- 1College of Animal and Veterinary Sciences, Southwest Minzu University, Chengdu, China
- 2Institute of Veterinary Drug and Feed Product Safety Testing, Zhengzhou Inspection and Testing Center of Product Quality, Zhengzhou, China
Introduction: To investigate the correlations among antibiotic resistance, virulence gene, phylogenetic group, and genetic diversity, providing essential data for Escherichia coli (E. coli) infection prevention and control in Tibetan pigs.
Methods: A total of 244 E. coli isolates were collected. Antimicrobial susceptibility was assessed using the microdilution method. PCR was used to detect antibiotic resistance genes (ARGs), virulence genes, and phylogenetic groups. Genetic diversity was analyzed using enterobacterial repetitive element sequence-based PCR. Enteroaggregative E. coli (EAEC) 5-12, a representative strain with multidrug resistance and strong biofilm-forming ability, harboring abundant virulence genes, was selected for whole-genome sequencing (WGS) to validate PCR results.
Results: Among the 244 isolates, 84.43% showed multidrug resistance (MDR), with the highest resistance rates for chloramphenicol (99.59%), sulfadiazine (96.31%), and sulfamethoxazole (93.85%). Twenty-five ARGs were detected, with ant(3’)-Ia, blaTEM, aac(3’)-II, floR, and qnrS exceeding 80% detection rates. Integrase genes intl1 and intl2 were found in 90.16% and 15.16% of isolates, respectively. Seventeen virulence genes were detected; bcsA (98.77%), fimC (89.75%), and agn43 (59.43%) were the most prevalent. A total of 106 virulence patterns were identified, with agn43/bcsA/fimC being predominant (17.92%). Most strains belonged to phylogenetic group A (45.90%), followed by B1 (34.43%), while 29 strains were unclassified. Sixty-four isolates were identified as diarrheagenic E. coli (DEC), predominantly enteroaggregative E. coli (EAEC, 90.63%). Biofilm-forming ability was categorized as strong (4.69%), moderate (21.88%), weak (59.38%), or absent (14.06%). Clustering based on 61.2% similarity grouped the 64 DEC into five clusters, with 84.38% in cluster II, which contained all strong biofilm producers.
Discussion: Antimicrobial resistance profiles of EAEC 5–12 confirmed that primarily confer resistance through antibiotic efflux, target alteration, and reduced permeability. These findings will contribute to further understanding the positive correlation between antibiotic resistance and pathogenicity in E. coli from Tibetan pig farms, shedding light on the rational use of antimicrobial agents and tackling the antibiotic resistance crisis in Tibetan pig breeding in Garze Tibetan Autonomous Prefecture, Sichuan, China.
1 Introduction
Escherichia coli (E. coli), a facultative anaerobe, is widely present in the gastrointestinal tracts of warm-blooded animals. When the immune response of the host is impaired, certain serotypes of E. coli can invade deeper tissues or organs, causing diseases such as diarrhea, pneumonia, and septicemia to acquire antibiotic resistance genes (ARGs) and virulence genes, adapt to environmental changes, and lead to gut microbiota disturbance (Geurtsen et al., 2022). Nearly 70% of antibiotics globally are used in animal production, with this widespread usage contributing significantly to bacterial resistance in pathogens like E. coli due to selective pressure and environment acquisition of resistance genes (Gasparrini et al., 2020; McKernan et al., 2021). China is the largest producer and user of antibiotics, with a large share applied in animal production and agriculture (Zhang et al., 2015). In 2023, E. coli isolated from Tibetan pigs in Nyingchi, Tibet, China, demonstrated resistance to multiple antibiotics (Cao et al., 2023). Similar resistance was observed in E. coli isolated from pigs, yak, beef and dairy cattle, reducing the efficacy of diminished the efficacy of β-lactams, quinolones, and aminoglycosides (Kylla et al., 2020; Wang et al., 2021).
Bacteria acquire resistance genes is a significant mechanism for the spread of resistance among bacteria. Integrons, a mobile genetic element, capture and integrate foreign genes, particularly resistance genes, through integrase enzymes (Hall and Collis, 1995).
E. coli acquires specific virulence factors through horizontal gene transfer and adaptive evolution, which enhances its adaptability and enables it to infect the host (Kaper et al., 2004; Arnold et al., 2022). Its pathogenicity is linked to virulence factors, typically encoded by genes on chromosomes, plasmids, or other genetic elements. Previous studies have shown that these factors are linked to gastrointestinal diseases (Singh et al., 2019).
E. coli is classified into phylogenetic groups based on the presence/absence of genes like chuA, yjaA, TspE4.C2, arpA, and trpA. Experimental results show differences in pathogenicity, phenotype, and genotype among strains from different phylogenetic groups (Tenaillon et al., 2010). Compared to other phylogenetic groups, E. coli in group B2 exhibits a longer survival time in the host’s intestine, which is suspected to be related to the virulence genes carried (Johnson and Kuskowski, 2000; Johnson and Stell, 2000; Escobar-Páramo et al., 2004). Potential pathogenic strains were also found in group D, while strains in groups A and B are mostly commensal E. coli (Picard et al., 1999; Duriez et al., 2001).
Tibetan pigs, physiologically adapted to high altitudes, are food animals vulnerable to outbreaks of fatal diarrhea caused by pathogenic E. coli, raising serious concerns for both animal and human health. Understanding the virulence genes, phylogroups, and phenotypic resistance characteristics in E. coli strains in Tibetan pigs is essential.
The linkages between virulence determinants and antibiotic resistance are still not clear despite several studies. It is important to assess the risk of antibiotic resistance and virulence factors on public health and this necessitates additional studies on such neglected food animals. This study aimed to investigate antibiotic resistance, virulence genes, and phylogenetic group distribution of E. coli isolated from Tibetan pig farms in Garze Tibetan Autonomous Prefecture, Sichuan, China. Findings provide foundational data on the relationship between antibiotic resistance and pathogenicity in E. coli from Tibetan pigs, supporting the rational use of antimicrobials and helping curb the spread of bacterial resistance in the Tibetan pig industry.
2 Materials and methods
2.1 Samples collection and reference strain
From 19 June to 6 October 2022, 301 samples (247 feces, 41 soil, and 23 water) were aseptically collected from Tibetan pigs in Garze Tibetan Autonomous Prefecture, Sichuan Province (Supplementary Table S1). Seven farms were sampled (Luding, 4 farms; Xiangcheng, 1 farm; and Daocheng, 4 farms) and samples were transported to Southwest Minzu University in ice-cooled containers for E. coli isolation and identification within 72 h. Anal swabs from normal animals, surface soil, and drain water were collected from intensive farms using sterile plastic bags. The reference strain ATCC25922 was kept in the Laboratory of Veterinary Pharmacology and Toxicology of Southwest Minzu University.
2.2 Antibacterial agents, medium and molecular biology reagents
Twenty-three antibacterial agents including Tetracyclines (Doxycycline, Oxytetracycline, Tetracycline), Sulfonamides (Sulfamethoxazole, Sulfadiazine), β-lactams (Amoxicillin/clavulanic acid, Ampicillin, Cefoxitin, Cefquinome, Oxacillin), Amphenicols (Chloramphenicol, Florfenicol), Rifamycins (Rifampin), Aminoglycosides (Gentamicin, Kanamycin, Spectinomycin, Streptomycin), Fluoroquinolones (Ciprofloxacin, Enrofloxacin, Nalidixic acid, Sarafloxacin), Polypeptides (Polymyxin B), Polyphosphates (Fosfomycin) were purchased from Shanghai YuanYe Biotechnology Co., Ltd. Tryptone soy broth (TSB), MacConkey agar (MAC), E. coli coliform chromogenic agar, Eosin methylene blue agar (EMB), Mueller-Hinton broth (MH), and Gram staining kits were provided by Qingdao Haibo Biotechnology Co., Ltd. molecular biology reagents for PCR including DL2000 DNA marker and 2×Taq Master Mix were purchased from Vazyme Biotech Co., Ltd (Nanjing, China).
2.3 Isolation, identification, and DNA extraction of E. coli
A suitable volume of environmental samples (0.2 g feces, 0.5 g soil, and 5 mL water) was placed into a test tube containing 5 mL of TSB and incubated for 12 h at 37°C. A loop of the bacterial suspension was streaked on MAC agar and incubated at 37°C for 12 h. Single colonies of moderate size with pink color were sequentially inoculated to EMB, E. coli/coliform color indicator medium, and MAC. The purified single colonies were subjected to the suspected E. coli via Gram staining and microscopic examination. PCR reaction targeting the β-glucosidase gene uidA (uidA-F: ATGCCAGTCCAGCGTTTTTGC and uidA-R: AAAGTGTGGGTCAATAATCAGGAAGTG) was amplified and the PCR products were subjected to the NCBI BLAST platform for sequence analysis.
DNA was extracted using the nuclease-free water boiling method and used as the DNA template in all the following molecular biological tests.
2.4 Antimicrobial sensitivity testing
The broth microdilution method used for the antimicrobial sensitivity profile and the obtained MICs (minimum inhibitory concentration) were interpreted according to the Clinical and Laboratory Standards Institute (CLSI) guidelines (CLSI, 2013). The reference strain ATCC25922 was used as a quality control. Briefly, dispense 100 µL of MH medium into each well in the columns from 1 to 12 and 100 µL in column 1 using a 300 µL electronic multichannel pipette (Eppendorf, Germany). Then, 100 µL of appropriate 4-fold concentrated stock solutions (5012 μg/mL) was pipetted into the wells in column 1 (antibiotic concentration will be diluted this way 1:1) and mixed, 100 µL of the mixed solutions in column 1 was withdrawn and moved into the column 2. Repeat the dilution procedure up to column 10 and additionally discard 100 µl of solutions from this last column. 100 µL of the suspension (106 CFU/mL) were inoculated in columns 1 to 10, columns 11 and 12 having a positive (no antibiotic) and negative growth control for medium sterility. Finally, transfer microtiter plates into the closable tray until inoculation is 12–16 h. Multi-drug resistance (MDR) was determined according to the reference (Magiorakos et al., 2021).
2.5 Detection of ARGs, integrase genes, and virulence genes
Specific primers for antibacterial resistance, integrase, and virulence genes were designed according to the literature (Supplementary Table 2) and synthesized by Sangon Biotech (Shanghai) Co., Ltd. Primers for the detection of ARGs and integrase genes were chosen based on the antibiotic categorizations. Virulence genes were chosen based on their functional characteristics. Singleplex PCR amplification reactions were carried out in 25 µL volumes comprising 2 µL genomic DNA, 12.5 µL 2×Taq Master Mix, 1 µL of each primer, and 8.5 µL ddH2O. The thermocycler conditions were as follows: denaturation at 95°C for 5 min followed by 35 cycles of denaturation at 95 °C for 30 s, variable annealing for 30 s, and extension at 72 °C for 15 s (Supplementary Tables S2 and S3). Finally, a final extension for 10 min at 72°C. The PCR products were analyzed using by 1% agarose gel electrophoresis staining with ethidium bromide. Concordance rates between drug-resistance phenotypes and genotypes were calculated, and the consistency rates of ARGs and integrase genes were assessed (An, 2024). Chi-Plot was used to investigate the possible association of two variables (https://www.chiplot.online/).
2.6 Phylogenetic analysis
Phylogenetic analysis of the isolated E. coli using a set of genes chuA, yjaA, TspE4.C2, arpA, arpAgpE, trpAgpC, trpBA were detected by multiplex PCR assays and the results were interpreted as previously described (Olivier et al., 2013). Primer information is listed in Supplementary Table S4. Quadruplex genotypes and steps for assigning E. coli isolates to phylogroups are listed in Supplementary Table S5.
2.7 Determination, genetic diversity, and biofilm formation of diarrheagenic E. coli (DEC)
DEC was determined according to the National Food Safety Standard GB/T 4789.6-2016 (National Health and Family Planning Commission of the People’s Republic of China, 2016). The criteria for diarrheagenic E. coli genetic typing are listed in Supplementary Table S6. ERIC-PCR typing was conducted to assess the genetic diversity of DEC by amplifying repetitive DNA sequences found in the intergenic regions and the primers information was shown in Supplementary Table S4.
ERIC-PCR reactions were performed in 25 μL volumes containing 1 μL of each primer, 12.5 μL of the master mix (CinnaGen, Iran), 2 μL of DNA template, and 8.5 μL of deionized water. The ERIC-PCR reaction program was performed following initial denaturation at 95°C for 5 min, with the next 35 cycles consisting of a denaturation step at 95°C for 1 min, annealing at 53°C for 1 min, extension at 72°C for 4 min, and a final extension for 10 min at 72°C. 5 μL of ERIC-PCR products were loaded on 1% agarose gel and conducted at 120 V for 30 min. Meanwhile, a 100-base pair-DNA marker was used as a standard measuring means. DNA bands were visualized with a ChemiDoc MP Imaging System (BIO-RAD).
ERIC-PCR bands were used to construct a dendrogram by converting into a binary matrix where each band is either present (1) or absent (0) for each isolate. The dendrogram for the 64 strains was constructed in NTSYS software using the Unweighted Pair Group Method with the Arithmetic mean (UPGMA) method.
The biofilm-forming ability of DEC was semi-quantitative determined using crystal violet assay (Chen et al., 2021), and the optical density threshold value (ODC) was set as the average value of the negative control plus three standard deviations. The biofilm formation ability was categorized as follows: OD570nm ≤ ODC indicates no biofilm formation, ODC < OD570nm ≤ 2 ODC indicates weak biofilm formation, 2 ODC < OD570nm ≤ 4ODC indicates moderate biofilm formation, and D570nm > 4 DC indicates strong biofilm formation.
2.8 Whole genome sequencing of EAEC 5-12
To confirm the presence of resistance-related genes and allelic variations in EAEC 5-12(multidrug resistance and strong biofilm-forming ability, harboring abundant virulence genes), the amino acid sequence from WGS data was analyzed using CARD’s Resistance Gene Identifier (RGI) software. A single colony was selected for enrichment in a 5 mL aliquot of TSB, and bacterial culture at the logarithmic phase was centrifuged at 4000 g for 10 min at 4°C. The supernatant was discarded, and the pellet was washed twice with sterile water. Samples were shipped with dry ice for Illumina sequencing (Beijing Novogene Bioinformatics Technology Co., Ltd). After sequencing and gene function prediction, the antimicrobial resistance genes were verified against the NCBI AMRFinderPlus database. For virulence genes, DIAMOND software was used to compare the non-redundant gene set with the VFDB core database, applying a cut-off of 80% identity and 70% coverage (parameters: BLASTP; E-value ≤ 1e-5).
2.9 Statistics and data analysis
Statistical analysis was performed using WHONET 5.6 and IBM SPSS Statistics 29. The chi-squared test and Fisher’s exact test were used to analyze differences, with P< 0.05 considered statistically significant.
3 Results
3.1 Isolation and identification of E. coli
Species identification was conducted using a combination of morphological and molecular biological methods (Figures 1a-c). The E. coli marker gene uidA was detected in 81.06% (244/301) of strains, with a molecular weight of approximately 1487 bp (Figure 1d). Of these, 64 strains (26.23%, 64/244) were identified as DEC. Among the isolates, the percentage of E. coli isolated from feces, soil, and water was 72.09% (217/301), 2.33% (7/301), and 6.64% (20/301), respectively.
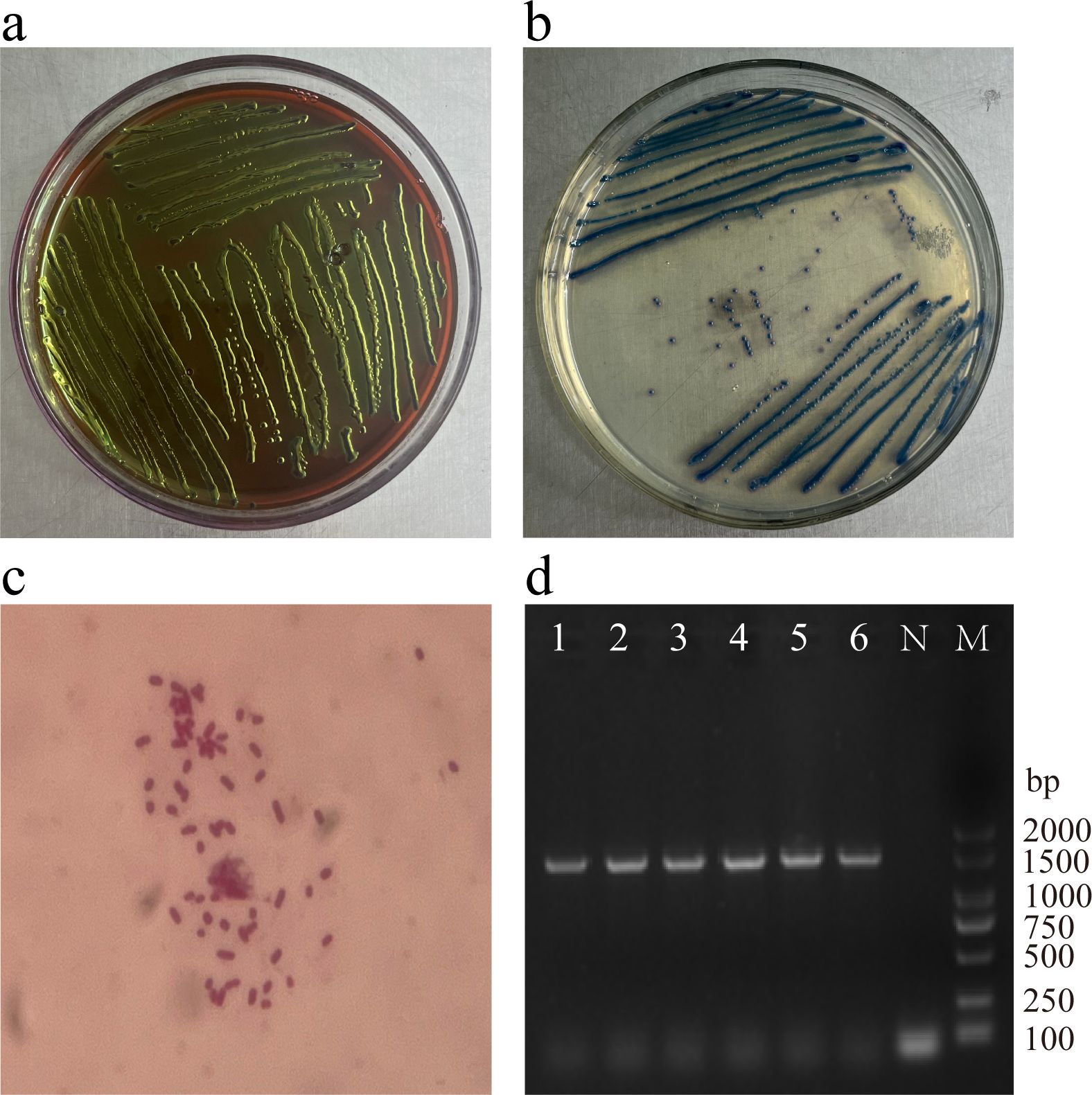
Figure 1. Identification of E. coli by combining morphological and molecular biological methods. (a) Colonies showing greenish metallic sheen on EMB agar; (b) Dark blue to violet colonies on E. coli coliform chromogenic agar; (c) E coli Colony Gram staining (1000×); (d) E. coli marker gene uidA (Lane 1-6: Representative strains. N, Negative control; M, DL 2000 DNA Marker).
3.2 Antimicrobial resistance and multi-drug resistance analysis
The antimicrobial sensitivity testing of 244 E. coli strains against 23 antibiotics is summarized in Table 1. The strains exhibited resistance to various antibiotics, including aminoglycosides, sulfonamides, and tetracyclines, with resistance rates exceeding 50%. The highest resistance rates were observed for CHL, SMZ, and SMX, posing 99.59%, 96.31%, and 93.85%, respectively. However, the isolated strains were sensitive to PLB (93.85%), CEF (91.8%), TIO (88.52%), CIP (88.11%), GEN (87.3%), KAM (85%). 84.43% (206/244) of the E. coli strains displayed MDR, with the highest proportion (32.52%, 67/206) being resistant to five classes of antibiotics (Figure 2).
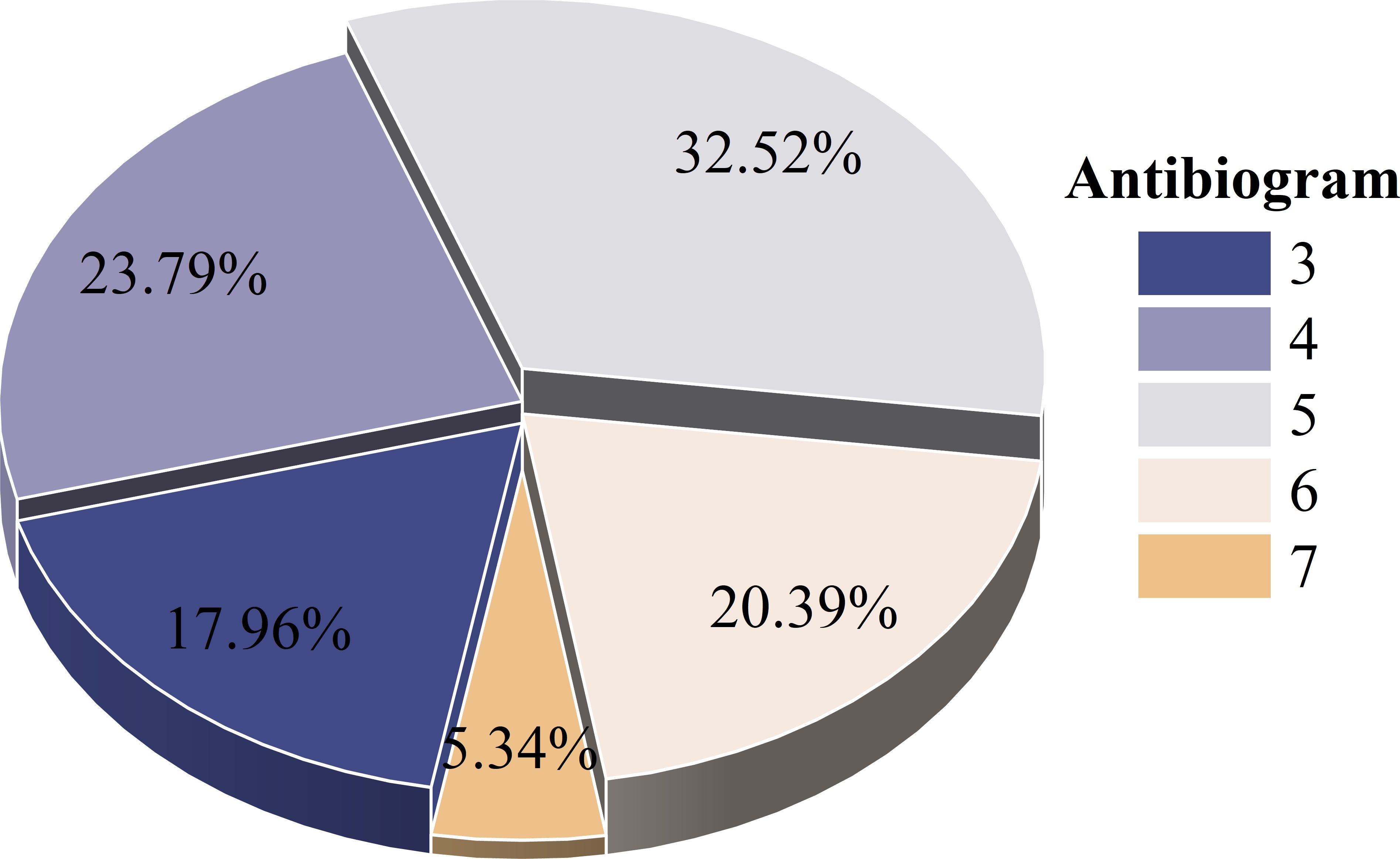
Figure 2. Antibiogram of MDR E. coli. (3–7 means that resistance to 3–7 kinds of antibiotics was tested).
Among the seven sampling sites, strains from Shangkuiwu Village and Lamu Village exhibited higher resistance rates to aminoglycosides and sulfonamides, followed by tetracyclines and some β-lactams. Resistant strains of all 23 antibiotics were detected in these villages. The resistance profiles of the other five sites were similar (Figure 3). Regional differences in the detection rates of antimicrobial agents were observed, with statistical significance (P< 0.05) for agents such as Ciprofloxacin, Gentamicin, and Sarafloxacin (Supplementary Table S7).
3.3 Detection of ARGs, integrase genes, and virulence genes
Twenty-five ARGs were positively detected (Figures 4, 5). The predicted fragment length (bp) and optimized annealing temperature (°C) are listed in Supplementary Table S2. For ARGs, detection rates exceeded 80% for ant (3’)-Ia (93.44%, 228/244), blaTEM (93.03%, 227/244), aac (3’)-II (91.80%, 224/244), floR (88.52%, 216/244), qnrS (86.07%, 210/244), sul3 (81.97%, 200/244), and cmlA (81.56%, 199/244). Correlation analysis between ARGs and phenotypes indicated a significant positive association between strains carrying ARGs and those without (Supplementary Table S8).
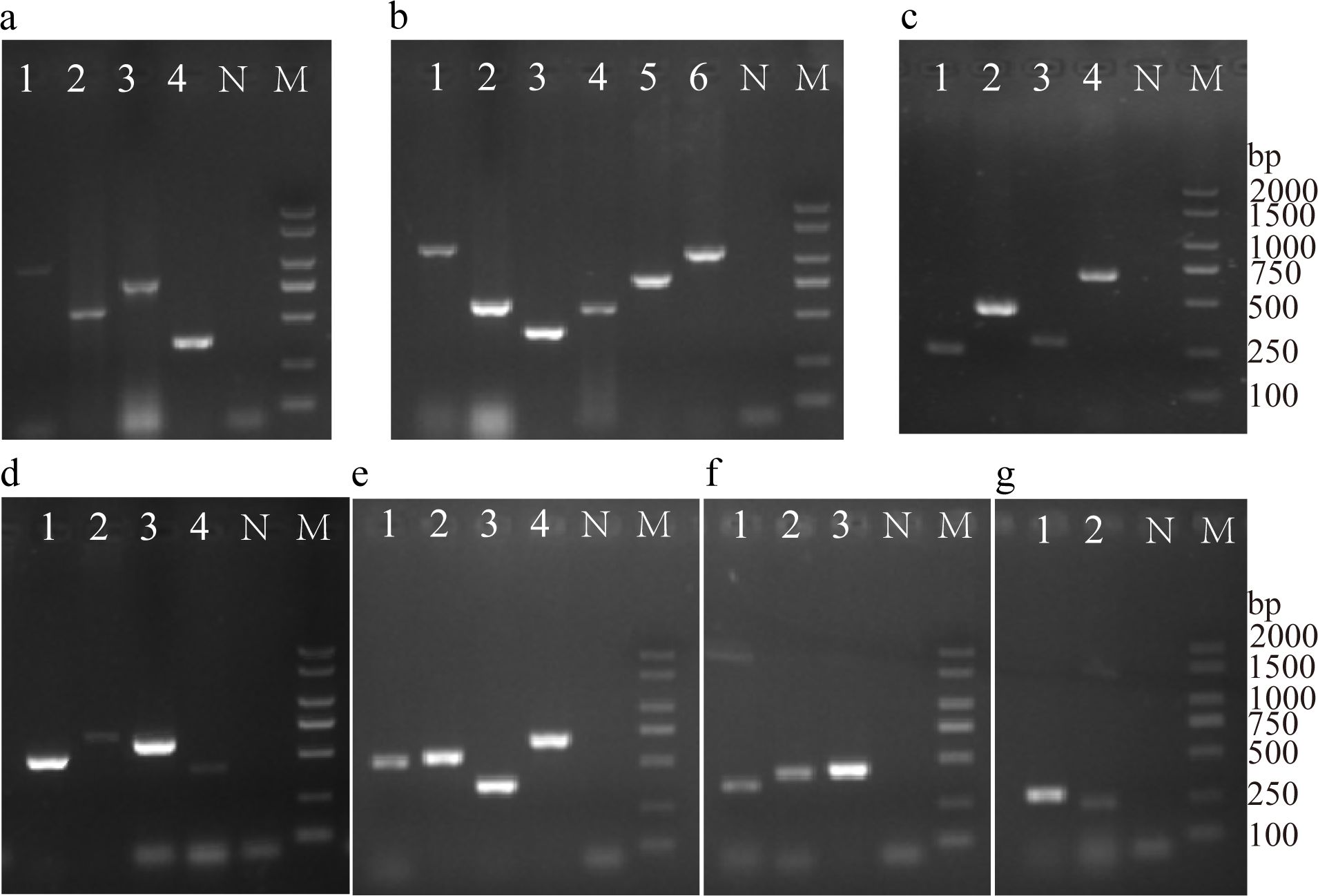
Figure 4. Electrophoresis of PCR amplification products of antibacterial resistance genes and integrase genes. (a) Tetracyclines (1-4, tetA, tetB, tetD, tetM); (b) β-lactams (1-6, blaCMY-2, blaCTX-M-U, blaDHA, blaOXA, blaSHV, blaTEM); (c) Aminoglycosides (1-4, aac(3’)-II, aadA2, ant(3’)-Ia, aph(3’)-VII); (d) Quinolones (1-4, aac(6’)-Ib, qnrA, qnrD, qnrS).; (e) Amphenicols (1-4, cat1, cat2, cmlA, floR); (f) Sulfonamides (1-3, sul1, sul2, sul3); (g) Integrase (1-2, intl1, intl2). (N, Negative control; M, DL 2000 DNA Marker).
The detection rates of intl1 and intl2 were 90.16% (220/244), and 15.16% (37/244), respectively. 14.34% (35/244) of the strains carried both intl1 and intl2 genes, and 9.02% (22/244) did not carry the intl1 and intl2 genes. Correlation analysis between ARGs and integrase genes was evaluated and shown in Supplementary Table S9. Overall, the consistency rate of the integrase gene intl1 was higher than that of intl2, and the detection rate of ARGs in strains carrying integrase genes differed significantly from the strains not carrying integrase genes.
Among the 17 positively detected virulence genes (Figure 6), the highest detection rates for bcsA, fimC, and agn43 genes were 98.77% (241/244), 89.75% (219/244), 59.43% (145/244), respectively. However, none of the isolates were positive for afa, bcsB, exhA, papC, stx1 and vat. The 244 E. coli posed106 virulence genotypes, exhibited the highest number of 11 virulence genes (agn43, astA, bcsA, colV, eaeA, fimC, hlyF, iss, ompT, sitA, tsh) and the lowest number of 1 virulence gene (bcsA). The predominant virulence pattern was agn43/bcsA/fimC (17.92%, 19/106) summarized in Supplementary Table S10.
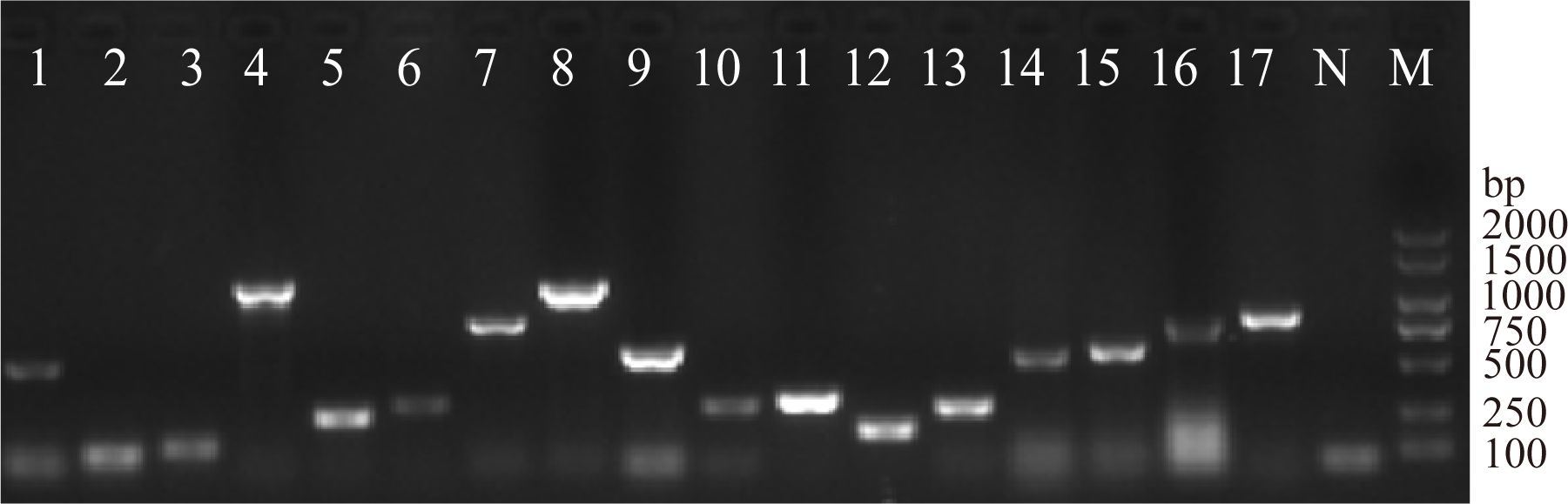
Figure 6. Electropherogram of the PCR amplification product of virulence genes. Lane 1, agn43; Lane 2, astA; Lane 3, bcsA; Lane 4, colV; Lane 5, eaeA; Lane 6, fimC; Lane 7, fyuA; Lane 8, hlyA; Lane 9, hlyF; Lane 10, irp2; Lane 11, iss; Lane 12, ler; Lane 13, LT; Lane 14, ompT; Lane 15, sitA; Lane 16, stx2; Lane 17, tsh; (N, Negative control; M, DL 2000 DNA Marker).
3.4 Phylogenetic analysis
Of the 244 strains, 45.90% (112/244) mainly belonged to group A, followed by group B1(34.43%), group B2(0.82%), group C (4.10%), group D (0.82%), group E (0.41%), and Clade I (1.64%). However, 29 strains could not be classified into any phylogenetic clusters (Supplementary Figure S1). Figure 7 revealed the distribution of virulence genes in 244 E. coli among the different phylogenetic groups, the most prevalent genes in group A were agn43, bcsA, eaeA, fimC, fyuA, irp2, sitA, and stx2, and those of B1 were higher in genes astA, colV, hlyA, hlyF, iss, ompT, and tsh. For ler and LT, the predominant groups were located in D and Clade I, respectively.
A total of 64 strains were screened and identified as DEC, the most predominant pathotype belonged to enteroaggregative E. coli (EAEC), accounting for 90.63% (58/64). Followed by enteropathogenic E. coli (EPEC), enterohemorrhagic E. coli (EHEC), and enterotoxigenic E. coli (ETEC), accounting for 4.69% (3/64), 3.12% (2/64), 1.56% (1/64), respectively. No enteroinvasive E. coli (EIEC) were identified in this study. Our results also revealed that EAEC strains (EAEC5-12) harbored various virulence genes relating to biofilm formation with a higher detection rate of virulence genes (Figure 8).
Among the 64 DEC isolates, most isolates exhibited moderate or weak biofilm-forming ability. Namely, 4.69% (3/64), 21.88% (14/64), 59.38% (38/64), and 14.06% (9/64) were classed as strong, moderate, weak, and absent producers of biofilm.
ERIC patterns based on molecular weight and markers were observed. Isolates with one to three similar or different band patterns were grouped, while those with differences in more than three bands were classified into separate types. Based on 61.2% similarity among strains, dendrograms grouped the 64 DEC isolates into five clusters (I, II, III, IV, and V). Of these, 84.38% were in cluster II, where all strong biofilm-forming strains were located (Table 2, Supplementary Figure 2).
3.5 Whole-genome sequencing
The detected antibiotic resistance profiles for EAEC5–12 largely agreed with the MIC results. Based on the verification against the NCBI AMRFinderPlus database, a total of 120 ARGs were ultimately selected, including those for tetracyclines, aminoglycosides, fluoroquinolones, glycopeptides, and macrolides, 71.67% were involved in antibiotic efflux, 14.17% were involved in antibiotic target alteration, and 15.16% were involved in multiple resistance mechanisms simultaneously (Figure 9).
Gene function annotations for EAEC 5–12 virulence genes showed 486 annotated virulence genes, including functions in adherence, antimicrobial activity/competitive advantage, biofilm formation, effector delivery systems, and others (Figure 10).
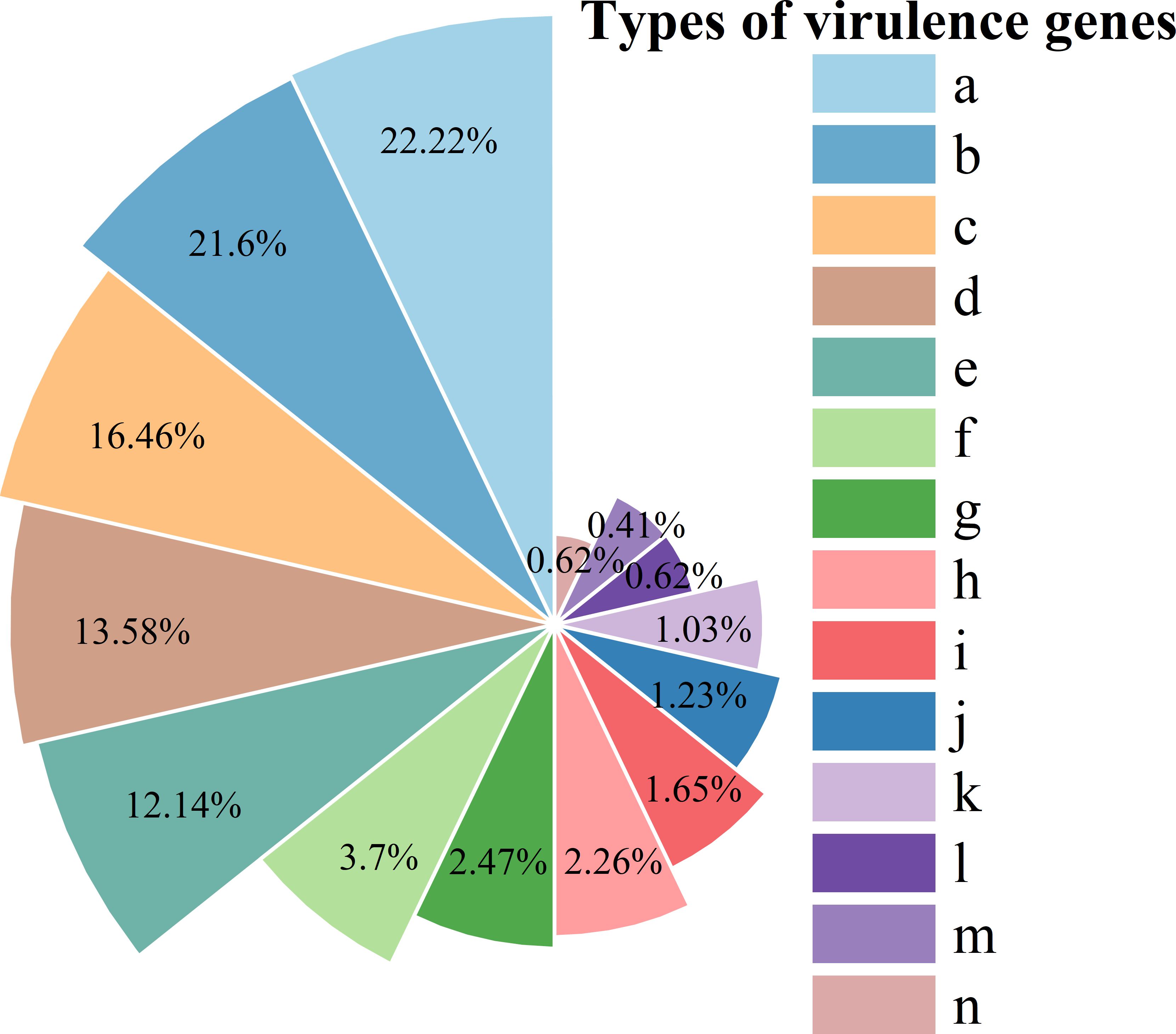
Figure 10. Classification of virulence genes. (a) adherence; (b) nutritional/metabolic factor; (c) immune modulation; (d) effector delivery system; (e) motility; (f) regulation; (g) antimicrobial activity/competitive advantage; (h) biofilm; (i) stress survival; (j) exotoxin; (k) others; (l) exoenzyme; (m) invasion; (n) post-translational modification.
4 Discussion
Bacterial resistance is a growing global issue, with MDR problems becoming increasingly severe. Economic and regulatory challenges contribute to the high prevalence of MDR bacteria in some developing countries. Given the limited research on antimicrobial resistance in Tibetan pigs, in this study, we observed a higher prevalence of multidrug-resistant E. coli with virulence factors in Tibetan pigs and the environmental samples (soil and drain water), providing a basis for comparison with reports from other regions and animal populations.
In this study, 84.43% of the 244 E. coli isolates from Tibetan were MDR and were resistant to 23 antibiotics with high resistance to sulfonamides, amphenicols, tetracyclines, and β-lactams, emphasizing the urgent need to improve drug management and avoid unnecessary antibiotic use in Tibetan pig farms. The result is aligned with a study in Bangladesh, where 98% of the tested strains were MDR (Jain et al., 2021) and another report indicates that resistance rates vary significantly by region and sample types (An, 2024).
Tibetan pig farming is a key economic sector in Garze Tibetan Autonomous Prefecture, Sichuan Province. According to the drug use information provided by the farmers, Ampicillin, Florfenicol, and Doxycycline are the most commonly used antibiotics in Tibetan pig farming due to their low cost and effectiveness. Data indicates that over 75% of tetracycline antibiotics or their metabolites enter the environment through waste, increasing selective pressure and promoting antibacterial resistance (Xu et al., 2021; Larsson and Flach, 2022). In early livestock practices, sulfonamides were often combined with β-lactams and tetracyclines (Sánchez-Osuna et al., 2018). Compared with other antibiotics, tetracyclines and sulfonamides are more readily adsorbed by soil and other natural organic matter (Kumar et al., 2005). Thus, surveillance is urgent to avoid the dissemination of antibiotic resistance in Garze Tibetan Autonomous Prefecture, Sichuan Province, China.
Bacterial resistance arises from multiple factors, necessitating a comprehensive, multifaceted approach to understanding its spread. The mobility of resistance genes enables their transfer between bacterial species via mobile genetic elements, facilitating the spread of pathogenic resistance genes (Mmh et al., 2021). Integrases are one of the major causes of multidrug resistance in gram-negative bacteria. Strains with class I integrase exhibit distinct resistance profiles compared to those lacking class I integrase (Sunde and Norström, 2006). We detected 41 ARGs associated with tetracyclines, β-lactams, aminoglycosides, fluoroquinolones, amphenicols, and sulfonamides, and 25 were positively detected in this study. Detection rates of ARGs were similar to a previous study, where resistance gene detection rates for β-lactams, aminoglycosides, tetracyclines, amphenicols, macrolides, sulfonamides, and polymyxins ranged from 50% to 96% (Peng et al., 2021). A study in India also found that isolates from various animals and their handlers carry multiple resistance genes (Mitra et al., 2024). Integrase genes, intl1 and intl2, have also been detected in pigs, chickens, and cattle (Wang et al., 2019; Yang et al., 2020). We also detected integrase genes, intl1 and intl2, with detection rates of 90.16% and 15.16%, respectively. Our results revealed a strong correlation between resistance genes and resistance phenotypes, with a significant difference between integrase gene-positive and gene-negative strains, highlighting the necessity to monitor integrase genes to prevent worsening antimicrobial resistance in Tibetan pigs.
In this experimental design, we detected 23 virulence genes, the results showed that 17 virulence genes (agn43, astA, bcsA、colV, eaeA, fimC, fyuA, hlyA, hlyF, irp2, iss, ler, LT, ompT, sitA, stx2, tsh) were positively detected, while 6 genes (afa, bcsB, ehxA, papC, stx1, and Vat) were not detected. Overall, our results indicate that most E. coli isolates are diarrheagenic pathotypes. Nevertheless, the occurrence of single or multiple virulence factors does not essentially signify its pathogenicity. Further studies via animal models or tissue cultures are needed to confirm the pathogenicity based on the observed virulence genotypes. Among the detected virulence genes, bcsA, fimC, and agn43 had the highest detection rates, with the agn43/bcsA/fimC combination being the most prevalent genotype, accounting for 17.92% (19/106). Agn43 encodes an adhesin that bacterial attachment to host cells (Sora et al., 2021). BcsA encodes bacterial cellulose synthase, which aids in biofilm formation and promotes adherence to host cells (Castiblanco and Sundin, 2018). FimC encodes the Type I flagellum adhesin, an adhesion factor critical for fimbriae synthesis; mutations in fimC can impair fimbriae formation (Gahlot et al., 2022). The above three virulence genes are all associated with biofilm formation, suggesting that E. coli isolated from Tibetan pigs may exert pathogenic effects through biofilm formation. The relationship between biofilm formation and virulence gene expression is needed in our future work.
Sixty-four strains were identified as DEC, with EAEC showing the highest isolation rate. Most of the 17 virulence genes were found in EAEC, likely due to its high prevalence. Phylogenetic analysis showed that the 17 detected virulence genes are mainly distributed in groups A and B1, aligning with Rehman et al.’s finding that virulence genes are predominantly found in group A (Rehman et al., 2017). Notably, studies have found that human-derived E. coli in group B2 is associated with the virulence genes fimH, irp2, kpsMTII (Monroy-Pérez et al., 2020). The discrepancies in these findings may be attributed to animal groups or geographical origin.
In this study, we developed a rapid and simple method to investigate the relationship between ERIC-PCR typing and biofilm formation. ERIC-PCR typing identified 11 separating DNA fragments of varying sizes ranging from 300 bp to 2000 bp. Based on a genetic similarity of 61.2%, 84.38% of the DEC were classified as cluster II, with all five clusters showing weak biofilm-forming ability. A study in Iran also found that DNA fragments of varying sizes of 115 tested strains ranged from 380 bp to 3280 bp, with different band distributions depending on the animal source: chicken samples primarily concentrated around 2800 bp, while sheep and cattle samples focused around 1200 bp (Ranjbar et al., 2017).
A total of 120 ARGs were predicted from CARD in EAEC 5-12, including genes for tetracyclines (tetA, tetB, tetD, and tetT), aminoglycosides (aadA5, aph (3’’)-Ib, and aph(6)-Id), fluoroquinolones (mfd, gyrA, and gyrB), amphenicols (cat1, cmlV, and mexN), β-lactams (CMY-63, mecC, and ompK37), and sulfonamides (sul2 and sul3). Additionally, resistance genes related to efflux pumps, macrolides, glycopeptides, and lacosamide were also predicted, and most were associated with efflux pumps. Although EAEC 5–12 is sensitive to PLB, it is predicted to carry the MCR-3 gene.
Adhesins are key proteins that facilitate pathogen-host binding, and the genes (fimA, fimB, fimC, etc.) carried by EAEC 5–12 play a role in the pathogen’s colonization process. The entA, entB, and fepA genes in EAEC 5–12 are nutritional/metabolic factors that supply essential nutrients to the pathogen. The ompA, LPS, and pbpG genes are involved in immune regulation, modulating the immune response to the pathogen. Through WGS and VFDB alignment analysis, fourteen virulence genes were predicted in EAEC 5-12, primarily including adhesins, nutritional/metabolic factors, and immune regulators. Most of the virulence genes mentioned above are linked to biofilm formation and pathogenicity, suggesting that effectively inhibiting biofilm formation could be a strategy to reduce pathogenicity in bacterial infections.
A limitation of this study is the small sample size, and our future work should increase sample sizes for WGS. Another important issue to address is that a systematic and comprehensive reference for the research on antimicrobial resistance based on machine learning methods and data mining techniques is urgent in our future work.
5 Conclusion
The present study highlights the important role of the Tibetan pigs as a potential reservoir of multidrug-resistant E. coli carrying a variety of virulence genes that evoke public health problems by spreading into the environment. The association between antibiotic resistance and virulence genes reveals that virulence characteristics in Tibetan pigs might be selected by antibiotic usage in Tibetan pig farms. In summary, it is urgent to enhance surveillance response systems for monitoring the rational use of antimicrobial agents in Tibetan pigs in Garze Tibetan Autonomous Prefecture, Sichuan province, China.
Data availability statement
The raw data supporting the conclusions of this article will be made available by the authors, without undue reservation.
Ethics statement
The animal studies were approved by Animals Care and Ethics Committee of Southwest Minzu University (Approval code: SMU- CAVS-240902001). The studies were conducted in accordance with the local legislation and institutional requirements. Written informed consent was obtained from the owners for the participation of their animals in this study.
Author contributions
XZ: Conceptualization, Data curation, Formal analysis, Methodology, Software, Validation, Visualization, Writing – original draft, Writing – review & editing. JL: Funding acquisition, Writing – review & editing. ZX: Data curation, Formal analysis, Software, Writing – review & editing. CC: Conceptualization, Funding acquisition, Resources, Supervision, Writing – review & editing.
Funding
The author(s) declare that financial support was received for the research and/or publication of this article. This work was funded by the Qinghai Provincial Model County Special Project for Rural Revitalization Science and Technology (2024-NK-X02) and Fundamental Research Funds for Central Universities, Southwest Minzu University (2025ZYN2025095).
Conflict of interest
The authors declare that the research was conducted in the absence of any commercial or financial relationships that could be construed as a potential conflict of interest.
Generative AI statement
The author(s) declare that no Generative AI was used in the creation of this manuscript.
Publisher’s note
All claims expressed in this article are solely those of the authors and do not necessarily represent those of their affiliated organizations, or those of the publisher, the editors and the reviewers. Any product that may be evaluated in this article, or claim that may be made by its manufacturer, is not guaranteed or endorsed by the publisher.
Supplementary material
The Supplementary Material for this article can be found online at: https://www.frontiersin.org/articles/10.3389/fcimb.2025.1526028/full#supplementary-material
References
An, S. (2024). Study on drug resistance genes of Klebsiella pneumoniae clinical isolates in Jilin Province. [master’s thesis]. Jilin Province: Jilin University. doi: 10.27162/d.cnki.gjlin2023.002349
Arnold, B. J., Huang, I. T., Hanage, W. P. (2022). Horizontal gene transfer and adaptive evolution in bacteria. Nat. Rev. Microbiol. 20, 206–218. doi: 10.1038/s41579-021-00650-4
Cao, Z., Qi, M., Shang, P., Zhang, H., Nawaz, S., Ghaffar, A., et al. (2023). Characterization, estimation of virulence and drug resistance of diarrheagenic Escherichia coli (DEC) isolated from Tibetan pigs. Microb. Pathog. 177, 106046. doi: 10.1016/j.micpath.2023.106046
Castiblanco, L. F., Sundin, G. W. (2018). Cellulose production, activated by cyclic di-GMP through BcsA and BcsZ, is a virulence factor and an essential determinant of the three-dimensional architectures of biofilms formed by Erwinia amylovora Ea1189. Mol. Plant Pathol. 19, 90–103. doi: 10.1111/mpp.12501
Chen, C., Li, Y., Tan, M., Wang, L., Huang, Z. (2021). Biofilm-forming phenotype, antibacterial resistance genes, integrase genes and virulence genes detection of Escherichia coli isolated from yaks and Tibetan pigs in northwest Sichuan Plateau. Agricultural Sciences in China. 54, 5144–5162. doi: 10.3864/j.issn.0578-1752.2021.23.018
Clinical Laboratory Standards Institute (2013). Performance standards for antimicrobial susceptibility testing; Twenty-third informational supplement (Wayne, PA: Clinical Laboratory Standards Institute (CLSI document M100-S23).
Duriez, P., Clermont, O., Bonacorsi, S., Bingen, E., Chaventré, A., Elion, J., et al. (2001). Commensal Escherichia coli isolates are phylogenetically distributed among geographically distinct human populations. Microbiol. (Reading) 147, 1671–1676. doi: 10.1099/00221287-147-6-1671
Escobar-Páramo, P., Clermont, O., Blanc-Potard, A.-B., Bui, H., Le Bouguénec, C., Denamur, E. (2004). A specific genetic background is required for acquisition and expression of virulence factors in Escherichia coli. Mol. Biol. Evol. 21, 1085–1094. doi: 10.1093/molbev/msh118
Gahlot, D. K., Taheri, N., MacIntyre, S. (2022). Diversity in genetic regulation of bacterial fimbriae assembled by the chaperone usher pathway. Int. J. Mol. Sci. 24, 161. doi: 10.3390/ijms24010161
Gasparrini, A. J., Markley, J. L., Kumar, H., Wang, B., Fang, L., Irum, S., et al. (2020). Tetracycline-inactivating enzymes from environmental, human commensal, and pathogenic bacteria cause broad-spectrum tetracycline resistance. Commun. Biol. 3, 241. doi: 10.1038/s42003-020-0966-5
Geurtsen, J., de Been, M., Weerdenburg, E., Zomer, A., McNally, A., Poolman, J. (2022). Genomics and pathotypes of the many faces of Escherichia coli. FEMS Microbiol. Rev. 46, fuac031. doi: 10.1093/femsre/fuac031
Hall, R. M., Collis, C. M. (1995). Mobile gene cassettes and integrons: capture and spread of genes by site-specific recombination. Mol. Microbiol. 15, 593–600. doi: 10.1111/j.1365-2958
Jain, P., Bepari, A. K., Sen, P. K., Rafe, T., Imtiaz, R., Hossain, M., et al. (2021). High prevalence of multiple antibiotic resistance in clinical E. coli isolates from Bangladesh and prediction of molecular resistance determinants using WGS of an XDR isolate. Sci. Rep. 11, 22859. doi: 10.1038/s41598-021-02251-w
Johnson, J. R., Kuskowski, M. (2000). Clonal origin, virulence factors, and virulence. Infect. Immun. 68, 424–425. doi: 10.1128/IAI.68.1.424-425.2000
Johnson, J. R., Stell, A. L. (2000). Extended virulence genotypes of Escherichia coli strains from patients with urosepsis in relation to phylogeny and host compromise. J. Infect. Dis. 181, 261–272. doi: 10.1086/315217
Kaper, J. B., Nataro, J. P., Mobley, H. L. (2004). Pathogenic escherichia coli. Nat. Rev. Microbiol. 2, 123–140. doi: 10.1038/nrmicro818
Kumar, K., Gupta, C., Chander, Y., Singh, A. K. (2005). Antibiotic use in agriculture and its impact on the terrestrial environment. Adv. Agron. 87, 1–54. doi: 10.1016/S0065-2113(05)87001-4
Kylla, H., Dutta, T. K., Roychoudhury, P., Subudhi, P. K., Lalhruaipuii, Lalsiamthara, J., et al. (2020). Characterization of porcine Enteropathogenic Escherichia Coli isolated in Northeastern India. J. Vet. Res. 64, 391–397. doi: 10.2478/jvetres-2020-0046
Larsson, D. G. J., Flach, C. F. (2022). Antibiotic resistance in the environment. Nat. Rev. Microbiol. 20, 257–269. doi: 10.1038/s41579-021-00649-x
Magiorakos, A.-P., Srinivasan, A., Carey, R. B., Carmeli, Y., Falagas, M. E., Giske, C. G., et al. (2021). Multidrug-resistant, extensively drug-resistant and pandrug-resistant bacteria: an international expert proposal for interim standard definitions for acquired resistance. Clin. Microbiol. Infect. 18, 268–281. doi: 10.1111/j.1469-0691.2011.03570.x
McKernan, C., Benson, T., Farrell, S., Dean, M. (2021). Antimicrobial use in agriculture: critical review of the factors influencing behavior. JAC Antimicrob. Resist. 3, dlab178. doi: 10.1093/jacamr/dlab178
Mitra, S. D., Shome, R., Bandopadhyay, S., Geddam, S., Kumar, A. M. P., Murugesan, D., et al. (2024). Genetic insights of antibiotic resistance, pathogenicity (virulence) and phylogenetic relationship of Escherichia coli strains isolated from livestock, poultry and their handlers - a one health snapshot. Mol. Biol. Rep. 51, 404. doi: 10.1007/s11033-024-09354-3
Mmh, E., M., A., P., L., Moa, S. (2021). Forecasting the dissemination of antibiotic resistance genes across bacterial genomes. Nat. Commun. 12, 2435. doi: 10.1038/s41467-021-22757-1
Monroy-Pérez, E., Cerón, A. B., Cortés, L. R. G., Alonso, N. N., Domínguez-Trejo, P., Hernández-Jaimes, T., et al. (2020). Virulence gene transcription, phylogroups, and antibiotic resistance of cervicovaginal pathogenic E. coli in Mexico. PloS One 15, e0234730. doi: 10.1371/journal.pone.0234730
National Health and Family Planning Commission of the People’s Republic of China. (2016). National food safety standard GB/T 4789.6-2016. Beijing: Standards Press of China.
Olivier, C., Julia K, C., Erick, D., David, M. (2013). The Clermont Escherichia coli phylo-typing method revisited: improvement of specificity and detection of new phylo-groups. Environ. Microbiol. Rep. 5, 58–65. doi: 10.1111/1758-2229.12019
Peng, J., Balasubramanian, B., Ming, Y., Niu, J., Yi, C., Ma, Y., et al. (2021). Identification of antimicrobial resistance genes and drug resistance analysis of Escherichia coli in the animal farm environment. J. Infect. Public Health 14, 1788–1795. doi: 10.1016/j.jiph.2021.10.025
Picard, B., Garcia, J. S., Gouriou, S., Duriez, P., Brahimi, N., Bingen, E., et al. (1999). The link between phylogeny and virulence in Escherichia coli extraintestinal infection. Infect. Immun. 67, 546–553. doi: 10.1128/IAI.67.2.546-553.1999
Ranjbar, R., Tabatabaee, A., Behzadi, P., Kheiri, R. (2017). Enterobacterial repetitive intergenic consensus polymerase chain reaction (ERIC-PCR) genotyping of Escherichia coli strains isolated from different animal stool specimens. Iran J. Pathol. 12, 25–34. doi: 10.30699/ijp.2017.21506
Rehman, M. U., Zhang, H., Iqbal, M. K., Mehmood, K., Huang, S., Nabi, F., et al. (2017). Antibiotic resistance, serogroups, virulence genes, and phylogenetic groups of Escherichia coli isolated from yaks with diarrhea in Qinghai Plateau, China. Gut Pathog. 9, 24. doi: 10.1186/s13099-017-0174-0
Sánchez-Osuna, M., Cortés, P., Barbé, J., Erill, I. (2018). Origin of the mobile dihydropteroate synthase gene determining sulfonamide resistance in clinical isolates. Front. Microbiol. 9, 3332. doi: 10.3389/fmicb.2018.03332
Singh, T., Singh, P. K., Das, S., Wani, S., Jawed, A., Dar, S. A. (2019). Transcriptome analysis of beta-lactamase genes in diarrheagenic Escherichia coli. Sci. Rep. 9, 3626. doi: 10.1038/s41598-019-40279-1
Sora, V. M., Meroni, G., Martino, P. A., Soggiu, A., Bonizzi, L., Zecconi, A. (2021). Extraintestinal pathogenic Escherichia coli: virulence factors and antibiotic resistance. Pathog 10, 1355. doi: 10.3390/pathogens10111355
Sunde, M., Norström, M. (2006). The prevalence of, associations between and conjugal transfer of antibiotic resistance genes in Escherichia coli isolated from Norwegian meat and meat products. J. Antimicrob. Chemother. 58, 741–747. doi: 10.1093/jac/dkl294
Tenaillon, O., Skurnik, D., Picard, B., Denamur, E. (2010). The population genetics of commensal Escherichia coli. Nat. Rev. Microbiol. 8, 207–217. doi: 10.1038/nrmicro2298
Wang, L., Wang, J., Wang, J., Zhu, L., Yang, L., Yang, R. (2019). Distribution characteristics of antibiotic resistant bacteria and genes in fresh and composted manures of livestock farms. Sci. Total Environ. 695, 133781. doi: 10.1016/j.scitotenv.2019.133781
Wang, W., Wei, X., Wu, L., Shang, X., Cheng, F., Li, B., et al. (2021). The occurrence of antibiotic resistance genes in the microbiota of yak, beef and dairy cattle characterized by a metagenomic approach. J. Antibiot 74, 508–518. doi: 10.1038/s41429-021-00425-2
Xu, L., Zhang, H., Xiong, P. (2021). Occurrence, fate, and risk assessment of typical tetracycline antibiotics in the aquatic environment: a review. Sci. Total Environ. 753, 141975. doi: 10.1016/j.scitotenv.2020.141975
Yang, Y., Wu, R., Hu, J., Xing, S., Huang, C., Mi, J., et al. (2020). Dominant denitrifying bacteria are important hosts of antibiotic resistance genes in pig farm anoxic-oxic wastewater treatment processes. Environ. Int. 143, 105897. doi: 10.1016/j.envint.2020.105897
Keywords: Tibetan pigs, diarrheagenic Escherichia coli, antibiotic resistance genes, integrase genes, virulence genes
Citation: Zhao X, Liang J, Xia Z and Chen C (2025) Antibiotic resistance, virulence gene, phylogenetic group and genetic diversity of Escherichia coli isolated from Tibetan pig farms in Garze Tibetan Autonomous Prefecture, Sichuan, China. Front. Cell. Infect. Microbiol. 15:1526028. doi: 10.3389/fcimb.2025.1526028
Received: 11 November 2024; Accepted: 11 April 2025;
Published: 12 May 2025.
Edited by:
Nasib Singh, Eternal University, IndiaReviewed by:
Mikaeel Young, Baylor University, United StatesKyriaki Chatzikyriakidou, Aristotle University of Thessaloniki, Greece
Copyright © 2025 Zhao, Liang, Xia and Chen. This is an open-access article distributed under the terms of the Creative Commons Attribution License (CC BY). The use, distribution or reproduction in other forums is permitted, provided the original author(s) and the copyright owner(s) are credited and that the original publication in this journal is cited, in accordance with accepted academic practice. No use, distribution or reproduction is permitted which does not comply with these terms.
*Correspondence: Chaoxi Chen, Y2hhb3hpODgzMkAxNjMuY29t
†These authors have contributed equally to this work