- 1Department of Microbiology, Immunology and Cell Biology, West Virginia University, Morgantown, WV, United States
- 2Health Effects Laboratory Division, National Institute for Occupational Safety and Health, Centers for Disease Control and Prevention, Morgantown, WV, United States
- 3Department of Civil, Environmental & Geodetic Engineering, College of Engineering, Ohio State University, Columbus, OH, United States
- 4Division of Environmental Health Sciences, College of Public Health, Ohio State University, Columbus, OH, United States
Background: Allergic airway disease (AAD) is a growing concern in industrialized nations and can be influenced by fungal exposures. Basidiomycota yeast species such as Cryptococcus neoformans are known to exacerbate allergic airway disease; however, recent indoor assessments have identified other Basidiomycota yeasts, including Vishniacozyma victoriae (syn. Cryptococcus victoriae), to be prevalent and potentially associated with asthma. Until now, the murine pulmonary immune response to repeated V. victoriae exposure was previously unexplored.
Objective: This study aimed to compare the immunological impact of repeated pulmonary exposure to Cryptococcus yeasts.
Methods: Mice were repeatedly exposed to an immunogenic dose of C. neoformans or V. victoriae via oropharyngeal aspiration. Bronchoalveolar lavage fluid (BALF) and lungs were collected to examine airway remodeling, inflammation, mucous production, cellular influx, and cytokine responses at 1 day and 21 days post final exposure. The responses to C. neoformans and V. victoriae were analyzed and compared.
Results: Following repeated exposure, both C. neoformans and V. victoriae cells were still detectable in the lungs 21 days post final exposure. Repeated C. neoformans exposure initiated myeloid and lymphoid cellular infiltration into the lung that worsened over time, as well as an IL-4 and IL-5 response compared to PBS-exposed controls. In contrast, repeated V. victoriae exposure induced a strong CD4+ T cell-driven lymphoid response that started to resolve by 21 days post final exposure.
Discussion: C. neoformans remained in the lungs and exacerbated the pulmonary immune responses as expected following repeated exposure. The persistence of V. victoriae in the lung and strong lymphoid response following repeated exposure were unexpected given its lack of reported involvement in AAD. Given the abundance in indoor environments and industrial utilization of V. victoriae, these results highlight the importance to investigate the impact of frequently detected fungal organisms on the pulmonary response following inhalational exposure. Moreover, it is important to continue to address the knowledge gap involving Basidiomycota yeasts and their impact on AAD.
1 Introduction
Allergic airway diseases including asthma, rhinitis, and similar respiratory morbidities have increased in incidence over time, specifically in industrialized nations such as the United States (Barnes, 2004; Bateman et al., 2008; Environment and Health Data Portal, 2020). These are complex inflammatory processes involving numerous cells and mediators that act directly or indirectly on the airway (Anderson, 2008). As a result, symptoms such as cough, chest tightness, shortness of breath, and wheezing may arise, which can impact quality of life and increase susceptibility to secondary pulmonary infections (Papi et al., 2018). This is particularly concerning during the COVID-19 pandemic, and it has been observed that individuals with severe asthma may be at increased risk for morbidity and mortality from COVID-19 infection (Howell et al., 2021).
Allergic asthma is the most diagnosed phenotype of asthma and is defined by sensitization to allergens, atopy, and is often triggered by environmental allergens (Eder et al., 2006). Individuals with allergic asthma are usually diagnosed early in life following symptom presentation and have increased total IgE and Th2 cytokine levels compared to non-allergic asthmatic counterparts (Schatz and Rosenwasser, 2014). Environmental allergens that are derived from microbial species present in indoor environments are often observed to influence allergic airway responses and lead to respiratory morbidities. Although pulmonary microbial exposures are well studied, most of the research has focused on the contribution of viruses and bacteria, and little is known about fungal exposures in general. For example, respiratory viruses such as rhinovirus frequently initiate asthma and are the most common trigger of asthma exacerbations (Jartti et al., 2020). Bacterial infections, such as Mycoplasma and Streptococcus pneumoniae, can impair mucous clearance, initiate goblet cell hyperplasia, and trigger IgE-mediated responses (Kraft, 2000) which exacerbate allergic airway diseases. However, it is becoming more evident that fungal microorganisms also contribute to allergic airway disease (Denning et al., 2014). A major caveat and reason the role of fungal species has not been recognized is the lack of standardized detection methods (Levetin et al., 2016; Green, 2018).
As Basidiomycota fungal species are not easily identifiable through traditional methods of fungal detection, these species have been largely overlooked, and as a result, vastly understudied. High throughput sequencing has changed the paradigm and consequently the identification of fungi in indoor environments contributing to human exposure (Alanio and Bretagne, 2014). Environmental assessments have emphasized the presence of Basidiomycota yeast species, specifically Cryptococcus (Adams et al., 2013; Dannemiller et al., 2014; Adams et al., 2015; Dannemiller et al., 2016). Cryptococcus yeasts include both pathogenic and nonpathogenic species. Cryptococcus neoformans is found in environments around the world, and is highly detected in pigeon droppings, and therefore, is commonly detected in urban areas (Levitz, 1991). C. neoformans is capable of growth at 37°C and has a rigid polysaccharide capsule that allows for the evasion of the immune system leading to cryptococcal infections primarily in immunocompromised individuals (Levitz, 1991; Idnurm and Lin, 2015; Campuzano and Wormley, 2018). Cryptococcus victoriae (since renamed Vishniacozyma victoriae) is highly prevalent in indoor environments (Pitkaranta et al., 2008; Dannemiller et al., 2016; Lutz et al., 2020; Rush et al., 2021). Vishniacozyma victoriae was isolated in the Antarctic (Montes et al., 1999) and has since been detected in indoor and outdoor environments worldwide (de Menezes et al., 2019). V. victoriae is also utilized for the control of post-harvest diseases of fruits in some semi-commercial settings in Argentina (Lutz et al., 2020). Unlike C. neoformans, V. victoriae grows optimally at lower temperatures (15°) but is known to tolerate a variety of environmental conditions. Additionally, V. victoriae does not have a polysaccharide capsule, which may contribute to its lack of pathogenicity. Studies have recently shown that V. victoriae produces a variety of compounds such as lipase, ergosterol, and linoleic acid (Rusinova-Videva et al., 2022). To date, V. victoriae research has not focused on the pulmonary immune response following exposure. Given the frequent and abundant detection in indoor environments, as well as its potential use in commercial settings, there is a crucial need to further examine the impact of exposure to V. victoriae.
Preliminary epidemiological-based studies suggest that Cryptococcus yeasts influence allergic airway diseases. Inhalation is the primary route of infection for C. neoformans (Campuzano and Wormley, 2018); however, pulmonary exposure to this yeast has also been shown to elicit allergic inflammation (Goldman and Huffnagle, 2009). One study using an ovalbumin model of allergic airway disease found that C. neoformans exposure exacerbated IgE responses and initiated eosinophil infiltration, goblet cell hyperplasia, and airway hyperreactivity (Goldman et al., 2006). Given the prevalence of C. neoformans in urban environments, it is important to note that individuals from these areas are also at a higher risk for asthma (Lin et al., 1999). Studies examining fungal exposure in health-based cohorts have indicated that low Cryptococcus diversity was highly associated with asthma development. Furthermore, previous studies found that V. victoriae concentrations in homes were inversely associated with asthma (Rush et al., 2021). Yet, a knowledge gap remains regarding the in vivo response to this frequently detected yeast species.
Given the inverse association of V. victoriae with asthma, as well as the differences between this species compared to C. neoformans, it is possible that exposure to V. victoriae influences pulmonary immune responses differently than C. neoformans. To better understand the impact exposure to V. victoriae may have on allergic airway disease, the pulmonary immune response to repeated exposure to each of these species was examined in parallel with C. neoformans. This was accomplished by repeatedly exposing mice to immunogenic doses of V. victoriae or C. neoformans via oropharyngeal aspiration, and then examining the physical changes, inflammation, mucous production, cellular influx, and cytokine response in the lungs of exposed mice at two different time points post-exposure. The immune responses following exposure to each yeast were characterized and compared to highlight the unique impacts of each exposure.
2 Materials and methods
2.1 Fungal cultivation and sample preparation
Vishniacozyma victoriae (ATCC MYA-305) was cultivated for exposures as previously described (Rush et al., 2021). Briefly, liquid cultures were grown in Potato Dextrose Broth (BD Biosciences, San Jose, CA) at 15°C. C. neoformans (ATCC 32045) was grown in liquid cultures in Yeast Mold Broth (BD Biosciences, San Jose, CA) at 25°C. Cells were collected during the logarithmic growth phase (approximately 48 hours post-inoculation for V. victoriae and 24 hours post-inoculation for C. neoformans), washed and diluted in phosphate buffered saline (PBS) at concentrations of 2x105 cells/mL and 2x107 cells/mL corresponding to 104 and 106 cells per 50 µL aspiration, respectively. Cells were diluted from fresh cultures each exposure day immediately prior to administration. Analysis of yeast growth kinetics at body temperature (37°C) compared to the optimal growth temperature was completed first by culturing V. victoriae from glycerol stock in 5 mL of PD broth at 15°C for 72 hours at 200 rpm. Flasks containing 100 mL of PD broth were inoculated with 100 µL of the liquid culture and incubated in a shaker incubator at either 15°C or 37°C at 150 rpm (n=2 per temperature). Optical density readings were obtained at various timepoints from 0 to 128 hours following inoculation using a SpectraMax M4 spectrophotometer (Molecular Devices, San Jose, CA). C. neoformans was cultured from glycerol stock in 5 mL of YM broth at 25°C for 72 hours at 200 rpm. Flasks containing 100 mL of YM broth were inoculated with 100 µL of the liquid culture and incubated in a shaker incubator at either 25°C or 37°C at 150 rpm (n=2 per temperature). Optical density readings at 600 nm were obtained at timepoints from 0 to 80 hours following inoculation.
2.2 Animal exposures
Randomly grouped 6- to 8-week-old female BALB/cJ mice (The Jackson Laboratory, Bar Harbor, ME) were used for all experiments. Preliminary studies demonstrated that female mice, in comparison to male mice, had a more severe allergic airway response to exposure, consistent with findings reported by Melgert et al. (2005). Mice included in the deposition study (n = 5 per group) were housed in the AAALAC International-accredited vivarium at the West Virginia University Health Science Center. Mice used in the repeated exposure and recovery study (n = 8-10 per group) were housed in the AAALAC International-accredited animal facility at the National Institute for Occupational Safety and Health (NIOSH). Mice received high-efficiency particulate absorbing (HEPA)-filtered air and were housed with 12-hour light/dark cycles and provided food and water ad libitum. All animal procedures were approved by the West Virginia University or CDC-Morgantown Animal Care and Use Committee.
Mice were exposed to yeast via oropharyngeal aspiration of a 50 µL yeast suspension prepared in PBS. For the deposition study, mice were anesthetized with isoflurane, exposed to one dose of yeast via oropharyngeal aspiration, and humanely euthanized approximately one hour later. Mice in the repeated exposure recovery study were anesthetized, exposed via oropharyngeal aspiration every other day for a total of six exposures, and then humanely euthanized 1 day or 21 days after the final exposure (Figure 1). All mice were humanely euthanized with an intraperitoneal injection of pentobarbital at 100-300 mg/kg body weight (Fatal Plus, Vortech Pharmaceuticals, Dearborn, MI). Serum, lung tissue, and bronchoalveolar lavage fluid (BALF) were collected following euthanasia for subsequent analyses.
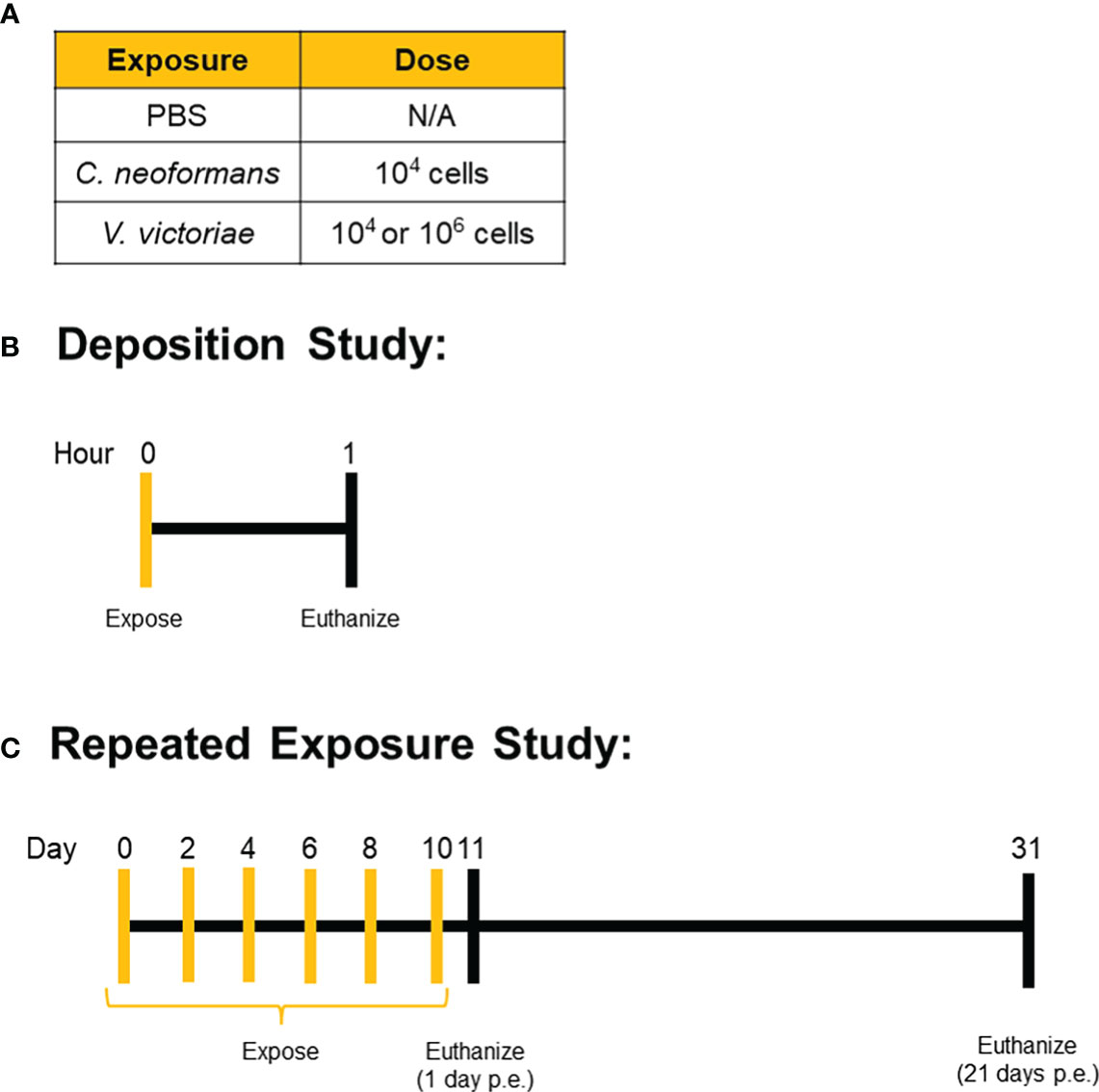
Figure 1 Exposure Paradigms. Mice were exposed to (A) set doses of either C. neoformans, V. victoriae, or PBS control via oropharyngeal aspiration. (B) Deposition was confirmed by exposing mice one time and then collecting tissue for analysis approximately 1 hour after the exposure. (C) For the repeated exposure study, mice were repeatedly exposed to the same dose of yeast or PBS control every other day for a total of six exposures, with tissue collection occurring either 1 day or 21 days after the final exposure.
2.3 Histopathological analysis
Capsule presence was examined by overloading murine lungs with approximately 108 C. neoformans or V. victoriae cells, grown at 25°C and 15°C, respectively, which were then fixed with 10% neutral buffered formalin, paraffin embedded, sectioned and then stained with mucicarmine stain. Lung sections were visualized and images were captured at 100X magnification under oil immersion using an Echo Rebel microscope (Echo, San Diego, CA). Right lung lobes (n= 3/group) from repeatedly exposed mice were inflated with 10% paraformaldehyde fixative, tied off, and collected. Lung tissue was then embedded in paraffin, sectioned, and stained with hematoxylin and eosin (H&E), Periodic-acid Schiff (PAS), Grocott’s methenamine silver (GMS) stain for histopathological evaluation by iHisto services (Salem, MA). H&E-stained sections were examined to evaluate lung architecture, inflammation severity and location, and inflammatory cell types. PAS-stained sections were analyzed to identify changes in airway epithelium, specifically the proliferation of goblet cells and mucous production. GMS-stained sections were examined for yeast organisms and GMS+ cytoplasmic granules. Scores were determined for various parameters based on a standard qualitative toxicologic scoring scale (0 – none, 1 – minimal, 2 – mild, 3 – moderate, 4 – marked, and 5 – severe). Slides were imaged and analyzed by a blinded histopathologist, and scores and findings were reported (Supplemental Table 1). Lastly, embedded lung tissues were also stained with mucicarmine to identify the presence or absence of a capsule surrounding the yeast cells.
2.4 Flow cytometric analysis of lung tissue and bronchoalveolar lavage fluid
Bronchoalveolar lavage fluid (BALF) was collected from mice as previously described (Croston et al., 2020). Briefly, BALF cells were collected in 2 mL of PBS, washed, and immediately underwent flow cytometric staining. To analyze the remaining cells in the lung tissue, BALF-depleted lungs were then processed for flow cytometry. Following BALF collection, the lungs were washed with PBS, minced, and incubated in digestion buffer (5% fetal bovine serum, 1 mg/mL collagenase, 30 µg/mL DNAse Type IV in Dulbecco’s Modified Eagle Medium) for 30 minutes at 37°C on a shaker. Digested lung tissue was filtered (0.2 uM), washed, filtered, and centrifuged at 400 x g to isolate lung cells. Lung cells then underwent flow cytometric staining. Reagent information can be found in Supplemental Table 2.
Cell pellets from lung tissue were resuspended in 1 mL red blood cell lysis buffer for 5 min at room temperature, then washed in FACS buffer (4% fetal bovine serum + 2mM ethylenediaminetetraacetic acid in PBS) and centrifuged at 400 x g for 5 min. Next, lung and BALF cells were blocked in Fc Block (10% Rat Serum, 5% anti-mouse CD16/CD32 clone 2.4G2 antibody in PBS) for 5 min at room temperature. Cells were then incubated with the surface stain cocktail (Supplemental Table 2) for 25 min in the dark at 4°C. Following a wash, the cells were then fixed with Cytofix for 10 min at room temperature, washed, and resuspended in 250 ul of FACS buffer. Data were acquired using an LSR II flow cytometer (BD Biosciences). Cell counts were calculated utilizing Spherotech AccuCount Beads per the manufacturer’s instructions. Flow cytometric analysis was performed utilizing FlowJo version 10.6 (FlowJo, Becton, Dickinson and Company, NJ). Reagent information can be found in Supplemental Table 1.
2.5 Cytokine multiplex
Frozen lung tissue was homogenized utilizing the TissueLyser II system (Qiagen, Hilden, Germany). Briefly, lung tissues were placed in 2 mL Eppendorf tubes containing Tissue Protein Extraction Reagent (T-PER, Thermo Fisher Scientific, Waltham, MA) with a 5 mm metal bead (OPS Diagnostics, Lebanon, NJ) and lysed for three 30 second cycles at 4.5 Hz. Homogenized samples were centrifuged at 1,400 rpm for 10 min and the supernatant was collected and stored at -80°C for later use. Cytokine levels of murine IL-4, IL-5, IL-13, IL-33, and Eotaxin were assessed using a custom ProcartaPlex Multiplex Immunoassay (Invitrogen, Waltham, MA). The plate was prepared per the manufacturer’s instructions and run on a Luminex 200 system (Luminex, Austin, TX). In-software analysis was performed, and cytokine concentrations were extrapolated from averages of technical duplicates.
2.6 Statistics
Statistical analyses were performed using GraphPad Prism version 9 (GraphPad, San Diego, CA). Comparisons between exposures within the same time point were analyzed by ordinary one-way ANOVA with Tukey’s multiple-comparisons test. Comparisons of the same exposure across the two time points were analyzed by ordinary one-way ANOVA with Sidak’s multiple comparisons test unless otherwise noted. ROUT analysis was run to identify outliers that were removed. Heat maps were created utilizing the HeatMapper web tool (Wishart Research Group, Alverta, Canada).
3 Results
3.1 Yeast characterization and deposition in murine lungs following oropharyngeal aspiration
Phenotypic evaluation and growth curves were conducted to determine how each yeast species grow in different conditions. Mucicarmine stain is commonly utilized to stain polysaccharide capsules present in C. neoformans cells (Vance, 1961). To determine whether the cells of each yeast species had a capsule surrounding them, murine lungs were overloaded with C. neoformans or V. victoriae cells and stained with mucicarmine. As expected, C. neoformans cells stained a dark magenta color (Supplemental Figure 1A), indicating positive staining of the polysaccharide capsule. In contrast, V. victoriae cells did not stain the same indicative magenta color (Supplemental Figure 1B), suggesting the absence of a capsule surrounding these cells in the growth conditions utilized. Growth curves at optimal and body temperature were also conducted to determine if each yeast could grow at 37°C and potentially proliferate in vivo. As expected, C. neoformans was able to grow at 37°C (Supplemental Figure 2A, red) whereas V. victoriae was not (Supplemental Figure 2B, red). To validate that oropharyngeal aspiration was an appropriate method for pulmonary yeast exposures, mice were exposed to a single dose of yeast and lung tissue was collected and stained with GMS to identify deposited yeast cells. Following a single exposure, yeast cells were detected in the lung tissue of exposed mice specifically within free alveolar spaces, or in some cases, within alveolar macrophages (Supplemental Figure 3). The deposition was localized to the terminal bronchioles and alveolar duct junctions or within alveolar spaces (Supplemental Figure 3). This confirmed that the oropharyngeal aspiration effectively delivered yeast into the lungs of mice.
3.2 C. neoformans and V. victoriae induced airway inflammation
Given the frequent detection of yeasts in indoor and outdoor environments, mice were repeatedly exposed to C. neoformans and V. victoriae, or PBS, to mimic a sustained pulmonary exposure over time (Levitz, 1991; Rush et al., 2021). One day following repeated PBS aspiration, mild inflammation, and mucous production were observed in the lungs of control mice (Figures 2A, B). Furthermore, the minor inflammatory response persisted 21-days post final exposure (Figures 2A, B). No GMS-positive cells were detected in the lungs at either time point following PBS exposure (Figure 2C). Along with mild mucous production, moderate perivascular and peribronchiolar inflammation was observed one day following repeated 104 C. neoformans exposure (Figures 2A, B). C. neoformans cells were also detected in the lung tissue as free cells and within alveolar histiocytes as shown by GMS and mucicarmine staining (Figure 2C; Supplementary Figure 4A). By 21-days post final C. neoformans exposure, the inflammation persisted, and granuloma formation was observed (Figure 2A). There was also moderate to marked mucous production and goblet cell hyperplasia present at 21 days post final exposure (Figure 2B). The yeast cells were still detectable at this time point, sometimes within lesions (Figure 2C; Supplementary Figure 4B), and increased in number as evidenced by histological counting. As an inflammatory response was evident following 104 C. neoformans exposure and for concerns of morbidity, a higher dose of C. neoformans was not considered. Together, these findings suggest that C. neoformans cells persist in the lungs of exposed mice and initiated a strong pulmonary inflammatory response over time.
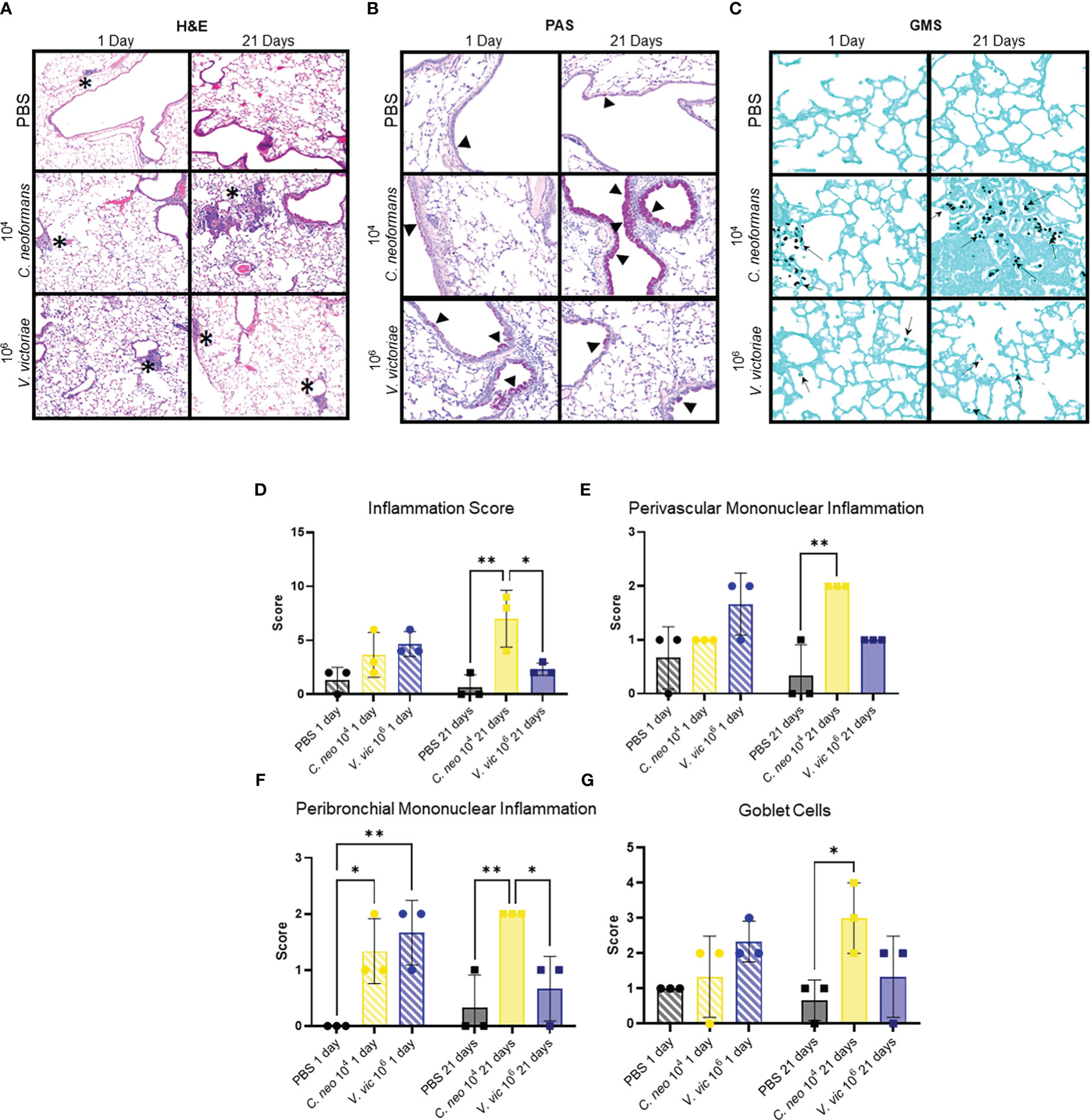
Figure 2 C. neoformans-induced inflammation worsens over time, whereas V. victoriae-induced inflammation resolves. (A–C) Representative micrographs of stained lung sections from mice one day (left) or 21 days (right) following repeated exposure to PBS (top), 104 C. neoformans (middle), or 106 V. victoriae cells (bottom). (A) H&E stain, 100x magnification. (B) PAS-stain, 200x magnification. (C) GMS-stain, 400x magnification. (D) Inflammation, (E) Perivascular Mononuclear Inflammation, (F) Peribronchial Mononuclear Inflammation and (G) Goblet Cells histology scores of lungs following repeated exposure to PBS (black), 104 C. neoformans cells (yellow) or 106 V. victoriae cells (blue) at 1 day post final exposure (circles, crossed bars, left) or 21 days post final exposure (squares, solid bars, right). n=3 per group, *P<0.05, **P < 0.01. P values were determined by ordinary one-way ANOVA with Tukey’s multiple comparisons test comparing each exposure together for the same timepoint.
Following repeated exposure to 106 V. victoriae cells, mice exhibited perivascular and peribronchiolar inflammation including nodular inflammation (Figure 2A). Moderate mucous production and goblet cell hyperplasia were present, and GMS-positive histiocytes were detected at 1-day post final exposure (Figures 2B, C). No mucicarmine-positive stained yeasts were visualized following V. victoriae exposure (data not shown). By 21 days post final V. victoriae exposure (106 cells), the inflammation and mucous production had started to resolve (Figures 2A, B). Still, GMS-positive histiocytes were detected in exposed mice (Figure 2C). In comparison to exposure to 104 C. neoformans cells, results indicated that V. victoriae cells also persist within histiocytes in the lung over time; however, the observed pulmonary inflammation started to resolve by 21 days post final exposure unlike with C. neoformans. Furthermore, mice did not lose significant weight and appeared healthy throughout the studies suggesting that an immunogenic response, but not a pathogenic infection, was achieved utilizing these doses and timeframes as intended.
Exposure to each of the yeasts elicited a mononuclear inflammatory response inclusive of lymphocytes and plasma cells (Figure 2A). C. neoformans exposure also elicited a mixed response including neutrophils and granuloma formation with macrophages and multinucleated giant cells (Figure 2A; Supplementary Table 2). Scores for total, perivascular mononuclear, and peribronchial mononuclear inflammation were provided by a blinded veterinary pathologist and results indicated that repeated exposure to 104 C. neoformans and 106 V. victoriae cells induced a similar pulmonary inflammatory response (Figures 2D–F). Furthermore, these exposure groups also had similar goblet cell scores (Figure 2G). Only C. neoformans-exposed mice had detectable free yeast cells, whereas all V. victoriae-exposed mice had GMS-positive histocytes (Supplementary Table 2). These results suggest that V. victoriae had been phagocytosed by immune cells.
Following repeated exposure to 104 V. victoriae cells, the same dose as C. neoformans, mice exhibited very little to no inflammation, mucous production, or goblet cell hyperplasia one day post final exposure (Figures 3A, B; Supplementary Table 2); however, GMS-positive histiocytes were present in the lung tissue (Figure 3C; Supplemental Table 2). By 21 days post final V. victoriae exposure (104 cells), little to no inflammation or mucous production remained (Figures 3D, E; Supplementary Table 2). Surprisingly, GMS-positive histiocytes were still detected in the lung (Figure 3F; Supplementary Table 2). Given that exposure to 104 V. victoriae cells did not elicit an immune response, a higher, immunogenic dose of 106 V. victoriae cells was used to further characterize the pulmonary immune response to V. victoriae.
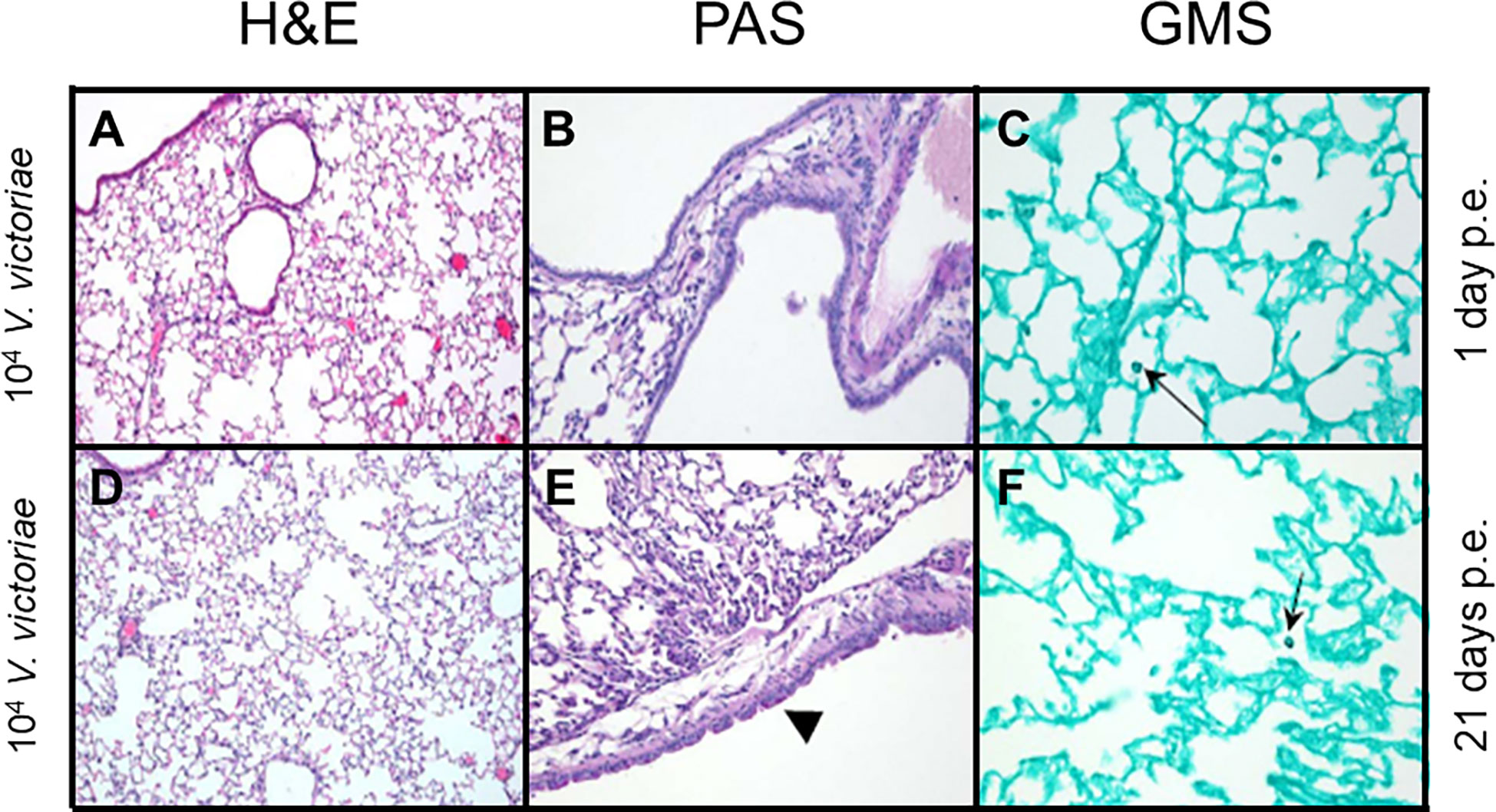
Figure 3 Inflammation, airway changes, and yeast infiltration in lungs of mice after repeated exposure to 104 V. victoriae cells. Representative micrographs of stained sections of lung from mice 1 day (top) and 21 days (bottom) following repeated exposure 104 V. victoriae cells. Goblet cells are by arrowheads, and yeast cells by arrows. (A, D) H&E stain, 100x magnification. (B, E) PAS-stain, 200x magnification. (C, F) GMS-stain, 400x magnification.
3.3 C. neoformans and V. victoriae impacts on myeloid populations in BALF
Cryptococcus neoformans has a polysaccharide capsule that protects the cells from phagocytosis (Yang et al., 2017), whereas V. victoriae does not; however, beta-glucans present in both yeast cell walls are known to stimulate innate immune cells (Goodridge et al., 2009). Given this, the impact of repeated exposure to each yeast on the BALF myeloid cell population was examined (Supplementary Figure 5A). Neither yeast significantly increased myeloid populations of interest in the BALF by one day post final exposure compared to PBS exposed mice (Figure 4). Repeated exposure to 106 V. victoriae cells increased Ly6Chi/med monocytes, CD11b+ DCs, and CD103+ DCs by 1-day post final exposure compared to C. neoformans exposure (Figures 4C–E).
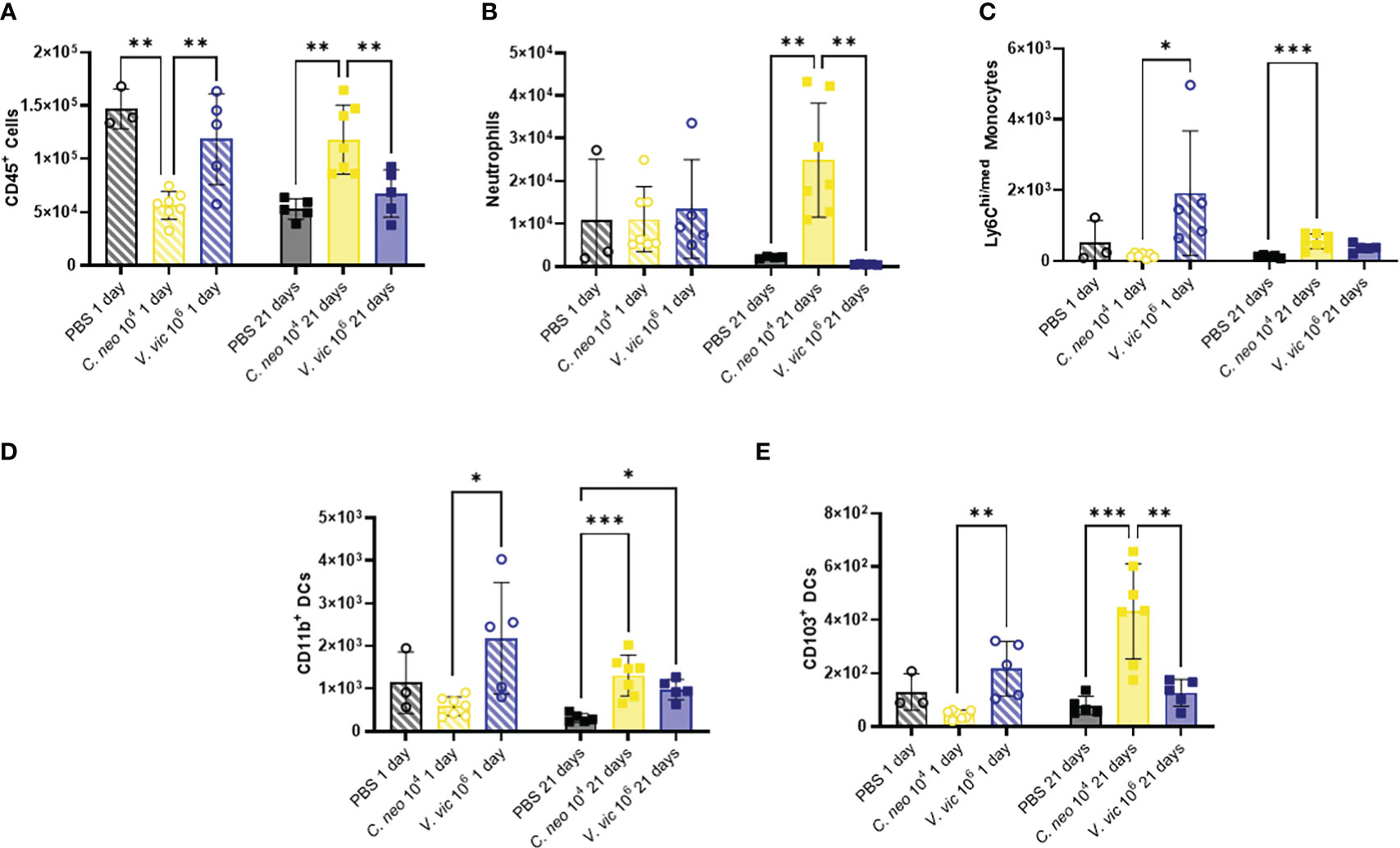
Figure 4 C. neoformans exposure has a stronger impact on myeloid populations in the BALF over time compared to V. victoriae. Cell quantifications (numbers) in BALF following repeated exposure to PBS (black), 104 C. neoformans cells (yellow) or 106 V. victoriae cells (blue) at 1 day post final exposure (circles, crossed bars, left) or 21 days post final exposure (squares, solid bars, right). (A) CD45+ Cells, (B) Neutrophils, (C) Ly6Chi/med Monocytes, (D) CD11b+ Dendritic Cells, and (E) CD103+ Dendritic Cells. n=3-7 per group, *P<0.05, **P < 0.01, ***P < 0.001. P values were determined by ordinary one-way ANOVA with Tukey’s multiple comparisons test comparing each exposure together for the same timepoint.
Repeated C. neoformans exposure increased total CD45+ cells, neutrophils, Ly6Chi/med monocytes, CD11b+ DCs and CD103+ DCs by 21 days post final exposure (Figure 4) compared to PBS control. Following repeated exposure to 106 V. victoriae cells, only a significant increase in CD11b+ DCs remained after 21 days post final exposure (Figure 4D) compared to PBS control. Surprisingly, neither yeast exposure increased eosinophil nor macrophage quantifications in the BALF (Supplementary Figure 6). There were no significant differences in cellular populations in the BAL-depleted lungs that was not evident in the BALF (Supplementary Figures 7–9). Taken together, these findings indicate that repeated exposure to 106 V. victoriae cells elicited a myeloid-driven immune response at 1-day post final exposure that was largely resolved by 21 days post final exposure, whereas repeated exposure to 104 C. neoformans cells had a delayed impact on myeloid immune responses.
3.4 Lymphoid populations in BALF following yeast exposures
Lymphocytes are known to play an important role in the immune response against C. neoformans (Muth and Murphy, 1995; He et al., 2017); therefore, lymphocytic cell populations were characterized (Supplementary Figure 5B). There were no significant differences in lymphoid cell populations in the BALF 1-day post final exposure between yeast-exposed mice and PBS controls (Figure 5). BALF lymphocyte populations increased 21 days following repeated yeast exposure. Specifically, repeated exposure to 104 C. neoformans cells resulted in increased total lymphocytes, B cells, Natural Killer cells, total T cells, CD4+ T cells, and CD8+ T cells compared to PBS exposed controls (Figure 5). In contrast, only total T cells and CD4+ T cells were increased by 21 days post final exposure following repeated exposure to the 106 cell dose of V. victoriae (Figures 5D, E). These findings suggest that repeated exposure to both yeast species elicited a strong lymphoid response, specifically CD4+ T cells, by 21 days post final exposure.
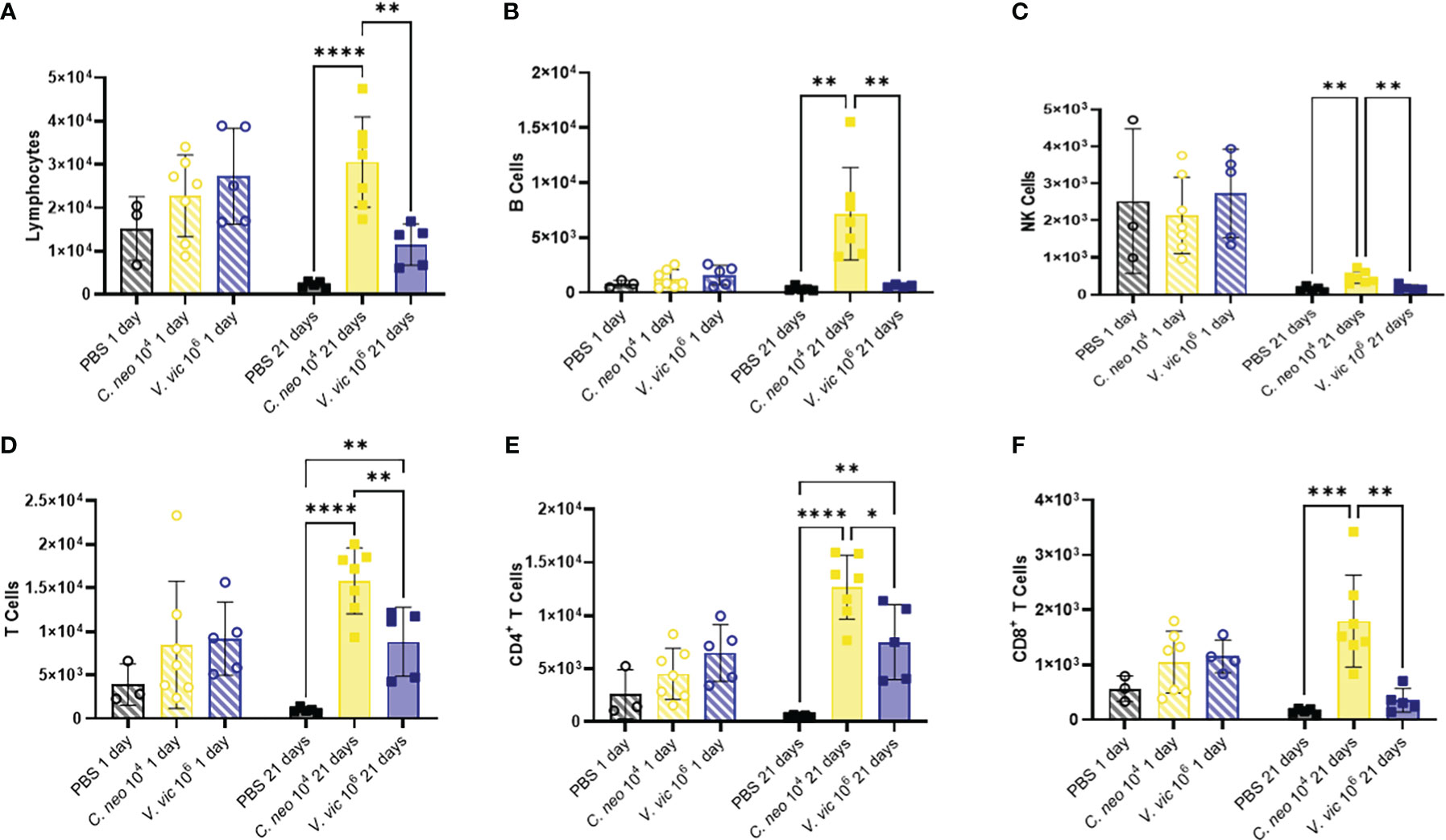
Figure 5 Lymphoid populations are elevated in the BALF 21 days following repeated yeast exposure. Cell populations in BALF following repeated exposure to PBS (black), 104 C. neoformans cells (yellow) or 106 V. victoriae cells (blue) at 1 day post final exposure (circles, crossed bars, left) or 21 days post final exposure (squares, solid bars, right). Cells = cell number. (A) Lymphocytes, (B) B Cells, (C) NK Cells, (D) T Cells, (E) CD4+ T Cells, and (F) CD8+ T Cells. n=3-7 per group, *P<0.05, **P < 0.01, ***P < 0.001, ****P < 0.0001. P values were determined by ordinary one-way ANOVA with Tukey’s multiple comparisons test comparing each exposure together for the same timepoint.
Research has shown that immune cell infiltration worsens over time following pulmonary exposure to C. neoformans (Goldman et al., 2006); however, no studies exist examining the potential exacerbation of, or recovery from, the cellular response following pulmonary exposure to environmental yeasts. Therefore, the differences in concentrations of cell populations between one-day and 21 days post final yeast exposure were examined. Repeated exposure to 104 C. neoformans cells continued to cause an increase in cell populations in the BALF after the final exposure. In contrast, the cellular impacts following repeated exposure to 106 V. victoriae cells start to decrease over time after the final exposure (Figure 6).
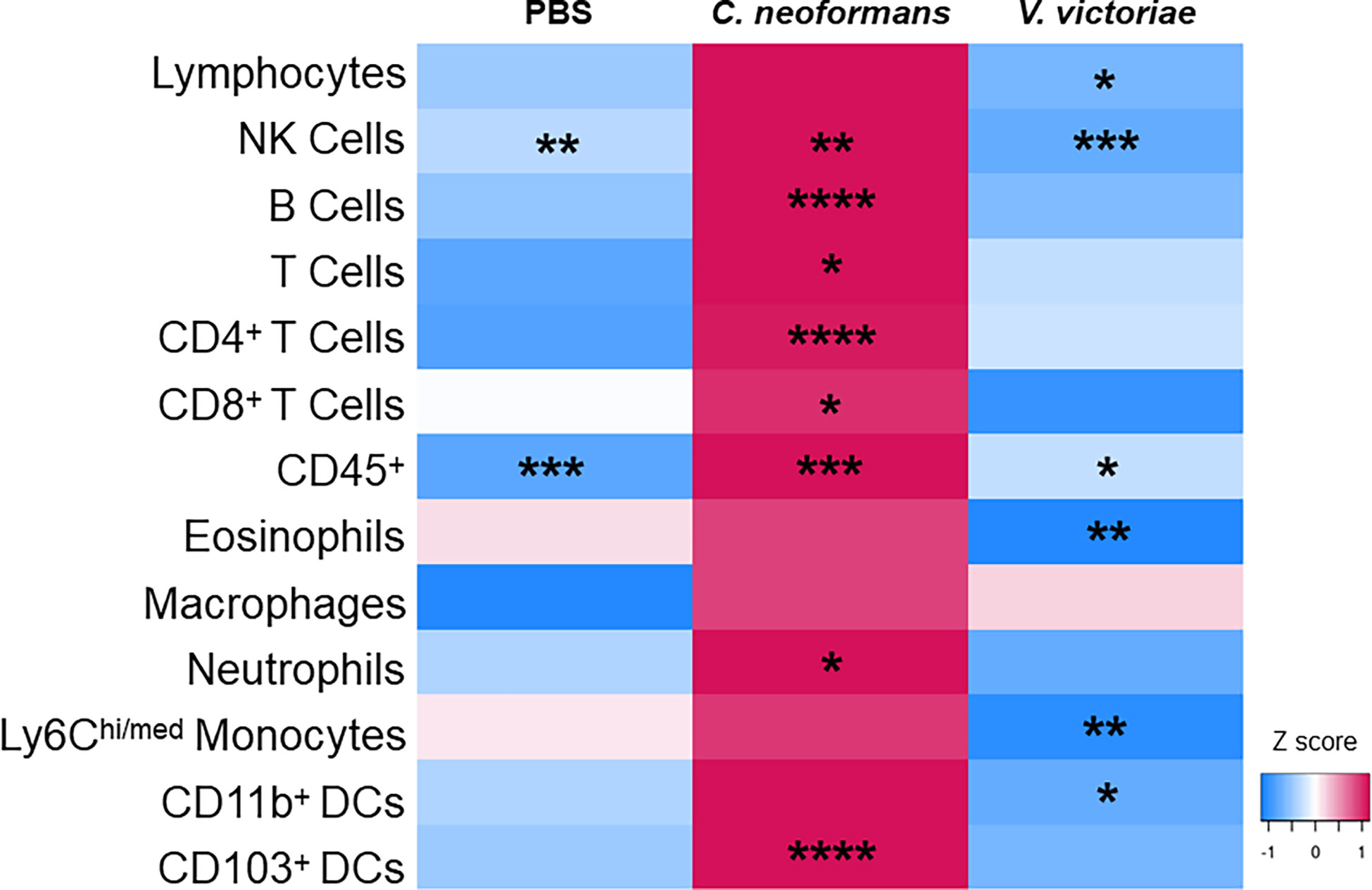
Figure 6 Repeated C. neoformans exposure continues to increase cell populations in the BALF at 21 days post final exposure. Mean cell quantifications in BALF following repeated exposure to PBS, 104 C. neoformans cells or 106 V. victoriae cells at 1 day post final exposure compared to 21 days post final exposure. n=3-7 per group. Values used for this heatmap were obtained by calculating the average quantities at 21 days post final exposure and subtracting the average quantities at 1 day post final exposure. A positive Z score (pink) indicates that the quantification of cells increased at 21 days post final exposure compared to 1 day post final exposure. Negative Z scores (blue) indicate that the cell quantifications decreased by 21 days post final exposure compared to 1 day post final exposure. Asterisks overlaying the heatmap indicate P values comparing the quantifications of each time point for the same exposure. *P<0.05, **P < 0.01, ***P < 0.001, ****P < 0.0001. P values were determined by ordinary one-way ANOVA with Sidak’s multiple comparisons test comparing the same exposure at 1 day post final exposure and 21 days post final exposure.
3.5 Lung cytokine responses following repeated yeast exposures
The Th2 cytokines IL-4, IL-5, and IL-13 play well-established roles in exacerbating allergic airway disease, mainly through their activation of effector cells and subsequent responses (Nakajima and Takatsu, 2007; Gour and Wills-Karp, 2015). Eotaxin is a potent chemoattractant for eosinophils and an important cell type in allergic airway pathogenesis (Conroy and Williams, 2001). Furthermore, IL-33 is a cytokine produced by cells of the lung that plays an important role in type-2 immunity and allergic inflammation (Chan et al., 2019). Given the significant influence of these cytokines in airway responses, a custom multiplex was developed to measure these cytokines in the lungs of mice repeatedly exposed to each yeast. Surprisingly, only IL-4 and IL-5 were elevated one day following repeated C. neoformans exposure compared to PBS control (Figure 7). There were slight, but nonsignificant, increases in the levels of IL-13, Eotaxin, and IL-33 one day post final exposure to either yeast compared to control. By 21 days post final exposure, there were no significant differences in the cytokine response of mice exposed to yeast or PBS control, suggesting that the cytokine response occurs during exposure and resolves by 21 days post final exposure.
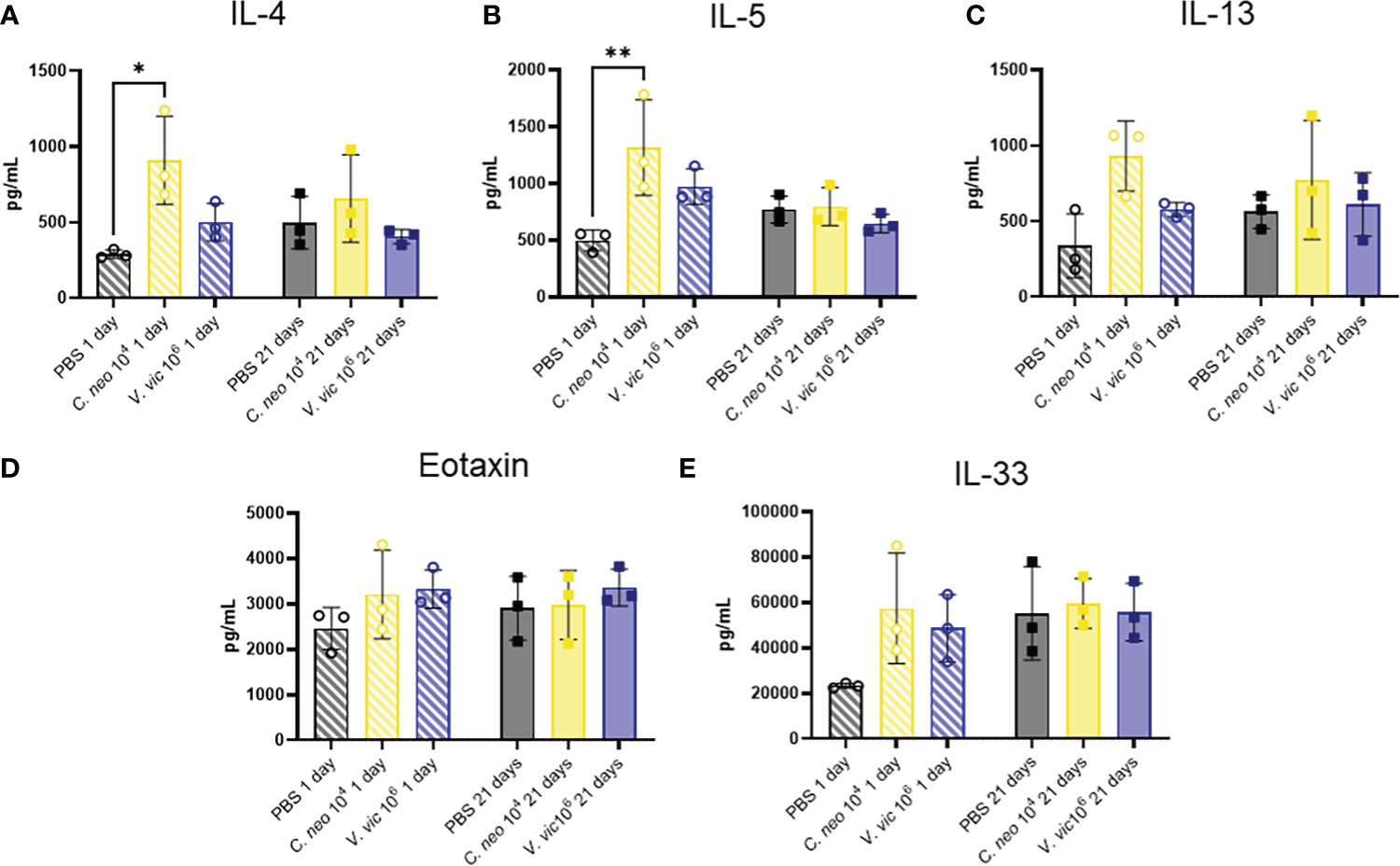
Figure 7 C. neoformans increases IL-4 and IL-5 in lung one day following repeated exposure. Cytokine concentrations in lung homogenate were determined via Luminex after repeated exposure to PBS (black), 104 C. neoformans cells (yellow) or 106 V. victoriae cells (blue) at 1 day post final exposure (circles, crossed bars, left) or 21 days post final exposure (squares, solid bars, right). (A) IL-4, (B) IL-5, (C) IL-13, (D) Eotaxin (CCL11), and (E) IL-33. n=3 per group, *P < 0.05, **P < 0.01. P values were determined by ordinary one-way ANOVA with Tukey’s multiple comparisons test comparing each exposure together for the same timepoint.
4 Discussion
Allergic airway diseases such as asthma are a growing concern in industrialized nations like the United States (Barnes, 2004) and environmental microbial exposures have been shown to influence these diseases (Black et al., 2000). The adverse human health effects associated with environmental fungal exposures have heightened public awareness, and the development of modern methods of fungal detection in indoor environments have highlighted the need to study yeasts (Dotterud et al., 1995; Park et al., 2008; Dannemiller et al., 2014; Dannemiller et al., 2016; Rush et al., 2021). To the authors’ knowledge, this is the first in vivo study examining the pulmonary immune response to the environmental yeast species, Vishniacozyma victoriae. This is an important first study given the frequent and abundant detection of this species in indoor environments and the use of this yeast in commercial sectors. This study sought to compare the pulmonary immunological impact following repeated exposure to V. victoriae and C. neoformans. The doses (104 and 106 cells) chosen for this study align with concentrations identified from environmental assessments (Levitz, 1991; Rush et al., 2021). Based on preliminary epidemiological findings, it was hypothesized that V. victoriae would initiate a different pulmonary immune response compared to C. neoformans.
Pulmonary C. neoformans exposures exacerbate allergic airway responses (Goldman et al., 2006; Campuzano and Wormley, 2018). In this study, repeated exposure to 104 C. neoformans cells elicited a mixed response including an influx of neutrophils and granuloma formation with macrophages and multinucleated giant cells by 21 days post final exposure. This response aligns with other studies examining this yeast that found granuloma formation following pulmonary C. neoformans exposure (Goldman et al., 2006). Unexpectedly, mice repeatedly exposed to 104 C. neoformans cells had decreased total CD45+ cells and macrophages in the BALF 1 day post final exposure compared to PBS-exposed controls. Given that macrophages are the most abundant immune cell in the lung, this response may be due to the ability of C. neoformans to downregulate macrophages (Siegemund and Alber, 2008). In addition, activated macrophages following C. neoformans exposure may have transitioned to a “foam cell” type within granulomas and would therefore be more difficult to remove with lavage (Normile et al., 2020), which could also explain the decrease in the macrophage population. While not assessed in these studies, C. neoformans has been reported to initiate polarization of macrophages towards an M2 phenotype (Eastman et al., 2015). These cells are known to promote allergic airway responses (Saradna et al., 2018) and should be further investigated following future Cryptococcus yeast exposure studies.
Repeated exposure to C. neoformans resulted in an increase in both CD11b+ and CD103+ DCs and total, CD4+, and CD8+ T cells. As CD11b+ and CD103+ DCs present antigen to CD4+ and CD8+ T cells, respectively, the increase in these populations agree with results from other studies that show C. neoformans exposure upregulates dendritic cell activity (Wozniak et al., 2006; Siegemund and Alber, 2008; Wozniak, 2018). Overall, repeated exposure to an immunogenic dose of 106 V. victoriae cells initiated an increased myeloid response 1 day post final exposure whereas exposure to 104 C. neoformans cells initiated an increased myeloid response by 21 days post final exposure. This result may be due to the presence of beta glucans in the cell wall of V. victoriae cells, which are known to stimulate innate immune responses via Dectin-1 (Goodridge et al., 2009; Camilli et al., 2018). However, unlike V. victoriae, C. neoformans is able to grow at body temperature (Supplemental Figure 2A) and has a capsule that hides the beta glucans (Supplemental Figure 1A) that serves as an immune evasion mechanism. The delayed myeloid response may be in response to in vivo replication of C. neoformans and renewed presence of beta glucans present in the yeast cell wall. Dectin-1 is a pattern recognition receptor highly expressed on dendritic cells (Goodridge et al., 2009) and when stimulated, initiates dendritic cells to produce inflammatory cytokines that are essential for anti-fungal immunity (Elder et al., 2017). This is consistent with the observation that dendritic cells increased 1 day post repeated exposure to 106 cells of V. victoriae and 21 days following repeated exposure to 104 C. neoformans cells.
Repeated exposure to 104 V. victoriae cells resulted in very little inflammation, airway remodeling, or cellular response (data not shown). This is not unexpected as V. victoriae lacks a capsule (Supplementary Figure 1B) and has not been previously determined to be pathogenic given its inability to replicate at body temperature (Supplementary Figure 2B) (Montes et al., 1999; de Menezes et al., 2019; Lutz et al., 2020; Rusinova-Videva et al., 2022). Following this observation and given that repeated exposure to 106 V. victoriae cells resulted in a stronger response, the immunogenic dose of 106 V. victoriae was chosen to further characterize the pulmonary immune response following exposure. The authors recognize that the comparison between the yeasts using the same dose would have been ideal; however, it was important that the selected dose initiate an immune response substantial enough to characterize mechanistically, regardless of the type of immune response. Increasing the dose of C. neoformans to mirror that of the increased V. victoriae dose could have resulted in infection and consequently, mortality. This was not the intended response of C. neoformans exposures for this study, but only to induce airway inflammation; therefore, the C. neoformans dose for exposure remained at 104 cells.
Although repeated exposure to C. neoformans initiated a strong lymphoid response including increased B cells, total T, CD4+ T and CD8+ T cells, only total and CD4+ T cells were increased compared to PBS-exposed controls by 21 days post final exposure to the immunogenic dose of V. victoriae (106 cells). This finding aligns with the increase in CD11b+ DCs resulting from V. victoriae exposure, as CD11b+ DCs present antigen to CD4+ T cells (Balan et al., 2019). The increase in CD11b+ DC activity and numbers of CD4+ T cells should be further investigated as allergen-specific CD4+ T cells are known drivers of allergic pulmonary responses, which may implicate the contribution of V. victoriae on allergic airway diseases such as asthma (Ling and Luster, 2016; Muehling et al., 2017; Jeong and Lee, 2021).
C. neoformans is able to persist in the lung and propagate in vivo (Goldman et al., 2000; Kozubowski and Heitman, 2012). The polysaccharide capsule surrounding C. neoformans cells is a known immune evasion mechanism (Campo et al., 2006); therefore, detectable yeast cells in the lung 21 days after final exposure was not surprising. The cellular immune responses in the BALF of mice repeatedly exposed to 104 C. neoformans cells persisted over time as these cellular populations continued to increase from 1 day to 21 days post final exposure. By 21 days post final exposure, the cellular immune response following repeated V. victoriae exposure (106 cells) started to resolve, unlike the response to 104 C. neoformans cells. This observation is supported by the lack of capsule surrounding V. victoriae (Supplementary Figure 1B) and the hypothesized detection and clearance of V. victoriae by the pulmonary immune cells. Consequently, the detection of engulfed V. victoriae cells in the lungs of exposed mice 21 days after the final exposure was unanticipated. This is of particular interest given the detection of V. victoriae in indoor environments and increasing use of this yeast in commercial settings (Lutz et al., 2020; Rusinova-Videva et al., 2022). Future studies should focus on the V. victoriae cell phagocytosis, digestion, and subsequent clearance by alveolar macrophages to better understand the temporal mechanisms underlying this process. Preliminary analyses conducted in this study suggest V. victoriae does not produce a capsule under optimal laboratory growth conditions or following repeated in vivo exposures. Vishniacozyma victoraie stains differ in regard to the presence of absence of a capsule (de Garcia et al., 2012). Examining the lack of a capsule formation by this yeast and the potential for capsule formation under certain conditions warrant future study (Vitale et al., 2012; Jang et al., 2022). Lastly, three PBS-exposed mice were reported to have GMS+ histiocyte granules. Given that these mice were not exposed to yeast, it is likely that these granules were either artifact or GMS+ stained blood cells, which has been previously reported (Adhya, 2019).
Oropharyngeal aspiration is a commonly utilized technique for reproducible pulmonary exposure (Kinaret et al., 2017). However, the isoflurane anesthesia and aspiration process has been shown to increase damage-associated molecular patterns (DAMPs) and subsequent inflammation in the lung following exposure (Crișan et al., 2016; Roh and Sohn, 2018). As such, mild inflammation and mucous production were observed in the lungs of control mice 1 day following repeated PBS aspiration. Additionally, inflammation scores were elevated in some of the PBS-exposed groups, which is consistent with the induction of DAMPs following aspiration (Tolle and Standiford, 2013). Cellular populations such as total CD45+ cells and NK cells were elevated in all groups at 1-day post final exposure. By 21 days post final exposure, these populations had significantly decreased in the PBS-exposed group. This decrease in cellular infiltrates suggests that the observed inflammatory response in the PBS-exposed group may be a result of the aspiration process itself. To better understand the influence of the aspiration process on the host response, future studies should include a naive group of mice that do not undergo aspiration. In addition, other methods of exposure may better mimic a natural route of exposure, such as utilization of an inhalation chamber. Although studies that use an acoustic generator system for the aerosolization of fungal particles exist (Buskirk et al., 2014; Nayak et al., 2018; Croston et al., 2020), the morphology and structure of yeasts may make these cells more difficult to aerosolize without compromising the immunogenic profile.
Previous reports suggested that V. victoriae may influence the pulmonary immune response differently than pathogenic C. neoformans, and that exposure to V. victoriae may protect against allergic airway diseases (Dannemiller et al., 2016; Rush et al., 2021). The results of the current study suggest that V. victoriae induced a unique pulmonary immune response compared to C. neoformans; however, it cannot be concluded that V. victoriae is protective. Exposure to a single yeast species, rather than exposure to a diversified test article, could partially account for the lack of a protective effect on the pulmonary immune response. Natural exposures contain a variety of fungal species (Adams et al., 2013; Behbod et al., 2015), and it is possible that the association between V. victoriae and asthma may be due to V. victoriae occupying an environmental niche, thus keeping an allergic fungal species from propagating. Alternatively, V. victoriae may require interactions with secondary metabolites from other fungal species in order to elicit a protective effect (Sheridan et al., 2015).
The lack of pronounced cytokine response following exposure to each yeast was unanticipated given that cytokines play an important role in early detection and subsequent clearance of Cryptococcus (Campuzano and Wormley, 2018). Moreover, the lack of a strong eosinophil response compared to PBS-exposed controls was also unexpected, although an increased trend following exposure to 106 V. victoriae cells may be appreciated. Given that samples were collected and analyzed at two timepoints, 1 day and 21 days post final exposure, it is possible that these responses are occurring at other timepoints and are therefore being missed. Future studies focused on examining the impact of these species in allergic airway disease models at various timepoints, including mixed fungal exposures that more closely mimic natural exposures in indoor environments, are warranted. Furthermore, measuring pulmonary function would also help to determine the impact of yeast exposure on respiratory health. Additionally, given that responses to the yeast are still detectable 21 days post final exposure, longer recovery timepoints may prove insightful.
In conclusion, V. victoriae and C. neoformans persisted in the lungs of mice following repeated exposure, and C. neoformans initiated a mixed myeloid and lymphoid response including granuloma formation that worsened over time. In contrast, repeated exposure to V. victoriae initiated a CD4+ T cell-driven lymphocytic response that began to resolve by 21 days post final exposure. Given the frequent detection, prevalence and use of V. victoriae in commercial settings, it is imperative that future studies further explore environmental yeast species and their impacts to continue to address this knowledge gap. The results of this study show that repeated exposure to Basidiomycota yeast species resulted in airway inflammation through different mechanisms. The unique inflammatory response elicited by V. victoriae further highlights the need for continued study. By examining the immune response to frequently detected species such as V. victoriae, the role of Basidiomycota yeast species in allergic airway disease can be better understood.
Data availability statement
The original contributions presented in the study are included in the article/Supplementary Material. Further inquiries can be directed to the corresponding author.
Ethics statement
The animal study was reviewed and approved by West Virginia University and CDC-Morgantown Animal Care and Use Committees.
Author contributions
RR, BG, KD, and TC conceived the overall project. RR cultivated the fungal organisms with input from KD. RR, CB, and TC performed the fungal exposures. AL analyzed mucicarmine stained slides and conducted the growth curve studies. RR, CB, AL, and TC performed euthanasia and tissue necropsy. RR performed flow cytometry panel design and analysis with assistance from CB. RR performed cytokine analyses. RR and TC wrote the manuscript with input from all the authors. All authors contributed to the article and approved the submitted version.
Funding
This study was supported in part by the Office of Graduate Research and Education at West Virginia University and an interagency agreement between NIOSH and NIEHS (AES 20003-001-00000) as a collaborative National Toxicology Program research activity. The findings and conclusions in this report are those of the author(s) and do not necessarily represent the official position of the National Institute for Occupational Safety and Health, Centers for Disease Control and Prevention.
Acknowledgments
The authors thank iHisto services (Salem, MA) for their consultation on the histopathological processing and analysis of this study.
Conflict of interest
The authors declare that the research was conducted in the absence of any commercial or financial relationships that could be construed as a potential conflict of interest.
Publisher’s note
All claims expressed in this article are solely those of the authors and do not necessarily represent those of their affiliated organizations, or those of the publisher, the editors and the reviewers. Any product that may be evaluated in this article, or claim that may be made by its manufacturer, is not guaranteed or endorsed by the publisher.
Supplementary material
The Supplementary Material for this article can be found online at: https://www.frontiersin.org/articles/10.3389/fcimb.2023.1067475/full#supplementary-material.
Abbreviations
AAALAC, American Association for Accreditation of Laboratory Animal Care; AAD, Allergic airway disease; BALF, Bronchoalveolar Lavage Fluid; FACS, Flow Cytometry Staining Buffer; NIOSH, National Institute for Occupational Safety and Health; PBS, Phosphate Buffered Saline; T-PER, Tissue Protein Extraction Reagent.
References
Adams, R. I., Miletto, M., Taylor, J. W., Bruns, T. D. (2013). The diversity and distribution of fungi on residential surfaces. PLoS One 8 (11), e78866. doi: 10.1371/journal.pone.0078866
Adams, R. I., Bhangar, S., Pasut, W., Arens, E. A., Taylor, J. W., Lindow, S. E., et al. (2015). Chamber bioaerosol study: outdoor air and human occupants as sources of indoor airborne microbes. PLoS One 10 (5), e0128022. doi: 10.1371/journal.pone.0128022
Adhya, A. K. (2019). Grocott methenamine silver positivity in neutrophils. J. cytology 36 (3), 184–184. doi: 10.4103/JOC.JOC_134_18
Alanio, A., Bretagne, S. (2014). Difficulties with molecular diagnostic tests for mould and yeast infections: where do we stand? Clin. Microbiol. Infect. 20 Suppl 6, 36–41. doi: 10.1111/1469-0691.12617
Anderson, G. P. (2008). Endotyping asthma: new insights into key pathogenic mechanisms in a complex, heterogeneous disease. Lancet (London England) 372 (9643), 1107–1119. doi: 10.1016/S0140-6736(08)61452-X
Balan, S., Saxena, M., Bhardwaj, N. (2019). “Chapter one - dendritic cell subsets and locations,” in International review of cell and molecular biology. Eds. Lhuillier, C., Galluzzi, L. (International Review of Cell and Molecular Biology: Academic Press), 1–68.
Barnes, P. J. (2004). The size of the problem of managing asthma. Respir. Med. 98 Suppl B, S4–S8. doi: 10.1016/j.rmed.2004.07.009
Bateman, E. D., Hurd, S. S., Barnes, P. J., Bousquet, J., Drazen, J. M., Fitzgerald, J. M., et al. (2008). Global strategy for asthma management and prevention: GINA executive summary. Eur. Respir. J. 31 (1), 143–178. doi: 10.1183/09031936.00138707
Behbod, B., Sordillo, J. E., Hoffman, E. B., Datta, S., Webb, T. E., Kwan, D. L., et al. (2015). Asthma and allergy development: contrasting influences of yeasts and other fungal exposures. Clin. Exp. Allergy 45 (1), 154–163. doi: 10.1111/cea.12401
Black, P. N., Udy, A. A., Brodie, S. M. (2000). Sensitivity to fungal allergens is a risk factor for life-threatening asthma. Allergy 55 (5), 501–504. doi: 10.1034/j.1398-9995.2000.00293.x
Buskirk, A. D., Green, B. J., Lemons, A. R., Nayak, A. P., Goldsmith, W. T., Kashon, M. L., et al. (2014). A murine inhalation model to characterize pulmonary exposure to dry aspergillus fumigatus conidia. PLoS One 9 (10), e109855. doi: 10.1371/journal.pone.0109855
Camilli, G., Eren, E., Williams, D. L., Aimanianda, V., Meunier, E., Quintin, J. (2018). Impaired phagocytosis directs human monocyte activation in response to fungal derived β-glucan particles. Eur. J. Immunol. 48 (5), 757–770. doi: 10.1002/eji.201747224
Campo, P., Kalra, H., Levin, L., Reponen, T., Olds, R., Lummus, Z., et al. (2006). Influence of dog ownership and high endotoxin on wheezing and atopy during infancy. J. Allergy Clin. Immunol. 118 (6), 1271–1278. doi: 10.1016/j.jaci.2006.08.008
Campuzano, A., Wormley, F. L. (2018). Innate immunity against cryptococcus, from recognition to elimination. J. Fungi (Basel) 4 (1). doi: 10.3390/jof4010033
Chan, B. C. L., Lam, C. W., Tam, L.-S., Wong, C. K. (2019). IL33: Roles in allergic inflammation and therapeutic perspectives. Front. Immunol. 10, 364. doi: 10.3389/fimmu.2019.00364
Conroy, D. M., Williams, T. J. (2001). Eotaxin and the attraction of eosinophils to the asthmatic lung. Respir. Res. 2 (3), 150. doi: 10.1186/rr52
Crișan, T. O., Netea, M. G., Joosten, L. A. (2016). Innate immune memory: Implications for host responses to damage-associated molecular patterns. Eur. J. Immunol. 46 (4), 817–828. doi: 10.1002/eji.201545497
Croston, T. L., Lemons, A. R., Barnes, M. A., Goldsmith, W. T., Orandle, M. S., Nayak, A. P., et al. (2020). Inhalation of stachybotrys chartarum fragments induces pulmonary arterial remodeling. Am. J. Respir. Cell Mol. Biol. 62 (5), 563–576. doi: 10.1165/rcmb.2019-0221OC
Dannemiller, K. C., Mendell, M. J., Macher, J. M., Kumagai, K., Bradman, A., Holland, N., et al. (2014). Next-generation DNA sequencing reveals that low fungal diversity in house dust is associated with childhood asthma development. Indoor Air 24 (3), 236–247. doi: 10.1111/ina.12072
Dannemiller, K. C., Gent, J. F., Leaderer, B. P., Peccia, J. (2016). Indoor microbial communities: Influence on asthma severity in atopic and nonatopic children. J. Allergy Clin. Immunol. 138 (1), 76–83.e1. doi: 10.1016/j.jaci.2015.11.027
de Garcia, V., Zalar, P., Brizzio, S., Gunde-Cimerman, N., van Broock, M. (2012). Cryptococcus species (Tremellales) from glacial biomes in the southern (Patagonia) and northern (Svalbard) hemispheres. FEMS Microbiol. Ecol. 82 (2), 523–539. doi: 10.1111/j.1574-6941.2012.01465.x
de Menezes, G. C. A., Amorim, S. S., Gonçalves, V. N., Godinho, V. M., Simões, J. C., Rosa, C. A., et al. (2019). Diversity, distribution, and ecology of fungi in the seasonal snow of Antarctica. Microorganisms 7 (10), 445. doi: 10.3390/microorganisms7100445
Denning, D. W., Pashley, C., Hartl, D., Wardlaw, A., Godet, C., Del Giacco, S., et al. (2014). Fungal allergy in asthma-state of the art and research needs. Clin. Trans. Allergy 4, 14–14. doi: 10.1186/2045-7022-4-14
Dotterud, L. K., Vorland, L. H., Falk, E. S. (1995). Viable fungi in indoor air in homes and schools in the sor-varanger community during winter. Pediatr. Allergy Immunol. 6 (4), 181–186. doi: 10.1111/j.1399-3038.1995.tb00282.x
Eastman, A. J., He, X., Qiu, Y., Davis, M. J., Vedula, P., Lyons, D. M., et al. (2015). Cryptococcal heat shock protein 70 homolog Ssa1 contributes to pulmonary expansion of cryptococcus neoformans during the afferent phase of the immune response by promoting macrophage M2 polarization. J. Immunol. 194 (12), 5999–6010. doi: 10.4049/jimmunol.1402719
Eder, W., Ege, M. J., von Mutius, E. (2006). The asthma epidemic. N Engl. J. Med. 355 (21), 2226–2235. doi: 10.1056/NEJMra054308
Elder, M. J., Webster, S. J., Chee, R., Williams, D. L., Hill Gaston, J. S., Goodall, J. C. (2017). β-glucan size controls dectin-1-Mediated immune responses in human dendritic cells by regulating IL-1β production. Front. Immunol. 8. doi: 10.3389/fimmu.2017.00791
Environment and Health Data Portal (2020) Asthma prevalence - children ever diagnosed with asthma (ages 0-13 years) - percentage, 2017, neighborhood (NYC KIDS)]. Available at: http://a816-dohbesp.nyc.gov/IndicatorPublic/VisualizationData.aspx?id=2392,4466a0,11,Map,Percentage,2017.
Goldman, D. L., Lee, S. C., Mednick, A. J., Montella, L., Casadevall, A. (2000). Persistent cryptococcus neoformans pulmonary infection in the rat is associated with intracellular parasitism, decreased inducible nitric oxide synthase expression, and altered antibody responsiveness to cryptococcal polysaccharide. Infect. Immun. 68 (2), 832–838. doi: 10.1128/IAI.68.2.832-838.2000
Goldman, D. L., Davis, J., Bommarito, F., Shao, X., Casadevall, A. (2006). Enhanced allergic inflammation and airway responsiveness in rats with chronic cryptococcus neoformans infection: potential role for fungal pulmonary infection in the pathogenesis of asthma. J. Infect. Dis. 193 (8), 1178–1186. doi: 10.1086/501363
Goldman, D. L., Huffnagle, G. B. (2009). Potential contribution of fungal infection and colonization to the development of allergy. Med. mycology 47 (5), 445–456. doi: 10.1080/13693780802641904
Goodridge, H. S., Wolf, A. J., Underhill, D. M. (2009). Beta-glucan recognition by the innate immune system. Immunol. Rev. 230 (1), 38–50. doi: 10.1111/j.1600-065X.2009.00793.x
Gour, N., Wills-Karp, M. (2015). IL-4 and IL-13 signaling in allergic airway disease. Cytokine 75 (1), 68–78. doi: 10.1016/j.cyto.2015.05.014
Green, B. J. (2018). Emerging insights into the occupational mycobiome. Curr. Allergy Asthma Rep. 18 (11), 62. doi: 10.1007/s11882-018-0818-2
He, Q., Ding, Y., Zhou, W., Li, H., Zhang, M., Shi, Y., et al. (2017). Clinical features of pulmonary cryptococcosis among patients with different levels of peripheral blood CD4+ T lymphocyte counts. BMC Infect. Dis. 17 (1), 768. doi: 10.1186/s12879-017-2865-z
Howell, D., Verma, H., Ho, K. S., Narasimhan, B., Steiger, D., Rogers, L., et al. (2021). Asthma and COVID-19: lessons learned and questions that remain. Expert Rev. Respir. Med. 15 (11), 1377–1386. doi: 10.1080/17476348.2021.1985763
Idnurm, A., Lin, X. (2015). Rising to the challenge of multiple cryptococcus species and the diseases they cause. Fungal Genet. Biol. 78, 1–6. doi: 10.1016/j.fgb.2015.05.002
Jang, E. H., Kim, J.-S., Yu, S.-R., Bahn, Y.-S. (2022). Unraveling capsule biosynthesis and signaling networks in cryptococcus neoformans. Microbiol. Spectr. 10 (6), e0286622. doi: 10.1128/spectrum.02866-22
Jartti, T., Bønnelykke, K., Elenius, V., Feleszko, W. (2020). Role of viruses in asthma. Semin. Immunopathol. 42 (1), 61–74. doi: 10.1007/s00281-020-00781-5
Jeong, J., Lee, H. K. (2021). The role of CD4(+) T cells and microbiota in the pathogenesis of asthma. Int. J. Mol. Sci. 22 (21). doi: 10.3390/ijms222111822
Kinaret, P., et al. (2017). Inhalation and oropharyngeal aspiration exposure to rod-like carbon nanotubes induce similar airway inflammation and biological responses in mouse lungs. ACS Nano 11 (1), 291–303. doi: 10.1021/acsnano.6b05652
Kozubowski, L., Heitman, J. (2012). Profiling a killer, the development of cryptococcus neoformans. FEMS Microbiol. Rev. 36 (1), 78–94. doi: 10.1111/j.1574-6976.2011.00286.x
Kraft, M. (2000). The role of bacterial infections in asthma. Clin. Chest Med. 21 (2), 301–313. doi: 10.1016/S0272-5231(05)70268-9
Levetin, E., Horner, W. E., Scott, J. A. (2016). Taxonomy of allergenic fungi. J. Allergy Clin. Immunology: In Pract. 4 (3), 375–385.e1. doi: 10.1016/j.jaip.2015.10.012
Levitz, S. M. (1991). The ecology of cryptococcus neoformans and the epidemiology of cryptococcosis. Rev. Infect. Dis. 13 (6), 1163–1169. doi: 10.1093/clinids/13.6.1163
Lin, S., Fitzgerald, E., Hwang, S.-A., Munsie, J. P., Stark, A. (. (1999). Asthma hospitalization rates and socioeconomic status in new York state (1987-1993). J. Asthma 36 (3), 239–251. doi: 10.3109/02770909909075407
Ling, M. F., Luster, A. D. (2016). Allergen-specific CD4(+) T cells in human asthma. Ann. Am. Thorac. Soc. 13 Suppl 1 (Suppl 1), S25–S30. doi: 10.1513/AnnalsATS.201507-431MG
Lutz, M. C., Lopes, C. A., Sosa, M. C., Sangorrín, M. P. (2020). Semi-commercial testing of regional yeasts selected from north Patagonia Argentina for the biocontrol of pear postharvest decays. Biol. Control 150, 104246. doi: 10.1016/j.biocontrol.2020.104246
Melgert, B. N., Postma, D. S., Kuipers, I., Geerlings, M., Luinge, M. A., Strate, B. W., et al. (2005). Female mice are more susceptible to the development of allergic airway inflammation than male mice. Clin. Exp. Allergy 35 (11), 1496–1503. doi: 10.1111/j.1365-2222.2005.02362.x
Montes, M. J., Belloch, C., Galiana, M., Garcia, M. D., Andrés, C., Ferrer, S., et al. (1999). Polyphasic taxonomy of a novel yeast isolated from antarctic environment; description of cryptococcus victoriae sp. nov. Syst. Appl. Microbiol. 22 (1), 97–105. doi: 10.1016/S0723-2020(99)80032-0
Muehling, L. M., Lawrence, M. G., Woodfolk, J. A. (2017). Pathogenic CD4(+) T cells in patients with asthma. J. Allergy Clin. Immunol. 140 (6), 1523–1540. doi: 10.1016/j.jaci.2017.02.025
Muth, S. M., Murphy, J. W. (1995). Direct anticryptococcal activity of lymphocytes from cryptococcus neoformans-immunized mice. Infect. Immun. 63 (5), 1637–1644. doi: 10.1128/iai.63.5.1637-1644.1995
Nakajima, H., Takatsu, K. (2007). Role of cytokines in allergic airway inflammation. Int. Arch. Allergy Immunol. 142 (4), 265–273. doi: 10.1159/000097357
Nayak, A. P., Croston, T. L., Lemons, A. R., Goldsmith, W. T., Marshall, N. B., Kashon, M., et al. (2018). Aspergillus fumigatus viability drives allergic responses to inhaled conidia. Ann. Allergy Asthma Immunol. 121 (2), 200–210.e2. doi: 10.1016/j.anai.2018.04.008
Normile, T. G., Bryan, A. M., Del Poeta, M. (2020). Animal models of cryptococcus neoformans in identifying immune parameters associated with primary infection and reactivation of latent infection. Front. Immunol. 11. doi: 10.3389/fimmu.2020.581750
Papi, A., Brightling, C., Pedersen, S. E., Reddel, H. K. (2018). Asthma. Lancet 391 (10122), 783–800. doi: 10.1016/S0140-6736(17)33311-1
Park, J. H., Cox-Ganser, J. M., Kreiss, K., White, S. K., Rao, C. Y. (2008). Hydrophilic fungi and ergosterol associated with respiratory illness in a water-damaged building. Environ. Health Perspect. 116 (1), 45–50. doi: 10.1289/ehp.10355
Pitkaranta, M., Meklin, T., Hyvärinen, A., Paulin, L., Auvinen, P., Nevalainen, A., et al. (2008). Analysis of fungal flora in indoor dust by ribosomal DNA sequence analysis, quantitative PCR, and culture. Appl. Environ. Microbiol. 74 (1), 233–244. doi: 10.1128/AEM.00692-07
Roh, J. S., Sohn, D. H. (2018). Damage-associated molecular patterns in inflammatory diseases. Immune Netw. 18 (4). doi: 10.4110/in.2018.18.e27
Rush, R. E., Cochran, S., Haines, S., Acosta, L., Divjan, A., Rundle, A., et al. (2021). Vishniacozyma victoriae (syn. cryptococcus victoriae) in the homes of asthmatic and non-asthmatic children in new York city. J. Expo Sci. Environ. Epidemiol 32 (1), 48–59. doi: 10.1016/j.jaci.2019.12.434
Rusinova-Videva, S., Ognyanov, M., Georgiev, Y., Kambourova, M., Adamov, A., Krasteva, V., et al. (2022). Production and chemical characterization of exopolysaccharides by Antarctic yeasts vishniacozyma victoriae and tremellomycetes sp. Appl. Sci. 12 (4), 1805. doi: 10.3390/app12041805
Saradna, A., Do, D. C., Kumar, S., Fu, Q.-L., Gao, P. (2018). Macrophage polarization and allergic asthma. Transl. Res. 191, 1–14. doi: 10.1016/j.trsl.2017.09.002
Schatz, M., Rosenwasser, L. (2014). The allergic asthma phenotype. J. Allergy Clin. Immunology: In Pract. 2 (6), 645–648. doi: 10.1016/j.jaip.2014.09.004
Sheridan, K. J., Dolan, S. K., Doyle, S. (2015). Endogenous cross-talk of fungal metabolites. Front. Microbiol. 5. doi: 10.3389/fmicb.2014.00732
Siegemund, S., Alber, G. (2008). Cryptococcus neoformans activates bone marrow-derived conventional dendritic cells rather than plasmacytoid dendritic cells and down-regulates macrophages. FEMS Immunol. Med. Microbiol. 52 (3), 417–427. doi: 10.1111/j.1574-695X.2008.00391.x
Tolle, L. B., Standiford, T. J. (2013). Danger-associated molecular patterns (DAMPs) in acute lung injury. J. Pathol. 229 (2), 145–156. doi: 10.1002/path.4124
Vance, A. M. (1961). The use of the mucicarmine stain for a rapid presumptive identification of cryptococcus from culture. Am. J. Med. Technol. 27, 125–128.
Vitale, R. G., Pascuccelli, V., Afeltra, J. (2012). Influence of capsule size on the in vitro activity of antifungal agents against clinical cryptococcus neoformans var. grubii strains. J. Med. Microbiol. 61 (Pt 3), 384–388. doi: 10.1099/jmm.0.036152-0
Wozniak, K. L. (2018). Interactions of cryptococcus with dendritic cells. J. fungi (Basel Switzerland) 4 (1), 36. doi: 10.3390/jof4010036
Wozniak, K. L., Vyas, J. M., Levitz, S. M. (2006). In vivo role of dendritic cells in a murine model of pulmonary cryptococcosis. Infect. Immun. 74 (7), 3817–3824. doi: 10.1128/IAI.00317-06
Keywords: yeast, fungi, inflammation, allergic disease, Vishniacozyma victoriae, Cryptococcus neoformans, exposure
Citation: Rush RE, Blackwood CB, Lemons AR, Dannemiller KC, Green BJ and Croston TL (2023) Persisting Cryptococcus yeast species Vishniacozyma victoriae and Cryptococcus neoformans elicit unique airway inflammation in mice following repeated exposure. Front. Cell. Infect. Microbiol. 13:1067475. doi: 10.3389/fcimb.2023.1067475
Received: 11 October 2022; Accepted: 31 January 2023;
Published: 14 February 2023.
Edited by:
Cheshta Sharma, The University of Texas Health Science Center at San Antonio, United StatesReviewed by:
Jennifer Geddes-McAlister, University of Guelph, CanadaAlthea Campuzano, University of Texas at San Antonio, United States
Copyright © 2023 Rush, Blackwood, Lemons, Dannemiller, Green and Croston. This is an open-access article distributed under the terms of the Creative Commons Attribution License (CC BY). The use, distribution or reproduction in other forums is permitted, provided the original author(s) and the copyright owner(s) are credited and that the original publication in this journal is cited, in accordance with accepted academic practice. No use, distribution or reproduction is permitted which does not comply with these terms.
*Correspondence: Tara L. Croston, eHp1OUBjZGMuZ292