- 1Faculty of Veterinary Medicine, University of Calgary, Calgary, AB, Canada
- 2Laboratorio de Bacteriología, Grupo de Sanidad Animal, Unidad Integrada INTA, Universidad Nacional de Mar del Plata (UNMdP), Balcarce, Buenos Aires, Argentina
- 3Physiology and Pharmacology, Cumming School of Medicine, University of Calgary, Calgary, AB, Canada
- 4McCaig Institute for Bone and Joint Health, Cumming School of Medicine, University of Calgary, Calgary, AB, Canada
- 5Université Clermont Auvergne, Institut national de recherche pour l'agriculture, l'alimentation et l'environnement, Clermont-Ferrand, France
- 6National Food Institute, Technical University of Denmark, Kongens Lyngby, Denmark
- 7Department of Clinical Studies, Ontario Veterinary College, University of Guelph, Guelph, ON, Canada
Introduction: Calves are highly susceptible to gastrointestinal infection with Cryptosporidium parvum (C. parvum), which can result in watery diarrhea and eventually death or impaired development. With little to no effective therapeutics, understanding the host’s microbiota and pathogen interaction at the mucosal immune system has been critical to identify and test novel control strategies.
Methods: Herein, we used an experimental model of C. parvum challenge in neonatal calves to describe the clinical signs and histological and proteomic profiling of the mucosal innate immunity and microbiota shifts by metagenomics in the ileum and colon during cryptosporidiosis. Also, we investigated the impact of supplemental colostrum feeding on C. parvum infection.
Results: We showed that C. parvum challenged calves experienced clinical signs including pyrexia and diarrhea 5 days post challenge. These calves showed ulcerative neutrophil ileitis with a proteomic signature driven by inflammatory effectors, including reactive oxygen species and myeloperoxidases. Colitis was also noticed with an aggravated mucin barrier depletion and incompletely filled goblet cells. The C. parvum challenged calves also displayed a pronounced dysbiosis with a high prevalence of Clostridium species (spp.) and number of exotoxins, adherence factors, and secretion systems related to Clostridium spp. and other enteropathogens, including Campylobacter spp., Escherichia sp., Shigella spp., and Listeria spp. Daily supplementation with a high-quality bovine colostrum product mitigated some of the clinical signs and modulated the gut immune response and concomitant microbiota to a pattern more similar to that of healthy unchallenged calves.
Discussion: C. parvum infection in neonatal calves provoked severe diarrheic neutrophilic enterocolitis, perhaps augmented due to the lack of fully developed innate gut defenses. Colostrum supplementation showed limited effect mitigating diarrhea but demonstrated some clinical alleviation and specific modulatory influence on host gut immune responses and concomitant microbiota.
Introduction
Neonatal calves are highly susceptible to Cryptosporidium parvum (C. parvum), a life-threatening apicomplexan protozoan parasite that causes watery diarrhea, dehydration, impaired gut absorption (Klein et al., 2008), and, in severe cases, impaired weight gain or death (Shaw et al., 2020). C. parvum is a zoonotic agent causing gastroenteritis in a diversity of vertebrates, including cattle, and it is a leading global cause of diarrhea, illness, and death in young children (Collaborators, 2017). Young children and immunocompromised individuals are at highest risk when in close contact with cattle, who are a main reservoir (Thomson et al., 2017). Cryptosporidiosis is a re-emerging food and waterborne disease for which currently there are no vaccines (Dumaine et al., 2020; Guo et al., 2022). Treatments usually rely on palliative oral electrolytes and a few labeled parasite-specific drugs (e.g., halofuginone) (Brainard et al., 2021), which have limited efficacy (Thomson et al., 2017; Riggs and Schaefer, 2020) and are legally restricted in some countries. Therefore, research has been focused on understanding cryptosporidiosis pathogenesis and gut-pathogen interactions, so that therapeutic targets or host anti-parasite immune mechanisms can be developed. However, the mucosal immune response during cryptosporidiosis in calves remains incompletely described due to a lack of consistent experimental models and limited tools to replicate the parasitic life cycle under laboratory conditions.
Bovine colostrum is essential for the protection of newborn calves. Its consumption within a short period of time after birth is critical for health (Chigerwe et al., 2015), long-term growth (Abuelo et al., 2021), and future productive performance (Armengol and Fraile, 2020). Oral administration of hyperimmune colostrum reduced shedding and clinical disease in lambs and calves (Perryman et al., 1999; Martín-Gómez et al., 2005; Askari et al., 2016). Such protective effects of colostrum have been largely attributed to the high concentration of pathogen-specific immunoglobulins G (IgGs); however, even non-hyperimmune colostrum has been shown to protect calves from undifferentiated diarrhea (Berge et al., 2009; Chamorro et al., 2017). This could be due to several important non-IgG biomolecules. Bovine colostrum contains epidermal growth factor (EGF) (Xiao et al., 2002) that sustains intestinal integrity (Clark et al., 2009) and inhibits C. parvum-induced intestinal disruption (Buret et al., 2003). Bovine colostrum is also abundant in lactoferrin (Stelwagen et al., 2009), lactoperoxidase, caseins, cathepsins, oligosaccharides (Arslan et al., 2021), and cathelicidins (Stelwagen et al., 2009; Isobe et al., 2013), which promote gut integrity in pigs (Otte et al., 2009; Yi et al., 2016). Other non-IgG biomolecules are non-coding RNAs and microRNAs (miRNAs) (Lau et al., 2001), present in the bovine mammary gland (Putz et al., 2019; Sun et al., 2019) and milk (Chen et al., 2010) in association with IgG (Ma et al., 2022), which are capable of epigenetically regulating gene expression of the host (Pritchard et al., 2012). However, research on bovine colostrum has been mostly focused on ensuring transfer of passive immunity and gut development in newborn calves, while its effect as a treatment at the onset of diarrheic cryptosporidiosis is unknown. This study aimed to determine the intestinal mucosal immune response and microbiome differences in calves experimentally inoculated with C. parvum and whether supplementation with a bovine colostrum product containing high levels of IgG would mitigate the disease.
Materials and methods
Sample cattle population
Male, Holstein, or crossbred Holstein x Ayrshire calves (n = 25) less than 1 day of age were acquired from pre-selected farms with no known history of diagnosed cryptosporidiosis. The number of calves per group (n = 6) represented a proof-of-concept experiment based on previous studies with successful C. parvum infection rates and development of colitis/diarrhea in challenged calves (Peeters et al., 1992). Only calves born by unassisted birth and without any overt signs of disease or congenital abnormalities were eligible for enrollment. Calves were transported within the first 12 hours of life to an isolation facility at the University of Calgary and, upon arrival, underwent a complete physical examination by a veterinarian or trained animal health technician. Only those deemed healthy were enrolled in the study. Within 4h of birth while still at the farm of origin, calves received one feeding of bovine colostrum (100 g of IgG, 1.4 liters; Calf’s Choice Total ® powder, Saskatoon Colostrum Company Ltd, Saskatoon, Saskatchewan, Canada) by bottle, followed by an additional second (n = 25) and third (n = 21) similar feeding of the same bovine colostrum product within the first 24h of life either at the farm or upon arrival to the university facility. The third colostrum feeding was introduced due to low serum total protein concentrations observed in the first four calves. These four calves that received only 200 g IgG were evenly distributed among non-challenged treatment groups. Calves had ad libitum access to water and were housed individually in indoor pens that allowed visual contact among calves but no physical contact. Pens had rubber sleeping pads and wood shaving bedding, which was changed daily. Strict biosecurity protocols were employed to prevent the spread of pathogens among calves.
Experimental groups
In a randomized controlled trial, calves were randomly allocated into one of four groups at 3–4 days of age: were randomly allocated into one of four groups: unchallenged (i.e., Sham) calves fed milk replacer (Sham/MR), unchallenged calves fed colostrum (Sham/C), C. parvum challenged calves fed milk replacer (C. parvum/MR), and C. parvum challenged calves fed colostrum (C. parvum/C). On the day of the experimental challenge (0-day post-challenge, dpc), calves in the C. parvum/MR and C. parvum/C groups were orally administered isolated peracetic acid-disinfected C. parvum oocysts (5 × 107 Iowa isolate from Dr. MW. Riggs, School of Animal and Comparative Biomedical Sciences, University of Arizona) preserved in 5 ml of phosphate buffer solution (PBS) and mixed with 25 ml of milk (Riggs and Schaefer, 2020). Sham/MR and Sham/C calves received the same volume of inert PBS and milk. After the challenge, Sham/MR and C.parvum/MR calves were fed 2 liters of milk replacer (22% crude protein, 17% crude fat, and 0.15% crude fiber; Grober ProGro Milk Replacer, Grober Nutrition, Cambridge, ON, Canada) three times per day (8 a.m., 2 p.m., and 7 p.m.) via nipple bucket. Beginning at 2 dpc, Sham/C and C. parvum/C calves were fed bovine colostrum (1 liter, 100 g IgG/L, 60% crude protein, 19% crude fat; Saskatoon Colostrum Company Ltd, Saskatoon, SK, Canada) mixed with 1 liter of milk replacer in the morning feeding (8 a.m.) and 2 liters of milk replacer at the 2 p.m. and 7 p.m. feedings. If calves did not complete their feeding in the morning, the remaining volume of milk replacer or colostrum plus milk replacer was administered by tube feeder to eliminate any variability in colostrum consumption. The experimental design is depicted in Figure 1.
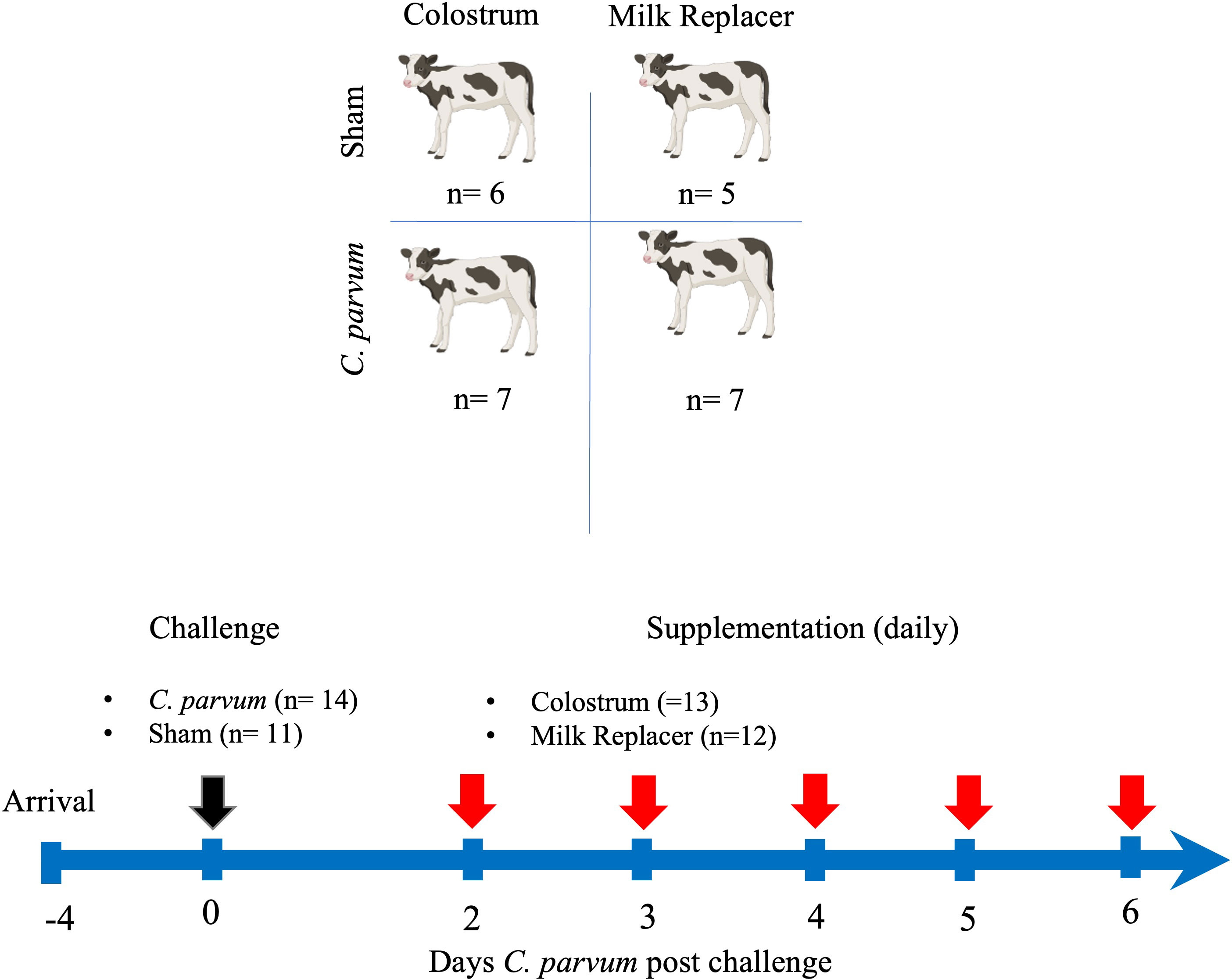
Figure 1 Experimental timeline. Schematic sequence of interventions, including groups of calves orally challenged (black arrow) with C. parvum (5 × 107 oocysts) or inert solution (Sham) that were fed with milk replacer (MR) (C. parvum/MR; n = 7 and Sham/MR; n = 5) or supplemented with colostrum (C) (1 liter in the morning) (C. parvum/C; n = 7 and Sham/C; n = 6) from 2 to 6 days post-challenge (dpc). Feedings with milk replacer or colostrum are indicated by red arrows. Calves were euthanized at 6 dpc.
Clinical examination and sampling of calves
Calves were examined daily, twice for Sham/MR and Sham/C calves and four times for C. parvum/MR and C. parvum/C calves, by trained individuals blinded to whether the calves were supplemented with colostrum or fed milk replacer only. Clinical parameters recorded included heart rate, respiratory rate, body temperature (normal: 38.5–39.5°C or abnormal: < 38.5 or ≥ 39.5°C), and a standardized health scoring system (Riggs and Schaefer, 2020) for attitude, hydration status, ability to rise, appetite, and fecal consistency with minor modifications (Table 1). Calves that reached an attitude, ability to rise, or hydration score of 2, or a fecal score of ≥ 3 received 50% of the subsequent meal as milk replacer and the other 50% as oral electrolyte solution (OES; V-lytes HE, Vetoquinol, Lavaltrie, Canada) (1:1) by nipple bottle or tube feeder. An attitude, ability to rise, or hydration score of 3, or an appetite score of 4 for two consecutive feedings was considered a humane end point, in which case the calf was euthanized by a veterinarian. The euthanasia protocol consisted of inducing anesthesia with xylazine and alfaxalone and then terminating calves using either potassium chloride (KCl) intravenously or a captive bolt gun.
Fecal samples were collected and stored at −20°C for polymerase chain reaction (PCR) identification of C. parvum (0, 2, 4, and 6 dpc and on the first day of required OES treatment), screening for other enteropathogens including Escherichia coli (E. coli) K99, Salmonella sp., and bovine rota- and coronavirus (0 and 6 dpc) (Animal Health Centre, Abbotsford, BC, Canada), and microbiome profiling (0, 4, and 6 dpc). At 6 dpc, calves were terminated as explained above and, within 1h post-mortem, ileum, mesenteric lymph nodes, and proximal spiral colon and respective fluids were sampled.
Gut and mucin barrier assessment
Colon and ileum samples (1 cm2) were washed twice in phosphate-buffered saline (PBS) and fixed in 10% neutral buffered formalin for 24h at room temperature. Tissues were dehydrated in increasing the concentrations of ethanol, embedded in paraffin wax, cut (5 μm), and mounted onto glass slides. Tissues were stained with hematoxylin (6765008; Thermo Fisher Scientific) and eosin (6766008; Thermo Fisher Scientific) (H&E) for histological examination and Alcian blue (Periodic acid–Schiff, PAS) for mucin layer assessment. For further study of goblet cell morphology and mucin barrier, lectin histochemistry specific against highly glycosylated proteins in mucin was conducted in formalin paraffined wax-embedded slides (Belote et al., 2018; Larzábal et al., 2020). Tissues were dewaxed and antigenic epitopes were heat-retrieved before incubating the slides with nuclei counterstain 4′,6-diamidino-2-phenylindole (DAPI) (Thermo Fisher Scientific, 1:1,000), and Alexa 647 conjugated wheat germ agglutinin (WGA) lectin (Thermo Fisher Scientific, 1:500 diluted in TBS buffer with bovine serum albumin (2% w/v); 20 min, room temperature). Slides stained with DAPI only served as control (Lindén et al., 2008). Slides were rinsed in distilled water, mounted with ProLong™ Gold Antifade Mountant (Thermo Fisher Scientific, Thermo Fisher Scientific, Waltham, MA, USA), and examined using a FluoView FV1000 confocal immunofluorescence microscope (Olympus Lifescience, Tokyo, Japan). Slides were examined, by individuals blinded to experimental group, for epithelial erosion, hemorrhage, leukocyte infiltration, and lectin blotted mucin barrier (Lindén et al., 2008; Erben et al., 2014) (Table 2). Images were taken with a ZEISS AXIO (ZEISS Innovation Center, Dublin, CA, USA) microscope (20X, NA 0.5) and analyzed by ZEN 2.6 (2018, Munich, Germany) software. Fluorescent WGA (green) was calculated using ImageJ 1.53c software (National Institute of Health, Bethesda, MD, USA) and represented as mean fluorescence intensity (MFI).
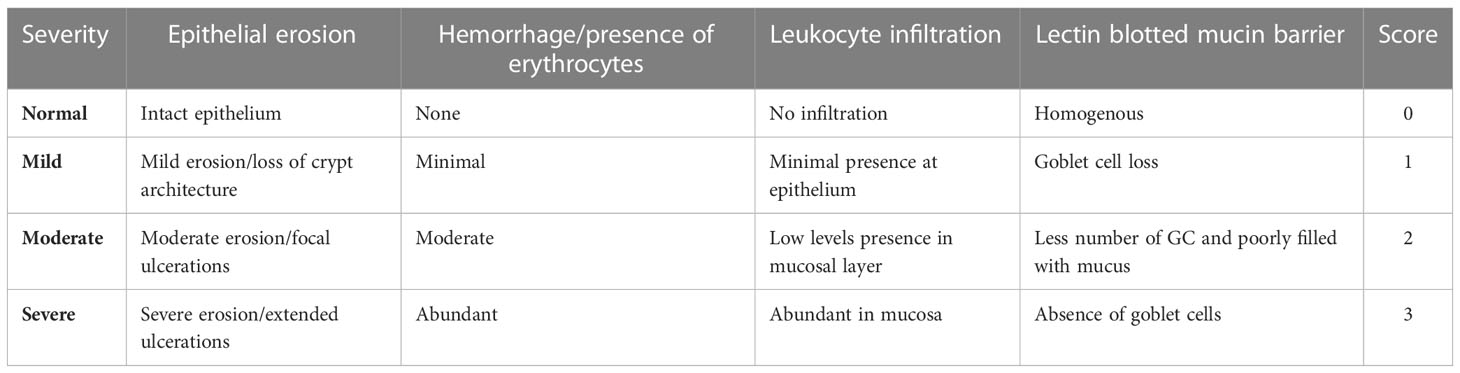
Table 2 Rubric for histological assessment of enterocolitis and mucin barrier in calves adapted from previous publications (Lindén et al., 2008; Erben et al., 2014).
Transcriptional expression of pro/anti−inflammatory cytokines and host defense peptides in ileum and colon
Relative messenger RNA (mRNA) concentration of cytokines IL-8, IL-10, IFN-γ, and TNF-α, and cathelicidin 5 were determined in ileum and colon by quantitative real-time polymerase chain reaction (qPCR) using pre-designed primers (RT2 qPCR Primer Assay, Qiagen) specific for bovine (# GADPH PPB00298A, HPRT1 PPB00330A, TNFα PPB00153A, INFγ PPB00336A, IL10 PPB339A, and CATH5 PPB00921). Total cellular RNA was extracted from tissue homogenates (Thermo Fisher Scientific, Waltham, MA). Each sample was treated with chloroform and centrifuged (10 min, 12,000g, 4°C) and nucleic acids precipitated by isopropanol. Pellets were washed with ice-cold 70% ethanol and resuspended in RNAse-free water to obtain complementary DNA (cDNA) (Quantabio reverse transcriptase, Thermo Fisher Scientific, Waltham, MA). Target gene mRNA values were corrected relative to the normalizer, GAPDH. Data were analyzed using the 2−ΔΔCT method (Bustin et al., 2009) and reported as mean fold change of target transcript levels in each experimental group.
Proteomic profiling in ileum
Ileum samples from each calf were subjected to a quantitative shotgun proteomic analysis including high-performance liquid chromatography (HPLC) and mass spectrometry. Tissues were lysed in a buffer composed of 1% SDS, 200 mM HEPES (pH 8.0), 100 mM ammonium bicarbonate, 10 mM EDTA, and protease inhibitor cOmplete tablets (Roche, Mannheim, Germany). Disulfide bonds of 100 μg of total protein were reduced with 10 mM Tris (2-carboxyethyl) phosphine hydrochloride (Thermo Fisher Scientific, Waltham, MA) at 55°C for 1 h. The proteins were alkylated by incubation with 15 mM iodoacetamide (VWR) for 25 min in the dark at room temperature. Proteins were precipitated out of solution by adding 600 μl of ice-cold acetone and incubated at 20°C overnight. Samples were centrifuged at 8,000g for 10 min before resuspension in 100 μl of 50 mM triethyl ammonium bicarbonate. Proteins were trypsinized (Thermo Fisher Scientific, Waltham, MA) overnight at a 1:10 enzyme-to-substrate ratio. For tandem mass tag (TMT) 6-plex labeling (TMTsixplex™ isobaric label reagent set, Thermo Fisher Scientific, Waltham, MA), 0.8 mg of TMT reagent was resuspended in 41 μl of acetonitrile and samples were spun down quickly at 2,000 rpm (380g) for 10 s and incubated at room temperature for 1 h. A total of four biological replicates per group (n = 4 calves per group) were labeled with TMT reagents and one TMT tag (131) contained the pooled samples [i.e., equal amounts of peptides (20 µg) of each sample per group] and served as internal standards for normalizing the data across the groups. For labeling, peptides were incubated with TMT reagents (room temperature, 1 h), and the reaction was quenched by adding 8 μl of 5% hydroxylamine and incubated for 15 min at 25°C. Peptides with different labels were combined before 100% formic acid was added to each sample to reach a volumetric concentration of 1% formic acid. Samples were spun at 5,000 rpm (2,350g) for 10 min and desalted using Sep-Pak C18 columns (Waters, 130 mg WAT023501). Sep-Pak columns were conditioned with 1 × 3 ml 90% methanol/0.1% TFA, 1× 2 ml 0.1% formic acid. Each sample was loaded onto a column and washed with 1× 3 ml 0.1% TFA/5% methanol. Peptides were eluted off the column with 1 × 1 ml 50% ACN/0.1% formic acid and lyophilized. Peptides were resuspended in 1% formic acid and a BCA assay (Thermo Fisher Scientific, Waltham, MA) was used to determine the concentration of peptide in each sample. Samples were dried down and stored at −80°C.
Liquid chromatography and mass spectrometry experiments were performed on an Orbitrap Fusion Lumos Tribrid mass spectrometer (Thermo Fisher Scientific, Waltham, MA) operated with Xcalibur (version 4.0.21.10) and coupled to a Thermo Scientific Easy-nLC (nanoflow Liquid Chromatography) 1200 system. Tryptic peptides (2 μg) were loaded onto a C18 trap (75 μm × 2 cm; Acclaim PepMap 100, P/N 164946; Thermo Fisher Scientific, Waltham, MA) at a flow rate of 2 μl/min of solvent A (0.1% formic acid and 3% acetonitrile in LC mass spectrometry grade water). Peptides were eluted using a 120-min gradient from 5 to 40% (5 to 28% in 105 min followed by an increase to 40% B in 15 min) of solvent B (0.1% formic acid in 80% LC–mass spectrometry grade acetonitrile) at a flow rate of 0.3 μl/min and separated on a C18 analytical column (75 µm × 50 cm; PepMap RSLC C18; P/N ES803; Thermo Fisher Scientific, Waltham, MA). Peptides were electrosprayed using 2.3 kV voltage into the ion transfer tube (300°C) of the Orbitrap Lumos operating in positive mode. The Orbitrap first performed a full mass spectrometry scan at a resolution of 120,000 FWHM to detect the precursor ion having a mass-to-charge ratio (m/z) between 375 and 1,575 and with a range of +2 and +4 charges. The Orbitrap AGC (Auto Gain Control) and the maximum injection time were set at 4 × 105 and 50 ms, respectively. The Orbitrap was operated using the top speed mode with a 3-s cycle time for precursor selection. The most intense precursor ions presenting a peptidic isotopic profile and having an intensity threshold of at least 2 × 104 were isolated using the quadrupole (isolation window of m/z 0.7) and fragmented with HCD (38% collision energy) in the ion routing Multipole. The fragment ions (MS2) were analyzed in the Orbitrap at a resolution of 15,000. The AGC, the maximum injection time, and the first mass were set at 1 × 105, 105 ms and 100, respectively. Dynamic exclusion was enabled for 45 s to avoid the acquisition of same precursor ions having a similar m/z ( ± 10 ppm).
For bioinformatic analysis, spectral data were matched to peptide sequences against a bovine and C. parvum UniProt protein database using the Andromeda algorithm (Cox et al., 2011) as implemented in the MaxQuant (Cox and Mann, 2008) software package v.1.6.0.1, at a peptide-spectrum match false discovery rate (FDR) of < 0.01. Search parameters included a mass tolerance of 20 p.p.m. for the parent ion, 0.5 Da for the fragment ion, carbamidomethylation of cysteine residues (+ 57.021464 Da), variable N-terminal modification by acetylation (+ 42.010565 Da), and variable methionine oxidation (+ 15.994915 Da). TMT 6-plex labels 126–131 were defined as labels for relative quantification. The cleavage site specificity was set to trypsin/P (search for free N terminus and for only lysine), with up to two missed cleavages allowed. An average of the normalized results for each group was calculated and followed by the ratio of each group comparison. The ratios were log (2) transformed, and the significant outlier cutoff values were determined after log (2) transformation by boxplot-and-whiskers analysis using the BoxPlotR tool.
Shotgun metagenomic sequencing in feces
Fecal samples for each calf collected at 0 and 6 dpc were sequenced using a KAPA Dual-indexed PCR-free library in a 6000 NovaSeq. The raw reads were trimmed using Trimmomatic (Bolger et al., 2014) and filtered to remove adapters and Bowtie (Langmead et al., 2009) with the latest Bos Taurus RefSeq assembly (GCF_002263795.1) to remove cow reads. Filtered reads were mapped to the virulence factor database (mgc.ac.cn/VFs/) and a customized Metagenome Assembled Genomes database using KMA (Clausen et al., 2018). Data integration for pathway and Gene Ontology (GO) enrichment was performed with Metascape (Zhou et al., 2019) and STRING-db (Szklarczyk et al., 2019). For virulence feature characterization, read counts were transformed by converting counts to proportions, dividing gene counts by gene length, and correcting them by dividing by the total counts of VFdb features for each sample. Virulence gene counts were converted to abundance tables and imported in R-studio for further analysis. For microbiota characterization, MAG (Metagenome Assembled Genomes) catalogs (database) representative of the predicted bacterial genomes in the samples were used. The MAGs were built using MEGAHIT assembler (Li et al., 2015) and autometa (Miller et al., 2019) and filtered using CheckM to remove the ones below 85% completeness and > 5% contamination (Parks et al., 2015). The newly built MAGs and the ones from a previous study (Teseo et al., 2022) using dRep (Olm et al., 2017) were compared, thus creating a non-redundant dataset of representative genomes for the samples. For abundance matrices, the read counts per sample were estimated using KMA and corrected by converting counts to proportions [(MAG contig counts/contig length)/average genome size] calculated using MicrobeCensus (Nayfach and Pollard, 2015).
Statistical analysis
Statistical analysis of clinical and histological data was performed with Prism (v9.0) (Graph Pad San Diego, CA, USA). The descriptive analysis was performed on all variables, and normality of the data was tested, when possible, by Kolmogorov–Smirnov test. Values were presented as mean and standard deviation (SD) or median and interquartile range (IQR), as appropriate based on the distribution. For consistency and comparisons among experimental groups, the average daily physical examination results were analyzed. Clinical parameters (i.e., heart rate, respiratory rate, temperature, hydration, attitude, appetite, fecal consistency, and ability to rise) were evaluated by repeated measures two-way analysis of variance (ANOVA), while histopathology scoring results were compared by one-way ANOVA. A Geisser–Greenhouse correction was applied when sphericity was not assumed due to the small sample size, followed by Tukey test for multiple comparisons. Clinical and histopathology scoring results are displayed as summary graphs of the means with bars representing SD or standard errors of the mean (SEM) as indicated in the figure legends. The number of OES treatments was compared by Chi-square or Fisher’s exact test, as appropriate.
For proteomic bioinformatic analysis, GO annotation, proteins basic functions, domain functional descriptions, and annotation of biological processes were studied by the UniProt-GOA database (http://www.ebi.ac.uk/GOA/), InterPro domain database (http://www.ebi.ac.uk/interpro/), and the Kyoto Encyclopedia of Genes and Genomes (KEGG) data- base (http://www.genome.jp/kaas-bin/kaas_main; http://www.kegg.jp/kegg/mapper.html). A two-tailed Fisher’s exact test was employed to test the enrichment of the differentially expressed proteins (DEPs) against all identified proteins. All the categories obtained after enrichment were collected and filtered for those categories that were at least enriched in one of the clusters with a P-value < 0.05. This filtered P-value matrix was transformed and clustered using one-way hierarchical clustering in Genesis. Clusters were visualized using the R Package pheatmap (https://cran.r-project.org/web/packages/cluster/). Pathway enrichment and protein networking were analyzed by an online meta-analysis tool (metascape.org) (Zhou et al., 2019). All DEPs’ database accession numbers or sequences were searched against STRING database (v11) for protein–protein interaction (PPI) analysis and interaction networks visualized in Cytoscape (Shannon et al., 2003). P-values of < 0.05 were considered statistically significant for all analyses [MaxQuant software (v.1.6.0.1) using a peptide FDR of 0.01].
For the metagenomic analysis, barplots were plotted using ggplot2 to visualize features overview. Differential representation analysis was performed using the indicspecies package. Alpha diversity was calculated using the absolute number of observed features (Sobs), and the Shannon index and groups were compared using the non-parametric Kruskal–Wallis tests. Beta-diversity ordination was calculated by either Bray–Curtis distances and non-metric dimensional scale (NMDS) or, alternatively, by imputing the 0s in the abundance matrices, CLR-transform them, and running principal component analysis. Significant contrasts were evaluated using the Adonis package in R.
Results
Clinical parameters and fecal shedding
A total of 25 calves (Sham/MR: n = 5; Sham/C: n = 6; C. parvum/MR: n = 7, C. parvum/C: n = 7) were enrolled in this study. The age at enrollment (i.e., day of sham inoculation or C. parvum inoculation) was not different for Sham/MR and Sham/C calves (mean = 3.6 days; SD = 1.1 days) compared with C. parvum/MR and C. parvum/C calves (mean = 4.4 days; SD = 1.3 days) calves (P = 0.5). Heart rates (range: 76 to 208 bpm; Figure 2A), respiratory rates (range: 20–88 bpm), appetite, hydration, and ability to rise slightly varied over time but did not differ substantially among experimental groups (P > 0.05). Calves in the C. parvum/MR group were more depressed (based on attitude score, P = 0.01) on 6 dpc when compared with Sham group calves, but calves in the C. parvum/C group were not significantly different from non-challenged groups (P > 0.2) (Figure 2B). Rectal temperatures were similar in all calves at enrollment (37.8–39.0°C) but increased in calves challenged with C. parvum (both C. parvum/MR and C. parvum/C) starting on 5 dpc compared with Sham calves (Figure 2C). Rectal temperatures in the C. parvum/MR calves were significantly higher on 5 and 6 dpc (P = 0.02; Figure 2C) when compared with Sham calves (Sham/MR and Sham/C), while there was no statistically significant difference among the C.parvum/C calves, Sham/MR, and Sham/C calves (P = 0.07). However, rectal temperatures of calves in the C.parvum/MR and C.parvum/C groups were similar 6 dpc (P = 0.9). Challenge with C. parvum provoked diarrhea as manifested by abnormal, loose to watery feces, regardless of colostrum supplementation in all but three calves. Average fecal scores on 5 and 6 dpc were significantly higher in C. parvum/MR and C. parvum/C calves (P < 0.005 and P < 0.05, respectively; Figure 2D) compared with scores of calves in the Sham groups.
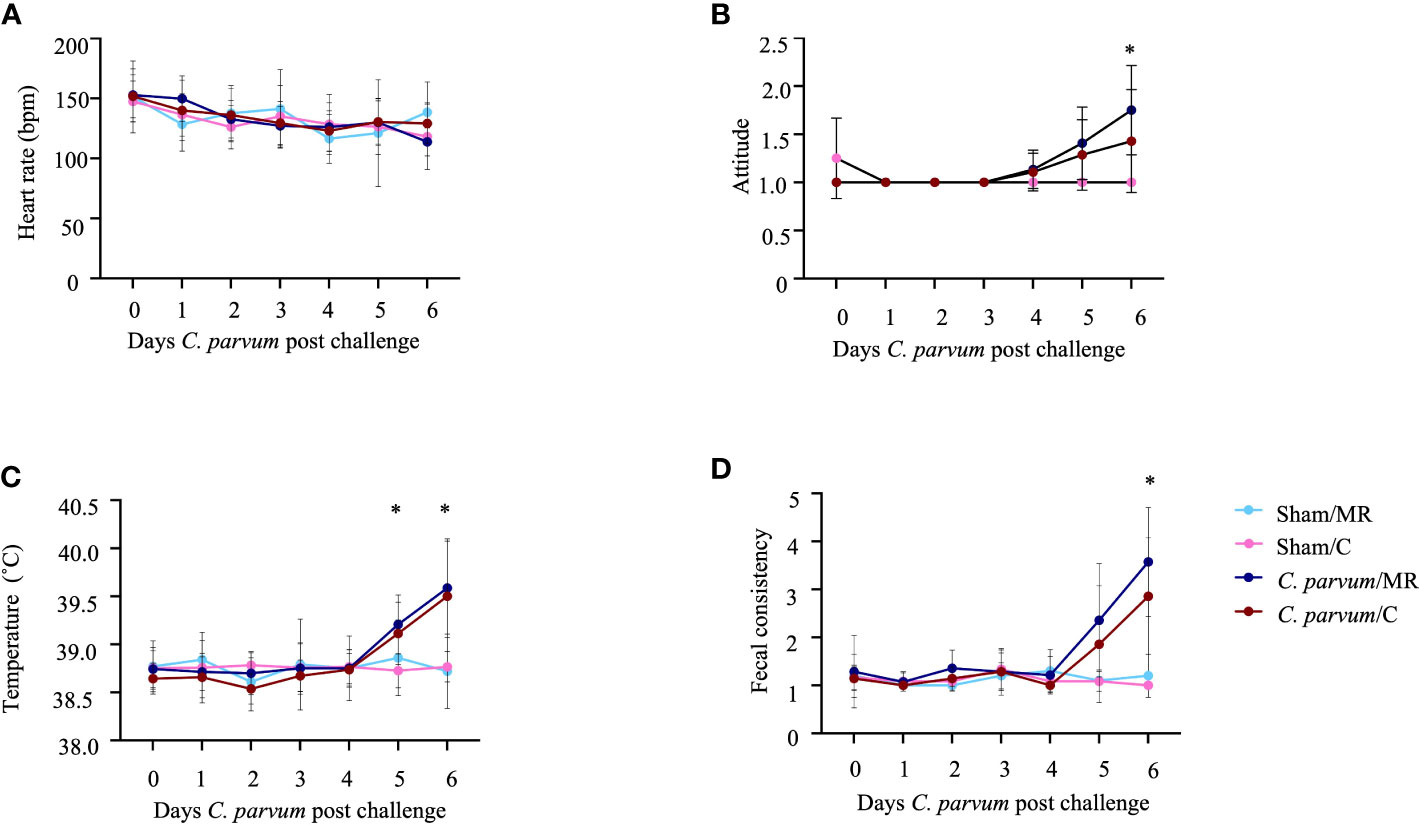
Figure 2 Clinical parameters in calves challenged with (C. parvum and supplemented with colostrum. (A) Heart rate. No significant differences among experimental groups. (B) Attitude score. Calves in the C parvum/MR group were significantly more depressed (P = 0.01) on 6 dpc when compared with Sham calves, whereas attitude scores of calves in the C. parvum/C group were not significantly different from Sham calves (P > 0.2). (C) Rectal temperature. Temperatures in the C. parvum/MR calves were significantly higher on 5 and 6 dpc (P = 0.02), compared with Sham calves, whereas there was no statistically significant difference between C. parvum/C calves and Sham calves (P = 0.07). Rectal temperatures of calves in the C.parvum/MR and C.parvum/C groups were similar 6 dpc (P = 0.9). (D) Fecal consistency. Average fecal scores on 5 and 6 dpc were significantly higher in C. parvum/MR and C. parvum/C calves (P < 0.005 and P < 0.05, respectively) compared with scores of calves in the Sham groups. Data are shown as mean ± SD. P < 0.05 (two-way ANOVA with post-hoc Tukey test for multiple comparisons) was considered significant.
None of the Sham calves met treatment criteria throughout the study period. Six and five of the seven calves in each of the C. parvum/MR and C. parvum/C groups required treatment with OES at least once, respectively. Calves in the C. parvum/MR group required significantly more OES treatments (P = 0.02) when compared with calves in the C. parvum/C group. Of the calves meeting treatment criteria, 10/12 were shedding C. parvum on the day of first treatment (C. parvum/MR: 6/6, C. parvum/C: 4/6). The first treatment occurred for one calf at 3 dpc (n = 1, C. parvum/C), for four calves at 4 dpc (n = 3, C. parvum/MR; n = 1, C. parvum/C), and six calves at 5 dpc (n = 3, C. parvum/MR; n = 3, C. parvum/C). From the total of 25 calves enrolled in this study, no calves had to be euthanized or excluded prior to the study end point at 6 dpc.
Regarding fecal shedding, no C. parvum DNA was detected on 2 dpc in C. parvum challenged groups but 4/7 C. parvum/MR calves (57%) and 1/7 (14%) C. parvum/C calves shed C. parvum at 4 dpc (P = 0.27, Table 3). At 6 dpc, the number of calves shedding C. parvum increased to 6/7 (86%) C. parvum/MR and 4/7 (57%) C. parvum/C calves (P = 0.56, Table 3). Both Sham/MR and Sham/C calves continued to have negative fecal C. parvum tests throughout the study.
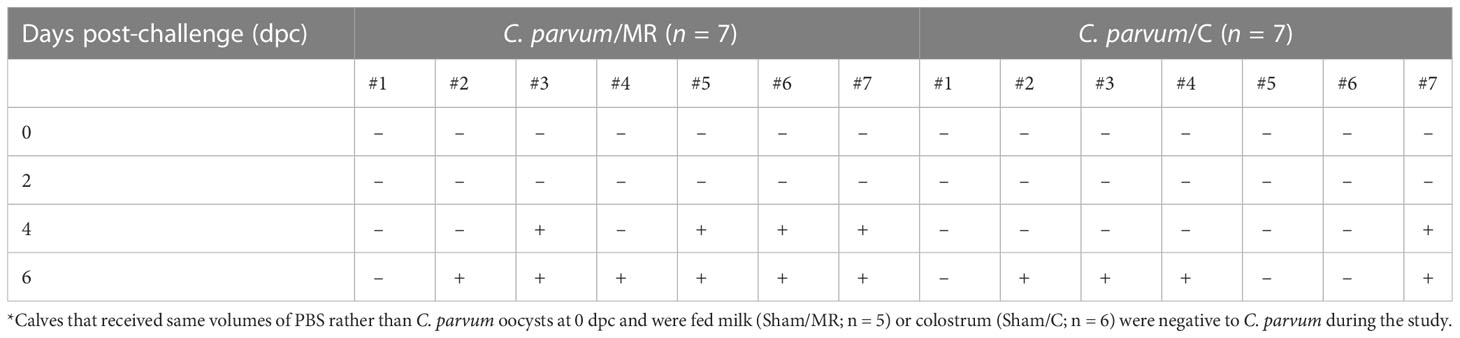
Table 3 Genomic detection (+ , present; - , not present) of C. parvum in feces of calves orally challenged with C. parvum oocysts (0-day post-challenge, dpc), which were fed milk replacer (C. parvum/MR) or colostrum (C. parvum/C).
Ileal innate immune and pathological response and proteomic signature
C. parvum challenged calves (C. parvum/MR and C. parvum/C) showed histological hallmarks of ileitis accompanied by villous atrophy and necrosis that was not ameliorated by feeding colostrum (Figure 3A). Ileitis in C. parvum/MR and C. parvum/C calves was manifested with severe grades of epithelial erosion, leukocyte infiltration in the lamina propria, and moderate mucosal hemorrhage (P < 0.05) (Figures 3B–D). The expression of pro-inflammatory cytokines (IL-8, IL-10, IFN-γ, and TNF-α mRNA expression) and cathelicidin 5 did not differ significantly in ilea of C. parvum challenged and unchallenged calves (Supplementary Figure S1A).
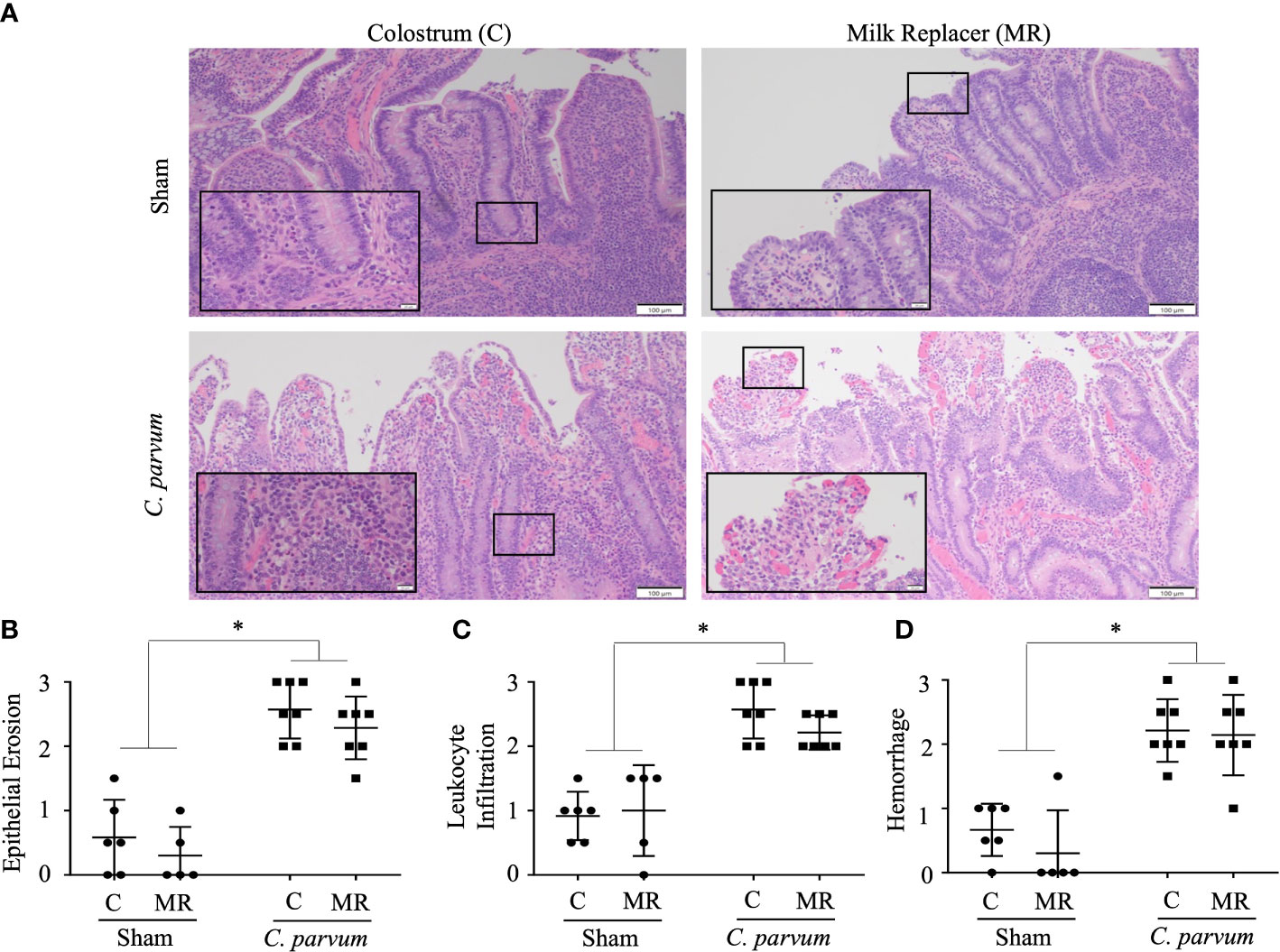
Figure 3 Grade of ileitis in newborn calves orally challenged by C. parvum and supplemented with milk replacer or colostrum. Sham and C. parvum (5 × 107 oocysts) challenged calves were fed with colostrum (Sham/C and C. parvum/C, respectively) or milk replacer (Sham/MR and C. parvum/MR, respectively) and terminated at 6 dpc. (A) Representative microphotographs of ilea (H&E staining) taken with a ZEISS AXIO microscope (20X, NA 0.5) and analyzed by ZEN 2.6 (2018) software. Severity of ileitis was blindly scored by the grade of (B) epithelial erosion, (C) leukocyte infiltration in the lamina propria, and (D) hemorrhage following a scale represented in Table 2. Data are shown as mean ± SEM. P < 0.05 (one-way ANOVA post-hoc Bonferroni correction for multiple group comparison or two-tailed Student’s t-test for two groups) was considered significant.
Quantitative global shotgun proteomic followed by pathway enrichment using Metascape (metascape.org) of the ilea (Figure 4A) identified 17 unique proteins that were upregulated and 14 proteins that were downregulated in Sham/C compared with Sham/MR ilea (Figure 4B and Supplementary Table S1). Those proteins in Sham/C ilea were related with two upregulated pathways: Rho GTPases and base excision repair, and one pathway downregulated: translation (Figure 4B and Supplementary Table S2 Sham/MR: Sham/C). Functional interactions between the DEPs as studied by STRING-db v11 (https://string-db.org) determined that four of the downregulated proteins in Sham/C (ATP-binding cassette subfamily F member 1 (ABCF1), glutamyl-prolyl-tRNA synthetase 1 (EPRS1), ribosomal protein L37a (RPL37A), and nuclear cap-binding protein subunit 1 (NCBP1), were clustered within a common translation pathway (Supplementary Figure S2A). Additionally, the upregulated expression of complement C1q-binding protein (C1QBP) showed to be related to both the upregulated and downregulated pathways in Sham/C ilea (Supplementary Table S2 Sham/MR: Sham/C).
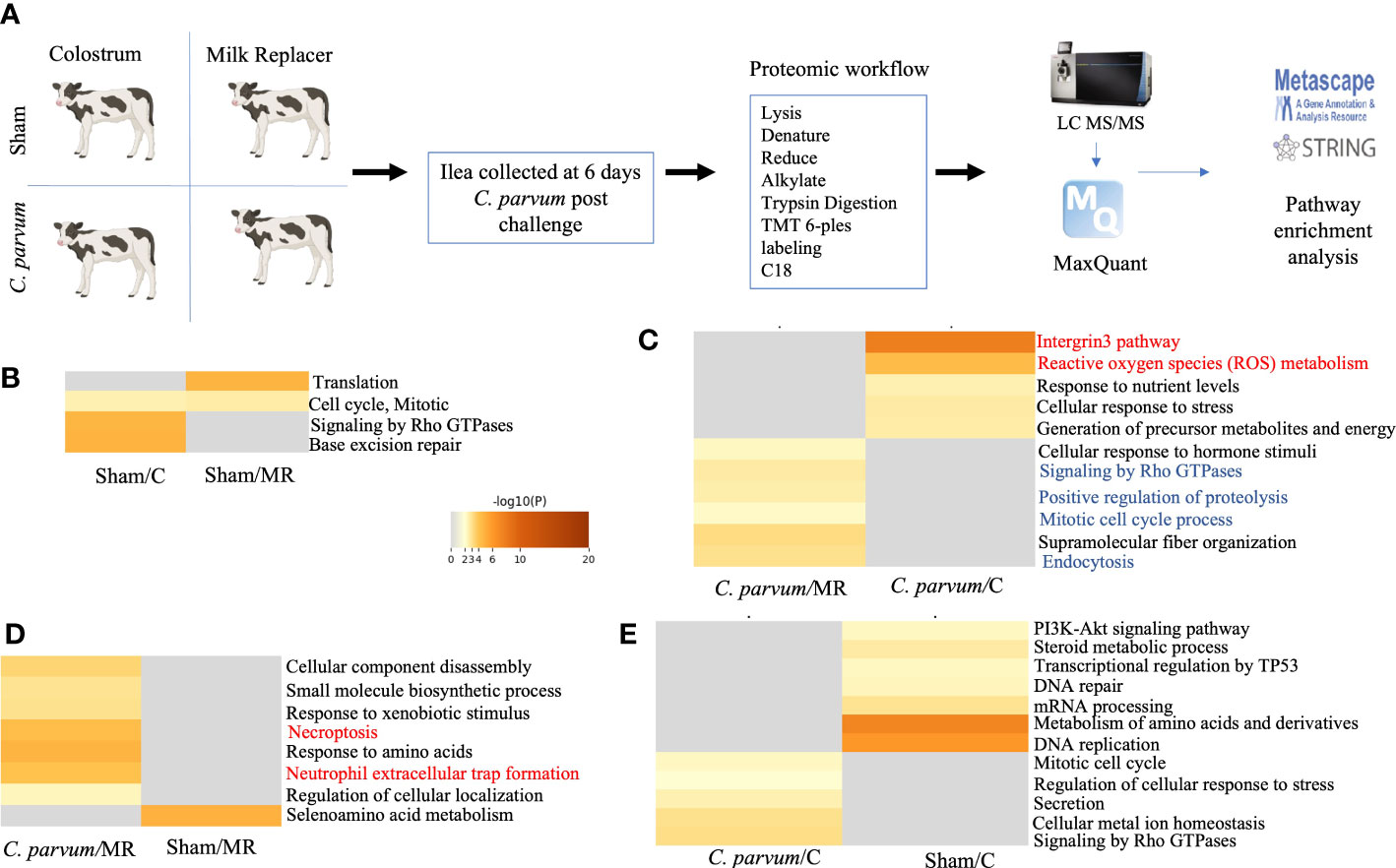
Figure 4 Proteomic immune-inflammatory signature in calves orally challenged by C. parvum and supplemented with milk replacer or colostrum. (A) Diagram of the proteomic workflow of different pathways in ilea from (B) Sham calves supplemented with colostrum or milk replacer (Sham/C vs. Sham/MR), (C) C. parvum challenged and supplemented with milk replacer or colostrum (C. parvum/MR vs. C. parvum/C, respectively), (D) C. parvum challenged or Sham supplemented with milk replacer C. parvum/MR vs. Sham/MR), and (E) C. parvum challenged or Sham supplemented with colostrum C. parvum/C vs. Sham/C). Ilea were collected at 6 dpc. Grade of red–yellow marks the level of upregulation.
The proteomic expression in ilea of calves challenged by C. parvum and supplemented with either colostrum or milk replacer were compared. C. parvum/C compared with C. parvum/MR ilea displayed 30 upregulated and 24 downregulated proteins (Figure 4C and Supplementary Table S1). GO functional enrichment analysis indicated that integrin 3 and ROS metabolic process pathways, including proteins such as lipocalin 2 (LCN), were enriched in C. parvum/C ilea (Figure 4C and Supplementary Table S2 C. parvum/C: C. parvum/MR). The downregulated pathways observed in C. parvum/C ilea included signaling by Rho GTPases, positive regulation of proteolysis, mitotic cell cycle process, and endocytosis (Figure 4C and Supplementary Table S2 C. parvum/C: C. parvum/MR).
Given significant differences in the proteomic profiles in ilea, from cytoskeleton rearrangement pathways in Sham calves to ROS inflammation in C. parvum challenged calves, the contribution of colostrum supplementation to those local immune responses was investigated. In the case of milk replacer only, C. parvum/MR ilea compared with Sham/MR counterparts showed 16 upregulated and 15 downregulated unique proteins and two upregulated pathways: necroptosis and neutrophil extracellular trap formation (Figure 4D and Supplementary Table S1). A protein–protein interaction network was mapped showing proteins in C. parvum/MR compared with Sham/MR ilea that were upregulated including neutrophil extracellular trap formation pathway with MPO, rac family small GTPase 2 (RAC2), and IQ motif containing GTPase-activating protein 2 (IQGAP2) functionally clustered together (Figure 4D; Supplementary Figure S2B, and Supplementary Table S2). Another noticeable cluster of upregulated proteins in C. parvum/MR ilea was composed of TOP2A, DNMT1, SUPT16H, and HIST1H2AJ proteins involved in cellular component disassembly and necroptosis (Figure 4D; Supplementary Figure S2B, and Supplementary Table S2 C. parvum/MR: Sham/MR). Among downregulated proteins in C. parvum/MR ilea, we found EPRS1, RPL23A, and RPS24, which clustered into seleno-acid metabolism pathways (Figure 4D; Supplementary Figure S2C, and Supplementary Table S2).
When colostrum supplementation was considered, C. parvum/C ilea showed 24 upregulated and 33 downregulated proteins compared with Sham/C, of which Rho GTPases and regulation of cellular response to stress pathways were upregulated in C. parvum/C ilea, but amino acid metabolism and DNA replication pathways were enriched in Sham/C ilea (Figure 4E and Supplementary Table S2 C. parvum/C: Sham/C). When addressing proteins specific for C. parvum, four unique upregulated and two downregulated C. parvum-derived proteins were identified in C. parvum/C ilea compared with C. parvum/MR (Supplementary Table S2 C. parvum/C: C. parvum/MR). These upregulated C. parvum proteins included proton-translocating NAD(P) (+) transhydrogenase and DNA helicase. Collectively, C. parvum induced grades of ileitis in young calves, whereas normal ilea expressed proteins related with cytoskeleton development and metabolism, ileitis provoked by C. parvum augmented proteins related with inflammation, neutrophil degranulation (ROS and MPO), and cellular necroptosis. The grade of ileitis was not ameliorated by colostrum supplementation or milk replacer. However, calves supplemented with colostrum sustained some proteins related with cytoskeleton dynamics compared with calves fed only milk replacer.
Colon innate immune and inflammatory responses
We next examined the colon, since this intestinal section can be colonized by C. parvum in severe immunosuppression or co-infections. For instance, while C. parvum typically infect the small intestine, C. parvum colonize the colons in gnobiotic pigs producing some degree of inflammation (Pereira et al., 2002). Likewise, C. parvum inhabit colons of neonatal mice co-infected with Plesiomonas shigelloides and C. parvum (Vitovec et al., 2001). We showed colons of calves challenged by C. parvum (C. parvum/MR and C. parvum/C) had areas of crypt hyperplasia along with dilated crypts and ulceration compared with Sham/C and Sham/MR colons, which displayed normal or minimally altered mucosa (Figure 5A). Colons from C. parvum/MR and C. parvum/C calves also showed similar severe epithelial erosion, infiltration of leukocytes in the lamina propria occasionally extending into the submucosa, and subepithelial extravasation of erythrocytes (Figures 5B–D). Transcription expression of IL-8, IL-10, IFN-γ, TNF-α, and cathelicidin 5 genes, on the other hand, did not show differences between C. parvum challenged and unchallenged colons (Supplementary Figure S1B).
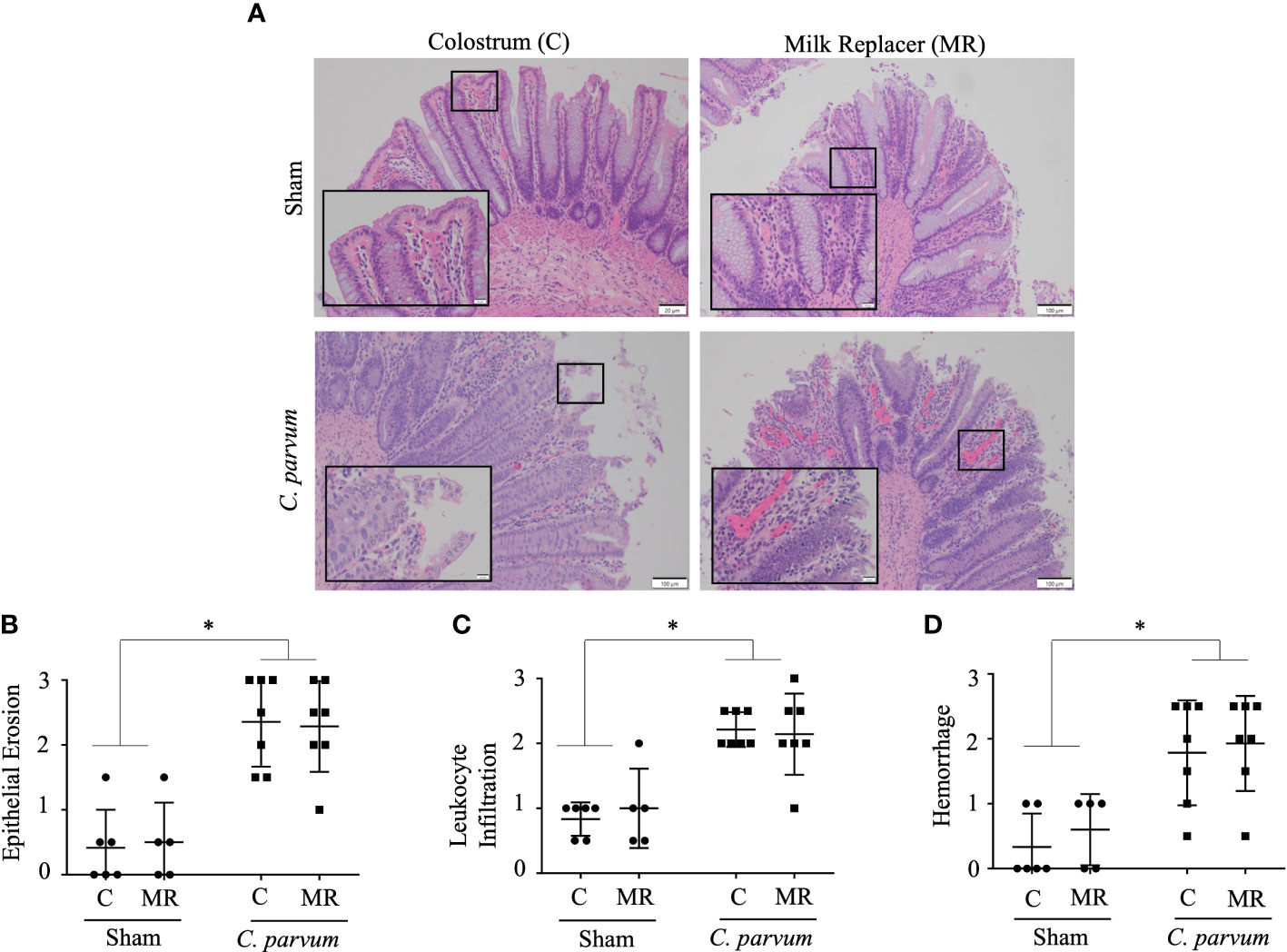
Figure 5 Grade of colitis in newborn calves orally challenged by C. parvum and supplemented with milk replacer or colostrum. Sham and C. parvum (5 × 107 oocysts) challenged calves were fed with colostrum (Sham/C and C. parvum/C, respectively) or milk replacer (Sham/MR and C. parvum/MR, respectively) and terminated at 6 dpc. (A) Representative microphotographs of colons (H&E staining) taken with a ZEISS AXIO microscope (20X, NA 0.5) and analyzed by ZEN 2.6 (2018) software. Severity of colitis was blindly scored by the grade of (B) epithelial erosion, (C) leukocyte infiltration in the lamina propria, and (D) hemorrhage following a scale represented in Table 2. Data are shown as mean ± SEM. P < 0.05 (one-way ANOVA post-hoc Bonferroni correction for multiple group comparison or two-tailed Student’s t-test for two groups) was considered significant.
C. parvum migrates during colonization through the intestinal mucin barrier, a gel forming glycoprotein-rich layer composed mostly of mucins, including MUC2 (Li et al., 2021). Thus, the parasitic effects on the colonic mucin barrier were evaluated by the terminal oligosaccharide α-2,3 and α-2,6 N-acetylneuraminic acids, also known as N-acetylglucosamine (α-D-GlcNAc and NeuNAc; sialic acid) and mucin-producing goblet cells. C. parvum challenged colons, regardless of colostrum supplementation (C. parvum/MR and C. parvum/C), exhibited very few and incompletely filled goblet cells, which are typically restricted to the crypt bases (Figure 6A). In contrast, the mucin barrier in colons from Sham calves (Sham/C and Sham/MR) showed many goblet cells, although the mucin layer was still discontinued in several areas (Figure 6A). Scoring the mucin layer denoted equally marked alterations in the mucin barrier in C. parvum/MR and C. parvum/C colons compared with Sham/C and Sham/MR counterparts (Figure 6B). When mucin terminal sialic acid residues were assessed by specific WGA lectins, Sham/C and Sham/MR colons showed a continuous thin layer of WGA+ goblet cells homogenously distributed along the surface of crypts (Figure 6C). Fewer WGA+ goblet cells restricted to the crypt bottom and lesser mucin content were detected in C. parvum/MR and C. parvum/C colons (Figure 6C). The amount of luminal mucus and WGA+ goblet cell number along the crypt (upper and lower thirds) did not reach significant differences among groups (Figures 6D–F; P > 0.05).
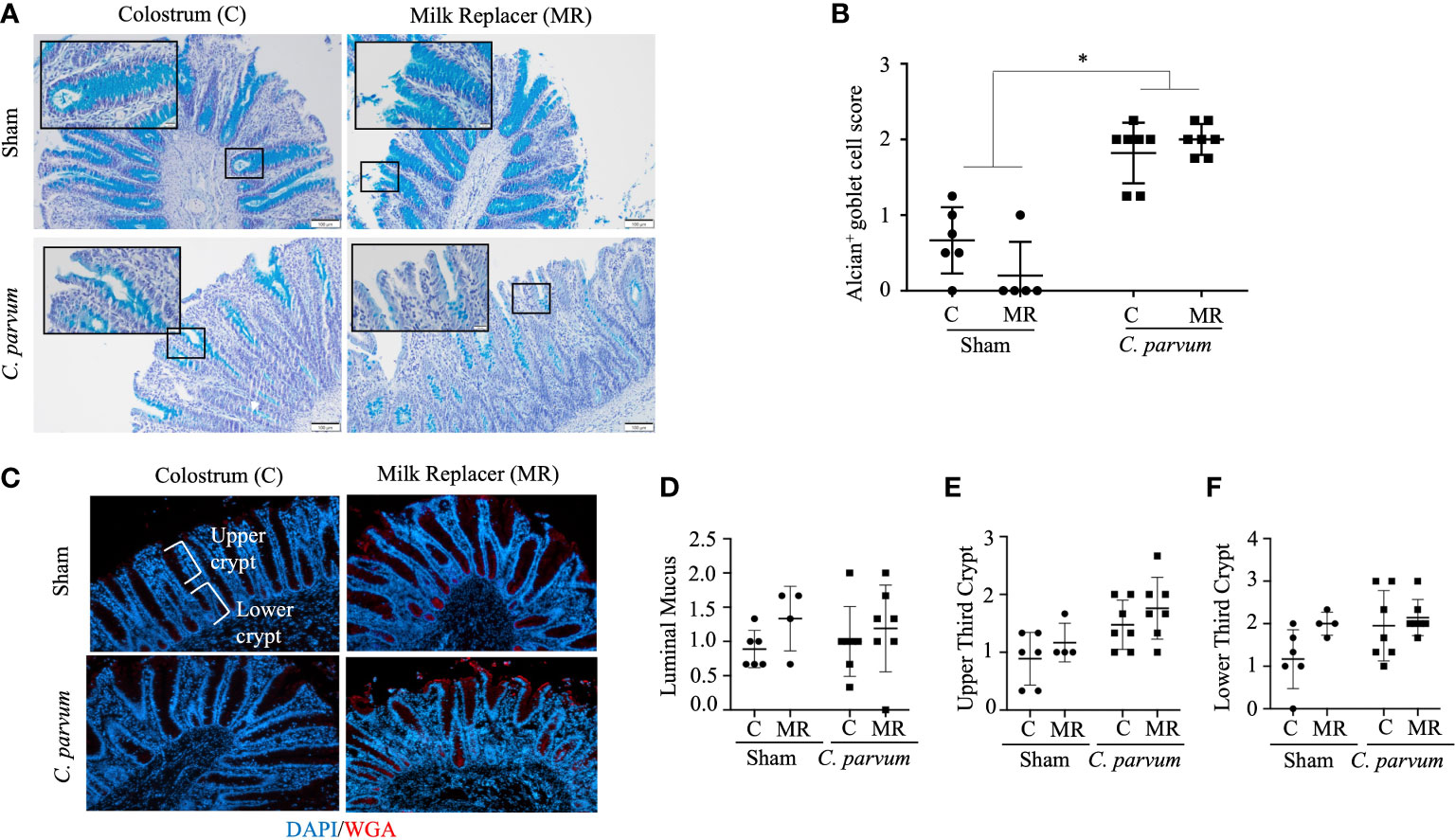
Figure 6 Mucin depletion in colons of calves orally challenged with C. parvum regardless of feeding regimen with milk replacer or colostrum. (A) Representative microphotographs of colonic goblet cells and (B) quantification of filled goblet cells per upper crypt (Alcian blue staining) in Sham and C. parvum (5 × 107 oocysts) challenged calves fed with colostrum (Sham/C and C. parvum/C, respectively) or milk replacer (Sham/MR and C. parvum/MR, respectively) and terminated at 6 dpc. (C) Representative microphotographs of colonic mucus stained with WGA+ (specific for sialic acid and N-acetylglucosamine) lectins, counterstained with DAPI. Scoring of the lectin blotting for (D) WGA+ as 0 = absence in crypts; 1 = presence restricted to crypt base; 2 = throughout crypt; 3 = throughout crypt and in lumen. The photographs were taken using a 20× magnification. Data are shown as mean ± SEM. P < 0.05 (one-way ANOVA post-hoc Bonferroni correction for multiple group comparison or two-tailed Student’s t-test for two groups) was considered significant.
Intestinal pathobiome
Genomic material from Bacillus sp. (n = 3), haemolytic E. coli (n = 7), Enterobacter cloacae (n = 1), Enterococcus spp. (n = 10), Klebsiella pneumoniae (n = 7), Lactobacillus spp. (n = 11), and Streptococcus spp. (n = 9) was detected in feces from all calves (n = 25) regardless of whether calves were challenged with C. parvum or supplemented with colostrum (P > 0.05, Table 4). All calves were PCR negative for E. coli K99, Salmonella spp., and bovine rota- and coronavirus.
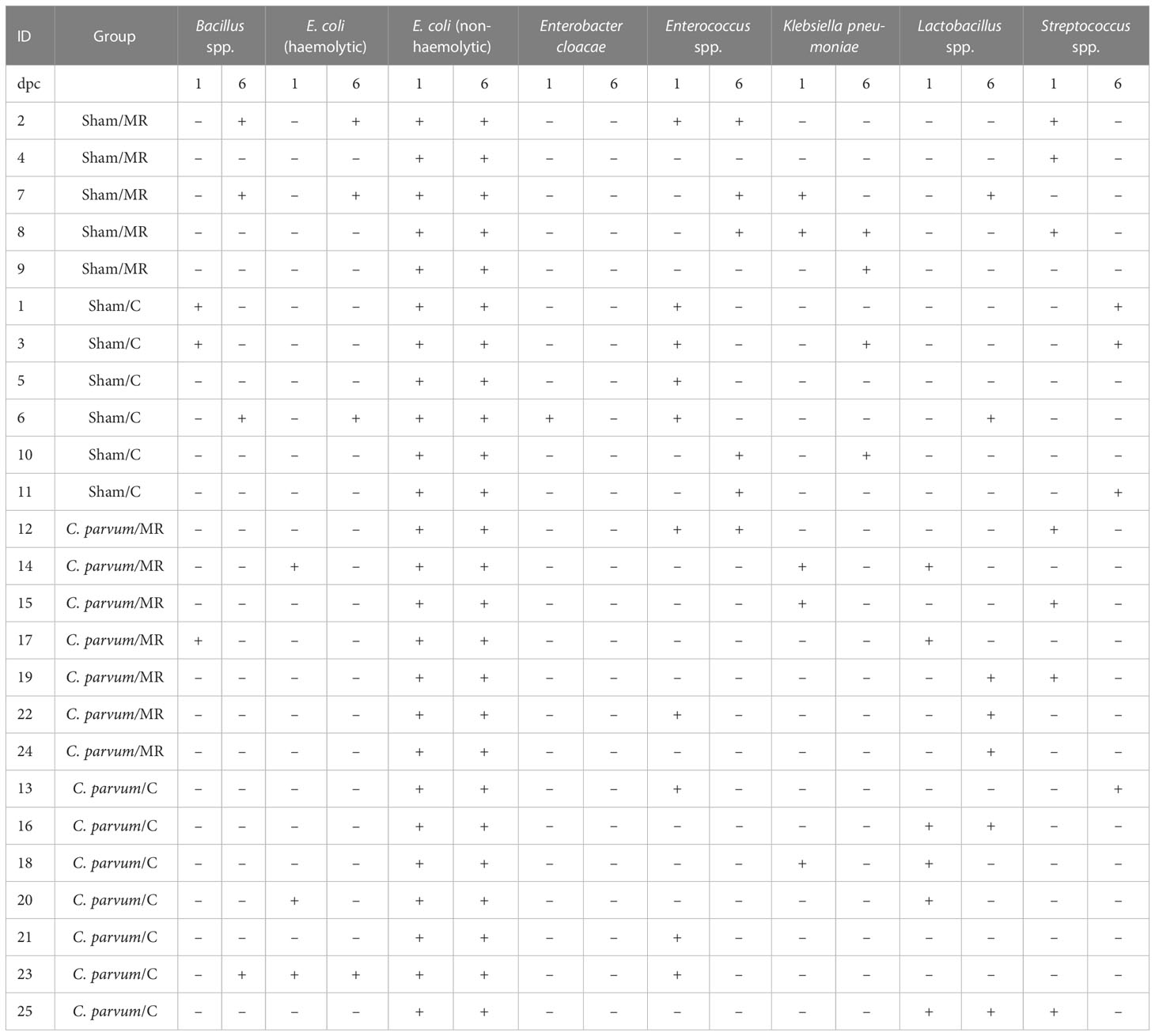
Table 4 Genomic presence (+ , present; - , not present) of aerobic bacteria in feces of Sham and C. parvum challenged calves (1- and 6-day post-challenge, dpc), which were fed with milk replacer (Sham/MR; n = 5; C. parvum/MR; n = 7) or colostrum (Sham/C; n = 6; C. parvum/C; n = 7).
By shotgun metagenomic sequencing assembled with previously reconstructed genomes (Teseo et al., 2022), and newly assembled MAGs with the data from this study, the entire microbial community and predicted virulence features were analyzed. From the metagenomic reads, 1,338 MAGs were mapped, and their abundance examined across fecal samples of 0 and 6 dpc. The alpha diversity differences were non-significant at 6 dpc (C. parvum vs. Sham P = 0.05; calf ID P = 0.157) (Figure 7A). In contrast, beta diversity ordination differed between C. parvum and Sham calf feces (Figure 7A) as demonstrated by different metrics (Bray Curtis vs. imputed, CLR and PCA; Supplementary Figure S3). Such beta diversity contrast was significant (P < 0.05), even though it explained only a small percent of the microbiome variation (R2 = 0.038) as most of it was driven by the natural variation between calves (R2 = 0.611).
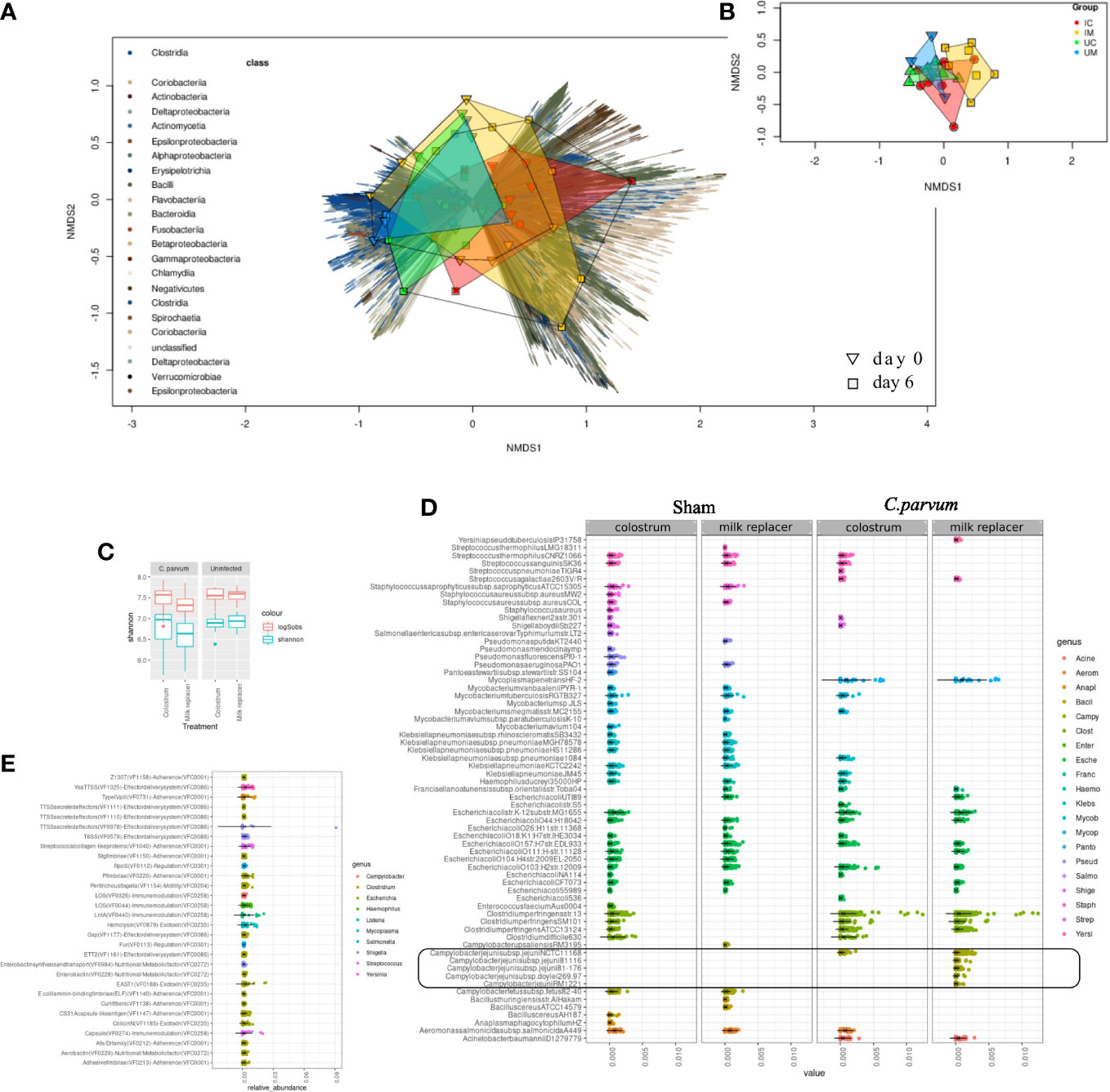
Figure 7 Distinct microbiome and pathobiome in newborn calves orally challenged by C. parvum and supplemented with milk replacer or colostrum. Microbiome (A, B) and pathobiome (C–E) changes were analyzed in feces from Sham and C. parvum (5 × 107 oocysts) challenged calves fed with colostrum (Sham/C and C. parvum/C, respectively) or milk replacer (Sham/MR and C. parvum/MR, respectively). (A) Non-metric Multidimensional Scaling (NMDS) ordination of Bray–Curtis dissimilarity of all feces collected at 0 (reverse triangle) and 6 (squares) dpc. Dissimilarity abundance matrix represents composition based on the abundance of a reference MAG catalog built for this study (see Methods). Vectors indicate the bacterial class of each MAG contig and are color coded (left). Samples are color coded (top left) based on feeding regimen*infection status and shapes connect samples of the same group. (B) A similar NMDS ordination using only fecal samples from 6 dpc (n = 25). (C) Alpha diversity (light green) and richness (red) of virulence features in the four groups (Sham/C, C. parvum/C, Sham/MR, and C. parvum/MR). Dots represent means. (D) Overview of the most abundant (top 100) predicted virulence features in all fecal samples from the groups (Sham/C, C. parvum/C, Sham/MR, and C. parvum/MR) after excluding the genus Aeromonas. (E) A differential representation analysis across the groups (Sham/C, C. parvum/C, Sham/MR, and C. parvum/MR) at 6 dpc (A–C). The features presented here are the ones that presented the sharpest contrasts between groups (indicspecies stat > 0.7, P < 0.05). Scatterplots show the abundance of the features in each sample. The rounded black square indicates the Campylobacter spp. features, which were exclusively present in C. parvum/MR calves.
C. parvum challenged calves showed a microbiome distinct from Sham calves but challenged calves that received colostrum displayed a microbiome profile more comparable with the one observed in Sham calves (Figure 7B). Shannon alpha diversity, pondering the predicted virulence features, differed in feces from C. parvum challenged and Sham calves (fdr corrected P = 0.027), while a decreased richness was also noticed using Sobs index (fdr corrected P < 0.01) (Figure 7C). Virulence feature diversity was not driven by the animal (calf ID; P > 0.05). Virulence factor Type IV pili related to an adherence factor (VF0476) was abundantly expressed. However, because VF0476 is commonly found in Aeromonas, a ubiquitous bacterium in animals and the environment (Pessoa et al., 2022), further downstream analyses were done after excluding it. This refined analysis showed matches with exotoxins, adherence factors, and secretion systems related to Campylobacter spp., Clostridium spp., Escherichia sp., Shigella spp., and Listeria spp. in C. parvum challenged calves (Figure 7D). Analysis of virulence features adjusted to 6 dpc between C. parvum challenged and Sham calves (equal number of samples with and without C. parvum sequences) showed most of the identified potential virulence features were reduced when C. parvum was present but increased alongside Clostridium spp. counts (Supplementary Figure S4). These virulence features included components related to the Type IV pili and two system components of Clostridium spp. (Supplementary Table S3). The combined effect of colostrum supplementation and C. parvum at 6 dpc revealed a unique increase of Campylobacter spp. in C. parvum/MR (Figure 7E). Taken together, the challenge with C. parvum in newborn calves induced a drastic shift on overall microbiome and pathobiome defined by virulence factors, in part contributed by Clostridium spp. overgrowth that was even more pronounced in calves fed with milk replacer.
Discussion
This study revealed gut innate immunological and microbiome signatures during cryptosporidiosis in neonatal calves and the effect of a daily regimen of colostrum supplementation on clinical and immune responses during the early establishment of C. parvum. The experimental model of C. parvum in neonates demonstrated that calves at this young age are extremely susceptible to cryptosporidiosis. Challenged calves experienced acute diarrhea and enterocolitis accompanied by depression and fever that required palliative oral treatment with electrolytes. Fever has been frequently reported in cryptosporidiosis, and we showed body temperature changed significantly in calves during the first week post-infection. The incubation time of 5 days for clinical signs agreed with data observed in 1-week-old calves experimentally challenged with C. parvum (Pohjola and Lindberg, 1986) and with the average 5–7 days observed in older calves (Abeywardena et al., 2015). Shedding of C. parvum after 4–6 dpc was expected based on other experimental studies reporting oocyte detection from 4 to 8 dpc (Pohjola and Lindberg, 1986). The high susceptibility to C. parvum in our calves resembles cryptosporidiosis in humans, where the disease is usually self-limiting in immunocompetent adult humans but is associated with severe diarrheal illness in children under 5 years old (Collaborators, 2017).
Colostrum has been proposed as therapeutic for unspecific diarrheas in pre-weaned calves (Carter et al., 2022), and colostrum administered with paromomycin in calves naturally infected with C. parvum impacted serum proteomes while reducing diarrhea and pathogen shedding in feces (Kacar et al., 2022). Our study deems that a prolonged colostrum supplementation instead of milk replacer, the usual diet for young calves in dairy production, did not categorically relieve diarrhea during the onset of cryptosporidiosis but did alleviate the concomitant fever and depression to some extent and was associated with lesser required OES treatments. This may potentially be attributed to the higher nutritional plane in calves supplemented with colostrum, which has previously been demonstrated to improve performance in calves infected with C. parvum (Ollivett et al., 2012). Of note, the limited sample size in this study could have precluded the finding of significant differences in fecal shedding among groups. Microscopically, challenged newborn calves, irrespective of feeding regimen, showed severe erosive and ulcerative ileitis, with accumulation of leukocytes in the lamina propria that extended to the colon. These lesions in colons of C. parvum challenged calves resembled those observed in murine ilea and colons inoculated with C. parvum oocysts (Al-Mathal and Alsalem, 2012), although those changes were not reported in previous experimentally challenged calves (Pohjola and Lindberg, 1986).
Because C. parvum oocyst encystation and colonization occur in the ileum (Al-Mathal and Alsalem, 2012), the local mucosal immunity during C. parvum infection in newborn calves was explored in this study. Alongside a leukocyte infiltration in the lamina propria of challenged calves, the proteomic data revealed C. parvum ileitis is characterized by an enriched ROS metabolic process pathway and the involvement of proteins associated with hypochlorous acid biosynthesis, lymphocyte aggregation, and thrombin-activated receptor signaling. Such mucosal alterations in the ilea of C.parvum challenged calves were not mitigated by colostrum supplementation. Neutrophil accumulation in the ileal mucosa alongside MPO activity was described in neonatal pigs mediated by the formation of superoxide (Zadrozny et al., 2006). The ilea of human patients with acquired immune deficiency syndrome (AIDS) also showed higher C. parvum infection with marked infiltration of neutrophils (Goodgame et al., 1995). However, the mucosal neutrophil influx would not be detrimental as neutrophils did not generate further peroxynitrite at mucosal surfaces and enhanced barrier integrity (Zadrozny et al., 2006). We also noted altered energy pathways in the ilea of C. parvum challenged calves in agreement with studies in the intestines of mice infected with C. parvum that showed depletion of glycolysis/citrate metabolite pathways (Karpe et al., 2021). Taken together, ileitis in C. parvum challenged neonates showed to course with active neutrophil inflammation and synthesis of ROS and other damaging inflammatory effectors.
Another consistent observation in C. parvum challenged calves was the depletion of the mucin barrier in the colon with extensive eroded surface areas, although sialic acid terminals were not particularly affected. The coating mucin conformed mostly by MUC2 secreted by goblet cells limits exposure to enteric pathogens (Bergstrom et al., 2010; Cobo et al., 2015; Cobo et al., 2017). Such lessened mucin barrier in challenged calves might have facilitated C. parvum colonization because bovine intestinal mucin and galactose-N-acetylgalactosamine (Gal/GalNAc) reduced C. parvum attachment to intestinal epithelia (Chen and LaRusso, 2000). Infection with E. coli O157:H7 in newborn calves also alters intestinal mucin barrier (Larzábal et al., 2020). Interestingly, even healthy control calves showed an impaired mucin layer in intestines, with fewer goblet cells restricted to crypt bottoms and thinner surfaces coated compared with adults. Butyrate and other short-chain fatty acids (SCFAs) contribute to mucus formation in the intestine during the first days of life (Liang et al., 2022), but butyrate-producing bacteria are not well established in the GI-tract of calves, as there is a transition from facultative anaerobes to anaerobic bacteria (Vlkova et al., 2006; Mayer et al., 2012). This could, at least in part, explain the difference in mucin between neonatal calves and slightly older calves, who show abundant mucin (Montagne et al., 2000a; Montagne et al., 2000b). Thus, the undeveloped mucin barrier in the gut this early in life infers a weakened constitutive defense with the conundrum of having to be settled during gut development but jeopardized by hyper mucin secretagogue effects of C. parvum. This would explain some of the susceptibility in neonates to cryptosporidiosis and other enteric diseases and highlights that dietary components could potentially promote intestinal mucin formation.
The general mechanisms of diarrheic enterocolitis implicate a disrupted intestinal epithelial integrity, mostly regulated by tight junction transmembrane proteins, that predisposes exaggerated amounts of flux escape, impaired water absorption, and microbial invasion (Walker et al., 2015; Liang et al., 2016). This study provides some evidence that C. parvum contributes to this “leaky gut syndrome” as ilea of healthy control calves displayed enriched proteins associated with actin and cytoskeleton dynamics, such as Rho GTPases and base excision repair pathways (Pradhan et al., 2021), that were lost during C. parvum infection. Disruptions in the intestinal architecture during C. parvum infection have been indeed observed in jejunums and ilea of mice which displayed upregulated actin, tubulin, and heat shock protein (Karpe et al., 2021). Additionally, C. parvum degrades occludin, claudin 4, and E-cadherin in murine enteroid-derived monolayer and intestines (Kumar et al., 2018). Pathogenic E. coli also disturbs genes associated with epithelial integrity in colons of newborn calves (He et al., 2022). Such overall rearrangements of the host cell cytoskeleton including aggregation and disassembly of actin would be key for the colonization of C. parvum (Yu et al., 2020) and other enteropathogens.
Enteric protozoa, including C. parvum, are known to promote shifts in microbial communities in infected animals. We showed C. parvum provoked a dysbiosis in neonatal calves, with higher occurrence of virulence genes attributed to exotoxins, adherence factors, and secretion systems from Clostridium spp. and other enteropathogens, including Campylobacter spp., Escherichia sp., Shigella spp., and Listeria spp. In agreement, calves naturally infected by C. parvum showed abundant Clostridium perfringens (Lucey et al., 2021), a bacterium associated with enterocolitis in suckling calves (Songer and Miskimmins, 2004). Additionally, the coexistence of C. parvum and Campylobacter spp. has been commonly reported in calves, and it would imply these enteropathogens synergize to rapidly colonize the gut of newborn calves and provoke diarrhea outbreaks (Grinberg et al., 2005). The documented co-occurrence of haemolytic E. coli (e.g., Shiga-like toxin producing E. coli) and C. parvum has been reported in calves associated with enteric infection outbreaks in children (Smith et al., 2004) and reinforce the role of cattle as reservoir of multiple enteric zoonotic pathogens. Cryptosporidiosis-induced dysbiosis has been previously reported in young mice (Mammeri et al., 2019) and calves (Ichikawa-Seki et al., 2019), which show an altered microbiota composition with increased Bacteroidetes and Fusobacteria, respectively. Similar gut dysbiosis was observed in C57BL/6J mice challenged by C. parvum, which displayed increased Faecalibaculum, Barnesiella, and Lactobacillus spp. in the small intestine and Ruminococcaceae in the caecum and colon (Karpe et al., 2021). This vulnerable microbiota shift in neonates seems to occur in other enteric diseases as well. A relative abundance of Escherichia sp. and Shigella spp. was noticed in colonic microbiota from newborn calves challenged by pathogenic E. coli (He et al., 2022) and in fecal microbiota of calves with naturally occurring diarrhea regardless of the pathogen (Gomez et al., 2017; Zeineldin et al., 2018; Jang et al., 2019; Gomez et al., 2022). Interestingly, we observed that microbiota from C. parvum challenged calves supplemented with colostrum could be more resilient compared with microbiota from calves fed only milk replacer. Colostrum ingestion is key immediately after birth, ensuring protective Bifidobacterium spp. and reducing opportunistic pathogenic E. coli and Shigella spp. in colons (Song et al., 2019). In addition, our finding supports that colostrum feeding may serve as a dietary intervention to regulate gut microbiota and improve gut health in neonatal calves (Malmuthuge et al., 2019). In fact, fresh colostrum reduced DNA copies of enteropathogens (C. perfringes, E. coli) in 7-day-old calves (Martin et al., 2021). Moreover, extended colostrum feeding for 3 days correlated with an abundance of mucosa-attached Lactobacillus spp. and E. coli in colons (Hromadkova et al., 2020). Our results support further studies to determine roles of colostrum to safeguard a healthy microbiome during enteric diseases in neonatal calves.
In summary, we described the damaging impact of C. parvum infection in neonatal calves, provoking severe diarrheic neutrophilic enterocolitis, perhaps augmented due to the lack of fully developed innate gut defenses at this young age. Colostrum supplementation (once per day after C. parvum challenge) showed limited effect mitigating diarrhea but had a positive effect on the associated, depression and required treatments in calves, as well as specific modulatory influence on host gut immune responses. Other colostrum formulations or regimes (e.g., doses and times) should be studied, exploring the abilities to regulate intestinal wellness further. For instance, cryptosporidiosis models with a lower infecting challenge could more accurately represent natural infections, given the marked susceptibility of newborn calves. Additionally, the use of colostrum beyond the first week of infection may better document the long-term impact of this management strategy on cryptosporidiosis diarrhea and associated clinical signs. Understanding of gut inflammatory and anti-parasite mechanisms during cryptosporidiosis and the testing of therapeutic alternatives remains critical due to the high mortality, severe outcomes, and zoonotic risk of C. parvum.
Data availability statement
The proteomic raw data is available in the ProteomeXchange database under project accession: PXD040269.
Ethics statement
The animal study was reviewed and approved by Canadian Guidelines for Animal Welfare (CGAW) and the University of Calgary Animal Care Committee (AC19-0051).
Author contributions
LG, SS, MC, and CW conducted the experiments in calves and acquired the clinical data. PL, DY, and AD contributed the proteomic assessment and analysis. KC and AH performed the histology and staining assessment and qPCR studies. PS, SO, and DG performed the microbiome analysis. LG, CW, and EC participated in conceiving the experiments and designed the experiments. LG, KC, and EC wrote the manuscript and prepared the figures. All authors contributed to the article and approved the submitted version.
Funding
This work was supported by an NSERC Discovery Grant (RGPAS-2017-507827), Alberta Government Major Innovation Fund (RCP-19-003-MIF), and Alberta Agriculture and Forestry (2018F050R, 2019F041R).
Acknowledgments
The authors thank Dr. Greg Muench and Greg Boorman from Veterinary Science Research Station for their assistance and guidance. during the studies with calves, the team of students who assisted extensively with data collection, particularly Benjamin Caddey and Cecilia Lucio Rodriguez. Deborah Schaefer (Research Specialist, School of Animal and Comparative Biomedical Sciences, University of Arizona) for providing resources and guidance manipulating C. parvum oocytes. Immunofluorescence studies were conducted in the Live Cell Imaging Facility, The Calvin, Phoebe and Joan Snyder Institute for Chronic Diseases, University of Calgary. Proteomic, liquid chromatography and mass spectrometry experiments were carried out by the Southern Alberta Mass Spectrometry (SAMS) core facility, University of Calgary.
Conflict of interest
The authors declare that the research was conducted in the absence of any commercial or financial relationships that could be construed as a potential conflict of interest.
Publisher’s note
All claims expressed in this article are solely those of the authors and do not necessarily represent those of their affiliated organizations, or those of the publisher, the editors and the reviewers. Any product that may be evaluated in this article, or claim that may be made by its manufacturer, is not guaranteed or endorsed by the publisher.
Supplementary material
The Supplementary Material for this article can be found online at: https://www.frontiersin.org/articles/10.3389/fcimb.2023.1165312/full#supplementary-material
References
Abeywardena, H., Jex, A. R., Gasser, R. B. (2015). A perspective on Cryptosporidium and Giardia, with an emphasis on bovines and recent epidemiological findings. Adv. Parasitol. 88, 243–301. doi: 10.1016/bs.apar.2015.02.001
Abuelo, A., Cullens, F., Hanes, A., Brester, J. L. (2021). Impact of 2 versus 1 colostrum meals on failure of transfer of passive immunity, pre-weaning morbidity and mortality, and performance of dairy calves in a large dairy herd. Anim. (Basel) 11(3), 782. doi: 10.3390/ani11030782
Al-Mathal, E. M., Alsalem, A. M. (2012). Pomegranate (Punica granatum) peel is effective in a murine model of experimental Cryptosporidium parvum. Exp. Parasitol. 131, 350–357. doi: 10.1016/j.exppara.2012.04.021
Armengol, R., Fraile, L. (2020). Feeding calves with pasteurized colostrum and milk has a positive long-term effect on their productive performance. Anim. (Basel) 10 (9), 1494. doi: 10.3390/ani10091494
Arslan, A., Duman, H., Kaplan, M., Uzkuc, H., Bayraktar, A., Erturk, M., et al. (2021). Determining total protein and bioactive protein concentrations in bovine colostrum. J. Vis. Exp 178. doi: 10.3791/63001
Askari, N., Shayan, P., Mokhber-Dezfouli, M. R., Ebrahimzadeh, E., Lotfollahzadeh, S., Rostami, A., et al. (2016). Evaluation of recombinant P23 protein as a vaccine for passive immunization of newborn calves against Cryptosporidium parvum. Parasite Immunol. 38, 282–289. doi: 10.1111/pim.12317
Belote, B. L., Tujimoto-Silva, A., Hummelgen, P. H., Sanches, A. W. D., Wammes, J. C. S., Hayashi, R. M., et al (2018). Histological parameters to evaluate intestinal health on broilers challenged with Eimeria and Clostridium perfringens with or without enramycin as growth promoter. Poult. Sci. 97, 2287–2294. doi: 10.3382/ps/pey064
Berge, A. C., Besser, T. E., Moore, D. A., Sischo, W. M. (2009). Evaluation of the effects of oral colostrum supplementation during the first fourteen days on the health and performance of preweaned calves. J. Dairy Sci. 92, 286–295. doi: 10.3168/jds.2008-1433
Bergstrom, K. S., Kissoon-Singh, V., Gibson, D. L., Ma, C., Montero, M., Sham, H. P., et al. (2010). Muc2 protects against lethal infectious colitis by disassociating pathogenic and commensal bacteria from the colonic mucosa. PloS Pathog. 6, e1000902. doi: 10.1371/journal.ppat.1000902
Bolger, A. M., Lohse, M., Usadel, B. (2014). Trimmomatic: a flexible trimmer for illumina sequence data. Bioinformatics 30, 2114–2120. doi: 10.1093/bioinformatics/btu170
Brainard, J., Hammer, C. C., Hunter, P. R., Katzer, F., Hurle, G., Tyler, K. (2021). Efficacy of halofuginone products to prevent or treat cryptosporidiosis in bovine calves: a systematic review and meta-analyses. Parasitology 148, 408–419. doi: 10.1017/S0031182020002267
Buret, A. G., Chin, A. C., Scott, K. G. (2003). Infection of human and bovine epithelial cells with Cryptosporidium andersoni induces apoptosis and disrupts tight junctional ZO-1: effects of epidermal growth factor. Int. J. Parasitol. 33, 1363–1371. doi: 10.1016/S0020-7519(03)00138-3
Bustin, S. A., Benes, V., Garson, J. A., Hellemans, J., Huggett, J., Kubista, M., et al. (2009). The MIQE guidelines: minimum information for publication of quantitative real-time PCR experiments. Clin. Chem. 55, 611–622. doi: 10.1373/clinchem.2008.112797
Carter, H. S. M., Steele, M. A., Costa, J. H. C., Renaud, D. L. (2022). Evaluating the effectiveness of colostrum as a therapy for diarrhea in preweaned calves. J. Dairy Sci. 105, 9982–9994. doi: 10.3168/jds.2022-22187
Chamorro, M. F., Cernicchiaro, N., Haines, D. M. (2017). Evaluation of the effects of colostrum replacer supplementation of the milk replacer ration on the occurrence of disease, antibiotic therapy, and performance of pre-weaned dairy calves. J. Dairy Sci. 100, 1378–1387. doi: 10.3168/jds.2016-11652
Chen, X., Gao, C., Li, H., Huang, L., Sun, Q., Dong, Y., et al. (2010). Identification and characterization of microRNAs in raw milk during different periods of lactation, commercial fluid, and powdered milk products. Cell Res. 20, 1128–1137. doi: 10.1038/cr.2010.80
Chen, X. M., LaRusso, N. F. (2000). Mechanisms of attachment and internalization of Cryptosporidium parvum to biliary and intestinal epithelial cells. Gastroenterology 118, 368–379. doi: 10.1016/S0016-5085(00)70219-8
Chigerwe, M., Hagey, J. V., Aly, S. S. (2015). Determination of neonatal serum immunoglobulin G concentrations associated with mortality during the first 4 months of life in dairy heifer calves. J. Dairy Res. 82, 400–406. doi: 10.1017/S0022029915000503
Clark, J. A., Gan, H., Samocha, A. J., Fox, A. C., Buchman, T. G., Coopersmith, C. M. (2009). Enterocyte-specific epidermal growth factor prevents barrier dysfunction and improves mortality in murine peritonitis. Am. J. Physiol. Gastrointest Liver Physiol. 297, G471–G479. doi: 10.1152/ajpgi.00012.2009
Clausen, P., Aarestrup, F. M., Lund, O. (2018). Rapid and precise alignment of raw reads against redundant databases with KMA. BMC Bioinf. 19, 307. doi: 10.1186/s12859-018-2336-6
Cobo, E. R., Kissoon-Singh, V., Moreau, F., Chadee, K. (2015). Colonic MUC2 mucin regulates the expression and antimicrobial activity of beta-defensin 2. Mucosal Immunol. 8, 1360–1372. doi: 10.1038/mi.2015.27
Cobo, E. R., Kissoon-Singh, V., Moreau, F., Holani, R., Chadee, K. (2017). MUC2 mucin and butyrate contribute to the synthesis of the antimicrobial peptide cathelicidin in response to Entamoeba histolytica- and dextran sodium sulfate-induced colitis. Infect. Immun. 85(3):e00905-16. doi: 10.1128/IAI.00905-16
Collaborators, G.B.D.D.D. (2017). Estimates of global, regional, and national morbidity, mortality, and aetiologies of diarrhoeal diseases: a systematic analysis for the global burden of disease study 2015. Lancet Infect. Dis. 17, 909–948. doi: 10.1016/S1473-3099(17)30276-1
Cox, J., Mann, M. (2008). MaxQuant enables high peptide identification rates, individualized p.p.b.-range mass accuracies and proteome-wide protein quantification. Nat. Biotechnol. 26 (12), 1367–1372. doi: 10.1038/nbt.1511
Cox, J., Neuhauser, N., Michalski, A., Scheltema, R. A., Olsen, J. V., Mann, M. (2011). Andromeda: a peptide search engine integrated into the MaxQuant environment. J. Proteome Res. 10 (4), 1794–805. doi: 10.1021/pr101065j
Dumaine, J. E., Tandel, J., Striepen, B. (2020). Cryptosporidium parvum. Trends Parasitol. 36, 485–486. doi: 10.1016/j.pt.2019.11.003
Erben, U., Loddenkemper, C., Doerfel, K., Spieckermann, S., Haller, D., Heimesaat, M. M., et al. (2014). A guide to histomorphological evaluation of intestinal inflammation in mouse models. Int. J. Clin. Exp. Pathol. 7, 4557–4576.
Gomez, D. E., Arroyo, L. G., Costa, M. C., Viel, L., Weese, J. S. (2017). Characterization of the fecal bacterial microbiota of healthy and diarrheic dairy calves. J. Vet. Intern. Med. 31, 928–939. doi: 10.1111/jvim.14695
Gomez, D. E., Li, L., Goetz, H., Macnicol, J., Gamsjaeger, L., Renaud, D. L. (2022). Calf diarrhea is associated with a shift from obligated to facultative anaerobes and expansion of lactate-producing bacteria. Front. Vet. Sci. 9, 846383. doi: 10.3389/fvets.2022.846383
Goodgame, R. W., Kimball, K., Ou, C. N., White, A. C., Jr., Genta, R. M., Lifschitz, C. H., et al. (1995). Intestinal function and injury in acquired immunodeficiency syndrome-related cryptosporidiosis. Gastroenterology 108, 1075–1082. doi: 10.1016/0016-5085(95)90205-8
Grinberg, A., Pomroy, W. E., Weston, J. F., Ayanegui-Alcerreca, A., Knight, D. (2005). The occurrence of Cryptosporidium parvum, Campylobacter and Salmonella in newborn dairy calves in the manawatu region of new Zealand. N Z Vet. J. 53, 315–320. doi: 10.1080/00480169.2005.36566
Guo, Y., Ryan, U., Feng, Y., Xiao, L. (2022). Emergence of zoonotic Cryptosporidium parvum in China. Trends Parasitol. 38, 335–343. doi: 10.1016/j.pt.2021.12.002
He, L., Wang, C., Simujide, H., Aricha, H., Zhang, J., Liu, B., et al. (2022). Effect of early pathogenic Escherichia coli infection on the intestinal barrier and immune function in newborn calves. Front. Cell Infect. Microbiol. 12, 818276. doi: 10.3389/fcimb.2022.818276
Hromadkova, J., Suzuki, Y., Pletts, S., Pyo, J., Ma, T., Chen, Y., et al. (2020). Effect of colostrum feeding strategies on the expression of neuroendocrine genes and active gut mucosa-attached bacterial populations in neonatal calves. J. Dairy Sci. 103, 8629–8642. doi: 10.3168/jds.2019-17710
Ichikawa-Seki, M., Motooka, D., Kinami, A., Murakoshi, F., Takahashi, Y., Aita, J., et al. (2019). Specific increase of Fusobacterium in the faecal microbiota of neonatal calves infected with Cryptosporidium parvum. Sci. Rep. 9, 12517. doi: 10.1038/s41598-019-48969-6
Isobe, N., Shibata, A., Kubota, H., Yoshimura, Y. (2013). Lingual antimicrobial peptide and lactoferrin concentrations and lactoperoxidase activity in bovine colostrum are associated with subsequent somatic cell count. Anim. Sci. J. 84, 751–756. doi: 10.1111/asj.12113
Jang, J. Y., Kim, S., Kwon, M. S., Lee, J., Yu, D. H., Song, R. H., et al. (2019). Rotavirus-mediated alteration of gut microbiota and its correlation with physiological characteristics in neonatal calves. J. Microbiol. 57, 113–121. doi: 10.1007/s12275-019-8549-1
Kacar, Y., Baykal, A. T., Aydin, L., Batmaz, H. (2022). Evaluation of the efficacy of cow colostrum in the treatment and its effect on serum proteomes in calves with cryptosporidiosis. Vet. Immunol. Immunopathol. 248, 110429. doi: 10.1016/j.vetimm.2022.110429
Karpe, A. V., Hutton, M. L., Mileto, S. J., James, M. L., Evans, C., Shah, R. M., et al. (2021). Cryptosporidiosis modulates the gut microbiome and metabolism in a murine infection model. Metabolites 11 (6), 380. doi: 10.3390/metabo11060380
Klein, P., Kleinova, T., Volek, Z., Simunek, J. (2008). Effect of Cryptosporidium parvum infection on the absorptive capacity and paracellular permeability of the small intestine in neonatal calves. Vet. Parasitol. 152, 53–59. doi: 10.1016/j.vetpar.2007.11.020
Kumar, A., Chatterjee, I., Anbazhagan, A. N., Jayawardena, D., Priyamvada, S., Alrefai, W. A., et al. (2018). Cryptosporidium parvum Disrupts intestinal epithelial barrier function via altering expression of key tight junction and adherens junction proteins. Cell Microbiol. 20, e12830. doi: 10.1111/cmi.12830
Langmead, B., Trapnell, C., Pop, M., Salzberg, S. L. (2009). Ultrafast and memory-efficient alignment of short DNA sequences to the human genome. Genome Biol. 10, R25. doi: 10.1186/gb-2009-10-3-r25
Larzábal, M., Da Silva, W. M., Multani, A., Vagnoni, L. E., Moore, D. P., Marin, M. S., et al. (2020). Early immune innate hallmarks and microbiome changes across the gut during Escherichia coli O157: H7 infection in cattle. Sci. Rep. 10, 21535. doi: 10.1038/s41598-020-78752-x
Lau, N. C., Lim, L. P., Weinstein, E. G., Bartel, D. P. (2001). An abundant class of tiny RNAs with probable regulatory roles in Caenorhabditis elegans. Science 294, 858–862. doi: 10.1126/science.1065062
Li, T., Liu, H., Jiang, N., Wang, Y., Wang, Y., Zhang, J., et al. (2021). Comparative proteomics reveals Cryptosporidium parvum manipulation of the host cell molecular expression and immune response. PloS Negl. Trop. Dis. 15, e0009949. doi: 10.1371/journal.pntd.0009949
Li, D., Liu, C. M., Luo, R., Sadakane, K., Lam, T. W. (2015). MEGAHIT: an ultra-fast single-node solution for large and complex metagenomics assembly via succinct de bruijn graph. Bioinformatics 31, 1674–1676. doi: 10.1093/bioinformatics/btv033
Liang, L., Liu, L., Zhou, W., Yang, C., Mai, G., Li, H., et al. (2022). Gut microbiota-derived butyrate regulates gut mucus barrier repair by activating the macrophage/WNT/ERK signaling pathway. Clin. Sci. (Lond) 136, 291–307. doi: 10.1042/CS20210778
Liang, G., Malmuthuge, N., Bao, H., Stothard, P., Griebel, P. J., Guan Le, L. (2016). Transcriptome analysis reveals regional and temporal differences in mucosal immune system development in the small intestine of neonatal calves. BMC Genomics 17, 602. doi: 10.1186/s12864-016-2957-y
Lindén, S. K., Florin, T. H., Mcguckin, M. A. (2008). Mucin dynamics in intestinal bacterial infection. PloS One 3, e3952. doi: 10.1371/journal.pone.0003952
Lucey, P. M., Lean, I. J., Aly, S. S., Golder, H. M., Block, E., Thompson, J. S., et al. (2021). Effects of mannan-oligosaccharide and Bacillus subtilis supplementation to preweaning Holstein dairy heifers on body weight gain, diarrhea, and shedding of fecal pathogens. J. Dairy Sci. 104, 4290–4302. doi: 10.3168/jds.2020-19425
Ma, T., Lin, W., Chen, Y., Cobo, E. R., Windeyer, C., Gamsjager, L., et al. (2022). Assessment of microRNA profiles in small extracellular vesicles isolated from bovine colostrum with different immunoblogulin G concentrations. J. Dairy Sci. Commun. 3, 328–333. doi: 10.3168/jdsc.2022-0225
Malmuthuge, N., Liang, G., Griebel, P. J., Guan, L. L. (2019). Taxonomic and functional compositions of the small intestinal microbiome in neonatal calves provide a framework for understanding early life gut health. Appl. Environ. Microbiol. 20, 172. doi: 10.1128/AEM.02534-18
Mammeri, M., Chevillot, A., Thomas, M., Julien, C., Auclair, E., Pollet, T., et al. (2019). Cryptosporidium parvum-infected neonatal mice show gut microbiota remodelling using high-throughput sequencing analysis: preliminary results. Acta Parasitol. 64, 268–275. doi: 10.2478/s11686-019-00044-w
Martin, C. C., De Oliveira, S., Costa, J., Baccili, C. C., Silva, B. T., Hurley, D. J., et al. (2021). Influence of feeding fresh colostrum from the dam or frozen colostrum from a pool on indicator gut microbes and the inflammatory response in neonatal calves. Res. Vet. Sci. 135, 355–365. doi: 10.1016/j.rvsc.2020.10.017
Martín-Gómez, S., Alvarez-Sánchez, M. A., Rojo-Vázquez, F. A. (2005). Oral administration of hyperimmune anti-Cryptosporidium parvum ovine colostral whey confers a high level of protection against cryptosporidiosis in newborn NMRI mice. J. Parasitol. 91, 674–678. doi: 10.1645/GE-3423
Mayer, M., Abenthum, A., Matthes, J. M., Kleeberger, D., Ege, M. J., Holzel, C., et al. (2012). Development and genetic influence of the rectal bacterial flora of newborn calves. Vet. Microbiol. 161, 179–185. doi: 10.1016/j.vetmic.2012.07.023
Miller, I. J., Rees, E. R., Ross, J., Miller, I., Baxa, J., Lopera, J., et al. (2019). Autometa: automated extraction of microbial genomes from individual shotgun metagenomes. Nucleic Acids Res. 47, e57. doi: 10.1093/nar/gkz148
Montagne, L., Toullec, R., Formal, M., Lalles, J. P. (2000a). Influence of dietary protein level and origin on the flow of mucin along the small intestine of the preruminant calf. J. Dairy Sci. 83, 2820–2828. doi: 10.3168/jds.S0022-0302(00)75181-2
Montagne, L., Toullec, R., Lalles, J. P. (2000b). Calf intestinal mucin: isolation, partial characterization, and measurement in ileal digesta with an enzyme-linked immunosorbent assay. J. Dairy Sci. 83, 507–517. doi: 10.3168/jds.S0022-0302(00)74910-1
Nayfach, S., Pollard, K. S. (2015). Average genome size estimation improves comparative metagenomics and sheds light on the functional ecology of the human microbiome. Genome Biol. 16, 51. doi: 10.1186/s13059-015-0611-7
Ollivett, T. L., Nydam, D. V., Linden, T. C., Bowman, D. D., Van Amburgh, M. E. (2012). Effect of nutritional plane on health and performance in dairy calves after experimental infection with Cryptosporidium parvum. J. Am. Vet. Med. Assoc. 241, 1514–1520. doi: 10.2460/javma.241.11.1514
Olm, M. R., Brown, C. T., Brooks, B., Banfield, J. F. (2017). dRep: a tool for fast and accurate genomic comparisons that enables improved genome recovery from metagenomes through de-replication. ISME J. 11, 2864–2868. doi: 10.1038/ismej.2017.126
Otte, J. M., Zdebik, A. E., Brand, S., Chromik, A. M., Strauss, S., Schmitz, F., et al. (2009). Effects of the cathelicidin LL-37 on intestinal epithelial barrier integrity. Regul. Pept. 156, 104–117. doi: 10.1016/j.regpep.2009.03.009
Parks, D. H., Imelfort, M., Skennerton, C. T., Hugenholtz, P., Tyson, G. W. (2015). CheckM: assessing the quality of microbial genomes recovered from isolates, single cells, and metagenomes. Genome Res. 25, 1043–1055. doi: 10.1101/gr.186072.114
Peeters, J. E., Villacorta, I., Vanopdenbosch, E., Vandergheynst, D., Naciri, M., Ares-Mazas, E., et al. (1992). Cryptosporidium parvum in calves: kinetics and immunoblot analysis of specific serum and local antibody responses (immunoglobulin a [IgA], IgG, and IgM) after natural and experimental infections. Infect. Immun. 60, 2309–2316. doi: 10.1128/iai.60.6.2309-2316.1992
Pereira, S. J., Ramirez, N. E., Xiao, L., Ward, L. A. (2002). Pathogenesis of human and bovine cryptosporidium parvum in gnotobiotic pigs. J. Infect. Dis. 186, 715–718. doi: 10.1086/342296
Perryman, L. E., Kapil, S. J., Jones, M. L., Hunt, E. L. (1999). Protection of calves against cryptosporidiosis with immune bovine colostrum induced by a cryptosporidium parvum recombinant protein. Vaccine 17, 2142–2149. doi: 10.1016/S0264-410X(98)00477-0
Pessoa, R. B. G., De Oliveira, W. F., Correia, M., Fontes, A., Coelho, L. (2022). Aeromonas and human health disorders: clinical approaches. Front. Microbiol. 13, 868890. doi: 10.3389/fmicb.2022.868890
Pohjola, S., Lindberg, L. A. (1986). Experimental cryptosporidiosis in mice, calves and chicken. Acta Vet. Scand. 27, 80–90. doi: 10.1186/BF03548561
Pradhan, R., Ngo, P. A., Martinez-Sanchez, L. D., Neurath, M. F., Lopez-Posadas, R. (2021). Rho GTPases as key molecular players within intestinal mucosa and GI diseases. Cells 10 (1), 66. doi: 10.3390/cells10010066
Pritchard, C. C., Cheng, H. H., Tewari, M. (2012). MicroRNA profiling: approaches and considerations. Nat. Rev. Genet. 13, 358–369. doi: 10.1038/nrg3198
Putz, E. J., Putz, A. M., Jeon, H., Lippolis, J. D., Ma, H., Reinhardt, T. A., et al. (2019). MicroRNA profiles of dry secretions through the first three weeks of the dry period from Holstein cows. Sci. Rep. 9, 19658. doi: 10.1038/s41598-019-56193-5
Riggs, M. W., Schaefer, D. A. (2020). Calf clinical model of cryptosporidiosis for efficacy evaluation of therapeutics. Methods Mol. Biol. 2052, 253–282. doi: 10.1007/978-1-4939-9748-0_15
Shannon, P., Markiel, A., Ozier, O., Baliga, N. S., Wang, J. T., Ramage, D., et al. (2003). Cytoscape: a software environment for integrated models of biomolecular interaction networks. Genome Res. 13, 2498–2504. doi: 10.1101/gr.1239303
Shaw, H. J., Innes, E. A., Morrison, L. J., Katzer, F., Wells, B. (2020). Long-term production effects of clinical cryptosporidiosis in neonatal calves. Int. J. Parasitol. 50, 371–376. doi: 10.1016/j.ijpara.2020.03.002
Smith, K. E., Stenzel, S. A., Bender, J. B., Wagstrom, E., Soderlund, D., Leano, F. T., et al. (2004). Outbreaks of enteric infections caused by multiple pathogens associated with calves at a farm day camp. Pediatr. Infect. Dis. J. 23, 1098–1104. doi: 10.1097/01.inf.0000145409.74116.e5
Song, Y., Malmuthuge, N., Li, F., Guan, L. L. (2019). Colostrum feeding shapes the hindgut microbiota of dairy calves during the first 12 h of life. FEMS Microbiol. Ecol. 95(1). doi: 10.1093/femsec/fiy203
Songer, J. G., Miskimmins, D. W. (2004). Clostridium perfringens Type e enteritis in calves: two cases and a brief review of the literature. Anaerobe 10, 239–242. doi: 10.1016/j.anaerobe.2004.05.001
Stelwagen, K., Carpenter, E., Haigh, B., Hodgkinson, A., Wheeler, T. T. (2009). Immune components of bovine colostrum and milk. J. Anim. Sci. 87, 3–9. doi: 10.2527/jas.2008-1377
Sun, H.-Z., Chen, Y., Guan, L. L. (2019). MicroRNA expression profiles across blood and different tissues in cattle. Sci. Data 6, 190013. doi: 10.1038/sdata.2019.13
Szklarczyk, D., Gable, A. L., Lyon, D., Junge, A., Wyder, S., Huerta-Cepas, J., et al. (2019). STRING v11: protein-protein association networks with increased coverage, supporting functional discovery in genome-wide experimental datasets. Nucleic Acids Res. 47, D607–D613. doi: 10.1093/nar/gky1131
Teseo, S., Otani, S., Brinch, C., Leroy, S., Ruiz, P., Desvaux, M., et al. (2022). A global phylogenomic and metabolic reconstruction of the large intestine bacterial community of domesticated cattle. Microbiome 10, 155. doi: 10.1186/s40168-022-01357-1
Thomson, S., Hamilton, C. A., Hope, J. C., Katzer, F., Mabbott, N. A., Morrison, L. J., et al. (2017). Bovine cryptosporidiosis: impact, host-parasite interaction and control strategies. Vet. Res. 48, 42. doi: 10.1186/s13567-017-0447-0
Vitovec, J., Aldova, E., Vladik, P., Krovacek, K. (2001). Enteropathogenicity of plesiomonas shigelloides and aeromonas spp. in experimental mono- and coinfection with cryptosporidium parvum in the intestine of neonatal BALB/c mice. Comp. Immunol. Microbiol. Infect. Dis. 24, 39–55. doi: 10.1016/S0147-9571(00)00012-6
Vlkova, E., Trojanova, I., Rada, V. (2006). Distribution of bifidobacteria in the gastrointestinal tract of calves. Folia Microbiol. (Praha) 51, 325–328. doi: 10.1007/BF02931825
Walker, M. P., Evock-Clover, C. M., Elsasser, T. H., Connor, E. E. (2015). Short communication: glucagon-like peptide-2 and coccidiosis alter tight junction gene expression in the gastrointestinal tract of dairy calves. J. Dairy Sci. 98, 3432–3437. doi: 10.3168/jds.2014-8919
Xiao, X., Xiong, A., Chen, X., Mao, X., Zhou, X. (2002). Epidermal growth factor concentrations in human milk, cow's milk and cow's milk-based infant formulas. Chin. Med. J. (Engl) 115, 451–454.
Yi, H., Zhang, L., Gan, Z., Xiong, H., Yu, C., Du, H., et al. (2016). High therapeutic efficacy of cathelicidin-WA against postweaning diarrhea via inhibiting inflammation and enhancing epithelial barrier in the intestine. Sci. Rep. 6, 25679. doi: 10.1038/srep25679
Yu, X., Guo, F., Mouneimne, R. B., Zhu, G. (2020). Cryptosporidium parvum Elongation factor 1alpha participates in the formation of base structure at the infection site during invasion. J. Infect. Dis. 221, 1816–1825. doi: 10.1093/infdis/jiz684
Zadrozny, L. M., Stauffer, S. H., Armstrong, M. U., Jones, S. L., Gookin, J. L. (2006). Neutrophils do not mediate the pathophysiological sequelae of Cryptosporidium parvum infection in neonatal piglets. Infect. Immun. 74, 5497–5505. doi: 10.1128/IAI.00153-06
Zeineldin, M., Aldridge, B., Lowe, J. (2018). Dysbiosis of the fecal microbiota in feedlot cattle with hemorrhagic diarrhea. Microb. Pathog. 115, 123–130. doi: 10.1016/j.micpath.2017.12.059
Keywords: Cryptosporidium parvum, cattle, enterocolitis, colostrum administration, microbiota
Citation: Gamsjäger L, Cirone KM, Schluessel S, Campsall M, Herik A, Lahiri P, Young D, Dufour A, Sapountzis P, Otani S, Gomez DE, Windeyer MC and Cobo ER (2023) Host innate immune responses and microbiome profile of neonatal calves challenged with Cryptosporidium parvum and the effect of bovine colostrum supplementation. Front. Cell. Infect. Microbiol. 13:1165312. doi: 10.3389/fcimb.2023.1165312
Received: 13 February 2023; Accepted: 05 April 2023;
Published: 03 May 2023.
Edited by:
Jiabo Ding, Institute of Animal Sciences (CAAS), ChinaReviewed by:
Gerardo Leotta, National Scientific and Technical Research Council (CONICET), ArgentinaRubén Darío Caffarena, National Institute for Agricultural Research (INIA), Uruguay
Copyright © 2023 Gamsjäger, Cirone, Schluessel, Campsall, Herik, Lahiri, Young, Dufour, Sapountzis, Otani, Gomez, Windeyer and Cobo. This is an open-access article distributed under the terms of the Creative Commons Attribution License (CC BY). The use, distribution or reproduction in other forums is permitted, provided the original author(s) and the copyright owner(s) are credited and that the original publication in this journal is cited, in accordance with accepted academic practice. No use, distribution or reproduction is permitted which does not comply with these terms.
*Correspondence: Eduardo R. Cobo, ZWNvYm9AdWNhbGdhcnkuY2E=
†Present address: Lisa Gamsjäger, Department of Population Health and Pathobiology, College of Veterinary Medicine, North Carolina State University, NC, Raleigh, United States