus on whether the canonical or non-canonical pyroptosis pathways are dominant in various cells under different stimuli and the inflammasomes that are involved in the canonical pyroptosis pathway. Recent advancements in technologies for gene knockout and knockin may facilitate the elucidation of the specific molecular mechanisms of pyroptosis in sepsis, providing new perspectives for the pathogenesis of sepsis and targets for treatments.
Considering that the role of pyroptosis varies in different stages of sepsis, it will be of great significance to pay attention to the mechanisms that regulate pyroptosis at multiple levels, such as epigenetics, transcription, and post-transcription. These mechanisms will provide further evidence for pyroptosis as a new factor in sepsis treatments. The application of omics technologies, such as proteomics, PTM omics, and bioinformatics, may offer clues for exploring the effects of these regulatory factors on pyroptosis and guide future experiments. In addition, further studies are needed before pyroptosis-related indicators can be used as biomarkers. The interactions between PCD pathways affect the fate of cells as well as inflammatory and immune responses. Exploring these potential links and their effects on sepsis will help develop novel treatment targets. Furthermore, exosomes carrying inflammasome components or ncRNAs can mediate the interactions between peripheral immune cells and organ-resident immune cells, as well as immune cells and organs, which may provide further potential therapeutic strategies.
Determining the role of pyroptosis in sepsis will provide new insights into its pathogenesis, diagnosis, and treatment. However, much research, especially clinical research, is needed to explore and identify the accurate molecular mechanisms and clinical value of pyroptosis in sepsis. This will contribute to revealing the diagnostic and therapeutic value of pyroptosis in sepsis. Future studies on the involvement of pyroptosis in sepsis may assess the following:
1) Detecting changes in severity and exploring the role of pyroptosis in all stages of sepsis and SAOD;
2) Involvement of various pyroptosis pathways in sepsis and SAOD;
3) Factors regulating pyroptosis at multiple levels;
4) Interactions among pyroptosis and other PCD pathways in sepsis;
5) Verifying pyroptosis-associated biomarkers for the early diagnosis of sepsis;
6) Experiments in vivo and clinical trials to explore therapeutic targets based on pyroptosis.
Author contributions
All authors participated in drafting or revising of the manuscript and approved the final manuscript.
Funding
This work was supported by the National Natural Science Foundation of China (82102254 to T-NZ and 82002021 to NY), the Doctoral Start-up Foundation of Liaoning Province (2021-BS-107 to T-NZ), and 345 Talent Program of Shengjing Hospital of China Medical University (M0691 to T-NZ and M0959 to NY).
Acknowledgments
All figures are created with BioRender.com.
Conflict of Interest
The authors declare that the research was conducted in the absence of any commercial or financial relationships that could be construed as a potential conflict of interest.
Publisher’s note
All claims expressed in this article are solely those of the authors and do not necessarily represent those of their affiliated organizations, or those of the publisher, the editors and the reviewers. Any product that may be evaluated in this article, or claim that may be made by its manufacturer, is not guaranteed or endorsed by the publisher.
Supplementary material
The Supplementary Material for this article can be found online at: https://www.frontiersin.org/articles/10.3389/fcimb.2022.962139/full#supplementary-material
Supplementary Figure 1 | Expression of the gasdermins family in different organs.
References
Aglietti, R. A., Estevez, A., Gupta, A., Ramirez, M. G., Liu, P. S., Kayagaki, N., et al. (2016). GsdmD p30 elicited by caspase-11 during pyroptosis forms pores in membranes. Proc. Natl. Acad. Sci. U. S. A. 113 (28), 7858–7863. doi: 10.1073/pnas.1607769113
An, L., Yang, T., Zhong, Y., Yin, Y., Li, W., Gao, H. (2021). Molecular pathways in sepsis-induced cardiomyocyte pyroptosis: Novel finding on long non-coding RNA ZFAS1/miR-138-5p/SESN2 axis. Immunol. Lett. 238, 47–56. doi: 10.1016/j.imlet.2021.07.003
Barriere, S. L., Lowry, S. F. (1995). An overview of mortality risk prediction in sepsis. Crit. Care Med. 23 (2), 376–393. doi: 10.1097/00003246-199502000-00026
Biasizzo, M., Kopitar-Jerala, N. (2020). Interplay between NLRP3 inflammasome and autophagy. Front. Immunol. 11. doi: 10.3389/fimmu.2020.591803
Boomer, J. S., To, K., Chang, K. C., Takasu, O., Osborne, D. F., Walton, A. H., et al. (2011). Immunosuppression in patients who die of sepsis and multiple organ failure. JAMA 306 (23), 2594–2605. doi: 10.1001/jama.2011.1829
Borkiewicz, L., Kalafut, J., Dudziak, K., Przybyszewska-Podstawka, A., Telejko, I. (2021). Decoding LncRNAs. Cancers (Basel) 13 (11):2643. doi: 10.3390/cancers13112643
Burgener, S. S., Schroder, K. (2020). Neutrophil extracellular traps in host defense. Cold Spring Harb. Perspect. Biol. 12 (7):a037028. doi: 10.1101/cshperspect.a037028
Busch, K., Kny, M., Huang, N., Klassert, T. E., Stock, M., Hahn, A., et al. (2021). Inhibition of the NLRP3/IL-1beta axis protects against sepsis-induced cardiomyopathy. J. Cachexia Sarcopenia Muscle 12 (6), 1653–1668. doi: 10.1002/jcsm.12763
Chen, K. W., Demarco, B., Broz, P. (2020). Pannexin-1 promotes NLRP3 activation during apoptosis but is dispensable for canonical or noncanonical inflammasome activation. Eur. J. Immunol. 50 (2), 170–177. doi: 10.1002/eji.201948254
Cheng, K. T., Xiong, S., Ye, Z., Hong, Z., Di, A., Tsang, K. M., et al. (2017). Caspase-11-mediated endothelial pyroptosis underlies endotoxemia-induced lung injury. J. Clin. Invest. 127 (11), 4124–4135. doi: 10.1172/JCI94495
Chen, Y. F., Hu, F., Wang, X. G., Tang, Z., Tang, H. X., Xu, M. (2021). MicroRNA-23a-5p is involved in the regulation of lipopolysaccharide-induced acute lung injury by targeting HSP20/ASK1. Oxid. Med. Cell. Longev. 2021, 9942557. doi: 10.1155/2021/9942557
Chen, Q., Kang, J., Fu, C. (2018). The independence of and associations among apoptosis, autophagy, and necrosis. Signal Transduct. Target. Ther. 3, 18. doi: 10.1038/s41392-018-0018-5
Chen, H., Li, Y., Wu, J., Li, G., Tao, X., Lai, K., et al. (2020a). RIPK3 collaborates with GSDMD to drive tissue injury in lethal polymicrobial sepsis. Cell Death Differ. 27 (9), 2568–2585. doi: 10.1038/s41418-020-0524-1
Chen, Y., Luo, R., Li, J., Wang, S., Ding, J., Zhao, K., et al. (2022). Intrinsic radical species scavenging activities of tea polyphenols nanoparticles block pyroptosis in endotoxin-induced sepsis. ACS Nano 16 (2), 2429–2441. doi: 10.1021/acsnano.1c08913
Chen, S., Tang, C., Ding, H., Wang, Z., Liu, X., Chai, Y., et al. (2020). Maf1 ameliorates sepsis-associated encephalopathy by suppressing the NF-kB/NLRP3 inflammasome signaling pathway. Front. Immunol. 11. doi: 10.3389/fimmu.2020.594071
Chen, Q., Yang, Y., Hou, J., Shu, Q., Yin, Y., Fu, W., et al. (2019). Increased gene copy number of DEFA1/DEFA3 worsens sepsis by inducing endothelial pyroptosis. Proc. Natl. Acad. Sci. U. S. A. 116 (8), 3161–3170. doi: 10.1073/pnas.1812947116
Chen, R., Zeng, L., Zhu, S., Liu, J., Zeh, H. J., Kroemer, G., et al. (2019). CAMP metabolism controls caspase-11 inflammasome activation and pyroptosis in sepsis. Sci. Adv. 5 (5), v5562. doi: 10.1126/sciadv.aav5562
Chen, L., Zhao, Y., Lai, D., Zhang, P., Yang, Y., Li, Y., et al. (2018). Neutrophil extracellular traps promote macrophage pyroptosis in sepsis. Cell Death Dis. 9 (6), 597. doi: 10.1038/s41419-018-0538-5
Chen, Q., Zheng, J., Wang, D., Liu, Q., Kang, L., Gao, X., et al. (2021). Nitrosonisoldipine is a selective inhibitor of inflammatory caspases and protects against pyroptosis and related septic shock. Eur. J. Immunol. 51 (5), 1234–1245. doi: 10.1002/eji.202048937
Chen, L., Zhong, X., Cao, W., Mao, M., Li, W., Yang, H., et al. (2021b). JQ1 as a BRD4 inhibitor blocks inflammatory pyroptosis-related acute colon injury induced by LPS. Front. Immunol. 12. doi: 10.3389/fimmu.2021.609319
Chi, T. F., Khoder-Agha, F., Mennerich, D., Kellokumpu, S., Miinalainen, I., Kietzmann, T., et al. (2020). Loss of USF2 promotes proliferation, migration and mitophagy in a redox-dependent manner. Redox Biol. 37, 101750. doi: 10.1016/j.redox.2020.101750
Cookson, B. T., Brennan, M. A. (2001). Pro-inflammatory programmed cell death. Trends Microbiol. 9 (3), 113–114. doi: 10.1016/s0966-842x(00)01936-3
Cui, J., Oehrl, S., Ahmad, F., Brenner, T., Uhle, F., Nusshag, C., et al. (2020). Detection of in vivo inflammasome activation for predicting sepsis mortality. Front. Immunol. 11. doi: 10.3389/fimmu.2020.613745
Das, G., Shravage, B. V., Baehrecke, E. H. (2012). Regulation and function of autophagy during cell survival and cell death. Cold Spring Harb. Perspect. Biol. 4 (6):a008813. doi: 10.1101/cshperspect.a008813
Deng, H., Chen, F., Wang, Y., Jiang, H., Dong, Z., Yuan, B., et al. (2020). The role of activated NLRP3 inflammatory body in acute kidney injury in rats caused by sepsis and NLRP3-TXNIP signaling pathway. Saudi J. Biol. Sci. 27 (5), 1251–1259. doi: 10.1016/j.sjbs.2020.03.018
Deng, M., Tang, Y., Li, W., Wang, X., Zhang, R., Zhang, X., et al. (2018). The endotoxin delivery protein HMGB1 mediates caspase-11-Dependent lethality in sepsis. Immunity 49 (4), 740–753. doi: 10.1016/j.immuni.2018.08.016
Deng, J., Tan, W., Luo, Q., Lin, L., Zheng, L., Yang, J. (2021). Long non-coding RNA MEG3 promotes renal tubular epithelial cell pyroptosis by regulating the miR-18a-3p/GSDMD pathway in lipopolysaccharide-induced acute kidney injury. Front. Physiol. 12. doi: 10.3389/fphys.2021.663216
Ding, J., Shao, F. (2017). SnapShot: The noncanonical inflammasome. Cell 168 (3), 544. doi: 10.1016/j.cell.2017.01.008
Evans, L., Rhodes, A., Alhazzani, W., Antonelli, M., Coopersmith, C. M., French, C., et al. (2021). Surviving sepsis campaign: International guidelines for management of sepsis and septic shock 2021. Intensive Care Med. 47 (11), 1181–1247. doi: 10.1007/s00134-021-06506-y
Evavold, C. L., Ruan, J., Tan, Y., Xia, S., Wu, H., Kagan, J. C. (2018). The pore-forming protein gasdermin d regulates interleukin-1 secretion from living macrophages. Immunity 48 (1), 35–44. doi: 10.1016/j.immuni.2017.11.013
Finethy, R., Dockterman, J., Kutsch, M., Orench-Rivera, N., Wallace, G. D., Piro, A. S., et al. (2020). Dynamin-related irgm proteins modulate LPS-induced caspase-11 activation and septic shock. EMBO Rep. 21 (11), e50830. doi: 10.15252/embr.202050830
Fleischmann, C., Scherag, A., Adhikari, N. K., Hartog, C. S., Tsaganos, T., Schlattmann, P., et al. (2016). Assessment of global incidence and mortality of hospital-treated sepsis. current estimates and limitations. Am. J. Respir. Crit. Care Med. 193 (3), 259–272. doi: 10.1164/rccm.201504-0781OC
Fleischmann-Struzek, C., Mellhammar, L., Rose, N., Cassini, A., Rudd, K. E., Schlattmann, P., et al. (2020). Incidence and mortality of hospital- and ICU-treated sepsis: Results from an updated and expanded systematic review and meta-analysis. Intensive Care Med. 46 (8), 1552–1562. doi: 10.1007/s00134-020-06151-x
Flusberg, D. A., Sorger, P. K. (2015). Surviving apoptosis: Life-death signaling in single cells. Trends Cell Biol. 25 (8), 446–458. doi: 10.1016/j.tcb.2015.03.003
Fu, Q., Wu, J., Zhou, X. Y., Ji, M. H., Mao, Q. H., Li, Q., et al. (2019). NLRP3/Caspase-1 pathway-induced pyroptosis mediated cognitive deficits in a mouse model of sepsis-associated encephalopathy. Inflammation 42 (1), 306–318. doi: 10.1007/s10753-018-0894-4
Galluzzi, L., Baehrecke, E. H., Ballabio, A., Boya, P., Bravo-San, P. J., Cecconi, F., et al. (2017). Molecular definitions of autophagy and related processes. EMBO J. 36 (13), 1811–1836. doi: 10.15252/embj.201796697
Galluzzi, L., Vitale, I., Aaronson, S. A., Abrams, J. M., Adam, D., Agostinis, P., et al. (2018). Molecular mechanisms of cell death: Recommendations of the nomenclature committee on cell death 2018. Cell Death Differ. 25 (3), 486–541. doi: 10.1038/s41418-017-0012-4
Garnacho-Montero, J., Palacios-Garcia, I., Diaz-Martin, A., Gutierrez-Pizarraya, A., Lopez-Sanchez, J. M., Gomez, E. A., et al. (2020). Sequential changes of NLRP3 inflammasome activation in sepsis and its relationship with death. Shock 54 (3), 294–300. doi: 10.1097/SHK.0000000000001521
Ge, Y., Xu, X., Liang, Q., Xu, Y., Huang, M. (2019). Alpha-mangostin suppresses NLRP3 inflammasome activation via promoting autophagy in LPS-stimulated murine macrophages and protects against CLP-induced sepsis in mice. Inflamm. Res. 68 (6), 471–479. doi: 10.1007/s00011-019-01232-0
Ghafouri-Fard, S., Khoshbakht, T., Hussen, B. M., Taheri, M., Arefian, N. (2021). Regulatory role of non-coding RNAs on immune responses during sepsis. Front. Immunol. 12. doi: 10.3389/fimmu.2021.798713
Gong, T., Yang, Y., Jin, T., Jiang, W., Zhou, R. (2018). Orchestration of NLRP3 inflammasome activation by ion fluxes. Trends Immunol. 39 (5), 393–406. doi: 10.1016/j.it.2018.01.009
Grootjans, S., Vanden, B. T., Vandenabeele, P. (2017). Initiation and execution mechanisms of necroptosis: An overview. Cell Death Differ. 24 (7), 1184–1195. doi: 10.1038/cdd.2017.65
Guo, T., Jiang, Z. B., Tong, Z. Y., Zhou, Y., Chai, X. P., Xiao, X. Z. (2020). Shikonin ameliorates LPS-induced cardiac dysfunction by SIRT1-dependent inhibition of NLRP3 inflammasome. Front. Physiol. 11. doi: 10.3389/fphys.2020.570441
Guo, H., Ni, M., Xu, J., Chen, F., Yao, Z., Yao, Y., et al. (2021). Transcriptional enhancement of GBP-5 by BATF aggravates sepsis-associated liver injury via NLRP3 inflammasome activation. FASEB J. 35 (6), e21672. doi: 10.1096/fj.202100234R
Guo, Q., Wu, Y., Hou, Y., Liu, Y., Liu, T., Zhang, H., et al. (2018). Cytokine secretion and pyroptosis of thyroid follicular cells mediated by enhanced NLRP3, NLRP1, NLRC4, and AIM2 inflammasomes are associated with autoimmune thyroiditis. Front. Immunol. 9. doi: 10.3389/fimmu.2018.01197
Gupta, S., Kaplan, M. J. (2016). The role of neutrophils and NETosis in autoimmune and renal diseases. Nat. Rev. Nephrol. 12 (7), 402–413. doi: 10.1038/nrneph.2016.71
Hagar, J. A., Powell, D. A., Aachoui, Y., Ernst, R. K., Miao, E. A. (2013). Cytoplasmic LPS activates caspase-11: Implications in TLR4-independent endotoxic shock. Science 341 (6151), 1250–1253. doi: 10.1126/science.1240988
Han, B., Xu, J., Shi, X., Zheng, Z., Shi, F., Jiang, F., et al. (2021). DL-3-n-Butylphthalide attenuates myocardial hypertrophy by targeting gasdermin d and inhibiting gasdermin d mediated inflammation. Front. Pharmacol. 12. doi: 10.3389/fphar.2021.688140
He, X., Fan, X., Bai, B., Lu, N., Zhang, S., Zhang, L. (2021). Pyroptosis is a critical immune-inflammatory response involved in atherosclerosis. Pharmacol. Res. 165, 105447. doi: 10.1016/j.phrs.2021.105447
Ho, J., Chan, H., Wong, S. H., Wang, M. H., Yu, J., Xiao, Z., et al. (2016). The involvement of regulatory non-coding RNAs in sepsis: A systematic review. Crit. Care 20 (1), 383. doi: 10.1186/s13054-016-1555-3
Hotchkiss, R. S., Karl, I. E. (2003). The pathophysiology and treatment of sepsis. N. Engl. J. Med. 348 (2), 138–150. doi: 10.1056/NEJMra021333
Hsu, S. K., Li, C. Y., Lin, I. L., Syue, W. J., Chen, Y. F., Cheng, K. C., et al. (2021). Inflammation-related pyroptosis, a novel programmed cell death pathway, and its crosstalk with immune therapy in cancer treatment. Theranostics 11 (18), 8813–8835. doi: 10.7150/thno.62521
Huang, W. (2017). MicroRNAs: Biomarkers, diagnostics, and therapeutics. Methods Mol. Biol. 1617, 57–67. doi: 10.1007/978-1-4939-7046-9_4
Huang, X., Feng, Z., Jiang, Y., Li, J., Xiang, Q., Guo, S., et al. (2019). VSIG4 mediates transcriptional inhibition of Nlrp3 and il-1beta in macrophages. Sci. Adv. 5 (1), u7426. doi: 10.1126/sciadv.aau7426
Huang, Y., Zang, K., Shang, F., Guo, S., Gao, L., Zhang, X. (2020). HMGB1 mediates acute liver injury in sepsis through pyroptosis of liver macrophages. Int. J. Burns Trauma 10 (3), 60–67.
Huet, O., Pickering, R. J., Tikellis, C., Latouche, C., Long, F., Kingwell, B., et al. (2017). Protective effect of inflammasome activation by hydrogen peroxide in a mouse model of septic shock. Crit. Care Med. 45 (2), e184–e194. doi: 10.1097/CCM.0000000000002070
Hu, J. J., Liu, X., Xia, S., Zhang, Z., Zhang, Y., Zhao, J., et al. (2020). FDA-Approved disulfiram inhibits pyroptosis by blocking gasdermin d pore formation. Nat. Immunol. 21 (7), 736–745. doi: 10.1038/s41590-020-0669-6
Humphries, F., Shmuel-Galia, L., Ketelut-Carneiro, N., Li, S., Wang, B., Nemmara, V. V., et al. (2020). Succination inactivates gasdermin d and blocks pyroptosis. Science 369 (6511), 1633–1637. doi: 10.1126/science.abb9818
Jiao, Y., Zhang, T., Zhang, C., Ji, H., Tong, X., Xia, R., et al. (2021). Exosomal miR-30d-5p of neutrophils induces M1 macrophage polarization and primes macrophage pyroptosis in sepsis-related acute lung injury. Crit. Care 25 (1), 356. doi: 10.1186/s13054-021-03775-3
Jin, L., Batra, S., Jeyaseelan, S. (2017). Deletion of nlrp3 augments survival during polymicrobial sepsis by decreasing autophagy and enhancing phagocytosis. J. Immunol. 198 (3), 1253–1262. doi: 10.4049/jimmunol.1601745
Juan, C. X., Mao, Y., Cao, Q., Chen, Y., Zhou, L. B., Li, S., et al. (2021). Exosome-mediated pyroptosis of miR-93-TXNIP-NLRP3 leads to functional difference between M1 and M2 macrophages in sepsis-induced acute kidney injury. J. Cell. Mol. Med. 25 (10), 4786–4799. doi: 10.1111/jcmm.16449
Kalbitz, M., Fattahi, F., Grailer, J. J., Jajou, L., Malan, E. A., Zetoune, F. S., et al. (2016). Complement-induced activation of the cardiac NLRP3 inflammasome in sepsis. FASEB J. 30 (12), 3997–4006. doi: 10.1096/fj.201600728R
Kambara, H., Liu, F., Zhang, X., Liu, P., Bajrami, B., Teng, Y., et al. (2018). Gasdermin d exerts anti-inflammatory effects by promoting neutrophil death. Cell Rep. 22 (11), 2924–2936. doi: 10.1016/j.celrep.2018.02.067
Kayagaki, N., Stowe, I. B., Lee, B. L., O'Rourke, K., Anderson, K., Warming, S., et al. (2015). Caspase-11 cleaves gasdermin d for non-canonical inflammasome signalling. Nature 526 (7575), 666–671. doi: 10.1038/nature15541
Kayagaki, N., Warming, S., Lamkanfi, M., Vande, W. L., Louie, S., Dong, J., et al. (2011). Non-canonical inflammasome activation targets caspase-11. Nature 479 (7371), 117–121. doi: 10.1038/nature10558
Kelley, N., Jeltema, D., Duan, Y., He, Y. (2019). The NLRP3 inflammasome: An overview of mechanisms of activation and regulation. Int. J. Mol. Sci. 20 (13):3328. doi: 10.3390/ijms20133328
Kim, M. J., Bae, S. H., Ryu, J. C., Kwon, Y., Oh, J. H., Kwon, J., et al. (2016). SESN2/sestrin2 suppresses sepsis by inducing mitophagy and inhibiting NLRP3 activation in macrophages. Autophagy 12 (8), 1272–1291. doi: 10.1080/15548627.2016.1183081
Lai, D., Tang, J., Chen, L., Fan, E. K., Scott, M. J., Li, Y., et al. (2018). Group 2 innate lymphoid cells protect lung endothelial cells from pyroptosis in sepsis. Cell Death Dis. 9 (3), 369. doi: 10.1038/s41419-018-0412-5
Lechtenberg, B. C., Mace, P. D., Riedl, S. J. (2014). Structural mechanisms in NLR inflammasome signaling. Curr. Opin. Struct. Biol. 29, 17–25. doi: 10.1016/j.sbi.2014.08.011
Lei, Y., Zhou, R., Sun, X., Tang, F., Gao, H., Chen, L., et al. (2021). The pannexin-1 channel regulates pyroptosis through autophagy in a mouse model of sepsis-associated encephalopathy. Ann. Transl. Med. 9 (24), 1802. doi: 10.21037/atm-21-6579
Liang, G., Zeng, M., Gao, M., Xing, W., Jin, X., Wang, Q., et al. (2022). LncRNA IGF2-AS regulates nucleotide metabolism by mediating HMGA1 to promote pyroptosis of endothelial progenitor cells in sepsis patients. Oxid. Med. Cell. Longev. 2022, 9369035. doi: 10.1155/2022/9369035
Li, J., Bai, Y., Tang, Y., Wang, X., Cavagnaro, M. J., Li, L., et al. (2022). A 4-Benzene-Indol derivative alleviates LPS-induced acute lung injury through inhibiting the NLRP3 inflammasome. Front. Immunol. 13. doi: 10.3389/fimmu.2022.812164
Li, L. L., Dai, B., Sun, Y. H., Zhang, T. T. (2020). The activation of IL-17 signaling pathway promotes pyroptosis in pneumonia-induced sepsis. Ann. Transl. Med. 8 (11), 674. doi: 10.21037/atm-19-1739
Li, P., Li, M., Lindberg, M. R., Kennett, M. J., Xiong, N., Wang, Y. (2010). PAD4 is essential for antibacterial innate immunity mediated by neutrophil extracellular traps. J. Exp. Med. 207 (9), 1853–1862. doi: 10.1084/jem.20100239
Li, Z., Liu, T., Feng, Y., Tong, Y., Jia, Y., Wang, C., et al. (2022). PPARgamma alleviates sepsis-induced liver injury by inhibiting hepatocyte pyroptosis via inhibition of the ROS/TXNIP/NLRP3 signaling pathway. Oxid. Med. Cell. Longev. 2022, 1269747. doi: 10.1155/2022/1269747
Li, D., Li, C., Wang, T., Zhang, C., Zhu, Z., Zhang, G., et al. (2021). Geranylgeranyl diphosphate synthase 1 knockdown suppresses NLRP3 inflammasome activity via promoting autophagy in sepsis-induced acute lung injury. Int. Immunopharmacol. 100, 108106. doi: 10.1016/j.intimp.2021.108106
Lima, H. J., Jacobson, L. S., Goldberg, M. F., Chandran, K., Diaz-Griffero, F., Lisanti, M. P., et al. (2013). Role of lysosome rupture in controlling Nlrp3 signaling and necrotic cell death. Cell Cycle 12 (12), 1868–1878. doi: 10.4161/cc.24903
Li, J., Ma, J., Li, M., Tao, J., Chen, J., Yao, C., et al. (2021). GYY4137 alleviates sepsis-induced acute lung injury in mice by inhibiting the PDGFRbeta/Akt/NF-kappaB/NLRP3 pathway. Life Sci. 271, 119192. doi: 10.1016/j.lfs.2021.119192
Ling, H., Li, Q., Duan, Z. P., Wang, Y. J., Hu, B. Q., Dai, X. G. (2021). LncRNA GAS5 inhibits miR-579-3p to activate SIRT1/PGC-1alpha/Nrf2 signaling pathway to reduce cell pyroptosis in sepsis-associated renal injury. Am. J. Physiol. Cell Physiol. 321 (1), C117–C133. doi: 10.1152/ajpcell.00394.2020
Li, Y. F., Sheng, H. D., Qian, J., Wang, Y. (2022). The Chinese medicine babaodan suppresses LPS-induced sepsis by inhibiting NLRP3-mediated inflammasome activation. J. Ethnopharmacol. 292, 115205. doi: 10.1016/j.jep.2022.115205
Li, T., Sun, H., Li, Y., Su, L., Jiang, J., Liu, Y., et al. (2022). Downregulation of macrophage migration inhibitory factor attenuates NLRP3 inflammasome mediated pyroptosis in sepsis-induced AKI. Cell Death Discov. 8 (1), 61. doi: 10.1038/s41420-022-00859-z
Liu, C., Cai, B., Li, D., Yao, Y. (2021). Wolf-hirschhorn syndrome candidate 1 facilitates alveolar macrophage pyroptosis in sepsis-induced acute lung injury through NEK7-mediated NLRP3 inflammasome activation. Innate Immun. 27 (6), 437–447. doi: 10.1177/17534259211035426
Liu, Y., Fang, Y., Chen, X., Wang, Z., Liang, X., Zhang, T., et al. (2020). Gasdermin e-mediated target cell pyroptosis by CAR T cells triggers cytokine release syndrome. Sci. Immunol. 5 (43):eaax7969. doi: 10.1126/sciimmunol.aax7969
Liu, J. J., Li, Y., Yang, M. S., Chen, R., Cen, C. Q. (2020). SP1-induced ZFAS1 aggravates sepsis-induced cardiac dysfunction via miR-590-3p/NLRP3-mediated autophagy and pyroptosis. Arch. Biochem. Biophys. 695, 108611. doi: 10.1016/j.abb.2020.108611
Liu, Z., Tan, K., Bu, L., Bo, L., Ni, W., Fei, M., et al. (2020). Tim4 regulates NALP3 inflammasome expression and activity during monocyte/macrophage dysfunction in septic shock patients. Burns 46 (3), 652–662. doi: 10.1016/j.burns.2019.08.016
Liu, B., Wang, Z., He, R., Xiong, R., Li, G., Zhang, L., et al. (2022). Buformin alleviates sepsis-induced acute lung injury via inhibiting NLRP3-mediated pyroptosis through an AMPK-dependent pathway. Clin. Sci. (Lond) 136 (4), 273–289. doi: 10.1042/CS20211156
Liu, R., Wang, S. C., Li, M., Ma, X. H., Jia, X. N., Bu, Y., et al. (2020). An inhibitor of DRP1 (Mdivi-1) alleviates LPS-induced septic AKI by inhibiting NLRP3 inflammasome activation. BioMed. Res. Int. 2020, 2398420. doi: 10.1155/2020/2398420
Liu, Y., Zhang, Y., Feng, Q., Liu, Q., Xie, J., Li, H., et al. (2021). GPA peptide attenuates sepsis-induced acute lung injury in mice via inhibiting oxidative stress and pyroptosis of alveolar macrophage. Oxid. Med. Cell. Longev. 2021, 5589472. doi: 10.1155/2021/5589472
Liu, X., Zhang, Z., Ruan, J., Pan, Y., Magupalli, V. G., Wu, H., et al. (2016). Inflammasome-activated gasdermin d causes pyroptosis by forming membrane pores. Nature 535 (7610), 153–158. doi: 10.1038/nature18629
Liu, T., Zhou, Y. T., Wang, L. Q., Li, L. Y., Bao, Q., Tian, S., et al. (2019). NOD-like receptor family, pyrin domain containing 3 (NLRP3) contributes to inflammation, pyroptosis, and mucin production in human airway epithelium on rhinovirus infection. J. Allergy Clin. Immunol. 144 (3), 777–787. doi: 10.1016/j.jaci.2019.05.006
Li, S., Wu, H., Han, D., Ma, S., Fan, W., Wang, Y., et al. (2018). A novel mechanism of mesenchymal stromal cell-mediated protection against sepsis: Restricting inflammasome activation in macrophages by increasing mitophagy and decreasing mitochondrial ROS. Oxid. Med. Cell. Longev. 2018, 3537609. doi: 10.1155/2018/3537609
Li, N., Xiong, R., He, R., Liu, B., Wang, B., Geng, Q. (2021). Mangiferin mitigates lipopolysaccharide-induced lung injury by inhibiting NLRP3 inflammasome activation. J. Inflamm. Res. 14, 2289–2300. doi: 10.2147/JIR.S304492
Li, X., Yao, L., Zeng, X., Hu, B., Zhang, X., Wang, J., et al. (2021). MiR-30c-5p alleviated pyroptosis during sepsis-induced acute kidney injury via targeting TXNIP. Inflammation 44 (1), 217–228. doi: 10.1007/s10753-020-01323-9
Li, N., Zhou, H., Wu, H., Wu, Q., Duan, M., Deng, W., et al. (2019). STING-IRF3 contributes to lipopolysaccharide-induced cardiac dysfunction, inflammation, apoptosis and pyroptosis by activating NLRP3. Redox Biol. 24, 101215. doi: 10.1016/j.redox.2019.101215
Lu, F., Chen, H., Hong, Y., Lin, Y., Liu, L., Wei, N., et al. (2021). A gain-of-function NLRP3 3'-UTR polymorphism causes miR-146a-mediated suppression of NLRP3 expression and confers protection against sepsis progression. Sci. Rep. 11 (1), 13300. doi: 10.1038/s41598-021-92547-8
Luo, J., Wang, F., Sun, F., Yue, T., Zhou, Q., Yang, C., et al. (2021). Targeted inhibition of FTO demethylase protects mice against LPS-induced septic shock by suppressing NLRP3 inflammasome. Front. Immunol. 12. doi: 10.3389/fimmu.2021.663295
Mandal, P., Feng, Y., Lyons, J. D., Berger, S. B., Otani, S., DeLaney, A., et al. (2018). Caspase-8 collaborates with caspase-11 to drive tissue damage and execution of endotoxic shock. Immunity 49 (1), 42–55. doi: 10.1016/j.immuni.2018.06.011
Martinez-Garcia, J. J., Martinez-Banaclocha, H., Angosto-Bazarra, D., de Torre-Minguela, C., Baroja-Mazo, A., Alarcon-Vila, C., et al. (2019). P2X7 receptor induces mitochondrial failure in monocytes and compromises NLRP3 inflammasome activation during sepsis. Nat. Commun. 10 (1), 2711. doi: 10.1038/s41467-019-10626-x
Miao, P., Ruiqing, T., Yanrong, L., Zhuwen, S., Huan, Y., Qiong, W., et al. (2022). Pyroptosis: A possible link between obesity-related inflammation and inflammatory diseases. J. Cell. Physiol. 237 (2), 1245–1265. doi: 10.1002/jcp.30627
Mizushima, N., Levine, B. (2010). Autophagy in mammalian development and differentiation. Nat. Cell Biol. 12 (9), 823–830. doi: 10.1038/ncb0910-823
Mortimer, L., Moreau, F., MacDonald, J. A., Chadee, K. (2016). NLRP3 inflammasome inhibition is disrupted in a group of auto-inflammatory disease CAPS mutations. Nat. Immunol. 17 (10), 1176–1186. doi: 10.1038/ni.3538
Murphy, T. L., Tussiwand, R., Murphy, K. M. (2013). Specificity through cooperation: BATF-IRF interactions control immune-regulatory networks. Nat. Rev. Immunol. 13 (7), 499–509. doi: 10.1038/nri3470
Orning, P., Weng, D., Starheim, K., Ratner, D., Best, Z., Lee, B., et al. (2018). Pathogen blockade of TAK1 triggers caspase-8-dependent cleavage of gasdermin d and cell death. Science 362 (6418), 1064–1069. doi: 10.1126/science.aau2818
Pai, M. H., Wu, J. M., Yang, P. J., Lee, P. C., Huang, C. C., Yeh, S. L., et al. (2020). Antecedent dietary glutamine supplementation benefits modulation of liver pyroptosis in mice with polymicrobial sepsis. Nutrients 12 (4):1086. doi: 10.3390/nu12041086
Papayannopoulos, V., Metzler, K. D., Hakkim, A., Zychlinsky, A. (2010). Neutrophil elastase and myeloperoxidase regulate the formation of neutrophil extracellular traps. J. Cell Biol. 191 (3), 677–691. doi: 10.1083/jcb.201006052
Pasparakis, M., Vandenabeele, P. (2015). Necroptosis and its role in inflammation. Nature 517 (7534), 311–320. doi: 10.1038/nature14191
Pillon, N. J., Chan, K. L., Zhang, S., Mejdani, M., Jacobson, M. R., Ducos, A., et al. (2016). Saturated fatty acids activate caspase-4/5 in human monocytes, triggering IL-1beta and IL-18 release. Am. J. Physiol. Endocrinol. Metab. 311 (5), E825–E835. doi: 10.1152/ajpendo.00296.2016
Praefcke, G. (2018). Regulation of innate immune functions by guanylate-binding proteins. Int. J. Med. Microbiol. 308 (1), 237–245. doi: 10.1016/j.ijmm.2017.10.013
Pu, Q., Gan, C., Li, R., Li, Y., Tan, S., Li, X., et al. (2017). Atg7 deficiency intensifies inflammasome activation and pyroptosis in pseudomonas sepsis. J. Immunol. 198 (8), 3205–3213. doi: 10.4049/jimmunol.1601196
Rahim, I., Sayed, R. K., Fernandez-Ortiz, M., Aranda-Martinez, P., Guerra-Librero, A., Fernandez-Martinez, J., et al. (2021). Melatonin alleviates sepsis-induced heart injury through activating the Nrf2 pathway and inhibiting the NLRP3 inflammasome. Naunyn Schmiedebergs Arch. Pharmacol. 394 (2), 261–277. doi: 10.1007/s00210-020-01972-5
Rathkey, J. K., Zhao, J., Liu, Z., Chen, Y., Yang, J., Kondolf, H. C., et al. (2018). Chemical disruption of the pyroptotic pore-forming protein gasdermin d inhibits inflammatory cell death and sepsis. Sci. Immunol. 3 (26):eaat2738. doi: 10.1126/sciimmunol.aat2738
Ren, C., Zhang, H., Wu, T. T., Yao, Y. M. (2017). Autophagy: A potential therapeutic target for reversing sepsis-induced immunosuppression. Front. Immunol. 8. doi: 10.3389/fimmu.2017.01832
Rhee, C., Dantes, R., Epstein, L., Murphy, D. J., Seymour, C. W., Iwashyna, T. J., et al. (2017). Incidence and trends of sepsis in US hospitals using clinical vs claims data 2009-2014. JAMA 318 (13), 1241–1249. doi: 10.1001/jama.2017.13836
Ruan, J., Xia, S., Liu, X., Lieberman, J., Wu, H. (2018). Cryo-EM structure of the gasdermin A3 membrane pore. Nature 557 (7703), 62–67. doi: 10.1038/s41586-018-0058-6
Ruhl, S., Broz, P. (2015). Caspase-11 activates a canonical NLRP3 inflammasome by promoting k(+) efflux. Eur. J. Immunol. 45 (10), 2927–2936. doi: 10.1002/eji.201545772
Ruhl, S., Shkarina, K., Demarco, B., Heilig, R., Santos, J. C., Broz, P. (2018). ESCRT-dependent membrane repair negatively regulates pyroptosis downstream of GSDMD activation. Science 362 (6417), 956–960. doi: 10.1126/science.aar7607
Saloura, V., Cho, H. S., Kiyotani, K., Alachkar, H., Zuo, Z., Nakakido, M., et al. (2015). WHSC1 promotes oncogenesis through regulation of NIMA-related kinase-7 in squamous cell carcinoma of the head and neck. Mol. Cancer Res. 13 (2), 293–304. doi: 10.1158/1541-7786.MCR-14-0292-T
Santos, J. C., Dick, M. S., Lagrange, B., Degrandi, D., Pfeffer, K., Yamamoto, M., et al. (2018). LPS targets host guanylate-binding proteins to the bacterial outer membrane for non-canonical inflammasome activation. EMBO J. 37 (6):e98089. doi: 10.15252/embj.201798089
Sarhan, J., Liu, B. C., Muendlein, H. I., Li, P., Nilson, R., Tang, A. Y., et al. (2018). Caspase-8 induces cleavage of gasdermin d to elicit pyroptosis during yersinia infection. Proc. Natl. Acad. Sci. U. S. A. 115 (46), E10888–E10897. doi: 10.1073/pnas.1809548115
Sarkar, A., Hall, M. W., Exline, M., Hart, J., Knatz, N., Gatson, N. T., et al. (2006). Caspase-1 regulates escherichia coli sepsis and splenic b cell apoptosis independently of interleukin-1beta and interleukin-18. Am. J. Respir. Crit. Care Med. 174 (9), 1003–1010. doi: 10.1164/rccm.200604-546OC
Semino, C., Carta, S., Gattorno, M., Sitia, R., Rubartelli, A. (2018). Progressive waves of IL-1beta release by primary human monocytes via sequential activation of vesicular and gasdermin d-mediated secretory pathways. Cell Death Dis. 9 (11), 1088. doi: 10.1038/s41419-018-1121-9
Sharif, H., Wang, L., Wang, W. L., Magupalli, V. G., Andreeva, L., Qiao, Q., et al. (2019). Structural mechanism for NEK7-licensed activation of NLRP3 inflammasome. Nature 570 (7761), 338–343. doi: 10.1038/s41586-019-1295-z
Sharma, D., Kanneganti, T. D. (2016). The cell biology of inflammasomes: Mechanisms of inflammasome activation and regulation. J. Cell Biol. 213 (6), 617–629. doi: 10.1083/jcb.201602089
Shenoy, A. R., Wellington, D. A., Kumar, P., Kassa, H., Booth, C. J., Cresswell, P., et al. (2012). GBP5 promotes NLRP3 inflammasome assembly and immunity in mammals. Science 336 (6080), 481–485. doi: 10.1126/science.1217141
Shi, C. S., Kehrl, J. H. (2016). Cytochrome c negatively regulates NLRP3 inflammasomes. PloS One 11 (12), e167636. doi: 10.1371/journal.pone.0167636
Shi, C. X., Wang, Y., Chen, Q., Jiao, F. Z., Pei, M. H., Gong, Z. J. (2020). Extracellular histone h3 induces pyroptosis during sepsis and may act through NOD2 and VSIG4/NLRP3 pathways. Front. Cell Infect. Microbiol. 10. doi: 10.3389/fcimb.2020.00196
Shi, J., Zhao, Y., Wang, Y., Gao, W., Ding, J., Li, P., et al. (2014). Inflammatory caspases are innate immune receptors for intracellular LPS. Nature 514 (7521), 187–192. doi: 10.1038/nature13683
Shi, J., Zhao, Y., Wang, K., Shi, X., Wang, Y., Huang, H., et al. (2015). Cleavage of GSDMD by inflammatory caspases determines pyroptotic cell death. Nature 526 (7575), 660–665. doi: 10.1038/nature15514
Silva, C., Wanderley, C., Veras, F. P., Sonego, F., Nascimento, D. C., Goncalves, A. V., et al. (2021). Gasdermin d inhibition prevents multiple organ dysfunction during sepsis by blocking NET formation. Blood 138 (25), 2702–2713. doi: 10.1182/blood.2021011525
Singer, M., Deutschman, C. S., Seymour, C. W., Shankar-Hari, M., Annane, D., Bauer, M., et al. (2016). The third international consensus definitions for sepsis and septic shock (Sepsis-3). JAMA 315 (8), 801–810. doi: 10.1001/jama.2016.0287
Sirito, M., Walker, S., Lin, Q., Kozlowski, M. T., Klein, W. H., Sawadogo, M. (1992). Members of the USF family of helix-loop-helix proteins bind DNA as homo- as well as heterodimers. Gene Expr. 2 (3), 231–240.
Sollberger, G., Choidas, A., Burn, G. L., Habenberger, P., Di Lucrezia, R., Kordes, S., et al. (2018). Gasdermin d plays a vital role in the generation of neutrophil extracellular traps. Sci. Immunol. 3 (26):eaar6689. doi: 10.1126/sciimmunol.aar6689
Song, N., Li, T. (2018). Regulation of NLRP3 inflammasome by phosphorylation. Front. Immunol. 9. doi: 10.3389/fimmu.2018.02305
Strasser, A., O'Connor, L., Dixit, V. M. (2000). Apoptosis signaling. Annu. Rev. Biochem. 69, 217–245. doi: 10.1146/annurev.biochem.69.1.217
Subramanian, S., Steer, C. J. (2019). Special issue: MicroRNA regulation in health and disease. Genes (Basel) 10 (6):457. doi: 10.3390/genes10060457
Sun, J., Ge, X., Wang, Y., Niu, L., Tang, L., Pan, S. (2022). USF2 knockdown downregulates THBS1 to inhibit the TGF-beta signaling pathway and reduce pyroptosis in sepsis-induced acute kidney injury. Pharmacol. Res. 176, 105962. doi: 10.1016/j.phrs.2021.105962
Sun, J., Sun, X., Chen, J., Liao, X., He, Y., Wang, J., et al. (2021). MicroRNA-27b shuttled by mesenchymal stem cell-derived exosomes prevents sepsis by targeting JMJD3 and downregulating NF-kappaB signaling pathway. Stem Cell Res. Ther. 12 (1), 14. doi: 10.1186/s13287-020-02068-w
Sun, Y. B., Zhao, H., Mu, D. L., Zhang, W., Cui, J., Wu, L., et al. (2019). Dexmedetomidine inhibits astrocyte pyroptosis and subsequently protects the brain in in vitro and in vivo models of sepsis. Cell Death Dis. 10 (3), 167. doi: 10.1038/s41419-019-1416-5
Swanson, K. V., Deng, M., Ting, J. P. (2019). The NLRP3 inflammasome: Molecular activation and regulation to therapeutics. Nat. Rev. Immunol. 19 (8), 477–489. doi: 10.1038/s41577-019-0165-0
Tan, J., Fan, J., He, J., Zhao, L., Tang, H. (2020). Knockdown of LncRNA DLX6-AS1 inhibits HK-2 cell pyroptosis via regulating miR-223-3p/NLRP3 pathway in lipopolysaccharide-induced acute kidney injury. J. Bioenerg. Biomembr. 52 (5), 367–376. doi: 10.1007/s10863-020-09845-5
Tang, J., Tu, S., Lin, G., Guo, H., Yan, C., Liu, Q., et al. (2020). Sequential ubiquitination of NLRP3 by RNF125 and cbl-b limits inflammasome activation and endotoxemia. J. Exp. Med. 217 (4):e20182091. doi: 10.1084/jem.20182091
Teng, Y., Li, N., Wang, Y., Sun, S., Hou, J., Chen, Y., et al. (2022). NRF2 inhibits cardiomyocyte pyroptosis via regulating CTRP1 in sepsis-induced myocardial injury. Shock 57 (4), 590–599. doi: 10.1097/SHK.0000000000001901
Thiam, H. R., Wong, S. L., Wagner, D. D., Waterman, C. M. (2020). Cellular mechanisms of NETosis. Annu. Rev. Cell Dev. Biol. 36, 191–218. doi: 10.1146/annurev-cellbio-020520-111016
Tian, Y., Li, P., Wu, Z., Deng, Q., Pan, B., Stringer, K. A., et al. (2021). Citrullinated histone h3 mediates sepsis-induced lung injury through activating caspase-1 dependent inflammasome pathway. Front. Immunol. 12. doi: 10.3389/fimmu.2021.761345
Tsuchiya, K., Nakajima, S., Hosojima, S., Thi, N. D., Hattori, T., Manh, L. T., et al. (2019). Caspase-1 initiates apoptosis in the absence of gasdermin d. Nat. Commun. 10 (1), 2091. doi: 10.1038/s41467-019-09753-2
Vanaja, S. K., Rathinam, V. A., Fitzgerald, K. A. (2015). Mechanisms of inflammasome activation: Recent advances and novel insights. Trends Cell Biol. 25 (5), 308–315. doi: 10.1016/j.tcb.2014.12.009
Vanaja, S. K., Russo, A. J., Behl, B., Banerjee, I., Yankova, M., Deshmukh, S. D., et al. (2016). Bacterial outer membrane vesicles mediate cytosolic localization of LPS and caspase-11 activation. Cell 165 (5), 1106–1119. doi: 10.1016/j.cell.2016.04.015
van der Poll, T., Lowry, S. F. (1995). Tumor necrosis factor in sepsis: Mediator of multiple organ failure or essential part of host defense? Shock 3 (1), 1–12. doi: 10.1097/00024382-199501000-00001
van der Poll, T., Shankar-Hari, M., Wiersinga, W. J. (2021). The immunology of sepsis. Immunity 54 (11), 2450–2464. doi: 10.1016/j.immuni.2021.10.012
Venet, F., Monneret, G. (2018). Advances in the understanding and treatment of sepsis-induced immunosuppression. Nat. Rev. Nephrol. 14 (2), 121–137. doi: 10.1038/nrneph.2017.165
Walkey, A. J., Lagu, T., Lindenauer, P. K. (2015). Trends in sepsis and infection sources in the united states. a population-based study. Ann. Am. Thorac. Soc 12 (2), 216–220. doi: 10.1513/AnnalsATS.201411-498BC
Wang, Y., Gao, W., Shi, X., Ding, J., Liu, W., He, H., et al. (2017). Chemotherapy drugs induce pyroptosis through caspase-3 cleavage of a gasdermin. Nature 547 (7661), 99–103. doi: 10.1038/nature22393
Wang, X., Li, X. L., Qin, L. J. (2021a). The lncRNA XIST/miR-150-5p/c-Fos axis regulates sepsis-induced myocardial injury via TXNIP-modulated pyroptosis. Lab. Invest. 101 (9), 1118–1129. doi: 10.1038/s41374-021-00607-4
Wang, B., Liu, Y., Jiang, R., Liu, Z., Gao, H., Chen, F., et al. (2022). Emodin relieves the inflammation and pyroptosis of lipopolysaccharide-treated 1321N1 cells by regulating methyltransferase-like 3 -mediated NLR family pyrin domain containing 3 expression. Bioengineered 13 (3), 6740–6749. doi: 10.1080/21655979.2022.2045836
Wang, Y. C., Liu, Q. X., Liu, T., Xu, X. E., Gao, W., Bai, X. J., et al. (2018). Caspase-1-dependent pyroptosis of peripheral blood mononuclear cells predicts the development of sepsis in severe trauma patients: A prospective observational study. Medicine (Baltimore) 97 (8), e9859. doi: 10.1097/MD.0000000000009859
Wang, X., Shi, J., Li, Z., Li, L., Zhang, R., Bai, Y., et al. (2021b). An 8-Hydroxy-Quinoline derivative protects against lipopolysaccharide-induced lethality in endotoxemia by inhibiting HMGB1-mediated caspase-11 signaling. Front. Pharmacol. 12. doi: 10.3389/fphar.2021.673818
Wang, Q., Wang, Y., Ding, J., Wang, C., Zhou, X., Gao, W., et al. (2020). A bioorthogonal system reveals antitumour immune function of pyroptosis. Nature 579 (7799), 421–426. doi: 10.1038/s41586-020-2079-1
Wang, Y., Yin, B., Li, D., Wang, G., Han, X., Sun, X. (2018). GSDME mediates caspase-3-dependent pyroptosis in gastric cancer. Biochem. Biophys. Res. Commun. 495 (1), 1418–1425. doi: 10.1016/j.bbrc.2017.11.156
Wang, L., Zhao, H., Xu, H., Liu, X., Chen, X., Peng, Q., et al. (2021). Targeting the TXNIP-NLRP3 interaction with PSSM1443 to suppress inflammation in sepsis-induced myocardial dysfunction. J. Cell. Physiol. 236 (6), 4625–4639. doi: 10.1002/jcp.30186
Wang, H., Zheng, Y., Huang, J., Li, J. (2021b). Mitophagy in antiviral immunity. Front. Cell Dev. Biol. 9. doi: 10.3389/fcell.2021.723108
Watany, M. M., Elmazny, M. I., Nasif, E. M. (2020). Interleukin-31 interaction with inflammasome: A promising diagnostic and prognostic panel for early sepsis identification in critically ill patients. Cytokine 131, 155102. doi: 10.1016/j.cyto.2020.155102
Xia, X., Wang, X., Zheng, Y., Jiang, J., Hu, J. (2019). What role does pyroptosis play in microbial infection? J. Cell. Physiol. 234 (6), 7885–7892. doi: 10.1002/jcp.27909
Xia, S., Zhang, Z., Magupalli, V. G., Pablo, J. L., Dong, Y., Vora, S. M., et al. (2021). Gasdermin d pore structure reveals preferential release of mature interleukin-1. Nature 593 (7860), 607–611. doi: 10.1038/s41586-021-03478-3
Xie, Y., Hou, W., Song, X., Yu, Y., Huang, J., Sun, X., et al. (2016). Ferroptosis: Process and function. Cell Death Differ. 23 (3), 369–379. doi: 10.1038/cdd.2015.158
Xue, Y., Enosi, T. D., Tan, W. H., Kay, C., Man, S. M. (2019). Emerging activators and regulators of inflammasomes and pyroptosis. Trends Immunol. 40 (11), 1035–1052. doi: 10.1016/j.it.2019.09.005
Yang, T., Feng, X., Zhao, Y., Zhang, H., Cui, H., Wei, M., et al. (2020). Dexmedetomidine enhances autophagy via alpha2-AR/AMPK/mTOR pathway to inhibit the activation of NLRP3 inflammasome and subsequently alleviates lipopolysaccharide-induced acute kidney injury. Front. Pharmacol. 11. doi: 10.3389/fphar.2020.00790
Yang, D., He, Y., Munoz-Planillo, R., Liu, Q., Nunez, G. (2015). Caspase-11 requires the pannexin-1 channel and the purinergic P2X7 pore to mediate pyroptosis and endotoxic shock. Immunity 43 (5), 923–932. doi: 10.1016/j.immuni.2015.10.009
Yang, W. S., Stockwell, B. R. (2016). Ferroptosis: Death by lipid peroxidation. Trends Cell Biol. 26 (3), 165–176. doi: 10.1016/j.tcb.2015.10.014
Yao, Y., Hu, X., Feng, X., Zhao, Y., Song, M., Wang, C., et al. (2019). Dexmedetomidine alleviates lipopolysaccharide-induced acute kidney injury by inhibiting the NLRP3 inflammasome activation via regulating the TLR4/NOX4/NF-kappaB pathway. J. Cell. Biochem. 120 (10), 18509–18523. doi: 10.1002/jcb.29173
Yi, Y. S. (2017). Caspase-11 non-canonical inflammasome: A critical sensor of intracellular lipopolysaccharide in macrophage-mediated inflammatory responses. Immunology 152 (2), 207–217. doi: 10.1111/imm.12787
Yuan, C., Wu, M., Xiao, Q., Zhao, W., Li, H., Zhong, Y., et al. (2021). Blocking Msr1 by berberine alkaloids inhibits caspase-11-dependent coagulation in bacterial sepsis. Signal Transduct. Target. Ther. 6 (1), 92. doi: 10.1038/s41392-021-00483-w
Zhang, K., Fan, C., Cai, D., Zhang, Y., Zuo, R., Zhu, L., et al. (2020). Contribution of TGF-Beta-Mediated NLRP3-HMGB1 activation to tubulointerstitial fibrosis in rat with angiotensin II-induced chronic kidney disease. Front. Cell Dev. Biol. 8. doi: 10.3389/fcell.2020.00001
Zhang, W., Tao, A., Lan, T., Cepinskas, G., Kao, R., Martin, C. M., et al. (2017). Carbon monoxide releasing molecule-3 improves myocardial function in mice with sepsis by inhibiting NLRP3 inflammasome activation in cardiac fibroblasts. Basic Res. Cardiol. 112 (2), 16. doi: 10.1007/s00395-017-0603-8
Zhang, Z., Zhang, Y., Xia, S., Kong, Q., Li, S., Liu, X., et al. (2020b). Gasdermin e suppresses tumour growth by activating anti-tumour immunity. Nature 579 (7799), 415–420. doi: 10.1038/s41586-020-2071-9
Zhao, Z., Wang, Y., Zhou, R., Li, Y., Gao, Y., Tu, D., et al. (2020). A novel role of NLRP3-generated IL-1beta in the acute-chronic transition of peripheral lipopolysaccharide-elicited neuroinflammation: Implications for sepsis-associated neurodegeneration. J. Neuroinflammation 17 (1), 64. doi: 10.1186/s12974-020-1728-5
Zhao, C., Zhao, W. (2020). NLRP3 inflammasome-a key player in antiviral responses. Front. Immunol. 11. doi: 10.3389/fimmu.2020.00211
Zhou, C. B., Fang, J. Y. (2019). The role of pyroptosis in gastrointestinal cancer and immune responses to intestinal microbial infection. Biochim. Biophys. Acta Rev. Cancer 1872 (1), 1–10. doi: 10.1016/j.bbcan.2019.05.001
Zhou, Z., He, H., Wang, K., Shi, X., Wang, Y., Su, Y., et al. (2020). Granzyme a from cytotoxic lymphocytes cleaves GSDMB to trigger pyroptosis in target cells. Science 368 (6494):eaaz7548. doi: 10.1126/science.aaz7548
Zhou, R., Tardivel, A., Thorens, B., Choi, I., Tschopp, J. (2010). Thioredoxin-interacting protein links oxidative stress to inflammasome activation. Nat. Immunol. 11 (2), 136–140. doi: 10.1038/ni.1831
Zhou, R., Yang, X., Li, X., Qu, Y., Huang, Q., Sun, X., et al. (2019). Recombinant CC16 inhibits NLRP3/caspase-1-induced pyroptosis through p38 MAPK and ERK signaling pathways in the brain of a neonatal rat model with sepsis. J. Neuroinflammation 16 (1), 239. doi: 10.1186/s12974-019-1651-9
Keywords: sepsis, pyroptosis, inflammation, cell death, sepsis-associated organ dysfunction
Citation: Wen R, Liu Y-P, Tong X-X, Zhang T-N and Yang N (2022) Molecular mechanisms and functions of pyroptosis in sepsis and sepsis-associated organ dysfunction. Front. Cell. Infect. Microbiol. 12:962139. doi: 10.3389/fcimb.2022.962139
Received: 06 June 2022; Accepted: 06 July 2022;
Published: 29 July 2022.
Edited by:
Alan G. Goodman, Washington State University, United StatesReviewed by:
Alan Y. Hsu, Boston Children’s Hospital and Harvard Medical School, United StatesShiyu Xia, Harvard University, United States
Copyright © 2022 Wen, Liu, Tong, Zhang and Yang. This is an open-access article distributed under the terms of the Creative Commons Attribution License (CC BY). The use, distribution or reproduction in other forums is permitted, provided the original author(s) and the copyright owner(s) are credited and that the original publication in this journal is cited, in accordance with accepted academic practice. No use, distribution or reproduction is permitted which does not comply with these terms.
*Correspondence: Tie-Ning Zhang, cmuztn@vip.qq.com; Ni Yang, yangni616@hotmail.com