- 1Department of Public Health and Infectious Diseases, Sapienza University of Rome, Rome, Italy
- 2Department of Experimental Medicine, University of Rome Tor Vergata, Rome, Italy
- 3Laboratory of Clinical Microbiology, Policlinico Tor Vergata, Rome, Italy
- 4Department of Promotion of Human Sciences and Quality of Life, San Raffaele Open University, Rome, Italy
- 5Laboratory of Microbiology, Istituto di Ricovero e Cura a Carattere Scientifico (IRCCS) San Raffaele Roma, Rome, Italy
- 6Scientific Department, Army Medical Center, Defense Institute for Biomedical Sciences, Rome, Italy
- 7Department of Public Health and Infectious Diseases, Laboratory Affiliated to Institute Pasteur Italia-Cenci Bolognetti Foundation, Sapienza University of Rome, Rome, Italy
- 8Department of Infectious Diseases, Istituto Superiore di Sanità, Rome, Italy
Introduction: Acinetobacter baumannii exhibits high genomic plasticity, enabling it to acquire virulence factors and antibiotic resistance (AR). Understanding its evolutionary adaptations is crucial for developing effective therapeutic strategies.
Methods: Thirty clinical isolates collected from two distinct time periods, defined as older (2010–2013), and recent (2022–2023),- were compared phenotypically (antibiotic resistance, growth, biofilm formation, desiccation tolerance, invasiveness) and genotypically (whole-genome sequencing).
Results: All isolates displayed an extensively drug-resistant phenotype. Overall, respiratory isolates harbored a higher content of antibiotic-resistant genes (ARGs), with older isolates showing 12.5% increases in the average number of ARGs compared to recent urine isolates (P = 0.02). More than 50% of the strains with faster growth, stronger biofilm formation, and increased lung cell invasiveness were recent respiratory isolates, while over 70% of older isolates showed greater desiccation tolerance and bladder cell invasiveness. Eleven virulence factor genes were shared between old and recent respiratory isolates, and eight were common between recent urinary and respiratory strains with no overlap among urinary isolates. Statistically significant positive correlations were observed between fast-growing and strong biofilm-forming respiratory isolates as well as their lung cell invasiveness. Conversely, negative correlations were found between collection time, isolation site, and host cell invasiveness. Analysis of macrocolony types revealed no link to phenotypic behavior.
Conclusion: Significant genetic variability was found between past and recent isolates. Older isolates had more genes involved in adhesion and nutrient uptake, while recent respiratory strains demonstrated increased biofilm formation and invasiveness, reflecting adaptation to clinical pressures. These findings highlight the dynamic evolution of A. baumannii, providing insights for future therapeutic strategies and infection control.
Introduction
Acinetobacter baumannii is a major opportunistic pathogen responsible for severe nosocomial infections worldwide, primarily affecting the lungs and bloodstream; however, the number of isolates collected from urinary sites has recently increased significantly compared to the past (Duan et al., 2024; Obenhuber et al., 2024), raising the possibility that either it was previously underestimated or that A. baumannii isolates adapted to urinary tract. Currently, respiratory and urinary isolates exhibit robust environmental and host persistence and several antibiotic resistance (AR) mechanisms (Yang et al., 2019; Zeighami et al., 2019). Moreover, transmission is not limited to vulnerable and critically ill patients in intensive care units (ICUs), but also to patients in general wards with long-term care treatment (Valencia-Martín et al., 2019; Alrahmany et al., 2022). According to European Antimicrobial Resistance Surveillance Network (EARS-Net) in 2023, 59% of the 8,429 Acinetobacter spp. invasive isolates collected from 25 European countries exhibited resistance to multiple classes of antibiotics (www.ecdc.europa.eu/en). As global mortality associated with multidrug-resistant (MDR) A. baumannii accounted for 132.000 cases in 2019 (www.iris.who.int) and is projected to increase further, new medical therapeutic countermeasures for this pathogen have been prioritized (Murray et al., 2022; Hu et al., 2023).
In the last decades, A. baumannii clinical isolates have been exposed to enormous stress conditions, particularly due to the extensive use of antibiotics. As a consequence, A. baumannii isolates undergo local clonal expansion and adapt progressively to environmental and host changes (Ambrosi et al., 2017; Ambrosi et al., 2020; Stracquadanio et al., 2022; Scribano et al., 2024). A. baumannii great genomic plasticity allowed the acquisition of a remarkable number of AR genes (ARGs) via horizontal gene transfer through plasmid splicing, integrons, transposons, and mutations (Hamidian and Hall, 2021; Ambrose et al., 2023). Other mechanisms, such as changes in the outer membrane proteins, target modification, and efflux pumps, further promote A. baumannii extraordinary resistance to antibiotics (Sarshar et al., 2021; Cain and Hamidian, 2023; Scribano et al., 2024). Acquirable traits allow A. baumannii isolates to adapt and persist in healthcare settings by forming biofilms, tolerating desiccation, improving motility and invasiveness. Isolates that are more adapted to local stresses expand clonally; currently, eleven international clones (IC) have been identified (Hansen et al., 2023; Karah et al., 2023). From epidemiological studies, it is evident that IC2 is the predominant and more spread clone, especially in Europe, whereas the others show a particular geographic dominance (Hansen et al., 2023; Karah et al., 2023; Müller et al., 2023). Several evidence demonstrated the fast evolution of this opportunistic pathogen in the healthcare environments and in the host during infection driven by stress exposure to achieve maximal adaptation (Holt et al., 2019; Penesyan et al., 2019; Pompilio et al., 2021). Therefore, the present study aimed to genotypically and phenotypically characterize a collection of endemic carbapenem-resistant A. baumannii clinical isolates, collected in two distinct periods nearly a decade apart to identify key features, including antibiotic resistance and virulence traits, to understand their successful microevolution in the nosocomial environment.
Methods
Bacterial isolates, growth curves, and antibiogram profiles
Thirty A. baumannii carbapenem-resistant isolates were collected from two periods (2010–2013) and (2022– 2023) from three hospitals in Rome, Italy, including Policlinico Umberto I (n=9), Policlinico Di Liegro (n=6), and Policlinico Tor Vergata (n=15). Inclusion criteria: Carbapenem-resistant A. baumannii strains isolated from respiratory or urinary specimens, reflecting prevalent infection sources and the high risk of progression to pan-drug resistance. Exclusion criteria: Strains not exhibiting carbapenem resistance; strains isolated from other clinical sources. A single A. baumannii strain per patient was included in this study and was isolated mainly from respiratory specimens, including sputum (n=6), bronchoalveolar lavage (BAL) (n=4), bronchoalveolar aspirate (BA) (n=12), whereas 8 from urinary infections (Table 1). Species were identified by MALDI-TOF mass spectrometry. Strains were grown on Luria-Bertani (LB) broth and agar plates (LA). Growth kinetics in 96 well microplates were determined by measuring cell density (OD600) for 16 h at 37°C in LB with vigorous shaking (200 rpm) in triplicate wells for each strain, using the plate reader BioTek SynergyHT equipped with the software GEN5™. Three independent experiments were performed. The reference strains ATCC 17978 and ATCC 19606 were used as controls for all the assays, while strain AB5075 for surface-associated motility only (Scribano et al., 2019). Antibiogram profiles were obtained using the VITEK®2 system (bioMérieux, Italia S.p.A, Grassina, Italy) with the AST-N397 panel, which included amikacin, gentamicin, tobramycin, imipenem, meropenem, piperacillin/tazobactam, ciprofloxacin, trimethoprim/sulfamethoxazole, and colistin. Minimum inhibitory concentration (MIC) values were interpreted according to the European Committee on Antimicrobial Susceptibility Testing (EUCAST v13.0, 2023). Detailed MIC values and breakpoints are provided in Table 2.”
Whole genome sequencing, molecular typing, antibiotic and virulence factors profiles
DNA was extracted using a Bacterial Genomic Isolation Kit (BioVision, USA). Libraries were prepared using the Nextera XT DNA kit and were sequenced on Illumina NextSeq500 and NextSeq2000 platforms using High Output Kit v2.5 (300 cycles) and P2 reagents (300 cycles), respectively; following the manufacturer’s instructions (Illumina, CA, USA). Raw reads (around 14M of reads per sample) were evaluated using FastQC v0.12.1 (2023–03–01) as post-sequencing Quality Control. Raw reads were filtered by Trimmomatic v0.39 (Bolger et al., 2014) with following parameters: ILLUMINACLIP: TruSeq3-PE.fa:2:30:10, LEADING:3, TRAILING:3, SLIDINGWINDOW:4:20, and MINLEN:36. A Phred quality score threshold of Q20 was applied for read filtering. The whole-genome shotgun sequences of the isolates generated in this study were deposited in GenBank under the BioProject accession number PRJNA1173090. The de novo assembly was performed by SPAdes Assembler v3.14.1 (2020–08–25) (Bankevich et al., 2012) on high-quality paired-ends reads for each isolate. Each genomic assembly contained contigs longer than 200 bp according to NCBI instructions (https://www.ncbi.nlm.nih.gov/genbank/wgsfaq/). ARGs were searched with AMRFinder Pathogen Detection (VERSION: 3.12.8, 2024-01-31.1). Multilocus Sequence Typing (MLST) with MLST Pasteur scheme (VERSION: 2024-03-09) (https://github.com/tseemann/mlst; accessed on March 2024). This scheme was selected for its high discriminatory power in A. baumannii, ability to provide stable sequence type (ST) assignments, and reliability in tracking clonal lineages across different hospitals and collection years. Additionally, it ensures comparability with previous epidemiological studies. DNA sequences of bacterial Virulence Factors (VFs) were retrieved from the Virulence Factors Database (VFDB). Virulence markers were searched with a minimal coverage of > 90% and an e-value cut off of 0.0000001 Analyses of the contigs sequences for each sample were performed using blastn v2.11.0+ (2020–10–11). Sequence analyses were performed using a commercial service (Bio-Fab Research srl, Roma, Italy).
Biofilm formation assay
Biofilm formation was assessed using the microtiter plate assay and categorized as biofilm-forming producers as previously described (Ambrosi et al., 2017). Three independent experiments were performed.
Desiccation tolerance assay
Overnight cultures were adjusted to OD600 ≈ 1.0, harvested by centrifugation, and washed twice with 2 ml of sterile water. Cell suspensions (10 µL) were deposited into a 96-well polystyrene microplate in triplicate and air-dried for 2–3 h in a biosafety cabinet until they were visibly dry. Uncovered plates were then placed in an airtight transparent plastic bag with silica beads and incubated for up to six days at 25°C with a relative humidity of 25 ± 2%. After incubation, the bacteria were rehydrated with water, gently scraped, plates rocked for 30 min to collect all cells from the bottom of the microplates, serially diluted in PBS and plated onto LB agar containing ampicillin (100 µg/ml). The percentage survival was calculated as:
Surfaced-associated motility, protease, and hemolytic activities
Surface-associated motility was evaluated by spotting 5 µL of an overnight culture on freshly prepared soft agar plates incubated at 37°C for 24 h., as previously described (Scribano et al., 2024). The motile clinical strains AB5075 and ATCC 17978, as well as the not motile ATCC 19606 were included as controls. Protease activity was assessed by spotting 5 µL on LB agar containing 1% skim milk. Pseudomonas aeruginosa PAO1 was included as the positive control. One microliter of the overnight culture was spotted onto Columbia agar plates (Becton Dickinson™, Milan, Italy) and incubated for 24 h to assess hemolytic activity and macrocolony types.
In vitro infection
The human A549 lung epithelial cell line type II (ATCC CCL185, lab collection) and bladder cell line 5637 HTB-9 (ATCC-LGC, Milan, Italy) were cultured in DMEM supplemented with 10% fetal bovine serum (FBS), 1% glutamine, 1% penicillin-streptomycin, and 50 µg/ml gentamycin. Cell monolayers on 35 mm culture dishes at a density of 2.5 x 105/ml were infected with clinical isolates at a multiplicity of infection (MOI) of 10 and incubated at 37°C with 5% CO2 for 2.5 h before adding colistin (10 µg/ml, BioChemica) to kill extracellular bacteria. After 48 h, cell monolayers were washed five times with PBS and lysed with 1 ml 0.1% Triton X-100. The recovered bacteria were serially diluted and plated onto LA plates to determine the number of colony-forming units (CFU)/ml.
Statistical analyses
GraphPad Prism Software (10.3.1) was used for statistical analyses. Normality was assessed using the Shapiro-Wilk test or Kolmogorov-Smirnov test. Phenotypic characteristics were analyzed using one-way ANOVA, and followed by Dunnett’s post hoc test for multiple comparisons. Pearson correlation was used to assess the relationships among phenotypic traits, with data transformed using the log (Y) function prior to analysis. To evaluate whether the number of antibiotic resistance genes (ARGs) influence MIC values, the same analysis was performed. Statistical significance for all tests was set at P< 0.05. Moreover, Supplementary Figures S1, S2 were generated with Microsoft Excel and GraphPad Prism, respectively.
Data availability
The data presented in the study are deposited in the NCBI GenBank repository, accession numbers PRJNA1173090.
Results
Antibiogram profiles and genomic content of ARGs
The minimal inhibition concentration (MIC) was assessed for all isolates, and values were interpreted according to EUCAST 2023 criteria (Table 2). Based on MIC values, all isolates were classified as extensively drug-resistant (XDR). Apart from carbapenem-resistance, few isolates (7/30) were susceptible to amikacin, whereas only one to tobramycin (Table 2). All isolates were susceptible to colistin. Overall, strains collected from urine were more resistant compared with respiratory isolates. EUCAST was chosen over clinical and laboratory standard institute (CLSI) as it is the standard in Europe, and provides continuously updated breakpoints based on pharmacokinetic and clinical data, ensuring a more precise and stringent classification of resistance, particularly for carbapenems. To associate the clinical breakpoints to genotypic data, the genome of each isolate was extracted and sequenced. According to the Pasteur scheme, all A. baumannii isolates belonged to ST2; but one, isolate BO29, belonging to ST632 (Ambrosi et al., 2016).
Genes directly and indirectly related to AR mechanisms were identified (Figure 1). Statistical analysis revealed a significant difference in the number of ARGs among isolates. On average, 46% (33/71) of conserved genes were harbored by older isolates, with older respiratory isolates exhibiting a 12.5% increase ARGs number compared to recent urine isolates (P = 0.02). Regarding the genes conferring resistance to aminoglycosides, 13 genes were found in the genomes’ isolates (Figure 1), thereby accounting for the high levels of resistance found in the MIC data (Table 2). Notably, the number of aminoglycoside resistance genes showed a strong positive correlation with the MIC of gentamicin (r = 0.43, P = 0.02) and amikacin (r = 0.70, P = 0.00002). Among these, the ant (3)-IIa variant was present in all isolates, followed by aph(3’)-Ib and aph (6)-Id (19/30), armA (18/19), aph(3’)-Ia (15/30), ant(2’)-Ia (10/30), aadA2 (9/30), aph(3’)-Via (7/30), aadA1 (6/30), aac (6)-Ib’ (5/30); aac (3)-Ia, aac(6’)-Ian, and aac (6)-Ib3 were found in single isolates. Interestingly, the overall content of aminoglycoside resistant genes was found less in recently isolated strains in comparison to old ones, particularly for urinary strains (Figure 1).
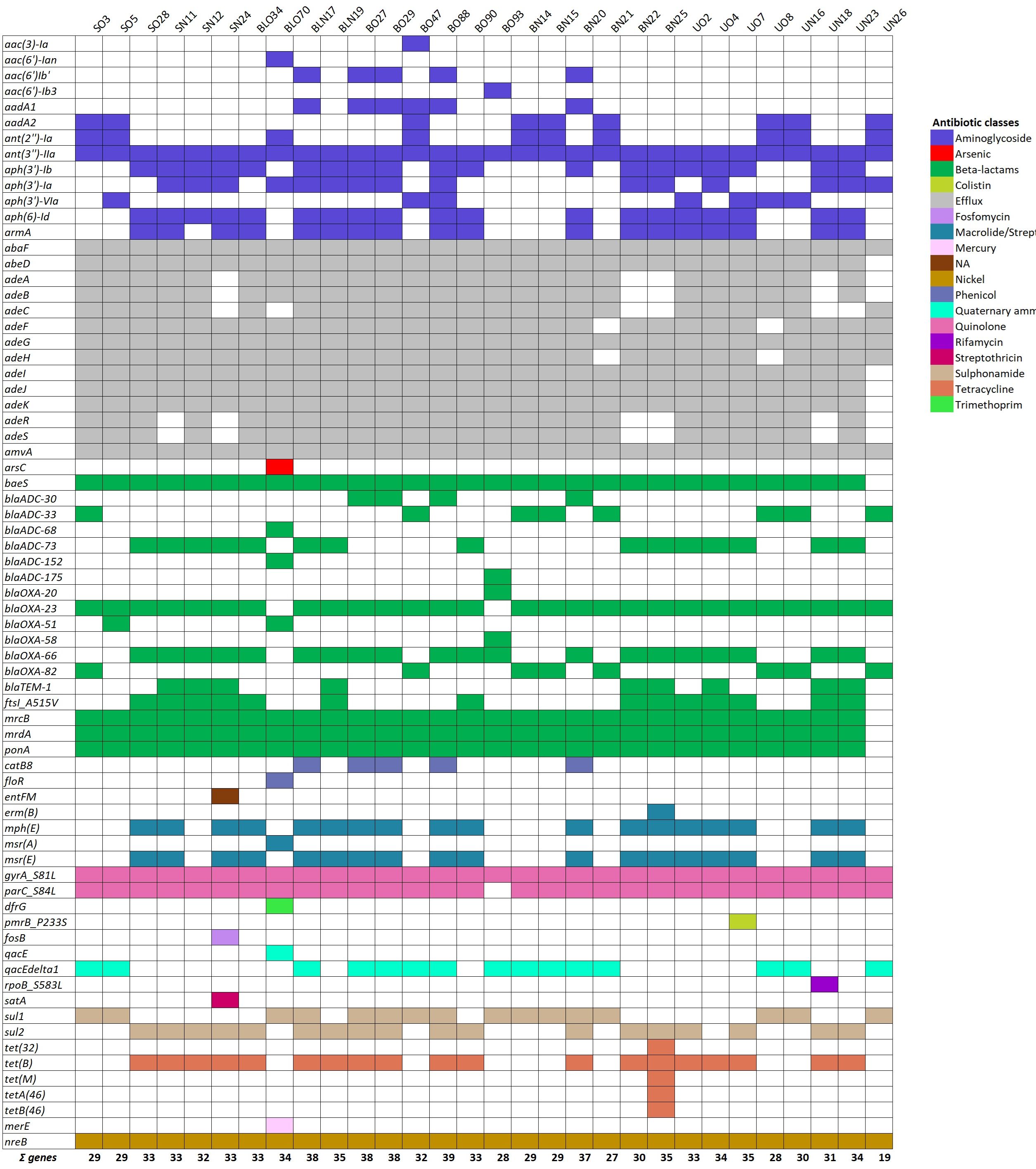
Figure 1. Genes directly and indirectly related to AR mechanisms detected in the isolate collection. Heat map of ARGs identified using whole genome sequence analysis. Color-code represents genes involved in the resistance of the different classes of antibiotics, heavy metals, and disinfectants, as indicated. NA, not applicable.
Among class D OXA-type carbapenemases, the blaOXA-23 gene was present in the vast majority of isolates (93%, 28/30) (Figure 1). Interestingly, the blaOXA-51-like carbapenemase gene, including blaOXA-66 and blaOXA-82 variants, was carried by all isolates. Conversely, the blaOXA-58 and blaOXA-20 genes were carried by one strain each (3%, 1/30), and the class A gene, blaTEM-1was found in 30% of isolates (9/30) (Figure 1). Several intrinsic Acinetobacter-derived cephalosporinases (ADCs) genes belonging to class C were found in the genomes of isolates (Figure 1). The most prevalent variants were blaADC-73(50%, 15/30), blaADC-33(27%, 8/30), and blaADC-30(13%, 4/30) (Figure 1). Other variants such as blaADC-152, and blaADC-175were identified only in old isolates BO70, and BO93, respectively (Figure 1). It was also identified the mutation A515V in the penicillin-binding protein 3, FtsI, an essential division component in fourteen isolates, which is associated with an increase in meropenem MIC values (Taguchi et al., 2019). From our data, the overall content of beta-lactamase and carbapenemase genes seems increased in recently collected strains from respiratory specimens, while it is stable among urinary isolates (Supplementary Figure S1A).
Moreover, the parC_S84L and gyrA_S81L genes conferring resistance to quinolones were found in 97 and 100% of the isolates, respectively (Figure 1). The sul1 and sul2 genes involved in sulfonamide resistance were less present, being both found in 53% of the genomes (16/30). The overall content of quinolone and sulfonamide resistance genes appears to have increased and decreased in recent respiratory strains, respectively. Conversely, while the content of quinolone resistance genes remains the same in both old and new urinary strains, there is an increase in sulfonamide resistance genes in recent urinary strains (Figure 1). Furthermore, five genes associated with tetracycline resistance mechanisms were identified, tet(B), tet(M), tet (32), tetA (46), and tetB (46), showing higher prevalence in recent respiratory isolates and lower prevalence in recent urinary strains (Figure 1). The tet(B) gene, an efflux pump (belonging to the major facilitator superfamily [MFS] superfamily) known to mediate resistance to tetracycline, doxycycline, and minocycline, was the most prevalent among our strain collection, accounting for 67% (20/30). The other tetracycline resistance genes, including tet (32), tet(M), conferring resistance to tetracycline by ribosomal protection, and tetAB (46), encoding a predicted heterodimeric ABC transporter was only described in Streptococcus australis (Warburton et al., 2013) were found only in strain BN25. To assess the contribution of these tetracycline resistance genes, we determined the MIC of tetracycline. BN25, BLN17, and UO7 exhibited MICs greater than 256 µg/ml, while other strains had MICs >16 µg/ml, highlighting the key role of Tet efflux pumps, including tetB (Huys et al., 2005).
Moreover, all isolates were colistin-susceptible, according to EUCAST breakpoints (https://www.eucast.org/). Notably, isolate UO7 carried the P233S mutation in the histidine kinase encoded by the pmrB gene, described as a key mutation for conferring resistance to polymyxins under certain conditions, accounting for the higher MIC value (Sun et al., 2020; Hahm et al., 2022). Although macrolide resistance was not tested, the A. baumannii common mph(E)-msr(E) operon was found in 57% of isolates (17/30). Differently, the erm(B), and msrA genes were individually found in only two isolates, BN25 and BO70, respectively (Figure 1). Overall, macrolide resistance gene content was found increased in recent respiratory isolates, and decreased in recent urinary strains (Supplementary Figure S1). Furthermore, the catB8 gene, encoding a chloramphenicol acetyltransferases protein type B (Liao et al., 2024), was identified in 17% (5/30) of isolates. Differently, the floR gene, conferring resistance to florfenicol and chloramphenicol, was found only in one isolate (Figure 1).
Several genes encoding multidrug efflux pumps were identified; the most prevalent ones were amvA and abaF, encoding MFS pumps, which were found in all isolates (Sharma et al., 2016; Short et al., 2021) (Figure 1). Differently, the adeC gene, encoding the outer membrane protein of the three-component efflux machinery of the resistance nodulation division (RND)-type superfamily (Salehi et al., 2021), was found in 80% of isolates (24/30). Interestingly, the mutation P116L in the regulatory gene adeR associated with AdeAB overexpression was identified in only one isolate (1/30), UO7 (Figure 1) (Marchand et al., 2004). The RND efflux pump adeFGH were highly present in all isolates, ranging from 100% for adeG to 97% (29/30) for adeF and adeH (Figure 1). In addition, the efflux systems involving qac genes (qacE and qacE delta1) that confers resistance to biocides were present in 3 (1/30) and 50% (15/30) of isolates, respectively (Shafaati et al., 2016). The nickel/cobalt tolerance gene nreB was found in all isolates (Neidhöfer et al., 2023). Overall, we found a slight decrease in the content of efflux pump genes in both groups of recent strains (Figure 1; Supplementary Figure S1). Other genes involved in resistance to heavy metal efflux (arsC, and merE), fosfomycin (abaF and fosB), trimethoprim (dfrG), rifamycin (rpoB_S583L), and streptothricin (satA), were the least prevalent among isolates (Figure 1). The highest content of ARGs was found in strain BO88 (39 genes), followed by strains BN17, BO29, and BO27 (Neidhöfer et al., 2023). According to the antibiogram profile, the most common genotype in strains displaying the highest MIC values was: ant(3’’)-IIa, abaF, abeD, adeABFGHJK, amvA, baeS, blaOXA-23, blaOXA-51 (variants included), mrcB, mrdA, ponA, gyrA_S81L, nreB, parC_S84L.
Growth rates and genomic content of key metabolic genes
The growth properties of the isolates were evaluated. The two reference strains ATCC 17978 and ATCC 19606 were included as controls. As shown in Figure 2, eleven isolates collected from 2022, and mainly isolated from the respiratory tract (7/11), showed a significant faster growth in comparison to both reference strains (P< 0.0001). Key genes related to A. baumannii metal ion metabolism uptake systems were analyzed (Figure 3), including the bar/bas/bau/entE (synthesis, secretion, and uptake acinetobactin/enterobactin, pbpG (penicillin-binding protein 7 (PBP7), fecIR/hemO/tonB (hemin or hemoglobin), pirA/slam/ybaN (transporters), znuABC (zinc acquisition system), zrlA (zinc peptidase), plc1/plc2/plcD (phospholipases), and lysR (transcriptional regulator) (Sheldon and Skaar, 2020; Bateman et al., 2021; Cook-Libin et al., 2022); basD, plcD, and znuABC were conserved in all isolates, while prevalent basAB, bauA, entE, fecI, hemO, and plc2 (29/30, 97%) (Figure 3). According to growth rates, the most common genotype in fastest-growing isolates was: basABD, bauA, entE, plc2, plcD, znuABC (Figures 2, 3).
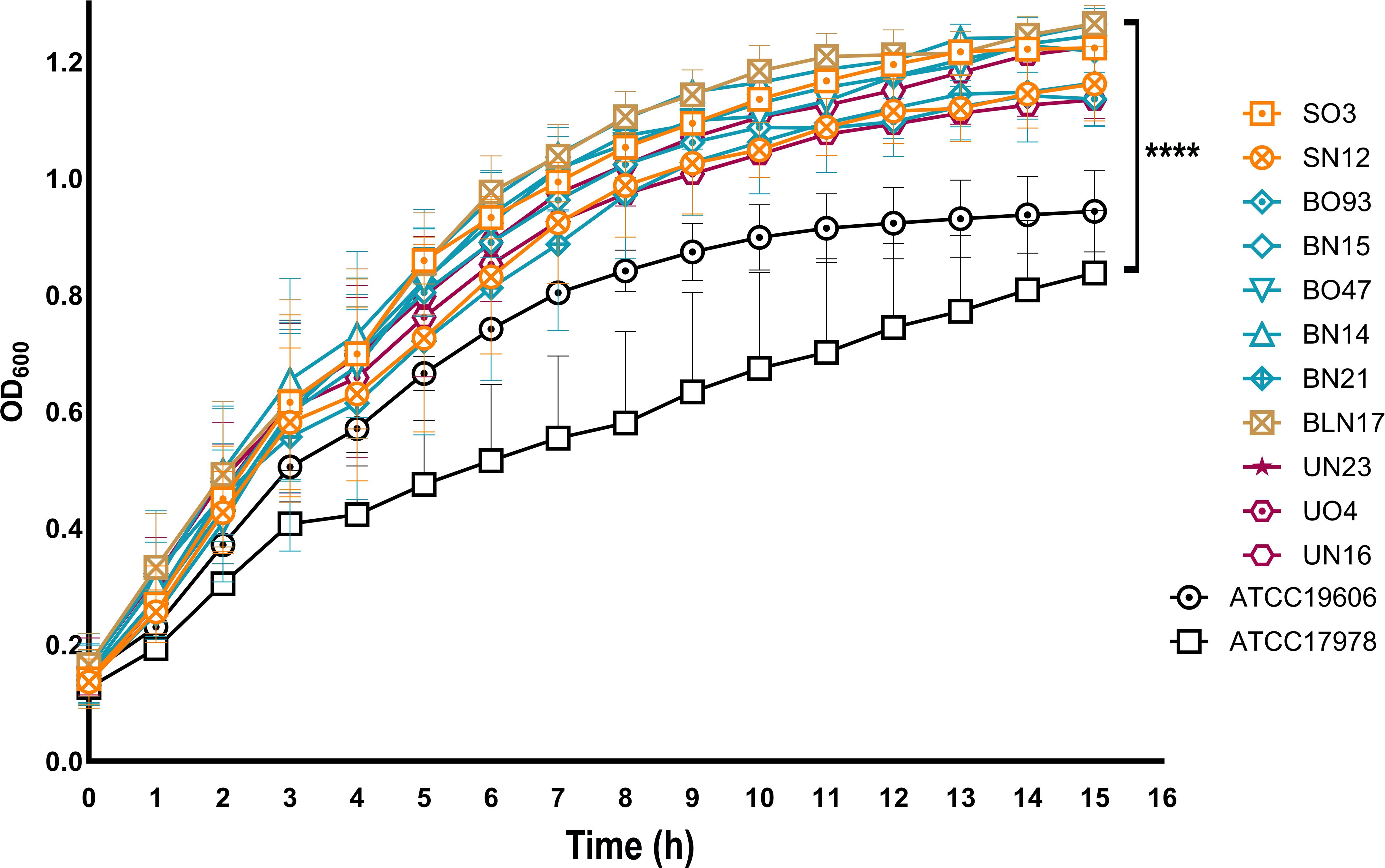
Figure 2. Growth rates of the clinical isolates. Overnight cultures were diluted to 1:100 in LB in a 96-wells microplate. The OD600 was determined every 30 min using a plate reader (BioTek SynergyHT). Only the growth curves of the 11 fastest-growing isolates, compared to both reference strains, are presented. Means and standard deviations were obtained from three independent experiments. ****P< 0.0001.
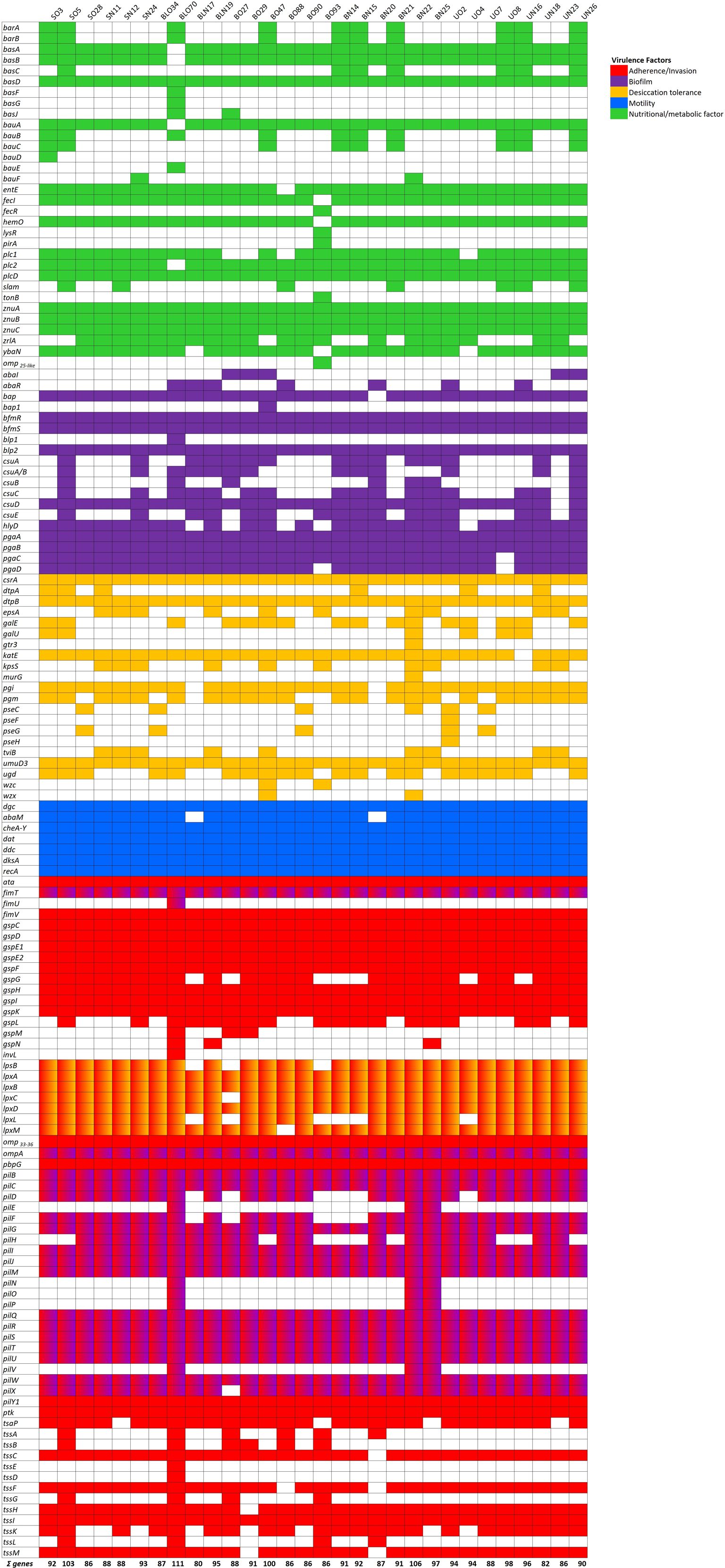
Figure 3. Genes directly and indirectly related to virulence detected in the isolate collection. Heat map of virulence genes identified using whole genome sequence analysis. Color-code represents genes involved in different aspects of virulence, adhesion, biofilm, secretion systems, exotoxins, immune modulation, and nutritional/metabolic factors, as indicated.
Biofilm-forming activity, desiccation tolerance and genomic content of related genes
The ability of A. baumannii clinical isolates to form biofilms represent crucial features in clinical settings. Therefore, the biofilm-forming ability on polystyrene microtiter plates of each isolate was measured by Crystal Violet staining (Figure 4). Isolates arbitrarily grouped according to A570/A600 values as weak (<0. 47), moderate (0.47-0.70), and strong biofilm producers (>0.70) accounted for 16.7% (5/30), moderate for 23.3% (7/30), and strong for 60.0% (18/30), respectively (Figure 4A). Nine isolates (SN24, BO47, BO88, BN15, BN14, BN21, BN22, UO2, and UO8) showed significant differences in biofilm formation compared with both reference strains, with the majority belonging to the recently collected BA specimens (71.4%, 5/7). Isolates BO88 and UO2 were significant only in comparison with ATCC 19606 reference strain (Figure 4A). Interestingly, we found a significant positive correlation between biofilm producer strains and amikacin resistance (r = 0.43, P = 0.016). Several genes that contribute to biofilm formation were searched, including bap/bap-like proteins (biofilm associate proteins), csuA-E (chaperone-usher type I pili), pil and fim genes (type 4 pili), pgaA-D genes (poly-β-1,6-N-acetylglucosamine), bfmRS (the two-component system), ompA (the major Gram-negative porin), hlyD (Type 1 Secretion System) and abaIRM (quorum sensing). All isolates carried the bpl2, bfmRS, ompA, csuD, pgaAB, pilBCGIJMQRSTUWY1, and fimTV genes (Figure 3). Interestingly, the bap gene was identified in all but one (the BN20 isolate), while bap1 and bpl1 were identified only in BO47 and BO70, respectively (Figure 3). According to the biofilm results, the genes shared among all the strains displaying the strongest biofilm-forming ability were: bap/blp2/bfmRS/csuD/pgaAB/fimTV/ompA/pilBCGIJlMlQRSTUWXY1 (9/9), with a prevalence of hlyD, pgaCD (8/9). Unexpectedly, the abaIR genes were found in a minority of strains, UN16, BLN17, BLN19, BLO70, and UO2, the latter only displaying a strong biofilm-forming activity (Figure 3). Conversely, the abaR gene, without abaI, was found only in BN20 and B088. Additionally, we found significant positive correlation between fast-growing and strong biofilm-forming isolates (r = 0.42, P = 0.022) (Supplementary Figure S2). Another critical feature for healthcare settings is A. baumannii desiccation tolerance since it allows long term survival, and therefore increases bacterial spread in the hospital environment (Farrow et al., 2018; Bashiri et al., 2021). Therefore, the tolerance to desiccation for 6 days was tested. Starting with an initial number of bacteria (approximately 108–109 CFU/ml/strain), the number of recovered bacteria varied (approximately 101–104 CFU/ml/strain) after six days of dryness and 25% of relative humidity. Seven isolates, SO3, BLN19, BN25, BO29, BO88, BO90, and UO8, displayed significant differences in their desiccation tolerance (Figure 4B). No reference strains were recovered. Among desiccation-tolerant strains, 57.1% (4/7) respiratory and 14.3% (1/7) urinary isolates, respectively, were retrieved before 2013. Genes directly and indirectly related to desiccation tolerance were searched, including bfmRS, csrA (carbon storage regulator A), epsA (exopolysaccharide), katE (catalase), recA (main recombinase), murG (peptidoglycan), lpx/lpsB (lipopolysaccharide), wzc/wzx/gtr3/kpsS/pgm/pse (capsule), galU/ugd/pgi/pgm (sugar precursors), dtpAB (hydrophilins), umuCD (error-prone DNA polymerase V), adeABC and adeIJK (efflux pumps) genes (Tipton et al., 2018; Singh et al., 2019; Green et al., 2022) (Figures 1, 3). Genes conserved in all isolates were csrA, dtpB, umuD3, lpxABD, recA and adeG, while prevalent pgm, katE, lpxCM, and adeIJK, (29/30, 97%) (Figure 3). According to the desiccation results, the most common genotype among the strains displaying the highest desiccation tolerance was: bfmRS/csrA/katE/lpxABCD/pgi/dtpB/umuD3/adeAB/adeIJK.
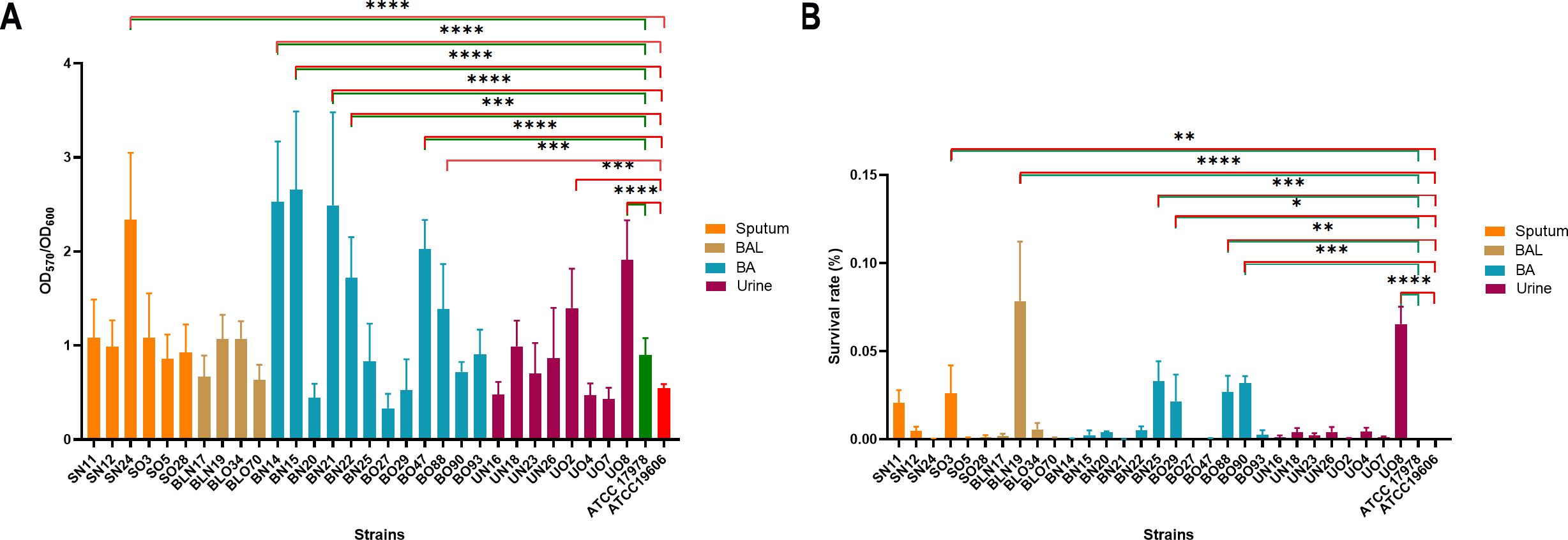
Figure 4. Ability of A. baumannii isolates to form biofilms and tolerate desiccation conditions. (A) A. baumannii strains were inoculated on polystyrene plates. After 48 h, quantification of biofilm formation was measured by the Crystal violet method (Ambrosi et al., 2017). Data are means ± standard deviation from at least three independent experiments, eight wells per strain (n=24). (B) Approximately 107–109 CFU/strain were inoculated in a 96 microplate. Plates were kept at 25°C with a relative humidity (RH) of 25 ± 3% for 6 days. Each isolate was recovered from three wells. Data are the ratio between the final and initial CFU/ml. Three independent experiments were performed in duplicate (n=6). Asterisks represent p values evaluated by one-way ANOVA in comparison to ATCC 17978 (green) and to ATCC 19606 (red); *P<0.05, **P<0.01, ***P<0.001, ****P<0.0001.
Surface-associated motility and genomic content of related genes
A. baumannii can move via surface-associated motility, an appendage-independent kind of movement (Jeong et al., 2024). Hence, the surface-associated motility of isolates was tested on soft agar plates. Isolates SN11, SN12, UN23, and BN25 displayed a not motile phenotype, while UO4, UO7, BO47, and BO90 a very slow motility (Figure 5). Conversely, the other isolates displayed surface-associated motility characterized by peculiar shapes, including tentacle-like (37%, 11/30), root-shaped (20%, 6/30), halo-shaped (10%, 3/30), and multi-layered (2/30) (Figure 5). Notably, the different patterns of motility were equally distributed between past and current isolates. Several genes have been shown to be involved in surface-associated motility, including ddc/dat (production of 1,3-diaminopropane), dgc (diguanylate cyclase), cheA/Y (two component system), dksA (DnaK suppressor protein), abaIRM, recA, ompA (Jeong et al., 2024). Interestingly, all the aforementioned genes were conserved in all isolates with the exception of abaM gene that was not found in BNL17 and BN20 (28/30, 93%) (Figure 3). According to the surface-associated motility, the most common genotype of motile isolates was: dgc, cheA-Y, dat, ddc, dksA and recA.
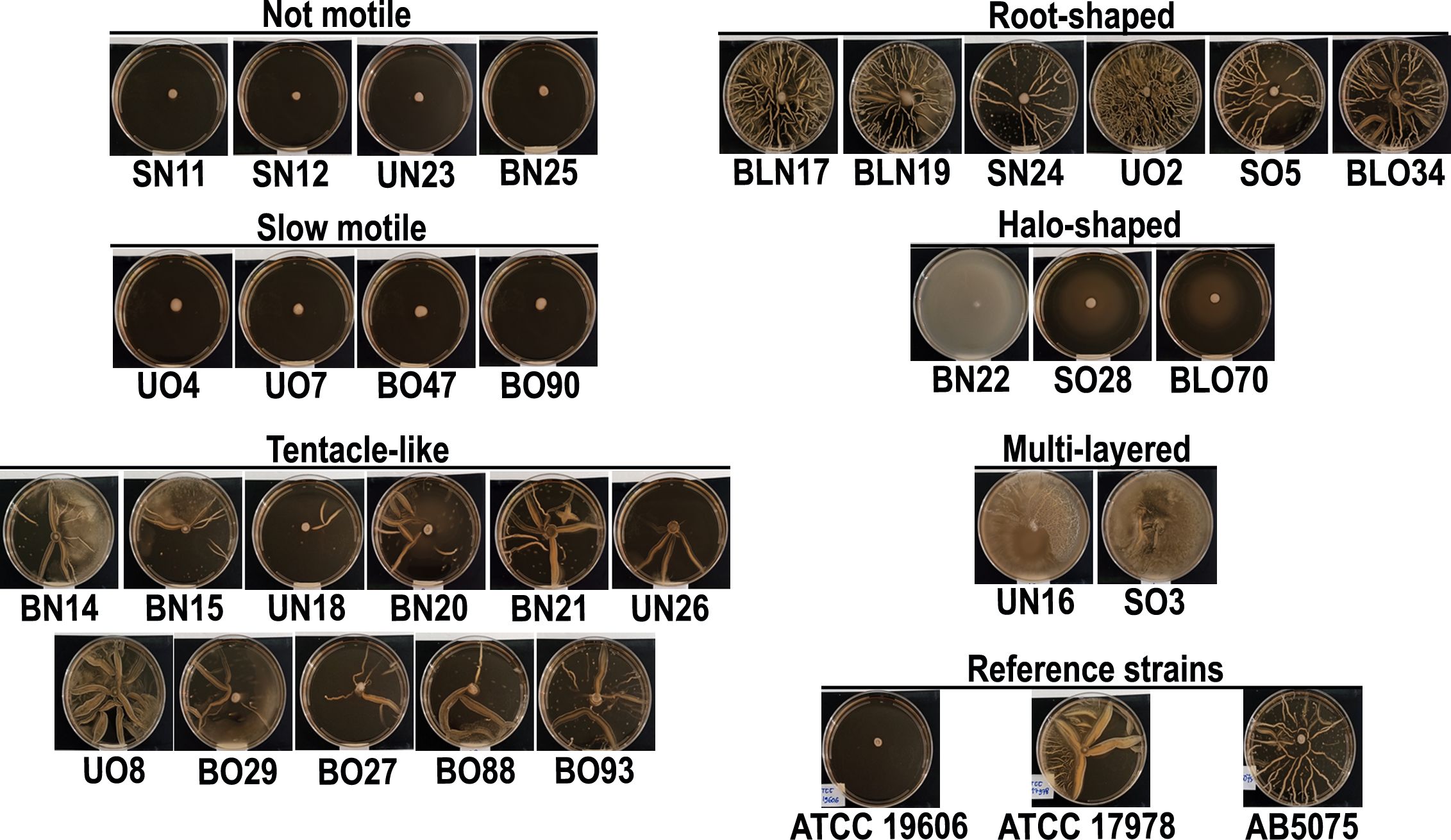
Figure 5. Surface-associated motility of clinical isolates. Each isolate was spotted on top of a soft agar plate. After 24 h of incubation at 37°C, plates were photographed. Three independent experiments were performed in duplicate (n=6). Representative images are shown. Reference strain AB5075 was included in the experiment for its different shape of surface-associated motility.
Host cell line invasiveness and genomic content of related genes
Some A. baumannii clinical isolates can enter epithelial cells to evade host immune responses and limit antibiotic accessibility (Ambrosi et al., 2020; Rubio et al., 2022). Therefore, infection experiments using human lung and bladder epithelial cells were conducted to quantify the number of intracellular bacteria after 48 hours (Figure 6). Compared to the two reference strains, ATCC 17978 and ATCC 19606, a significantly higher invasive capacity in lung epithelial cells was observed in 23% (7/30) of the tested strains, most of which were retrieved from respiratory specimens (5/7, 71%), reaching values greater than 3 × 106 CFU/ml (Figure 6A). Additionally, the majority of these invasive strains were recently isolated (6/7, 86%). The same experiment with bladder epithelial cells revealed that 27% of the analyzed strains could invade this host cell line (8/30). In this experimental set, eight isolates demonstrated greater invasiveness compared to both reference strains, 75% (6/8) of these were old strains and 38% (3/8) were retrieved from the urinary tract (Figure 6B). Intriguingly, among invasive strains, SN11, and UO8 were able to invade both host epithelial models, suggesting a cell-type non-specific invasion phenotype (Figure 6). Genes directly and indirectly related to invasiveness were searched (Figure 3), including ata (Acinetobacter trimeric autotransporter), gsp (T2SS), tss (T6SS), invL (adhesin), pbpG, lps, omp33-36/ompA, plc, pil genes. According to the invasion experiments, the most common genotype displayed by invasive isolates was: ata, fimTV, gspCDE1E2FHIK, lpsABCDM, omp33-36, ompA, pbpG, pilBCGIJMQRSTUWXY1, ptk, and tssCFHIM (Figure 3). Unexpectedly, invL was found only in BO70 (Figure 3). No major genetic differences between the cell-type non-specific invasion phenotypes and the other invasive isolates were found (Figure 3). Notably, significant negative correlations were found between urinary (r = -0.44, P = 0.014) and recent isolates (r = -0.36, P = 0.048) and the invasiveness of lung and bladder epithelial cells, respectively. Moreover, we found significant positive correlation (r = 0.40, P = 0.030) between strong biofilm-forming isolates and invasiveness of A549 cells (Supplementary Figure S2).
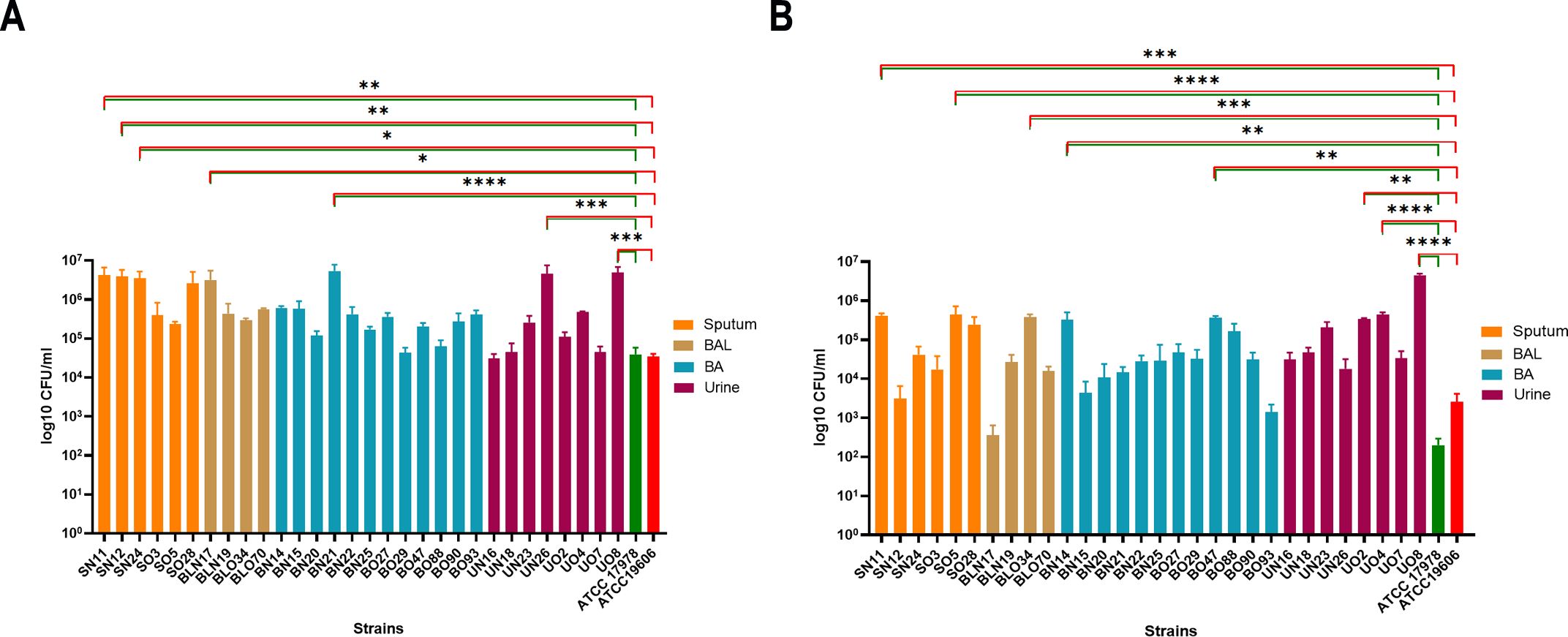
Figure 6. Ability of clinical isolates to be internalized in lung and bladder epithelial cells. Semi-confluent A549 (A) and HTB-9 (B) cell monolayers were infected with each isolate or ATCC 17978 or ATCC 19606 reference strains using a multiplicity of infection (MOI) of 10. Uninfected cells were used as control. After 2 h of adhesion, the medium was supplemented with colistin (10 µg/ml) to kill extracellular bacteria. Intracellular bacteria were enumerated after additional 48-h of incubation. Data (CFU/ml) are means ± standard deviation from at least five independent experiments, in duplicate (n=10). Asterisks represent p values evaluated by one-way ANOVA in comparison to ATCC 17978 (green) and to ATCC 19606 (red); *P<0.05, **P<0.01, ***P<0.001, ****P<0.0001.
Proteolytic and hemolytic activities and genomic content of related genes
As an additional assay of virulence traits, protease and hemolytic activities were assayed on skim milk and blood agar plates, respectively. No proteolytic and hemolytic activities could be detected in the clinical isolates in comparison with Pseudomonas aeruginosa PAO1 and Escherichia coli H20P used as positive controls (Ambrosi et al., 2019). The hlyD gene, reported to be a putative hemolysis-related gene (Schramm et al., 2019), was found in 83% (25/30) of the isolates (Figure 3). Additionally, among the plc genes (Fiester et al., 2016), plc1 was absent only in isolates BO29 and UO4, while plc2 was absent only in BO70 (Figure 3). Conversely, plcD was common to all isolates (Figure 3). We noticed a high degree of heterogeneity among the clinical isolates in the shape of the macrocolonies (Valcek et al., 2023); in particular, four previously reported and one new macrocolony types (MTs) were identified (Valcek et al., 2023) (Figure 7). This new macrocolony shape (MT G), shared by 50% of the strains (15/30), was characterized by a deep opaque center expanding to the round edge (Figure 7; Table 3). Interestingly, both plc2 and plcD were shared among strains displaying MT G (Figure 3). No morphology resembling types A and C was observed in any of our isolates (Valcek et al., 2023). The second prevalent MT was MT E, followed by MT F; only one isolate had a MT B (BN15), and one MT D (BN20) (Figure 7; Table 3). This MT pattern was homogeneously shared between past and recent isolates (8 and 7, respectively). Additionally, no correlation between MTs and surface-associated motility patterns was found (Figures 5, 7; Supplementary Figure S2) (r = 0.080, P = 0.256).

Figure 7. Macrocolony types (MTs) of clinical isolates on blood agar plates. One µl of each isolate was spotted on Columbia Agar with 5% sheep blood. After 24 h, macrocolonies were photographed. Representative images of one representative isolate per different MTs. Scale bar, 1 cm.
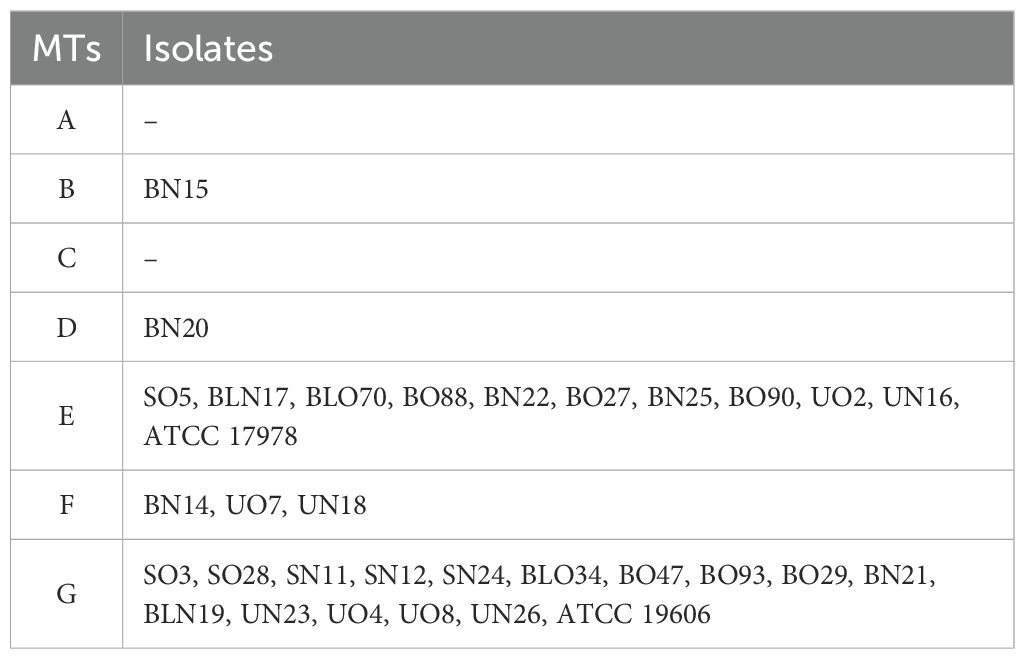
Table 3. Macrocolony types (MTs) (Valcek et al., 2023).
Discussion
From an evolutionary perspective, A. baumannii demonstrates remarkable adaptability, acquiring a wide range of virulence factors through high genomic plasticity. Understanding how this pathogen evolves to gain virulence and AR is critical for developing strategies to combat this public health threat. Thirty clinical isolates, 22 respiratory, and 8 urinary, were analyzed. The proportion of strains aligns with previous reports, where respiratory isolates dominate (39.5%) over uropathogenic isolates ranging from 6.1% to 29.3% (Bagińska et al., 2021). In line with current reports, the ST2 was the most represented in our collection (Hansen et al., 2023; Müller et al., 2023). All the isolates displayed an XDR phenotype, with a high number of ARGs in each genome (Table 2; Figure 1). Among the 71 identified ARGs, old isolates have a higher gene content (> 30) with respect to recent ones (27 vs. –19 genes, respectively), particularly old respiratory isolates exhibited 12.5% increases in the average number of ARGs compared to recent urine isolates (P = 0.02) (Figure 1; Supplementary Figure S1A). Vice versa, recent respiratory isolates carried an overall higher content of resistance genes for beta-lactams, carbapenems, quinolones, and tetracyclines, compared with old ones (Figure 1). Increased resistance to quinolones and carbapenems is in accordance with the ECDC report of invasive strains (https://atlas.ecdc.europa.eu/public/index.aspx). Furthermore, the number of aminoglycoside resistance genes positively correlated with the MIC of gentamicin (r = 0.43, P = 0.02) and amikacin (r = 0.70, P = 0.00002), supporting their role in conferring resistance. Notably, recent respiratory isolates exhibited lower amikacin MICs, likely due to the reduced prevalence of aminoglycoside resistance genes (Table 2; Figure 1). In the past, the synergistic effects of combined treatment with aminoglycosides and carbapenems or colistin for A. baumannii infections were exploited (Zhang et al., 2023). Hence, the lower prevalence of aminoglycoside resistance genes in our recent strains may reflect the decline in the use of this therapeutic approach, given its lack of significant improvement in clinical outcomes (Shields et al., 2023).
The lower content of macrolide resistance genes in recent isolates may be linked to the WHO’s 2017 recommendation against macrolides (www.iris.who.int, Figure 1). The genetic adjustments made by recent isolates over old ones might be due to lower contacts with donor species and/or that global and local antibiotic guidelines changes for carbapenem-resistant A. baumannii (Kim et al., 2021). Notably, all isolates remained susceptible to colistin; however, two isolates (SO5 and UO7) had MICs near the resistance breakpoint (EUCAST 2023), highlighting the presence of two colistin-heteroresistant strains. EUCAST clinical breakpoints were used for antibiotic susceptibility testing, as they are standard in Europe and facilitate interlaboratory comparisons; however, it is worth mentioning that, although EUCAST and CLSI breakpoints are aligned for carbapenem-resistant A. baumannii, notable discrepancies between the two methodologies exist for specific aminoglycoside and tetracycline subclasses, which should be duly considered (Farshadzadeh et al., 2018; Yang et al., 2019; Bulens et al., 2024; Halim et al., 2024). Moreover, the content of genes encoding efflux pumps was stable among isolates. While the adeE gene, typically of Acinetobacter nosocomialis, was missing, the majority of isolates (25/30) carried the adeB gene, which was found shared among bacteria belonging to the Acinetobacter genus (Hou et al., 2012; Cosgaya et al., 2019).
Distinctive traits of recent isolates include faster growth (P< 0.0001), a partial association with MT G (7/11), an average of 16.9 nutritionally-related genes, a strong biofilm-forming activity, and a lower desiccation tolerance than older ones (Figures 2–4A). These findings recall the behavior of multi-drug resistant (MDR) A. baumannii environmental isolates, in which this phenotype comes with a fitness cost that causes a significantly decrease in desiccation tolerance even with strong biofilm activity (Greene et al., 2016). Being these clinical isolates, a possible explanation is that these strains experienced less desiccation stress pressures in their growth environment Seven isolates, mostly from the past respiratory collection, survived six days of desiccation with an average survival rate of 3.44% (Figure 4B). Among the 21 desiccation-related genes identified, these isolates carried an average of 8.6 genes (Figure 3). Notably, the BfmR-dependent hydrophilin dtpB gene, involved in desiccation tolerance (Green et al., 2022), was found in all strains (Figure 3). However, only two strains, BO88 and UO8, retained both biofilm-forming and desiccation tolerance abilities (Figures 3, 4). Desiccation tolerance was reported to be a typical characteristic of clinical A. baumannii strains (Hamidian et al., 2022). However, from our data, it seems that the hospital environment may not exert desiccation pressure, offering enough water or humidity for bacterial survival and growth, potentially selecting mainly for stronger biofilm-forming strains.
The positive correlation found between growth rate and biofilm formation (r = 0.42, P = 0.022) corroborates previous findings that biofilm development depends on bacterial growth rates (Farshadzadeh et al., 2018). Conversely, urinary strains, while having the highest nutritionally-related gene content, were not proficient biofilm formers (Figure 4A). In dominant and non-dominant A. baumannii STs, despite a very similar content and expression patterns of biofilm-related genes, such as adeFGH, bap, csu, pga/b/c/d, abaR, and abaI, the actual biofilm-forming activity was not significantly different (Kong et al., 2023). Strong biofilm-formers had an average of 12.3 biofilm genes (range: 8-15, Figure 3; Supplementary Figure S1B), and it seems that at least 8 genes are sufficient for proficient biofilm formation. Moreover, the positive correlation between strong biofilm producers and invasion of lung epithelial cells (r = 0.40, P = 0.030) strengthen the notion that biofilm matrix can effectively shield bacteria, allowing persistence, and, eventually, invasion host cells (Yang et al., 2019).
Surface-associated motility was observed in 26 isolates, with variability from slow to hypermotile phenotypes (Figure 5). Variability in surface-associated morphotypes suggests that efflux pumps, quorum-sensing molecules and surfactant-like compounds and proteins may influence motility and phenotypic diversity (Clemmer et al., 2011; Cosgaya et al., 2019; Pérez-Varela et al., 2019). Seven genes related to surface-associated motility were identified (Supplementary Figure S1B) (Blaschke et al., 2021; Corral et al., 2021; Jeong et al., 2024), but two isolates (BN20 and BLN17) lacked the abaM gene (Figure 3). The lack of surface-associated motility of isolate BLN17 could be due to the absence of genes encoding the AdeRS two-component system that regulates the RND efflux pump AdeABC, as previously described (De Silva and Kumar, 2018). Chemotaxis in E. coli is subject to a behavioral variability and phenotypic diversity (Waite et al., 2018); E. coli swimming depends on external signal processing as well as the proton motif force and the levels of individual expression of the involved proteins, known as potential modulators of chemotactic variability (Waite et al., 2018). Although A. baumannii lacks flagella, it is plausible that variability of surface-associated motility could be due to the to a combination of the activity of efflux pumps that, in turn, cause a variable secretion of surfactants, proteins or quorum sensing molecules (Clemmer et al., 2011; Pérez-Varela et al., 2019; Gaona et al., 2024).
Thirteen isolates demonstrated higher invasiveness into epithelial cells compared to reference strains (Figure 6). Recent respiratory isolates were more invasive in lung cells, while older isolates showed more invasiveness in bladder cells, with observed invasive colony numbers of 4 x 106 and 1 x 106, respectively (Figure 6). Interestingly, two strains, SN11 and UO8, exhibited a non-specific cell-type invasion phenotype, being capable of invading both cell lines (Figure 6). Overall, these results suggest a shift in recent respiratory strains toward lung tropism in vitro, reflecting their preferred colonization/infection site in vivo (Bagińska et al., 2021). Several bacterial pathogens exhibit host cell tropism, dependent on specific surface appendages/proteins that ensure the interaction between pathogens and their respective hosts, like type 1 fimbriae for uropathogenic E. coli (Sarshar et al., 2020). Among 63 conserved genes corresponded to invasiveness, 79% were across all isolates, encoding components of lipopolysaccharide (LPS), type II secretion system (T2SS), type IV secretion system (T4SS), and type VI secretion system (T6SS) (Figure 3; Supplementary Figure S1B). Several of these genes were shown to be involved in adhesion/invasion in A. baumannii (De Silva and Kumar, 2018; Ambrosi et al., 2020; Sarshar et al., 2021). Notably, the invL gene, encoding an invasin-like adhesin dependent on T2SS, involved in adhesion to urothelial cell cultures, was identified only strain BO47 (Jackson-Litteken et al., 2022) (Figure 3). Differently, the T2SS genes were found in all strains (gspCDE1E2FHIK), underlining the importance of this system for A. baumannii physiology and pathogenesis; indeed, T2SS contributes to A. baumannii in vivo fitness as well as protection to the human bactericidal activity (Johnson et al., 2016; Waack et al., 2017). Thus, other T2SS-dependent proteins might be involved in A. baumannii invasiveness; moreover, the high degree of conservation makes this an ideal target for innovative anti-A. baumannii therapeutics. It is worth mentioning that this study has some potential limitations, including a limited sample size, as well as geographical and time constraints. However, this study provides evidence of significant adaptations driven by clinical pressures. The findings herein reported could contribute to highlight key traits that could be targeted for improved infection control and treatment strategies.
In conclusion, significant genetic variability was observed between past and recent isolates, with 71.2% of identified genes distributed across all strains. Interestingly, 19 genes were unique to the old respiratory strains (Figure 8), mainly involved in adhesion and nutrient uptake (e.g. fimU, gspM, tssBDEG, basFGJ, bauDE, bap1, wzc, pseG, lysR, fecR, pirA, tonB, and omp25-like). The overlap between old and recent respiratory isolates was limited to 11 shared genes, including pilE, pilNOPV, gspN, tssAL, tagX, pseC, and wzx. Eight genes were conserved across recent urinary and respiratory strains (tviB, kpsS, hemO, epsA, fecI, ybaN, hlyD, and slam). Remarkably, no gene overlap was observed between past and recent urinary isolates, justifying the marked difference in the phenotypic traits. Old isolates were more desiccation-tolerant, and metabolically variable likely due to adaptation to broader nutrient sources and environmental conditions. In contrast, recent respiratory isolate exhibited increased growth, biofilm formation, and invasiveness, reflecting the dynamic evolution to clinical stress pressures. Overall, these findings support the genetic adaptation of A. baumannii in response to environmental pressures, including antibiotic use and host immune responses. Understanding these genetic changes will provide valuable insights for future therapeutic approaches and infection control strategies.
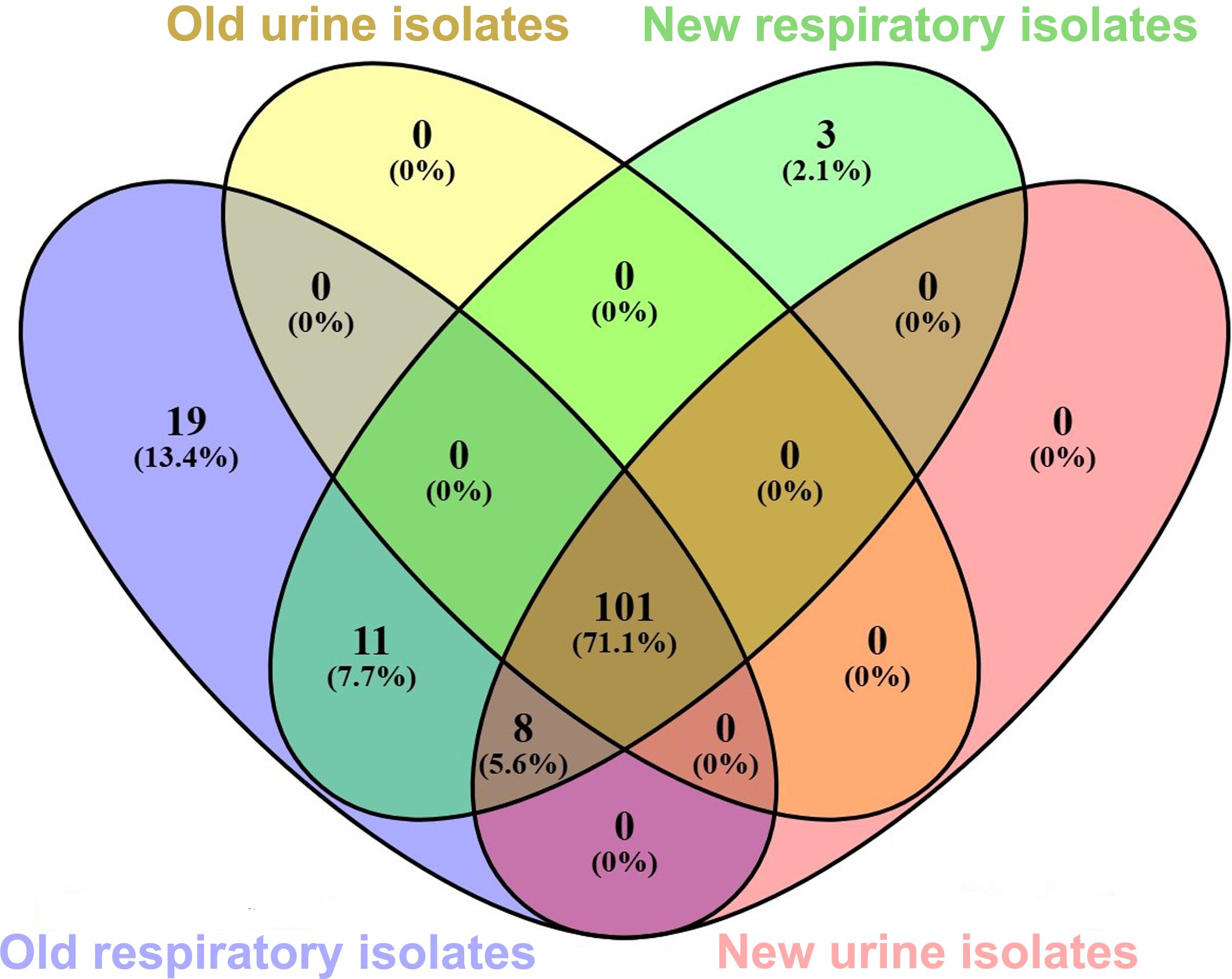
Figure 8. Venn diagram showing the number of common genes shared among the isolate collection. Each group is depicted by a different oval color, as indicated. Genes included are those reported in Figures 1 and 3. Numbers in the non-overlapping regions show the number of genes unique to that strain group. The given percentage was calculated by considering the total number of genes (142) as 100%. The graph was generated using Venn 2.1. online software.
Data availability statement
The datasets presented in this study can be found in online repositories. The names of the repository/repositories and accession number(s) can be found below: https://www.ncbi.nlm.nih.gov/genbank/, PRJNA1173090.
Ethics statement
Ethical approval was not required for the studies on humans in accordance with the local legislation and institutional requirements because only commercially available established cell lines were used.
Author contributions
ADT: Data curation, Formal analysis, Investigation, Methodology, Writing – review & editing. CD: Formal analysis, Investigation, Writing – review & editing, Data curation, Methodology. DS: Conceptualization, Data curation, Formal analysis, Funding acquisition, Investigation, Methodology, Writing – review & editing. CF: Formal analysis, Investigation, Writing – review & editing. DL: Formal analysis, Investigation, Writing – review & editing. SF: Data curation, Methodology, Software, Writing – review & editing. LC: Data curation, Methodology, Software, Writing – review & editing. ML: Data curation, Methodology, Software, Writing – review & editing. FL: Data curation, Methodology, Software, Writing – review & editing. LN: Funding acquisition, Supervision, Writing – review & editing. ATP: Funding acquisition, Supervision, Writing – review & editing. CA: Conceptualization, Data curation, Formal analysis, Funding acquisition, Investigation, Methodology, Writing – original draft.
Funding
The author(s) declare that financial support was received for the research and/or publication of this article. This work was supported by funding from the Italian Ministry of Health (Ricerca Corrente) IRCCS San Raffaele Roma to CA. Salary of DS was supported by POR Lazio FSE2014-2020, from Italian Defense Ministry within the National Plan of Military Research (PNRM) Project n. a2017.129 entitled “Analisi Genomica Resistoma Infezioni Nosocomiali dei Teatri Operativi-AGRINTO”and Sapienza Ateneo funds (RP12218162DFBF0A and RP1221816196D9A2) to CA and to DS, respectively.
Acknowledgments
The authors want to acknowledge support for this project from the Dani Di Giò Foundation-Onlus, Rome, Italy. A special thanks to Dr. Valentina Totino for sharing with us A. baumannii clinical isolates.
Conflict of interest
The authors declare that the research was conducted in the absence of any commercial or financial relationships that could be construed as a potential conflict of interest.
Generative AI statement
The author(s) declare that no Generative AI was used in the creation of this manuscript.
Publisher’s note
All claims expressed in this article are solely those of the authors and do not necessarily represent those of their affiliated organizations, or those of the publisher, the editors and the reviewers. Any product that may be evaluated in this article, or claim that may be made by its manufacturer, is not guaranteed or endorsed by the publisher.
Supplementary material
The Supplementary Material for this article can be found online at: https://www.frontiersin.org/articles/10.3389/fcimb.2025.1527488/full#supplementary-material
References
Alrahmany, D., Omar, A. F., Alreesi, A., Harb, G., Ghazi, I. M. (2022). Acinetobacter baumannii infection-related mortality in hospitalized patients: risk factors and potential targets for clinical and antimicrobial stewardship interventions. Antibiotics. 11, 1086. doi: 10.3390/antibiotics11081086
Ambrose, S. J., Hamidian, M., Hall, R. M. (2023). The extensively antibiotic resistant ST111 Acinetobacter baumannii isolate RBH2 carries an extensive mobile element complement of plasmids, transposons and insertion sequences. Plasmid. 128, 102707. doi: 10.1016/j.plasmid.2023.102707
Ambrosi, C., Aleandri, M., Giordano, A., Scribano, D., Marazzato, M., Zagaglia, C., et al. (2016). Molecular characterization of extensively drug-resistant Acinetobacter baumannii: First report of a new sequence type in Italy. J. OF Global ANTIMICROBIAL RESISTANCE 7, 154–156. doi: 10.1016/j.jgar.2016.10.002
Ambrosi, C., Sarshar, M., Aprea, M. R., Pompilio, A., Di Bonaventura, G., Strati, F., et al. (2019). Colonic adenoma-associated Escherichia coli express specific phenotypes. Microbes AND INFECTION 21, 305–312. doi: 10.1016/j.micinf.2019.02.001
Ambrosi, C., Scribano, D., Aleandri, M., Zagaglia, C., Di Francesco, L., Putignani, L., et al. (2017). Acinetobacter baumannii virulence traits: a comparative study of a novel sequence type with other Italian endemic international clones. Front. Microbiol. 8, 1977. doi: 10.3389/fmicb.2017.01977
Ambrosi, C., Scribano, D., Sarshar, M., Zagaglia, C., Singer, B. B., Palamara, A. T. (2020). Acinetobacter baumannii targets human carcinoembryonic antigen-related cell adhesion molecules (CEACAMs) for invasion of pneumocytes. MSYSTEMS. 5, 1–17. doi: 10.1128/mSystems.00604-20
Bagińska, N., Cieślik, M., Górski, A., Jończyk-Matysiak, E. (2021). The role of antibiotic resistant A. baumannii in the pathogenesis of urinary tract infection and the potential of its treatment with the use of bacteriophage therapy. Antibiotics (Basel Switzerland). 10, 1–15. doi: 10.3390/antibiotics10030281
Bankevich, A., Nurk, S., Antipov, D., Gurevich, A. A., Dvorkin, M., Kulikov, A. S., et al. (2012). SPAdes: a new genome assembly algorithm and its applications to single-cell sequencing. J. Comput. Biol. 19, 455–477. doi: 10.1089/cmb.2012.0021
Bashiri, S., Lucidi, M., Visaggio, D., Capecchi, G., Persichetti, L., Cincotti, G., et al. (2021). Growth phase-and desiccation-dependent Acinetobacter baumannii morphology: an atomic force microscopy investigation. Langmuir. 37, 1110–1119. doi: 10.1021/acs.langmuir.0c02980
Bateman, T. J., Shah, M., Ho, T. P., Shin, H. E., Pan, C., Harris, G., et al. (2021). A Slam-dependent hemophore contributes to heme acquisition in the bacterial pathogen Acinetobacter baumannii. Nat. Commun. 12, 6270. doi: 10.1038/s41467-021-26545-9
Blaschke, U., Skiebe, E., Wilharm, G. (2021). Novel genes required for surface-associated motility in Acinetobacter baumannii. Curr. Microbiol. 78, 1509–1528. doi: 10.1007/s00284-021-02407-x
Bolger, A. M., Lohse, M., Usadel, B. (2014). Trimmomatic: a flexible trimmer for Illumina sequence data. Bioinformatics. 30, 2114–2120. doi: 10.1093/bioinformatics/btu170
Bulens, S. N., Campbell, D., McKay, S. L., Vlachos, N., Burgin, A., Burroughs, M., et al. (2024). Carbapenem-resistant Acinetobacter baumannii complex in the United States—An epidemiological and molecular description of isolates collected through the Emerging Infections Program, 2019. Am. J. Infection Control 52, 1035–1042. doi: 10.1016/j.ajic.2024.04.184
Cain, A. K., Hamidian, M. (2023). Portrait of a killer: Uncovering resistance mechanisms and global spread of Acinetobacter baumannii. PloS Pathogens. 19, e1011520. doi: 10.1371/journal.ppat.1011520
Clemmer, K. M., Bonomo, R. A., Rather, P. N. (2011). Genetic analysis of surface motility in Acinetobacter baumannii. Microbiology. 157, 2534. doi: 10.1099/mic.0.049791-0
Cook-Libin, S., Sykes, E. M., Kornelsen, V., Kumar, A. (2022). Iron acquisition mechanisms and their role in the virulence of Acinetobacter baumannii. Infection Immunity 90, e00223–e00222. doi: 10.1128/iai.00223-22
Corral, J., Pérez-Varela, M., Sánchez-Osuna, M., Cortés, P., Barbé, J., Aranda, J. (2021). Importance of twitching and surface-associated motility in the virulence of Acinetobacter baumannii. Virulence. 12, 2201–2213. doi: 10.1080/21505594.2021.1950268
Cosgaya, C., Ratia, C., Marí-Almirall, M., Rubio, L., Higgins, P. G., Seifert, H., et al. (2019). In vitro and in vivo Virulence Potential of the Emergent Species of the Acinetobacter baumannii (Ab) Group. Front. Microbiol. 10, 2429. doi: 10.3389/fmicb.2019.02429
De Silva, P. M., Kumar, A. (2018). Effect of sodium chloride on surface-associated motility of acinetobacter baumannii and the role of adeRS two-component system. J. membrane Biol. 251, 5–13. doi: 10.1007/s00232-017-9985-7
Duan, Z., Li, X., Li, S., Zhou, H., Hu, L., Xia, H., et al. (2024). Nosocomial surveillance of multidrug-resistant Acinetobacter baumannii: a genomic epidemiological study. Microbiol. Spectrum. 12, e02207–e02223. doi: 10.1128/spectrum.02207-23
Farrow, J. M., III, Wells, G., Pesci, E. C. (2018). Desiccation tolerance in Acinetobacter baumannii is mediated by the two-component response regulator BfmR. PloS One 13, e0205638. doi: 10.1371/journal.pone.0205638
Farshadzadeh, Z., Taheri, B., Rahimi, S., Shoja, S., Pourhajibagher, M., Haghighi, M. A., et al. (2018). Growth rate and biofilm formation ability of clinical and laboratory-evolved colistin-resistant strains of acinetobacter baumannii. Front. Microbiol. 9, 153. doi: 10.3389/fmicb.2018.00153
Fiester, S. E., Arivett, B. A., Schmidt, R. E., Beckett, A. C., Ticak, T., Carrier, M. V., et al. (2016). Iron-regulated phospholipase C activity contributes to the cytolytic activity and virulence of acinetobacter baumannii. PloS One 11, e0167068. doi: 10.1371/journal.pone.0167068
Gaona, M., Corral, J., Campoy, S., Barbé, J., Pérez Varela, M., Aranda, J. (2024). The novel MFS efflux pump SxtP, regulated by the LysR-type transcriptional activator SxtR, is involved in the susceptibility to sulfamethoxazole/trimethoprim (SXT) and the pathogenesis of Acinetobacter baumannii. Antimicrob. Agents Chemother. 68 (10), e0071224. doi: 10.1128/aac.00712-24
Green, E. R., Fakhoury, J. N., Monteith, A. J., Pi, H., Giedroc, D. P., Skaar, E. P. (2022). Bacterial hydrophilins promote pathogen desiccation tolerance. Cell Host Microbe 30, 975–87. e7. doi: 10.1016/j.chom.2022.03.019
Greene, C., Vadlamudi, G., Newton, D., Foxman, B., Xi, C. (2016). The influence of biofilm formation and multidrug resistance on environmental survival of clinical and environmental isolates of Acinetobacter baumannii. Am. J. infection control 44, e65–e71. doi: 10.1016/j.ajic.2015.12.012
Hahm, C., Chung, H.-S., Lee, M. (2022). Whole-genome sequencing for the characterization of resistance mechanisms and epidemiology of colistin-resistant Acinetobacter baumannii. PloS One 17, e0264335. doi: 10.1371/journal.pone.0264335
Halim, J., Carr, R. A., Fliorent, R., Jonnalagadda, K., Kurbonnazarova, M., Kaur, M., et al. (2024). Combinations of antibiotics effective against extensively-and pandrug-resistant Acinetobacter baumannii patient isolates. Microorganisms. 12, 1353. doi: 10.3390/microorganisms12071353
Hamidian, M., Hall, R. M. (2021). Dissemination of novel Tn 7 family transposons carrying genes for synthesis and uptake of fimsbactin siderophores among Acinetobacter baumannii isolates. Microbial Genomics 7, 000548. doi: 10.1099/mgen.0.000548
Hamidian, M., Maharjan, R. P., Farrugia, D. N., Delgado, N. N., Dinh, H., Short, F. L., et al. (2022). Genomic and phenotypic analyses of diverse non-clinical Acinetobacter baumannii strains reveals strain-specific virulence and resistance capacity. Microb. Genom 8, 1–19. doi: 10.1099/mgen.0.000765
Hansen, F., Porsbo, L. J., Frandsen, T. H., Kaygisiz, A. N. S., Roer, L., Henius, A. E., et al. (2023). Characterization of carbapenemase-producing Acinetobacter baumannii isolates from danish patients 2014–2021: detection of a new international clone–IC11. Int. J. Antimicrobial Agents 62, 106866. doi: 10.1016/j.ijantimicag.2023.106866
Holt, K. E., Kenyon, J. J., Hamidian, M., Schultz, M. B., Pickard, D. J., Dougan, G., et al. (2019). Corrigendum: Five decades of genome evolution in the globally distributed, extensively antibiotic-resistant Acinetobacter baumannii global clone 1. Microbial Genomics 5. doi: 10.1099/mgen.0.000280
Hou, P. F., Chen, X. Y., Yan, G. F., Wang, Y. P., Ying, C. M. (2012). Study of the correlation of imipenem resistance with efflux pumps AdeABC, AdeIJK, AdeDE and AbeM in clinical isolates of Acinetobacter baumannii. Chemotherapy. 58, 152–158. doi: 10.1159/000335599
Hu, Y., Zhang, X., Deng, S., Yue, C., Jia, X., Lyu, Y. (2023). Non-antibiotic prevention and treatment against Acinetobacter baumannii infection: Are vaccines and adjuvants effective strategies? Front. Microbiol. 14, 1049917. doi: 10.3389/fmicb.2023.1049917
Huys, G., Cnockaert, M., Vaneechoutte, M., Woodford, N., Nemec, A., Dijkshoorn, L., et al. (2005). Distribution of tetracycline resistance genes in genotypically related and unrelated multiresistant Acinetobacter baumannii strains from different European hospitals. Res. Microbiol. 156, 348–355. doi: 10.1016/j.resmic.2004.10.008
Jackson-Litteken, C. D., Di Venanzio, G., Le, N.-H., Scott, N. E., Djahanschiri, B., Distel, J. S., et al. (2022). InvL, an invasin-like adhesin, is a type II secretion system substrate required for Acinetobacter baumannii uropathogenesis. MBio. 13, e00258–e00222. doi: 10.1128/mbio.00258-22
Jeong, G.-J., Khan, F., Tabassum, N., Kim, Y.-M. (2024). Motility of Acinetobacter baumannii: Regulatory systems and controlling strategies. Appl. Microbiol. Biotechnol. 108, 3. doi: 10.1007/s00253-023-12975-6
Johnson, T. L., Waack, U., Smith, S., Mobley, H., Sandkvist, M. (2016). Acinetobacter baumannii is dependent on the type II secretion system and its substrate LipA for lipid utilization and in vivo fitness. J. bacteriology. 198, 711–719. doi: 10.1128/JB.00622-15
Karah, N., Faille, N., Grenier, F., Fayad, A. A., Higgins, P. G., Al-Hassan, L., et al. (2023). Emergence of Acinetobacter baumannii International Clone 10 predominantly found in the Middle East. bioRxiv. 2023, 10. 09.561570. doi: 10.1101/2023.10.09.561570
Kim, M., Park, J., Park, W. (2021). Genomic and phenotypic analyses of multidrug-resistant Acinetobacter baumannii NCCP 16007 isolated from a patient with a urinary tract infection. Virulence. 12, 150–164. doi: 10.1080/21505594.2020.1867421
Kong, X., Chen, T., Guo, L., Zhou, Y., Lu, P., Xiao, Y. (2023). Phenotypic and genomic comparison of dominant and nondominant sequence-type of Acinetobacter baumannii isolated in China. Front. Cell Infect. Microbiol. 13, 1118285. doi: 10.3389/fcimb.2023.1118285
Liao, J., Qi, Q., Kuang, L., Zhou, Y., Xiao, Q., Liu, T., et al. (2024). Chloramphenicol binding sites of acinetobacter baumannii chloramphenicol acetyltransferase catB8. ACS Infect. Diseases. 10, 870–878. doi: 10.1021/acsinfecdis.3c00359
Marchand, I., Damier-Piolle, L., Courvalin, P., Lambert, T. (2004). Expression of the RND-type efflux pump AdeABC in Acinetobacter baumannii is regulated by the AdeRS two-component system. Antimicrobial Agents chemotherapy. 48, 3298–3304. doi: 10.1128/AAC.48.9.3298-3304.2004
Müller, C., Reuter, S., Wille, J., Xanthopoulou, K., Stefanik, D., Grundmann, H., et al. (2023). A global view on carbapenem-resistant Acinetobacter baumannii. Mbio. 14, e02260–e02223. doi: 10.1128/mbio.02260-23
Murray, C. J., Ikuta, K. S., Sharara, F., Swetschinski, L., Aguilar, G. R., Gray, A., et al. (2022). Global burden of bacterial antimicrobial resistance in 2019: a systematic analysis. Lancet 399, 629–655. doi: 10.1016/S0140-6736(21)02724-0
Neidhöfer, C., Neuenhoff, M., Jozič, R., Atangcho, B., Unsleber, S., Neder, U., et al. (2023). Exploring clonality and virulence gene associations in bloodstream infections using whole-genome sequencing and clinical data. Front. Cell. Infection Microbiol. 13, 1274573. doi: 10.3389/fcimb.2023.1274573
Obenhuber, T., Scheier, T. C., Stutz, T., Hug, M., Fontein, D., Kaiser, A., et al. (2024). An outbreak of multi-drug-resistant Acinetobacter baumannii on a burns ICU and its control with multi-faceted containment measures. J. Hosp. Infection. 146, 102–108. doi: 10.1016/j.jhin.2024.01.002
Penesyan, A., Nagy, S. S., Kjelleberg, S., Gillings, M. R., Paulsen, I. T. (2019). Rapid microevolution of biofilm cells in response to antibiotics. NPJ biofilms microbiomes. 5, 34. doi: 10.1038/s41522-019-0108-3
Pérez-Varela, M., Corral, J., Aranda, J., Barbé, J. (2019). Roles of efflux pumps from different superfamilies in the surface-associated motility and virulence of acinetobacter baumannii ATCC 17978. Antimicrob. Agents Chemother. 63, 1–11. doi: 10.1128/AAC.02190-18
Pompilio, A., Scribano, D., Sarshar, M., Di Bonaventura, G., Palamara, A. T., Ambrosi, C. (2021). Gram-negative bacteria holding together in a biofilm: the Acinetobacter baumannii way. Microorganisms. 9, 1353. doi: 10.3390/microorganisms9071353
Rubio, T., Gagné, S., Debruyne, C., Dias, C., Cluzel, C., Mongellaz, D., et al. (2022). Incidence of an Intracellular Multiplication Niche among Acinetobacter baumannii Clinical Isolates. Msystems. 7, e00488–e00421. doi: 10.1128/msystems.00488-21
Salehi, B., Ghalavand, Z., Yadegar, A., Eslami, G. (2021). Characteristics and diversity of mutations in regulatory genes of resistance-nodulation-cell division efflux pumps in association with drug-resistant clinical isolates of Acinetobacter baumannii. Antimicrobial Resistance Infection Control 10, 1–12. doi: 10.1186/s13756-021-00924-9
Sarshar, M., Behzadi, P., Ambrosi, C., Zagaglia, C., Palamara, A. T., Scribano, D. (2020). FimH and anti-adhesive therapeutics: A disarming strategy against uropathogens. ANTIBIOTICS. 9, 1–16. doi: 10.3390/antibiotics9070397
Sarshar, M., Behzadi, P., Scribano, D., Teresa, P. A., Ambrosi, C. (2021). Acinetobacter baumannii: an ancient commensal with weapons of a pathogen. PATHOGENS. 10, 1–25. doi: 10.3390/pathogens10040387
Schramm, S. T. J., Place, K., Montaña, S., Almuzara, M., Fung, S., Fernandez, J. S., et al. (2019). Genetic and phenotypic features of a novel acinetobacter species, strain A47, isolated from the clinical setting. Front. Microbiol. 10, 1375. doi: 10.3389/fmicb.2019.01375
Scribano, D., Cheri, E., Pompilio, A., Di Bonaventura, G., Belli, M., Cristina, M., et al. (2024). Acinetobacter baumannii OmpA-like porins: functional characterization of bacterial physiology, antibiotic-resistance, and virulence. Commun. Biol. 7, 948. doi: 10.1038/s42003-024-06645-0
Scribano, D., Marzano, V., Levi Mortera, S., Sarshar, M., Vernocchi, P., Zagaglia, C., et al. (2019). Insights into the Periplasmic Proteins of Acinetobacter baumannii AB5075 and the Impact of Imipenem Exposure: A Proteomic Approach. Int J Mol Sci. 20 (14), 3451. doi: 10.3390/ijms20143451
Shafaati, M., Boroumand, M., Nowroozi, J., Amiri, P., Kazemian, H. (2016). Correlation between qacE and qacEΔ1 efflux pump genes, antibiotic and disinfectant resistant among clinical isolates of E. coli. Recent patents anti-infective Drug discovery. 11, 189–195. doi: 10.2174/1574891X11666160815094718
Sharma, A., Sharma, R., Bhattacharyya, T., Bhando, T., Pathania, R. (2016). Fosfomycin resistance in Acinetobacter baumannii is mediated by efflux through a major facilitator superfamily (MFS) transporter—AbaF. J. Antimicrobial Chemotherapy. 72, 68–74. doi: 10.1093/jac/dkw382
Sheldon, J. R., Skaar, E. P. (2020). Acinetobacter baumannii can use multiple siderophores for iron acquisition, but only acinetobactin is required for virulence. PloS pathogens. 16, e1008995. doi: 10.1371/journal.ppat.1008995
Shields, R. K., Paterson, D. L., Tamma, P. D. (2023). Navigating available treatment options for carbapenem-resistant Acinetobacter baumannii-calcoaceticus complex infections. Clin. Infect. Dis. 76, S179–SS93. doi: 10.1093/cid/ciad094
Short, F. L., Liu, Q., Shah, B., Clift, H. E., Naidu, V., Li, L., et al. (2021). The Acinetobacter baumannii disinfectant resistance protein, AmvA, is a spermidine and spermine efflux pump. Commun. Biol. 4, 1114. doi: 10.1038/s42003-021-02629-6
Singh, J. K., Adams, F. G., Brown, M. H. (2019). Diversity and function of capsular polysaccharide in Acinetobacter baumannii. Front. Microbiol. 9, 3301. doi: 10.3389/fmicb.2018.03301
Stracquadanio, S., Bonomo, C., Marino, A., Bongiorno, D., Privitera, G. F., Bivona, D. A., et al. (2022). Acinetobacter baumannii and cefiderocol, between cidality and adaptability. Microbiol. Spectrum. 10, e02347–e02322. doi: 10.1128/spectrum.02347-22
Sun, B., Liu, H., Jiang, Y., Shao, L., Yang, S., Chen, D. (2020). New mutations involved in colistin resistance in Acinetobacter baumannii. Msphere. 5, 1–14. doi: 10.1128/msphere.00895-19
Taguchi, A., Welsh, M. A., Marmont, L. S., Lee, W., Sjodt, M., Kruse, A. C., et al. (2019). FtsW is a peptidoglycan polymerase that is functional only in complex with its cognate penicillin-binding protein. Nat. Microbiol. 4, 587–594. doi: 10.1038/s41564-018-0345-x
Tipton, K. A., Chin, C.-Y., Farokhyfar, M., Weiss, D. S., Rather, P. N. (2018). Role of capsule in resistance to disinfectants, host antimicrobials, and desiccation in Acinetobacter baumannii. Antimicrobial Agents Chemotherapy. 62, 1–6. doi: 10.1128/aac.01188-18
Valcek, A., Philippe, C., Whiteway, C., Robino, E., Nesporova, K., Bové, M., et al. (2023). Phenotypic Characterization and Heterogeneity among Modern Clinical Isolates of Acinetobacter baumannii. Microbiol. Spectr. 11, e0306122. doi: 10.1128/spectrum.03061-22
Valencia-Martín, R., Gonzalez-Galan, V., Álvarez-Marín, R., Cazalla-Foncueva, A., Aldabó, T., Gil-Navarro, M. V., et al. (2019). A multimodal intervention program to control a long-term Acinetobacter baumannii endemic in a tertiary care hospital. Antimicrobial Resistance Infection Control 8, 1–11. doi: 10.1186/s13756-019-0658-4
Waack, U., Johnson, T. L., Chedid, K., Xi, C., Simmons, L. A., Mobley, H. L., et al. (2017). Targeting the type II secretion system: development, optimization, and validation of a high-throughput screen for the identification of small molecule inhibitors. Front. Cell. Infection Microbiol. 7, 380. doi: 10.3389/fcimb.2017.00380
Waite, A. J., Frankel, N. W., Emonet, T. (2018). Behavioral variability and phenotypic diversity in bacterial chemotaxis. Annu. Rev. biophysics. 47, 595–616. doi: 10.1146/annurev-biophys-062215-010954
Warburton, P. J., Ciric, L., Lerner, A., Seville, L. A., Roberts, A. P., Mullany, P., et al. (2013). TetAB (46), a predicted heterodimeric ABC transporter conferring tetracycline resistance in Streptococcus australis isolated from the oral cavity. J. Antimicrobial Chemotherapy. 68, 17–22. doi: 10.1093/jac/dks351
Yang, Z., Liu, X., Shi, Y., Yin, S., Shen, W., Chen, J., et al. (2019). Characterization and genome annotation of a newly detected bacteriophage infecting multidrug-resistant Acinetobacter baumannii. Arch. virology. 164, 1527–1533. doi: 10.1007/s00705-019-04213-0
Yang, C. H., Su, P. W., Moi, S. H., Chuang, L. Y. (2019). Biofilm formation in acinetobacter baumannii: genotype-phenotype correlation. Molecules. 24, 1–12. doi: 10.3390/molecules24101849
Zeighami, H., Valadkhani, F., Shapouri, R., Samadi, E., Haghi, F. (2019). Virulence characteristics of multidrug resistant biofilm forming Acinetobacter baumannii isolated from intensive care unit patients. BMC Infect. diseases. 19, 1–9. doi: 10.1186/s12879-019-4272-0
Keywords: Acinetobacter baumannii, motility, biofilm, desiccation, host-pathogen interaction, phenotypic and genotypic comparison
Citation: Tagueha AD, D’Agostini C, Scribano D, Fiorilla C, Limongi D, Fillo S, Corrent L, Lipari M, Lista F, Nencioni L, Palamara AT and Ambrosi C (2025) A decade of genomic and phenotypic adaptation of carbapenem-resistant Acinetobacter baumannii. Front. Cell. Infect. Microbiol. 15:1527488. doi: 10.3389/fcimb.2025.1527488
Received: 13 November 2024; Accepted: 08 April 2025;
Published: 30 April 2025.
Edited by:
Sandip Patil, Shenzhen Children’s Hospital, ChinaReviewed by:
Humberto Barrios Camacho, National Institute of Public Health, MexicoAlfizah Hanafiah, Universiti Kebangsaan Malaysia Medical Center (UKMMC), Malaysia
Copyright © 2025 Tagueha, D’Agostini, Scribano, Fiorilla, Limongi, Fillo, Corrent, Lipari, Lista, Nencioni, Palamara and Ambrosi. This is an open-access article distributed under the terms of the Creative Commons Attribution License (CC BY). The use, distribution or reproduction in other forums is permitted, provided the original author(s) and the copyright owner(s) are credited and that the original publication in this journal is cited, in accordance with accepted academic practice. No use, distribution or reproduction is permitted which does not comply with these terms.
*Correspondence: Cecilia Ambrosi, Y2VjaWxpYS5hbWJyb3NpQHVuaXJvbWE1Lml0
†These authors have contributed equally to this work
‡ORCID: Cartesio D’Agostini, https://orcid.org/0000-0001-9199-3340