- 1Institute for Microbiology, University of Veterinary Medicine Hannover, Foundation, Hannover, Germany
- 2Department of Biotechnology and Biomedicine, Section for Medical Biotechnology, Technical University of Denmark, Kgs. Lyngby, Denmark
- 3Clinic for Poultry, University of Veterinary Medicine Hannover, Foundation, Hannover, Germany
Respiratory infections of livestock represent a major health issue for the animals and cause high economic losses for the farmers. Still, little is known about the intricate interactions between host cells and the many different pathogens that cause respiratory diseases, leaving a substantial knowledge gap to be filled in order to develop effective therapies. Immortalized cell lines and two-dimensional cultures of primary respiratory epithelial cells do not reflect the complex architecture and functionality of the respiratory tract tissues. Thus, it is essential to develop and apply appropriate primary cell culture systems to study respiratory diseases. In human research, the use of complex cell culture systems, such as air-liquid interface (ALI) cultures, organoids and lung-on-chip, has proceeded significantly during the last years, whereas in veterinary research, these models are only rarely used. Nevertheless, there are several three-dimensional, primary cell culture systems available to study respiratory infections of livestock. Here, we give an overview on models that are currently used in this field: nasal mucosa explants, tracheal organ cultures, ALI cultures, and precision-cut lung slices. All these models align with the 3R principle, as they can replace animal experiments to some extent and the tissue material for these culture systems can be obtained from abattoirs or veterinary research facilities. We aim to encourage other researchers to use these versatile cell culture systems to drive investigations of respiratory tract infections of livestock forward. Finally, these models are not limited to infection research, but can also be applied in other research fields and can be transferred to other animal species than livestock.
1 Introduction
Respiratory infections of livestock cause major problems with regard to animal welfare and economic losses. In order to improve understanding of host-pathogen interactions, extensive research on pathogens, virulence factors, and the host’s immune response towards infection with respiratory pathogens is necessary. One of the most common methods to study host-pathogen interactions is the use of experimental animal models. However, this is ethically debatable mainly due to animal welfare, limited value for studying the early stages of disease, and, when using laboratory animals, the restricted transferability to the natural host species. Moreover, reproducibility of results, required time, and practicability make in vivo studies challenging (Steukers et al., 2011; Di Teodoro et al., 2018). Thus, suitable in vitro and ex vivo models, which resemble the in vivo situation and apply the 3R (replacement, reduction, and refinement) principle of Russel and Burch (Russell and Burch, 1959) are mandatory to study host-pathogen interactions.
Immortalized cell lines and two-dimensional (2D) cell culture models do not reflect the complexity of the respiratory tract and are therefore suboptimal for the study of host-pathogen interactions. Luckily, more reliable in vitro models of the respiratory tract have been developed and improved significantly during the last years, especially in human research due to the COVID-19 pandemic. Commonly used in vitro models in human research are primary human airway epithelial cells cultured under air-liquid interface (ALI) conditions, organoids, lung-on-chip, and precision-cut lung slices (PCLS) (Dichtl et al., 2024; Mahieu et al., 2024). However, the establishment and maintenance of organoids and lung-on-chip is quite challenging and expensive, and in veterinary science, these models are yet only rarely used.
Detailed reviews on complex human cell cultures systems have been published in the past (Cao et al., 2021; Viana et al., 2022) but to our knowledge, no reviews on primary cell culture systems from livestock are available. Thus, in this review, we present four different primary cell culture systems of the upper and lower respiratory tract that have been proven suitable for the study of host-pathogen interactions in respiratory infections of livestock – nasal mucosa explants (NME), tracheal organ cultures (TOC), ALI cultures, and PCLS. We focus on bacterial respiratory infections as there is currently a lack of studies on veterinary bacterial pathogens, and we want to encourage researchers to fill this knowledge gap in the future by using these in vitro/ex vivo models instead of animal experiments. For each model, we describe preparation and maintenance, possible applications, as well as limitations and future perspectives.
2 Nasal mucosa explants
NMEs for the study of diverse aspects of respiratory colonization and infection have been established from various livestock species, including pigs (Pol et al., 1991; Glazenburg et al., 1995; Glorieux et al., 2007; Van Poucke et al., 2010; Frydas et al., 2013; Tulinski et al., 2013; Starbæk et al., 2018), horses (Vandekerckhove et al., 2009; Glorieux et al., 2012; Vairo et al., 2013; Baghi and Nauwynck, 2014; Van Cleemput et al., 2019), cattle (Niesalla et al., 2009; Steukers et al., 2012; Yang et al., 2019), and sheep (Mazzetto et al., 2020; Zheng et al., 2023), but also humans (Read et al., 1995; Jackson et al., 1996; Jang et al., 2005; Wang et al., 2012; Cantero et al., 2013b; Gordhan et al., 2023). The nasal mucosa is the site of first contact for many pathogens and colonizing bacteria. Being able to study these pathogens using models that mimic this anatomical site is therefore of high importance, and the application of nasal mucosal tissue explant culture in the study of the initial interactions between host and pathogen thus have obvious benefits. NMEs are a 3R compliant ex vivo model as many NMEs can be obtained from one animal, thus attaining both replacement and reduction relative to applying in vivo experimentation. Moreover, in contrast to cell culture using immortalized or even primary nasal epithelial cells, NMEs carry the benefit of retaining the sophisticated three-dimensional (3D) structure of the nasal mucosal epithelium.
2.1 Preparation and maintenance
A guiding protocol for preparation and inoculation of NMEs can be found in Supplementary Material 1.
The NME model is easily accessible to researchers, as it would usually not require any highly specialized lab equipment and can be set up in any standard cell culture lab. Tissue may be obtained at veterinary research facilities as the animals head will often be medical “waste” from experimental/educational surgeries, or it can be obtained from slaughterhouses. Euthanization of animals solely for the purpose of harvesting tissue for NMEs can thereby be avoided. NMEs are obtained by exposing the interior of the nasal cavity and carefully stripping the mucosal layer from the cartilage of e.g. the nasal septum and/or conchae (Figures 1). Tissue sheets are divided into samples of equal size, often approximately 2.5 cm² (Glazenburg et al., 1995), to be cultured individually. Depending on the species, age, and size, a fairly large number (often > 20) of NMEs may be obtained from one animal. The preparation of individual NMEs is usually followed by an acclimatization period of up to 24 hours. Exposure to pathogen(s) to start infection or colonization of NMEs is often achieved by incubating the tissue fully submerged in medium containing bacteria or virus for approx. 1–2 hours (Figure 1). Early studies including the use of NMEs have reported maintaining the tissue fully submerged in culture medium throughout the experiment (Pol et al., 1991; Glazenburg et al., 1995), but the more common practice is to place the NME apical side up on a scaffold and maintain it in an air-liquid interphase culture, mimicking in vivo conditions (Figure 1). Various types of scaffolds have been described, including fine-meshed metal gauze scaffolds (Glorieux et al., 2007; Oh et al., 2023; Stadejek et al., 2023), gelfoam blocks saturated with culture medium (Jang et al., 2005; Cantero et al., 2013b), cell-strainers adapted to serve as scaffold (Starbæk et al., 2018), transwell culture plate inserts (Zheng et al., 2023), and agarose plugs (Niesalla et al., 2009). It is often reported that a combination of RPMI-1640 medium and Dulbecco’s Modified Eagle Medium (DMEM) is used for culturing NMEs, usually supplemented with antibiotics when applying NMEs for the study of viral pathogens. For the study of bacterial pathogens, the acclimatization process may take place in the presence of antibiotics, but prior infection a thorough washing and an additional period of culturing in antibiotics-free medium are essential (Tulinski et al., 2013). Moreover, it has to be taken into account that animals often received antibiotics in the days prior to euthanization.
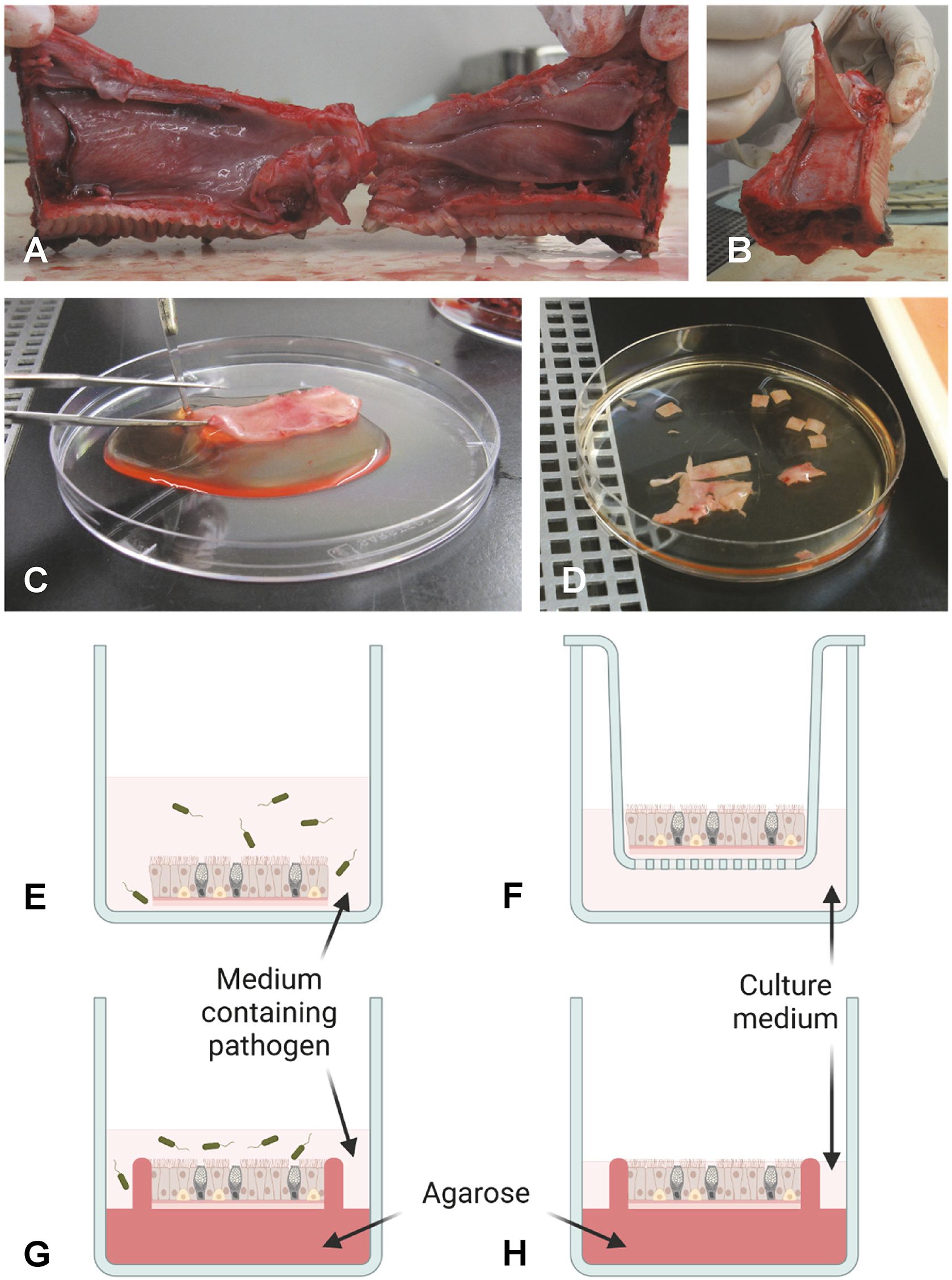
Figure 1. Schematic overview of preparation and culturing of NMEs. As an example, a pig snout is shown in (A, B). To obtain NMEs, the interior of the nasal cavity is exposed (A) and the mucosal layer is stripped from the cartilage (B, C). The tissue is then cut into appropriately sized pieces (NMEs) (D) and inoculated by submerging the NME in medium containing pathogen (E). Following incubation and washing, the NME is placed on a scaffold (shown here as a transwell culture plate insert) and cultured at the air-liquid interphase (F). Alternatively, a polarized setup can be employed. Prior to inoculation, the NME can be embedded in agarose to occlude the basolateral and side surfaces of the NME, thus allowing infection to occur only from the apical side (G). Following incubation and washing, the embedded NME is cultured at the air-liquid interphase in fresh medium (H). Drawings in panels G and H have been prepared taking inspiration from Frydas et al (Frydas et al., 2013). Photos provided by L. Brogaard, Department of Biotechnology and Biomedicine, Section for Medical Biotechnology, Technical University of Denmark, Kgs. Lyngby, Denmark. Created by L. Brogaard in BioRender. https://BioRender.com/dwhk64n.
Importantly, the natural route of infection should be considered when applying the pathogen(s), i.e. either from the apical or the basolateral surface of the epithelium. To ensure access for the pathogen only to the apical side of NME during inoculation, polarized NME cultures have been established from pigs and horses, as well as humans (Read et al., 1995; Frydas et al., 2013; Vairo et al., 2013). To achieve this, the tissue is embedded in agarose, leaving only the epithelial surface exposed during inoculation, thus more closely mimicking the in vivo situation (Figures 1E).
Viability of the tissue is commonly assessed by confirming ciliary beating and checking for apoptotic cells by staining. Tissue integrity can be evaluated by monitoring tissue morphometrics, e.g. cell composition and epithelial thickness. These parameters indicate that the NME model is stable and viable in culture for up to 96 hours after isolation or even longer (Vandekerckhove et al., 2009; Van Poucke et al., 2010; Zhao et al., 2016).
2.2 Applications
Studies employing NMEs for investigation of viral pathogens far outnumber studies of bacterial pathogens. Studies on herpesviruses dominate equine and bovine NME literature, while porcine NMEs have been used for the study of influenza viruses, porcine reproductive and respiratory syndrome virus, African swine fever virus, as well as herpesvirus (pseudorabies virus). However, porcine as well as ovine NMEs have also been used for investigation of bacterial colonization. Porcine NMEs have been demonstrated to be a useful tool for investigation of Staphylococcus aureus colonization (Tulinski et al., 2013), and have been used to provide temporal resolution of S. aureus gene expression during the early phase of colonization and evaluate the effect of bacteriophage treatment on reduction of livestock-associated methicillin-resistant S. aureus colonization (Tulinski et al., 2014; Verstappen et al., 2016). In ovine NMEs, the antiviral effect of bacterial colonizers of the nasal mucosa has been investigated, suggesting that Bacillus subtilis-induced expression of antiviral factors contributed to restricting pseudorabies virus infection in the NME model (Zheng et al., 2023). The studies of bacteriophage treatment against S. aureus and antiviral effect of B. subtilis highlight the application of NMEs for evaluation of intervention strategies against pathogens that target the nasal mucosa, even though such studies are yet sparse. In addition to those already mentioned, just one other study investigating the antiviral effect of A-5021 [(10S,20R)-9-[[10,20-bis(hydroxymethyl)cycloprop-10-yl]methyl]guanine] on equine herpesvirus-1 in equine NMEs has utilized this potential of the model (Glorieux et al., 2012). Also highlighted by the study of the antiviral effect of B. subtilis in ovine NMEs is that NMEs may provide an excellent tool for investigating co-infections or interactions between colonizers and (opportunistic) pathogens. The nasal mucosal microbiota is a complex and diverse community, and any infection by an inhaled or opportunistic pathogen would always occur in the presence of colonizers that may potentially exacerbate or inhibit the infection. The fact that NMEs can be colonized by relevant bacteria in vitro promotes their application in this context. However, this possibility has so far not been extensively explored in livestock NMEs, whereas human NMEs have been utilized to investigate the effect of influenza B virus on the association of Neisseria meningitidis with the nasal mucosa and to demonstrate the role of herpes simplex virus type 1 in facilitating S. aureus invasion of the nasal mucosa (Read et al., 1999; Wang et al., 2012).
2.3 Limitations and future perspectives
Inherently, the NME model will comprise tissue resident immune cells, such as dendritic cells and macrophages, as well as mucus-secreting goblet cells, which can be significant for the model’s ability to accurately mimic the conditions encountered by a pathogen or commensal colonizer during first and early contact with the host’s nasal mucosa. However, it has to be considered that recruitment of circulating lymphocytes to the site of infection does obviously not occur which limits its use for studying immune responses during later stages of infection.
Most studies employing NMEs have been focused on characterizing aspects of the pathogen under investigation, e.g. replication dynamics, infectivity, tissue and cell tropism, and pathogen gene expression dynamics during the infection. Some studies have also utilized the NME model to investigate the host response during infection. Human NMEs have been successfully used to characterize the innate immune response to S. aureus biofilm formation, showing that the bacterium induces a predominantly pro-inflammatory state in the nasal mucosa early in the process (Cantero et al., 2013a, Cantero et al., 2015). Various aspects of the host response during viral infections have also been investigated using livestock-derived NMEs, e.g. the migration of tissue resident immune cells in equine NMEs during equine herpesvirus-1 infection and the potential relevance for systemic viral spread (Baghi and Nauwynck, 2014; Zhao et al., 2017). Porcine NMEs have been used to characterize host antiviral gene expression during influenza A virus infection in a study that, in part, investigated the ability of the NME model to accurately mimic the in vivo antiviral innate immune response. Moreover, this study also evaluated technical aspects such as inter-experiment variation and importantly the effect on host transcription of keeping the nasal mucosal tissue in culture for several days (Starbæk et al., 2018). The authors concluded that the process of harvesting and culturing the tissue had significant impact on expression of genes related to e.g. inflammation and apoptosis, even in the absence of viral infection. This is important when using the NME model for investigation of pathogen or host gene expressions, as the culturing process itself may influence the results. Nonetheless, the NME model has been proved a valuable tool in facilitating sophisticated investigation of e.g., viral replication and host cell tropism, viral-bacterial co-/super-infection, effect of biofilm on host nasal mucosa, mapping host and pathogen transcriptional landscapes during colonization or infection, and efficiency of antiviral drug candidates in inhibition of viral infection and migration. The NME model does however seem somewhat under-utilized for investigation of bacterial infection and colonization, but it has potential to become a relevant tool for this purpose in the future.
3 Tracheal organ cultures
TOC have been described in the literature from human (Dolin and Smith, 1975) and various animal species, including laboratory animals (Wolterbeek et al., 1996; Kishimoto et al., 2021), domestic animals (Leeming et al., 2006) as well as from livestock (Thomas et al., 1987; DeBey and Ross, 1994; Niang et al., 1998). Notably, the majority of these publications is about TOC from chicken and other birds. This could be due to the fact that suitable culture conditions for air-liquid interface (ALI) cultures of the bird’s respiratory tract have not been published so far and only few studies describe the use of precision-cut lung slices (PCLS) of immature chicken embryos to investigate pathogen-host interactions (Abd El Rahman et al., 2010; Bryson et al., 2020). Thus, in poultry research, TOC represent an alternative model to investigate the avian upper respiratory tract in the context of infections with especially viruses, bacteria, but also parasites and have been used for over 50 years (Colwell and Lukert, 1969; Abdul-Wahab et al., 1996; Zhang et al., 2012; Knab et al., 2020). TOC were also applied in the context of pharmacological investigations addressing decongestants and mucolytic agents or mycotoxins (Cardeilhac et al., 1972; Dudley and Cherry, 1977). TOC can be considered a highly reproducible ex vivo model with a cell composition closely mimicking the in vivo situation in birds and other animal species. However, in this review, we will focus on the preparation and application of TOC in poultry research as literature on other primary cell culture models in this research area is sparse.
3.1 Preparation and maintenance
TOC can be prepared from embryos or post-hatched birds (de Wit et al., 2013; Dowgier and Bickerton, 2020), the latter playing an important role in the evaluation of vaccine efficacy against especially infectious bronchitis virus infection in chickens. TOC have been prepared from various bird species (Colwell et al., 1973; Petersen et al., 2012) and species-specific but also age-related differences may be observed in the duration and intensity of ciliary activity. Pheasant TOC have shown ciliary activity up to 70 days, and TOC derived from chicken embryos maintained ciliary activity longer compared to day-old chicks (Gerganov and Surtmadzhiev, 1982a). For the collection of the trachea, embryos at about 1–2 days before hatch or post-hatch birds are humanely sacrificed. The trachea is immediately carefully removed and placed in pre-warmed medium (for example Medium 199 with Hank’s salts supplemented with antibiotics). Subsequently, the trachea will be placed in pre-warmed medium in a petri dish on sterile filter paper (Figures 2). Ex vivo the trachea will be stripped off connective tissue (Figure 2), and subsequently rings of about 1 mm thickness will be cut manually with a sharp microtome blade or with a tissue chopper (Figure 2) (Hennion, 2015). The rings are placed in pre-warmed medium. Single rings can be incubated in 5 ml sterile plastic tubes with 1 ml medium (Figure 2), and incubated at 37-38°C in an overhead rotating rack at the lowest rotating speed or in roller cell culture bottles to prevent accumulation of mucus within the lumen of the ring (Jones and Hennion, 2008; Hennion, 2015), which prolongs survival of the rings compared to static cultures. After about four days, when stress related (innate immune) responses of the TOC due to the preparation process have waned (Reemers et al., 2009), infection studies can be initiated. Importantly, it has to be considered that even from embryos the tracheae may not be fully sterile, although bacteria may not be detected by classical microbiological procedures, microflora may be identified by Illumina sequencing (Taylor et al., 2020; Ding et al., 2022; Faldynova et al., 2024). Therefore, some effects of this microflora on the structural and non-structural cells including innate immune reactions of the TOC cannot be ruled out. Tissue can remain viable at least four weeks, or, depending on the age or species the TOC were collected from, shorter or longer (Cherry and Taylor-Robinson, 1970). Viability can be confirmed by ciliary activity, which is investigated by using an inverted microscope. For evaluation of ciliary beats, the ring is divided into 10% sections. The ciliary activity is documented for each section, which adds up to total ciliary activity. About 100% ciliary activity is expected for intact TOC, while it wanes in infected or dying tissue until total ciliostasis with or without loss of cilia may be observed. Depending on the experimental protocol, the media may have to be changed when cultures are maintained for an extended period.
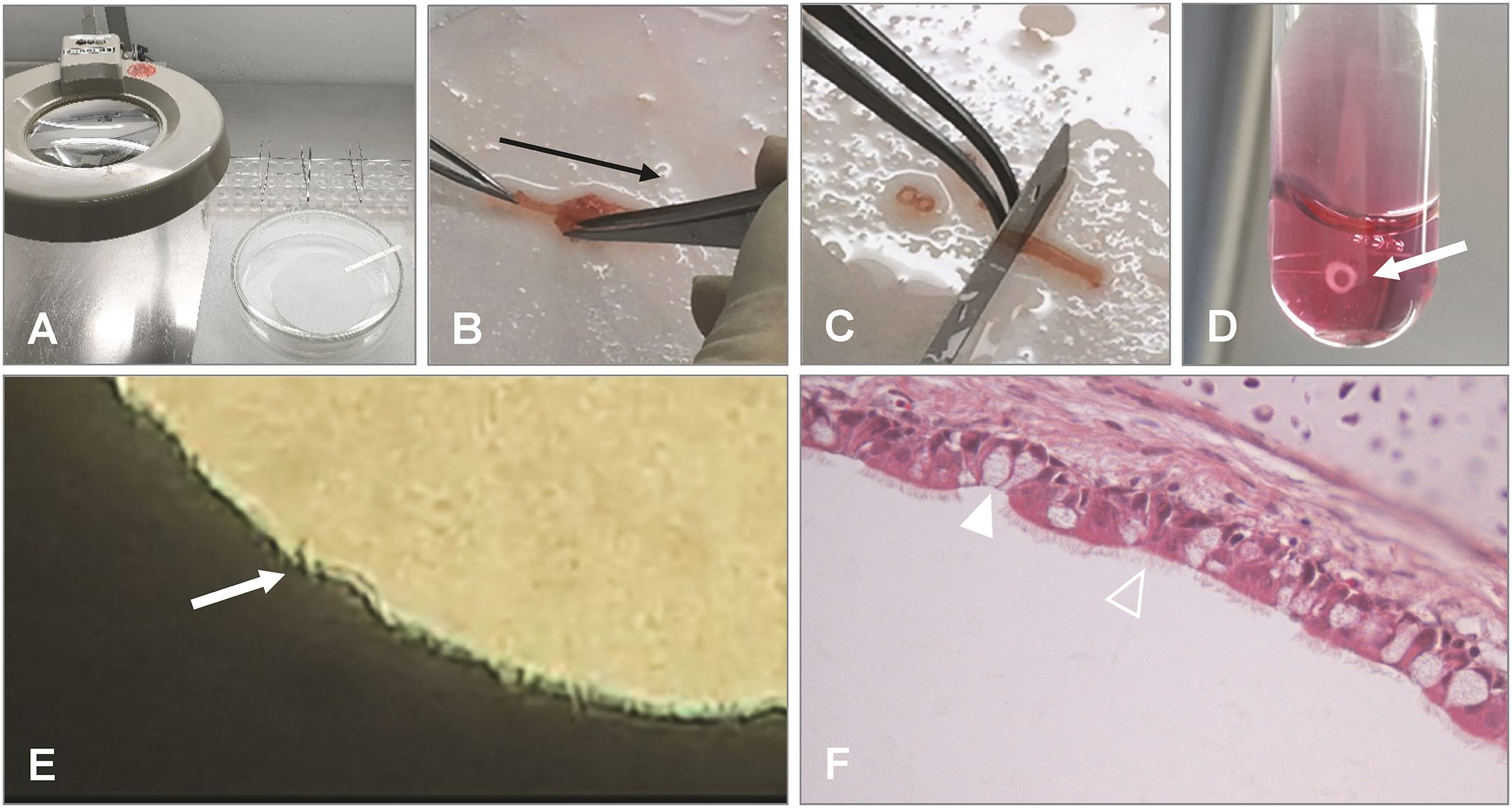
Figure 2. Preparation of chicken TOC. (A) Preparation should be done under a magnification glass in a petri dish with pre-warmed medium and on filter paper. (B) After removal of the trachea from the embryo or post hatch bird, connective tissue is stripped off (black arrow indicates direction of stripping). (C) Cutting of the trachea into 0.8–1 mm evenly thick rings with a sharp blade manually or with a tissue chopper. (D) Placement of the ring in pre-warmed medium (arrow indicates TOC) and incubation in an overhead rotator at 37°C. (E) Microscopical evaluation of the tracheal ring after incubation for ciliary activity using an inverted microscope (arrow indicates cilia and a clear lumen of the ring without mucus accumulation). (F) Histological section of the TOC showing goblet cells (filled arrowhead) and epithelial cells with cilia (open arrowhead). Photos provided by N. Rüger and S. Rautenschlein, Clinic for Poultry, University of Veterinary Medicine Hannover.
For a detailed protocol, the reader is referred to the publication by Hennion et al. (Hennion, 2015).
3.2 Application
TOC have traditionally been used for virus propagation and titration (Cook et al., 1976; Dowgier and Bickerton, 2020), but they are also applicable for the investigation of host-pathogen interactions. Ciliary activity is an important read-out parameter to assess the impact of an infection on the tissue viability. Thus, only rings with full ciliary activity should be used for experimental infections. Histopathological examination of the TOC can be performed to identify pathogen-induced lesion development including loss of cilia and epithelial cells, edema of the epithelial layer or changes in the number of goblet cells (Figure 2). Pathogens may be identified by (microbiological) culture, molecular methods (including quantitative PCR (qPCR), in situ hybridization) or by antigen staining using specific peroxidase- or fluorescence-labeled antibodies. TOC structures involved in the pathogen-host-interaction may be visualized by antibodies, lectins, or specific staining procedures with for example Alcian blue for mucus (Winter et al., 2008; Zhong et al., 2024). By using confocal microscopy, pathogen and host structures can be detected simultaneously. Besides the detection of the pathogen by electron microscopy or molecular methods, also the expression of immune related genes including Toll-like receptors, interaction of pathogens with respective ligands, and immune responses can be investigated by reverse transcription quantitative real-time PCR (RT-qPCR) or microarray systems (Reemers et al., 2009; Barjesteh et al., 2016). Enzyme-linked immunosorbent assays (ELISA) or bioactivity assays for the detection of soluble factors released by the TOC into the supernatant are rarely used, as most systems are less sensitive than molecular methods, and the individual TOC is very small that only low levels of molecules of interest may be found in the medium.
3.3 Limitations and future perspectives
The most significant limitation in investigations of host-pathogen-interaction in TOC is the lack of the specific immune response. Although residual numbers of T and B cells may be found at the beginning of the culture of TOC, these cells have a short survival time without the addition of specific growth factors (Kothlow et al., 2008; Linti et al., 2024). However, TOC provide an excellent model for host-pathogen investigations of not only birds in the order galliformes such as turkeys, chicken or quail but have also been applied for columbiformes and anseriformes of different species (Colwell et al., 1973; Kocan et al., 1978; Gerganov and Surtmadzhiev, 1982b; Abdul-Wahab et al., 1996; Petersen et al., 2012). We expect that TOC may also be used for other bird species in the future. TOC can be used for the investigation of innate immune responses, which has been shown after mono- and co-infection with various pathogens (Chhabra et al., 2016; Sid et al., 2016; Ruger et al., 2021), and for the comparison of strains of pathogens (Raj and Jones, 1996). The use of TOC allows a statistically meaningful number of replicates and an easy comparison of genotypes and bird species with respect to questions related to pathogenesis under well controlled and unified conditions (Petersen et al., 2012; Hartmann et al., 2015), which would be difficult under in vivo conditions. Though, variation between experiments using different birds or embryonated eggs from different parents cannot be fully excluded due to genetic variations of the donor animals and variable microbiota colonizing the respiratory tract of the donor. An additional limitation is the cellular complexity of the TOC, which may not allow pinning down the cell type associated with for example expressed cytokines/chemokines in tissue homogenates. Therefore, single cell RNA sequencing technologies are expected to be used increasingly for the analysis of complex tissues including TOC.
4 Primary respiratory epithelial cells cultured under air-liquid interface conditions
It is commonly accepted that primary cells derived from the host species are in general more reliable than immortalized cell lines, which differ genetically and phenotypically from the original host cell (Alge et al., 2006; Pan et al., 2009). However, the 2D submerged culture of primary respiratory epithelial cells does not reflect the sophisticated architecture and the expression profile of the native respiratory epithelium (Kaartinen et al., 1993; Pezzulo et al., 2011). Moreover, these cultures lack the most important defense mechanism of the respiratory tract, the mucociliary clearance mechanism (Ostrowski and Nettesheim, 1995; Gray et al., 1996). In contrast, primary respiratory epithelial cells cultured under ALI conditions develop a well-differentiated pseudostratified epithelium, characterized by the formation of an epithelial barrier and by the presence of ciliated, mucus producing (goblet cells), and basal cells, thereby mimicking the in vivo airway epithelium very closely (Whitcutt et al., 1988). Despite some differences regarding the cell population and the immune competence of ALI cultures compared to the in vivo respiratory epithelium, the general gene expression and (patho-) physiological reactions are similar (Ross et al., 2007; Dvorak et al., 2011; Pezzulo et al., 2011; Mathis et al., 2013).
ALI cultures with respiratory epithelial cells have been used frequently for various applications in different fields of respiratory research, e.g., toxicology, pharmacokinetics, pathology, virology, and bacteriology. In contrast to human ALI cultures, respiratory epithelial cells from livestock are usually readily available at low costs from slaughterhouses. Nevertheless, the reproducibility of primary cells is low due to a high interindividual variability (Abraham et al., 2011; Stewart et al., 2012). Moreover, the development of a well-differentiated respiratory epithelium in vitro is a complex process, comprising the initial attachment, proliferation, and polarization of the cells, thickening of the cell layer and mucociliary differentiation, as well as dedifferentiation, cell death and remodeling of the cell layer (Gray et al., 1996), which makes the establishment and maintenance of ALI cultures quite challenging.
For a comprehensive characterization of caprine, ovine, and bovine ALI cultures the reader is referred to recent respective publications (O’Boyle et al., 2017; Cozens et al., 2018a, Cozens et al., 2018b; O’Boyle et al., 2018; Strassle et al., 2021). This review aims to give an overview of the preparation and maintenance as well as possible applications of ALI cultures from livestock.
4.1 Preparation and maintenance
The general procedure of ALI culture preparation and maintenance is similar for all host species. However, there are some species-specific differences in the medium supplementation or culture conditions, which will be highlighted in the following sections. A detailed protocol for the preparation of ALI cultures with primary porcine respiratory epithelial cells can be found in Supplementary Material 2.
The lungs from livestock can be easily and cost-effectively obtained from abattoirs. After removing the connective tissue (Figure 3), trachea/bronchi are transferred to a “digestion buffer” - medium (e.g., DMEM) supplemented with protease, DNase, antibiotics, and antimycotics - and incubated for up to 72 hours (h) at 4°C (Figure 3). The supplements and the incubation time differ between the species and/or the research groups (Supplementary Table 1). Another possibility to dissociate the cells is the incubation in 0.25% trypsin-0.6 mM EDTA at 37°C for 2 h (Abraham et al., 2011). Afterwards, the cells are harvested either by centrifugation (Radi and Ackermann, 2004), by rinsing the tissue pieces (Oslund et al., 2010), by agitation (Mao et al., 2009), or by scraping the cells from the tissue with a scalpel blade (Figure 3) (Schwab et al., 2010). To remove cell detritus and mucosal residues, it is recommended to wash the cell suspension and to filter it through a cell strainer (40-70 µm) (Schwab et al., 2010). Fibroblast contamination can be reduced by incubation of the cell suspension in non-coated plastic cell culture dishes at 37°C for 1–2 h, as fibroblasts rapidly adhere to plastics (Mao et al., 2009). The non-adherent epithelial cells can then be transferred to “submerged growth medium” (SGM; Supplementary Table 2) to propagate them in collagen-coated tissue culture flasks (Figure 3) or to transfer them directly onto collagen-coated permeable membrane inserts (Supplementary Table 3) in a transwell system consisting of two chambers (apical and basal; Figure 3). The epithelial cells are kept under submerged conditions until confluence (approximately 2–7 days; Figure 3) and are then introduced to ALI conditions. For this, the medium is removed from the apical chamber (“air”) and “ALI medium” (Supplementary Table 4) is added to the basal chamber (“liquid”; Figure 3). At the interface of air and liquid, the respiratory epithelial cells can build a well-differentiated pseudostratified epithelium, consisting of ciliated, mucus producing, and basal cells, within an average of three weeks (Figure 3). During differentiation, the medium in the basal chamber is changed every 2–3 days and the cells should be washed at least once per week with neutral buffer solution (e.g., PBS, HBSS) to maintain the homeostatic balance of mucus and cells. Usually, the fully differentiated epithelium remains stable for additional three weeks, allowing long-term experiments (O’Boyle et al., 2017; Cozens et al., 2018b). Notably, Strässle et al. described caprine ALI cultures that were stable for up to two months – afterwards, the ciliary activity decreased and cells died (Strassle et al., 2021).
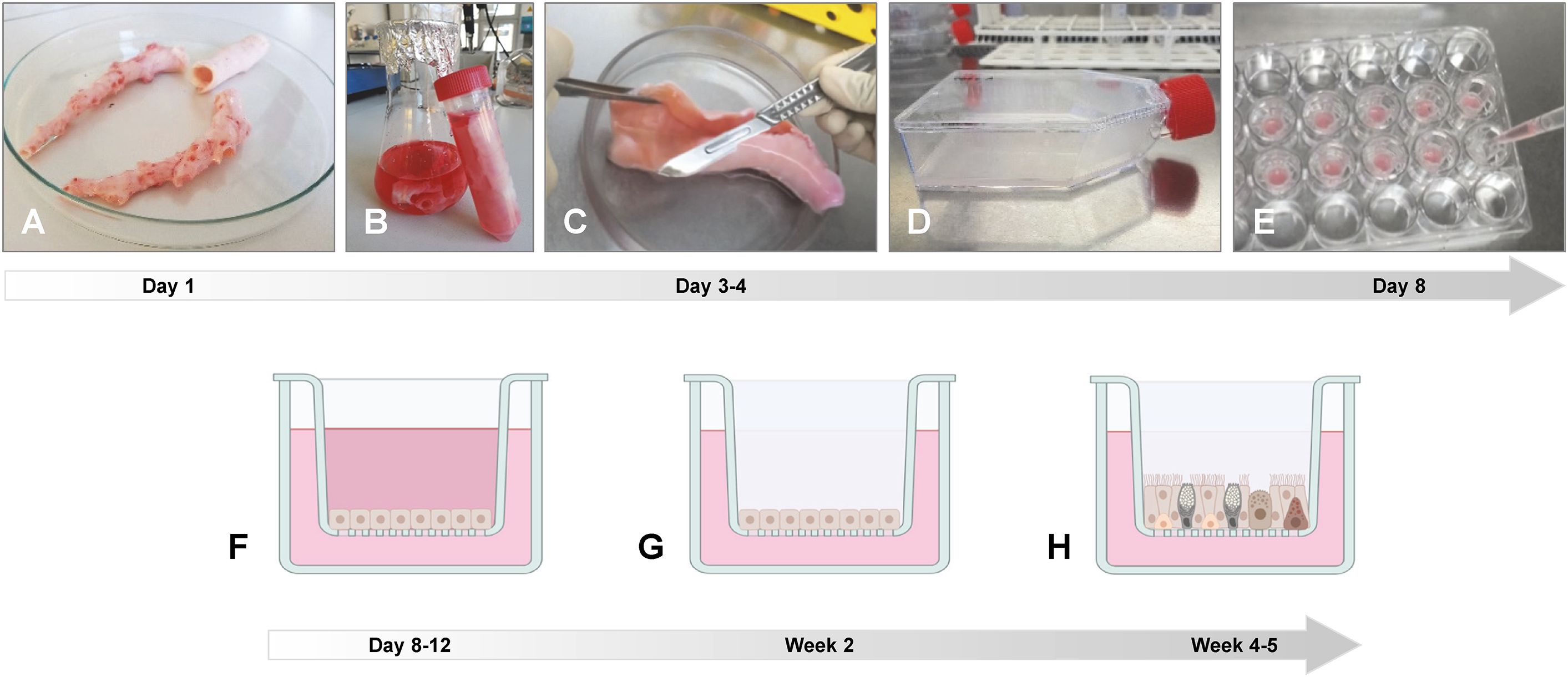
Figure 3. Preparation of ALI cultures from porcine trachea/bronchi. (A) Lungs from slaughtered animals from the abattoir are transported on ice to the laboratory and the trachea and/or the main bronchi are separated from the connective lung tissue. (B) The trachea and/or the main bronchi are incubated in digestion buffer at 4°C for up to three days. (C) Epithelial cells are scraped off from the luminal surface using a scalpel blade and transferred to DMEM supplemented with 10% FBS. After filtration and washing, cells are incubated in non-coated plastic cell culture dishes at 37°C for 1–2 h to reduce fibroblast contamination. (D) Non-adherent epithelial cells are then transferred to a collagen I-coated cell culture flask and incubated at 37°C until cells are confluent. (E) Epithelial cells are detached from the cell culture flask and seeded on collagen IV-coated polycarbonate membranes with a pore size of 0.4 µm and (F) incubated under submerged conditions for four days. (G) Medium from the apical compartment is removed and (H) the epithelial cells differentiate under ALI conditions within 3–4 weeks. Photos provided by (D) Schaaf, Institute for Microbiology, University of Veterinary Medicine Hannover, Germany. Created by D. Schaaf in BioRender. https://BioRender.com/b62a774.
The composition of the SGM is essential for the growth of respiratory epithelial cells (Mao et al., 2009) and can be decisive for the success of cell differentiation under ALI conditions (O’Boyle et al., 2018). The presence of serum in the SGM promotes the proliferation of respiratory epithelial cells (Mao et al., 2009) and seems to be important for the subsequent differentiation under ALI conditions (Abraham et al., 2011). However, serum is also reported to induce squamous differentiation of respiratory epithelial cells (Lechner et al., 1984), indicating that the concentration of serum or serum substituent (e.g., Ultroser G) in the “ALI medium” has to be adjusted very carefully. According to our literature research, the optimum serum concentration in SGM is 5-10% fetal bovine serum (FBS) or 0.5 mg/ml bovine serum albumin (BSA; Supplementary Table 2) and in “ALI medium” 0.5 mg/ml BSA or 2% Ultroser G (Supplementary Table 4).
Proliferation and differentiation of respiratory epithelial cells is a complex process, which requires the precise supplementation of growth factors and hormones in the “ALI medium”, and even though some studies used commercially available media successfully (Wang et al., 2020; Genna et al., 2023; Muller et al., 2023), they sometimes fail to support differentiation (Luengen et al., 2020). Epidermal growth factor, retinoic acid, and triiodo-L-thyronine are all components that should be carefully adjusted in the “ALI medium” to ensure that they optimally support proliferation and differentiation without causing any adverse effects (Gray et al., 1996; Yoon et al., 1997; Cozens et al., 2018a; O’Boyle et al., 2018).
In addition to growth factors and hormones, the physical properties of the membrane insert, such as material (e.g., PC, PET, PTFE), pore size, pore density, and coating of the membrane might affect the differentiation of respiratory epithelial cells (Davenport and Nettesheim, 1996; Widdicombe et al., 2003; Lee et al., 2011; Cozens et al., 2018a; O’Boyle et al., 2018). A high pore-density, allowing the sufficient availability of nutrients across the membrane, was required for optimal differentiation of bovine bronchial and ovine tracheal epithelial cells (Cozens et al., 2018a; O’Boyle et al., 2018). However, the pore-density of the membrane insert is often not indicated by the manufacturer.
Oxygen tension is supposed to influence proliferation and differentiation of respiratory epithelial cells, as it is reported for neural and mesenchymal stem cells (Chen et al., 2007; Panchision, 2009; Liu et al., 2015). Cozens et al. and O’Boyle et al. tested different oxygen concentrations (7%, 14%, and 21%) and found that optimal ciliation and proliferation of bovine bronchial and ovine tracheal epithelial cells occurred at 14% oxygen (Cozens et al., 2018a; O’Boyle et al., 2018).
Even though passaged primary respiratory epithelial cells retain their ability to differentiate into ciliated and mucus-producing cells, they differ from freshly isolated cells, as they completely dedifferentiate under submerged conditions resulting in a homogenous cell population. Passaged cells have been shown to establish cell-cell-connections more efficiently (Abraham et al., 2011) but are delayed in the development of ciliated cells compared to freshly isolated cells (Karp et al., 2002; Lee et al., 2005; Wiszniewski et al., 2006).
Finally, a common challenge with primary cell culture systems is the high variability between the donor individuals (Abraham et al., 2011; Stewart et al., 2012; Russell et al., 2018; Luengen et al., 2020), which can be only addressed by an appropriate number of biological and technical replicates. However, this variability reflects the differences between individuals among the same species occurring also in vivo, indicating that this culture system is mimicking the in vivo situation reliably.
4.2 Applications
This section describes a selection of possible read-out methods and applications for ALI cultures with respiratory epithelial cells from livestock. In contrast to human ALI cultures, literature about livestock ALI cultures is limited. However, methods used for human ALI cultures may also be transferred to ALI cultures from livestock.
Measurement of the trans-epithelial electrical resistance (TEER) is the most common method to evaluate the epithelial barrier integrity of the respiratory epithelial cell layer. Regardless of the species origin, TEER usually peaks during the first days under ALI conditions, then decreases and stays stable for the remainder of the culturing (Lam et al., 2011; Bateman et al., 2013; Meng et al., 2016; O’Boyle et al., 2017; Cozens et al., 2018b; Wang et al., 2018). Barrier functionality can also be assessed by the measurement of a dextran flux across the cell layer (Kreft et al., 2015; Ramezanpour et al., 2016; Su et al., 2020) or immunofluorescence staining of tight or adherens junctions (Figure 4; Supplementary Table 5). Furthermore, immunofluorescence staining or immunohistochemistry allow the visualization of ciliated cells, mucus producing cells, and basal cells (Supplementary Table 5). Hematoxylin-eosin staining of cross sections indicates the cellular composition, thickness of the barrier, and the extent of ciliation (Figure 4) (Abraham et al., 2011; Bateman et al., 2013; O’Boyle et al., 2017; Cozens et al., 2018b; Wang et al., 2018; Strassle et al., 2021), whereas the periodic acid-Schiff (PAS) reaction colors the mucus (Oslund et al., 2010; Schwab et al., 2010; Abraham et al., 2011; O’Boyle et al., 2017). When using transparent membrane inserts, cell layers as well as cilia can be monitored by conventional light microscopy (Schwab et al., 2010; Abraham et al., 2011; Wang et al., 2018; Strassle et al., 2021). Transmission electron microscopy (TEM) (Mao et al., 2009; Khoufache et al., 2010; Oslund et al., 2010; Schwab et al., 2010; Ma et al., 2016; O’Boyle et al., 2017; Cozens et al., 2018b; Wang et al., 2020) as well as scanning electron microscopy (SEM; Figure 4) (Mao et al., 2009; Abraham et al., 2011; Lam et al., 2011; Xue et al., 2015; Meng et al., 2016; O’Boyle et al., 2017; Cozens et al., 2018b; Wang et al., 2018; Strassle et al., 2021; Genna et al., 2023) allows the ultrastructural visualization of ciliated and goblet cells. Additionally, the presence of mucus producing cells may also be estimated by the expression of mucins by RT-qPCR (Oslund et al., 2010; Schwab et al., 2010).
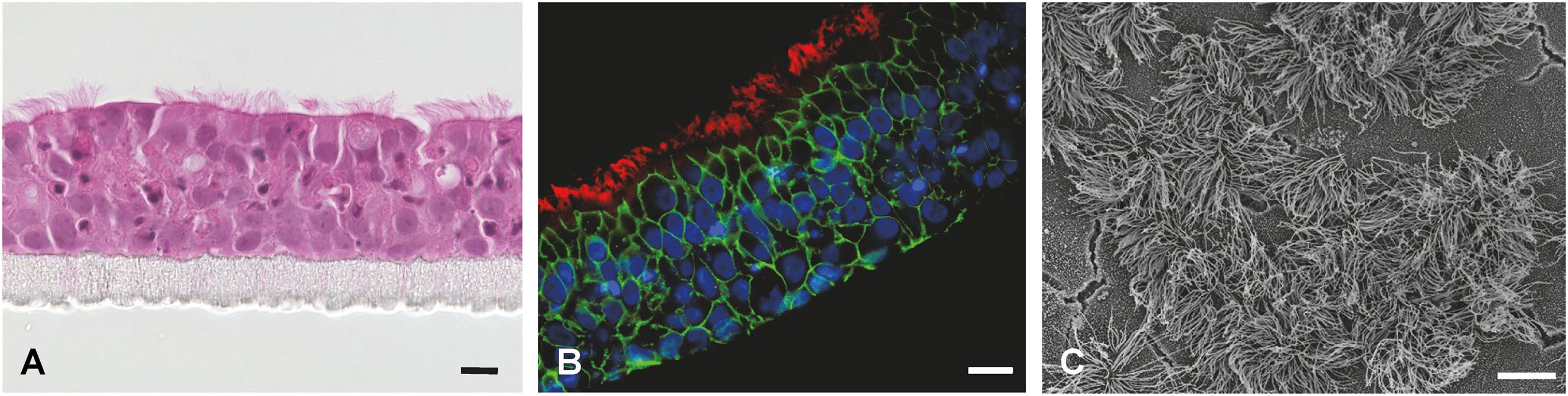
Figure 4. Fully differentiated primary porcine bronchial epithelial cells (PBEC) under ALI conditions. (A) Hematoxylin-eosin staining of PBEC (D. Schaaf, Institute for Microbiology, University of Veterinary Medicine Hannover, Germany). (B) Immunofluorescence staining of PBEC under ALI conditions. Visualization of cilia (β-tubulin, red), tight junctions (β-catenin, green), and nuclei (DAPI, blue) (D. Schaaf, Institute for Microbiology, University of Veterinary Medicine Hannover, Germany). (C) SEM of PBEC (M. Rohde, Helmholtz Center for Infection Research, Braunschweig, Germany). Bars represent 10 µm.
The lactate dehydrogenase (LDH) release assay is a commercial assay commonly used to determine the damage induced by a pathogen or a substance and can be easily applied to ALI cultures (Li et al., 2016; Meng et al., 2016; Su et al., 2020; Qin et al., 2023). In order to examine the immunomodulatory or inflammatory response of the host cells, the activation of inflammatory pathways as well as the induction of cytokines/chemokines can be analyzed by RT-qPCR (Khoufache et al., 2010; Quintana et al., 2011; Xue et al., 2015) or Western blotting (Ma et al., 2016). In addition, cytokine and/or chemokine levels in the supernatant can be determined using ELISA (Jiang et al., 2017; Wang et al., 2018).
For bacterial infection studies, it is feasible to determine the bacterial replication rate, e.g., by plating of supernatant and cell lysates (Meng et al., 2016), by qPCR (Wang et al., 2020), or by color-changing units (Strassle et al., 2021), and to visualize attachment of the pathogen to respiratory epithelial cells via immunofluorescence staining (Meng et al., 2019).
ALI cultures with primary respiratory epithelial cells from livestock can be applied to study the biology of the airway epithelium and respiratory tract diseases (Oslund et al., 2010; Quintana et al., 2011; Abugisisa et al., 2023), to investigate interactions between host cells and viral (Goris et al., 2009; Bateman et al., 2013; Kirchhoff et al., 2014; Wu et al., 2016; Van Cleemput et al., 2017; Yang et al., 2019; Peng et al., 2020; Shin et al., 2020; Gultom et al., 2021; Peng et al., 2021; Muller et al., 2023), bacterial (Xue et al., 2015; Li et al., 2016; Ma et al., 2016; Meng et al., 2016; Jiang et al., 2017; Su et al., 2020; Wang et al., 2020; Strassle et al., 2021; Qin et al., 2023), or fungal (Khoufache et al., 2010) pathogens, to study co-infections of the respiratory tract (Meng et al., 2019), or they might be used for drug transport studies (Lin et al., 2007; Min et al., 2016) or toxicological risk assessment (Friesen et al., 2022). Moreover, ALI cultures from livestock, especially from swine, can provide a suitable model for the human respiratory tract (Lam et al., 2011; Genna et al., 2023).
Finally, ALI cultures with primary respiratory epithelial cells from livestock offer numerous possibilities of applications and read-out methods, a lot more than mentioned above, and are therefore promising in vitro models to study the biology of the respiratory epithelium and respiratory diseases.
4.3 Limitations and future perspectives
Most ALI cultures consist only of epithelial cells, which does not reflect the in vivo respiratory tract appropriately, as it also comprises immune cells, fibroblasts, chondrocytes, endothelial cells, etc. To address this aspect, co-culture systems (e.g., respiratory epithelial cells and fibroblasts) have been developed (Albers et al., 2015; Abs et al., 2019). These studies showed that co-cultivation with fibroblasts improves the differentiation of porcine tracheal and equine bronchial epithelial cells. Co-culture systems including fibroblasts and/or immune cells are also reported for human ALI cultures (Harrington et al., 2014; Kletting et al., 2018; De Rudder et al., 2020; Friesen et al., 2022). Leach et al. developed a 3D airway ‘organ tissue equivalent’ by including pulmonary fibroblasts, solubilized lung extracellular matrix, and hydrogel substrate with tunable stiffness and porosity (Leach et al., 2023). Another factor, which can affect the epithelial development and the immune response but is only rarely addressed in in vitro systems, is the respiratory microbiota (De Rudder et al., 2020). Furthermore, most ALI cultures from livestock consist of epithelial cells from the conducting airways (mainly trachea and bronchi), whereas we found only one article about bovine alveolar epithelial cells, co-cultured with bovine pulmonary arterial endothelial cells (Lee and Chambers, 2019). Since the epithelium of gas-exchanging airways differs from that of the conducting airways, it might be interesting to include also ALI cultures consisting of alveolar epithelial cells in future studies.
Remarkably, to our knowledge no reports about ALI cultures with respiratory epithelial cells from poultry exist. Although it was shown that primary avian tracheal epithelial cells develop to ciliated cells, goblet cells, and basal cells under submerged conditions (Shen et al., 2010), they fail to build a pseudostratified epithelium comparable to the native avian respiratory tract. Regarding the occurrence of respiratory pathogens (e.g., avian influenza virus, Mycoplasma gallisepticum) leading to increased mortality in poultry, it might be worthwhile to establish the ALI culture system for avian respiratory epithelial cells to investigate host-pathogen interactions in vitro.
Overall, ALI cultures with respiratory epithelial cells from livestock represent a major area of development and a promising cell culture system for future studies in the field of respiratory research.
5 Precision-cut lung slices
PCLS are 3D ex vivo organ models, which closely mimic the in vivo situation and have significant impact and value in translational science (Alsafadi et al., 2020). Since first described by Placke and Fisher in 1987 (Placke and Fisher, 1987), PCLS have been established from lungs of different animal species including humans during the last decades. PCLS have advantages over cell culture as they reflect the structural and functional heterogeneity of lung tissue. The microarchitecture of lung tissue including vessels, airways, and nerves, which are all embedded in the lung parenchyma, is intact and enables investigations under physiological conditions (Martin et al., 1996; Schleputz et al., 2011). In addition, the morphological organization of the cellular components and their response to stimuli are very close to the in vivo situation in the lung (Hays et al., 2003; Harrigan et al., 2004; Henjakovic et al., 2008). PCLS are composed of various cell types, which can be classified as structural (such as epithelial, endothelial, lymphatic, smooth muscle, and fibroblastic cells) and non-structural cell types, mainly cells of the immune system (like macrophages, neutrophils, dendritic cells, T cells, and B cells) (Lyons-Cohen et al., 2017; Niehof et al., 2017). Additional advantages of PCLS include the ease of preparation, low costs, good reproducibility, and the stability of cells ex vivo (Ruigrok et al., 2019; Weldearegay et al., 2019; Ram-Mohan et al., 2020). Moreover, PCLS serve as an efficient and rapid screening method to identify suitable experimental animal models, thereby reducing the necessity for large-scale animal usage (Gerhards et al., 2021).
During our literature research, we found that PCLS from livestock have been used in different research areas, e.g., lung physiology and pathophysiology, pharmacology and gene therapy, as well as respiratory infection research. Whereas several studies on viral respiratory pathogens have been published during the last twenty years, only few publications on bacterial respiratory infections of livestock can be found (Weldearegay et al., 2019; Qin et al., 2021; Votsch et al., 2021).
Recently, there have been several comprehensive reviews on PCLS with regard to human lung biology and disease modelling as well as the investigation of therapeutic targets (Liu et al., 2019; Alsafadi et al., 2020; Viana et al., 2022; Lam et al., 2023). Thus, in this review we focus on PCLS as a powerful tool to investigate respiratory bacterial infections of livestock.
5.1 Preparation and maintenance
A detailed protocol for the preparation of porcine PCLS can be found in Supplementary Material 3.
PCLS are prepared following standard procedures, which implement the use of low-melting agarose prepared with buffer or cell culture medium (e.g., RPMI 1640 medium) (Placke and Fisher, 1987). The concentration of agarose varies from 0.4% to 3% - lower concentrations should be used for small and fragile lobes, whereas higher percentages can be used for lungs from humans and bigger animals (Alsafadi et al., 2020). Lungs from livestock can be easily obtained from a local slaughterhouse. The cranial and accessory lobes (big/adult animals) or the whole lung (small/young animals) can be used, respectively. The lung lobes/lungs are filled slowly with warm (37°C) low-melting agarose via a cannula introduced in the (main) bronchus/trachea, until all the air spaces are filled with agarose (Figure 5). These lobes are then kept on ice or in chilled buffer until the agarose inside the tissue solidifies. Once the agarose becomes solid, thin tissue slices (150-500 µm) can be prepared using a tissue slicer or vibratome (Figure 5). Optional, before slicing, cylindrical tissue sections can be punched out of the solidified tissue by using a tissue-coring tool to prepare round tissue slices that fit into well plates. The agarose is removed from the airways by washing, e.g., on a shaker or by bubbling with a normoxic gas mixture (Paddenberg et al., 2014) (Figure 5). Finally, the slices are maintained in cell culture medium (e.g., RPMI 1640 medium), supplemented with antibiotics and antimycotics to eliminate contaminating bacteria and fungi, and can remain viable for up to two weeks (Neuhaus et al., 2017; Temann et al., 2017; Weldearegay et al., 2019) or even ≥ 40 days (chicken) (Bryson et al., 2020). Medium should be changed every day to minimize the effect of endogenously released mediators (Lambermont et al., 2014) as well as to remove any residual agarose. Depending on the pathogen used for the experimental infection, the medium should be changed to a medium without antibiotics/antimycotics, at least one day before infection.
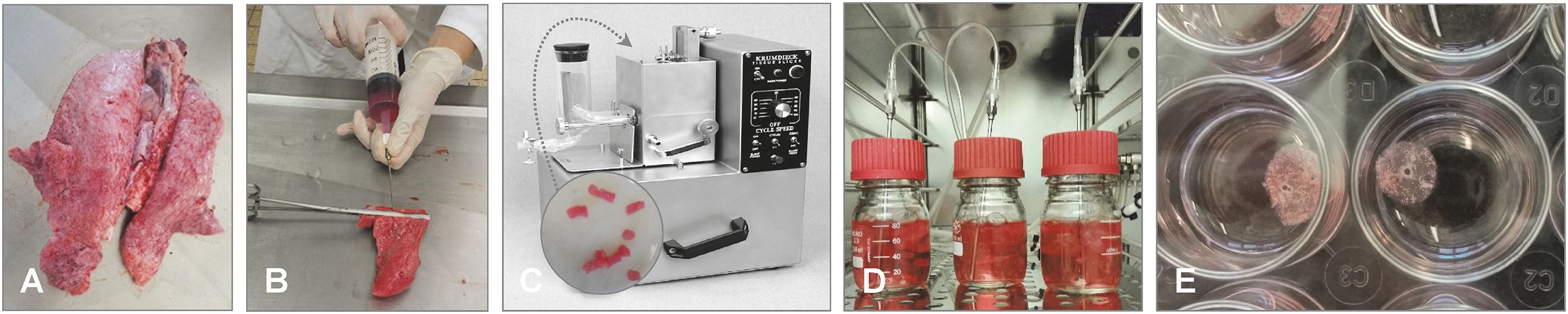
Figure 5. Preparation of porcine PCLS. (A) Lungs from slaughtered animals from the abattoir are transported on ice to the laboratory. (B) The cranial lobes of apparently healthy lungs are dissected and filled with 37°C low melting-point agarose through a cannula introduced in the bronchus. Filled lung lobes are kept on ice until the agarose becomes solid. (C) By using a tissue coring tool, cylindrical pieces of lung tissue with a bronchioles in the middle are punched out and cut into approximately 300 µm thin tissue slices by using a tissue slicer or vibratome (here: Krumdieck tissue slicer, model MD 4000-01; TSE Systems, Chesterfield, MO, USA). (D) The tissue slices are transferred to cell culture medium (e.g., RPMI) supplemented with antibiotics and antimycotics and bubbled with a normoxic gas mixture at 37°C to remove the agarose from the airway lumen. (E) PCLS can be maintained for up to two weeks in cell culture medium (e.g., RPMI) supplemented with antibiotics and antimycotics in, e.g., a 24-well plate. Photos provided by D. Schaaf, Institute for Microbiology, University of Veterinary Medicine Hannover, Germany.
5.2 Applications
Ciliary activity is the major means of controlling tissue vitality using light microscopy (Votsch et al., 2021). However, to quantify the impact of a pathogen or other agents on the ciliary motility, the ciliary beat frequency has to be determined by high-speed video microscopy, as described by Dresdner and Wong in 1985 (Dresdner and Wong, 1985). Viability may also be assessed using metabolic tests (e.g., WST-1 or MTS assay) (Neuhaus et al., 2017; Weldearegay et al., 2019) and cytotoxicity assays (e.g., LDH-release assay) (Remot et al., 2021; Votsch et al., 2021; Gaudino et al., 2023; Qin et al., 2023), as well as live-dead staining (Neuhaus et al., 2017; Gaudino et al., 2023) and flow cytometry (Gaudino et al., 2023).
To quantify bronchoconstriction following infection or drug administration, the bronchial cavity areas can be measured by light microscopy (Qin et al., 2021) or tissue traction microscopy can be applied to measure contractile forces of airway smooth muscles (Ram-Mohan et al., 2020).
Histopathological examinations give important information on the overall tissue structure and alterations due to infection or treatment with other agents (Weldearegay et al., 2019; Qin et al., 2021; Votsch et al., 2021) (Figure 6). In contrast, immunohistochemistry or immunofluorescence staining allows the identification and visualization of specific tissue structures (e.g., cilia, adherence junctions) or pathogens, thereby enabling the localization of the pathogen on the tissue slice as well as the documentation of host cell-pathogen interactions (Meng et al., 2015; Weldearegay et al., 2019; Remot et al., 2021; Votsch et al., 2021; Qin et al., 2023) (Figure 6). Ultrastructural visualization of tissue structures and pathogens is possible with SEM (Votsch et al., 2021) (Figure 6) or TEM (Weldearegay et al., 2019; Gaudino et al., 2023).
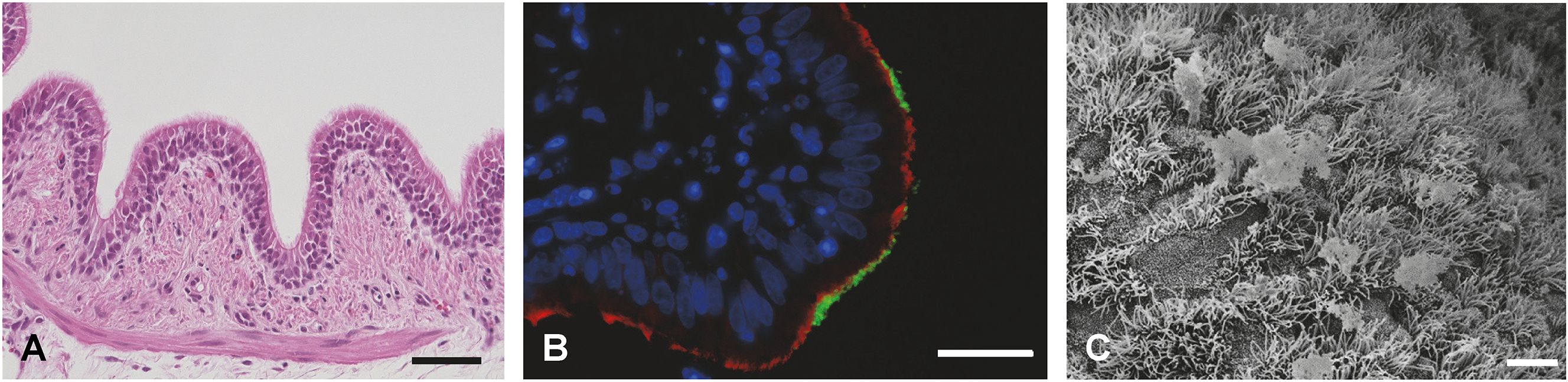
Figure 6. Visualization of PCLS. (A) Hematoxylin-eosin staining of porcine PCLS; bar represents 50 µm (R. Spriewald, Institute for Microbiology, University of Veterinary Medicine Hannover, Germany). (B) Immunofluorescence staining of porcine PCLS infected with Bordetella bronchiseptica. Bacteria are shown in green, cilia (β-tubulin) in red, and nuclei (DAPI) in blue; bar represents 20 µm (D. Schaaf, Institute for Microbiology, University of Veterinary Medicine Hannover, Germany). (C) SEM of porcine PCLS; bar represents 5 µm (M. Rohde, Helmholtz Center for Infection Research, Braunschweig, Germany).
Even though emerging analysis methods, such as mass spectrometry and next generation sequencing, have so far mainly focused on human PCLS, this demonstrates their applicability across species for RNA-sequencing (Huang et al., 2019; Stegmayr et al., 2021; Crue et al., 2023) as well as for proteomic and metabolomics analyses (Mossina et al., 2017; Khan et al., 2021). These methods would significantly advance the study of host-pathogen interactions in respiratory tract infections of livestock.
Transcriptomic studies using microarray analysis and PCLS from different species revealed a number of interesting results, which could be utilized in identifying multifactorial mode of drug action in the treatment of infectious diseases (Reamon-Buettner et al., 2019) as well as a better understanding of innate immune responses towards infectious agents (Weldearegay et al., 2023; Li et al., 2024). Furthermore, the host’s immune response upon infection can be evaluated by cytokine expression profiles and the identification of specific immune pathways using RT-qPCR (Remot et al., 2021; Gaudino et al., 2023; Weldearegay et al., 2023) or by detection of cytokines in the supernatant using ELISA, Luminex xMAP® Technology, or Western blotting (Dresen et al., 2021; Remot et al., 2021; Gaudino et al., 2023; Qin et al., 2023; Weldearegay et al., 2023).
The number of colonizing bacteria can either be determined by plating of supernatants and tissue lysates on agar plates (Meng et al., 2015; Remot et al., 2021; Votsch et al., 2021; Qin et al., 2023), by (duplex) qPCR (Weldearegay et al., 2019; Hanske et al., 2023), or by RT-qPCR (Gaudino et al., 2023). Moreover, the presence of virulence (-associated) factors during infection of PCLS can be analyzed by Western blotting (Dresen et al., 2021; Votsch et al., 2021) or RT-qPCR (Weldearegay et al., 2023).
The methods described here represent only a small selection of what is possible with this versatile ex vivo model, as methods described for human PCLS can likewise be applied to PCLS from animals.
5.3 Limitations and future perspectives
Although PCLS provide important information on host-pathogen interactions, one limitation of PCLS is that the slices, including the airways, are submerged in a liquid medium, which does not represent the in vivo situation, where the airways and alveoli are largely filled with air. In pharmacological studies, the route of drug administration remains to be a challenge since slices are submerged in medium containing the drug/compound of interest. This complicates assessment of the effect of administration routes, e.g. systemic delivery or local application in vivo (Liu et al., 2019). Similarly, regarding respiratory infections, the natural (i.e., nasal) route of infection cannot be mimicked with this model.
Another important limitation of the PCLS model is the lack of immune cell recruitment and adaptive immunity. Even though resident immune cells responsible for host defense mechanisms are present, their activation and release of cytokines may not initiate subsequent differentiation and recruitment of other immune cells towards the site of infection, which limits the extent of immune response using PCLS (Liu et al., 2019; Viana et al., 2022). However, the early innate immune response can be studied in PCLS and alterations in the inflammatory response upon infection with (bacterial) pathogens have been reported in different studies (Remot et al., 2021; Gaudino et al., 2023; Qin et al., 2023; Weldearegay et al., 2023).
The viability of cells in PCLS being on average restricted to two weeks (Neuhaus et al., 2017; Temann et al., 2017; Weldearegay et al., 2019) limits the application of PCLS for chronic infection and toxicity studies (Viana et al., 2022). In order to address this, Bailey and colleagues used poly(ethylene glycol)-based hydrogel platforms to embed the PCLS and successfully prolonged the life span of viable PCLS (Bailey et al., 2020).
For more efficient utilization of PCLS, some research groups tested different methods of preservation such as cold or hypothermic storage and cryopreservation and found that structural and functional stability including immune functions were to a great extent maintained (Rosner et al., 2014; Bai et al., 2016; Watson et al., 2016; Tigges et al., 2021; Patel et al., 2023; Melo-Narvaez et al., 2025).
Despite these limitations, PCLS provide a useful tool to study host cell-pathogen interactions as well as the early pulmonary immune response during mono- and co-infection of the respiratory tract in livestock.
6 Conclusion and outlook
In the present review, we summarized preparation, maintenance, and applications of four different primary cell culture systems of the upper and lower respiratory tract in the context of respiratory infections in livestock. While especially PCLS and ALI cultures are widely used in human health research, the use of the four models described here in veterinary research is still scarce.
The main advantages of these models are listed in Table 1. The nearly unlimited access to the starting material (organs and tissues from slaughtered animals), simplifies the examination of adequate numbers of biological and technical replicates. In addition, early steps in host-pathogen interaction can be studied with high temporal resolution, which is difficult to achieve in in vivo experimental infections due to the high demand of laboratory animals in this type of experiments. The tissue based systems are derived from the upper (NME, TOC) and lower (PCLS) respiratory tract, which allows the comparison of region-specific epithelial cell type reactions.
Existing limitations (Table 1), like the limited life span, the lack of immune cell recruitment and adaptive immunity, and limited genetic manipulation methods are subjects of ongoing and future improvements, e.g., by transfection of PCLS or epithelial cells growing under ALI conditions.
In summary, the four primary cell culture systems offer the potential for relatively high throughput examination of specific aspects of host-pathogen interaction in respiratory mono- and co-infections. They apply to the 3R principle (replacement), thereby reducing ethical implications compared to in vivo studies and can bridge the gap between classical 2D in vitro models and experimental animal models.
Future applications are not restricted to classical respiratory infections in livestock, but also zoonotic pathogens like Streptococcus suis or even the characterisation of organs from genetically multi-modified pigs in the field of xenotransplantation might be interesting.
Author contributions
DS: Funding acquisition, Visualization, Writing – original draft, Writing – review & editing. YW: Conceptualization, Writing – original draft, Writing – review & editing. LB: Visualization, Writing – original draft, Writing – review & editing. SR: Visualization, Writing – original draft, Writing – review & editing. JM: Conceptualization, Writing – review & editing. PV-W: Conceptualization, Writing – review & editing.
Funding
The author(s) declare that financial support was received for the research and/or publication of this article. This work was financially supported by the Deutsche Forschungsgemeinschaft (DFG; SCHA 2406/1-1). Furthermore, we acknowledge financial support by the Open Access Publication Fund of the University of Veterinary Medicine Hannover, Foundation.
Conflict of interest
The authors declare that the research was conducted in the absence of any commercial or financial relationships that could be construed as a potential conflict of interest.
Generative AI statement
The author(s) declare that no Generative AI was used in the creation of this manuscript.
Publisher’s note
All claims expressed in this article are solely those of the authors and do not necessarily represent those of their affiliated organizations, or those of the publisher, the editors and the reviewers. Any product that may be evaluated in this article, or claim that may be made by its manufacturer, is not guaranteed or endorsed by the publisher.
Supplementary material
The Supplementary Material for this article can be found online at: https://www.frontiersin.org/articles/10.3389/fcimb.2025.1565513/full#supplementary-material
Abbreviations
2D, two-dimensional; 3D, three-dimensional; ALI, air-liquid interface; ELISA, enzyme-linked immunosorbent assay; LDH, lactate dehydrogenase; NME, nasal mucosa explants; PBEC, porcine bronchial epithelial cells; PCLS, precision-cut lung slices; qPCR, quantitative PCR; RT-qPCR, reverse transcription quantitative real-time PCR; SEM, scanning electron microscopy; SGM, submerged growth medium; TEER, trans-epithelial electrical resistance; TEM, transmission electron microscopy; TOC, tracheal organ cultures.
References
Abd El Rahman, S., Winter, C., El-Kenawy, A., Neumann, U., Herrler, G. (2010). Differential sensitivity of well-differentiated avian respiratory epithelial cells to infection by different strains of infectious bronchitis virus. J. Virol 84, 8949–8952. doi: 10.1128/JVI.00463-10
Abdul-Wahab, O. M., Ross, G., Bradbury, J. M. (1996). Pathogenicity and cytadherence of Mycoplasma imitans in chicken and duck embryo tracheal organ cultures. Infect. Immun. 64, 563–568. doi: 10.1128/iai.64.2.563-568.1996
Abraham, G., Zizzadoro, C., Kacza, J., Ellenberger, C., Abs, V., Franke, J., et al. (2011). Growth and differentiation of primary and passaged equine bronchial epithelial cells under conventional and air-liquid-interface culture conditions. BMC Vet Res. 7, 26. doi: 10.1186/1746-6148-7-26
Abs, V., Bonicelli, J., Kacza, J., Zizzadoro, C., Abraham, G. (2019). Equine bronchial fibroblasts enhance proliferation and differentiation of primary equine bronchial epithelial cells co-cultured under air-liquid interface. PLoS One 14, e0225025. doi: 10.1371/journal.pone.0225025
Abugisisa, L., Royse, E. X., Kemp, M. W., Jobe, A. H., Hillman, N. H. (2023). Preterm ovine respiratory epithelial cell responses to mechanical ventilation, lipopolysaccharide, and interleukin-13. Am. J. Physiol. Lung Cell Mol. Physiol. 324, L815–L824. doi: 10.1152/ajplung.00355.2022
Albers, S., Thiebes, A. L., Gessenich, K. L., Jockenhoevel, S., Cornelissen, C. G. (2015). Differentiation of respiratory epithelium in a 3-dimensional co-culture with fibroblasts embedded in fibrin gel. Multidiscip Respir. Med. 11, 6. doi: 10.1186/s40248-016-0046-3
Alge, C. S., Hauck, S. M., Priglinger, S. G., Kampik, A., Ueffing, M. (2006). Differential protein profiling of primary versus immortalized human RPE cells identifies expression patterns associated with cytoskeletal remodeling and cell survival. J. Proteome Res. 5, 862–878. doi: 10.1021/pr050420t
Alsafadi, H. N., Uhl, F. E., Pineda, R. H., Bailey, K. E., Rojas, M., Wagner, D. E., et al. (2020). Applications and approaches for three-dimensional precision-cut lung slices. Disease modeling and drug discovery. Am. J. Respir. Cell Mol. Biol. 62, 681–691. doi: 10.1165/rcmb.2019-0276TR
Baghi, H. B., Nauwynck, H. J. (2014). Impact of equine herpesvirus type 1 (EHV-1) infection on the migration of monocytic cells through equine nasal mucosa. Comp. Immunology Microbiol. Infect. Dis. 37, 321–329. doi: 10.1016/j.cimid.2014.09.004
Bai, Y., Krishnamoorthy, N., Patel, K. R., Rosas, I., Sanderson, M. J., Ai, X. (2016). Cryopreserved human precision-cut lung slices as a bioassay for live tissue banking. A viability study of bronchodilation with bitter-taste receptor agonists. Am. J. Respir. Cell Mol. Biol. 54, 656–663. doi: 10.1165/rcmb.2015-0290MA
Bailey, K. E., Pino, C., Lennon, M. L., Lyons, A., Jacot, J. G., Lammers, S. R., et al. (2020). Embedding of precision-cut lung slices in engineered hydrogel biomaterials supports extended ex vivo culture. Am. J. Respir. Cell Mol. Biol. 62, 14–22. doi: 10.1165/rcmb.2019-0232MA
Barjesteh, N., Alkie, T. N., Hodgins, D. C., Nagy, E., Sharif, S. (2016). Local innate responses to TLR ligands in the chicken trachea. Viruses-Basel 8, 207. doi: 10.3390/v8070207
Bateman, A. C., Karasin, A. I., Olsen, C. W. (2013). Differentiated swine airway epithelial cell cultures for the investigation of influenza A virus infection and replication. Influenza Other Respir. Viruses 7, 139–150. doi: 10.1111/j.1750-2659.2012.00371.x
Bryson, K. J., Garrido, D., Esposito, M., McLachlan, G., Digard, P., Schouler, C., et al. (2020). Precision cut lung slices: a novel versatile tool to examine host-pathogen interaction in the chicken lung. Vet Res. 51, 2. doi: 10.1186/s13567-019-0733-0
Cantero, D., Cooksley, C., Bassiouni, A., Tran, H. B., Roscioli, E., Wormald, P. J., et al. (2015). Staphylococcus aureus biofilms induce apoptosis and expression of interferon-γ, interleukin-10, and interleukin-17A on human sinonasal explants. Am. J. Rhinology Allergy 29, 23–28. doi: 10.2500/ajra.2015.29.4130
Cantero, D., Cooksley, C., Bassiouni, A., Wormald, P. J., Vreugde, S. (2013a). Staphylococcus aureus biofilm activates the nucleotide-binding oligomerization domain containing 2 (Nod2) pathway and proinflammatory factors on a human sinonasal explant model. Int. Forum Allergy Rhinology 3, 877–884. doi: 10.1002/alr.21213
Cantero, D., Cooksley, C., Jardeleza, C., Bassiouni, A., Jones, D., Wormald, P. J., et al. (2013b). A human nasal explant model to study Staphylococcus aureus biofilm in vitro. Int. Forum Allergy Rhinology 3, 556–562. doi: 10.1002/alr.21146
Cao, X., Coyle, J. P., Xiong, R., Wang, Y., Heflich, R. H., Ren, B., et al. (2021). Invited review: human air-liquid-interface organotypic airway tissue models derived from primary tracheobronchial epithelial cells-overview and perspectives. In Vitro Cell Dev. Biol. Anim 57, 104–132. doi: 10.1007/s11626-020-00517-7
Cardeilhac, P. T., Nair, K. P., Colwell, W. M. (1972). Tracheal organ cultures for the bioassay of nanogram quantities of mycotoxins. J. Assoc Off Anal. Chem. 55, 1120–1121. doi: 10.1093/jaoac/55.5.1120
Chen, H. L., Pistollato, F., Hoeppner, D. J., Ni, H. T., McKay, R. D., Panchision, D. M. (2007). Oxygen tension regulates survival and fate of mouse central nervous system precursors at multiple levels. Stem Cells 25, 2291–2301. doi: 10.1634/stemcells.2006-0609
Cherry, J. D., Taylor-Robinson, D. (1970). Large-quantity production of chicken embryo tracheal organ cultures and use in virus and mycoplasma studies. Appl. Microbiol 19, 658–662. doi: 10.1128/am.19.4.658-662.1970
Chhabra, R., Kuchipudi, S. V., Chantrey, J., Ganapathy, K. (2016). Pathogenicity and tissue tropism of infectious bronchitis virus is associated with elevated apoptosis and innate immune responses. Virology 488, 232–241. doi: 10.1016/j.virol.2015.11.011
Colwell, W. M., Ashley, R. C., Simmons, D. G., Hamilton, P. B. (1973). The relative in vitro sensitivity to aflatoxin B1 of tracheal organ cultures prepared from day-old chickens, ducks, Japanese quail, and Turkeys. Avian Dis. 17, 166–172. doi: 10.2307/1588934
Colwell, W. M., Lukert, P. D. (1969). Effects of avian infectious bronchitis virus (IBV) on tracheal organ cultures. Avian Dis. 13, 888–894. doi: 10.2307/1588596
Cook, J. K., Darbyshire, J. H., Peters, R. W. (1976). The use of chicken tracheal organ cultures for the isolation and assay of avian infectious bronchitis virus. Arch. Virol 50, 109–118. doi: 10.1007/BF01318005
Cozens, D., Grahame, E., Sutherland, E., Taylor, G., Berry, C. C., Davies, R. L. (2018a). Development and optimization of a differentiated airway epithelial cell model of the bovine respiratory tract. Sci. Rep. 8, 853. doi: 10.1038/s41598-017-19079-y
Cozens, D., Sutherland, E., Marchesi, F., Taylor, G., Berry, C. C., Davies, R. L. (2018b). Temporal differentiation of bovine airway epithelial cells grown at an air-liquid interface. Sci. Rep. 8, 14893. doi: 10.1038/s41598-018-33180-w
Crue, T., Lee, G. Y., Peng, J. Y., Schaunaman, N., Agraval, H., Day, B. J., et al. (2023). Single cell RNA-sequencing of human precision-cut lung slices: A novel approach to study the effect of vaping and viral infection on lung health. Innate Immun. 29, 61–70. doi: 10.1177/17534259231181029
Davenport, E. A., Nettesheim, P. (1996). Regulation of mucociliary differentiation of rat tracheal epithelial cells by type I collagen gel substratum. Am. J. Respir. Cell Mol. Biol. 14, 19–26. doi: 10.1165/ajrcmb.14.1.8534482
DeBey, M. C., Ross, R. F. (1994). Ciliostasis and loss of cilia induced by Mycoplasma hyopneumoniae in porcine tracheal organ cultures. Infect. Immun. 62, 5312–5318. doi: 10.1128/iai.62.12.5312-5318.1994
De Rudder, C., Calatayud Arroyo, M., Lebeer, S., Van de Wiele, T. (2020). Dual and triple epithelial coculture model systems with donor-derived microbiota and THP-1 macrophages to mimic host-microbe interactions in the human sinonasal cavities. mSphere 5, 916–919. doi: 10.1128/mSphere.00916-19
de Wit, J. J., Boelm, G. J., van Gerwe, T. J., Swart, W. A. (2013). The required sample size in vaccination-challenge experiments with infectious bronchitis virus, a meta-analysis. Avian Pathol. 42, 9–16. doi: 10.1080/03079457.2012.751485
Dichtl, S., Posch, W., Wilflingseder, D. (2024). The breathtaking world of human respiratory in vitro models: Investigating lung diseases and infections in 3D models, organoids, and lung-on-chip. Eur. J. Immunol. 54, e2250356. doi: 10.1002/eji.202250356
Ding, P., Liu, H. C., Tong, Y. Y., He, X., Yin, X., Yin, Y. L., et al. (2022). Developmental change of yolk microbiota and its role on early colonization of intestinal microbiota in chicken embryo. Animals 12. doi: 10.3390/ani12010016
Di Teodoro, G., Marruchella, G., Di Provvido, A., Orsini, G., Ronchi, G. F., D’Angelo, A. R., et al. (2018). Respiratory explants as a model to investigate early events of contagious bovine pleuropneumonia infection. Vet Res. 49, 5. doi: 10.1186/s13567-017-0500-z
Dolin, R., Smith, H. A. (1975). Antiviral activity of adenine arabinoside and iododeoxyuridine in human fetal intestinal and tracheal organ cultures. J. Infect. Dis. 132, 287–295. doi: 10.1093/infdis/132.3.287
Dowgier, G., Bickerton, E. (2020). The preparation of chicken tracheal organ cultures and their application for ciliostasis test, growth kinetics studies, and virus propagation. Methods Mol. Biol. 2203, 97–106. doi: 10.1007/978-1-0716-0900-2_8
Dresdner, R. D., Wong, L. B. (1985). Measurement of ciliary beat frequency using high-speed video microscopy. ISA Trans. 24, 33–38.
Dresen, M., Schenk, J., Berhanu Weldearegay, Y., Votsch, D., Baumgartner, W., Valentin-Weigand, P., et al. (2021). Streptococcus suis induces expression of cyclooxygenase-2 in porcine lung tissue. Microorganisms 9, 366. doi: 10.3390/microorganisms9020366
Dudley, J. P., Cherry, J. D. (1977). The effect of mucolytic agents and topical decongestants on the ciliary activity of chicken tracheal organ cultures. Pediatr. Res. 11, 904–906. doi: 10.1203/00006450-197708000-00010
Dvorak, A., Tilley, A. E., Shaykhiev, R., Wang, R., Crystal, R. G. (2011). Do airway epithelium air-liquid cultures represent the in vivo airway epithelium transcriptome? Am. J. Respir. Cell Mol. Biol. 44, 465–473. doi: 10.1165/rcmb.2009-0453OC
Faldynova, M., Prikrylova, H., Sebkova, A., Volf, J., Karasova, D., Crhanova, M., et al. (2024). Contact with adult hens affects the composition of skin and respiratory tract microbiota in newly hatched chicks. Poult Sci. 103, 103302. doi: 10.1016/j.psj.2023.103302
Friesen, A., Fritsch-Decker, S., Hufnagel, M., Mulhopt, S., Stapf, D., Weiss, C., et al. (2022). Gene expression profiling of mono- and co-culture models of the respiratory tract exposed to crystalline quartz under submerged and air-liquid interface conditions. Int. J. Mol. Sci. 23, 7773. doi: 10.3390/ijms23147773
Frydas, I. S., Verbeeck, M., Cao, J., Nauwynck, H. J. (2013). Replication characteristics of porcine reproductive and respiratory syndrome virus (PRRSV) European subtype 1 (Lelystad) and subtype 3 (Lena) strains in nasal mucosa and cells of the monocytic lineage: Indications for the use of new receptors of PRRSV (Lena). Veterinary Res. 44, 73. doi: 10.1186/1297-9716-44-73
Gaudino, M., Lion, A., Sagne, E., Nagamine, B., Oliva, J., Terrier, O., et al. (2023). The Activation of the RIG-I/MDA5 Signaling Pathway upon Influenza D Virus Infection Impairs the Pulmonary Proinflammatory Response Triggered by Mycoplasma bovis Superinfection. J. Virol 97, e0142322. doi: 10.1128/jvi.01423-22
Genna, V. G., Adamo, D., Galaverni, G., Lepore, F., Boraldi, F., Quaglino, D., et al. (2023). Validation of airway porcine epithelial cells as an alternative to human in vitro preclinical studies. Sci. Rep. 13, 16290. doi: 10.1038/s41598-023-43284-7
Gerganov, G., Surtmadzhiev, K. (1982a). Comparative study of tracheal organ cultures obtained from different bird donors. Vet Med. Nauki 19, 11–20.
Gerganov, G., Surtmadzhiev, K. (1982b). Cultivation of various avian viruses in pheasant trachea organ cultures and chick embryos. Vet Med. Nauki 19, 18–24.
Gerhards, N. M., Cornelissen, J., van Keulen, L. J. M., Harders-Westerveen, J., Vloet, R., Smid, B., et al. (2021). Predictive value of precision-cut lung slices for the susceptibility of three animal species for SARS-CoV-2 and validation in a refined hamster model. Pathogens 10, 824. doi: 10.3390/pathogens10070824
Glazenburg, K. L., Peeters, B. P., Pol, J. M., Gielkens, A. L., Moormann, R. J. (1995). Construction and properties of pseudorabies virus recombinants with altered control of immediate-early gene expression. J. Virol. 69, 189–197. doi: 10.1128/jvi.69.1.189-197.1995
Glorieux, S., Vandekerckhove, A. P., Goris, N., Yang, X. Y., Steukers, L., Van de Walle, G. R., et al. (2012). Evaluation of the antiviral activity of (1’S,2’R)-9-[[1’,2’-bis(hydroxymethyl)cycloprop-1’-yl]methyl]guanine (A-5021) against equine herpesvirus type 1 in cell monolayers and equine nasal mucosal explants. Antiviral Res. 93, 234–238. doi: 10.1016/j.antiviral.2011.11.016
Glorieux, S., Van den Broeck, W., van der Meulen, K. M., Van Reeth, K., Favoreel, H. W., Nauwynck, H. J. (2007). In vitro culture of porcine respiratory nasal mucosa explants for studying the interaction of porcine viruses with the respiratory tract. J. Virological Methods 142, 105–112. doi: 10.1016/j.jviromet.2007.01.018
Gordhan, B. G., Herrera, C., Pillay, A. D., Seiphetlo, T., Ealand, C. S., Machowski, E., et al. (2023). Evaluation of a human mucosal tissue explant model for SARS-CoV-2 replication. PLoS One 18. doi: 10.1371/journal.pone.0291146
Goris, K., Uhlenbruck, S., Schwegmann-Wessels, C., Kohl, W., Niedorf, F., Stern, M., et al. (2009). Differential sensitivity of differentiated epithelial cells to respiratory viruses reveals different viral strategies of host infection. J. Virol 83, 1962–1968. doi: 10.1128/JVI.01271-08
Gray, T. E., Guzman, K., Davis, C. W., Abdullah, L. H., Nettesheim, P. (1996). Mucociliary differentiation of serially passaged normal human tracheobronchial epithelial cells. Am. J. Respir. Cell Mol. Biol. 14, 104–112. doi: 10.1165/ajrcmb.14.1.8534481
Gultom, M., Licheri, M., Laloli, L., Wider, M., Strassle, M., V’Kovski, P., et al. (2021). Susceptibility of well-differentiated airway epithelial cell cultures from domestic and wild animals to severe acute respiratory syndrome coronavirus 2. Emerg Infect. Dis. 27, 1811–1820. doi: 10.3201/eid2707.204660
Hanske, J., Heller, M., Schnee, C., Weldearegay, Y. B., Franzke, K., Jores, J., et al. (2023). A new duplex qPCR-based method to quantify Mycoplasma mycoides in complex cell culture systems and host tissues. J. Microbiol Methods 211, 106765. doi: 10.1016/j.mimet.2023.106765
Harrigan, J. A., Vezina, C. M., McGarrigle, B. P., Ersing, N., Box, H. C., Maccubbin, A. E., et al. (2004). DNA adduct formation in precision-cut rat liver and lung slices exposed to benzo[a]pyrene. Toxicol. Sci. 77, 307–314. doi: 10.1093/toxsci/kfh030
Harrington, H., Cato, P., Salazar, F., Wilkinson, M., Knox, A., Haycock, J. W., et al. (2014). Immunocompetent 3D model of human upper airway for disease modeling and in vitro drug evaluation. Mol. Pharm. 11, 2082–2091. doi: 10.1021/mp5000295
Hartmann, S., Sid, H., Rautenschlein, S. (2015). Avian metapneumovirus infection of chicken and Turkey tracheal organ cultures: comparison of virus-host interactions. Avian Pathol. 44, 480–489. doi: 10.1080/03079457.2015.1086974
Hays, A. M., Lantz, R. C., Witten, M. L. (2003). Correlation between in vivo and in vitro pulmonary responses to jet propulsion fuel-8 using precision-cut lung slices and a dynamic organ culture system. Toxicol. Pathol. 31, 200–207. doi: 10.1080/01926230390183689
Henjakovic, M., Sewald, K., Switalla, S., Kaiser, D., Muller, M., Veres, T. Z., et al. (2008). Ex vivo testing of immune responses in precision-cut lung slices. Toxicol. Appl. Pharmacol. 231, 68–76. doi: 10.1016/j.taap.2008.04.003
Hennion, R. M. (2015). The preparation of chicken tracheal organ cultures for virus isolation, propagation, and titration. Methods Mol. Biol. 1282, 51–56. doi: 10.1007/978-1-4939-2438-7_5
Huang, X., Li, L., Ammar, R., Zhang, Y., Wang, Y., Ravi, K., et al. (2019). Molecular characterization of a precision-cut rat lung slice model for the evaluation of antifibrotic drugs. Am. J. Physiol. Lung Cell Mol. Physiol. 316, L348–L357. doi: 10.1152/ajplung.00339.2018
Jackson, A. D., Cole, P. J., Wilson, R. (1996). Comparison of Haemophilus influenzae Type b Interaction with Respiratory Mucosa Organ Cultures Maintained with an Air Interface or Immersed in Medium. Infect. Immun. 64, 2353–2355. doi: 10.1128/iai.64.6.2353-2355.1996
Jang, Y. J., Lee, S. H., Kwon, H. J., Chung, Y. S., Lee, B. J. (2005). Development of rhinovirus study model using organ culture of turbinate mucosa. J. Virological Methods 125, 41–47. doi: 10.1016/j.jviromet.2004.12.004
Jiang, Z., Song, F., Li, Y., Xue, D., Deng, G., Li, M., et al. (2017). Capsular polysaccharide is a main component of mycoplasma ovipneumoniae in the pathogen-induced toll-like receptor-mediated inflammatory responses in sheep airway epithelial cells. Mediators Inflammation 2017, 9891673. doi: 10.1155/2017/9891673
Jones, B. V., Hennion, R. M. (2008). The preparation of chicken tracheal organ cultures for virus isolation, propagation, and titration. Methods Mol. Biol. 454, 103–107. doi: 10.1007/978-1-59745-181-9_9
Kaartinen, L., Nettesheim, P., Adler, K. B., Randell, S. H. (1993). Rat tracheal epithelial cell differentiation in vitro. In Vitro Cell Dev. Biol. Anim 29, 481–492. doi: 10.1007/BF02639383
Karp, P. H., Moninger, T. O., Weber, S. P., Nesselhauf, T. S., Launspach, J. L., Zabner, J., et al. (2002). An in vitro model of differentiated human airway epithelia. Methods for establishing primary cultures. Methods Mol. Biol. 188, 115–137. doi: 10.1385/1-59259-185-X:115
Khan, M. M., Poeckel, D., Halavatyi, A., Zukowska-Kasprzyk, J., Stein, F., Vappiani, J., et al. (2021). An integrated multiomic and quantitative label-free microscopy-based approach to study pro-fibrotic signalling in ex vivo human precision-cut lung slices. Eur. Respir. J. 58, 2000221. doi: 10.1183/13993003.00221-2020
Khoufache, K., Cabaret, O., Farrugia, C., Rivollet, D., Alliot, A., Allaire, E., et al. (2010). Primary in vitro culture of porcine tracheal epithelial cells in an air-liquid interface as a model to study airway epithelium and Aspergillus fumigatus interactions. Med. Mycol 48, 1049–1055. doi: 10.3109/13693786.2010.496119
Kirchhoff, J., Uhlenbruck, S., Goris, K., Keil, G. M., Herrler, G. (2014). Three viruses of the bovine respiratory disease complex apply different strategies to initiate infection. Vet Res. 45, 20. doi: 10.1186/1297-9716-45-20
Kishimoto, I., Ohnishi, H., Yamahara, K., Nakagawa, T., Yamashita, M., Omori, K., et al. (2021). Insulin-like growth factor 1 promotes the extension of Tracheal Epithelium in an in Vitro Tracheal organ culture model. Auris Nasus Larynx 48, 441–450. doi: 10.1016/j.anl.2020.09.017
Kletting, S., Barthold, S., Repnik, U., Griffiths, G., Loretz, B., Schneider-Daum, N., et al. (2018). Co-culture of human alveolar epithelial (hAELVi) and macrophage (THP-1) cell lines. ALTEX 35, 211–222. doi: 10.14573/altex.1607191
Knab, R., Petersen, H., Lin, H. J., Meixner, M., Rautenschlein, S., Jung, A. (2020). In vitro characterization and genetic diversity of Bordetella avium field strains. Avian Pathol. 49, 36–46. doi: 10.1080/03079457.2019.1660305
Kocan, A. A., Daubney, G. A., Kocan, K. M. (1978). Structural changes associated with type-A influenza in Mallard duck tracheal organ culture. Avian Dis. 22, 535–541. doi: 10.2307/1589311
Kothlow, S., Morgenroth, I., Tregaskes, C. A., Kaspers, B., Young, J. R. (2008). CD40 ligand supports the long-term maintenance and differentiation of chicken B cells in culture. Dev. Comp. Immunol. 32, 1015–1026. doi: 10.1016/j.dci.2008.01.012
Kreft, M. E., Jerman, U. D., Lasic, E., Hevir-Kene, N., Rizner, T. L., Peternel, L., et al. (2015). The characterization of the human cell line Calu-3 under different culture conditions and its use as an optimized in vitro model to investigate bronchial epithelial function. Eur. J. Pharm. Sci. 69, 1–9. doi: 10.1016/j.ejps.2014.12.017
Lam, M., Lamanna, E., Organ, L., Donovan, C., Bourke, J. E. (2023). Perspectives on precision cut lung slices-powerful tools for investigation of mechanisms and therapeutic targets in lung diseases. Front. Pharmacol. 14. doi: 10.3389/fphar.2023.1162889
Lam, E., Ramke, M., Groos, S., Warnecke, G., Heim, A. (2011). A differentiated porcine bronchial epithelial cell culture model for studying human adenovirus tropism and virulence. J. Virol Methods 178, 117–123. doi: 10.1016/j.jviromet.2011.08.025
Lambermont, V. A., Schleputz, M., Dassow, C., Konig, P., Zimmermann, L. J., Uhlig, S., et al. (2014). Comparison of airway responses in sheep of different age in precision-cut lung slices (PCLS). PLoS One 9, e97610. doi: 10.1371/journal.pone.0097610
Leach, T., Gandhi, U., Reeves, K. D., Stumpf, K., Okuda, K., Marini, F. C., et al. (2023). Development of a novel air-liquid interface airway tissue equivalent model for in vitro respiratory modeling studies. Sci. Rep. 13, 10137. doi: 10.1038/s41598-023-36863-1
Lechner, J. F., Haugen, A., McClendon, I. A., Shamsuddin, A. M. (1984). Induction of squamous differentiation of normal human bronchial epithelial cells by small amounts of serum. Differentiation 25, 229–237. doi: 10.1111/j.1432-0436.1984.tb01361.x
Lee, D., Chambers, M. (2019). A co-culture model of the bovine alveolus. F1000Res 8, 357. doi: 10.12688/f1000research.18696.2
Lee, S., Kim, J., Park, T. J., Shin, Y., Lee, S. Y., Han, Y. M., et al. (2011). The effects of the physical properties of culture substrates on the growth and differentiation of human embryonic stem cells. Biomaterials 32, 8816–8829. doi: 10.1016/j.biomaterials.2011.07.058
Lee, M. K., Yoo, J. W., Lin, H., Kim, Y. S., Kim, D. D., Choi, Y. M., et al. (2005). Air-liquid interface culture of serially passaged human nasal epithelial cell monolayer for in vitro drug transport studies. Drug Delivery 12, 305–311. doi: 10.1080/10717540500177009
Leeming, G., Meli, M. L., Cripps, P., Vaughan-Thomas, A., Lutz, H., Gaskell, R., et al. (2006). Tracheal organ cultures as a useful tool to study Felid herpesvirus 1 infection in respiratory epithelium. J. Virol Methods 138, 191–195. doi: 10.1016/j.jviromet.2006.07.010
Li, Y., Jiang, Z., Xue, D., Deng, G., Li, M., Liu, X., et al. (2016). Mycoplasma ovipneumoniae induces sheep airway epithelial cell apoptosis through an ERK signalling-mediated mitochondria pathway. BMC Microbiol 16, 222. doi: 10.1186/s12866-016-0842-0
Li, S., Lu, Y., Yang, S., Wang, C., Yang, J., Huang, X., et al. (2024). Porcine lung tissue slices: a culture model for PRCV infection and innate immune response investigations. AMB Express 14, 57. doi: 10.1186/s13568-024-01717-0
Lin, H., Li, H., Cho, H. J., Bian, S., Roh, H. J., Lee, M. K., et al. (2007). Air-liquid interface (ALI) culture of human bronchial epithelial cell monolayers as an in vitro model for airway drug transport studies. J. Pharm. Sci. 96, 341–350. doi: 10.1002/jps.20803
Linti, A. E., Gobel, T. W., Fruh, S. P. (2024). Chicken gammadelta T cells proliferate upon IL-2 and IL-12 treatment and show a restricted receptor repertoire in cell culture. Front. Immunol. 15. doi: 10.3389/fimmu.2024.1325024
Liu, G., Betts, C., Cunoosamy, D. M., Aberg, P. M., Hornberg, J. J., Sivars, K. B., et al. (2019). Use of precision cut lung slices as a translational model for the study of lung biology. Respir. Res. 20, 162. doi: 10.1186/s12931-019-1131-x
Liu, J., Hao, H., Xia, L., Ti, D., Huang, H., Dong, L., et al. (2015). Hypoxia pretreatment of bone marrow mesenchymal stem cells facilitates angiogenesis by improving the function of endothelial cells in diabetic rats with lower ischemia. PLoS One 10, e0126715. doi: 10.1371/journal.pone.0126715
Luengen, A. E., Kniebs, C., Buhl, E. M., Cornelissen, C. G., Schmitz-Rode, T., Jockenhoevel, S., et al. (2020). Choosing the right differentiation medium to develop mucociliary phenotype of primary nasal epithelial cells in vitro. Sci. Rep. 10, 6963. doi: 10.1038/s41598-020-63922-8
Lyons-Cohen, M. R., Thomas, S. Y., Cook, D. N., Nakano, H. (2017). Precision-cut mouse lung slices to visualize live pulmonary dendritic cells. J. Vis Exp. 122). doi: 10.3791/55465
Ma, Y., Han, F., Liang, J., Yang, J., Shi, J., Xue, J., et al. (2016). A species-specific activation of Toll-like receptor signaling in bovine and sheep bronchial epithelial cells triggered by Mycobacterial infections. Mol. Immunol. 71, 23–33. doi: 10.1016/j.molimm.2016.01.004
Mahieu, L., Van Moll, L., De Vooght, L., Delputte, P., Cos, P. (2024). In vitro modelling of bacterial pneumonia: a comparative analysis of widely applied complex cell culture models. FEMS Microbiol Rev. 48. doi: 10.1093/femsre/fuae007
Mao, H., Wang, Y., Yuan, W., Wong, L. B. (2009). Ciliogenesis in cryopreserved mammalian tracheal epithelial cells cultured at the air-liquid interface. Cryobiology 59, 250–257. doi: 10.1016/j.cryobiol.2009.07.012
Martin, C., Uhlig, S., Ullrich, V. (1996). Videomicroscopy of methacholine-induced contraction of individual airways in precision-cut lung slices. Eur. Respir. J. 9, 2479–2487. doi: 10.1183/09031936.96.09122479
Mathis, C., Poussin, C., Weisensee, D., Gebel, S., Hengstermann, A., Sewer, A., et al. (2013). Human bronchial epithelial cells exposed in vitro to cigarette smoke at the air-liquid interface resemble bronchial epithelium from human smokers. Am. J. Physiol. Lung Cell Mol. Physiol. 304, L489–L503. doi: 10.1152/ajplung.00181.2012
Mazzetto, E., Bortolami, A., Fusaro, A., Mazzacan, E., Maniero, S., Vascellari, M., et al. (2020). Replication of influenza D viruses of bovine and swine origin in ovine respiratory explants and their attachment to the respiratory tract of bovine, sheep, goat, horse, and swine. Front. Microbiol. 11. doi: 10.3389/fmicb.2020.01136
Melo-Narvaez, M. C., Golitz, F., Jain, E., Gote-Schniering, J., Stoleriu, M. G., Bertrams, W., et al. (2025). Cold storage of human precision-cut lung slices in TiProtec preserves cellular composition and transcriptional responses and enables on-demand mechanistic studies. Respir. Res. 26, 57. doi: 10.1186/s12931-025-03132-w
Meng, F., Tong, J., Votsch, D., Peng, J. Y., Cai, X., Willenborg, M., et al. (2019). Viral Coinfection Replaces Effects of Suilysin on Streptococcus suis Adherence to and Invasion of Respiratory Epithelial Cells Grown under Air-Liquid Interface Conditions. Infect. Immun. 87. doi: 10.1128/IAI.00350-19
Meng, F., Wu, N. H., Nerlich, A., Herrler, G., Valentin-Weigand, P., Seitz, M. (2015). Dynamic virus-bacterium interactions in a porcine precision-cut lung slice coinfection model: swine influenza virus paves the way for streptococcus suis infection in a two-step process. Infect. Immun. 83, 2806–2815. doi: 10.1128/IAI.00171-15
Meng, F., Wu, N. H., Seitz, M., Herrler, G., Valentin-Weigand, P. (2016). Efficient suilysin-mediated invasion and apoptosis in porcine respiratory epithelial cells after streptococcal infection under air-liquid interface conditions. Sci. Rep. 6, 26748. doi: 10.1038/srep26748
Min, K. A., Rosania, G. R., Kim, C. K., Shin, M. C. (2016). Functional and cytometric examination of different human lung epithelial cell types as drug transport barriers. Arch. Pharm. Res. 39, 359–369. doi: 10.1007/s12272-015-0704-6
Mossina, A., Lukas, C., Merl-Pham, J., Uhl, F. E., Mutze, K., Schamberger, A., et al. (2017). Cigarette smoke alters the secretome of lung epithelial cells. Proteomics 17, 1600243. doi: 10.1002/pmic.201600243
Muller, M., Fischer, K., Woehnke, E., Zaeck, L. M., Pronnecke, C., Knittler, M. R., et al. (2023). Analysis of nipah virus replication and host proteome response patterns in differentiated porcine airway epithelial cells cultured at the air-liquid interface. Viruses 15, 961. doi: 10.3390/v15040961
Neuhaus, V., Schaudien, D., Golovina, T., Temann, U. A., Thompson, C., Lippmann, T., et al. (2017). Assessment of long-term cultivated human precision-cut lung slices as an ex vivo system for evaluation of chronic cytotoxicity and functionality. J. Occup. Med. Toxicol. 12, 13. doi: 10.1186/s12995-017-0158-5
Niang, M., Rosenbusch, R. F., DeBey, M. C., Niyo, Y., Andrews, J. J., Kaeberle, M. L. (1998). Field isolates of Mycoplasma ovipneumoniae exhibit distinct cytopathic effects in ovine tracheal organ cultures. Zentralbl Veterinarmed A 45, 29–40. doi: 10.1111/j.1439-0442.1998.tb00798.x
Niehof, M., Hildebrandt, T., Danov, O., Arndt, K., Koschmann, J., Dahlmann, F., et al. (2017). RNA isolation from precision-cut lung slices (PCLS) from different species. BMC Res. Notes 10, 121. doi: 10.1186/s13104-017-2447-6
Niesalla, H. S., Dale, A., Slater, J. D., Scholes, S. F. E., Archer, J., Maskell, D. J., et al. (2009). Critical assessment of an in vitro bovine respiratory organ culture system: A model of bovine herpesvirus-1 infection. J. Virological Methods 158, 123–129. doi: 10.1016/j.jviromet.2009.02.001
O’Boyle, N., Sutherland, E., Berry, C. C., Davies, R. L. (2017). Temporal dynamics of ovine airway epithelial cell differentiation at an air-liquid interface. PLoS One 12, e0181583. doi: 10.1371/journal.pone.0181583
O’Boyle, N., Sutherland, E., Berry, C. C., Davies, R. L. (2018). Optimisation of growth conditions for ovine airway epithelial cell differentiation at an air-liquid interface. PLoS One 13, e0193998. doi: 10.1371/journal.pone.0193998
Oh, D., Han, S., Tignon, M., Balmelle, N., Cay, A. B., Griffioen, F., et al. (2023). Differential infection behavior of African swine fever virus (ASFV) genotype I and II in the upper respiratory tract. Veterinary Res. 54, 121–121. doi: 10.1186/s13567-023-01249-8
Oslund, K. L., Adamson, G., Wu, R. (2010). Evaluation of MUC5AC expression and upregulation in airway epithelial cells of horses. Am. J. Vet Res. 71, 690–696. doi: 10.2460/ajvr.71.6.690
Ostrowski, L. E., Nettesheim, P. (1995). Inhibition of ciliated cell differentiation by fluid submersion. Exp. Lung Res. 21, 957–970. doi: 10.3109/01902149509031773
Paddenberg, R., Mermer, P., Goldenberg, A., Kummer, W. (2014). Videomorphometric analysis of hypoxic pulmonary vasoconstriction of intra-pulmonary arteries using murine precision cut lung slices. J. Vis Exp. 83), e50970. doi: 10.3791/50970
Pan, C., Kumar, C., Bohl, S., Klingmueller, U., Mann, M. (2009). Comparative proteomic phenotyping of cell lines and primary cells to assess preservation of cell type-specific functions. Mol. Cell Proteomics 8, 443–450. doi: 10.1074/mcp.M800258-MCP200
Panchision, D. M. (2009). The role of oxygen in regulating neural stem cells in development and disease. J. Cell Physiol. 220, 562–568. doi: 10.1002/jcp.21812
Patel, V. S., Amin, K., Wahab, A., Marimoutou, M., Ukishima, L., Alvarez, J., et al. (2023). Cryopreserved human precision-cut lung slices provide an immune competent pulmonary test system for “on-demand Use long-term cultures. Toxicol. Sci. 191, 253–265. doi: 10.1093/toxsci/kfac136
Peng, J. Y., Punyadarsaniya, D., Shin, D. L., Pavasutthipaisit, S., Beineke, A., Li, G., et al. (2020). The cell tropism of porcine respiratory coronavirus for airway epithelial cells is determined by the expression of porcine aminopeptidase N. Viruses 12, 1211. doi: 10.3390/v12111211
Peng, J. Y., Shin, D. L., Li, G., Wu, N. H., Herrler, G. (2021). Time-dependent viral interference between influenza virus and coronavirus in the infection of differentiated porcine airway epithelial cells. Virulence 12, 1111–1121. doi: 10.1080/21505594.2021.1911148
Petersen, H., Matrosovich, M., Pleschka, S., Rautenschlein, S. (2012). Replication and adaptive mutations of low pathogenic avian influenza viruses in tracheal organ cultures of different avian species. PLoS One 7, e42260. doi: 10.1371/journal.pone.0042260
Pezzulo, A. A., Starner, T. D., Scheetz, T. E., Traver, G. L., Tilley, A. E., Harvey, B. G., et al. (2011). The air-liquid interface and use of primary cell cultures are important to recapitulate the transcriptional profile of in vivo airway epithelia. Am. J. Physiol. Lung Cell Mol. Physiol. 300, L25–L31. doi: 10.1152/ajplung.00256.2010
Placke, M. E., Fisher, G. L. (1987). Adult peripheral lung organ culture–a model for respiratory tract toxicology. Toxicol. Appl. Pharmacol. 90, 284–298. doi: 10.1016/0041-008x(87)90336-x
Pol, J. M. A., Quint, W. G. V., Kok, G. L., Broekhuysen-Davies, J. M. (1991). Pseudorabies virus infections in explants of porcine nasal mucosa. Res. Veterinary Sci. 50, 45–53. doi: 10.1016/0034-5288(91)90052-P
Qin, L., Meng, F., He, H., Li, S., Zhang, H., Sun, Y., et al. (2023). Inflammation plays a critical role in damage to the bronchiolar epithelium induced by Trueperella pyogenes in vitro and in vivo. Infect. Immun. 91, e0027323. doi: 10.1128/iai.00273-23
Qin, L., Meng, F., He, H., Yang, Y. B., Wang, G., Tang, Y. D., et al. (2021). A virulent trueperella pyogenes isolate, which causes severe bronchoconstriction in porcine precision-cut lung slices. Front. Vet Sci. 8. doi: 10.3389/fvets.2021.824349
Quintana, A. M., Landolt, G. A., Annis, K. M., Hussey, G. S. (2011). Immunological characterization of the equine airway epithelium and of a primary equine airway epithelial cell culture model. Vet Immunol. Immunopathol 140, 226–236. doi: 10.1016/j.vetimm.2010.12.008
Radi, Z. A., Ackermann, M. R. (2004). Growth of differentiated ovine tracheal epithelial cells in vitro. J. Vet Med. A Physiol. Pathol. Clin. Med. 51, 167–170. doi: 10.1111/j.1439-0442.2004.00620.x
Raj, G. D., Jones, R. C. (1996). An in vitro comparison of the virulence of seven strains of infectious bronchitis virus using tracheal and oviduct organ cultures. Avian Pathol. 25, 649–662. doi: 10.1080/03079459608419172
Ramezanpour, M., Moraitis, S., Smith, J. L., Wormald, P. J., Vreugde, S. (2016). Th17 cytokines disrupt the airway mucosal barrier in chronic rhinosinusitis. Mediators Inflammation 2016, 9798206. doi: 10.1155/2016/9798206
Ram-Mohan, S., Bai, Y., Schaible, N., Ehrlicher, A. J., Cook, D. P., Suki, B., et al. (2020). Tissue traction microscopy to quantify muscle contraction within precision-cut lung slices. Am. J. Physiol. Lung Cell Mol. Physiol. 318, L323–L330. doi: 10.1152/ajplung.00297.2019
Read, R. C., Fox, A., Miller, K., Gray, T., Jones, N., Borrow, R., et al. (1995). Experimental infection of human nasal mucosal explants with Neisseria meningitidis. J. Med. Microbiol. 42, 353–361. doi: 10.1099/00222615-42-5-353
Read, R. C., Goodwin, L., Parsons, M. A., Silcocks, P., Kaczmarski, E. B., Parker, A., et al. (1999). Coinfection with influenza B virus does not affect association of neisseria meningitidis with human nasopharyngeal mucosa in organ culture. Infection And Immun. 67, 3082–3086. doi: 10.1128/IAI.67.6.3082-3086.1999
Reamon-Buettner, S. M., Niehof, M., Hirth, N., Danov, O., Obernolte, H., Braun, A., et al. (2019). Transcriptomic analysis reveals priming of the host antiviral interferon signaling pathway by bronchobini((R)) resulting in balanced immune response to rhinovirus infection in mouse lung tissue slices. Int. J. Mol. Sci. 20, 2242. doi: 10.3390/ijms20092242
Reemers, S. S., Koerkamp, M. J. G., Holstege, F. C., van Eden, W., Vervelde, L. (2009). Cellular host transcriptional responses to influenza A virus in chicken tracheal organ cultures differ from responses in infected trachea. Veterinary Immunol. Immunopathology 132, 91–100. doi: 10.1016/j.vetimm.2009.04.021
Remot, A., Carreras, F., Coupe, A., Doz-Deblauwe, E., Boschiroli, M. L., Browne, J. A., et al. (2021). Mycobacterial Infection of Precision-Cut Lung Slices Reveals Type 1 Interferon Pathway Is Locally Induced by Mycobacterium bovis but Not M. tuberculosis in a Cattle Breed. Front. Vet Sci. 8. doi: 10.3389/fvets.2021.696525
Rosner, S. R., Ram-Mohan, S., Paez-Cortez, J. R., Lavoie, T. L., Dowell, M. L., Yuan, L., et al. (2014). Airway contractility in the precision-cut lung slice after cryopreservation. Am. J. Respir. Cell Mol. Biol. 50, 876–881. doi: 10.1165/rcmb.2013-0166MA
Ross, A. J., Dailey, L. A., Brighton, L. E., Devlin, R. B. (2007). Transcriptional profiling of mucociliary differentiation in human airway epithelial cells. Am. J. Respir. Cell Mol. Biol. 37, 169–185. doi: 10.1165/rcmb.2006-0466OC
Ruger, N., Sid, H., Meens, J., Szostak, M. P., Baumgartner, W., Bexter, F., et al. (2021). New Insights into the Host-Pathogen Interaction of Mycoplasma gallisepticum and Avian Metapneumovirus in Tracheal Organ Cultures of Chicken. Microorganisms 9, 2407. doi: 10.3390/microorganisms9112407
Ruigrok, M. J. R., Tomar, J., Frijlink, H. W., Melgert, B. N., Hinrichs, W. L. J., Olinga, P. (2019). The effects of oxygen concentration on cell death, anti-oxidant transcription, acute inflammation, and cell proliferation in precision-cut lung slices. Sci. Rep. 9, 16239. doi: 10.1038/s41598-019-52813-2
Russell, W. M. S., Burch, R. L. (1959). The Principles of Humane Experimental Technique. (UK: reprinted 1992: Universities Federation for Animal Welfare, Wheathampstaed).
Russell, A. L., Lefavor, R., Durand, N., Glover, L., Zubair, A. C. (2018). Modifiers of mesenchymal stem cell quantity and quality. Transfusion 58, 1434–1440. doi: 10.1111/trf.14597
Schleputz, M., Uhlig, S., Martin, C. (2011). Electric field stimulation of precision-cut lung slices. J. Appl. Physiol. (1985) 110, 545–554. doi: 10.1152/japplphysiol.00409.2010
Schwab, U. E., Fulcher, M. L., Randell, S. H., Flaminio, M. J., Russell, D. G. (2010). Equine bronchial epithelial cells differentiate into ciliated and mucus producing cells in vitro. In Vitro Cell Dev. Biol. Anim 46, 102–106. doi: 10.1007/s11626-009-9258-6
Shen, C. I., Wang, C. H., Liao, J. W., Hsu, T. W., Kuo, S. M., Su, H. L. (2010). The infection of primary avian tracheal epithelial cells with infectious bronchitis virus. Vet Res. 41, 6. doi: 10.1051/vetres/2009054
Shin, D. L., Yang, W., Peng, J. Y., Sawatsky, B., von Messling, V., Herrler, G., et al. (2020). Avian influenza A virus infects swine airway epithelial cells without prior adaptation. Viruses 12, 589. doi: 10.3390/v12060589
Sid, H., Hartmann, S., Petersen, H., Ryll, M., Rautenschlein, S. (2016). Mycoplasma gallisepticum modifies the pathogenesis of influenza A virus in the avian tracheal epithelium. Int. J. Med. Microbiol 306, 174–186. doi: 10.1016/j.ijmm.2016.04.001
Stadejek, W., Chiers, K., Van Reeth, K. (2023). Infectivity and transmissibility of an avian H3N1 influenza virus in pigs. Veterinary Res. 54, 4–4. doi: 10.1186/s13567-022-01133-x
Starbæk, S. M. R., Brogaard, L., Dawson, H. D., Smith, A. D., Heegaard, P. M. H., Larsen, L. E., et al. (2018). Animal models for influenza A virus infection incorporating the involvement of innate host defenses: enhanced translational value of the porcine model. ILAR J. 59, 323–337. doi: 10.1093/ilar/ily009
Stegmayr, J., Alsafadi, H. N., Langwinski, W., Niroomand, A., Lindstedt, S., Leigh, N. D., et al. (2021). Isolation of high-yield and -quality RNA from human precision-cut lung slices for RNA-sequencing and computational integration with larger patient cohorts. Am. J. Physiol. Lung Cell Mol. Physiol. 320, L232–L240. doi: 10.1152/ajplung.00401.2020
Steukers, L., Vandekerckhove, A. P., Van den Broeck, W., Glorieux, S., Nauwynck, H. J. (2011). Comparative analysis of replication characteristics of BoHV-1 subtypes in bovine respiratory and genital mucosa explants: a phylogenetic enlightenment. Vet Res. 42, 33. doi: 10.1186/1297-9716-42-33
Steukers, L., Vandekerckhove, A. P., Van den Broeck, W., Glorieux, S., Nauwynck, H. J. (2012). Kinetics of BoHV-1 dissemination in an in vitro culture of bovine upper respiratory tract mucosa explants. ILAR journal/National Res. Council Institute Lab. Anim. Resour. 53, E43–E54. doi: 10.1093/ilar.53.1.43
Stewart, C. E., Torr, E. E., Mohd Jamili, N. H., Bosquillon, C., Sayers, I. (2012). Evaluation of differentiated human bronchial epithelial cell culture systems for asthma research. J. Allergy (Cairo) 2012, 943982. doi: 10.1155/2012/943982
Strassle, M., Laloli, L., Gultom, M., V’Kovski, P., Stoffel, M. H., Crespo Pomar, S., et al. (2021). Establishment of caprine airway epithelial cells grown in an air-liquid interface system to study caprine respiratory viruses and bacteria. Vet Microbiol 257, 109067. doi: 10.1016/j.vetmic.2021.109067
Su, A., Tong, J., Fu, Y., Muller, S., Weldearegay, Y. B., Becher, P., et al. (2020). Infection of bovine well-differentiated airway epithelial cells by Pasteurella multocida: actions and counteractions in the bacteria-host interactions. Vet Res. 51, 140. doi: 10.1186/s13567-020-00861-2
Taylor, K. J. M., Ngunjiri, J. M., Abundo, M. C., Jang, H., Elaish, M., Ghorbani, A., et al. (2020). Respiratory and gut microbiota in commercial Turkey flocks with disparate weight gain trajectories display differential compositional dynamics. Appl. Environ. Microbiol 86. doi: 10.1128/AEM.00431-20
Temann, A., Golovina, T., Neuhaus, V., Thompson, C., Chichester, J. A., Braun, A., et al. (2017). Evaluation of inflammatory and immune responses in long-term cultured human precision-cut lung slices. Hum. Vaccin Immunother 13, 351–358. doi: 10.1080/21645515.2017.1264794
Thomas, L. H., Howard, C. J., Parsons, K. R., Anger, H. S. (1987). Growth of Mycoplasma bovis in organ cultures of bovine foetal trachea and comparison with Mycoplasma dispar. Vet Microbiol 13, 189–200. doi: 10.1016/0378-1135(87)90044-7
Tigges, J., Eggerbauer, F., Worek, F., Thiermann, H., Rauen, U., Wille, T. (2021). Optimization of long-term cold storage of rat precision-cut lung slices with a tissue preservation solution. Am. J. Physiol. Lung Cell Mol. Physiol. 321, L1023–L1035. doi: 10.1152/ajplung.00076.2021
Tulinski, P., Duim, B., Wittink, F. R., Jonker, M. J., Breit, T. M., van Putten, J. P., et al. (2014). Staphylococcus aureus ST398 gene expression profiling during ex vivo colonization of porcine nasal epithelium. BMC Genomics 15, 915. doi: 10.1186/1471-2164-15-915
Tulinski, P., Fluit, A. C., van Putten, J. P. M., de Bruin, A., Glorieux, S., Wagenaar, J. A., et al. (2013). An ex vivo porcine nasal mucosa explants model to study MRSA colonization. PLoS One 8. doi: 10.1371/journal.pone.0053783
Vairo, S., Van Den Broeck, W., Favoreel, H., Scagliarini, A., Nauwynck, H. (2013). Development and use of a polarized equine upper respiratory tract mucosal explant system to study the early phase of pathogenesis of a European strain of equine arteritis virus. Veterinary Res. 44, 22. doi: 10.1186/1297-9716-44-22
Van Cleemput, J., Poelaert, K. C. K., Laval, K., Maes, R., Hussey, G. S., Van den Broeck, W., et al. (2017). Access to a main alphaherpesvirus receptor, located basolaterally in the respiratory epithelium, is masked by intercellular junctions. Sci. Rep. 7, 16656. doi: 10.1038/s41598-017-16804-5
Van Cleemput, J., Poelaert, K. C. K., Laval, K., Nauwynck, H. J. (2019). Unravelling the first key steps in equine herpesvirus type 5 (EHV5) pathogenesis using ex vivo and in vitro equine models. Veterinary Res. 50, 13. doi: 10.1186/s13567-019-0630-6
Vandekerckhove, A., Glorieux, S., Broeck, W. V. D., Gryspeerdt, A., van der Meulen, K. M., Nauwynck, H. J. (2009). In vitro culture of equine respiratory mucosa explants. Veterinary J. 181, 280–287. doi: 10.1016/j.tvjl.2008.03.027
Van Poucke, S. G. M., Nicholls, J. M., Nauwynck, H. J., Van Reeth, K. (2010). Replication of avian, human and swine influenza viruses in porcine respiratory explants and association with sialic acid distribution. Virol. J. 7, 38. doi: 10.1186/1743-422X-7-38
Verstappen, K. M., Tulinski, P., Duim, B., Fluit, A. C., Carney, J., Van Nes, A., et al. (2016). The effectiveness of bacteriophages against methicillin-resistant Staphylococcus aureus ST398 nasal colonization in pigs. PLoS One 11. doi: 10.1371/journal.pone.0160242
Viana, F., O’Kane, C. M., Schroeder, G. N. (2022). Precision-cut lung slices: A powerful ex vivo model to investigate respiratory infectious diseases. Mol. Microbiol 117, 578–588. doi: 10.1111/mmi.14817
Votsch, D., Willenborg, M., Baumgartner, W., Rohde, M., Valentin-Weigand, P. (2021). Bordetella bronchiseptica promotes adherence, colonization, and cytotoxicity of Streptococcus suis in a porcine precision-cut lung slice model. Virulence 12, 84–95. doi: 10.1080/21505594.2020.1858604
Wang, H., He, L., Liu, B., Feng, Y., Zhou, H., Zhang, Z., et al. (2018). Establishment and comparison of air-liquid interface culture systems for primary and immortalized swine tracheal epithelial cells. BMC Cell Biol. 19, 10. doi: 10.1186/s12860-018-0162-3
Wang, X. D., Zhang, N., Glorieux, S., Holtappels, G., Vaneechoutte, M., Krysko, O., et al. (2012). Herpes simplex virus type 1 infection facilitates invasion of Staphylococcus aureus into the nasal mucosa and nasal polyp tissue. PLoS One 7. doi: 10.1371/journal.pone.0039875
Wang, H., Zhang, Z., Xie, X., Liu, B., Wei, Y., Gan, Y., et al. (2020). Paracellular Pathway-Mediated Mycoplasma hyopneumoniae Migration across Porcine Airway Epithelial Barrier under Air-Liquid Interface Conditions. Infect. Immun. 88, 1128. doi: 10.1128/IAI.00470-20
Watson, C. Y., Damiani, F., Ram-Mohan, S., Rodrigues, S., de Moura Queiroz, P., Donaghey, T. C., et al. (2016). Screening for chemical toxicity using cryopreserved precision cut lung slices. Toxicol. Sci. 150, 225–233. doi: 10.1093/toxsci/kfv320
Weldearegay, Y. B., Brogaard, L., Nerlich, A., Schaaf, D., Heegaard, P. M. H., Valentin-Weigand, P. (2023). Transcriptional host responses to infection with streptococcus suis in a porcine precision-cut lung slice model: between-strain differences suggest association with virulence potential. Pathogens 13, 4. doi: 10.3390/pathogens13010004
Weldearegay, Y. B., Muller, S., Hanske, J., Schulze, A., Kostka, A., Ruger, N., et al. (2019). Host-pathogen interactions of mycoplasma mycoides in caprine and bovine precision-cut lung slices (PCLS) models. Pathogens 8, 82. doi: 10.3390/pathogens8020082
Whitcutt, M. J., Adler, K. B., Wu, R. (1988). A biphasic chamber system for maintaining polarity of differentiation of cultured respiratory tract epithelial cells. In Vitro Cell Dev. Biol. 24, 420–428. doi: 10.1007/bf02628493
Widdicombe, J. H., Sachs, L. A., Finkbeiner, W. E. (2003). Effects of growth surface on differentiation of cultures of human tracheal epithelium. In Vitro Cell Dev. Biol. Anim 39, 51–55. doi: 10.1290/1543-706X(2003)039<0051:EOGSOD>2.0.CO;2
Winter, C., Herrler, G., Neumann, U. (2008). Infection of the tracheal epithelium by infectious bronchitis virus is sialic acid dependent. Microbes Infect. 10, 367–373. doi: 10.1016/j.micinf.2007.12.009
Wiszniewski, L., Jornot, L., Dudez, T., Pagano, A., Rochat, T., Lacroix, J. S., et al. (2006). Long-term cultures of polarized airway epithelial cells from patients with cystic fibrosis. Am. J. Respir. Cell Mol. Biol. 34, 39–48. doi: 10.1165/rcmb.2005-0161OC
Wolterbeek, A. P., Ciotti, M. A., Schoevers, E. J., Roggeband, R., Baan, R. A., Feron, V. J., et al. (1996). Influence of growth factors and medium composition on benzo[a]pyrene- and vitamin A-induced cell proliferation and differentiation in hamster tracheal epithelium in organ culture. Toxicol. In Vitro 10, 359–369. doi: 10.1016/0887-2333(96)00010-0
Wu, N. H., Yang, W., Beineke, A., Dijkman, R., Matrosovich, M., Baumgartner, W., et al. (2016). The differentiated airway epithelium infected by influenza viruses maintains the barrier function despite a dramatic loss of ciliated cells. Sci. Rep. 6, 39668. doi: 10.1038/srep39668
Xue, D., Ma, Y., Li, M., Li, Y., Luo, H., Liu, X., et al. (2015). Mycoplasma ovipneumoniae induces inflammatory response in sheep airway epithelial cells via a MyD88-dependent TLR signaling pathway. Vet Immunol. Immunopathol 163, 57–66. doi: 10.1016/j.vetimm.2014.11.008
Yang, B., Xie, J., Van Cleemput, J., Wei, R., Opsomer, G., Nauwynck, H. J. (2019). Gammaherpesvirus BoHV-4 infects bovine respiratory epithelial cells mainly at the basolateral side. Vet Res. 50, 11. doi: 10.1186/s13567-019-0629-z
Yoon, J. H., Gray, T., Guzman, K., Koo, J. S., Nettesheim, P. (1997). Regulation of the secretory phenotype of human airway epithelium by retinoic acid, triiodothyronine, and extracellular matrix. Am. J. Respir. Cell Mol. Biol. 16, 724–731. doi: 10.1165/ajrcmb.16.6.9191474
Zhang, S., Jian, F., Zhao, G., Huang, L., Zhang, L., Ning, C., et al. (2012). Chick embryo tracheal organ: a new and effective in vitro culture model for Cryptosporidium baileyi. Vet Parasitol 188, 376–381. doi: 10.1016/j.vetpar.2012.03.049
Zhao, J., Negussie, H., Laval, K., Poelaert, K. C. K., Nauwynck, H. J. (2016). Dual infections of equine herpesvirus 1 and equine arteritis virus in equine respiratory mucosa explants. Virus Res. 220, 104–111. doi: 10.1016/j.virusres.2016.04.013
Zhao, J., Poelaert, K. C. K., Van Cleemput, J., Nauwynck, H. J. (2017). CCL2 and CCL5 driven attraction of CD172a+ monocytic cells during an equine herpesvirus type 1 (EHV-1) infection in equine nasal mucosa and the impact of two migration inhibitors, rosiglitazone (RSG) and quinacrine (QC). Veterinary Res. 48, 14. doi: 10.1186/s13567-017-0419-4
Zheng, J., Lin, J., Ma, Y., Yang, C., Zhong, Q., Li, Y., et al. (2023). Establishment of sheep nasal mucosa explant model and its application in antiviral research. Front. Microbiol. 14. doi: 10.3389/fmicb.2023.1124936
Keywords: nasal mucosa explants, tracheal organ cultures, precision-cut lung slices, air-liquid interface cultures, respiratory tract infections, organotypic models, livestock diseases
Citation: Weldearegay YB, Brogaard L, Rautenschlein S, Meens J, Valentin-Weigand P and Schaaf D (2025) Primary cell culture systems to investigate host-pathogen interactions in bacterial respiratory tract infections of livestock. Front. Cell. Infect. Microbiol. 15:1565513. doi: 10.3389/fcimb.2025.1565513
Received: 18 February 2025; Accepted: 14 April 2025;
Published: 09 May 2025.
Edited by:
Percy Schröttner, Technische Universität Dresden, GermanyReviewed by:
Marta Alonso-Hearn, Basque Research and Technology Alliance (BRTA), SpainMaria Camila Melo-Narváez, University of Marburg, Germany
Copyright © 2025 Weldearegay, Brogaard, Rautenschlein, Meens, Valentin-Weigand and Schaaf. This is an open-access article distributed under the terms of the Creative Commons Attribution License (CC BY). The use, distribution or reproduction in other forums is permitted, provided the original author(s) and the copyright owner(s) are credited and that the original publication in this journal is cited, in accordance with accepted academic practice. No use, distribution or reproduction is permitted which does not comply with these terms.
*Correspondence: Désirée Schaaf, ZGVzaXJlZS5zY2hhYWZAdGloby1oYW5ub3Zlci5kZQ==