- Department of Microbiology and Immunology, Faculty of Pharmacy, October University for Modern Sciences and Arts (MSA), Giza, Egypt
Acinetobacter baumannii is a leading cause of biofilm-associated infections, particularly catheter-related bloodstream infections (CRBSIs) that are mostly recalcitrant to antimicrobial therapy. One approach to reducing the burden of CRBSIs is inhibiting biofilm formation on catheters. Owing to their prodigious microbial diversity, bacterial endophytes might be a valuable source of biosurfactants, which are known for their great capacity to disperse microbial biofilms. With this in mind, our study aimed to screen bacterial endophytes from plants growing on the banks of the River Nile for the production of powerful biosurfactants capable of reducing the ability of A. baumannii to form biofilms on central venous catheters (CVCs). This was tested on multidrug- and extensive drug-resistant (M/XDR) clinical isolates of A. baumannii that belong to high-risk global clones and on a standard strain of A. baumannii ATCC 19606. The drop collapse and oil dispersion assays were employed in screening the cell-free supernatants (CFS) of all endophytes for biosurfactant activity. Of the 44 bacterial endophytes recovered from 10 plants, the CFS of Bacillus amyloliquefaciens Cp24, isolated from Cyperus papyrus, showed the highest biosurfactant activity. The crude biosurfactant extract of Cp24 showed potent antibacterial activity with minimum inhibitory concentrations (MICs) ranging from 0.78 to 1.56 mg/ml. It also showed significant antibiofilm activity (p-value<0.01). Sub-MICs of the extract could reduce biofilm formation by up to 89.59%, while up to 87.3% of the preformed biofilms were eradicated by the MIC. A significant reduction in biofilm formation on CVCs impregnated with sub-MIC of the extract was demonstrated by CV assay and further confirmed by scanning electron microscopy. This was associated with three log10 reductions in adhered bacteria in the viable count assay. GC-MS analysis of the crude biosurfactant extract revealed the presence of several compounds, such as saturated, unsaturated, and epoxy fatty acids, cyclopeptides, and 3-Benzyl-hexahydro-pyrrolo [1, 2-a] pyrazine-1,4-dione, potentially implicated in the potent biosurfactant and antibiofilm activities. In the present study, we report the isolation of a B. amyloliquefaciens endophyte from the plant C. papyrus that produces a biosurfactant with potent antibiofilm activity against MDR/XDR global clones of A. baumannii. The impregnation of CVCs with the biosurfactant was demonstrated to reduce biofilms and, hence, proposed as a potential strategy for reducing CRBSIs.
1 Introduction
Acinetobacter baumannii (A. baumannii) has become a global threat in healthcare settings and a leading cause of healthcare-associated infections (Gedefie et al., 2021). This successful nosocomial pathogen is known for its adaptable genetic machinery that is capable to accumulate resistance genes and to acquire multidrug-, extensive drug-, and pan-drug-resistance phenotypes (Rolain et al., 2013; Wasfi et al., 2021; Zafer et al., 2021). In addition, it has remarkable environmental resilience partly due to its simple growth requirements and resistance to desiccation (Peleg et al., 2012).
One of the hallmark characteristics of A. baumannii is the propensity to form biofilms in which they live in surface-attached communities (Eze et al., 2018). Biofilm-embedded A. baumannii is properly shielded from antibiotics, immunity factors, and harsh environmental conditions. The close proximity within biofilms further enhances the acquisition of foreign genes through horizontal gene transfer. Hence, A. baumannii is a frequent cause of biofilm-related, particularly catheter-related bloodstream infections (CRBSI) and ventilator-associated pneumonia (VAP) (Gedefie et al., 2021). Such infections are mostly associated with devastating outcomes and are exceedingly resistant to a wide range of antimicrobial treatment modalities, posing a great challenge to infectious disease practitioners (Mansouri et al., 2013; Abd El-Rahman et al., 2023; Lafuente Cabrero et al., 2023).
Biofilm-related A. baumannii infections are mostly seen in intensive care units (ICU), where life support systems (e.g.; mechanical ventilation) and indwelling medical devices (e.g.; vascular and urinary catheters) are widely used (Lynch et al., 2017). A central venous catheter (CVC) insertion is the most common invasive procedure that leads to A. baumannii infections in ICUs (Castilho et al., 2017). Even using extreme aseptic techniques, the percutaneous insertion of catheters was found to permit the attachment of bacteria. This then progresses to biofilm formation and planktonic dispersion into the bloodstream, causing CRBSIs (Pathak et al., 2018). An estimated 250,000 bloodstream infections occur annually, and most are related to the presence of intravascular devices. In the United States, CRBSIs are still experienced by tens of thousands of patients annually, resulting in thousands of deaths and adding billions of dollars to the cost of the country’s healthcare system (Guenezan et al., 2018). The likelihood of developing CRBSI was found to be increased by chemotherapy, immunosuppression, and long-term catheterization (Lafuente Cabrero et al., 2023). Previous studies have reported biofilm formation capacity in up to 76% of CVC devices reported to have A. baumannii (Nahar et al., 2013; Sanchez et al., 2013; Duarte et al., 2016; Castilho et al., 2017). Consequently, researchers have been long looking for innovative ways to maintain CVCs biofilm-free.
Catheter coating or impregnation with various compounds that prevent biofilm formation and bacterial colonization is one of the approaches proposed by many authors to reduce the incidence of CRBSIs. While antimicrobial agents have been commonly used for coating or impregnation of catheters (Wang et al., 2018; Neethu et al., 2020; Sivanandan, 2020; Corrêa Carvalho et al., 2022), only partial clinical efficacy was shown by some antimicrobial-coated catheters, particularly against MDR pathogens (Mansouri et al., 2013). In addition, the use of antimicrobial-treated catheters might contribute to the evolution of antimicrobial resistance (Donlan, 2011). Hence, antibiofilm compounds represent good alternatives to antimicrobial agents, as they inhibit biofilms without exerting selection pressure on bacterial growth and thus reduce the development of antibiotic resistance (Amer et al., 2022, Wang et al., 2018; Neethu et al., 2020). Examples include catheter pretreatment with bacteriophages, surfactants, or enzymes (Donlan, 2011).
Biosurfactants are among the promising candidates for application in inhibiting bacterial biofilms (Banat et al., 2014). Biosurfactants, also named green surfactants, are surface active agents of biological origin. They are amphiphilic in nature, having hydrophilic and hydrophobic parts. They are non-toxic and biodegradable and do not accumulate in the environment. Microbial biosurfactants have been gaining much attention, owing to their chemical properties and stability under several environmental conditions (Eras-Muñoz et al., 2022). These properties make them relevant molecules for applications in combating many diseases and as potential therapeutic agents.
Endophytes are a class of endosymbiotic microorganisms that colonize plants and serve as stores for unique bioactive secondary metabolites, such as alkaloids, phenolic acids, quinones, steroids, saponins, tannins, terpenoid, and biosurfactants (Gouda et al., 2016; Ashitha et al., 2020; Marchut-Mikołajczyk et al., 2021). Endophytes can stimulate plant growth, facilitate the de novo synthesis of biologically active compounds, such as antibiotics, biosurfactants, and phytohormones, increase the host’s resistance to stressful environmental conditions, and increase the resistance of the host plant to pathogens and pests (Marchut-Mikołajczyk et al., 2021). Research into the biodiversity of endophytic strains for novel metabolites may lead to the discovery of new drugs, potentially contributing to the effective treatment of diseases in humans, plants, and animals (Ryan et al., 2008). Ongoing discoveries on the variety of metabolites produced by endophytes and their promising applications show that endophytes have inspired research in the development of biotechnological solutions. These solutions span from the exploration to the manufacture of industrially relevant metabolites that could help identify long-lasting sustainable solutions for the economic exploitation of biosurfactants that reduce biofilm formation, (Tidke et al., 2019).
Thus, the present study aimed to screen endophytes from Egypt for the ability to produce a powerful biosurfactant that can inhibit biofilms of MDR and XDR A. baumannii and to investigate the potential application of this biosurfactant in reducing biofilm formation on CVCs.
2 Materials and methods
2.1 Clinical strains and growth conditions
Five clinical isolates of multidrug- and extensive drug-resistant A. baumannii and a standard strain A. baumannii ATCC® 19606 were included in this study. The clinical isolates were recovered from different clinical specimens of patients admitted to Kasr Al-Ainy Hospital, which were collected in a previous study conducted by Hamed et al. (2022). As part of the previous study, the multilocus sequence typing (MLST) revealed that these isolates belong to high-risk global clones (GCs), as shown in Table 1. Bacterial cultures were routinely grown in Luria-Bertani (LB) medium at 37°C for 24 hours.

Table 1 MLSTs and GCs of the clinical isolates included in the current study (Hamed et al., 2022).
2.2 Collection of plants and isolation of endophytes
From April to November 2019, samples from different plants growing along the banks of the River Nile were randomly collected and screened for biosurfactant-producing bacterial endophytes. For endophyte isolation, fresh and healthy samples of each plant were collected, stored in sealed plastic bags, and delivered to the laboratory on the same day of collection for further processing. All samples were surface sterilized in the method described by Katoch et al. (2017), with some modifications. Briefly, the samples were thoroughly washed under tap water and distilled water, then dried using tissue paper. All washed plant materials were cut into 5 cm segments that were surface sterilized by sequential immersion in 70% ethanol (v/v) for 3 min, 1% sodium hypochlorite (v/v) for 12 minutes, and then 70% ethanol (v/v) for additional 30 sec. Finally, the segments were rinsed three times in sterile distilled water to remove residual sterilant and then left to dry under an airflow cabinet until complete drying. The plant segments were aseptically cut using sterile surgical scalpels into 2 mm-thick segments that were placed on trypticase soy agar (TSA) plates, ensuring direct contact of the cut edges with the culture media. The plates were incubated at 30°C for 5 days. To confirm the effectiveness of the surface sterilization process, 50 µl samples of the last distilled water rinse were cultured on TSA, incubated at the same conditions, and checked daily for the growth of surface contaminants. Morphologically distinct bacterial colonies growing under the plant parts were isolated to obtain pure colonies. Endophyte isolates were preserved in trypticase soy broth (TSB) supplemented with 25% (v/v) glycerol at -20°C.
2.3 Screening the endophytes for biosurfactant production
Endophytes were screened for biosurfactant production after the preparation of cell-free supernatant (CFS) using the methods described by Amer et al. (2021). The turbidity of an overnight culture of endophytes grown in TSB was adjusted to be equivalent to an OD600 of 1. The diluted culture was then used to inoculate fresh TSB to achieve a final dilution of 1:100. Flasks were then incubated at 30°C with shaking at 120 rpm for 4 days. CFS was prepared by centrifugation at 10,000 rpm for 10 min at 4°C, followed by filtration using a 0.22 µm filter (Millipore, Bedford, MA, USA). These were then screened for their biosurfactant activity using the drop collapse, oil displacement, and emulsification assays described below.
2.3.1 Drop collapse assay
The drop collapse assay was carried out as described by Patel et al. (2021). First, a drop (35 µl) of the CFS was placed on the surface of a parafilm. Biosurfactant production was indicated by the spreading or collapse of the drop within 15 min (Patel et al., 2021).
2.3.2 Oil displacement test
Isolates that showed positive drop collapse assay were further subjected to the oil displacement assay that was carried out following the procedure described by Joe et al. (2019). Briefly, 50 ml of distilled water was added to a Petri dish. This was overlaid by a thin layer of castor oil (100 µl). Approximately 10 µl of the CFS was added to the center of the oil layer. After 30 seconds, the oil surface was observed for the emergence of a clear zone. Uninoculated TSB and Triton X-100 were used as negative and positive controls, respectively.
The isolate with CFS causing the greatest displacement of the oil layer was selected for further testing.
2.3.3 Emulsification assay
The emulsification assay confirmed the biosurfactant production potential of the endophyte that showed the highest oil displacement activity. Following the method described by Satpute et al. (2010), equal parts of the CFS and castor oil were combined by vortexing for 2 minutes before being left to stand for 24 hours. The emulsification activity was indicated by the emulsification index (% EI24), which was calculated according to the following formula:
2.4 Molecular identification of the bacterial endophyte-producing biosurfactant
The bacterial endophyte showing the highest emulsification activity was identified by its microscopic morphology, followed by molecular analysis based on the 16S rRNA gene sequence. DNA was extracted using the GeneJET Genomic DNA Purification Kit (Thermo Fisher Scientific Inc., USA) as per the manufacturer’s instructions. The 16S rRNA gene was amplified by the universal pair of primers designed by Weisburg et al. (1991): 27F (5′AGAGTTTGATCCTGGCTCAG3′) and 1492R (5′CGGTTACCTTGTTACGACTT3′). The purified PCR product was then sequenced by Macrogen® (Seoul, South Korea) using ABI 3730xl DNA Analyzer. Gene sequences were compared to sequences in the National Center for Biotechnology Information (NCBI) database using the nucleotide Basic Local Alignment Search Tool (BLASTn). Gene sequences with high similarity to that determined in the study were retrieved and genetic diversity was analyzed using Molecular Evolutionary Genetics Analysis version 11.0 (MEGA 11). The phylogenetic tree was constructed by the maximum parsimony method (Tamura et al., 2013).
2.5 Preparation of the crude biosurfactant from the endophyte Cp24
For extraction of the crude biosurfactant, the CFS was prepared in the same way as in the preliminary screening for biosurfactant production. The CFS was acidified to pH 2.5 using HCl (5 N) and stored overnight at 4°C for precipitation of the biosurfactant compounds. The crude biosurfactant was then extracted twice from the acidified supernatant by shaking with double volumes of ethyl acetate (EtOAc) (Patel et al., 2021). The ethyl acetate extract was pooled and dried under a vacuum in a rotary flash evaporator (Heidolph, Germany) at 45°C. Based on the solubility, the dried extract was dissolved in 20% (v/v) Dimethylsulfoxide (DMSO) to obtain a stock solution of 50 mg/ml. The final concentrations of DMSO in all experiments were confirmed to not affect bacterial growth.
2.6 Assessment of the antibacterial activity of the crude biosurfactant against A. baumannii
The antibacterial activity of the crude biosurfactant extract against A. baumannii was evaluated using the broth microdilution assay. The minimum inhibitory concentrations (MICs) of the crude biosurfactant were determined according to the guidelines of the Clinical and Laboratory Standards Institute (CLSI) (CLSI, 2015). In 96-well microtiter plates, two-fold serial dilutions of the crude extract were prepared in Muller Hinton Broth (MHB; Oxoid, UK) to final concentrations ranging from 0.01 to 12.5 mg/ml. All wells were inoculated with approximately 5×105 CFU ml−1 of each test strain. After overnight incubation at 37°C, the MICs were visually recorded. Negative controls were prepared with MHB containing DMSO at the same concentrations as the extract.
2.7 Assessment of the antibiofilm activity of the crude biosurfactant against A. baumannii
2.7.1 Effect of the crude biosurfactant on bacterial adherence and biofilm formation
The ability of clinical strains to form biofilm was assayed using a crystal violet stain as described by Amer et al. (2021). Briefly, overnight cultures of A. baumannii strains adjusted to a count of 1.5 x 108 CFU/ml were diluted 1:50 in LB broth supplemented by 1% (w/v) glucose. Then 200 µl of the dilute cultures were inoculated into a 96-well flat-bottomed polystyrene microtiter plate (Greiner Bio One, Germany) The plates were incubated in static conditions for 24 h at 37°C. Following incubation, the planktonic microbial growth was then measured at a wavelength of 600 nm (ODgrowthPlanktonic) using an ELISA plate reader (Stat Fax®2100) Awareness Technology (Palm City, FA, USA). The wells were then washed three times with phosphate-buffered saline (PBS, pH=7.4) to remove unadhered or loosely adhered cells. After air drying, biofilms were stained with 0.1% (w/v) crystal violet (CV) solution for 15 min. The plates were then washed with water to rinse off the excess stain. The CV stain bound to the adherent cells was then solubilized by 33% glacial acetic acid and the biofilm biomass was quantified colorimetrically (ODCV Biofilm) at 570 nm. To reduce background signals, a blank containing an uninoculated medium was included and measured (ODgrowth Blank and ODCV Blank). The biofilm index (BFI) of each clinical strain was calculated using the following equation:
Isolates were classified into non-adherent, weak, moderate, and strong biofilm-forming isolates according to the semiquantitative classification of biofilm production as described by Naves et al. (2008). The effect of the crude biosurfactant on biofilm formation by A. baumannii was tested as described by Amer et al. (2021). A volume of 100 µl of the diluted culture was inoculated into a 96-well flat-bottomed polystyrene microtiter plate (Greiner Bio-one®, Germany) containing equal volumes of the crude biosurfactant at concentrations equivalent to the MIC, reaching a final concentration of 0.5X MIC. Negative controls containing DMSO at the same final concentrations as in the crude biosurfactant (control) were also included. Biofilm was stained by crystal violet and BFI was determined. The antibiofilm activity of the crude biosurfactant was expressed as percentage biofilm inhibition (%BI) that was calculated according to the following formula:
Where BFI (test) and BFI (control) are the BFIs of each strain in the presence and absence of the crude biosurfactant, respectively.
2.7.2 Effect of the crude biosurfactant on established biofilms
The efficacy of the crude biosurfactant in eradicating established biofilms was assessed using the method, with slight modifications, described by Lemos et al. (2018). First, 100 µl of overnight cultures of A. baumannii adjusted to 106 cells/ml in LBG were transferred to 96-well microtiter plates to form biofilms (Lemos et al., 2018). After overnight incubation in static conditions at 37°C, planktonic cells were delicately removed, and the wells were washed three times with PBS. The adherent cells remaining in the wells were then treated with 200 µl of the crude biosurfactant at concentrations equivalent to MIC. Wells treated with DMSO at the same final concentrations as in the crude biosurfactant served as control. The plates were incubated at 37°C for an additional 24 h, after which the supernatants were removed, and wells were washed three times using PBS. The residual biofilms were quantified using two methods, namely, crystal violet staining as described in section 2.7.1 and viable count assays as described by Ziemyte et al. (2020). Using the crystal violet staining method, the biofilm eradication percentage was calculated using the following formula (Patel et al, 2021):
Where ODCV Control is the absorbance reading of control; ODCV Test is the absorbance reading of biosurfactant treated biofilm.
To determine the number of viable biofilm-embedded bacteria after treatment with the crude biosurfactant, the wells were filled with 200 µl PBS and adherent cells were detached by sonication for 5 min. The viable count was determined using the drop plate method as described by Herigstad et al. (2001). Tenfold serial dilutions were prepared and 10 µl were plated onto MacConkey agar plates in triplicates, and incubated at 37°C for 24 h. The biofilm eradication percentage was expressed as a log10 reduction of the viable count in the biofilms treated by the crude biosurfactant compared to the negative control (Amer et al., 2022).
2.8 Evaluation of the antibiofilm effect of crude biosurfactant on central venous catheters (CVCs) in vitro
The catheter model was performed with a triple-lumen polyurethane CVC (Amecath® Ref. No. CTLC-0720-KGSN, Ameco Medical Industries, Egypt). In this model, the biosurfactant-impregnated CVC was challenged by the A. baumannii strain (M02) that showed the highest capacity for biofilm formation.
2.8.1 Preparation of the biosurfactant-impregnated CVC
The CVC was divided into 1-cm-long segments. Each was impregnated with the crude biosurfactant at a final concentration of 0.5X MIC (0.78 mg/ml) and kept at room temperature for 24 h (Amer et al., 2022). The catheters were then air-dried to restore their original size. Control segments were impregnated with DMSO at the same concentration used in the test.
2.8.2 Antibiofilm assay
The inhibitory effect of the crude biosurfactant on the ability of A. baumannii M02 (strong biofilm-forming isolate) to form biofilm on the CVC was evaluated according to the method, with slight modifications, described by Raad et al. (2012). First, the biosurfactant-impregnated and the control segments of the CVC were conditioned by plasma from a human volunteer (one of the authors). For this purpose, all CVC segments were placed in a sterile 12-well culture plate containing 2 ml of human plasma and incubated for 24 h at 37°C. The plasma was then replaced by LBG inoculated by ~1X106 CFU/ml of the test strain and the plate was incubated at 37°C for an additional 24 h. After incubation, the catheter sections were gently washed with sterile PBS to remove the non-adhered planktonic cells. The biofilms formed on the control and the biosurfactant-impregnated CVC segments were quantified by crystal violet staining and the viable count assay as described by Amer et al. (2022). For a detachment of the biofilm-embedded cells to determine the remaining viable bacteria on the catheter surface, CVC segments were sonicated in 1 ml PBS for 15 min, followed by 5 min vortexing. Aliquots of PBS were used for viable bacterial counting.
2.8.3 Scanning electron microscope analysis of biofilms on CVC
The efficacy of the CVC biosurfactant-impregnation against A. baumannii M02 biofilm was further confirmed through scanning electron microscope (SEM) analysis. Biofilms of A. baumannii M02 were allowed to develop on the biosurfactant-impregnated and the control CVC segments, as described in the antibiofilm assay. The segments were washed with PBS and then fixed using 2.5% glutaraldehyde. This was followed by gradual dehydration using increasing concentrations of 20, 40, 60, 80, and 100% ethanol. The dehydrated samples were plated by gold sputter for examination under SEM (Quanta™ 250 FEG, Thermo Fischer Scientific; New Hampshire, USA) (Yassin et al., 2019).
2.9 Cytotoxicity assay of the biosurfactant crude extract
The cytotoxicity assay of the crude biosurfactant extract was adapted from the ISO 10993-5 protocol. The assay was carried out using healthy human skin fibroblast cells obtained from Nawah Scientific Inc. (ATCC CCL-75) (Hou et al., 2020; Ivanova et al., 2021).
The biosurfactant crude extract was embedded in serum-free Dulbecco’s Modified Eagle Medium (DMEM) overnight at 37°C. At the same time, the human fibroblast cell line was cultured in DMEM supplemented with 10% v/v fetal bovine serum (FBS) and 1% w/v penicillin−streptomycin for 24 h at 37°C, 5% v/v CO2.
After achieving the confluence, 100 µl of the cell suspension (5X103 cells/ml) was seeded in each well of the 96-well plate. Then, the cells were incubated overnight in a humidified atmosphere (>90% humidity) with 5% CO2 at 37°C. Cells were treated with another aliquot of 100 μl media containing biosurfactant extract at various concentrations. After 72 h of treatment, cells were fixed by replacing media with 150 μl of 10% trichloroacetic acid (TCA) and incubated at 4°C for 1 h. The TCA solution was removed, and the cells were washed 5 times with PBS. The viability of the cells was evaluated using sulforhodamine B (SRB) (0.4% w/v) assay as described by Allam et al. (2018), and color intensity was measured at wavelength 540 nm. The percentage of cell viability was calculated using the following formula: Viability (%) =
2.10 Ex vivo hemolysis assay of the biosurfactant crude extract
The ex vivo hemolysis assay was conducted according to the method, with some modifications, described by Zhou et al. (2017). Briefly, freshly collected human red blood cells (RBCs) were centrifuged for 10 min at 2500 rpm, then washed three times and diluted to a final concentration of 5% v/v in sterile PBS (pH 7.4). Afterward, 500 µl of the diluted RBCs were added to 500 µl of crude biosurfactant extract at a final concentration of 0.5X MIC (0.78 mg/ml). Triton X-100 (0.1% in PBS), which can lyse RBCs completely, was used as the positive control while PBS buffer was used as the negative control. Shaking and incubation of samples at 37°C for 1 h was done and then samples were centrifuged again at 2500 rpm for 15 min. The supernatant was collected and transferred to wells of a microtiter plate, and its optical density was recorded by an ELISA plate reader at 545 nm. Sterile PBS (pH 7.4) was used as a blank. The same test was repeated with the addition of biosurfactant and DMSO-impregnated catheter to diluted RBCs. The hemolysis percentage was estimated using the following equation:
where As, An, and Ap are the absorbances of the sample, negative, and positive controls, respectively. The assay was calculated as the mean ± standard deviation of three replicates.
2.11 Gas chromatography-mass spectrophotometry analysis
Five milligrams of the extract dry weight was mixed with 120 µl silylating agent (N,O- Bis (tert-butyl dimethyl silyl) acetamide and incubated at 60°C for 30 minutes and analyzed by gas chromatography. The GC apparatus (Shimadzu®, Kyoto, Japan) coupled with a QP2010 Rtx-5MS was used to identify the structural analog of the crude biosurfactant. Helium was employed as a carrier gas, and a total of 10 µl of the sample was added to the apparatus. The runtime was 45 minutes with a flow rate of 1.24 ml/min. The oven was maintained at a temperature ranging from 60 to 260°C. The data were processed by matching the mass spectra and retention indices of peaks with references to retention index and mass spectra from the National Institute of Standards and Technology (NIST) library.
2.12 Statistical analysis
Statistical analysis was performed using GraphPad Prism 8.0.0 for Windows (GraphPad Software Inc., CA, USA). Independent samples t-test and two-way ANOVA (analysis of variance) were employed to analyze the statistical differences between crude biosurfactant and DMSO-treated cultures, where p<0.05 was considered to be statistically significant.
3 Results
3.1 Screening for biosurfactant-producing endophytic bacteria
In all, 10 plant samples were collected throughout the study period from different locations along the banks of the River Nile. A total of 44 bacterial endophytes were isolated from these plants. The plant species, their sites of collection, and the morphology of the isolates recovered from each plant are listed in Supplementary Table 1. CFSs of all endophytes were screened for their biosurfactant activity via different qualitative and quantitative assays. Of all endophytes that showed a positive drop collapse test, the CFS of an endophyte coded “Cp24” showed the highest biosurfactant activity. It produced a clear zone of 74 mm diameter in the oil dispersion assay and an %EI24 of 60 ± 2.5. Cp24 was isolated from the leaf of Cyperus papyrus collected from the Pharaonic Village in Cairo (29.9973° N, 31.2148° E). The growth of Cp24 from the leaf fragments of C. papyrus on TSA plates is shown in Figure 1.
3.2 Identification of endophytic isolate-producing biosurfactant
The endophytic isolate Cp24 grew on TSA plates as rough, opaque, fuzzy yellow to slightly orange colonies with jagged edges. Under a light microscope, it appeared as Gram-positive rods arranged in chains. The nucleotide sequence of the 16S rRNA gene carried by Cp24 showed the highest similarity to the corresponding gene of strain B. amyloliquefaciens HY-5 (GenBank accession: KY886133.1; percent similarity: 97.68%). A phylogenetic tree based on the 16S rRNA sequence of Cp24 and highly similar strains retrieved from the NCBI database showed that Cp24 was clustered with the same strain (Figure 2). Accordingly, Cp24 was tentatively identified as B. amyloliquefaciens.
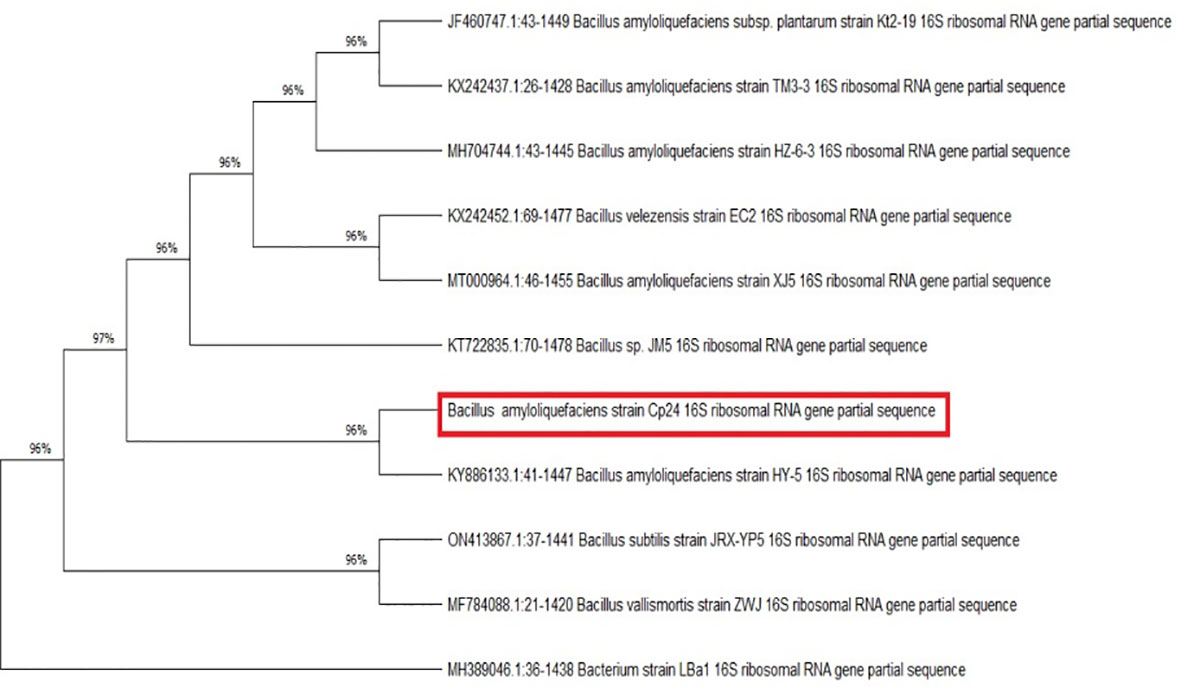
Figure 2 Evolutionary relationships between B. amyloliquefaciens Cp24 and highly similar strains. The phylogenetic tree was constructed by the maximum parsimony method (1000 replicates).
3.3 Antibacterial activity of the crude biosurfactant against A. baumannii isolates
The crude biosurfactant extract of Cp24 (50 mg/ml) displayed considerable antibacterial activity against four MDR and XDR A. baumannii isolates in addition to the standard strain (ATCC® 19606). The MIC values of the crude biosurfactant in the tested strains ranged from 0.78 to 1.56 mg/ml, as shown in Table 2.
3.4 Sub-MIC concentration of crude biosurfactant extract significantly inhibits A. baumannii biofilm formation
All tested A. baumannii isolates and the standard strain displayed strong biofilm-forming capacity, with BFI ranging from 1.25 to 2.2. The antibiofilm potential of B. amyloliquefaciens Cp24 crude biosurfactant was determined by its ability to impair the biofilm formation of the tested strains and inhibit their adhesion ability to the surface without any effect on their growth. Our results showed that the B. amyloliquefaciens Cp24 crude biosurfactant efficiently inhibited biofilm formation of all the tested strains at half of its MIC. At this concentration, the biofilm formation among all the treated A. baumannii isolates was significantly inhibited (p-value<0.0001), with the percentage of inhibition ranging from 71.6 to 89.59% (Figure 3).
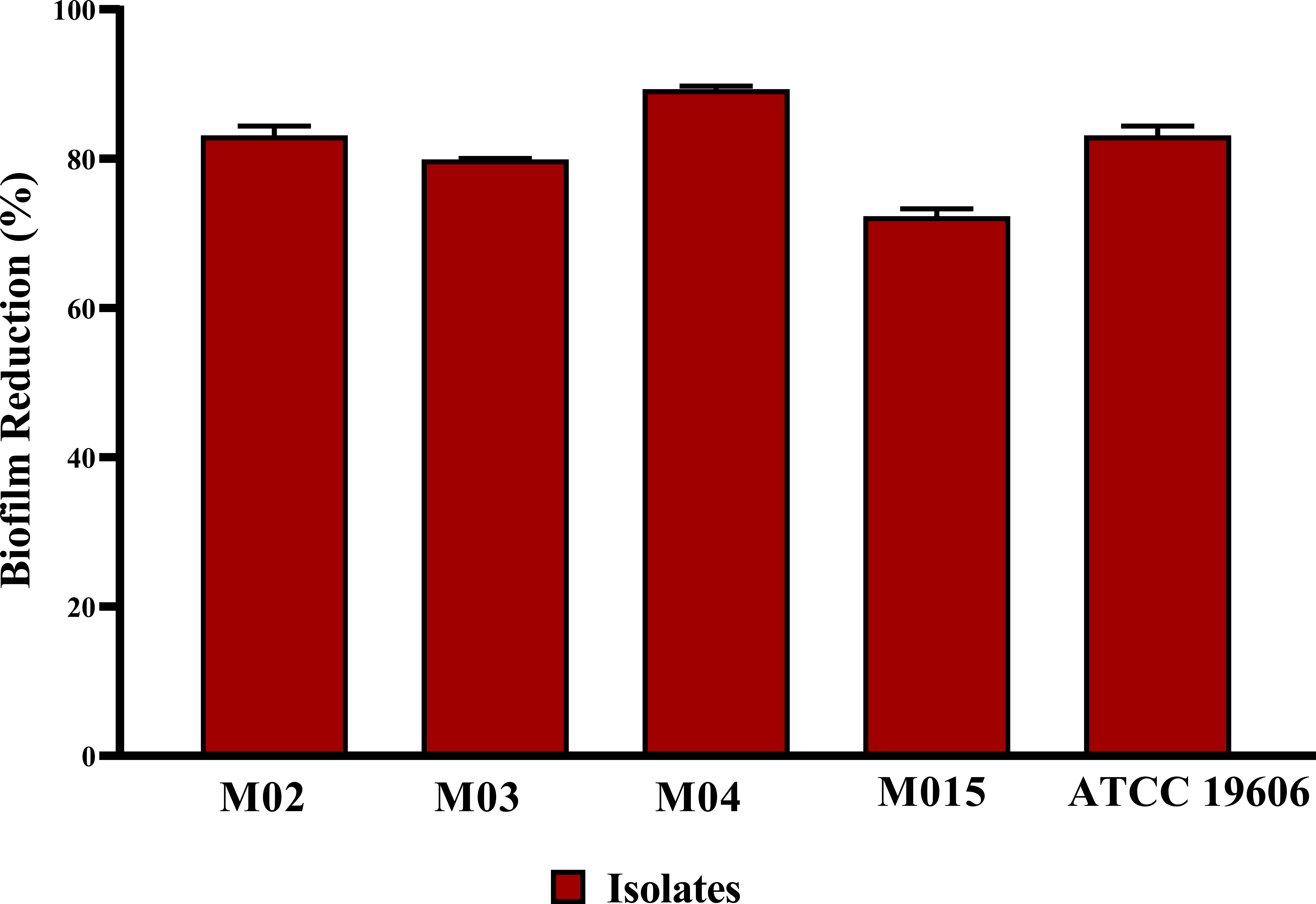
Figure 3 Quantification of the inhibitory effect of B. amyloliquefaciens crude biosurfactant on biofilms formation of A. baumannii isolates using crystal violet assay, showing the average reduction percentage of biofilm formation of A. baumannii isolates treated with 0.5X MIC of the crude biosurfactant Cp24 extract. Data represent the mean of at least three biological replicates, and error bars show the standard deviation.
3.5 Eradication effect of biosurfactant crude extract on established biofilm of A. baumannii
The antibiofilm potential of B. amyloliquefaciens Cp24 crude biosurfactant was further determined by its ability to impair the preformed biofilms of the tested strains. Our results showed that the B. amyloliquefaciens Cp24 crude biosurfactant efficiently disrupted the preformed biofilms. At the MIC concentrations, the eradication of the preformed biofilms by the B. amyloliquefaciens Cp24 crude biosurfactant ranged from 44.6% to 87.3% among all the tested strains (Figure 4).
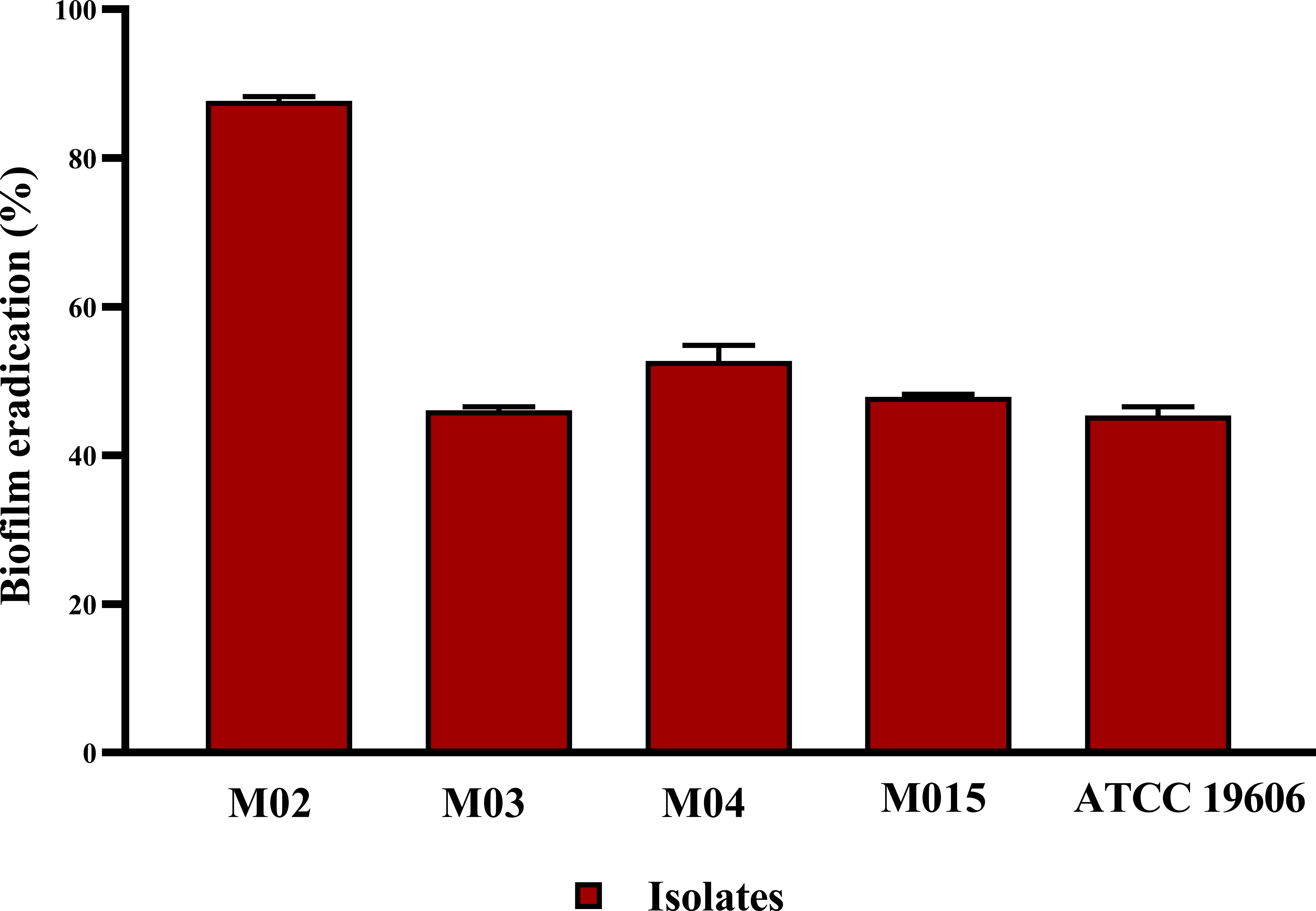
Figure 4 Quantification of the inhibitory effect of B. amyloliquefaciens crude biosurfactant on biofilms eradication of A. baumannii isolates using crystal violet assay, showing the average biofilm eradication percentage of established biofilms A. baumannii isolates. Data represent the mean of at least three biological replicates, and error bars show the standard deviation.
3.6 B. amyloliquefaciens CVCs impregnated with crude biosurfactant have a significant in vitro antibiofilm activity against A. baumannii
Catheter impregnated with 0.5X MIC of the crude biosurfactant extract caused a significant reduction in the biofilm by three log10 cycles for the clinical isolate M02. The obtained results revealed that the viability of A. baumannii isolates M02 embedded in the biofilm were remarkably reduced upon coating by the Cp24 crude biosurfactant (Figure 5). The results obtained from the biofilm biomass quantification assay revealed that the impregnated CVCs caused a 43% reduction in biofilm formation.
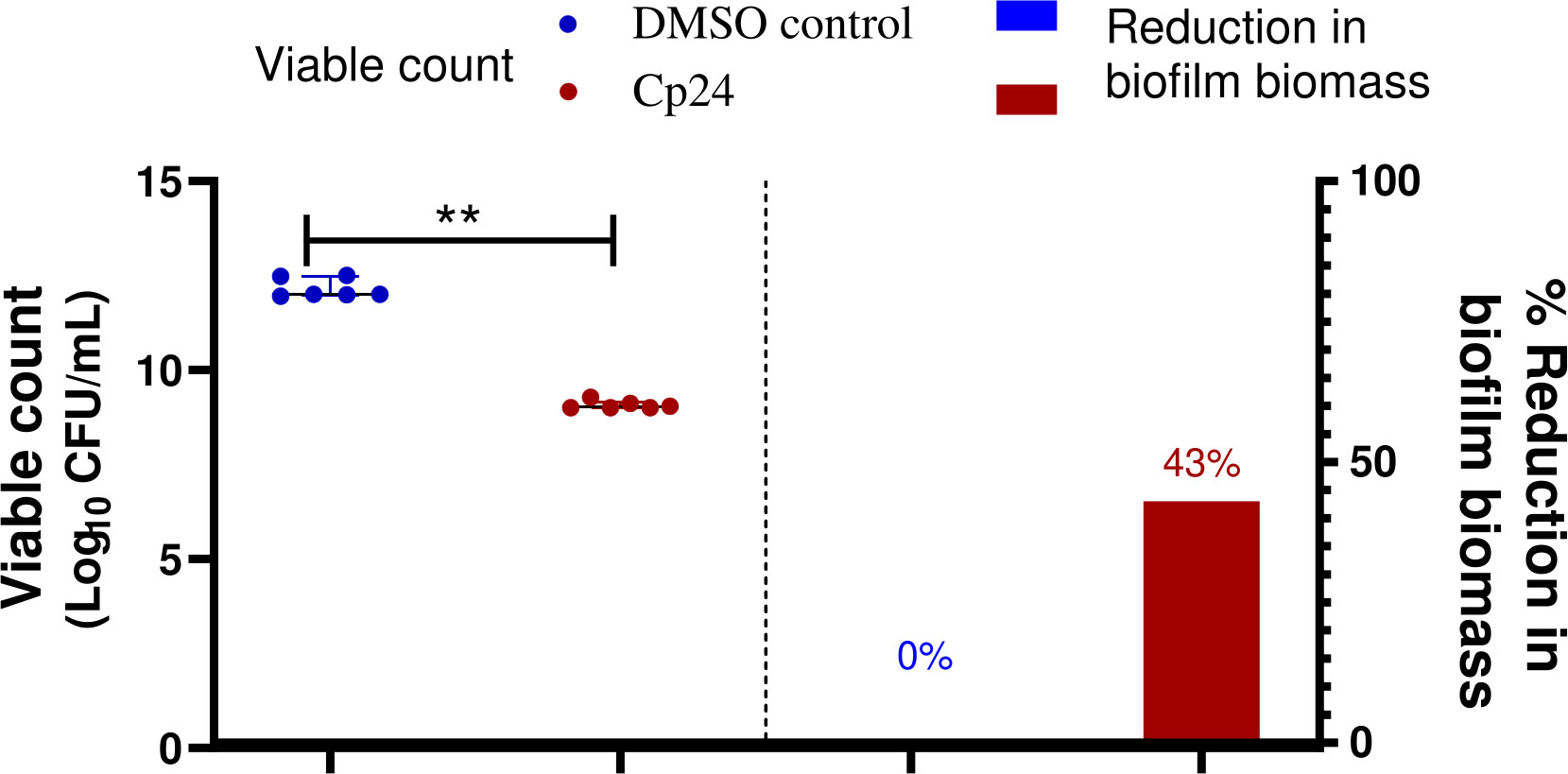
Figure 5 Quantification of the reduction in A. baumannii biofilm on crude biosurfactant extract (Cp24) impregnated CVCs compared to DMSO control. The percentage reduction in biofilm biomass was measured by crystal violet assay. The reduction in adhered viable bacterial counts of A. baumannii isolate M02 was measured by the drop plate method after 24 h of incubation. Data are represented as median with interquartile range and statistical difference was determined by student’s t-test, where statistical significance is represented by **p< 0.01.
3.7 SEM analysis revealed obvious antibiofilm activity of the impregnated CVC with the crude biosurfactant
The inhibition of biofilm formation on the impregnated CVC with the crude biosurfactant was confirmed by visualization using SEM analysis. A. baumannii growing on the control CVC surface showed higher bacterial cell density, while impregnated CVC showed scattered aggregation of fewer cells than the control (Figure 6).
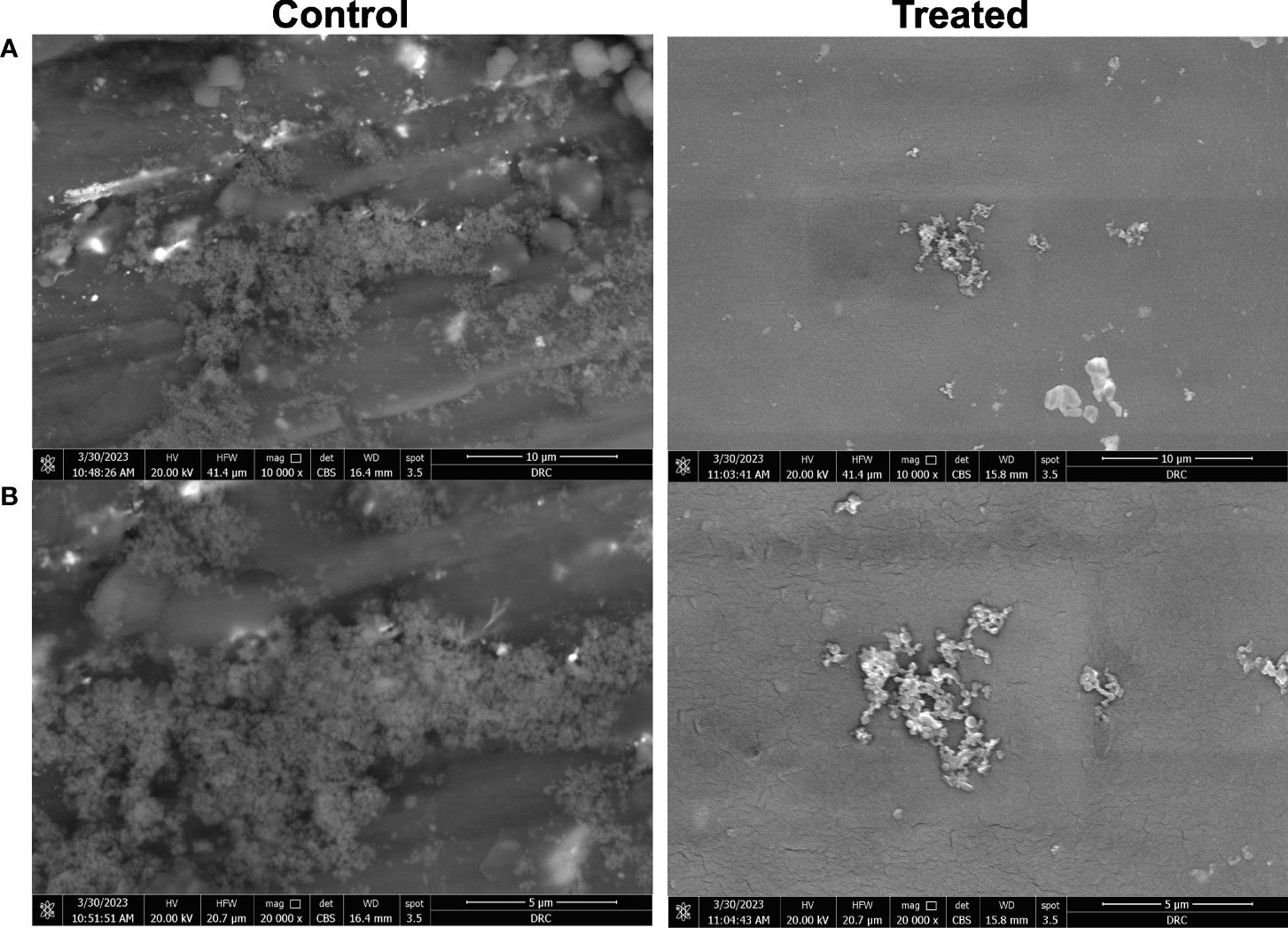
Figure 6 Microscopic visualization of biofilm formation by A. baumannii isolate M02 on CVC segments using scanning electron microscope (SEM). Biofilm formation on the catheter treated by impregnation with 0.5X MIC (0.78 mg/ml) of the crude biosurfactant extract (Cp24) was compared to the control DMSO-treated catheter. Visualization using SEM was done at magnifications of (A) 10,000 X and (B) 20,000 X.
3.8 Cytotoxicity assay for biosurfactant crude extract
No substantial change was observed in the viability of skin fibroblast cells at sub-MIC concentrations of the crude biosurfactant extract when compared to the untreated control cells. The viability of fibroblast cells at the concentration used in catheter impregnation (0.78 mg/ml) was more than 90% (Figure 7).
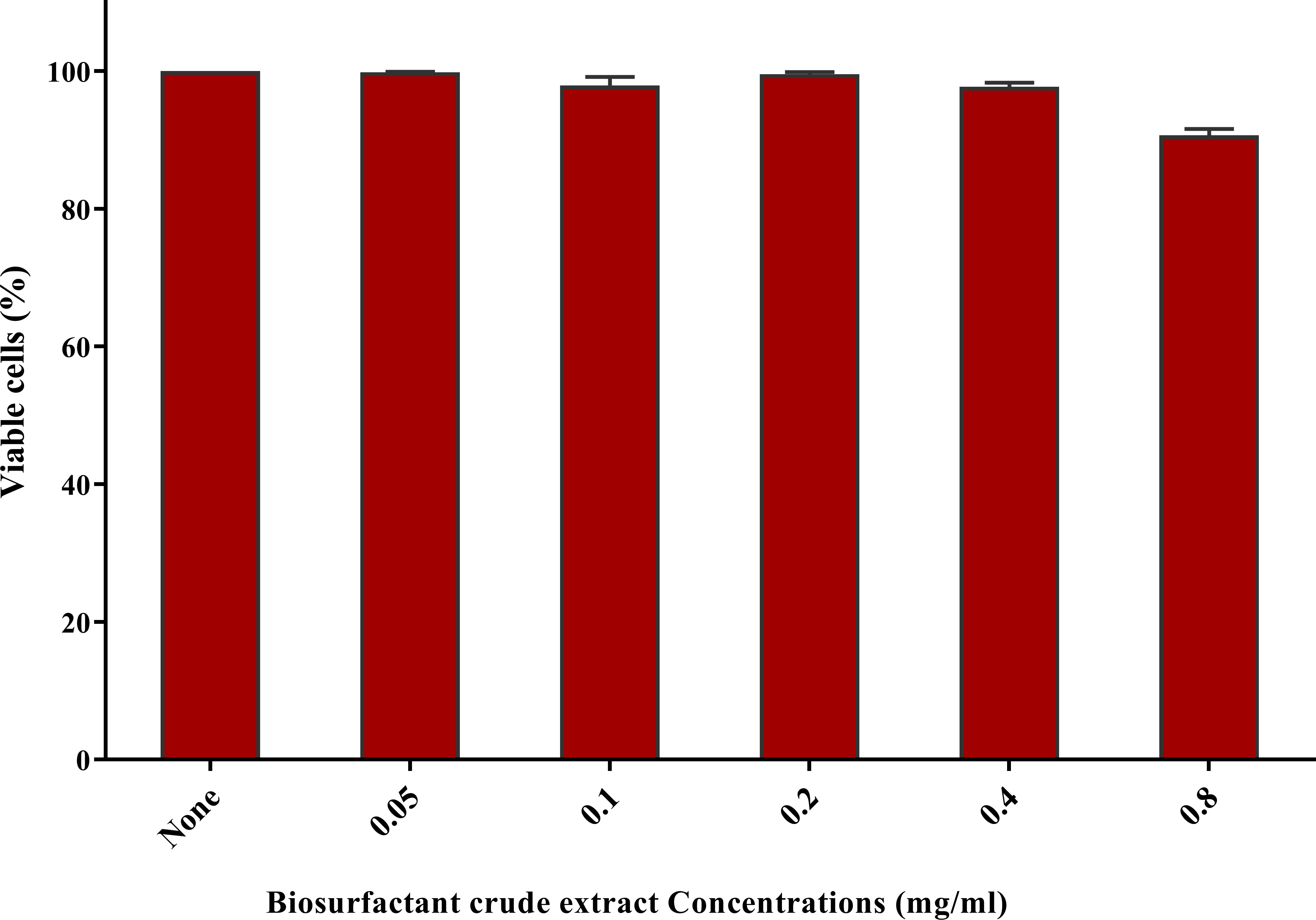
Figure 7 Cytotoxicity assay on human fibroblast cells after 24 h exposure to the nutrient medium interacting with serial dilutions of biosurfactant crude extract (0.05-0.8 mg/ml). Cytotoxicity was tested by sulforhodamine B (SRB) (0.4% w/v) and represented as the percentage of remaining viable cells after applying the treatment.
3.9 Ex vivo hemolysis assay of the biosurfactant crude extract
The potential hemolytic activity induced by the crude biosurfactant of B. amyloliquefaciens Cp24 was investigated as an indication of ex vivo blood biocompatibility. The Triton X 100 was used as a positive control and turned red color due to hemolysis, resulting in the release of hemoglobin (Hb) from the red blood cells (RBCs), whereas the negative control (PBS solutions) did not show visible hemolysis. Crude biosurfactant at a concentration of 0.78 mg/ml showed hemolysis of 6.6%. However, impregnated catheter with an equivalent concentration (0.78 mg/ml) caused a lower hemolytic effect, of 2.3%.
3.10 Gas Chromatography-Mass Spectrometry (GC–MS) Analysis
The analytical technique GC–MS consists of gas chromatography coupled with mass spectroscopy and it was used for the detection of various compounds present in the B. amyloliquefaciens Cp24 crude biosurfactant. The GC–MS chromatogram showed different peaks, indicating the presence of different compounds (Figure 8). Major peak compounds at the respective retention time were identified from the standard library compound, and are shown in Table 3.
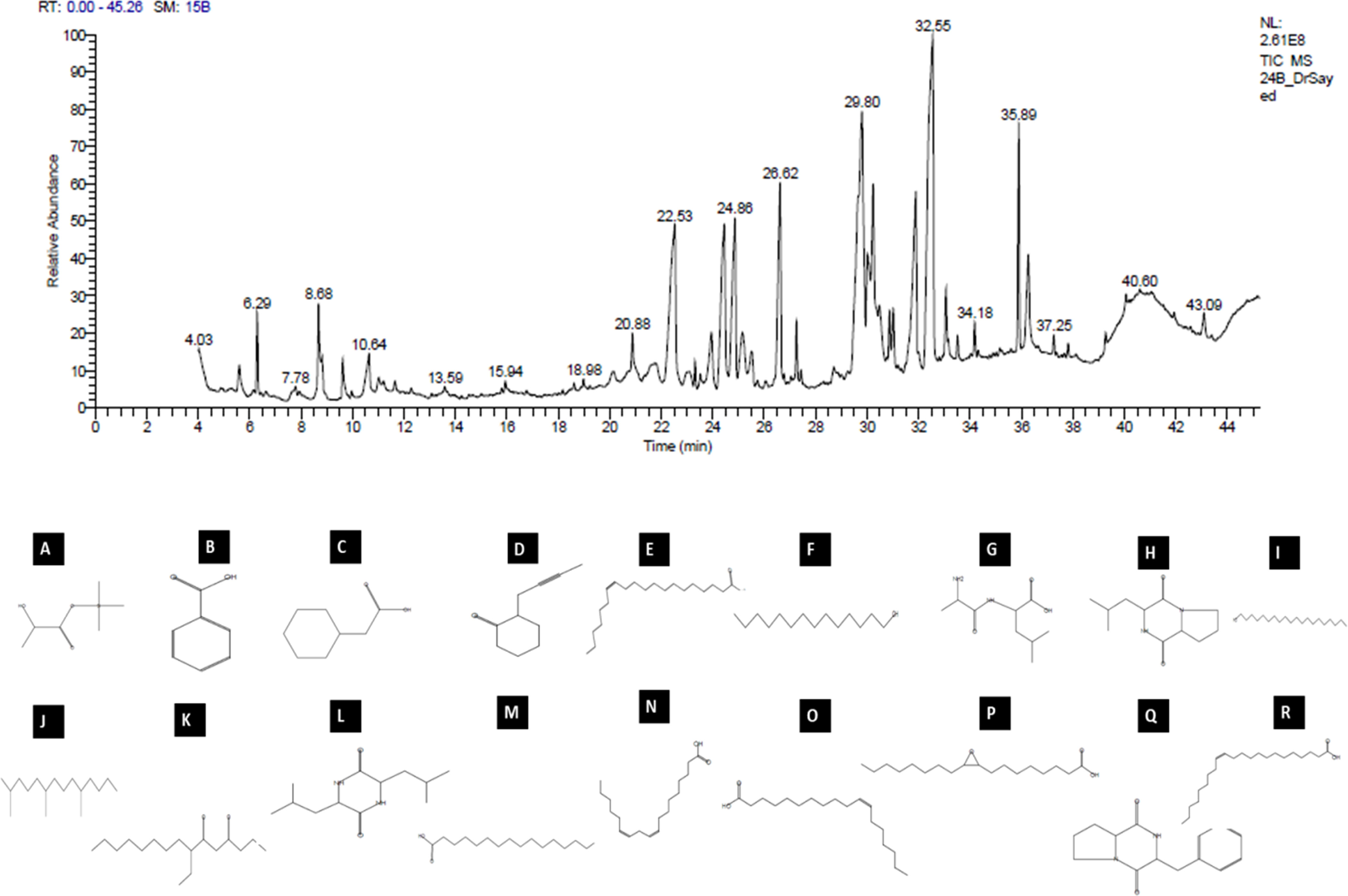
Figure 8 GC–MS analysis of the crude biosurfactants derived from B. amyloliquefaciens Cp24 endophyte. Identified compounds: (A) D-(-)-Lactic acid; (B) Benzoic acid (C) Benzeneacetic acid; (D) 2-(2-butynyl) Cyclohexanone; (E) cis-13-Eicosenoic acid; (F) 1-Tetradecanol; (G) dl-Alanyl-l-leucine; (H) [Cyclo (D-Leu-L-Pro)]; (I) 1-Eicosanol, (J) 2,6,10-Trimethyltetradecane (Farnesan); (K) 7-Ethyl-4,6-heptadecandione;(L) [Cyclo(Leu-Leu)]; (M) n-Hexadecanoic acid; (N) 9,12-Octadecadienoic acid; (O) cis-Vaccenic acid; (P) cis- (Epoxyoleic acid); (Q) 3-Benzyl-hexahydro-pyrrolo[1, 2-a]pyrazine-1,4-dione; (R) Erucic acid.
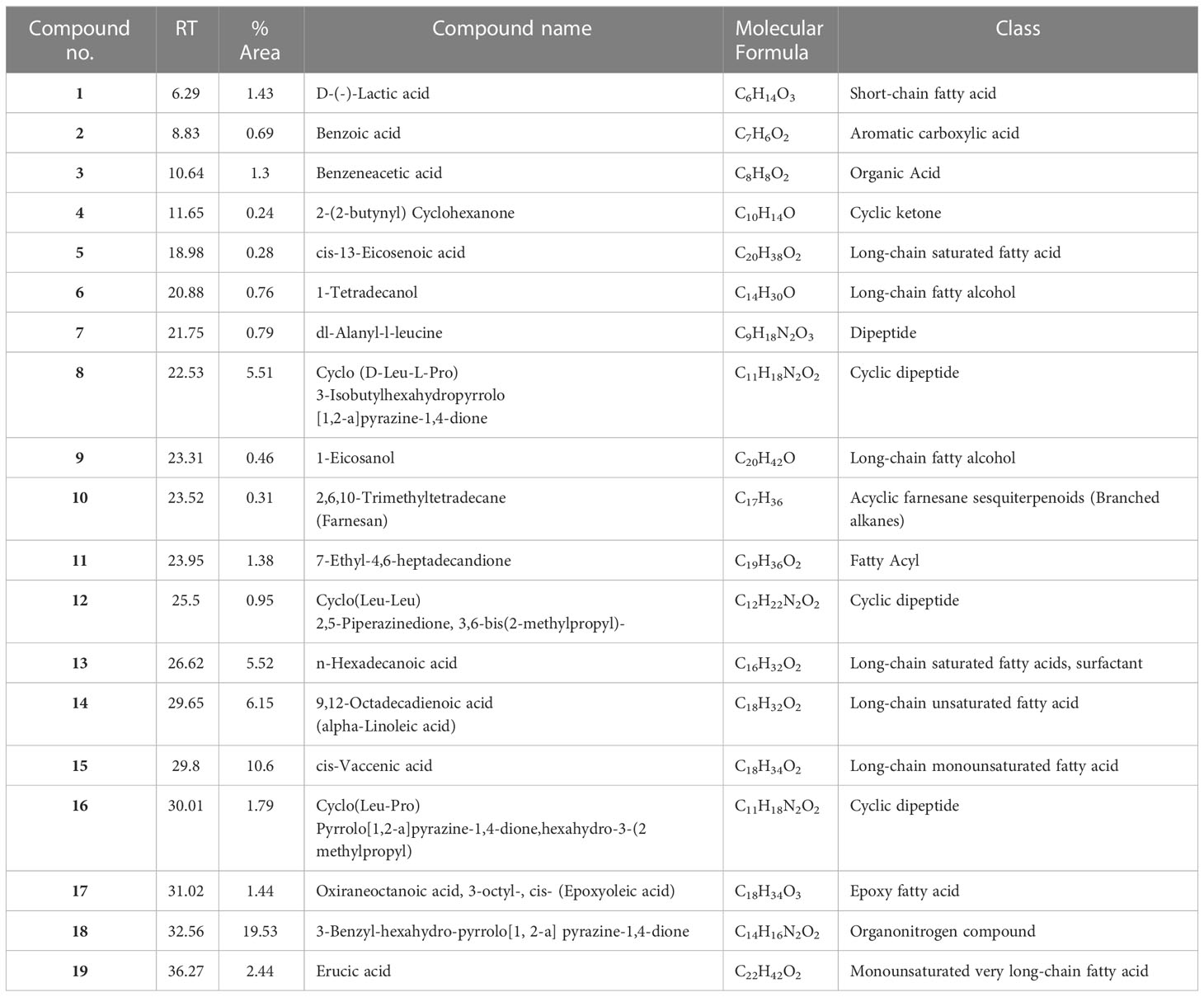
Table 3 Major constituents of the crude biosurfactants of B. amyloliquefaciens Cp24 endophyte using GC–MS.
4 Discussion
In the present study, we aimed to screen the antibiofilm activity of endophytes that produce biosurfactants against multidrug-resistant A. baumannii isolates through the collection of plant samples along the banks of the River Nile. From 10 collected plant samples, a total of 44 bacterial cultures were isolated and screened for biosurfactant activity. Endophytic isolate Cp24, isolated from Cyperus papyrus, showed strong and instant biosurfactant activity and was selected for further study. Cyperus papyrus, commonly named papyrus and used by Ancient Egyptians as a writing surface, belongs to the Cyperaceae family and is one of the plants that are widespread in the African subtropical and tropical wetlands (Mburu et al., 2015). Little scientific studies are available on this plant and its associated endophytes.
Endophytic isolate Cp24 was further identified using 16s rRNA sequencing and found to be Bacillus amyloliquefaciens. Endophytic B. amyloliquefaciens is a well-known endophyte that has been used for biological control against crop diseases and insect pests. It is found to be a safe microorganism with proven excellence in plant colonization (Liu et al., 2017). Previous studies showed the antimicrobial activity of B. amyloliquefaciens through the production of secondary metabolites, such as low-molecular-weight lipopeptides, polyenes, phospholipids, amino acids, nucleic acids, and polyketides, as well as antimicrobial proteins (Koumoutsi et al., 2004; Luo et al., 2022).
We tested the effect of the produced biosurfactant against A.baumanni, which is the causative agent for many serious infections, including endocarditis, meningitis, necrotizing fasciitis, sepsis, urinary tract infections, skin and/or soft tissue infections, and pneumonia. Its ability to survive is significantly increased by the creation of biofilms, which also renders them resistant to desiccation and antimicrobial treatment. Microbial adherence to biotic and abiotic surfaces such as catheters, ventilators, or even gloves promotes the spread of the infection from one individual to another (Vijayashree Priyadharsini et al., 2018). In the current study, the crude biosurfactant of B. amyloliquefaciens Cp24 showed considerable antibacterial effects against all the tested A. baumannii bacterial strains with MIC ranging from 0.78 to 1.56 mg/ml. Similarly, the antimicrobial activity of several biosurfactants has been previously reported (Gomaa, 2013; De Giani et al., 2021). Moreover, reports suggest that biosurfactants can prevent the growth of biofilms and microbial adherence without exhibiting antibacterial action (Tahmourespour et al., 2011; Hamza et al., 2017). The Cp24 crude biosurfactant was also tested for its antibiofilm activity against both biofilm formation and eradication of established biofilms. The antibiofilm activity against all A. baumannii isolates reached up to 71.6-89.59% at 0.5X MIC (p-value<0.01). Additionally, it successfully eradicated the preformed A. baumannii biofilms with a range of 44.6% to 87.3% among all the tested strains. Although the particular mechanisms underlying the antibiofilm activity of biosurfactants are not yet fully known, biosurfactants may impact the interactions between microorganisms and surfaces in several ways, including (1) modifying the surface’s physicochemical characteristics, such as surface energy, hydrophobicity, and surface charge, which lessens microbial adhesion (Giri et al., 2019); (2) downregulating the biofilm-related gene expression (Ohadi et al., 2020); (3) rendering the biofilms more soluble, which favors bacterial detachment (e Silva et al., 2017); and (4) decreasing biofilm formation through the interference with quorum sensing (Paraszkiewicz et al., 2021).
Numerous studies have shown that the previous coating of catheter surfaces with biosurfactants (surface conditioning) reduced microbial adhesion and colonization (Falagas and Makris, 2009; Janek et al., 2018). Therefore, our study also aimed to investigate the in vitro antibiofilm efficacy of CVC impregnated with a crude biosurfactant. Applying biosurfactants for catheter treatment reduced biofilm formation by 43%, whereas a previous study by Janek et al. (2018) showed a reduction in adherence by 70% for weak biofilm-forming Escherichia coli. The extracted biosurfactant reduced the number of viable cells adhering to CVCs by three log10 cycles, whereas previous studies on biosurfactant-coated catheters showed a reduction in the viable count by one log10 (Rodrigues et al., 2004). In addition to their ability to reduce biofilm formation, the crude biosurfactant exhibited, at its sub-MICs, no cytotoxic effect on human cell lines nor hemolytic activity against human blood, which makes it safe for use. The cytocompatibility and safety of biosurfactants have been reported in previous studies (Rodrigues, 2011; Gupta et al., 2017).
The GC-MS analysis of the Cp24 crude biosurfactant revealed the presence of 19 compounds. The nature of the majority of detected compounds was the fatty acids and peptides components of the lipopeptide biosurfactant, which conforms with the previously identified nature of B. amyloliquefaciens biosurfactants as a lipopeptide surfactant (Sarwar et al., 2018; Wu et al., 2022). The complex nature of biosurfactants and their mosaic distribution of polarity, as well as branched or circular structures, give them remarkable physical properties compared to synthetic surfactants. Therefore, biosurfactants, such as surfactin, with their cyclic nature and dynamic surface properties can combine densely at the interface, increasing their surface activity and providing them with properties that make them suitable for many potential applications (Otzen, 2017).
Cyclic peptides detected in the Cp24 extract have been detected as products by many Bacillus sp. and have been reported for many potential applications in the agricultural, pharmaceutical, and biotechnology industries due to their dynamic surface properties (Sarwar et al., 2018). Previous studies showed that the diketopiperazines (DKPs) cyclopeptides ((Cyclo(Leu-Pro) and Cyclo (Leu-Leu)), similar to those detected in our extract, inhibit quorum sensing mechanisms and biofilm formation by various microorganisms, such as soft rot-causing pathogen Lelliottia amnigena RCE and P.aeruginosa (Rashiya et al., 2021; Kachhadia et al., 2022). Additionally, the presence of nitrogen atoms in DKPs makes them physiologically more stable compared to their counterpart lactones (Almohaywi et al., 2019).
There are three primary FA groups produced by Bacillus species: branched-chain FAs, straight-chain FAs, and complex FA types, such as cyclic, hydroxyl, or epoxy FAs. The fatty acid (FA) composition of bacterial cells differs depending on the species, and, as an adaptation to environmental changes is crucial for bacterial growth, the FA composition of the cell’s membrane fluctuates according to the environment. The crude biosurfactant extract is composed of a mixture of fatty acids with a range of short-chain fatty acids (Lactic acid) to long-chain fatty acids, varying from C16 to C22 saturated (cis-13-eicosenoic acid and n-hexadecanoic acid) and unsaturated fatty acids (9,12-octadecadienoic acid, cis-vaccenic acid, and erucic acid). In addition, it also contained epoxy oleic acid. Previous studies showed that some Bacillus species are capable of producing epoxy FAs, which are FAs with one or two epoxy groups with antibacterial capabilities (Diomandé et al., 2015). Unsaturated fatty acids, such as cis-9-hexadecenoic acid and cis-9-tetradecenoic acid, were reported to inhibit the quorum sensing system and subsequently reduce the biofilm formation and suppress motility in V. cholerae and A. baumannii ATCC 17978 (Nicol et al., 2018). Previous studies revealed that fatty acids and their derivatives reduced the virulence characteristics of Chromobacterium violaceum (Santhakumari et al., 2017) and Vibrio Sp. (Pérez-López et al., 2018). Higher molecular-weight biosurfactants displayed higher emulsification activity, according to a study by Martins and Martins (2018).
The compound 3-Benzyl-hexahydro-pyrrolo[1, 2-a]pyrazine-1,4-dione represents 19% of the Cp24 extract component and it was previously identified in Exiguobacterium indicum SJ16 extract isolated from the rhizosphere of Cyperus laevigatus. It showed significant quorum sensing inhibition against the reference Chromobacterium violaceum CV026 strain and also inhibited the biofilm formation of P. aeruginosa (Singh et al., 2019).
5 Conclusion
In conclusion, this study demonstrates that impregnating catheters with crude biosurfactant of endophytic B. amyloliquefaciens strain Cp24 effectively reduces biofilm formation of A. baumannii, a multidrug-resistant bacterium that belongs to global clones and has a strong biofilm-forming capacity. An additional advantage is the safety of this compound on human cell lines and its reduced hemolysis activity. GC-MS analysis confirmed the production of lipopeptides with cyclic peptide moiety providing them with dynamic surface properties that increased their surface activity. In addition, the presence of long-chain and epoxy-type fatty acids was observed, which reduced virulence factors and exhibited quorum sensing inhibition activity, therefore reducing biofilm formation. Further studies should evaluate this approach by in vivo analysis via long-term catheterization in animal models and study the possible synergistic activity of these fatty acids with other antivirulence compounds to increase their activity.
Data availability statement
The original contributions presented in the study are included in the article/Supplementary Material. Further inquiries can be directed to the corresponding author.
Ethics statement
The study was performed in accordance with relevant guidelines and regulations and has been approved by the Ethics Committee of October University for Modern Sciences and Arts with the reference number M1/EC1/2023PD.
Author contributions
All authors conceptualized the work, performed the experiments, analyzed the results, edited the manuscript, contributed to the article, and approved the submitted version.
Acknowledgments
The authors wish to thank Prof. Shahira M. Ezzat, Professor of Phytochemistry, Faculty of Pharmacy, Cairo University and October University for Modern Sciences and Arts for aiding in the identification of some plants. The authors extend their appreciation to Dr. Rana M. Merghany, Department of Pharmacognosy, National Research Centre for providing some of the plants included in the study.
Conflict of interest
The authors declare that the research was conducted in the absence of any commercial or financial relationships that could be construed as a potential conflict of interest
Publisher’s note
All claims expressed in this article are solely those of the authors and do not necessarily represent those of their affiliated organizations, or those of the publisher, the editors and the reviewers. Any product that may be evaluated in this article, or claim that may be made by its manufacturer, is not guaranteed or endorsed by the publisher.
Supplementary material
The Supplementary Material for this article can be found online at: https://www.frontiersin.org/articles/10.3389/fcimb.2023.1210195/full#supplementary-material
References
Abd El-Rahman, O. A., Rasslan, F., Hassan, S. S., Ashour, H. M., Wasfi, R. (2023). The RND efflux pump gene expression in the biofilm formation of acinetobacter baumannii. Antibiotics (Basel) 12, 419. doi: 10.3390/antibiotics12020419
Allam, R. M., Al-Abd, A. M., Khedr, A., Sharaf, O. A., Nofal, S. M., Khalifa, A. E., et al. (2018). Fingolimod interrupts the cross talk between estrogen metabolism and sphingolipid metabolism within prostate cancer cells. Toxicol. Lett. 291, 77–85. doi: 10.1016/j.toxlet.2018.04.008
Almohaywi, B., Yu, T. T., Iskander, G., Chan, D. S. H., Ho, K. K. K., Rice, S., et al. (2019). Dihydropyrrolones as bacterial quorum sensing inhibitors. Bioorg Med. Chem. Lett. 29, 1054–1059. doi: 10.1016/j.bmcl.2019.03.004
Amer, M. A., Ramadan, M. A., Attia, A. S., Wasfi, R. (2021). Indole derivatives obtained from Egyptian enterobacter sp. soil isolates exhibit antivirulence activities against uropathogenic Proteus mirabilis. Antibiotics (Basel) 10, 363. doi: 10.3390/antibiotics10040363
Amer, M. A., Wasfi, R., Attia, A. S., and Ramadan, M. A. (2022). Silicone Foley catheters impregnated with microbial indole derivatives inhibit crystalline biofilm formation by Proteus mirabilis. Front. Cell. Infection Microbiol. 12. doi: 10.3389/fcimb.2022.1010625
Ashitha, A., Radhakrishnan, E. K., Mathew, J. (2020). Characterization of biosurfactant produced by the endophyte burkholderia sp. WYAT7 and evaluation of its antibacterial and antibiofilm potentials. J. Biotechnol. 313, 1–10. doi: 10.1016/j.jbiotec.2020.03.005
Banat, I. M., De Rienzo, M. A., Quinn, G. A. (2014). Microbial biofilms: biosurfactants as antibiofilm agents. Appl. Microbiol. Biotechnol. 98, 9915–9929. doi: 10.1007/s00253-014-6169-6
Castilho, S. R. A., Godoy, C. S. M., Guilarde, A. O., Cardoso, J. L., Andre, M. C. P., Junqueira-Kipnis, A.P., et al. (2017). Acinetobacter baumannii strains isolated from patients in intensive care units in goiânia, Brazil: molecular and drug susceptibility profiles. PLoS One 12, e0176790. doi: 10.1371/journal.pone.0176790
CLSI (2015). “Methods for dilution antimicrobial susceptibility tests for bacteria that grow aerobically; approved standard–tenth edition,” in CLSI document M07-A10 (Wayne: PA Clinical and Laboratory Standards Institute).
Corrêa Carvalho, G., Miguel Sábio, R., Spósito, L., De Jesus Andreoli Pinto, T., Chorilli, M. (2022). An overview of the use of central venous catheters impregnated with drugs or with inorganic nanoparticles as a strategy in preventing infections. Int. J. Pharmaceutics 615, 121518. doi: 10.1016/j.ijpharm.2022.121518
De Giani, A., Zampolli, J., Di Gennaro, P. (2021). Recent trends on biosurfactants with antimicrobial activity produced by bacteria associated with human health: different perspectives on their properties, challenges, and potential applications. Front. Microbiol. 12. doi: 10.3389/fmicb.2021.655150
Diomandé, S. E., Nguyen-The, C., Guinebretière, M. H., Broussolle, V., Brillard, J. (2015). Role of fatty acids in bacillus environmental adaptation. Front. Microbiol. 6. doi: 10.3389/fmicb.2015.00813
Donlan, R. M. (2011). Biofilm elimination on intravascular catheters: important considerations for the infectious disease practitioner. Clin. Infect. Dis. 52, 1038–1045. doi: 10.1093/cid/cir077
Duarte, A., Ferreira, S., Almeida, S., Domingues, F. C. (2016). Clinical isolates of acinetobacter baumannii from a Portuguese hospital: PFGE characterization, antibiotic susceptibility and biofilm-forming ability. Comp. Immunol. Microbiol. Infect. Dis. 45, 29–33. doi: 10.1016/j.cimid.2016.02.002
Eras-Muñoz, E., Farré, A., Sánchez, A., Font, X., Gea, T. (2022). Microbial biosurfactants: a review of recent environmental applications. Bioengineered 13, 12365–12391. doi: 10.1080/21655979.2022.2074621
Eze, E. C., Chenia, H. Y., El Zowalaty, M. E. (2018). Acinetobacter baumannii biofilms: effects of physicochemical factors, virulence, antibiotic resistance determinants, gene regulation, and future antimicrobial treatments. Infect. Drug Resist. 11, 2277–2299. doi: 10.2147/IDR.S169894
Falagas, M. E., Makris, G. C. (2009). Probiotic bacteria and biosurfactants for nosocomial infection control: a hypothesis. J. Hosp Infect. 71, 301–306. doi: 10.1016/j.jhin.2008.12.008
Gedefie, A., Demsis, W., Ashagrie, M., Kassa, Y., Tesfaye, M., Tilahun, M., et al. (2021). Acinetobacter baumannii biofilm formation and its role in disease pathogenesis: a review. Infect. Drug Resist. 14, 3711–3719. doi: 10.2147/IDR.S332051
Giri, S. S., Ryu, E. C., Sukumaran, V., Park, S. C. (2019). Antioxidant, antibacterial, and anti-adhesive activities of biosurfactants isolated from bacillus strains. Microb. Pathog. 132, 66–72. doi: 10.1016/j.micpath.2019.04.035
Gomaa, E. Z. (2013). Antimicrobial activity of a biosurfactant produced by bacillus licheniformis strain M104 grown on whey. Braz. Arch. Biol. Technol. 56. doi: 10.1590/S1516-89132013000200011
Gouda, S., Das, G., Sen, S. K., Shin, H. S., Patra, J. K. (2016). Endophytes: a treasure house of bioactive compounds of medicinal importance. Front. Microbiol. 7. doi: 10.3389/fmicb.2016.01538
Guenezan, J., Drugeon, B., Marjanovic, N., Mimoz, O. (2018). Treatment of central line-associated bloodstream infections. Crit. Care 22, 303. doi: 10.1186/s13054-018-2249-9
Gupta, S., Raghuwanshi, N., Varshney, R., Banat, I. M., Srivastava, A. K., Pruthi, P. A., et al. (2017). Accelerated in vivo wound healing evaluation of microbial glycolipid containing ointment as a transdermal substitute. BioMed. Pharmacother. 94, 1186–1196. doi: 10.1016/j.biopha.2017.08.010
Hamed, S. M., Hussein, A. F. A., Al-Agamy, M. H., Radwan, H. H., Zafer, M. M. (2022). Genetic configuration of genomic resistance islands in acinetobacter baumannii clinical isolates from Egypt. Front. Microbiol. 13. doi: 10.3389/fmicb.2022.878912
Hamza, F., Satpute, S., Banpurkar, A., Kumar, A. R., Zinjarde, S. (2017). Biosurfactant from a marine bacterium disrupts biofilms of pathogenic bacteria in a tropical aquaculture system. FEMS Microbiol. Ecol. 93. doi: 10.1093/femsec/fix140
Herigstad, B., Hamilton, M., Heersink, J. (2001). How to optimize the drop plate method for enumerating bacteria. J. Microbiol. Methods 44, 121–129. doi: 10.1016/s0167-7012(00)00241-4
Hou, Z., Wu, Y., Xu, C., Reghu, S., Shang, Z., Chen, J., et al. (2020). Precisely structured nitric-Oxide-Releasing copolymer brush defeats broad-spectrum catheter-associated biofilm infections In vivo. ACS Cent. Sci. 6, 2031–2045. doi: 10.1021/acscentsci.0c00755
Ivanova, A., Ivanova, K., Perelshtein, I., Gedanken, A., Todorova, K., Milcheva, R., et al. (2021). Sonochemically engineered nano-enabled zinc oxide/amylase coatings prevent the occurrence of catheter-associated urinary tract infections. Materials Sci. Engineering: C 131, 112518. doi: 10.1016/j.msec.2021.112518
Janek, T., Krasowska, A., Czyznikowska, Z., Lukaszewicz, M. (2018). Trehalose lipid biosurfactant reduces adhesion of microbial pathogens to polystyrene and silicone surfaces: an experimental and computational approach. Front Microbiol 9, 2441. doi: 10.3389/fmicb.2018.02441
Joe, M., Gomathi, R., Benson, A., Devaraj, S., Rengasamy, P., Henry, A., et al. (2019). Simultaneous application of biosurfactant and bioaugmentation with rhamnolipid-producing shewanella for enhanced bioremediation of oil-polluted soil. Appl. Sci. 9, 3773. doi: 10.3390/app9183773
Kachhadia, R., Kapadia, C., Singh, S., Gandhi, K., Jajda, H., Alfarraj, S., et al. (2022). Quorum sensing inhibitory and quenching activity of bacillus cereus RC1 extracts on soft rot-causing bacteria lelliottia amnigena. ACS Omega 7, 25291–25308. doi: 10.1021/acsomega.2c02202
Katoch, M., Phull, S., Vaid, S., Singh, S. (2017). Diversity, phylogeny, anticancer and antimicrobial potential of fungal endophytes associated with monarda citriodora l. BMC Microbiol. 17, 44. doi: 10.1186/s12866-017-0961-2
Koumoutsi, A., Chen, X. H., Henne, A., Liesegang, H., Hitzeroth, G., Franke, P., et al. (2004). Structural and functional characterization of gene clusters directing nonribosomal synthesis of bioactive cyclic lipopeptides in bacillus amyloliquefaciens strain FZB42. J. Bacteriol 186, 1084–1096. doi: 10.1128/jb.186.4.1084-1096.2004
Lafuente Cabrero, E., Terradas Robledo, R., Civit Cunado, A., Garcia Sardelli, D., Hidalgo Lopez, C., Giro Formatger, D., et al. (2023). Risk factors of catheter- associated bloodstream infection: systematic review and meta-analysis. PLoS One 18, e0282290. doi: 10.1371/journal.pone.0282290
Lemos, A. S. O., Campos, L. M., Melo, L., Guedes, M., Oliveira, L. G., Silva, T. P, et al. (2018). Antibacterial and antibiofilm activities of psychorubrin, a pyranonaphthoquinone isolated from mitracarpus frigidus (Rubiaceae). Front. Microbiol. 9. doi: 10.3389/fmicb.2018.00724
Liu, H., Carvalhais, L. C., Crawford, M., Singh, E., Dennis, P. G., Pieterse, C. M. J, et al. (2017). Inner plant values: diversity, colonization and benefits from endophytic bacteria. Front. Microbiol. 8. doi: 10.3389/fmicb.2017.02552
Luo, X., Sun, J., Lu, Y. (2022). Research progress in the biosynthesis, antimicrobial mechanism, and application of lipopeptides inBacillus amyloliquefaciens. Sci. Technol. Food Industry 43, 462–470. doi: 10.13386/j.issn1002-0306.2021100259
Lynch, J. P., Zhanel, G. G., Clark, N. M. (2017). Infections due to acinetobacter baumannii in the ICU: treatment options. Semin. Respir. Crit. Care Med. 38, 311–325. doi: 10.1055/s-0037-1599225
Mansouri, M. D., Hull, R. A., Stager, C. E., Cadle, R.M., Darouiche, R. O. (2013). In vitro activity and durability of a combination of an antibiofilm and an antibiotic against vascular catheter colonization. Antimicrob. Agents Chemother. 57, 621–625. doi: 10.1128/AAC.01646-12
Marchut-Mikołajczyk, O., Drożdżyński, P., Polewczyk, A., Smułek, W., Antczak, T. (2021). Biosurfactant from endophytic bacillus pumilus 2A: physicochemical characterization, production and optimization and potential for plant growth promotion. Microbial Cell Factories 20, 40. doi: 10.1186/s12934-021-01533-2
Martins, P. C., Martins, V. G. (2018). Biosurfactant production from industrial wastes with potential remove of insoluble paint. Int. Biodeterioration Biodegradation 127, 10–16. doi: 10.1016/j.ibiod.2017.11.005
Mburu, N., Rousseau, D. P. L., Van Bruggen, J. J. A., Lens, P. N. L. (2015). Use of the macrophyte Cyperus papyrus in wastewater treatment. In: ed. J. Vymazal The Role of Natural and Constructed Wetlands in Nutrient Cycling and Retention on the Landscape (Cham: Springer International Publishing), 293–313. doi: 10.1007/978-3-319-08177-9_20
Nahar, A., Anwar, S., Miah, M. (2013). Association of biofilm formation with antimicrobial resistance among the acinetobacter species in a tertiary care hospital in Bangladesh. J. Med. 14, 284–32. doi: 10.3329/jom.v14i1.14533
Naves, P., Del Prado, G., Huelves, L., Gracia, M., Ruiz, V., Blanco, J., et al. (2008). Measurement of biofilm formation by clinical isolates of escherichia coli is method-dependent. J. Appl. Microbiol. 105, 585–590. doi: 10.1111/j.1365-2672.2008.03791.x
Neethu, S., Midhun, S. J., Radhakrishnan, E. K., Jyothis, M. (2020). Surface functionalization of central venous catheter with mycofabricated silver nanoparticles and its antibiofilm activity on multidrug resistant acinetobacter baumannii. Microb. Pathog. 138, 103832. doi: 10.1016/j.micpath.2019.103832
Nicol, M., Alexandre, S., Luizet, J. B., Skogman, M., Jouenne, T., Salcedo, S. P., et al. (2018). Unsaturated fatty acids affect quorum sensing communication system and inhibit motility and biofilm formation of acinetobacter baumannii. Int. J. Mol. Sci. 19, 214. doi: 10.3390/ijms19010214
Ohadi, M., Forootanfar, H., Dehghannoudeh, G., Eslaminejad, T., Ameri, A., Shakibaie, M., et al. (2020). Antimicrobial, anti-biofilm, and anti-proliferative activities of lipopeptide biosurfactant produced by acinetobacter junii B6. Microb. Pathog. 138, 103806. doi: 10.1016/j.micpath.2019.103806
Otzen, D. E. (2017). Biosurfactants and surfactants interacting with membranes and proteins: same but different? Biochim. Biophys. Acta (BBA) - Biomembranes 1859, 639–649. doi: 10.1016/j.bbamem.2016.09.024
Paraszkiewicz, K., Moryl, M., Płaza, G., Bhagat, D., S, K. S., Bernat, P., et al (2021). Surfactants of microbial origin as antibiofilm agents. Int. J. Environ. Health Res. 31, 401–420. doi: 10.1080/09603123.2019.1664729
Patel, M., Siddiqui, A. J., Hamadou, W. S., Surti, M., Awadelkareem, A. M., Ashraf, S. A., et al (2021). Inhibition of bacterial adhesion and antibiofilm activities of a glycolipid biosurfactant from lactobacillus rhamnosus with its physicochemical and functional properties. Antibiotics (Basel) 10, 1546. doi: 10.3390/antibiotics10121546
Pathak, R., Bierman, S. F., D'arnaud, P. (2018). Inhibition of bacterial attachment and biofilm formation by a novel intravenous catheter material using an in vitro percutaneous catheter insertion model. Med. Devices (Auckl) 11, 427–432. doi: 10.2147/MDER.S183409
Peleg, A. Y., De Breij, A., Adams, M. D., Cerqueira, G. M., Mocali, S., Galardini, M., et al (2012). The success of acinetobacter species; genetic, metabolic and virulence attributes. PloS One 7, e46984. doi: 10.1371/journal.pone.0046984
Pérez-López, M., García-Contreras, R., Soto-Hernández, M., Rodríguez-Zavala, J. S., Martínez-Vázquez, M., Prado-Galbarro, F. J, et al. (2018). Antiquorum sensing activity of seed oils from oleaginous plants and protective effect during challenge with chromobacterium violaceum. J. Med. Food 21, 356–363. doi: 10.1089/jmf.2017.0080
Raad, I., Mohamed, J. A., Reitzel, R. A., Jiang, Y., Raad, S., Al Shuaibi, M., et al (2012). Improved antibiotic-impregnated catheters with extended-spectrum activity against resistant bacteria and fungi. Antimicrob. Agents Chemother. 56, 935–941. doi: 10.1128/aac.05836-11
Rashiya, N., Padmini, N., Ajilda, A. a. K., Prabakaran, P., Durgadevi, R., Veera Ravi, A., et al. (2021). Inhibition of biofilm formation and quorum sensing mediated virulence in pseudomonas aeruginosa by marine sponge symbiont brevibacterium casei strain alu 1. Microb. Pathog. 150, 104693. doi: 10.1016/j.micpath.2020.104693
Rodrigues, L. R. (2011). Inhibition of bacterial adhesion on medical devices. Adv. Exp. Med. Biol. 715, 351–367. doi: 10.1007/978-94-007-0940-9_22
Rodrigues, L., Van Der Mei, H. C., Teixeira, J., Oliveira, R. (2004). Influence of biosurfactants from probiotic bacteria on formation of biofilms on voice prostheses. Appl. Environ. Microbiol. 70, 4408–4410. doi: 10.1128/aem.70.7.4408-4410.2004
Rolain, J. M., Diene, S. M., Kempf, M., Gimenez, G., Robert, C., Raoult, D. (2013). Real-time sequencing to decipher the molecular mechanism of resistance of a clinical pan-drug-resistant acinetobacter baumannii isolate from marseille, France. Antimicrob. Agents Chemother. 57, 592–596. doi: 10.1128/AAC.01314-12
Ryan, R. P., Germaine, K., Franks, A., Ryan, D.J., Dowling, D.N.. (2008). Bacterial endophytes: recent developments and applications. FEMS Microbiol. Lett. 278, 1–9. doi: 10.1111/j.1574-6968.2007.00918.x
Sanchez, C. J., Mende, K., Beckius, M. L., Akers, K. S., Romano, D. R., Wenke, J. C, et al. (2013). Biofilm formation by clinical isolates and the implications in chronic infections. BMC Infect. Dis. 13, 47. doi: 10.1186/1471-2334-13-47
Santhakumari, S., Nilofernisha, N. M., Ponraj, J. G., Pandian, S. K., Ravi, A. V. (2017). In vitro and in vivo exploration of palmitic acid from synechococcus elongatus as an antibiofilm agent on the survival of artemia franciscana against virulent vibrios. J. Invertebr Pathol. 150, 21–31. doi: 10.1016/j.jip.2017.09.001
Sarwar, A., Brader, G., Corretto, E., Aleti, G., Ullah, M. A., Sessitsch, A, et al. (2018). Qualitative analysis of biosurfactants from bacillus species exhibiting antifungal activity. PloS One 13, e0198107. doi: 10.1371/journal.pone.0198107
Satpute, S. K., Banpurkar, A. G., Dhakephalkar, P. K., Banat, I. M., Chopade, B. A. (2010). Methods for investigating biosurfactants and bioemulsifiers: a review. Crit. Rev. Biotechnol. 30, 127–144. doi: 10.3109/07388550903427280
Silva, E., Carvalho, J. W. P., Aires, C. P., Nitschke, M. (2017). Disruption of staphylococcus aureus biofilms using rhamnolipid biosurfactants. J. Dairy Sci. 100, 7864–7873. doi: 10.3168/jds.2017-13012
Singh, V. K., Mishra, A., Jha, B. (2019). 3-Benzyl-Hexahydro-Pyrrolo[1,2-a]Pyrazine-1,4-Dione extracted from exiguobacterium indicum showed anti-biofilm activity against pseudomonas aeruginosa by attenuating quorum sensing. Front. Microbiol. 10. doi: 10.3389/fmicb.2019.01269
Sivanandan, S. (2020). Do antimicrobial-impregnated central venous catheters prevent nosocomial bloodstream infection in neonates? Acta Paediatrica 109, 1907–1908. doi: 10.1111/apa.15268
Tahmourespour, A., Salehi, R., Kasra Kermanshahi, R. (2011). Lactobacillus acidophilus-derived biosurfactant effect on GTFB and GTFC expression level in streptococcus mutans biofilm cells. Braz. J. Microbiol. 42, 330–339. doi: 10.1590/s1517-83822011000100042
Tamura, K., Stecher, G., Peterson, D., Filipski, A., Kumar, S. (2013). MEGA6: molecular evolutionary genetics analysis version 6.0. Mol. Biol. And Evol. 30, 2725–2729. doi: 10.1093/molbev/mst197
Tidke, S. A., Kiran, S., Giridhar, P., Gokare, R. A. (2019). “Current understanding and future perspectives of endophytic microbes vis-a-vis production of secondary metabolites,” in Endophytes and secondary metabolites. Ed. Jha, S. (Cham: Springer International Publishing), 459–474.
Vijayashree Priyadharsini, J., Smiline Girija, A. S., Paramasivam, A. (2018). In silico analysis of virulence genes in an emerging dental pathogen a. baumannii and related species. Arch. Oral. Biol. 94, 93–98. doi: 10.1016/j.archoralbio.2018.07.001
Wang, H., Tong, H., Liu, H., Wang, Y., Wang, R., Gao, H, et al. (2018). Effectiveness of antimicrobial-coated central venous catheters for preventing catheter-related blood-stream infections with the implementation of bundles: a systematic review and network meta-analysis. Ann. Intensive Care 8, 71. doi: 10.1186/s13613-018-0416-4
Wasfi, R., Rasslan, F., Hassan, S. S., Ashour, H. M., Abd El-Rahman, O. A. (2021). Co-Existence of carbapenemase-encoding genes in acinetobacter baumannii from cancer patients. Infect. Dis. Ther. 10, 291–305. doi: 10.1007/s40121-020-00369-4
Weisburg, W. G., Barns, S. M., Pelletier, D. A., Lane, D. J. (1991). 16S ribosomal DNA amplification for phylogenetic study. J. Bacteriol 173, 697–703. doi: 10.1128/jb.173.2.697-703.1991
Wu, B., Xiu, J., Yu, L., Huang, L., Yi, L., Ma, Y. (2022). Biosurfactant production by bacillus subtilis SL and its potential for enhanced oil recovery in low permeability reservoirs. Sci. Rep. 12, 7785. doi: 10.1038/s41598-022-12025-7
Yassin, M. A., Elkhooly, T. A., Elsherbiny, S. M., Reicha, F. M., Shokeir, A. A. (2019). Facile coating of urinary catheter with bio-inspired antibacterial coating. Heliyon 5, e02986–e02986. doi: 10.1016/j.heliyon.2019.e02986
Zafer, M. M., Hussein, A. F. A., Al-Agamy, M. H., Radwan, H. H., Hamed, S. M. (2021). Genomic characterization of extensively drug-resistant NDM-producing acinetobacter baumannii clinical isolates with the emergence of novel bla ADC-257. Front. Microbiol. 12. doi: 10.3389/fmicb.2021.736982
Zhou, C., Wu, Y., Thappeta, K. R. V., Subramanian, J. T. L., Pranantyo, D., Kang, E. -T, et al. (2017). In vivo anti-biofilm and anti-bacterial non-leachable coating thermally polymerized on cylindrical catheter. ACS Appl. Materials Interfaces 9, 36269–36280. doi: 10.1021/acsami.7b07053
Keywords: Acinetobacter baumannii, global clones, endophytes, central venous catheter (CVC), biosurfactant, antibiofilm, Bacillus amyloliquefaciens, Papyrus
Citation: Amer MA, Wasfi R and Hamed SM (2023) Biosurfactant from Nile Papyrus endophyte with potential antibiofilm activity against global clones of Acinetobacter baumannii. Front. Cell. Infect. Microbiol. 13:1210195. doi: 10.3389/fcimb.2023.1210195
Received: 21 April 2023; Accepted: 20 June 2023;
Published: 13 July 2023.
Edited by:
Rachna Singh, Panjab University, IndiaReviewed by:
Ashraf Kariminik, Islamic Azad University Kerman, IranAnjna Kumari, Panjab University, India
Copyright © 2023 Amer, Wasfi and Hamed. This is an open-access article distributed under the terms of the Creative Commons Attribution License (CC BY). The use, distribution or reproduction in other forums is permitted, provided the original author(s) and the copyright owner(s) are credited and that the original publication in this journal is cited, in accordance with accepted academic practice. No use, distribution or reproduction is permitted which does not comply with these terms.
*Correspondence: Reham Wasfi, cndhc2ZpQG1zYS5lZHUuZWc=