Interactions between microbiota and cervical epithelial, immune, and mucus barrier
- 1Department of Obstetrics and Gynaecology, Tianjin Medical University General Hospital, Tianjin, China
- 2Tianjin Key Laboratory of Female Reproductive Health and Eugenic, Department of Gynecology and Obstetrics, Tianjin Medical University General Hospital, Tianjin, China
The female reproductive tract harbours hundreds of bacterial species and produces numerous metabolites. The uterine cervix is located between the upper and lower parts of the female genital tract. It allows sperm and birth passage and hinders the upward movement of microorganisms into a relatively sterile uterus. It is also the predicted site for sexually transmitted infection (STI), such as Chlamydia, human papilloma virus (HPV), and human immunodeficiency virus (HIV). The healthy cervicovaginal microbiota maintains cervical epithelial barrier integrity and modulates the mucosal immune system. Perturbations of the microbiota composition accompany changes in microbial metabolites that induce local inflammation, damage the cervical epithelial and immune barrier, and increase susceptibility to STI infection and relative disease progression. This review examined the intimate interactions between the cervicovaginal microbiota, relative metabolites, and the cervical epithelial-, immune-, and mucus barrier, and the potent effect of the host-microbiota interaction on specific STI infection. An improved understanding of cervicovaginal microbiota regulation on cervical microenvironment homeostasis might promote advances in diagnostic and therapeutic approaches for various STI diseases.
Introduction
Cervicovaginal microbiota have a mutual relationship with the host. The lower genital tract of women provides an ecological niche for bacterial colonisation. Lactobacillus spp. predominates the microbial flora in a healthy female genital tract, which confers several benefits to the host. It ferments the glucose and maltose of the vaginal epithelial cells to produce lactic acid which maintains vaginal pH at 3.8~4.5. Lactic acid and its associated acidic microenvironment help regulate inflammation. Lactobacilli also secrete bacteriocin (Scillato et al., 2021), which inhibits the proliferation of other microorganisms and has strong epithelial cell adhesion ability, thereby competing with pathogens for living space and nutrient uptake (Kwok et al., 2006). Female genital microbiota can be tightly regulated by host factors such as age, menstrual cycle, sex steroid hormones, race, living habits, immunity, and genetic polymorphism. Microbial metabolites, components, and bacteria can interact with host epithelial cells and resident immune cells, leading to alterations in the local ecosystem and affecting defences against pathogen infection and disease progression. The dominant Lactobacilli flora maintains the health of the reproductive tract, whereas dysbiosis of the microbiota, wherein Lactobacilli are significantly reduced or absent and replaced by pathogenic bacteria is indicative of the development of reproductive tract-associated diseases (Smith and Ravel, 2017).
The female reproductive tract (FRT) can be divided into the lower FRT (vagina and ectocervix) which contains a large microbial presence, and the upper FRT (endocervix, uterus, and oviduct) which is relatively sterile. The cervix includes the ecto- and endocervix and plays a specific role in FRT. On the one hand, the cervix acts as a channel through which human sperm can migrate into the uterus, and as an infant passage after dramatic changes during gestation and parturition. On the other hand, the cervix and mucus should prevent microorganisms in the lower reproductive tract which may induce pelvic inflammatory disease (PID). It is also the site of attack of specific sexually transmitted infection (STI) pathogens including Chlamydia trachomatis (CT), human papillomavirus (HPV), and human immunodeficiency virus (HIV).
A healthy cervicovaginal microbiota (CVM) and the acidic environment formed by the associated metabolites maintain cervical epithelial barrier integrity, stabilise the mucosal immune system, and balance host defence and tolerance with microbes, metabolites, components, and attachment to host cells. In contrast, microbiota dysbiosis and its accompanying changes in microbial metabolites induce an inflammatory response, cause damage to cervical cells, disrupt cervical epithelial and mucosal barriers, stimulate the immune system, and cause an imbalance in cervical homeostasis, thereby inducing various STI diseases. This review highlights recent advances in the understanding of the interactions between the microbiota and the cervical mucosal barrier (epithelial, mucus, and immune), and discusses the implications for cervical-specific STIs.
Normal physiology state
Anatomy and histology of the cervix
The cervix is a cylindrical structure approximately 2-cm long, with the cervical canal in the centre, the inner orifice opening to the uterus, and the outer opening to the vagina. The cervix is divided into the ectocervix and endocervix depending on whether it is exposed to the vagina. The cervix is composed of stroma and epithelial cells separated by a basement membrane. The underlying stroma of the cervix is predominately an extracellular matrix, including type I and type II collagen, and a small amount of type IV collagen (Leppert, 1995). Approximately 70% of the cervix’s extracellular matrix is composed of type I collagen, which plays a crucial role in maintaining tissue integrity (Yellon, 2019). Type IV collagen can be found in the basement membrane as well as enriched in the placenta (Lithgow et al., 2022). Other components included elastin, proteoglycans, hyaluronan, fibroblasts, and scattered immune cells (Tantengco et al., 2021a). Epithelial cells are region-specific, and the endocervix is covered by columnar epithelial cells. The ectocervix and vagina are covered by continuous stratified non-keratinised squamous epithelial cells. The area between the endocervix and ectocervix is the transformation zone, which is covered by squamous columnar epithelial cells. Intercellular junctions and the epithelial cells formed cervical epithelial barrier. The main connections between epithelial cells include tight junctions, adherent junctions, and desmosome junctions (Blaskewicz et al., 2011). Columnar epithelial cells are mainly composed of tight junctions that are directly regulated by oestrogens, cytokines, and growth factors (Grant and Wira, 2003; Wira et al., 2010). The squamous epithelium mainly consists of adhesive junctions and desmosome junctions, while tight junctions are lacking, allowing small molecules to pass through the intercellular space. Pathogenic microorganisms can contact Langerhans cells and CD4+ T cells distributed in the cervix (Hladik et al., 2007). In addition, epithelial cells can act as antigen-presenting cells (APCs) to recognise pathogenic microorganisms and secrete immune components such as antibiotics, cytokines, and chemokines.
Cervical mucus barrier
Cervical mucus is produced by goblet/secretory cells around the crypts in the endocervical canal (Han et al., 2017) and contains mucins (MUCs), immunoglobulins, plasma proteins, lipids, sterols, carbohydrates, inorganic ions, and water. Mucin is a large O-linked glycosylated protein and is the main component of the cervical mucus (Radtke et al., 2012). Currently, 20 mucins are known, which are divided into gels such as MUC5AC, MUC5B, and MUC6, transmembrane proteins such as MUC1 and MUC16, and small soluble molecules (Andersch-Björkman et al., 2007; Radtke et al., 2012). MUC5B and MUC5AC are the main mucins of the cervix (Gipson et al., 1999; Gipson et al., 2001), and MUC5B is the main mucin that affects the properties of the cervical mucus. During the follicular phase, the amount of mucus increases with increasing oestrogen levels, and the mucus gradually becomes thinner. During ovulation, the mucus levels increase by 10–20 times, MUC5B expression reaches its highest level, and the secretion becomes watery, which facilitates the entry of sperm into the upper reproductive tract (Han et al., 2021). During the luteal phase, MUC5B expression decreases owing to the effect of progesterone, and the cervical mucus gradually thickens (Gipson et al., 2001). The levels of immune factors such as IgG/IgA, lactoferrin, interleukin-10 (IL-10), and antimicrobial peptides also show a bimodal distribution with the menstrual cycle (Ulcova-Gallova, 2010), with higher levels in the early stage of the follicular phase, followed by 10-1,000 times decreases in the middle of menstruation, and increases in the later stage of menstruation. However, the levels of IFN-γ, IL-4, IL-6, and IL-12 did not show periodic changes (White et al., 1997; Han et al., 2017). Hughes performed a meta-analysis include 31 article mainly CVL samples, found that antibodies, CC−type chemokines, MMPs, IL−6, IL−16, IL−1RA, G−CSF, GNLY, and ICAM1 were lower in the luteal phase than the follicular phase. IL−1α, HBD−2, and HBD−3 were elevated in the luteal phase. CXCL8, 9, and 10, interferons, TNF, SLPI, elafn, lysozyme, lactoferrin, and IL-1β, 2, 10,12, 13, and 17A show minimal change. Although some constituents showing cyclic variations increase the vulnerability of sperm passaging and pathogen ascent, some cytokines did not change with the cell cycle which may compensate for the risk of ascending infection (mucus increase) during ovaluation (De Tomasi et al., 2019).
The cervical mucus can play a barrier role in both physical and immunological aspects. First, mucin and other substances capture pathogenic microorganism particles and slow down the diffusion speed to gain time for local immune responses (Boukari et al., 2009; Shukair et al., 2013). Second, immune factors such as IgG, IgA, lactoferrin, and SLPI in cervical mucus can inhibit the adhesion and invasion of cervical epithelial cells by pathogenic microorganisms such as CT, and HIV (Radtke et al., 2012; Filardo et al., 2019).
Distribution of local immune cells in the cervix
Studies on cervical immune cells mainly focus on cervical tissue and secretion samples. Neutrophils are the most prevalence immune cells found in cytobrush secretion samples, supported by studies from non-pregnant- (Mohammadi et al., 2020) and pregnant women (Hunter et al., 2016); this differs from samples collected from biopsies (Trifonova et al., 2014). Both cervical and vaginal tissues are mainly composed of APCs and T cells, and most of them are effector memory- or effector T cells, indicating that the cervical region is an organ dominated by cellular immunity (Trifonova et al., 2014). The content of APCs and T cells is higher in the cervix than those in the vagina, with the transformation zone and endocervix having the largest and smallest amounts, respectively (Pudney et al., 2005). In the ectocervix, 37–55% are APC cells (most are macrophages), followed by dendritic cells (DCs), and 23–43% are T lymphocytes. The number of APCs in the endocervix is similar to that in the ectocervix, however, T lymphocytes are approximately half of those in the ectocervix (Trifonova et al., 2014). CD8+ T cells are slightly more abundant than CD4+ T cells, with the latter accounting for approximately 50% of the total CD3+ cells in the endocervix and ectocervix. Th17 cells with CCR5 and CD90 co-expression are the main CD4+T cell population in the cervix (Rodriguez-Garcia et al., 2014).
Human cellular immunity works through the following processes: local APCs recognise pathogenic microorganisms, present antigens to lymphoid tissues, and T cells are stimulated and differentiate into effector T cells in local tissues. Subsequently, some effector T cells develop into memory T cells, which are divided into central memory T cells (TCM) that are stored in the extralymphatic tissue, and effector memory T cells (TEM) which can travel between the blood and extralymphatic tissue and play a role in circulating immune surveillance. Recent work found that some memory T cells also reside in local tissues and become tissue-resident memory T cells (TRMs) (Gebhardt et al., 2013; Sathaliyawala et al., 2013). An increasing number of studies confirmed that circulating memory cells fail to approach the genital tract mucosa at a steady state, while TRMs can quickly play a local immune role without being recirculated through lymphoid tissue (Iijima and Iwasaki, 2014). CD8+TRM (CD69+CD103+) is the predominant fraction in CD8+T cells of cervicovaginal tissue, while others are CD69+ single positive and double negative CD69−CD103− cells, which are also defined as inflammatory mucosal T cells (Tim) (Pattacini et al., 2019). Most CD8+TRMs in the mouse reproductive tract express CD103, which binds to E-cadherin and promotes TRM retention within certain epithelial compartments (Rosato et al., 2017). Only a few CD4+TRMs express CD103, and CD4+TRMs are mainly distributed within memory lymphocyte clusters (MLCs). Once TRM recognises the cognate peptide, it releases cytokines IL-2, TNFα, and IFNγ, thereby upregulating adhesion molecules and chemokines and promoting the recruitment of circulating memory T cells and B cells into tissues (Schenkel et al., 2014; Rosato et al., 2017).
Studies on the effects of the menstrual cycle and menopause on cervical lymphocytes are scarce and controversial. Several studies found that the total number of CD8+T and CD4+T cells in the cervix does not change with the menstrual cycle and menopause (Pudney et al., 2005), and the toxicity of CD8+T cells is unaffected (Rodriguez-Garcia et al., 2020). In addition, endometrial CD8+T cells are inhibited by an increase in transforming growth factor beta (TGF-β) in the secretory phase, but cervical CD8+T cells are insensitive to TGF-β regulation. This means that the cervix can still play an immunoprotective role in preventing pathogenic microorganisms from ascending when the secretory endometrium is in an immunosuppressed state due to embryo implantation (Rodriguez-Garcia et al., 2020). However, other studies show that the menstrual cycle and age can affect the number and function of certain lymphocyte subsets. For example, the level of chemokine (C-C motif) ligand 2 CCL2 and the proportion of local CD4+TRM in the cervix increases during the follicular period (Boily-Larouche et al., 2019). CCL2 is a chemokine found in monocytes/macrophages, T cells, and TRM. The inhibitory effect of progesterone on CCL2 decreases during the follicular phase, and more CD4+TRMs are recruited to the local cervix (Hall and Klein, 2017; Boily-Larouche et al., 2019). In addition, the abundance of CD8+TRM and DC cells in the cervical tissue of postmenopausal women decreases with age (Rodriguez-Garcia et al., 2018). This may be the reason for the increased susceptibility of postmenopausal women to STI (Ghosh et al., 2014) and the second peak of HPV infection at the age of 45 (Rodriguez-Garcia et al., 2018).
Normal cervicovaginal microbiome
The microbiota of the lower genital tract is identified more frequently than that of the endocervix. Using self-collected vaginal secretions samples, five “communities state types” (CSTs) are classified according to the composition and proportion of vaginal microbiota by Ravel et al. Specifically, CST-I, CST-II, CST-III, and CST-V corresponds to a predominance of Lactobacillus crispatus, L. gasseri, L. iners, and L. jensenii, respectively, whereas CST-IV is characterised by a combination of various facultative anaerobes with a low abundance of Lactobacilli. CST IV-A comprises Anaerococcus, Prevotella, Corynebacterium, Peptoniphilus, Finegoldia, and Streptococcus. CST IV-B is characterised by Atopobium, Gardnerella, Mobiluncus, Sneathia, Megasphaera, and other bcteria in the order Clostridiales (Ravel et al., 2011). In 2020, they comprehensively re-identified common vaginal CSTs in 1975 women with different ethnicities; black, white, Hispanic, and Asian. CST IV is divided into CST IV-A, IV-B, and IV-C. CST IV-A has a high level of Candidatus Lachnocurva vaginae (formerly called bacterial vaginosis-associated bacterium-1, BVAB1) and a moderate amount of Gardnerella vaginalis, whereas IV-B has a high abundance of G. vaginalis and a low amount of Ca. L. vaginae. Both IV-A and IV-B contain moderate levels of Atopobium vaginae. CST IV-C is characterised by other diverse anaerobic bacteria and is divided into five sub-CSTs: CST IV-C0 is a homogeneous community with a moderate level of Prevotella spp., CST IV-C1, C2, C4 with Streptococcus spp., Enterococcus spp., Staphylococcus spp, as a dominated flora respectively. CST IV-C3 with Bifidobacterium spp. as dominant flora (France et al., 2020). In addition, “cervicotype” (CT) was proposed by Anathar et al. (Anahtar et al., 2015) by analysing the microbiota community structure of ectocervical swabs. CT1 is primarily dominated by non-iners Lactobacillus, CT2 is dominated by L. iners, CT3 by Gardnerella, and CT4 by diverse anaerobes associated with BV. Buck et al. further defined the structure of vaginal flora with “vagitype” (Fettweis et al., 2019). Although both vagitypes and dominant bacteria refer to bacterial species with a relative abundance of over 30%, each sample can have over two dominant bacterial species, and vagitypes refer to the most dominant and functional bacterial species. Normal vaginal flora is often composed of only one or two dominant bacteria due to the strong adhesion ability of Lactobacillus.
The microbiota in the vagina, endocervix, and uterine cavity of the same female show continuity. The overall microbiota biomass shows a downward trend and bacterial diversity gradually increases. The microbiota in the endocervix is more similar to the vagina than the uterus and is dominated by Lactobacillus. However, the proportion of Lactobacillus in the endocervix is lower than that in the vagina, and the relative abundance of Bacteroides, Pseudomonas, and Prevotella spp. increases. The relative abundance of Lactobacillus in the uterus is lower than in the vagina and endocervix (Chen et al., 2017; Zhang et al., 2021; Wang et al., 2021a). It is suggested that microflora quantity and composition is altered with the rise in genital tract position. The cervix is located between the vagina and uterus, and the characteristics of its microflora are between those of the vagina and uterus, suggesting that it is the transformation zone of bacteria in the reproductive tract.
CVM can be dynamic with the menstrual cycle and age. During menses, increased diversity (Krog et al., 2022) and decreased Lactobacillus abundances (Song et al., 2020) were observed. During the follicular and luteal phases, the abundance of Lactobacillus is expanded, and the vaginal microbiome of more women becomes dominated by Lactobacillus due to the increase of estradiol (Gajer et al., 2012; Song et al., 2020; Jie et al., 2021; Krog et al., 2022; Oerlemans et al., 2022). In postmenopausal women, species richness was decreased, but species diversity was significantly increased (Kim et al., 2021). The abundance of lactobacillus is decreased, while the proportion of anaerobic bacteria is increased (Gliniewicz et al., 2019; Kim et al., 2021). The composition of these communities resembles those of premenopausal women with BV and is correlated with symptoms of vaginal discomfort (Shardell et al., 2021; Mitchell et al., 2021a). However, the bacteria associated with BV nugent score in postmenopausal women were different from premenopausal women, which demonstrated there are differences in microbial community with premenopausal women with BV (Mitchell et al., 2021b).
Many studies also demonstrate that CVM is associated with disease treatment outcomes. For example, some studies reveal that low baseline Lactobacillus (Xiao et al., 2022), L.iners.(Zhou et al., 2022), high G.vaginalis and A. vaginae (Bradshaw et al., 2006; Ferreira et al., 2017) was associated with BV treatment failure and recurrence, although some reports show inconsistent effect. The discrepancy is partly due to different study designs, populations and technology. In addition, the different virulence of species (Mollin et al., 2022; Zhou et al., 2022), and the dynamics of the species in CVM (Xiao et al., 2019), rather than the genus as a whole maybe provide more information to monitor the treatment outcome.
Interaction between microbiota and cervical barrier
Microbiome dysbiosis and metabolite alterations
The microbiota and metabolites changed during cervicovaginal dysbiosis. Lactobacilli are the dominant species in healthy conditions and exclusively utilise sugars such as glycogen and glycogen hydrolysates as carbon and nitrogen sources to produce lactic acid (Amabebe and Anumba, 2018). Lactobacilli produce lactic acid, bacteriocins, and biosurfactants to protect the cervicovaginal environment. Bacterial vaginosis (BV) is one of the most common dysbiosis in the vagina, characterised by a decrease in Lactobacillus and an increase in anaerobic bacteria, such as G. vaginalis, Atopobium spp., and Prevotella spp., which correspond to CST IV-A, IV-B, and IV-C0. Aerobic vaginitis (AV) is a vaginal dysbiosis characterised by the loss of Lactobacillus and an increase in aerobes such as Enterococcus spp., Escherichia coli, Staphylococcus spp., and Streptococcus spp. identified by culture and bacterial sequencing (Wang et al., 2020), which may correspond to CST IV-C1, IV-C2, and IV-C4. A healthy metabolite environment positively correlates with the metabolism of lysolipids, phospholipids, glutathione, and glycogen, but negatively correlates with the metabolism of biogenic amines, lysine, and histidine, and is characterised by high lactic acid levels. Both L. crispatus and L. gasseri produce L-type and D-type lactic acids, L. jensenii only produces D-type lactate, and L. iners only produces L-type lactate (Witkin et al., 2013). L. iners also secretes the cholesterol-dependent cytotoxin, haemolysin. This explains why the dominant vaginal bacteria in healthy women are mostly L. crispatus, followed by L. gasseri and L. jensenii, while L. iners is often associated with pathogenic bacteria, for instance, G. vaginalis. Metabolites associated with BV positively correlate with the metabolism of biogenic amines, lysine, and histidine; however, they negatively correlate with lipid-, glutathione-, and glycogen metabolism. The levels of biogenic amines (putrescine, cadaverine and trimethylamine) and short-chain fatty acids (SCFAs) (especially acetate, butyrate and formate) in BV are high, while the levels of some amino acids (tyrosine and glutamate) in BV are low (Srinivasan et al., 2015; Vitali et al., 2015; Watson and Reid, 2018; Borgogna et al., 2020). The metabolite signature of AV is less characterised than that of BV. A study on premature rupture of membranes (PROM) patients shows that the glycolytic metabolite GalNAc (N-acetylgalactosamine) and sucrose negatively correlate with Streptococcus, Chlamydia, Prevotella, Staphylococcus, Mycobacterium, and Enterobacter genera, which supports the reduction of lactic acid (Liu et al., 2021b). In addition, Streptococcus spp. dominated communities contain slightly higher acetate levels (Gajer et al., 2012).
Immune response induced by cervicovaginal microbiota on cervix
The effect of cervicovaginal bacteria on the cervix is less pronounced than on the vagina. Cervical epithelial cells and immune cells recognize and sense pathogens through pattern recognition receptors (PRRs). Cell-surface PRRs toll-like receptors (TLRs) 1,2,4,5,6 and 3,7,9 (Aflatoonian and Fazeli, 2008; Benjelloun et al., 2020) and intracellular cytosolic pathogen sensing receptors Nod-like receptors (NLRs), NOD1, NOD2, RIG-1, and MDA5 (Ghosh et al., 2013) have been found in the cervix mucosa. TLR1, TLR2, and TLR4-6 detect microbial ligands, TLR2 and TLR4 can recognize Gram-positive and Gram-negative bacteria, respectively, and regulate the release of downstream cytokines. NOD1 and NOD2 can recognize intracellular PAMPs that can enter the cell through phagocytosis or membrane pores (Anton et al., 2022). BV and associated pathogens, such as Prevotella and Gardnerella, have been shown that associated with the expressions of TLRs and NLRs, especially TLR2/4 (Anahtar et al., 2015; Anton et al., 2022; Dong et al., 2022; Gerson et al., 2022). BV-associated bacteria can induce immune response of cervical cells through the TLR2/4−activated signalling pathways (Zariffard et al., 2005; Anton et al., 2022). In addition, due to G. vaginalis can trigger the NLRP3 infammasome in macrophages and monocytes (Vick et al., 2014; Xiang et al., 2021), it is possible that NOD signalling may be another passway of G. vaginalis-mediated infammation in cervicovaginal epithelial cells (Anton et al., 2022).
Clinical studies of the relationship between cervical cytokines and CVM sequencing are listed in Table 1. Most studies show that the BV-associated microbiome (CST IV) and elevated microbiota diversity are related to an increase cervical IL-1α and IL-1β, and to a lesser extent granulocyte-macrophage colony-stimulating factor (GM-CSF) and IL-10 (Mitchell and Marrazzo, 2014; Shannon et al., 2017), which was similarly with vagina (Shannon et al., 2017). The L. iners dominant microbiome is associated with increased IP-10 and MIG compared with CST I/II (Anahtar et al., 2015; Shannon et al., 2017). Clinical data of IL-6, IL-8, and IL-10 cytokines and chemokines in the BV-associated microbiome are inconsistent in the vagina and cervix (Mitchell and Marrazzo, 2014). Discrepancies in immune molecules may be attributed to the diversity of microbes and hosts (Łaniewski and Herbst-Kralovetz, 2021). Several studies have explored the role of a single bacterium in epithelial immunometabolic characteristics using a 3D cervical epithelial model. Among the common vaginal bacteria, Lactobacillus (especially L. crispatus) usually plays a protective role in immunomodulation, G. vaginalis or Prevotella bivia usually induces a relatively low inflammatory response, whereas A. vaginae and Sneathia amnii elicit more robust cytokine responses (Eade et al., 2012; Gardner et al., 2020; Łaniewski and Herbst-Kralovetz, 2021). L. crispatus did not induce an inflammatory response or alter the epithelial barrier integrity when cocultured with 3D cervical models. P. bivia and G. vaginalis strains only induce IL-6, TNF-α, IL-1α, and MMP-9, whereas A. vaginae and S. amnii induce multiple proinflammatory molecules, including IL-6, IL-8, IP-10, MCP-1, MIP-3α, RANTES, MMP-10, MMP-1, and sFas. Polymicrobial infection with four BV-associated bacteria leads to a mixed profile and extra activation of IL-1β, a critical cytokine usually raised in women with BV (Łaniewski and Herbst-Kralovetz, 2021). Other BVAB such as Eggerthella sp. only causes an increase in IL-1α; Mobiluncus mulieris increases IL-1α, IL-6, IL-8, MCP-1, and TNF-α; while Megasphaera micronuciformis increases IL-1α, IL-1β, IL-1RA, TNF-α, IL-6, and sFasL (McKenzie et al., 2021). Cervical epithelial cells (especially endocervical epithelial cells) exhibit a more robust immune response than vaginal cells after stimulation by BVAB bacteria. The cervix and the transformation zone of the upper and lower reproductive tracts has a higher ability to generate inflammatory responses than that of the vagina, thus guaranteeing the relative sterility of the upper reproductive tract (Eade et al., 2012).
Research on common cervicovaginal bacteria in immune cells has mainly focused on DC cells, macrophages, and T cell recruitment and differentiation. The effect of G. vaginalis on DC cell stimulation and the inflammatory response is not obvious. G. vaginalis and its supernatants induce THP-1 macrophages to differentiate into the M1 phenotype which is involved in defence against bacterial infections, elevated reactive oxygen species (ROS) levels, and stimulation of the NF-κB/STAT1 pathway (Liu et al., 2021a). This causes THP-1 cell pyroptosis by promoting the formation of the NLRP3 inflammasome, resulting in cytokine secretion. Other BVABs such as Megasphaera elsdenii and Prevotella timonensis significantly promote the maturation of DC cells, the differentiation of T cells into the pro-inflammatory Th1 type, and the increase of IL-1β, IL-6, IL-8, IL-12p40, and TNF-α (van Teijlingen et al., 2020). The increase in Th1 pro-inflammatory cytokines Il-1β, IL-6, and IL-8 recruits Th1 and Th17 pro-inflammatory CD4+T cells, effector memory CD8+T cells, and leucocytes (Deruaz and Luster, 2015). In contrast, vaginal Lactobacillus inhibits the pro- or inflammatory response of epithelial or immune cells (Jan et al., 2012) and promotes M2 macrophages polarisation which is involved in tissue repair and wound healing (Lin et al., 2021), promotes the differentiation of CD4+T cells toward immunosuppressed Treg cells (Jang et al., 2017), but has no effect on DC maturation (van Teijlingen et al., 2020).
Immune response induced by cervicovaginal metabolites on cervix
The most representative metabolites in the cervicovaginal tract are lactic acid and SCFAs. Lactic acid and other products such as extracellular polysaccharides (EPS) exhibit anti-inflammatory effects on both cervical epithelial and immune cells, while the effect of SCFAs is not well understood.
EPS secreted by vaginal L. gasseri strains G10 and H15 inhibit the inflammatory factor TNF-α and promote the increase in the anti-inflammatory factor IL-10 (Sungur et al., 2017). Coculture of protonated L/D lactate with cervical epithelial cells results in a decrease in IL-6 and IL-8, and an increase in IL-1RA and IL-1β (Hearps et al., 2017). IL-1RA is expressed more highly than IL-1β, and is an antagonist of IL-1β, which inhibits its pro-inflammatory effect (Hearps et al., 2017). Delgado-Diaz et al. (Delgado-Diaz et al., 2019) simulated the organic acid composition of healthy or BV women (normal: 33 mM L- lactic acid, 4 mM acetate, 1 mM succinate, pH 3.9; BV: 100 mM acetate, 20 mM succinate, 4 mM propionate, 4 mM butyrate, pH 7). Normal organic acid dampening TLR-elicited stimulation of IP-10 MIP-3α, IL-6 of cervicovaginal epithelial cells. Regarding the effect of lactic acid on immune cells, tumour-related studies demonstrate that lactic acid has an immunosuppressive role by inhibiting the differentiation of monocytes and T cells, activity of cytotoxic CD8+T cells (CTLs), maturation of DC cells, and promoting polarisation of macrophages towards the M2 type (Ma et al., 2020).
The effect of SCFAs is studied more fully in the gut than in the female reproductive tract. SCFAs act as an energy source and immune modulator of the intestinal cell. Most studies show that SCFAs (especially butyrate) restore intestinal barrier function in inflammatory conditions by exhibiting anti-inflammatory effects in intestinal mucosa and inducing tight junction protein expression (Parada et al., 2019), although some studies show contradictory results. For instance, SCFA (acetic, butyric, and propionic acids) cocultured with peripheral blood mononuclear cells (PBMCs) and neutrophils significantly enhance TLR2 and TLR7 and induces IL-8 and TNF-α in a time- and dose-dependent manner (Mirmonsef et al., 2012). Propionate and butyrate also increased in vitro transmigration of neutrophils (Vinolo et al., 2009). SCFAs induces both effector and regulatory T cells by suppressing histone deacetylases and regulating the mTOR-S6K pathway (Arpaia et al., 2013; Park et al., 2015). The difference depends on the type, concentration and pH of the SCFAs and cell type. In the female reproductive tract aspect, BV organic acids (especially acetic and butyric acids) enhance the secretion of TNF-α after TLR1/2/3 stimulation of cervicovaginal epithelial cells but inhibit the production of IL-6, RANTES, and IP-10 (Delgado-Diaz et al., 2019). The exact role of SCFA in the reproductive tract immune cells remains to be elucidated.
The effect of cervicovaginal dysbiosis on epithelial barrier disruption
Clinical proteomic and transcriptional studies show that CVM is associated with the disruption of the cervical epithelial barrier (Table 2). Dysbiosis of vaginal flora may disrupt the epithelial barrier by inducing an inflammatory response.
Borgdorff et al. divided vaginal microbiota composition into four groups according to bacterial diversity: group 1 (L. crispatus-dominated), group 2 (L. iners-dominated), group 3 (moderate dysbiosis, mixture of BV), and group 4 (severe dysbiosis, all BV-positive). An increase in the vaginal microbiota group causes proteomic changes including cytoskeleton alterations (increasing actin-organising proteins, decreasing keratins and cornified envelope proteins), increasing lactate dehydrogenase (LDH) A/B as markers of cell death, proinflammatory cytokines, and proteolytic activity, together with decreasing immunoglobulin G1/2, AMP imbalances, and mucus alterations (increasing MUC5B and 5AC) (Borgdorff et al., 2016). Additionally, the cervicovaginal microbiome influences certain menstrual cycle-dependent changes to the cervical epithelial barrier, where hormone-associated reduction of the epithelial barrier protein RPTN is amplified in women without Lactobacilli during the luteal phase (Bradley et al., 2018). Other proteome studies demonstrate that CST III/IV or BV have differentially expressed proteins involved in the cytoskeleton, keratinisation alteration, epidermis development, and immune response compared with healthy women (Cruciani et al., 2013; Zevin et al., 2016; Ferreira et al., 2018). The inflammatory response contributes to the disruption of the cervicovaginal epithelium. Arnold et al. found elevated cytokine expression is positively associated with neutrophil proteases (MMP-9 and MMP-8), reduced antiproteases (SPINK5, SPINK7, SLPI), and altered cytoskeleton, epithelial differentiation, and keratinisation pathways. IL-1β, MIP-3α, and IL-8 display the strongest correlations with MMP-8 and MMP-9, indicating reduced barrier integrity (Mohammadi et al., 2022). IL-1β activates p38 and JNK signalling, leading to decreased tight junctions and disruption of mammary epithelial intergrity (Kobayashi et al., 2021). TNF-α and lipopolysaccharide (LPS) increase apoptosis, necrosis, and senescence of cervical epithelial cells (Tantengco et al., 2021a). The dysbiotic microbiome induces an inflammatory response which contributes to the disruption of cervicovaginal epithelial cells.
In addition, CVM and its metabolites directly influence the epithelial barrier by inducing oxidative stress, altering miRNA expression, and promoting cell cycle arrest and apoptosis. BV-associated bacteria induce oxygen stress intermediates when cocultured with a 3D cervical epithelial model which affects the epithelial barrier integrity (Wang et al., 2021b). P. bivia and S. amnii increase 2-hydroxyglutarate, 2-hydroxybutyrate, and citrulline levels, which are correlated to the activity of inducible nitric oxide synthase (iNOS) (Łaniewski and Herbst-Kralovetz, 2021). Eggerthella sp. and M. mulieris elevate sphingolipids and 2-hydroxybutyrate and decrease cysteinylglycine and cysteinylglycine disulphide (McKenzie et al., 2021). Veillonella atypica and Veillonella montpellierensis significantly accumulate histamine. Fusobacterium gonidiaformans and Fusobacterium nucleatum increase 2-hydroxyglutarate, induce cysteine- and methionine metabolic pathways, pro-inflammatory lipids, and genotoxic hydrogen sulfide (Maarsingh et al., 2022). 2-hydroxyglutarate is a metabolite marker of oxidative stress. The depletion of two intermediates in the glutathione synthesis pathway (cysteinylglycine and cysteinylglycine disulfide) indicates increased glutathione biosynthesis and an increase in ROS levels (McKenzie et al., 2021). The distribution of ROS or oxidative stress pathways in human cervical epithelial cells and cervical stromal cells is linked to sterile inflammation (increased IL-6). This is mediated by p38 mitogen-activated protein kinase activation which promotes cell cycle arrest and cell necrosis death (Tantengco et al., 2021b), and is associated with lipid peroxidation and epithelial tight junction disruptions (Yamamoto et al., 2021). Histamine decreases tight junctions (ZO-1 in nasal epithelia and E-cadherin in pulmonary epithelia) (Salliss et al., 2021). The increase in sphingolipids also reflects disruption of the epithelial barrier.
BV-associated bacterial supernatants also affect the alteration of epithelial barrier function. Supernatants derived from G. vaginalis and L. iners increase ectocervical and endocervical cell permeability, cleave E-cadherin, and elevate miR-143 and miR-145 expression, which downregulate cell adhesion genes, JAM-A and FSCN1, decrease cell proliferation, and increase apoptosis (Anton et al., 2017). M. mulieris supernatant also increases cell permeability (Dude et al., 2020). In contrast, lactic acid increase the expression of barrier proteins claudin1, claudin4 of cervicovaginal epithelial cells (Delgado-Diaz et al., 2022), L. crispatus supernatants also can mitigate the disruption of cervical epithelial barrier induced by LPS or G. vaginalis and reversal of G. vaginalis-induced increase in miRNA expression (Anton et al., 2018). G. vaginalis secretes haemolysin (vaginolysin), a cholesterol-dependent pore-forming toxin that increases the membrane permeability of host cells and causes K+ efflux, activates caspase-1, and contributes to apoptosis (Muñoz-Planillo et al., 2013; Vick et al., 2014). The increase of sFasL (an apoptosis-related protein) in cervicovaginal lavages of women with vaginal dysbiosis demonstrates the damage and apoptosis of cervicovaginal epithelial cells. Taken together, vaginal dysbiosis bacteria can directly induce epithelial barrier disruption through oxidative stress and miRNA alteration, and promote cell cycle arrest, apoptosis, and necrosis. An indirect effect is also realized by the secretion of harmful metabolites and causing immune disorders (Figure 1).
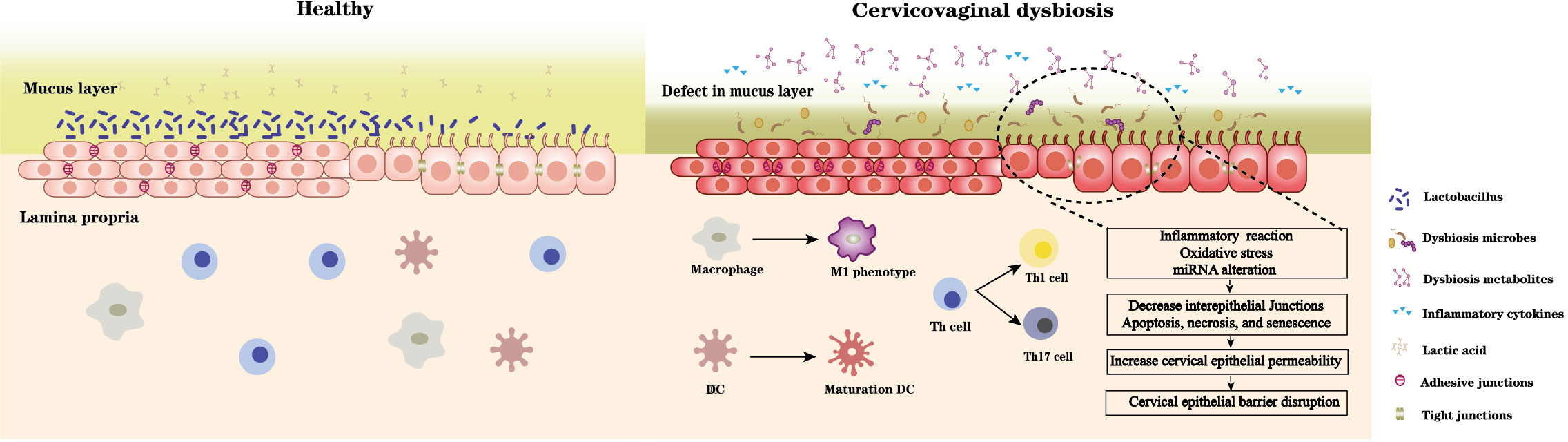
Figure 1 A healthy Lactobacillus dominant microbiota and the acidic environment formed by the associated metabolites especially lactic acid maintain cervical epithelial barrier integrity, stabilise the mucosal immune system. In contrast, microbiota dysbiosis and its accompanying changes in microbial metabolites can 1) damage mucus layer; 2) imbalance immune system: promote immune cells differentiate towards proinflammatory type and induce pro- and inflammatory cytokines secretion; 3) disrupt cervical epithelial barrier: induce inflammation reaction, oxidative stress, miRNA alteration of cervical epithelial cell, decrease intracellular junctions, and promote cell cycle arrest, apoptosis, and necrosis. Thus, increase the epithelial permeability and disrupt barrier function.
The effect of cervicovaginal microbiota on mucus and cervical remodeling
Mucus and mucins play a role in protecting mucosal surfaces, protecting the epithelium from contact, and removing intruders by trapping (Hansson, 2020). Mucins provide an attachment site and a nutrient source for some bacteria. For example, isolated vaginal Lactobacilli have shown the ability to bind mucin (Leccese Terraf et al., 2014; Ahire et al., 2021). Vaginal Lactobacilli contain genes encoding proteins that allow adhesion to mucins, including mucus adhesion promoting protein (MapA), mucus-binding protein (MubI), cell and mucus-binding protein (CmbA), modulator of adhesion and biofilm formation (mabA), and mucus binding factor (MBF) (Leccese Terraf et al., 2014). Lactobacilli isolated from the gut contain several mucus adhesion proteins, including mucus-binding protein (MUB), MucBP domain, pili, SIpa, MapA, and EF-Tu. These adhesion proteins include two main patterns: MUB, the MucBP domain, and pili are cell wall-anchored proteins that covalently bond to the cell wall; MapA, EF-Tu, and ATP-binding cassette (ABC) transporters are multifunctional proteins (so-called moonlighting proteins) that, in addition to performing their primary intracellular functions, also act as adhesion molecules (Nishiyama et al., 2016).
BVAB alters the cervical mucus by stimulating mucin production and degrading highly glycosylated proteins. Clinical studies show that the levels of MUC1, MUC4, MUC5AC, MUC5B, and MUC7 are significantly higher in women with BV than in women with normal or intermediate Nugent scores (Borgdorff et al., 2016; Moncla et al., 2016). Bacterial and viral products and TNF-α increase the mRNA expression of MUC1, MUC4, and MUC16 in a 3D endocervical model (Radtke et al., 2012). An increase in MUC expression enhances the ability of epithelia to form a protective barrier, thus promoting bacterial clearance. Sialidase and glycosulfatase levels and activity increase in BV patients compared with normal women (Gipson et al., 1999; Gipson et al., 2001; Wiggins et al., 2002; Ng et al., 2021). G. vaginalis strains, Prevotella species, and S. amnii liberate or consume sialic acids (Łaniewski and Herbst-Kralovetz, 2021). Three sialidases are identified in G. vaginalis strains: NanH1 (formerly sialidase A), NanH2, and NanH3. Among these, NanH2 and NanH3 have higher ability against sialic acid substrates in many different molecular contexts, such as 2-3- and 2-6-linked sialic acids, and N- and O-linked sialoglycans found on SIgA and mucin (Robinson et al., 2019). The only Prevotella spp. that contains a gene encoding α-N-acetylglucosaminidase (an enzyme that cleaves O-glycans of type 3 mucins) is P. timonensis; consequently, this species has the greatest sialidase activity in this genus (Ilhan et al., 2020).
Vaginal dysbiosis is associated with cervical shortening (Di Paola et al., 2020) and PTB (Gerson et al., 2022). The cervix needs to be extensively remodelled during pregnancy to allow a full term foetus to pass through the birth canal, which is divided into softening, ripening, dilation/labour, and postpartum repair. Both the cervical epithelium and stroma undergo changes during this process. Cervical epithelia proliferate in preparation for parturition and regulate the expression of aquaporin water channels to maintain fluid balance, epithelial permeability, and a protective barrier. This regulates solute transport through the intracellular junctional complex and protects the cervical stroma and upper reproductive tract against invasion of pathogens (Timmons et al., 2007). Stromal changes include increasing hyaluronan content and collagen solubility (Read et al., 2007), and loosening of the collagen matrix (Yellon, 2019).
The potential mechanisms by which bacterial infections affect cervical remodelling mainly involve metalloproteinases. G. vaginalis, A. vaginae, P. bivia, and P. asaccharolytica can induce or secrete MMP-1, 9 and 10, which shows the ability to degrade collagen (type I and IV), gelatin, casein, and fibrinogen (Łaniewski and Herbst-Kralovetz, 2021; McGregor et al., 1986; Łaniewski and Herbst-Kralovetz, 2021; Short et al., 2021; Tantengco et al., 2021a), while L. crispatus has no effect on type I collagen, casein, and fibrinogen, which inhibits clot formation (Lithgow et al., 2022). The colonisation of the mouse reproductive tract by G. vaginalis increases mucin expression, dispersion of collagen fibres, and alters cervical biomechanical properties which indicates a more rapid cervical remodeling (Sierra et al., 2018). The ability to secrete or induce metalloproteases and degrade collagen highlights their potential to alter reproductive tissue structure and harm human pregnancy through premature cervical remodelling, clotting disruption, and foetal membrane weakening.
The effect of cervicovaginal microbiota on STI infection
CT infection
Chlamydia trachomatis (CT) is the most prevalent sexually transmitted bacterial infection worldwide, with approximately 131 million new cases occurring annually (Kreisel et al., 2021). In some cases, it ascends from the cervix to the uterus and fallopian tubes, leading to severe reproductive pathology (Brunham and Rekart, 2009). It is characterised by an asymptomatic infection and a high reinfection rate. The transmission rates of CT are between 25–40%. Only 50% of women will naturally resolve the CT infection within one year (Morré et al., 2002; Molano et al., 2005). CVM and its products may serve as important cofactors for infection susceptibility, clearance, or reinfection.
BV is the most frequent vaginal infection and is found in 20–48% of CT-infected women (Wiesenfeld et al., 2003; Filardo et al., 2019). A growing number of sequencing studies show the association of cervicovaginal microbiota with CT infection (Table 3). A recent meta-analysis reveals a trend towards a positive association between low Lactobacilli microbiota and HPV and CT infection, suggesting a potential protective role of high Lactobacilli microbiota (Tamarelle et al., 2019). Lactobacilli including L. crispatus, L. gasseri, L. jensenii, L. reuteri, and L. aviarius are reduced in both the cervical and vaginal microbiota of CT-positive women (Filardo et al., 2017). Asymptomatic CT-positive patients are more likely to have L. crispatus than women with CT-correlated symptoms. In contrast, BVAB, L. iners, and increased species richness and diversity are associated with CT infections (Filardo et al., 2017). A specific bacterial network characterised by BV-associated bacteria include, G. vaginalis, Prevotella amnii, Prevotella buccalis, P. timonensis, Aerococcus christensenii, and Vatica guangxiensis is a potential biomarker of CT (Filardo et al., 2019). In addition, L. iners-dominated CVM is an independent risk factor for CT infection compared to L. crispatus-dominant CVM (van der Veer et al., 2017; van Houdt et al., 2018).
Some studies have explored the mechanisms of CVM in CT treatment and clearance. Mott et al. (Mott et al., 2021) evaluated the potential impact of BV and metronidazole treatment on CT clearance and found that CT clearers were more likely to have a Lactobacillus-dominant vaginal microbiota after metronidazole treatment compared to non-clearers. The diverse anaerobe dominant group has higher IL-1α and IL-1β levels, while the L. crispatus or L. iners dominant group has higher IL-6 and IP-10 levels, which is consistent with CT clearers. IP-10 can result in a rapid influx of T cells which may aid CT clearance. IP-10 can be abrogated in endocervical epithelial cell secretion after CT infection. Cervicovaginal dysbiosis and BV treatment can drive cytokine changes, which may modulate CT clearance. Although L. iners dominant flora and its relative cytokine IP10 may help CT clearance. L. iners and G. vaginalis have a higher level of resistance to azithromycin and, thus could be selected for post-treatment, whereas sensitive Lactobacillus spp. are diminished (Tamarelle et al., 2020). The instability of the L. iners dominant flora, its ease of conversion to BV-associated flora, and the potential risk factors for CT infection suggest that the risk of STI in women may not be reduced after antibiotic treatment.
IFN-γ induced persistence of CT in epithelial cells is another issue through which CVM can impact the pathogenesis of CT. CT is characterized by two distinct forms, including extracellular infectious basic bodies (EBs) and intracellular dividing reticulums (RBs). CT is a tryptophan auxotroph. IFN-γ produced by infiltrating T and NK cells induces the activation of the enzyme IDO1, which depletes the tryptophan into kynurenine. Lacking tryptophan can arrest CT in intracellular RBs, resulting in morphologically aberrant, viable but non-cultivable, persistent growth form (Beatty et al., 1994; Aiyar et al., 2014; Ziklo et al., 2019). CT contain a tryptophan synthase operon (trpA, trpB and trpR genes) that can produce tryptophan from indole. Lactobacillus spp. do not produce indole, however, many Prevotella spp. can produce indole and promotes CT infection in the presence of IFN-γ (Ziklo et al., 2016). Besides Prevotella spp., Porphyromonas asaccharolytica, Propionibacterium acnes, Fusobacterium nucleatum, Faecalibacterium prausnitzii, Enterococcus faecalis, Peptoniphilus harei, and Escherichia coli are also found the ability of indole-producing (Ziklo et al., 2018). In addition to tryptophan depletion, a recent study found that IFN-γ also can down regulate c-Myc, the key regulator of host cell metabolism, resulting in the reduction of TCA cycle intermediates and nucleotides to prevent chlamydial replication and promoting persistence (Rajeeve et al., 2020; Vollmuth et al., 2022). Constitutive expression of c-Myc can rescue CT from persistence induced by IFN-γ. Dong et al. (Dong et al., 2022) proved that the abundance of Prevotella correlates with the expression of cervical TLR4, NF‐κB, C‐myc, and hTERT genes. In contrast, Lactobacillus abundance showed negative correlations with these genes. Whether CVM can impact IFN-γ involved CT pathogenesis and persistence through C-Myc remain to be verified.
Most experimental studies have concentrated on the protective effect of Lactobacillus spp. towards CT infections. CT replicates only intracellularly; therefore, attachment and entry are essential in CT infection and pathogenesis. (Mastromarino et al., 2014). L. brevis and L. salivarius can co-aggregate with CT and compete with HeLa cells, thereby reducing the proportion of EB and inhibiting EB absorption. The intracellular multiplication of CT are inhibited by L. brevis and L. salivarius (independent of the pH) (Mastromarino et al., 2014). Vaginal Lactobacilli inhibit CT adhesion and infectivity in human epithelial cells and macrophages (Rizzo et al., 2015; Parolin et al., 2018). Incubation of HeLa cells with L. crispatus BC5 cells reduces polar lipids and α5β1 integrin subunit exposure in the epithelial plasma membrane. The interaction of CT with the β1 integrin subunit of host epithelial cells is a mechanism for EB binding, invasion, and signalling (Stallmann and Hegemann, 2016).
Lactobacillus supernatants inhibit CT infectivity; L. crispatus is the most potent while L. iners is the least effective (Edwards et al., 2019; Chen et al., 2021). The supernatants of D-lactate-producing Lactobacillus spp.(L. crispatus and L. jensenii) modulate the expression of multiple genes related to cell proliferation including decreasing miR-193b and histone deacetylase 4 (HDAC4) which are required for CT-induced proliferation during infection. HDACs can repress gene transcription of cyclin-dependent kinase inhibitor 1A (CDKN1A), which in turn inhibits the activity of cyclin-dependent kinase 4 (CDK4) and thus the cell cycle. Downregulation of HDAC4 by L. crispatus and L. jensenii supernatants led to an increase of CDKN1A and lower host cell proliferation. Therefore, these extracts help reduce susceptibility to CT infection (Edwards et al., 2019). D-lactic acid is the most potent metabolite and could compensate for the inhibitory effect of L. iners on CT detection. Lactoferrin is another metabolite that inhibits CT entry into host cells and downregulates IL-6/IL-8 synthesis. Its inhibitory role is proven by the administration to pregnant women asymptomatically infected with CT (Sessa et al., 2017). Lactobacilli and their products also modulate the host inflammatory reaction after CT infection. For instance, L. crispatus and its supernatant reduce production of IL-6, IL-8, and TNF-α and increase IL-10 production in CT-infected HeLa and J774 cells (Rizzo et al., 2015). Lactobacilli mixtures significantly reduce CT-induced cytokine production (TNF-α, IFN-γ, and IL-1β) in the mouse vagina and the severity of hydrosalpinx, oviduct inflammation, and dilatation (Chen et al., 2022). L. crispatus reduces the levels of pro-inflammatory cytokines to reduce inflammatory symptoms, which may explain why asymptomatic CT-positive patients are more likely to have L. crispatus than women with CT-correlated symptoms.
HPV infection and relative disease
Most existing epidemiological studies support a positive association between vaginal infection and HPV acquisition, persistence, and cervical intraepithelial neoplasia (CIN) progression. Many case-control-, cross-sectional-, longitudinal-, and meta-analysis studies demonstrate that BV is the most relevant vaginal infection. A meta-analysis including 13 studies demonstrated that the factors related to HPV infection are BV, vulvovaginal candidiasis (VVC), CT, and ureaplasma urealyticum (UU), with BV also related to CIN. Another study also showed that moderate-to-severe AV are correlated with CIN (Plisko et al., 2021). A network meta-analysis of the microbiome including 11 longitudinal and cross-sectional studies showed that a low Lactobacilli-dominated CST and greater microbiota diversity are strongly associated with HPV compared with L. crispatus. L. iners also showed higher odds of HPV infection than L. crispatus (Norenhag et al., 2020; Usyk et al., 2020; Zhang et al., 2022). Women who acquire HPV16 are more likely to transition between microbial communities than women without a previous history of HPV infection (Moscicki et al., 2020). Taken together, vaginal dysbiosis associated with CST, higher diversity, and unstable transition properties are associated with HPV infection and progression (Supplementary Table 1). Most studies have shown the harmful role of BVAB in HPV infection and related diseases, including Gardnerella, Prevotella, Atopobium, Sneathia, and Mycoplasma, although a few studies show inconsistent results, which is the same as that of L. iners. Another longitudinal cohort study found that depletion of Lactobacillus spp. and the existence of specific anaerobic bacteria (including Megasphaera spp., P. timonensis and G. vaginalis) are associated with persistence and slower regression of CIN2 (Mitra et al., 2020).
As previously discussed, BV-associated bacteria can induce cervical inflammation, influence local immunity, and disrupt the epithelial barrier. This impact may contribute to HPV infection and disease progression. HPV infection also promotes cervicovaginal microbiota imbalance by influencing the expression of mucosal host defence peptides. Lebeau et al. demonstrated that HPV E7 oncoprotein substantially inhibits host defence peptide expression including HβD1, 2, 4, HD-5/6, SLPI, S100A7, and elafin by interacting with NF-κB and Wnt/β-catenin signalling pathways. All these peptides have antimicrobial activity against G. vaginalis except HβD1. Meanwhile, S100A7 and elafins expressed by the cervical/vaginal squamous mucosa can be cleaved, internalised, and used as an amino acid source by Lactobacilli, which leads to its survival. Consequently, the amino acid source supporting the survival of Lactobacillus species is considerably decreased, promoting an unbalanced cervicovaginal flora (Lebeau et al., 2022). This is consistent with work showing that HPV infections result in an increase in vaginal bacterial richness and diversity and a decrease in the percentage of Lactobacilli; this causes a shift from CST III to CST IV, despite the status of CINs (Chen et al., 2020).
CVM can affect HPV infection by modulating the inflammatory environment. The antiviral-specific immune response to HPV infection needs the cooperation of CD4+T helper cells and cytotoxic CD8+T cells (CTLs). High levels of IFN-γ secreted by Th1 cells potentiate the cytotoxic activity of CTLs and target pathogen-infected cells. IFN-γ also promotes the expression of intracellular antiviral genes that block viral replication (Hickey et al., 2011). In contrast, Th17 and IL-17 are associated with an enhanced cervical immune response during HPV infection, result in the progression of cervical lesions (Sahu and Khare, 2021). The number of Th17 cells in the blood and the level of IL-17 in tissue homogenates of the cervix increases at different stages of cervical lesions (Xue et al., 2018). Nicolò et al. stimulated SiHa and CaSki cells with heat-inactivated bacteria to explore the relevant changes in cytokines: Lactobacilli (especially L. gasseri and L. jensenii) significantly induce IFN-γ levels, while L. ines and G. vaginalis increase IL-17 in addition to IFN-γ (Nicolò et al., 2021). An increase in IL-17 and IL-17-inducing cytokines (IL-23 and IL-1β) are observed in the cervicovaginal lavage obtained from women with CST-IV-dominated microbiota (Gosmann et al., 2017). Besides G. vaginalis, Streptococcus anginosus, Staphylococcus, and Mycoplasmataceae correlated with an increase in IL-17, cervical lesions, and cancer (Fan et al., 2021b; Manzanares-Leal et al., 2022). In contrast, dominant flora composed of L. gasseri and L. jensenii are associated with HPV clearance and the prevention of low-grade cervical dysplasia progression (Brotman et al., 2014; Mitra et al., 2015). In addition to IL-17, continuous low IL-1β/IP-10 (BV cytokine signature), predicts HR-HPV clearance, and an elevated TNF-α/MIP-1β signature is associated with CIN2+ progression (Usyk et al., 2022).
Regarding the role of cervicovaginal organic acids in HPV and related diseases, many experts have explored whether Lactobacilli and their suppernant have a protective effect against disease. L. crispatus culture supernatants promote healing of damaged vaginal epithelium (Takada et al., 2018). G. vaginalis supernatants inhibit wound healing of cervical cell monolayers compared to L. iners (Zevin et al., 2016). L. crispatus, L. rhamnosus, L. gasseri, and L. jensenii supernatants reduce the expression of autophagy genes ATG14, BECN1, and cyclin A, cyclin-dependent kinase 2 (CDK2), and HPV E6 and E7 to inhibit cervical cancer cells activity (Motevaseli et al., 2016; Ghanavati et al., 2020). Although most studies demonstrate the protective role of Lactobacilli products, lactic acid has conflicting results. Lactate and butyrate inhibit HDACs, thereby promoting histone acetylation by histone acetylases. Acetylated histones induce chromatin relaxation, resulting in an increased DNA repair rate (Wagner et al., 2015; Wagner et al., 2017), and recruitment of DNA-dependent protein kinase catalytic subunits (DNA-PKcs) to the nucleus. Cervical epithelial cells bear the lactate hydroxycarboxylic acid receptor 1 (HCA1/GPR81). Lactate stimulates surface HCA1 and induces cAMP signalling and the cAMP/EPAC/PKA-dependent shuttling of DNA-PKcs. DNA-PKcs sense foreign or damaged self-DNA and are essential components of the non-homologous end-joining pathway which protects cells from lentiviral (HPV and HIV-1) transduction, thus restricting viral oncogenic and/or cytopathic potential (Wagner et al., 2021). In addition, core fucosylation levels are significantly reduced in the serum, exfoliated cervical cells, and tumour tissues from patients with cervical cancer. L. iners metabolites and lactate activate the Wnt pathway by means of the lactic acid-HCA1 complex, increase the level of core fucosylation, thereby inhibiting the proliferation and migration of cervical cancer cells (Fan et al., 2021a). In contrast, lactic acid (a major metabolite in glycolytic tumour cells) can be imported to fuel mitochondrial respiration (Warburg, 1956). Lactate induces the expression of TGF-β2 in gastric and lung cancers which upregulates the expression and activation of MMP-2, thereby promoting tumour cell metastasis. It also promotes angiogenesis by upregulating HIF-1α and VEGF (Kolev et al., 2008). In addition, the immunomodulatory effect of lactate on T cells, macrophages, and CTL may lead to tumour immune escape (Colegio et al., 2014; Brand et al., 2016). Detection of high lactate levels among human cervical cancers predicted tumour metastasis, recurrence and restricted of patient survival (Walenta et al., 2000). Li et al. demonstrated that lactate induces migration and invasion of SiHa cells via the miR-774/ARHGAP5 axis, although the effect is diminished due to partial inhibition of E6 and E7 expression (Li et al., 2019). However, the exact roles of lactic acid in cervical cancer with respect to HPV protein expression, proliferation, and migration of cancer cells remain unclear.
HIV infection
Most studies show that BV and low Lactobacilli CVM are associated with an increased risk of HIV acquisition (Atashili et al., 2008), transmission (Cohen et al., 2012), and a risk factor for intrapartum HIV trasmission(Atashili et al., 2008), and adverse pregnancy outcome(Short et al., 2020; Mwenda et al., 2021). A nested case-control study including five cohorts shows concentration-dependent associations between Eggerthella species type 1, Gemella asaccharolytica, Leptotrichia/Sneathia, Megasphaera spp., and Mycoplasma hominis with increased odds of HIV acquisition (Eastment and McClelland, 2018). Other studies also demonstrate that Prevotella melaninogenica, V. montpellierensis, Mycoplasma, P. bivia, Sneathia sanguinegens (Gosmann et al., 2017), and L.iners (Taku et al., 2022) are associated with HIV acquisition. HIV with unsuppressed viral loads are more likely to have higher abundance of Megasphaera genomosp.1, A. vaginae, and Clostridiales sp. compared to healthy and recurrent BV patients (Elwood et al., 2020). Relative studies are summarized in Supplementary Table 2.
The impact of the cervix immune response induced by the BV-associated microbiome on HIV acquisition is complicated and conflicting. On the one hand, the increase in BV-associated microbiota and inflammatory cytokines indicates a disruption of the epithelial barrier associated with HIV genital tract shedding and the recruitment of HIV target cells. Abnormally high levels of several cytokines such as IL-1β, IL-6, MIP-1α, and TNF-α are associated with HIV disease progression (Herold et al., 2013) and contribute to HIV persistence during antiretroviral treatment (ART) by promoting residual levels of viral production (Vandergeeten et al., 2012). BV-associated flora are associated with endo- (Gosmann et al., 2017) and ectocervix (Thurman et al., 2015) CD4+CCR5+T cells compared to healthy flora. Elevated inflammatory cytokine levels are associated with an increased frequency of cervical CD4+T cell (Arnold et al., 2016; Gosmann et al., 2017). Activated CD4+T cells and GD T cells are affected by vaginal flora and are associated with HIV infection. GD1 T cells are protective in the genital tract and GD2 T cells are targets for HIV entry. Cervical GD1 T cells and GD2 T cells are lower and higher in women with BV than in those with normal vaginal flora, respectively which predisposes women with HIV acquisition (Alcaide et al., 2016). On the other hand, mucosal IgG and IgM elevated with genital inflammation, IgM neutralises and reduces HIV infections and directly binds to CD4+T cells and chemokine receptor CCR5, hindering HIV entry (Sobia et al., 2021). Some bacteria, such as L. gasseri, L. crispatus, V. montpellierensis, P. amnii, and S. amnii are positively correlated with the frequency of mucosal-activated natural killer (NK) cells that coordinate the early control of HIV infection (Munusamy et al., 2021). The exact effect of CVM on HIV infection via local immune responses remains further explored.
CVM also plays a role in the pharmacokinetics of pre-exposure prophylaxis (PrEP) drugs. However, previous studies reported inconsistent results on this. Some clinical trials using topical 1% tenofovir (TFV) vaginal gel in the CAPRISA 004 cohort and TFV vaginal film in the FAME 04 cohort found that women with low Lactobacilli flora have reduced mucosal concentrations of TFV, which potentially reduces the efficacy of PrEP drugs (Klatt et al., 2017). BV cervicovaginal larvae and G. vaginalis reduce the uptake of PrEP drugs by Jurkat cells, and increase TFV degradation and HIV infection compared to normal cervicovaginal larvae and L. crispatus (Cheu et al., 2020). However, another study examined the effect of CVM on TFV vaginal ring which can continuously deliver for approximately 14 days, and showed a positively correlates between CST IVA/B and high level of TFV during the first two months of use (Thurman et al., 2022). Nevertheless, individuals with a high abundance of Prevotella spp. or G. vaginalis show reduced TFV/TFV-diphosphate concentrations in the vaginal fluid and tissues in the third month. In addition, pH drives TFV release since it affects TFV transport into human cells (Johnson et al., 2012; Taneva et al., 2018). The authors speculate that a higher pH in vivo increases aqueous solubility, and more TFV will dissolve from the formulated paste inside the vaginal ring, which may explain the positive correlation between TFV and CST IVA/B in the first two months.
Protonated L-lactic acid is a major anti-HIV metabolite of Lactobacilli (Kyongo et al., 2015; Ñahui et al., 2017; Tyssen et al., 2018). 0.3% w/w L-lactic acid shows a 17 times higher anti-HIV activity than that of D-lactic acid. Moreover, a physiological concentration of L-lactic acid can inactivate HIV in the presence of 50% cervix vaginal secretions and 0.75% semen. The anti-HIV activity of L-lactic acid is pH dependent, and can be abrogated under neutral pH (Aldunate et al., 2013). Healthy cervicovaginal mucus inhibits HIV activity and transmission. HIV spreads in the form of free viral particles and cellular contact. Although L-lactic acid has a stronger ability to inhibit HIV activity than D-lactic acid, the HIV-1 virion can be greatly trapped in CVM with high D-lactic acid concentration and an L. crispatus-dominant microbiota (Nunn et al., 2015). HIV virions have increased mobility in L. iners dominant mucus and BV mucus which is related to the levels of D-lactic acid and total lactic acid. The trapping of HIV-1 viruses in the cervicovaginal mucus is mainly based on adhesive interactions. The addition of lactic acid to the cervicovaginal mucus with reduced adhesive properties did not restore HIV adhesion (Nunn et al., 2015). Therefore, the reduction in mucoadhesive interactions might be due to an increased amount of mucin-degrading enzymes that Thus, the reduction in adhesive interactions may be due to elevated levels of mucin-degrading enzymes which disturb HIV-mucin interactions.
The release of extracellular vesicles (EVs) is another approach which protects HIV infection mediated by Lactobacilli (Ñahui et al., 2019). EVs constitute a lipid bilayer membrane carrying numerous bioactive molecules and are considered to be important mediators of cell–cell communication (Costantini et al., 2022; Nagakubo et al., 2019). Lactobacillus-derived EVs (L. crispatus BC3 and L. gasseri BC12) bind to the HIV-1 Env protein, leading to a reduction of HIV-1 entry and binding to target cells. Nevertheless, EVs released by other gram-positive species (Staphylococcus aureus, G. vaginalis, Enterococcus faecium, and Enterococcus faecalisi) also protect human cervicovaginal tissues ex vivo and isolated cells from HIV-1 infection by blocking HIV-1-cell receptor interactions which are related to the steric hindrance of gp120 or gp120 modification (Costantini et al., 2022).
Confounders affect microbiome sequencing
Several confounders may account for the discrepancies across studies among the clinical sample sequencing researches. First, the difference in the baseline characters of subjects, such as ethnicity and geographical region, age, menstrual state, previous hormone and antibiotic treatment history can influence the composition of the reproductive tract microbiome. One example is that some studies about microbiota sequencing on CT infection show inconsistence on Aerococcus christensenii and diversity (Balle et al., 2018; Di Pietro et al., 2018; Cheong et al., 2019). Confounders such as different region, ethnicity and age may account for these bias. Second, sampling site and methodology may also have influence on microbiome sequencing as well as cytokines detection. As discussed before, there are difference in the microbiome composition of vagina wall, posterior fornix and endocervix, as well as sampling device (Kim et al., 2009; Virtanen et al., 2017; Kero et al., 2023). Cytokine and protein concentration were also different collected by endocervical swabs, lavage samples, and vaginal swabs (Dezzutti et al., 2011). Third, sequencing method, laboratory and data processing differ across studies, such as DNA extraction, sequencing target, platform, and annotation database may also impact the sequencing, metabolome and proteome result (Theodoridis et al., 2012; Schnaars et al., 2018; Mattei et al., 2019; Li and Sui, 2021; Sirichoat et al., 2021). For instance, the richness and diversity of cervical microbiota increased in HR-HPV positive women compared with healthy control by 16S rRNA sequencing, however, the difference were not obvious in metagenomic sequencing (Fang et al., 2022). Despite the discrepancies in the result of some specific bacteria in different sequencing studies due to these confounders, presence or increased relative abundances of Lactobacillus spp. are generally associated with decreased risk of female reproductive STI; BV (CST IV vaginal microbiota), and particular BVAB have been found to be associated with increased risk.
Conclusion and outlook
Recent accumulating studies have shown complex interactions between CVM, metabolites, and host cells in the cervical ecosystem. Here, we focused on the crosstalk between CVM and cervical epithelial-, immune-, and mucus barriers.
Generally, Lactobacillus-dominant microbiota exhibit immunomodulatory effects on cervical epithelial and immune cells, maintain cervical epithelial integrity, and protect cervical CT, HPV, and HIV infection with respect to infection, treatment, and clearance. CVM dysbiosis induces an inflammatory response in cervical epithelial and immune cells in microbe-specific modes and directly induces epithelial barrier disruption by inducing oxidative stress, miRNA alteration, and promoting cell cycle arrest, apoptosis, and necrosis. Furthermore, an indirect response may occur through the secretion of harmful metabolites and causes immune disorders. Dysregulation of cervical cytokine and immune cell profiles induced by CVM dysbiosis is also associated with STI infection and relative disease development. Lactobacillus binds mucin, while BVAB stimulates mucin expression and degrades these highly glycosylated proteins which decrease the ability to trap and remove pathogens. BVABs also degrade collagen, gelatin, and casein, and alter cervical biomechanical properties which cause rapid cervical remodelling and harm human pregnancy.
Lactic acid is the most well-studied and potent normal CVM metabolite in maintaining cervicovaginal barrier homeostasis, and inhibiting CT infection and HIV activity; however, its exact role in HPV infection and cervical cancer remains unclear. The specific mechanisms involved in the Lactobacillus, culture supernatant and lactic acid in anti-STI infection mainly include 1) reduces polar lipids and α5β1 integrin subunit exposure in the epithelial plasma membrane, inhibit EB binding and invasion in epithelial cell; 2) inhibit HDACs so that inhibit host cell proliferation, and induce chromatin relaxation, increase DNA repair rate; 3) recruit DNA-PKcs to the nucleus, which can sense foreign or damaged self-DNA, protects cells from lentiviral (HPV and HIV-1) transduction; 4) reduce the expression of autophagy genes and HPV E6 and E7 to inhibit cervical cancer cells activity. Contradictory mechanisms mainly include 1) can be used as fuel mitochondrial respiration in glycolytic tumour cells; 2) promote MMP-2, HIF-1α, VEGF, miR-774/ARHGAP5 axis which promote metastasis, angiogenesis, migration and invasion; 3) immunomodulatory effect on T cells, macrophages, and CTL may lead to tumour immune escape. The association between BVAB, culture supernatant and SCFAs with STI infection usually related with the cytokines change and immune cells recruitment induced by the inflammatory response. The specific mechanisms in the influence on cervical STI disease are less documented.
Going forward, although some studies have observed the interactions between CVM, their metabolites, with host microenvironment using omics technology, the mechanistic understanding towards how these microbiota and their metabolites influence STI infections are lack. For instance, the bioactive metabolites which can interact with bacteria, STI pathogens and host epithelial and immune cell and are less identified, as well as the possible passways. A better understanding can provide information in the developing and optimizing of diagnostics, treatment strategies and drugs, probiotics, postbiotics and vaginal flora transplantation. The innumerable hypotheses generated from these omics studies should be verified by in vitro and in vivo studies. A major gap towards these issue is a lack of suitable in vitro and animal experimental models (Bjornson-Hooper et al., 2022; Mahajan et al., 2022). The microbiome, cervicovaginal epithelium and immune reaction differ between human, mice and non-human primates. Exploring suitable models simulate female reproductive tract environment is essential to better understand the intimate interaction between CVM, their metabolites and cervical host cell. Advanced tools including metagenomics, metatranscriptomics, and metabolomics will provide insights into the characteristics of functional microbiomes and metabolites, determine the potential mechanisms used to connect with host cells, and further form interaction networks in health and disease. Future studies involving molecular detection technology in human samples, cells, and animal biological studies will aid in the discovery of novel diagnostic and therapeutic targets for female reproductive diseases.
Author contributions
MD, CH, and FX conceived the study question, and all authors were involved in the study design. MD,YD created the first draft of the manuscript. JB, HuaL, XM, BL, CW, HuiL, and AF made substantial contributions to drafting the article and revising it critically. All authors contributed to the article and approved the submitted version.
Funding
This work was supported by the National Natural Science Fund of China (Grant No. 82071674), the Tianjin Municipal Science and Technology Commission Special Foundation for Science and Technology Major Projects in the Control and Prevention of Major Diseases (Grant No. 18ZXDBSY00200).
Conflict of interest
The authors declare that the research was conducted in the absence of any commercial or financial relationships that could be construed as a potential conflict of interest.
Publisher’s note
All claims expressed in this article are solely those of the authors and do not necessarily represent those of their affiliated organizations, or those of the publisher, the editors and the reviewers. Any product that may be evaluated in this article, or claim that may be made by its manufacturer, is not guaranteed or endorsed by the publisher.
Supplementary material
The Supplementary Material for this article can be found online at: https://www.frontiersin.org/articles/10.3389/fcimb.2023.1124591/full#supplementary-material
References
Aflatoonian, R., Fazeli, A. (2008). Toll-like receptors in female reproductive tract and their menstrual cycle dependent expression. J. Reprod. Immunol. 77 (1), 7–13. doi: 10.1016/j.jri.2007.03.014
Ahire, J. J., Sahoo, S., Kashikar, M. S., Heerekar, A., Lakshmi, S. G., Madempudi, R. S. (2021). In vitro assessment of lactobacillus crispatus UBLCp01, lactobacillus gasseri UBLG36, and lactobacillus johnsonii UBLJ01 as a potential vaginal probiotic candidate. Probiotics Antimicrob. Proteins. doi: 10.1007/s12602-021-09838-9
Aiyar, A., Quayle, A. J., Buckner, L. R., Sherchand, S. P., Chang, T. L., Zea, A. H., et al. (2014). Influence of the tryptophan-indole-IFNγ axis on human genital chlamydia trachomatis infection: role of vaginal co-infections. Front. Cell Infect. Microbiol. 4. doi: 10.3389/fcimb.2014.00072
Alcaide, M. L., Strbo, N., Romero, L., Jones, D. L., Rodriguez, V. J., Arheart, K., et al. (2016). Bacterial vaginosis is associated with loss of gamma delta T cells in the female reproductive tract in women in the Miami women interagency HIV study (WIHS): A cross sectional study. PloS One 11 (4), e0153045. doi: 10.1371/journal.pone.0153045
Aldunate, M., Tyssen, D., Johnson, A., Zakir, T., Sonza, S., Moench, T., et al. (2013). Vaginal concentrations of lactic acid potently inactivate HIV. J. Antimicrob. Chemother. 68 (9), 2015–2025. doi: 10.1093/jac/dkt156
Amabebe, E., Anumba, D. O. C. (2018). The vaginal microenvironment: The physiologic role of lactobacilli. Front. Med. 5. doi: 10.3389/fmed.2018.00181
Anahtar, M. N., Byrne, E. H., Doherty, K. E., Bowman, B. A., Yamamoto, H. S., Soumillon, M., et al. (2015). Cervicovaginal bacteria are a major modulator of host inflammatory responses in the female genital tract. Immunity 42 (5), 965–976. doi: 10.1016/j.immuni.2015.04.019
Andersch-Björkman, Y., Thomsson, K. A., Holmén, L. J. M., Ekerhovd, E., Hansson, G. C. (2007). Large Scale identification of proteins, mucins, and their O-glycosylation in the endocervical mucus during the menstrual cycle. Mol. Cell Proteomics 6 (4), 708–716. doi: 10.1074/mcp.M600439-MCP200
Anton, L., DeVine, A., Sierra, L.-J., Brown, A. G., Elovitz, M. A. (2017). miR-143 and miR-145 disrupt the cervical epithelial barrier through dysregulation of cell adhesion, apoptosis and proliferation. Sci. Rep. 7 (1), 3020. doi: 10.1038/s41598-017-03217-7
Anton, L., Ferguson, B., Friedman, E. S., Gerson Kristin, D., Brown Amy, G., Elovitz, M. A. (2022). Gardnerella vaginalis alters cervicovaginal epithelial cell function through microbe-specific immune responses. Microbiome 10 (1), 119. doi: 10.1186/s40168-022-01317-9
Anton, L., Sierra, L.-J., DeVine, A., Barila, G., Heiser, L., Brown Amy, G., et al. (2018). Lactobacillus crispatusCommon cervicovaginal microbial supernatants alter cervical epithelial function: Mechanisms by which contributes to cervical health. Front. Microbiol. 9. doi: 10.3389/fmicb.2018.02181
Arnold, K. B., Burgener, A., Birse, K., Romas, L., Dunphy, L. J., Shahabi, K., et al. (2016). Increased levels of inflammatory cytokines in the female reproductive tract are associated with altered expression of proteases, mucosal barrier proteins, and an influx of HIV-susceptible target cells. Mucosal Immunol. 9 (1), 194–205. doi: 10.1038/mi.2015.51
Arpaia, N., Campbell, C., Fan, X., Dikiy, S., van der Veeken, J., Deroos, P., et al. (2013). Metabolites produced by commensal bacteria promote peripheral regulatory T-cell generation. Nature 504 (7480), 451–455. doi: 10.1038/nature12726
Atashili, J., Poole, C., Ndumbe, P. M., Adimora Adaora, A., Smith, J. S. (2008). Bacterial vaginosis and HIV acquisition: A meta-analysis of published studies. AIDS 22 (12), 1493–1501. doi: 10.1097/QAD.0b013e3283021a37
Balle, C., Konstantinus, I. N., Jaumdally, S. Z., Havyarimana, E., Lennard, K., Esra, R., et al. (2020). Hormonal contraception alters vaginal microbiota and cytokines in south African adolescents in a randomized trial. Nat. Commun. 11 (1), 5578. doi: 10.1038/s41467-020-19382-9
Balle, C., Lennard, K., Dabee, S., Barnabas, S. L., Jaumdally, S. Z., Gasper, M. A., et al. (2018). Endocervical and vaginal microbiota in south African adolescents with asymptomatic chlamydia trachomatis infection. Sci. Rep. 8 (1), 11109. doi: 10.1038/s41598-018-29320-x
Beatty, W. L., Belanger, T. A., Desai, A. A., Morrison, R. P., Byrne, G. I. (1994). Tryptophan depletion as a mechanism of gamma interferon-mediated chlamydial persistence. Infect. Immun. 62 (9), 3705–3711. doi: 10.1128/iai.62.9.3705-3711.1994
Benjelloun, F., Quillay, H., Cannou, C., Marlin, R., Madec, Y., Fernandez, H., et al. (2020). Activation of toll-like receptors differentially modulates inflammation in the human reproductive tract: Preliminary findings. Front. Immunol. 11. doi: 10.3389/fimmu.2020.01655
Bjornson-Hooper, Z. B., Fragiadakis, G. K., Spitzer, M. H., Chen, H., Madhireddy, D., Hu, K., et al. (2022). A comprehensive atlas of immunological differences between humans, mice, and non-human primates. Front. Immunol. 13. doi: 10.3389/fimmu.2022.867015
Blaskewicz, C. D., Pudney, J., Anderson, D. J. (2011). Structure and function of intercellular junctions in human cervical and vaginal mucosal epithelia. Biol. Reprod. 85 (1), 97–104. doi: 10.1095/biolreprod.110.090423
Boily-Larouche, Geneviève., Lajoie, J., Dufault, B., Omollo, K., Cheruiyot, J., Njoki, J., et al. (2019). Characterization of the genital mucosa immune profile to distinguish phases of the menstrual cycle: Implications for HIV susceptibility. J. Infect. Dis. 219 (6), 856–866. doi: 10.1093/infdis/jiy585
Borgdorff, H., Gautam, R., Armstrong, S. D., Xia, D., Ndayisaba, G. F., van Teijlingen, N. H., et al. (2016). Cervicovaginal microbiome dysbiosis is associated with proteome changes related to alterations of the cervicovaginal mucosal barrier. Mucosal Immunol. 9 (3), 621–633. doi: 10.1038/mi.2015.86
Borgogna, J. C., Shardell, M. D., Santori, E. K., Nelson, T. M., Rath, J. M., Glover, E. D., et al. (2020). The vaginal metabolome and microbiota of cervical HPV-positive and HPV-negative women: A cross-sectional analysis. BJOG 127 (2), 182–192. doi: 10.1111/1471-0528.15981
Boukari, Hacène., Brichacek, B., Stratton, P., Mahoney, S. F., Lifson, J. D., Margolis, L., et al. (2009). Movements of HIV-virions in human cervical mucus. Biomacromolecules 10 (9), 2482–2488. doi: 10.1021/bm900344q
Bradley, F., Birse, K., Hasselrot, K., Noël-Romas, L., Introini, A., Wefer, H., et al. (2018). The vaginal microbiome amplifies sex hormone-associated cyclic changes in cervicovaginal inflammation and epithelial barrier disruption. Am. J. Reprod. Immunol. 80 (1), e12863. doi: 10.1111/aji.12863
Bradshaw, C. S., Tabrizi, S. N., Fairley, C. K., Morton, A. N., Rudland, E., Garland, S. M. (2006). The association of atopobium vaginae and gardnerella vaginalis with bacterial vaginosis and recurrence after oral metronidazole therapy. J. Infect. Dis. 194 (6), 828–836. doi: 10.1086/506621
Brand, A., Singer, K., Koehl, G. E., Kolitzus, M., Schoenhammer, G., Thiel, A., et al. (2016). LDHA-associated lactic acid production blunts tumor immunosurveillance by T and NK cells. Cell Metab. 24 (5), 657–671. doi: 10.1016/j.cmet.2016.08.011
Brotman, R. M., Shardell, M. D., Gajer, P., Tracy, J.K., Zenilman, J. M., Ravel, J., et al. (2014). Interplay between the temporal dynamics of the vaginal microbiota and human papillomavirus detection. J. Infect. Dis. 210 (11), 1723–1733. doi: 10.1093/infdis/jiu330
Brunham, R. C., Rekart, M. L. (2009). Considerations on chlamydia trachomatis disease expression. FEMS Immunol. Med. Microbiol. 55 (2), 162–166. doi: 10.1111/j.1574-695X.2008.00509.x
Chen, H., Min, S., Wang, Li., Zhao, L., Luo, F., Lei, W., et al. (2022). Lactobacillus modulates chlamydia infectivity and genital tract pathology in vitro and in vivo. Front. Microbiol. 13. doi: 10.3389/fmicb.2022.877223
Chen, Y., Qiu, X., Wang, W., Li, D., Wu, A., Hong, Z., et al. (2020). Human papillomavirus infection and cervical intraepithelial neoplasia progression are associated with increased vaginal microbiome diversity in a Chinese cohort. BMC Infect. Dis. 20 (1), 629. doi: 10.1186/s12879-020-05324-9
Chen, C., Song, X., Wei, W., Zhong, H., Dai, J., Lan, Z., et al. (2017). The microbiota continuum along the female reproductive tract and its relation to uterine-related diseases. Nat. Commun. 8 (1), 875. doi: 10.1038/s41467-017-00901-0
Chen, H., Wang, Li., Zhao, L., Luo, L., Min, S., Wen, Y., et al. (2021). Chlamydia trachomatisAlterations of vaginal microbiota in women with infertility and infection. Front. Cell Infect. Microbiol. 11. doi: 10.3389/fcimb.2021.698840
Cheong, H. C., Yap, P. S. X., Chong, C. W., Cheok, Y. Y., Lee, C. Y. Q., Tan, G. M. Y., et al. (2019). Diversity of endocervical microbiota associated with genital chlamydia trachomatis infection and infertility among women visiting obstetrics and gynecology clinics in Malaysia. PloS One 14 (11), e0224658. doi: 10.1371/journal.pone.0224658
Cheu, R. K., Gustin, A. T., Lee, C., Schifanella, L., Miller, C. J., Ha, A., et al. (2020). Impact of vaginal microbiome communities on HIV antiretroviral-based pre-exposure prophylaxis (PrEP) drug metabolism. PloS Pathog. 16 (12), e1009024. doi: 10.1371/journal.ppat.1009024
Chiu, S.-F., Huang, P.-J., Cheng, W.-H., Huang, C.-Y., Chu, L. J., Lee, C.-C., et al. (2021). Chlamydia trachomatis vaginal microbiota of the sexually transmitted infections caused by and in women with vaginitis in Taiwan. Microorganisms 9 (9), 1864. doi: 10.3390/microorganisms9091864
Cohen, C. R., Lingappa, J. R., Baeten, J. M., Ngayo, M. O., Spiegel, C. A., Hong, T., et al. (2012). Bacterial vaginosis associated with increased risk of female-to-male HIV-1 transmission: A prospective cohort analysis among African couples. PloS Med. 9 (6), e1001251. doi: 10.1371/journal.pmed.1001251
Colegio, O. R., Chu, N.-Q., Szabo, A. L., Chu, T., Rhebergen, A. M., Jairam, V., et al. (2014). Functional polarization of tumour-associated macrophages by tumour-derived lactic acid. Nature 513 (7519), 559–563. doi: 10.1038/nature13490
Costantini, P. E., Vanpouille, C., Firrincieli, A., Cappelletti, M., Margolis, L., Ñahui Palomino, R. A. (2022). Extracellular vesicles generated by gram-positive bacteria protect human tissues ex vivo from HIV-1 infection. Front. Cell. infection Microbiol. 11. doi: 10.3389/fcimb.2021.822882
Cruciani, F., Wasinger, V., Turroni, S., Calanni, F., Donders, G., Brigidi, P., et al. (2013). Proteome profiles of vaginal fluids from women affected by bacterial vaginosis and healthy controls: outcomes of rifaximin treatment. J. Antimicrob. Chemother. 68 (11), 2648–2659. doi: 10.1093/jac/dkt244
Delgado-Diaz, D. J., Jesaveluk, B., Hayward, J. A., Tyssen, D., Alisoltani, A., Potgieter, M., et al. (2022). Lactic acid from vaginal microbiota enhances cervicovaginal epithelial barrier integrity by promoting tight junction protein expression. Microbiome 10 (1), 141. doi: 10.1186/s40168-022-01337-5
Delgado-Diaz, D. J., Tyssen, D., Hayward, J. A., Gugasyan, R., Hearps, A. C., Tachedjian, G. (2019). Distinct immune responses elicited from cervicovaginal epithelial cells by lactic acid and short chain fatty acids associated with optimal and non-optimal vaginal microbiota. Front. Cell Infect. Microbiol. 9 (undefined). doi: 10.3389/fcimb.2019.00446
Deruaz, M., Luster, A. D. (2015). Chemokine-mediated immune responses in the female genital tract mucosa. Immunol. Cell Biol. 93 (4), 347–354. doi: 10.1038/icb.2015.20
De Tomasi, J. B., Opata, M. M., Chishimba, N. M. (2019). Immunity in the cervix: Interphase between immune and cervical epithelial cells. J. Immunol. Res. 2019 (undefined), 7693183. doi: 10.1155/2019/7693183
Dezzutti, C. S., Hendrix, C. W., Marrazzo, J. M., Pan, Z., Wang, L., Louissaint, N., et al. (2011). Performance of swabs, lavage, and diluents to quantify biomarkers of female genital tract soluble mucosal mediators. PloS One 6 (8), e23136. doi: 10.1371/journal.pone.0023136
Di Paola, M., Seravalli, V., Paccosi, S., Linari, C., Parenti, A., De Filippo, C., et al. (2020). Identification of vaginal microbial communities associated with extreme cervical shortening in pregnant women. J. Clin. Med. 9 (11), 3621. doi: 10.3390/jcm9113621
Di Pietro, M., Filardo, S., Porpora, M. G., Recine, N., Latino, M. A., Sessa, R. (2018). HPV/Chlamydia trachomatis co-infection: Metagenomic analysis of cervical microbiota in asymptomatic women. New Microbiol. 41 (1), 34–41.
Dong, B., Huang, Y., Cai, H., Chen, Y., Li, Y., Zou, H., et al. (2022). Prevotella as the hub of the cervicovaginal microbiota affects the occurrence of persistent human papillomavirus infection and cervical lesions in women of reproductive age via host NF-κB/C-myc. J. Med. Virol. 94 (11), 5519–5534. doi: 10.1002/jmv.28001
Dude, C. M., Saylany, A., Brown, A., Elovitz, M., Anton, L. (2020). Microbial supernatants from mobiluncus mulieris, a bacteria strongly associated with spontaneous preterm birth, disrupts the cervical epithelial barrier through inflammatory and miRNA mediated mechanisms. Anaerobe 61, 102127. doi: 10.1016/j.anaerobe.2019.102127
Eade, C. R., Diaz, C., Wood, M. P., Anastos, K., Patterson, B. K., Gupta, P., et al. (2012). Identification and characterization of bacterial vaginosis-associated pathogens using a comprehensive cervical-vaginal epithelial coculture assay. PloS One 7 (11), e50106. doi: 10.1371/journal.pone.0050106
Eastment, M. C., McClelland, R. S. (2018). Vaginal microbiota and susceptibility to HIV (London, England: AIDS) 32, 687–698. doi: 10.1097/QAD.0000000000001768
Edwards, V. L., Smith, S. B., McComb, E. J., Tamarelle, J., Ma, B., Humphrys, M. S., et al. (2019). The cervicovaginal microbiota-host interaction modulates chlamydia trachomatis infection. mBio 10 (4), e01548–19. doi: 10.1128/mBio.01548-19
Elwood, C., Albert, A., McClymont, E., Wagner, E., Mahal, D., Devakandan, K., et al. (2020). Different and diverse anaerobic microbiota were seen in women living with HIV with unsuppressed HIV viral load and in women with recurrent bacterial vaginosis: A cohort study. BJOG 127 (2), 250–259. doi: 10.1111/1471-0528.15930
Fan, Q., Wu, Y., Li, M., An, F., Yao, L., Wang, M., et al. (2021). Lactobacillus spp. create a protective micro-ecological environment through regulating the core fucosylation of vaginal epithelial cells against cervical cancer. Cell Death Dis. 12 (12), 1094. doi: 10.1038/s41419-021-04388-y
Fang, B., Li, Q., Wan, Z., Ouyang, Z., Zhang, Q. (2022). Exploring the association between cervical microbiota and HR-HPV infection based on 16S rRNA gene and metagenomic sequencing. Front. Cell Infect. Microbiol. 12. doi: 10.3389/fcimb.2022.922554
Farr Zuend, N. C., Tobin Nicole, H., Vera, T., Kotyrba, L, Noël-Romas, L., Birse, K., et al. (2020). Pregnancy associates with alterations to the host and microbial proteome in vaginal mucosa. Am. J. Reprod. Immunol. 83 (6), e13235. doi: 10.1111/aji.13235
Ferreira, C. S. T., da Silva, MárciaGuimarães., de Pontes, LetíciaG., Dos Santos, L. D., Marconi, C. (2018). Protein content of cervicovaginal fluid is altered during bacterial vaginosis. J. Low Genit Tract Dis. 22 (2), 147–151. doi: 10.1097/LGT.0000000000000367
Ferreira, C. S. T., Donders, G. G., Parada, C., Tristão, A. D. R., Fernandes, T., Da Silva, M. G., et al. (2017). Treatment failure of bacterial vaginosis is not associated with higher loads of atopobium vaginae and gardnerella vaginalis. J. Med. Microbiol. 66 (8), 1217–1224. doi: 10.1099/jmm.0.000561
Fettweis, J. M., Serrano, M. G., Brooks, J. P., Edwards, D. J., Girerd, P. H., Parikh, H. I., et al. (2019). The vaginal microbiome and preterm birth. Nat. Med. 25 (6), 1012–1021. doi: 10.1038/s41591-019-0450-2
Filardo, S., Di Pietro, M., Porpora, M. G., Recine, N., Farcomeni, A., Latino, M. A., et al. (2017). Diversity of cervical microbiota in asymptomatic chlamydia trachomatis genital infection: A pilot study. Front. Cell. infection Microbiol. 7. doi: 10.3389/fcimb.2017.00321
Filardo, S., Di Pietro, M., Tranquilli, G., Latino, M. A., Recine, N., Porpora, M. G., et al. (2019). Selected immunological mediators and cervical microbial signatures in women with chlamydia trachomatis infection. mSystems 4 (4), e00094–19. doi: 10.1128/mSystems.00094-19
France, M. T., Ma, B., Gajer, P., Brown, S., Humphrys, M. S., Holm, J. B., et al. (2020). VALENCIA: A nearest centroid classification method for vaginal microbial communities based on composition. Microbiome 8 (1), 166. doi: 10.1186/s40168-020-00934-6
Gajer, P., Brotman, R. M., Bai, G., Sakamoto, J., Schütte, U. M., Zhong, X., et al. (2012). Temporal dynamics of the human vaginal microbiota. Sci. Trans. Med. 4 (132), 132ra52. doi: 10.1126/scitranslmed.3003605
Gardner, J. K., Łaniewski, Paweł., Knight, A., Haddad, L. B., Swaims-Kohlmeier, A., Herbst-Kralovetz, M. M. (2020). Interleukin-36γ is elevated in cervicovaginal epithelial cells in women with bacterial vaginosis and in vitro after infection with microbes associated with bacterial vaginosis. J. Infect. Dis. 221 (6), 983–988. doi: 10.1093/infdis/jiz514
Gebhardt, T., Mueller, S. N., Heath, W. R., Carbone, F. R. (2013). Peripheral tissue surveillance and residency by memory T cells. Trends Immunol. 34 (1), 27–32. doi: 10.1016/j.it.2012.08.008
Gerson, K. D., Anton, L., Ferguson, B., Ravel, J., Burris, H. H., Elovitz, M. A. (2022). Gardnerella vaginalis induces matrix metalloproteinases in the cervicovaginal epithelium through TLR-2 activation. J. Reprod. Immunol. 152, 103648. doi: 10.1016/j.jri.2022.103648
Ghanavati, R., Asadollahi, P., Shapourabadi, M. B., Razavi, S., Talebi, M., Rohani, M. (2020). Inhibitory effects of lactobacilli cocktail on HT-29 colon carcinoma cells growth and modulation of the notch and wnt/β-catenin signaling pathways. Microb. Pathog. 139, 103829. doi: 10.1016/j.micpath.2019.103829
Ghosh, M., Rodriguez-Garcia, M., Wira, C. R. (2014). The immune system in menopause: pros and cons of hormone therapy. J. Steroid Biochem. Mol. Biol. 142, 171–175. doi: 10.1016/j.jsbmb.2013.09.003
Ghosh, M., Shen, Z., Fahey, J. V., Crist, S. G., Patel, M., Smith, J. M., et al. (2013). Pathogen recognition in the human female reproductive tract: Expression of intracellular cytosolic sensors NOD1, NOD2, RIG-1, and MDA5 and response to HIV-1 and neisseria gonorrhea. Am. J. Reprod. Immunol. 69 (1), 41–51. doi: 10.1111/aji.12019
Gipson, I. K., Moccia, R., Spurr-Michaud, S., Argüeso, P., Gargiulo, A. R., Hill, J. A., et al. (2001). The amount of MUC5B mucin in cervical mucus peaks at midcycle. J. Clin. Endocrinol. Metab. 86 (2), 594–600. doi: 10.1210/jcem.86.2.7174
Gipson, I. K., Spurr-Michaud, S., Moccia, R., Zhan, Q., Toribara, N., Ho, S. B., et al. (1999). MUC4 and MUC5B transcripts are the prevalent mucin messenger ribonucleic acids of the human endocervix. Biol. Reprod. 60 (1), 58–64. doi: 10.1095/biolreprod60.1.58
Gliniewicz, K., Schneider, G. M., Ridenhour, B. J., Williams, C. J., Song, Y., Farage, M. A., et al. (2019). Comparison of the vaginal microbiomes of premenopausal and postmenopausal women. Front. Microbiol. 10. doi: 10.3389/fmicb.2019.00193
Gosmann, C., Anahtar, M. N., Handley, S. A., Farcasanu, M., Abu-Ali, G., Bowman, B. A., et al. (2017). Lactobacillus-deficient cervicovaginal bacterial communities are associated with increased HIV acquisition in young south African women. Immunity 46 (1), 29–37. doi: 10.1016/j.immuni.2016.12.013
Grant, K. S., Wira, C. R. (2003). Effect of mouse uterine stromal cells on epithelial cell transepithelial resistance (TER) and TNFalpha and TGFbeta release in culture. Biol. Reprod. 69 (3), 1091–1098. doi: 10.1095/biolreprod.103.015495
Gustin, A. T., Thurman Andrea, R., Chandra, N., Schifanella, L., Alcaide, M., Fichorova, R., et al. (2022). Recurrent bacterial vaginosis following metronidazole treatment is associated with microbiota richness at diagnosis. Am J Obstet Gynecol. 226(2), 225.e1–225.e15. doi: 10.1016/j.ajog.2021.09.018
Hall, O. J., Klein, S. L. (2017). Progesterone-based compounds affect immune responses and susceptibility to infections at diverse mucosal sites. Mucosal Immunol. 10 (5), 1097–1107. doi: 10.1038/mi.2017.35
Han, L., Park, D., Reddy, A., Wilmarth, P. A., Jensen, J. T. (2021). Comparing endocervical mucus proteome of humans and rhesus macaques. Proteomics Clin. Appl. 15 (4), e2100023. doi: 10.1002/prca.202100023
Han, L., Taub, R., Jensen, J. T. (2017). Cervical mucus and contraception: what we know and what we don't. Contraception 96 (5), 310–321. doi: 10.1016/j.contraception.2017.07.168
Hansson, G. C. (2020). Mucins and the Microbiome. Annu Rev Biochem, 89(undefined), 769–793. doi: 10.1146/annurev-biochem-011520-105053
Hearps, A. C., Tyssen, D., Srbinovski, D., Bayigga, L., Diaz, D. J. D., Aldunate, M., et al. (2017). Vaginal lactic acid elicits an anti-inflammatory response from human cervicovaginal epithelial cells and inhibits production of pro-inflammatory mediators associated with HIV acquisition. Mucosal Immunol. 10 (6), 1480–1490. doi: 10.1038/mi.2017.27
Herold, B. C., Keller, M. J., Shi, Q., Hoover, D. R., Carpenter, C. A., Huber, A., et al. (2013). Plasma and mucosal HIV viral loads are associated with genital tract inflammation in HIV-infected women. J. Acquir. Immune Defic. Syndr. 63 (4), 485–493. doi: 10.1097/QAI.0b013e3182961cfc
Hickey, D. K., Patel, M. V., Fahey, J. V., Wira, C. R. (2011). Innate and adaptive immunity at mucosal surfaces of the female reproductive tract: stratification and integration of immune protection against the transmission of sexually transmitted infections. J. Reprod. Immunol. 88 (2), 185–194. doi: 10.1016/j.jri.2011.01.005
Hladik, F., Sakchalathorn, P., Ballweber, L., Lentz, G., Fialkow, M., Eschenbach, D., et al. (2007). Initial events in establishing vaginal entry and infection by human immunodeficiency virus type-1. Immunity 26 (2), 257–270. doi: 10.1016/j.immuni.2007.01.007
Hunter, P. J., Sheikh, S., David, A. L., Peebles, D. M., Klein, N. (2016). Cervical leukocytes and spontaneous preterm birth. J. Reprod. Immunol. 113, 42–49. doi: 10.1016/j.jri.2015.11.002
Iijima, N., Iwasaki, A. (2014). T Cell memory. a local macrophage chemokine network sustains protective tissue-resident memory CD4 T cells. Science 346 (6205), 93–98. doi: 10.1126/science.1257530
Ilhan, Z. E., Łaniewski, P., Tonachio, A., Herbst-Kralovetz, M. M. (2020). Members of prevotella genus distinctively modulate innate immune and barrier functions in a human three-dimensional endometrial epithelial cell model. J. Infect. Dis. 222 (12), 2082–2092. doi: 10.1093/infdis/jiaa324
Jan, R. L., Yeh, K. C., Hsieh, M. H., Lin, Y. L., Kao, H. F., Li, P. H., et al. (2012). Lactobacillus gasseri suppresses Th17 pro-inflammatory response and attenuates allergen-induced airway inflammation in a mouse model of allergic asthma. Br. J. Nutr. 108 (1), 130–139. doi: 10.1017/S0007114511005265
Jang, S.-E., Jeong, J.-J., Choi, S.-Y., Kim, H., Han, M. J., Kim, D.-H. (2017). Lactobacillus rhamnosus HN001 and lactobacillus acidophilus la-14 attenuate gardnerella vaginalis-infected bacterial vaginosis in mice. Nutrients 9 (6), 531. doi: 10.3390/nu9060531
Jie, Z., Chen, C., Hao, L., Li, F., Song, L., Zhang, X., et al. (2021). Life history recorded in the vagino-cervical microbiome along with multi-omics. Genomics Proteomics Bioinf. 20 (2), 304–321. doi: 10.1016/j.gpb.2021.01.005
Joag, V., Obila, O., Gajer, P., Scott Milcah, C., Dizzell, S., Humphrys, M., et al. (2019). Impact of standard bacterial vaginosis treatment on the genital microbiota, immune milieu, and ex vivo human immunodeficiency virus susceptibility. Clin. Infect. Dis. 68 (10), 1675–1683. doi: 10.1093/cid/ciy762
Johnson, T. J., Clark, M. R., Albright, T. H., Nebeker, J. S., Tuitupou, A. L., Clark, J. T., et al. (2012). A 90-day tenofovir reservoir intravaginal ring for mucosal HIV prophylaxis. Antimicrob. Agents Chemother. 56 (12), 6272–6283. doi: 10.1128/AAC.01431-12
Kawahara, R., Fujii, T., Kukimoto, I., Nomura, H., Kawasaki, R., Nishio, E., et al. (2021). Changes to the cervicovaginal microbiota and cervical cytokine profile following surgery for cervical intraepithelial neoplasia. Sci Rep 11(1), 2156. doi: 10.1038/s41598-020-80176-6
Kero, K., Hieta, N., Kallonen, T., Ahtikoski, A., Laine, H. K., Rautava, J., et al. (2023). Optimal sampling and analysis methods for clinical diagnostics of vaginal microbiome. Eur. J. Clin. Microbiol. Infect. Dis. 42 (2), 201–208. doi: 10.1007/s10096-022-04545-x
Kim, S., Seo, H., Rahim, M. A., Lee, S., Kim, Y. S., Song, H. Y. (2021). Changes in the microbiome of vaginal fluid after menopause in Korean women. J. Microbiol. Biotechnol. 31 (11), 1490–1500. doi: 10.4014/jmb.2106.06022
Kim, T. K., Thomas, S. M., Ho, M., Sharma, S., Reich, C. I., Frank, J. A., et al. (2009). Heterogeneity of vaginal microbial communities within individuals. J. Clin. Microbiol. 47 (4), 1181–1189. doi: 10.1128/JCM.00854-08
Klatt, N. R., Cheu, R., Birse, K., Zevin, A. S., Perner, M., Noël-Romas, L., et al. (2017). Vaginal bacteria modify HIV tenofovir microbicide efficacy in African women. Science 356 (6341), 938–945. doi: 10.1126/science.aai9383
Kobayashi, K., Matsunaga, K., Tsugami, Y., Wakasa, H., Nishimura, T. (2021). IL-1β is a key inflammatory cytokine that weakens lactation-specific tight junctions of mammary epithelial cells. Exp. Cell Res. 409 (2), 112938. doi: 10.1016/j.yexcr.2021.112938
Kolev, Y., Uetake, H., Takagi, Y., Sugihara, K. (2008). Lactate dehydrogenase-5 (LDH-5) expression in human gastric cancer: Association with hypoxia-inducible factor (HIF-1alpha) pathway, angiogenic factors production and poor prognosis. Ann. Surg. Oncol. 15 (8), 2336–2344. doi: 10.1245/s10434-008-9955-5
Kreisel, K. M., Weston, E. J., St Cyr, S. B., Spicknall, I. H. (2021). Estimates of the prevalence and incidence of chlamydia and gonorrhea among US men and women 2018. Sex Transm Dis. 48 (4), 222–231. doi: 10.1097/OLQ.0000000000001382
Krog, M. C., Hugerth, L. W., Fransson, E., Bashir, Z., Nyboe Andersen, A., Edfeldt, G., et al. (2022). The healthy female microbiome across body sites: Effect of hormonal contraceptives and the menstrual cycle. Hum. Reprod. 37 (7), 1525–1543. doi: 10.1093/humrep/deac094
Kwok, L., Stapleton, A. E., Stamm, W. E., Hillier, S. L., Wobbe, C. L., Gupta, K. (2006). Adherence of lactobacillus crispatus to vaginal epithelial cells from women with or without a history of recurrent urinary tract infection. J. Urol. 176 (5), 2050–2054. doi: 10.1016/j.juro.2006.07.014
Kyongo, J. K., Crucitti, T., Menten, J., Hardy, L., Cools, P., Michiels, J., et al. (2015). Cross-sectional analysis of selected genital tract immunological markers and molecular vaginal microbiota in Sub-Saharan African women, with relevance to HIV risk and prevention. Clin. Vaccine Immunol. 22 (5), 526–538. doi: 10.1128/CVI.00762-14
Łaniewski, P., Herbst-Kralovetz, M. M. (2021). Bacterial vaginosis and health-associated bacteria modulate the immunometabolic landscape in 3D model of human cervix. NPJ Biofilms Microbiomes 7 (1), 88. doi: 10.1038/s41522-021-00259-8
Łaniewski, P., Barnes, D., Goulder, A., Cui, H., Roe Denise, J., Chase Dana, M., et al. (2018). Linking cervicovaginal immune signatures, HPV and microbiota composition in cervical carcinogenesis in non-Hispanic and Hispanic women. Sci. Rep. 8(1), 7593. doi: 10.1038/s41598-018-25879-7
Lebeau, A., Bruyere, D., Roncarati, P., Peixoto, P., Hervouet, E., Cobraiville, G., et al. (2022). HPV infection alters vaginal microbiome through down-regulating host mucosal innate peptides used by lactobacilli as amino acid sources. Nat. Commun. 13 (1), 1076. doi: 10.1038/s41467-022-28724-8
Leccese Terraf, M. C., Mendoza, L. M., Juárez Tomás, M. S., Silva, C., Nader-Macías, M. E. F. (2014). Phenotypic surface properties (aggregation, adhesion and biofilm formation) and presence of related genes in beneficial vaginal lactobacilli. J. Appl. Microbiol. 117 (6), 1761–1772. doi: 10.1111/jam.12642
Leppert, P. C. (1995). Anatomy and physiology of cervical ripening. Clin. Obstet Gynecol 38 (2), 267–279. doi: 10.1097/00003081-199506000-00009
Li, C., Jia, L., Yu, Y., Jin, L. (2019). Lactic acid induced microRNA-744 enhances motility of SiHa cervical cancer cells through targeting ARHGAP5. Chem. Biol. Interact. 298 (undefined), 86–95. doi: 10.1016/j.cbi.2018.10.027
Li, B., Sui, L. (2021). Metabolic reprogramming in cervical cancer and metabolomics perspectives. Nutr. Metab. (Lond) 18 (1), 93. doi: 10.1186/s12986-021-00615-7
Lin, F., Zhang, B., Shi, Q., Liang, J., Wang, X., Lian, X., et al. (2021). The conditioned medium of lactobacillus rhamnoides GG regulates Microglia/Macrophage polarization and improves functional recovery after spinal cord injury in rats. BioMed. Res. Int. 2021 (undefined), 3376496. doi: 10.1155/2021/3376496
Lithgow, K. V., Buchholz, V. C.H., Ku, E., Konschuh, S., D'Aubeterre, A., Sycuro, L. K. (2022). Protease activities of vaginal porphyromonas species disrupt coagulation and extracellular matrix in the cervicovaginal niche. NPJ Biofilms Microbiomes 8 (1), 8. doi: 10.1038/s41522-022-00270-7
Liu, L., Chen, Yu., Chen, J.-L., Xu, H.-J., Zhan, H.-Y., Chen, Z., et al. (2021b). Integrated metagenomics and metabolomics analysis of third-trimester pregnant women with premature membrane rupture: A pilot study. Ann. Transl. Med. 9 (23), 1724. doi: 10.21037/atm-21-5539
Liu, C.-W., Su, B.-C., Chen, J.-Y. (2021a). Gardnerella vaginalisTilapia piscidin 4 (TP4) reprograms M1 macrophages to M2 phenotypes in cell models of -induced vaginosis. Front. Immunol. 12 (undefined). doi: 10.3389/fimmu.2021.773013
López-Filloy, M., Cortez Flor, J., Gheit, T., Cruz Cruz, Y. O., Cruz-Talonia, F., Chávez-Torres, M., et al. (2022). Altered vaginal microbiota composition correlates with human papillomavirus and mucosal immune responses in women with symptomatic cervical ectopy. Front. Cell. Infect. Microbiol. 12(undefined), 884272. doi: 10.3389/fcimb.2022.884272
Ma, L.-N., Huang, X.-B., Muyayalo, K. P., Mor, G., Liao, A.-H. (2020). Lactic acid: A novel signaling molecule in early pregnancy? Front. Immunol. 11 (undefined). doi: 10.3389/fimmu.2020.00279
Maarsingh, J. D., Łaniewski, P., Herbst-Kralovetz, M. M. (2022). Immunometabolic and potential tumor-promoting changes in 3D cervical cell models infected with bacterial vaginosis-associated bacteria. Commun. Biol. 5 (1), 725. doi: 10.1038/s42003-022-03681-6
Mahajan, G., Doherty, E., To, T., Sutherland, A., Grant, J., Junaid, A., et al. (2022). Vaginal microbiome-host interactions modeled in a human vagina-on-a-chip. Microbiome 10 (1), 201. doi: 10.1186/s40168-022-01400-1
Manzanares-Leal, G. L., Coronel-Martínez, J. A., Rodríguez-Morales, M., Rangel-Cuevas, Iván., Bustamante-Montes, L. P., Sandoval-Trujillo, H., et al. (2022). Preliminary identification of the aerobic cervicovaginal microbiota in Mexican women with cervical cancer as the first step towards metagenomic studies. Front. Cell Infect. Microbiol. 12 (undefined). doi: 10.3389/fcimb.2022.838491
Mastromarino, P., Di Pietro, M., Schiavoni, G., Nardis, C., Gentile, M., Sessa, R. (2014). Effects of vaginal lactobacilli in chlamydia trachomatis infection. Int. J. Med. Microbiol. 304 (null), 654–661. doi: 10.1016/j.ijmm.2014.04.006
Mattei, V., Murugesan, S., Al Hashmi, M., Mathew, R., James, N., Singh, P., et al. (2019). Evaluation of methods for the extraction of microbial DNA from vaginal swabs used for microbiome studies. Front. Cell Infect. Microbiol. 9. doi: 10.3389/fcimb.2019.00197
McGregor, J. A., Lawellin, D., Franco-Buff, A., Todd, J. K., Makowski, E. L. (1986). Protease production by microorganisms associated with reproductive tract infection. Am. J. Obstet Gynecol 154 (1), 109–114. doi: 10.1016/0002-9378(86)90404-7
McKenzie, R., Maarsingh, J. D., Łaniewski, P., Herbst-Kralovetz, M. M. (2021). Immunometabolic analysis of mobiluncus mulieris and eggerthella sp. reveals novel insights into their pathogenic contributions to the hallmarks of bacterial vaginosis. Front. Cell. infection Microbiol. 11. doi: 10.3389/fcimb.2021.759697
Mirmonsef, P., Zariffard, M. R., Gilbert, D., Makinde, H., Landay, A. L., Spear, G. T. (2012). Short-chain fatty acids induce pro-inflammatory cytokine production alone and in combination with toll-like receptor ligands. Am. J. Reprod. Immunol. 67 (5), 391–400. doi: 10.1111/j.1600-0897.2011.01089.x
Mitchell, C. M., Ma, N., Mitchell, A. J., Wu, M. C., Valint, D. J., Proll, S., et al. (2021a). Association between postmenopausal vulvovaginal discomfort, vaginal microbiota, and mucosal inflammation. Am. J. Obstet Gynecol 225 (2), 159.e151–159.e115. doi: 10.1016/j.ajog.2021.02.034
Mitchell, C., Marrazzo, J. (2014). Bacterial vaginosis and the cervicovaginal immune response. Am. J. Reprod. Immunol. (New York N.Y. 1989) 71 (6), 555–563. doi: 10.1111/aji.12264
Mitchell, C. M., Srinivasan, S., Ma, N., Reed, S. D., Wu, M. C., Hoffman, N. G., et al. (2021b). Bacterial communities associated with abnormal Nugent score in postmenopausal versus premenopausal women. J. Infect. Dis. 223 (12), 2048–2052. doi: 10.1093/infdis/jiaa675
Mitra, A., MacIntyre, D. A., Lee, Y. S., Smith, A., Marchesi, J. R., Lehne, B, et al. (2015). Cervical intraepithelial neoplasia disease progression is associated with increased vaginal microbiome diversity. Sci. Rep. 5 (undefined), 16865. doi: 10.1038/srep16865
Mitra, A., MacIntyre, D. A., Ntritsos, G., Smith, A., Tsilidis, K. K., Marchesi, J. R., et al. (2020). The vaginal microbiota associates with the regression of untreated cervical intraepithelial neoplasia 2 lesions. Nat. Commun. 11 (1), 1999. doi: 10.1038/s41467-020-15856-y
Mohammadi, A., Bagherichimeh, S., Choi, Y., Fazel, A., Tevlin, E., Huibner, S., et al. (2022). Immune parameters of HIV susceptibility in the female genital tract before and after penile-vaginal sex. Commun. Med. (Lond) 2 (undefined), 60. doi: 10.1038/s43856-022-00122-7
Mohammadi, A., Bagherichimeh, S., Perry, M. C., Fazel, A., Tevlin, E., Huibner, S., et al. (2020). The impact of cervical cytobrush sampling on cervico-vaginal immune parameters and microbiota relevant to HIV susceptibility. Sci. Rep. 10 (1), 8514. doi: 10.1038/s41598-020-65544-6
Molano, M., Meijer, C. J.L.M., Weiderpass, E., Arslan, A., Posso, H., Franceschi, S., et al. (2005). The natural course of chlamydia trachomatis infection in asymptomatic Colombian women: A 5-year follow-up study. J. Infect. Dis. 191 (6), 907–916. doi: 10.1086/428287
Mollin, A., Katta, M., Sobel, J., Akins, R. J. P. O. (2022). Association of key species of vaginal bacteria of recurrent bacterial vaginosis patients before and after oral metronidazole therapy with short- and long-term clinical outcomes. PloS One 17 (7), e0272012. doi: 10.1371/journal.pone.0272012
Moncla, B. J., Chappell, C. A., Debo, B. M., Meyn, L. A. (2016). The effects of hormones and vaginal microflora on the glycome of the female genital tract: Cervical-vaginal fluid. PloS One 11 (7), e0158687. doi: 10.1371/journal.pone.0158687
Morré, S. A., van den Brule, A. J.C., Rozendaal, L., Boeke, A.J. P., Voorhorst Feja J., de Blok, S., et al. (2002). The natural course of asymptomatic chlamydia trachomatis infections: 45% clearance and no development of clinical PID after one-year follow-up. Int. J. STD AIDS null (undefined), 12–18. doi: 10.1258/095646202762226092
Moscicki, A. B., Shi, B., Huang, H., Barnard, E., Li, H. (2020). Cervical-vaginal microbiome and associated cytokine profiles in a prospective study of HPV 16 acquisition, persistence, and clearance. Front. Cell. infection Microbiol. 10. doi: 10.3389/fcimb.2020.569022
Motevaseli, E., Azam, R., Akrami, S. M., Mazlomy, M., Saffari, M., Modarressi, M. H., et al. (2016). The effect of lactobacillus crispatus and lactobacillus rhamnosusCulture supernatants on expression of autophagy genes and HPV E6 and E7 oncogenes in the HeLa cell line. Cell J. 17 (4), 601–607. doi: 10.22074/cellj.2016.3833
Mott, P. D., Taylor, C. M., Lillis, R. A., Ardizzone, C. M., Albritton, H. L., Luo, M., et al. (2021). Differences in the genital microbiota in women who naturally clear chlamydia trachomatis infection compared to women who do not clear; a pilot study. Front. Cell. infection Microbiol. 11. doi: 10.3389/fcimb.2021.615770
Muñoz-Planillo, R., Kuffa, P., Martínez-Colón, G., Smith, B. L., Rajendiran, T. M., Núñez, G. (2013). K⁺ efflux is the common trigger of NLRP3 inflammasome activation by bacterial toxins and particulate matter. Immunity 38 (6), 1142–1153. doi: 10.1016/j.immuni.2013.05.016
Munusamy, P. S., Thiruvengadam, K., Tellapragada, C., Ambikan, A. T., Narayanan, A., Kathirvel, S., et al. (2021). Deciphering the role of mucosal immune responses and the cervicovaginal microbiome in resistance to HIV infection in HIV-exposed seronegative (HESN) women. Microbiol. Spectr. 9 (2), e0047021. doi: 10.1128/Spectrum.00470-21
Mwenda, N., Nduati, R., Kosgey, M., Kerich, G. (2021). Effect of bacterial vaginosis (BV)-HIV-1 Co-existence on maternal and infant health: A secondary data analysis. Front. Pediatr. 9 (undefined). doi: 10.3389/fped.2021.544192
Nagakubo, T., Nomura, N., Toyofuku, M. (2019). Cracking open bacterial membrane vesicles. Front. Microbiol. 10 (undefined). doi: 10.3389/fmicb.2019.03026
Ñahui, P. R. A., Vanpouille, C., Laghi, L., Parolin, C., Melikov, K., Backlund, P., et al. (2019). Extracellular vesicles from symbiotic vaginal lactobacilli inhibit HIV-1 infection of human tissues. Nat. Commun. 10 (1), 5656. doi: 10.1038/s41467-019-13468-9
Ñahui, P. R. A., Zicari, S., Vanpouille, C., Vitali, B., Margolis, L. (2017). LactobacillusVaginal inhibits HIV-1 replication in human tissues. Front. Microbiol. 8 (undefined). doi: 10.3389/fmicb.2017.00906
Ng, S., Chen, M., Kundu, S., Wang, X., Zhou, Z., Zheng, Z., et al. (2021). Large-Scale characterisation of the pregnancy vaginal microbiome and sialidase activity in a low-risk Chinese population. NPJ biofilms microbiomes 7 (1), 89. doi: 10.1038/s41522-021-00261-0
Nicolò, S., Tanturli, M., Mattiuz, G., Antonelli, A., Baccani, I., Bonaiuto, C., et al. (2021). Vaginal lactobacilli and vaginal dysbiosis-associated bacteria differently affect cervical epithelial and immune homeostasis and anti-viral defenses. Int. J. Mol. Sci. 22 (12), 6487. doi: 10.3390/ijms22126487
Nishiyama, K., Sugiyama, M., Mukai, T. (2016). Adhesion properties of lactic acid bacteria on intestinal mucin. Microorganisms 4 (3), 34. doi: 10.3390/microorganisms4030034
Norenhag, J., Du, J., Olovsson, M., Verstraelen, H., Engstrand, L., Brusselaers, N. (2020). The vaginal microbiota, human papillomavirus and cervical dysplasia: A systematic review and network meta-analysis. BJOG 127 (2), 171–180. doi: 10.1111/1471-0528.15854
Nunn, K. L., Wang, Y.-Y., Harit, D., Humphrys, M. S., Ma, B., Cone, R., et al. (2015). Enhanced trapping of HIV-1 by human cervicovaginal mucus is associated with lactobacillus crispatus-dominant microbiota. mBio 6 (5), e01084–e01015. doi: 10.1128/mBio.01084-15
Oerlemans, E., Ahannach, S., Wittouck, S., Dehay, E., De Boeck, I., Ballet, N., et al. (2022). Impacts of menstruation, community type, and an oral yeast probiotic on the vaginal microbiome. mSphere 7 (5), e0023922. doi: 10.1128/msphere.00239-22
Parada, V. D., de la Fuente, M. K., Landskron, G., González, MaríaJ., Quera, R., Dijkstra, G., et al. (2019). Short chain fatty acids (SCFAs)-mediated gut epithelial and immune regulation and its relevance for inflammatory bowel diseases. Front. Immunol. 10 (undefined). doi: 10.3389/fimmu.2019.00277
Park, J., Kim, M., Kang, S. G., Jannasch, A. H., Cooper, B., Patterson, J., et al. (2015). Short-chain fatty acids induce both effector and regulatory T cells by suppression of histone deacetylases and regulation of the mTOR-S6K pathway. Mucosal Immunol. 8 (1), 80–93. doi: 10.1038/mi.2014.44
Parolin, C., Frisco, G., Foschi, C., Giordani, B., Salvo, M., Vitali, B., et al. (2018). Lactobacillus crispatus BC5 interferes with infectivity through integrin modulation in cervical cells. Front. Microbiol. 9 (undefined). doi: 10.3389/fmicb.2018.02630
Pattacini, L., Woodward, D. A., Czartoski, J., Mair, F., Presnell, S., Hughes, S. M., et al. (2019). A pro-inflammatory CD8+ T-cell subset patrols the cervicovaginal tract. Mucosal Immunol. 12 (5), 1118–1129. doi: 10.1038/s41385-019-0186-9
Plisko, O., Zodzika, J., Jermakova, I., Pcolkina, K., Prusakevica, A., Liepniece-Karele, I., et al. (2021). Aerobic vaginitis-underestimated risk factor for cervical intraepithelial neoplasia. Diagnostics (Basel) 11 (1), 97. doi: 10.3390/diagnostics11010097
Pudney, J., Quayle, A. J., Anderson, D. J. (2005). Immunological microenvironments in the human vagina and cervix: Mediators of cellular immunity are concentrated in the cervical transformation zone. Biol. Reprod. 73 (6), 1253–1263. doi: 10.1095/biolreprod.105.043133
Radtke, A. L., Quayle, A. J., Herbst-Kralovetz, M. M. (2012). Microbial products alter the expression of membrane-associated mucin and antimicrobial peptides in a three-dimensional human endocervical epithelial cell model. Biol. Reprod. 87 (6), 132. doi: 10.1095/biolreprod.112.103366
Raimondi, S., Candeliere, F., Amaretti, A., Foschi, C., Morselli, S., Gaspari, V., et al. (2021). Chlamydia trachomatis vaginal and anal microbiome during infections. Pathogens 10 (10), 1347. doi: 10.3390/pathogens10101347
Rajeeve, K., Vollmuth, N., Janaki-Raman, S., Wulff, T. F., Baluapuri, A., Dejure, F. R., et al. (2020). Reprogramming of host glutamine metabolism during chlamydia trachomatis infection and its key role in peptidoglycan synthesis. Nat. Microbiol. 5 (11), 1390–1402. doi: 10.1038/s41564-020-0762-5
Ravel, J., Gajer, P., Abdo, Z., Schneider, G. M., Koenig, S. S., McCulle, S. L., et al. (2011). Vaginal microbiome of reproductive-age women. Proc. Natl. Acad. Sci. United States America 108 Suppl 1 (Suppl 1), 4680–4687. doi: 10.1073/pnas.1002611107
Read, C. P., Word, R.A., Ruscheinsky, M. A., Timmons, B. C., Mahendroo, M. S. (2007). Cervical remodeling during pregnancy and parturition: Molecular characterization of the softening phase in mice. Reproduction 134 (2), 327–340. doi: 10.1530/REP-07-0032
Rizzo, A., Fiorentino, M., Buommino, E., Donnarumma, G., Losacco, A., Bevilacqua, N. (2015). Lactobacillus crispatus mediates anti-inflammatory cytokine interleukin-10 induction in response to chlamydia trachomatis infection in vitro. Int. J. Med. Microbiol. 305 (8), 815–827. doi: 10.1016/j.ijmm.2015.07.005
Robinson, L. S., Schwebke, J., Lewis, W. G., Lewis, A. L. (2019). Gardnerella vaginalisIdentification and characterization of NanH2 and NanH3, enzymes responsible for sialidase activity in the vaginal bacterium. J. Biol. Chem. 294 (14), 5230–5245. doi: 10.1074/jbc.RA118.006221
Rodriguez-Garcia, M., Barr, F. D., Crist, S. G., Fahey, J. V., Wira, C. R. (2014). Phenotype and susceptibility to HIV infection of CD4+ Th17 cells in the human female reproductive tract. Mucosal Immunol. 7 (6), 1375–1385. doi: 10.1038/mi.2014.26
Rodriguez-Garcia, M., Fortier, J. M., Barr, F. D., Wira, C. R. (2018). Aging impacts CD103 CD8 T cell presence and induction by dendritic cells in the genital tract. Aging Cell 17 (3), e12733. doi: 10.1111/acel.12733
Rodriguez-Garcia, M., Shen, Z., Fortier, J. M., Wira, C. R. (2020). Differential cytotoxic function of resident and non-resident CD8+ T cells in the human female reproductive tract before and after menopause. Front. Immunol. 11 (undefined). doi: 10.3389/fimmu.2020.01096
Rosato, P. C., Beura, L. K., Masopust, D. (2017). Tissue resident memory T cells and viral immunity. Curr. Opin. Virol. 22, 44–50. doi: 10.1016/j.coviro.2016.11.011
Sahu, U., Khare, P. (2021). Role of interleukin-17 in human papillomavirus infection and associated malignancies. Microb. Pathog. 161 (null), 105294. doi: 10.1016/j.micpath.2021.105294
Salliss, M. E., Maarsingh, J. D., Garza, C., Łaniewski, P., Herbst-Kralovetz, M. M. (2021). Veillonellaceae family members uniquely alter the cervical metabolic microenvironment in a human three-dimensional epithelial model. NPJ biofilms microbiomes 7 (1), 57. doi: 10.1038/s41522-021-00229-0
Sathaliyawala, T., Kubota, M., Yudanin, N., Turner, D., Camp, P., Thome, J. J.C., et al. (2013). Distribution and compartmentalization of human circulating and tissue-resident memory T cell subsets. Immunity 38 (1), 187–197. doi: 10.1016/j.immuni.2012.09.020
Schenkel, J. M., Fraser, K. A., Beura, L. K., Pauken, K. E., Vezys, V., Masopust, D. (2014). T Cell memory. resident memory CD8 T cells trigger protective innate and adaptive immune responses. Science 346 (6205), 98–101. doi: 10.1126/science.1254536
Schnaars, V., Dörries, M., Hutchins, M., Wöhlbrand, L., Rabus, R. (2018). What's the difference? 2D DIGE image analysis by DeCyderTM versus SameSpotsTM. J. Mol. Microbiol. Biotechnol. 28 (3), 128–136. doi: 10.1159/000494083
Scillato, M., Spitale, A., Mongelli, G., Privitera, G. F., Mangano, K., Cianci, A., et al. (2021). Antimicrobial properties of lactobacillus cell-free supernatants against multidrug-resistant urogenital pathogens. Microbiologyopen 10 (2), e1173. doi: 10.1002/mbo3.1173
Serebrenik, J., Wang, T, Hunte, R., Srinivasan, S., McWalters, J., Tharp Gregory, K., et al. (2021). Differences in vaginal microbiota, host transcriptome, and proteins in women with bacterial vaginosis are associated with metronidazole treatment response. J. Infect. Dis. 224 (12), 2094–2104. doi: 10.1093/infdis/jiab266
Sessa, R., Di Pietro, M., Filardo, S., Bressan, A., Rosa, L., Cutone, A., et al. (2017). Effect of bovine lactoferrin on chlamydia trachomatis infection and inflammation. Biochem. Cell Biol. 95 (1), 34–40. doi: 10.1139/bcb-2016-0049
Shannon, B., Gajer, P., Yi, T. J., Ma, B., Humphrys, M. S., Thomas-Pavanel, J., et al. (2017). Distinct effects of the cervicovaginal microbiota and herpes simplex type 2 infection on female genital tract immunology. J. Infect. Dis. 215 (9), 1366–1375. doi: 10.1093/infdis/jix088
Shardell, M., Gravitt, P. E., Burke, A. E., Ravel, J., Brotman, R. M. (2021). Association of vaginal microbiota with signs and symptoms of the genitourinary syndrome of menopause across reproductive stages. J. Gerontol A Biol. Sci. Med. Sci. 76 (9), 1542–1550. doi: 10.1093/gerona/glab120
Short, C.-E. S., Brown, R. G., Quinlan, R., Lee, Y. S., Smith, A., Marchesi, J. R., et al. (2020). Lactobacillus-depleted vaginal microbiota in pregnant women living with HIV-1 infection are associated with increased local inflammation and preterm birth. Front. Cell Infect. Microbiol. 10 (undefined). doi: 10.3389/fcimb.2020.596917
Short, C.-E. S., Quinlan, R. A., Wang, X., Preda, V. G., Smith, A., Marchesi, J. R., et al. (2021). Vaginal microbiota, genital inflammation and extracellular matrix remodelling collagenase: MMP-9 in pregnant women with HIV, a potential preterm birth mechanism warranting further exploration. Front. Cell Infect. Microbiol. 11 (undefined). doi: 10.3389/fcimb.2021.750103
Shukair, S. A., Allen, S. A., Cianci, G. C., Stieh, D. J., Anderson, M. R., Baig, S. M., et al. (2013). Human cervicovaginal mucus contains an activity that hinders HIV-1 movement. Mucosal Immunol. 6(2), 427–34. doi: 10.1038/mi.2012.87.
Sierra, L.-J., Brown, A. G., Barilá, G. O., Anton, L., Barnum, C. E., Shetye, S. S., et al. (2018). Colonization of the cervicovaginal space with gardnerella vaginalis leads to local inflammation and cervical remodeling in pregnant mice. PloS One 13 (1), e0191524. doi: 10.1371/journal.pone.0191524
Sirichoat, A., Sankuntaw, N., Engchanil, C., Buppasiri, P., Faksri, K., Namwat, W., et al. (2021). Comparison of different hypervariable regions of 16S rRNA for taxonomic profiling of vaginal microbiota using next-generation sequencing. Arch. Microbiol. 203 (3), 1159–1166. doi: 10.1007/s00203-020-02114-4
Smith, S. B., Ravel, J. (2017). The vaginal microbiota, host defence and reproductive physiology. J. Physiol. 595 (2), 451–463. doi: 10.1113/JP271694
Sobia, P., Pillay, T., Liebenberg, L. J.P., Sivro, A., Mansoor, L. E., Osman, F., et al. (2021). Higher mucosal antibody concentrations in women with genital tract inflammation. Sci. Rep. 11 (1), 23514. doi: 10.1038/s41598-021-02954-0
Song, S. D., Acharya, K. D., Zhu, J. E., Deveney, C. M., Walther-Antonio, M. R. S., Tetel, M. J., et al. (2020). Daily vaginal microbiota fluctuations associated with natural hormonal cycle, contraceptives, diet, and exercise. mSphere 5 (4), e00593–20. doi: 10.1128/mSphere.00593-20
Srinivasan, S., Morgan, M. T., Fiedler, T. L., Djukovic, D., Hoffman, N. G., Raftery, D., et al. (2015). Metabolic signatures of bacterial vaginosis. mBio 6 (2), e00204–15. doi: 10.1128/mBio.00204-15
Stallmann, S., Hegemann, J. H. (2016). The chlamydia trachomatis Ctad1 invasin exploits the human integrin β1 receptor for host cell entry. Cell Microbiol. 18 (5), 761–775. doi: 10.1111/cmi.12549
Sungur, T., Aslim, B., Karaaslan, C., Aktas, B. (2017). Impact of exopolysaccharides (EPSs) of lactobacillus gasseri strains isolated from human vagina on cervical tumor cells (HeLa). Anaerobe 47, 137–144. doi: 10.1016/j.anaerobe.2017.05.013
Takada, K., Komine-Aizawa, S., Kuramochi, T., Ito, S., Trinh, Q. D., Pham Ngan, T. K., et al. (2018). Lactobacillus crispatus accelerates re-epithelialization in vaginal epithelial cell line MS74. Am. J. Reprod. Immunol. 80 (3), e13027. doi: 10.1111/aji.13027
Taku, O., Onywera, H., Mbulawa, Z. Z. A., Businge, C. B., Meiring, T. L., Williamson, A. L. (2022). Molecular identification of cervical microbes in HIV-negative and HIV-positive women in an African setting using a customized bacterial vaginosis microbial DNA quantitative PCR (qPCR) array. Microbiol. Spectr. 10 (3), e0222921. doi: 10.1128/spectrum.02229-21
Tamarelle, J., Ma, B., Gajer, P., Humphrys, M. S., Terplan, M., Mark, K. S., et al. (2020). Nonoptimal vaginal microbiota after azithromycin treatment for chlamydia trachomatis infection. J. Infect. Dis. 221 (4), 627–635. doi: 10.1093/infdis/jiz499
Tamarelle, J., Thiébaut, A. C. M., de Barbeyrac, B., Bébéar, C., Ravel, J., Delarocque-Astagneau, E. (2019). The vaginal microbiota and its association with human papillomavirus, chlamydia trachomatis, neisseria gonorrhoeae and mycoplasma genitalium infections: A systematic review and meta-analysis. Clin. Microbiol. Infect. 25 (1), 35–47. doi: 10.1016/j.cmi.2018.04.019
Taneva, E., Sinclair, S., Mesquita, P. Mm., Weinrick, B., Cameron, S. A., Cheshenko, N., et al. (2018). Vaginal microbiome modulates topical antiretroviral drug pharmacokinetics. JCI Insight 3 (13), e99545. doi: 10.1172/jci.insight.99545
Tantengco, O. A. G., Richardson, L. S., Medina, P. M. B., Han, A., Menon, R. (2021a). Organ-on-chip of the cervical epithelial layer: A platform to study normal and pathological cellular remodeling of the cervix. FASEB J. 35 (4), e21463. doi: 10.1096/fj.202002590RRR
Tantengco, O. A. G., Vink, J., Medina, P. M. B., Menon, R. (2021b). Oxidative stress promotes cellular damages in the cervix: Implications for normal and pathologic cervical function in human pregnancy†. Biol. Reprod. 105 (1), 204–216. doi: 10.1093/biolre/ioab058
Theodoridis, G., Gika, H., Want, E., Wilson, I. (2012). Liquid chromatography-mass spectrometry based global metabolite profiling: A review. Anal. Chim. Acta 711, 7–16. doi: 10.1016/j.aca.2011.09.042
Thurman, A. R., Kimble, T., Herold, B., Mesquita, P. M.M., Fichorova, R. N., Dawood, H. Y., et al. (2015). Bacterial vaginosis and subclinical markers of genital tract inflammation and mucosal immunity. AIDS Res. Hum. Retroviruses 31 (11), 1139–1152. doi: 10.1089/aid.2015.0006
Thurman, A. R., Ravel, J., Gajer, P., Marzinke, M. A., Ouattara, L. A., Jacot, T., et al. (2022). Vaginal microbiota and mucosal pharmacokinetics of tenofovir in healthy women using a 90-day Tenofovir/Levonorgestrel vaginal ring. Front. Cell Infect. Microbiol. 12 (undefined). doi: 10.3389/fcimb.2022.799501
Timmons, B. C., Mitchell, S. M., Gilpin, C., Mahendroo, M. S. (2007). Dynamic changes in the cervical epithelial tight junction complex and differentiation occur during cervical ripening and parturition. Endocrinology 148 (3), 1278–1287. doi: 10.1210/en.2006-0851
Trifonova, R. T., Lieberman, J., van Baarle, D. (2014). Distribution of immune cells in the human cervix and implications for HIV transmission. Am. J. Reprod. Immunol. 71 (3), 252–264. doi: 10.1111/aji.12198
Tyssen, D., Wang, Y.-Y., Hayward, J. A., Agius, P. A., DeLong, K., Aldunate, M., et al. (2018). Anti-HIV-1 activity of lactic acid in human cervicovaginal fluid. mSphere 3 (4), e00055–18. doi: 10.1128/mSphere.00055-18
Ulcova-Gallova, Z. (2010). Immunological and physicochemical properties of cervical ovulatory mucus. J. Reprod. Immunol. 86 (2), 115–121. doi: 10.1016/j.jri.2010.07.002
Usyk, M., Schlecht, N. F., Pickering, S., Williams, L., Sollecito, C. C., Gradissimo, A., et al. (2022). molBV reveals immune landscape of bacterial vaginosis and predicts human papillomavirus infection natural history. Nat. Commun. 13 (1), 233. doi: 10.1038/s41467-021-27628-3
Usyk, M., Zolnik, C. P., Castle, P. E., Porras, C., Herrero, R., Gradissimo, A., et al. (2020). Cervicovaginal microbiome and natural history of HPV in a longitudinal study. PloS Pathog. 16 (3), e1008376. doi: 10.1371/journal.ppat.1008376
Vandergeeten, C., Fromentin, R., Chomont, N. (2012). The role of cytokines in the establishment, persistence and eradication of the HIV reservoir. Cytokine Growth factor Rev. 23 (4-5), 143–149. doi: 10.1016/j.cytogfr.2012.05.001
van der Veer, C., Bruisten, S. M., van der Helm, J. J., de Vries, H. J.C., van Houdt, R. (2017). The cervicovaginal microbiota in women notified for chlamydia trachomatis infection: A case-control study at the sexually transmitted infection outpatient clinic in Amsterdam, the Netherlands. Clin. Infect. Dis. 64 (1), 24–31. doi: 10.1093/cid/ciw586
van Houdt, R., Ma, B., Bruisten, S. M., Speksnijder, A. G.C.L., Ravel, J., de Vries, H. J.C. (2018). Lactobacillus iners-dominated vaginal microbiota is associated with increased susceptibility to infection in Dutch women: A case-control study. Sex Transm Infect. 94 (2), 117–123. doi: 10.1136/sextrans-2017-053133
van Teijlingen, N. H., Helgers, L. C., Zijlstra-Willems, E. M., van Hamme, J. L., Ribeiro, C. M.S., Strijbis, K., et al. (2020). Vaginal dysbiosis associated-bacteria megasphaera elsdenii and prevotella timonensis induce immune activation via dendritic cells. J. Reprod. Immunol. 138 (undefined), 103085. doi: 10.1016/j.jri.2020.103085
Vick, E. J., Park, H. S., Huff, K. A., Brooks, K. M., Farone, A. L., Farone, M. B. (2014). Gardnerella vaginalis triggers NLRP3 inflammasome recruitment in THP-1 monocytes. J. Reprod. Immunol. 106, 67–75. doi: 10.1016/j.jri.2014.08.005
Vinolo, M., Rodrigues, H., Hatanaka, E., Hebeda, C., Farsky, S., Curi, R. (2009). Short-chain fatty acids stimulate the migration of neutrophils to inflammatory sites. Clin. Sci. (Lond) 117 (9), 331–338. doi: 10.1042/CS20080642
Virtanen, S., Kalliala, I., Nieminen, P., Salonen, A. (2017). Comparative analysis of vaginal microbiota sampling using 16S rRNA gene analysis. PloS One 12 (7), e0181477. doi: 10.1371/journal.pone.0181477
Vitali, B., Cruciani, F., Picone, G., Parolin, C., Donders, G., Laghi, L. (2015). Vaginal microbiome and metabolome highlight specific signatures of bacterial vaginosis. Eur. J. Clin. Microbiol. Infect. Dis. 34 (12), 2367–2376. doi: 10.1007/s10096-015-2490-y
Vollmuth, N., Schlicker, L., Guo, Y., Hovhannisyan, P., Janaki-Raman, S., Kurmasheva, N., et al. (2022). C-myc plays a key role in IFN-γ-induced persistence of chlamydia trachomatis. Elife 11, e76721. doi: 10.7554/eLife.76721
Wagner, W., Ciszewski, W. M., Kania, K. D. (2015). L- and d-lactate enhance DNA repair and modulate the resistance of cervical carcinoma cells to anticancer drugs via histone deacetylase inhibition and hydroxycarboxylic acid receptor 1 activation. Cell Commun. Signal 13 (undefined), 36. doi: 10.1186/s12964-015-0114-x
Wagner, W., Kania, K. D., Ciszewski, W. M. (2017). Stimulation of lactate receptor (HCAR1) affects cellular DNA repair capacity. DNA Repair (Amst) 52 (undefined), 49–58. doi: 10.1016/j.dnarep.2017.02.007
Wagner, W., Sobierajska, K., Kania, K. D., Paradowska, E., Ciszewski, W. Michał. (2021). Lactate suppresses retroviral transduction in cervical epithelial cells through DNA-PKcs modulation. Int. J. Mol. Sci. 22 (24), 13194. doi: 10.3390/ijms222413194
Walenta, S., Wetterling, M., Lehrke, M., Schwickert, G., Sundfør, K., Rofstad, E. K., et al. (2000). High lactate levels predict likelihood of metastases, tumor recurrence, and restricted patient survival in human cervical cancers. Cancer Res. 60 (4), 916–921. doi: 10.1016/j.diagmicrobio.2019.114981
Wang, X.-H., Song, T.-Z., Zheng, H.-Y., Li, Y.-H., Zheng, Y.-T. (2021b). Jejunal epithelial barrier disruption triggered by reactive oxygen species in early SIV infected rhesus macaques. Free Radic. Biol. Med. 177 (undefined), 143–155. doi: 10.1016/j.freeradbiomed.2021.10.026
Wang, R., Zhou, G., Wu, L., Huang, X., Li, Y., Luo, B., et al. (2021a). in vitroThe microbial composition of lower genital tract may affect the outcome of fertilization-embryo transfer. Front. Microbiol. 12 (undefined). doi: 10.3389/fmicb.2021.729744
Wang, C., Fan, A., Li, H., Yan, Y., Qi, W., Wang, Y., et al. (2020). Vaginal bacterial profiles of aerobic vaginitis: A case-control study. Diagn Microbiol Infect Dis 96(4), 114981. doi: 10.1016/j.diagmicrobio.2019.114981
Warburg, O. (1956). On the origin of cancer cells. Science 123(3191), 309–14. doi: 10.1126/science.123.3191.309
Watson, E., Reid, G. (2018). Metabolomics as a clinical testing method for the diagnosis of vaginal dysbiosis. Am. J. Reprod. Immunol. 80 (2), e12979. doi: 10.1111/aji.12979
White, H. D., Crassi, K. M., Givan, A. L., Stern, J. E., Gonzalez, J. L., Memoli, V. A., et al. (1997). CD3+ CD8+ CTL activity within the human female reproductive tract: Influence of stage of the menstrual cycle and menopause. J. Immunol. 158 (6), 3017–3027. doi: 10.4049/jimmunol.158.6.3017
Wiesenfeld, H. C., Hillier, S. L., Krohn, M. A., Landers, D. V., Sweet, R. L. (2003). Bacterial vaginosis is a strong predictor of neisseria gonorrhoeae and chlamydia trachomatis infection. Clin. Infect. Dis. 36 (5), 663–668. doi: 10.1086/367658
Wiggins, R., Millar, M. R., Soothill, P. W., Hicks, S. J., Corfield, A. P. (2002). Application of a novel human cervical mucin-based assay demonstrates the absence of increased mucinase activity in bacterial vaginosis. Int. J. STD AIDS 13 (11), 755–760. doi: 10.1258/095646202320753709
Wira, C. R., Fahey, J. V., Ghosh, M., Patel, M. V., Hickey, D. K., Ochiel, D. O. (2010). Sex hormone regulation of innate immunity in the female reproductive tract: the role of epithelial cells in balancing reproductive potential with protection against sexually transmitted pathogens. Am. J. Reprod. Immunol. 63 (6), 544–565. doi: 10.1111/j.1600-0897.2010.00842.x
Witkin, S. S., Mendes-Soares, H., Linhares, I. M., Jayaram, A., Ledger, W. J., Forney, L. J. (2013). Influence of vaginal bacteria and d- and l-lactic acid isomers on vaginal extracellular matrix metalloproteinase inducer: Implications for protection against upper genital tract infections. mBio 4 (4), e00460–e00413. doi: 10.1128/mBio.00460-13
Xiang, N., Yin, T., Chen, T. (2021). Gardnerella vaginalis induces NLRP3 inflammasome-mediated pyroptosis in macrophages and THP-1 monocytes. Exp. Ther. Med. 22 (4), 1174. doi: 10.3892/etm.2021.10609
Xiao, B., Qin, H., Mi, L., Zhang, D. (2022). Correlation analysis of vaginal microbiome changes and bacterial vaginosis plus vulvovaginal candidiasis mixed vaginitis prognosis. Front. Cell Infect. Microbiol. 12. doi: 10.3389/fcimb.2022.860589
Xiao, B., Wu, C., Song, W., Niu, X., Qin, N., Liu, Z., et al. (2019). Association analysis on recurrence of bacterial vaginosis revealed microbes and clinical variables important for treatment outcome. Front. Cell Infect. Microbiol. 9. doi: 10.3389/fcimb.2019.00189
Xue, J., Wang, Y., Chen, C., Zhu, X., Zhu, H., Hu, Y. (2018). Effects of Th17 cells and IL-17 in the progression of cervical carcinogenesis with high-risk human papillomavirus infection. Cancer Med. 7 (2), 297–306. doi: 10.1002/cam4.1279
Yamamoto, N., Kan-O, K., Tatsuta, M., Ishii, Y., Ogawa, T., Shinozaki, S., et al. (2021). Incense smoke-induced oxidative stress disrupts tight junctions and bronchial epithelial barrier integrity and induces airway hyperresponsiveness in mouse lungs. Sci. Rep. 11 (1), 7222. doi: 10.1038/s41598-021-86745-7
Yellon, S. M. (2019). Immunobiology of cervix ripening. Front. Immunol. 10 (undefined). doi: 10.3389/fimmu.2019.03156
Zariffard, M.R., Novak, R. M., Lurain, N., Sha, B. E., Graham, P., Spear, G. T. (2005). Induction of tumor necrosis factor- alpha secretion and toll-like receptor 2 and 4 mRNA expression by genital mucosal fluids from women with bacterial vaginosis. J. Infect. Dis. 191 (11), 1913–1921. doi: 10.1086/429922
Zevin, A. S., Xie, I. Y., Birse, K., Arnold, K., Romas, L., Westmacott, G., et al. (2016). Microbiome composition and function drives wound-healing impairment in the female genital tract. PloS Pathog. 12 (9), e1005889. doi: 10.1371/journal.ppat.1005889
Zhang, Z., Li, T., Zhang, D., Zong, X., Bai, H., Bi, H., et al. (2021). Distinction between vaginal and cervical microbiota in high-risk human papilloma virus-infected women in China. BMC Microbiol. 21 (1), 90. doi: 10.1186/s12866-021-02152-y
Zhang, Y., Xu, X., Yu, L., Shi, X., Min, M., Xiong, L., et al. (2022). Vaginal microbiota changes caused by HPV infection in Chinese women. Front. Cell Infect. Microbiol. 12 (undefined). doi: 10.3389/fcimb.2022.814668
Zhou, R., Lu, J., Wang, J., Xiao, B. (2022). Vaginal lactobacillus iners abundance is associated with outcome in antibiotic treatment of bacterial vaginosis and capable of inhibiting gardnerella. Front. Cell Infect. Microbiol. 12. doi: 10.3389/fcimb.2022.1033431
Ziklo, N., Huston, W. M., Taing, K., Katouli, M., Timms, P. (2016). In vitro rescue of genital strains of chlamydia trachomatis from interferon-γ and tryptophan depletion with indole-positive, but not indole-negative prevotella spp. BMC Microbiol. 16 (1), 286. doi: 10.1186/s12866-016-0903-4
Ziklo, N., Huston, W. M., Taing, K., Timms, P. (2019). High expression of IDO1 and TGF-β1 during recurrence and post infection clearance with chlamydia trachomatis, are independent of host IFN-γ response. BMC Infect. Dis. 19 (1), 218. doi: 10.1186/s12879-019-3843-4
Keywords: cervicovaginal microbiota, uterine cervix, epithelial, immune, mucus, chlamydia trachomatis, human papilloma virus, human immunodeficiency virus
Citation: Dong M, Dong Y, Bai J, Li H, Ma X, Li B, Wang C, Li H, Qi W, Wang Y, Fan A, Han C and Xue F (2023) Interactions between microbiota and cervical epithelial, immune, and mucus barrier. Front. Cell. Infect. Microbiol. 13:1124591. doi: 10.3389/fcimb.2023.1124591
Received: 15 December 2022; Accepted: 31 January 2023;
Published: 24 February 2023.
Edited by:
Tingtao Chen, Nanchang University, ChinaReviewed by:
Bingbing Xiao, First Hospital, Peking University, ChinaXinyue Hu, Nanchang University, China
Long Sui, Fudan University, China
Copyright © 2023 Dong, Dong, Bai, Li, Ma, Li, Wang, Li, Qi, Wang, Fan, Han and Xue. This is an open-access article distributed under the terms of the Creative Commons Attribution License (CC BY). The use, distribution or reproduction in other forums is permitted, provided the original author(s) and the copyright owner(s) are credited and that the original publication in this journal is cited, in accordance with accepted academic practice. No use, distribution or reproduction is permitted which does not comply with these terms.
*Correspondence: Cha Han, tjhancha@163.com; Fengxia Xue, xuefengxia@tmu.edu.cn
†These authors have contributed equally to this work