- 1Stazione Zoologica Anton Dohrn, Naples, Italy
- 2Centre For Life's Origins and Evolution, University College London, London, United Kingdom
Neurons and pancreatic endocrine cells have a common physiology and express a similar toolkit of transcription factors during development. To explain these common features, it has been hypothesized that pancreatic cells most likely co-opted a pre-existing gene regulatory program from ancestral neurons. To test this idea, we looked for neurons with a “pre-pancreatic” program in an early-branched deuterostome, the sea urchin. Only vertebrates have a proper pancreas, however, our lab previously found that cells with a pancreatic-like signature are localized within the sea urchin embryonic gut. We also found that the pancreatic transcription factors Xlox/Pdx1 and Brn1/2/4 co-localize in a sub-population of ectodermal cells. Here, we find that the ectodermal SpLox+ SpBrn1/2/4 cells are specified as SpSoxC and SpPtf1a neuronal precursors that become the lateral ganglion and the apical organ neurons. Two of the SpLox+ SpBrn1/2/4 cells also express another pancreatic transcription factor, the LIM-homeodomain gene islet-1. Moreover, we find that SpLox neurons produce the neuropeptide SpANP2, and that SpLox regulates SpANP2 expression. Taken together, our data reveal that there is a subset of sea urchin larval neurons with a gene program that predated pancreatic cells. These findings suggest that pancreatic endocrine cells co-opted a regulatory signature from an ancestral neuron that was already present in an early-branched deuterostome.
Introduction
Complex organisms have more cell types than structurally simple ones. In many cases, functionally distinct cell types show remarkably similar gene programs. This shared program can be the result of a common evolutionary ancestor cell or of convergent evolution. Another possibility is the co-option of parts of gene networks from an ancestral cell to a new cell that leads to a parallel use of the same gene repertoire. Pancreatic endocrine β-cells and neurons are an example of different cell types that share a similar gene program but exert different functions. These two cell types share many remarkable features (1–7). Some features are common to all endocrine cells, like the ability of producing polypeptide hormones (1, 2), neurotransmitters and their receptors (3, 8), while other are specific of pancreatic β-cells, like mRNA expression and chromatin methylation pattern similar to neurons (5). Many genes expressed in neuronal development are also expressed in the development of pancreatic β-cells (7), like the homeodomain protein Isl1 (9), the bHLH transcription factors neurogenins (10–12), and the homeobox transcription factor PDX1 (13, 14).
All of these aspects lead to the idea that gut cells co-opted a neuronal transcriptional program leading to the evolution of β-cells (15). While all deuterostomes have a nervous system, the discrete pancreas with fully-developed β-cells is a vertebrate specific organ (16). Therefore, gut cells must have adopted part of a neuronal program before the vertebrate ancestor appeared. When did gut cells adopt a neuronal program? Was this program common to all neurons, or rather distinctive of only some neuronal populations? An approach to answer these questions is to identify neurons that express pancreatic toolkit genes in invertebrates that have gut cells with a pancreatic-like program. Together with vertebrates, chordates include tunicates and cephalochordates. Both groups have gut cells that express insulin-like peptides (ILPs). The cephalochordate amphioxus has an ILP localized in the gut and the mesoderm (17, 18), while tunicates have cells producing ILPs in the gut and the nervous system (19–21). Besides the ILP expression pattern, there are no data on the presence of a pancreatic-like gene regulatory program in cephalochordates and tunicates.
The sister group of chordates includes the echinoderms, a clade of early-branching deuterostomes like the sea urchin Strongylocentrotus purpuratus. The sea urchin's simple development and available genetic tools enable comparative studies on cell specification and gene regulatory networks with other deuterostomes, such as vertebrates (22, 23).
Although sea urchin larvae do not have a true pancreas, we previously found that there are subsets of gut cells that express a pancreatic program similar to the exocrine pancreas (24). We next asked whether in the same animal there are neurons that express the transcription factors co-opted by pancreatic β-cells (summary of the genes discussed in this paper in Supplementary Table 1). For simplicity, we name the neuronal genes that are expressed in the pancreas “pre-pancreatic” genes. To date, the only cells in sea urchins known to express the neuronal transcription factors co-opted by the pancreas are a group of 3–4 ectodermal cells that express SpLox, the sea urchin homolog of Pdx1 (25). In vertebrate embryos, Pdx1 is expressed in two domains: within the duodenum and the developing pancreatic endoderm (26) and in the neural cells during brain development (27). The SpLox ectodermal cells co-express the gene SpBrn1/2/4 (25). SpBrn1/2/4 is a member of the POU3 family. The human genes Brn1, Brn2, Brn4, and Oct6 are all equal co-orthologs of the sea urchin gene SpBRN1/2/4 (28). In mammals, Brn4 is involved in the specification of glucagon producing α-cells (10, 29), while for instance Oct6 is expressed in distinct mouse brain regions (30, 31). However, besides SpLox and SpBrn1/2/4 cells in the ciliary band, we lack information on the presence of neurons expressing pre-pancreatic gene. In addition, although many features of neurogenesis in sea urchins have been explained (32–36), we still know little about the diversity of neuronal subtypes. Our hypothesis is that if a pre-pancreatic neuronal subtype exists, then the gut cells that gave rise to the endocrine pancreas co-opted a gene program specific of one neuronal subtype, rather than a generic one. To this aim, we asked whether there are neurons with a gene program that resembles the pancreatic one in the sea urchin. Here we describe new subtypes of neurons that display a pre-pancreatic regulatory fingerprint and are marked by SpLox. We define these neurons as “pre-pancreatic neurons,” because these are the cells that express the gene program co-opted by the pancreas.
Results
SpLox is Expressed in Lateral Ganglion and Apical Organ Neurons
In situ hybridization data have previously shown that the SpLox gene is expressed in the endoderm (37) and also in three ectodermal cells together with SpBrn1/2/4 (25). SpLox orthologs (Pdx/xlox) are involved in both nervous system and pancreas development (26, 27). Hence, SpLox is an important gene to investigate the regulatory state of neurons that express pre-pancreatic genes. Using an anti-SpLox antibody (38), we define the SpLox+Brn1/2/4 cells in the ciliary band in relation with Synaptotagmin B (SynB), a marker of differentiated neurons in invertebrates (39). Our data revealed that the SpLox+SpBrn1/2/4+ cells were lateral ganglion neurons (Figures 1A–C). At 96 h post-fertilization (hpf), SpLox was localized in two of the left lateral ganglia and one to two right lateral ganglion cells (Figures 1A,A',A” oral view; Figures 1B,B',B” left view; Figures 1C,C',C” right view). SpLox protein was localized in the nucleus only, consistent with its function as a transcription factor.
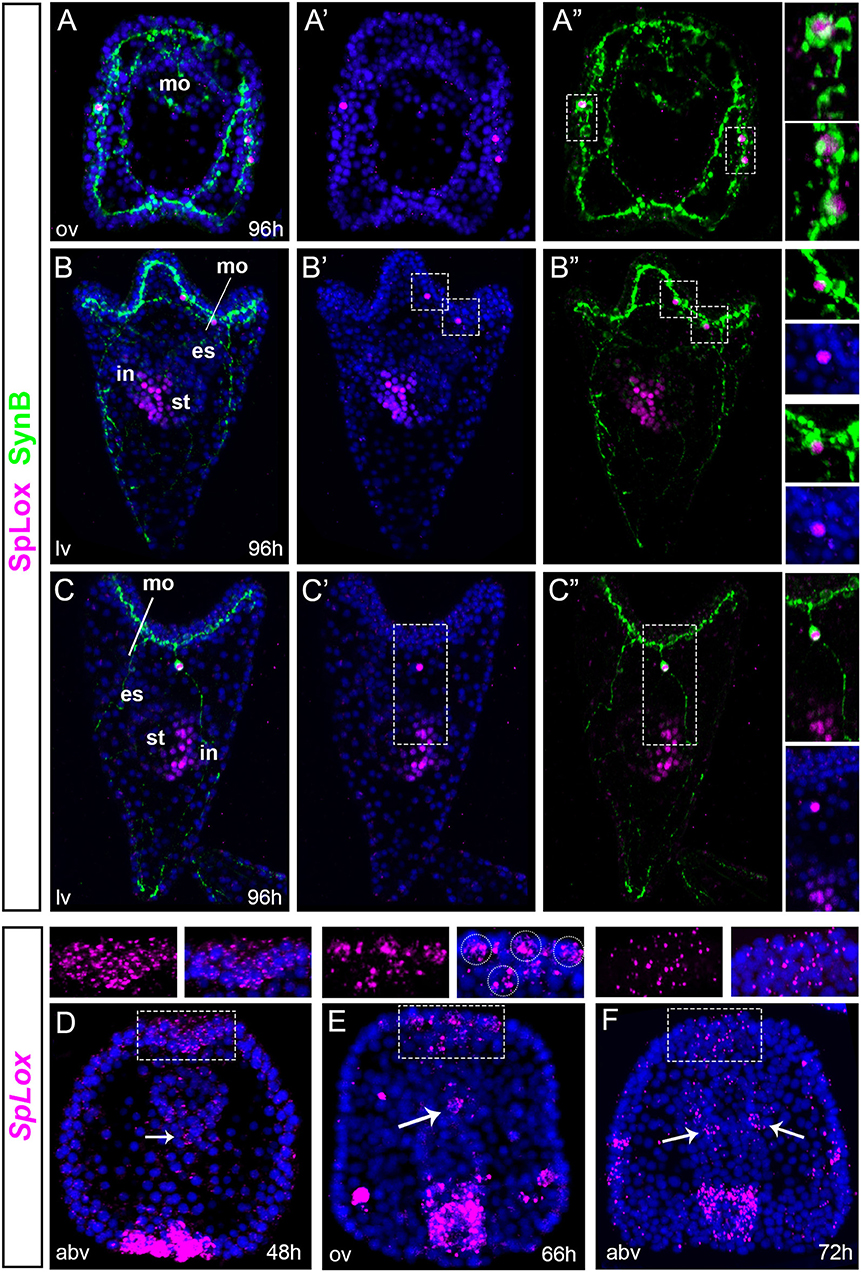
Figure 1. SpLox is expressed in lateral ganglion neurons. (A–C”) SpLox (magenta) and SynB (green) protein localization in late larvae. Nuclei are labeled blue with DAPI. In (A”,B”,C”) nuclei are omitted in order to see the long and interconnected network formed by the neurite projections. Insets on the right show SpLox expression in the nucleiof left lateral ganglia (A,B) and right lateral ganglia (A,C). (D–F) Are fluorescent in situ hybridization (FISH) showing the localization of SpLox mRNA in the apical plate in late gastrula (A), prism (B) and early larva (C) stages. White dashed line boxes are magnifications of the apical plate area. White arrowheads indicate SpLox localization in the foregut. All pictures are full projections of merged confocal stacks. Nuclei are stained with DAPI and depicted in blue. es, esophagus; in, intestine; mo, mouth; st, stomach; abv, aboral view; lv, lateral view; ov, oral view.
Next, we identified previously undescribed cells that expressed SpLox transcripts. First, in gastrulae we found cells within the apical plate were SpLox mRNA was diffused (Figure 1D). At 66 hpf the signal narrowed down to a distinct group of 3–4 cells of the apical organ (Figure 1E) and by 72 hpf SpLox expression was faint and diffused again (Figure 1F). Second, we identified distinct cells of the foregut that expressed SpLox (Figures 1E,F, white arrows). Thus, we found that the previously identified SpLox cells below the ciliary band are lateral ganglia neurons, and that SpLox is also dynamically expressed in the apical organ and foregut up to early larval stages.
Scattered Ciliary Band Neurons Express Single Pre-pancreatic Genes
We examined the expression of pre-pancreatic transcription factors in ectodermal cells that give rise to neurons. For some of these vertebrate genes there are multiple paralogs, while the sea urchin genome features only one paralog. Neurogenins are transcription factors expressed early in endocrine pancreas precursors (40, 41) and in neuronal differentiation (42, 43). The only sea urchin neurogenin ortholog is SpNgn. In gastrulae, SpNgn was expressed at the animal pole and in individual cells located within the ciliary band (Figure 2A). This expression increased at early pluteus stage where several SpNgn cells were found throughout the ciliary band (Figure 2D). Islet is another vertebrate gene that is expressed in the developing pancreas and in the nervous system (9, 44). In sea urchin gastrulae, SpIsl was expressed broadly in the oral ectoderm, particularly on the right side (Figure 2B). The expression increased during development and at the larval stage, SpIsl was expressed throughout the ciliary band and a few cells of the post-oral ectoderm (Figure 2E). SpIsl was also expressed in the foregut and in a group of cells in the upper and lower lip (Figure 2E). Last, we analyzed the expression profile of SpNeuroD. The SpNeuroD vertebrate orthologs are key regulators of neuronal terminal differentiation and pancreas development (45–49). In gastrulae, SpNeuroD was widely expressed in the oral ectoderm (Figure 2C). In early larvae, SpNeuroD was detected at low levels in several cells throughout the ciliary band and the apical organ (Figure 2F).
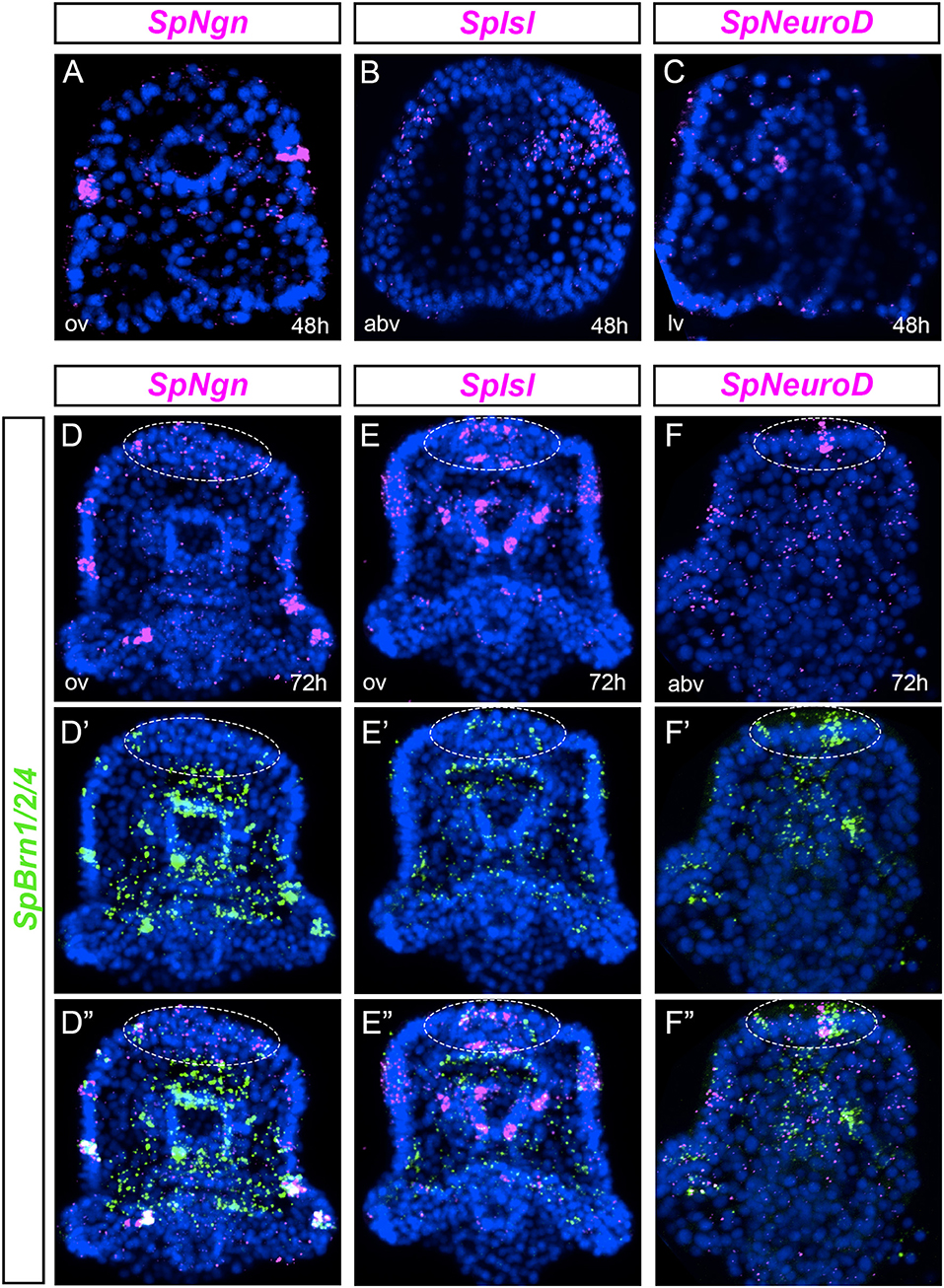
Figure 2. Co-expression analysis of markers of pancreatic transcription factors and SpBrn1/2/4 define unique neurons. (A–C) FISH of SpNgn, SpIsl, and SpNeuroD in gastrulae. (D–F”) Double fluorescent in situ hybridization (FISH) of SpNgn, SpIsl, and SpNeuroD with SpBrn1/2/4 combined with nuclear staining (DAPI, blue) in early larvae. White dashed-line circles highlight the apical organ region. All images are obtained as stacks of merged confocal Z sections. Split and combined channels of single confocal sections are provided to show that genes are expressed in the same cells. abv, aboral view; av, aboral view; lv, lateral view; ov, oral view.
Given that SpNgn, SpIsl and SpNeuroD were all expressed in ectodermal cells, we asked if those cells were neurons that co-express SpBrn1/2/4, recently identified as a key gene of the sea urchin neural specification process (50). We found that most of the SpNgn positive ciliary band cells co-expressed SpBrn1/2/4 (Figure 2D”). Similarly, SpIsl cells of the ciliary band, apical organ and foregut were SpBrn1/2/4+ (Figure 2E”). Last, the SpNeuroD apical organ cells co-expressed also SpBrn1/2/4 (Figure 2F”). These data reveal that in early larvae there are scattered ciliary band neurons that express pre-pancreatic transcription factors, like SpNgn, SpIsl and SpNeuroD.
A Pancreatic Signature Is Turned on in a Subset of Neuronal Precursors
We aimed to understand whether a pre-pancreatic regulatory state was active in neuronal precursor cells. We first tested whether SpLox was active in progenitors that express SpSoxC, an early marker of neuronal development (50, 51). SpSoxC was already expressed in mid gastrula before SpLox is expressed in the ectoderm (Figure 3A). SpLox appeared in late gastrula stage in SpSoxC neural precursors of the apical plate and ciliary band (Figure 3B, yellow arrowheads). Additionally, the SpLox cells in the foregut also expressed SpSoxC (Figure 3B, white arrowheads). We used SpBrn1/2/4 as a second marker for neuronal precursors. Besides the known co-expression of SpLox and SpBrn1/2/4 in the ciliary band (25), we found that from gastrula stage SpLox and SpBrn1/2/4 co-expressed in at least two cells of the apical plate (Figure 3C).
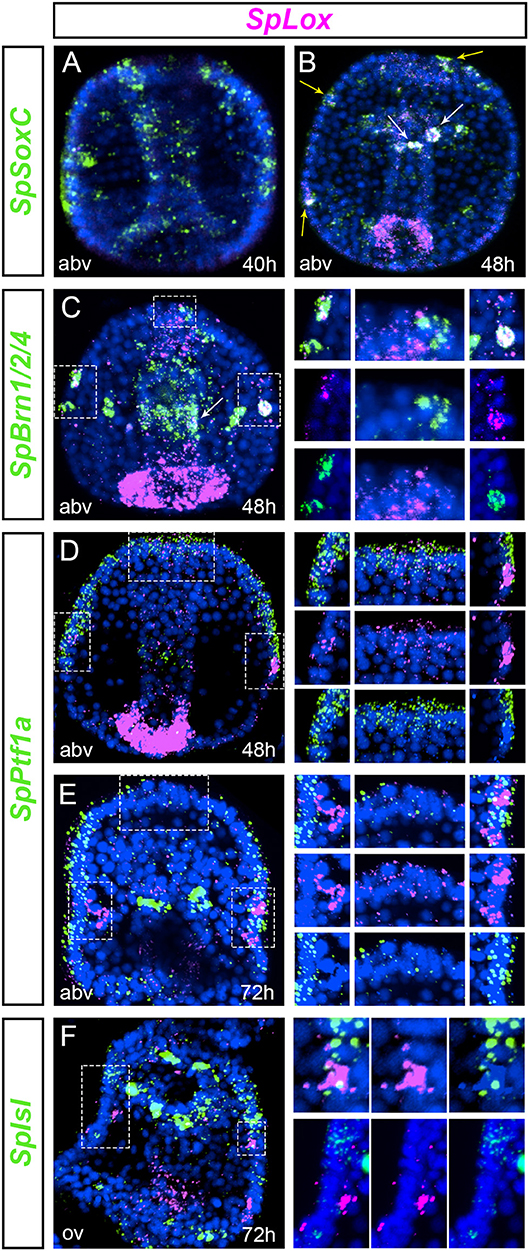
Figure 3. Cells with a pre-pancreatic regulatory state. (A,B) double FISH of SpLox and the proneural gene SpSoxC in middle to late gastrula. White arrowheads show SpLox co-localization with SpSoxC in the foregut, yellow arrowheads mark neurons in the apical plate and ciliary band that co-express SpSoxC and SpLox. (C) double FISH of SpLox and SpBrn1/2/4 in mid gastrula. Insets on the right are magnifications of (Continued)
Next, we tested which other pancreatic genes were expressed in the SpLox neurons. In vertebrates, Ptf1a is expressed early in pancreas development in the same cells that express the Pdx1 gene, the ortholog of SpLox in vertebrates (52). We previously found that ectodermal cells transiently expressed SpPtf1a until gastrula stage (53). We therefore tested if the SpLox ectodermal cells expressed also SpPtf1a. Double in situ hybridization revealed that cells in the apical plate and in the left and right lateral ganglion expressed both SpLox and SpPtf1a (Figures 3D,E). In particular, only in the left lateral ganglion precursors SpPtf1a expression was high in the adjacent cells and weak in the SpLox cells (Figure 3E). These same left lateral ganglion precursors also expressed SpIsl (Figure 3F). As for SpPtf1a, we noticed that SpIsl expression was higher in adjacent cells rather than in the SpLox neurons themselves (Figure 3F, insets on the right). Conversely, we found that SpLox neurons did not express SpNgn or SpNeuroD (data not shown).
Altogether, we found that apical organ, foregut and ciliary band neurons express SpLox after SpSoxC is activated. Apical plate and ciliary band cells express also SpPtf1a until gastrula stage. In the early larva at least two neurons are SpLox+SpBrn1/2/4+SpIsl+, likely representing a novel specialized neuroendocrine cell. Our results show that the left and right lateral ganglion neurons do not possess exactly the same molecular signature, suggesting separate functions.
The “Pre-pancreatic” Neurons Produce the Neuropeptide SpANP2
In order to identify terminal differentiation genes of the SpLox neurons, we looked at the expression of the sea urchin neuropeptides described by Woods et al. and Rowe et al. (54). In particular, SpAN expression pattern resembled that of SpLox+SpBrn1/2/4+ neurons. To better understand the nature of the SpAN expressing cells, we developed an antibody to SpANP2 protein and performed double immunostaining with anti-SynB antibody (gene name SpAN, protein name SpANP2). In larvae, SpANP2 localized in three to four apical organ neurons, lateral ganglia and postoral neurons (Figures 4A,B). The SpANP2+ apical organ neurons were serotoninergic and expressed also SpBrn1/2/4 (Supplementary Figure 1). Besides the ectodermal expression, SpANP2 was localized in the coelomic pouches (Figures 4A”,B”). To test whether SpANP2 immunofluorescence marked cells that produced the neuropeptide, and not target cells, we double stained larvae for SpAN mRNA and SpANP2 protein. We found that in early larvae the neuropeptide expression recapitulated the expression of the RNA transcripts (Figure 4C). In particular, while the mRNA was localized throughout the cells, the protein accumulated at the cell apical side (Figure 4C, white dashed line box), suggesting SpANP2 is secreted in vesicles. The same mRNA and protein expression was present also in late larvae (Figure 4D). We also found that SpAN neurons in the apical organ and ciliary band were secretory because they expressed SpMist, known marker of exocrine cells (55) (Figure 4E).
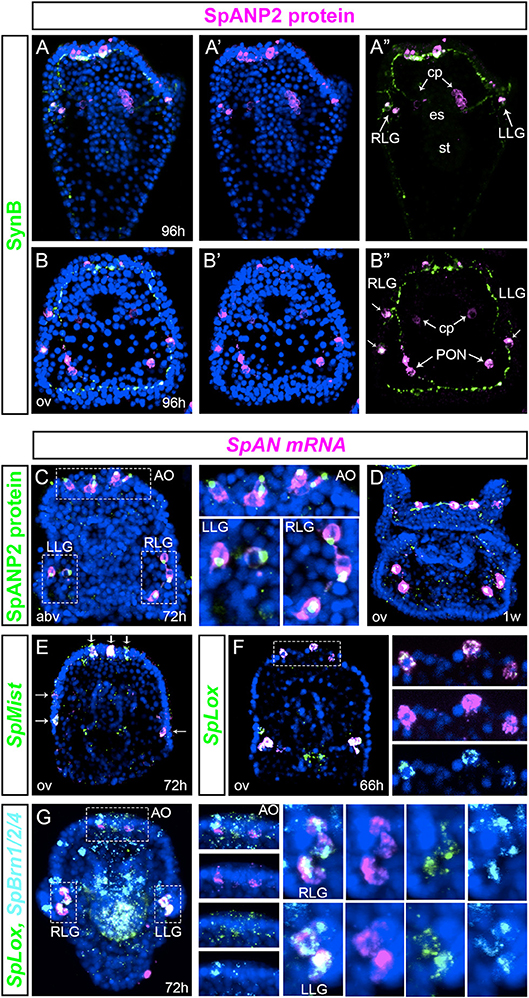
Figure 4. Transcript expression and protein localization of the neuropeptide SpANP2. (A,B)” SpANP2 (magenta) and SynB (green) immunolocalization in late larvae. (A–A)” is a frontal view, (B–B)” is a dorsal view. (C,D) SpANP2 transcripts (FISH, in magenta) and protein (immunofluorescence, in green) localization in early larva (C) and in 1-week old larva (D). White dashed line boxes in (C) mark the apical organ region, the left and the right lateral ganglia that are magnified on the right. Note that the protein accumulates close to the neuritis extension. (E) Double FISH of SpAN (magenta) with SpMist (green) showing that cells producing SpAN mRNA are secretory endocrine cells. White arrows indicate co-expression. (F) Double FISH of SpAN (magenta) and SpLox (green) at prism stage (66 h). White dashed line box marks the apical organ region. Insets on the right show three distinct cells where SpLox and SpAN are co-expessed. (G) Triple FISH shows the expression pattern of SpBrn1/2/4 (cyan), SpLox (green), and Sppnp5 (magenta). Insets on the right show single channels for each gene. The 72 h pluteus is oriented abanal along the A/V axis. Pictures are all full projection of merged confocal stacks; nuclei are labeled blue with DAPI. AO, apical organ; cp, coelomic pouches; es, esophagus; LLG, left lateral ganglion; PON, post-oral neurons; st, stomach; RLG, right lateral ganglion; abv, aboral view); ov (oral view).
We next tested whether SpLox neurons expressed SpAN. We found that the SpLox apical organ and lateral ganglion neurons expressed SpAN (Figures 4F,G). To further test that those were the SpLox+SpBrn1/2/4 neurons we performed a triple FISH experiment and confirmed that SpAN was expressed in all the SpLox+SpBrn1/2/4 neurons (Figure 4G and insets on the right).
Taken together, our results revealed that SpLox marks secretory pre-pancreatic apical organ and lateral ganglion neurons that produce and secrete the neuropeptide SpANP2. Only the SpLox neurons in the foregut did not produce the SpANP2 neuropeptide.
SpLox Controls SpAN Expression
Since SpLox neurons expressed also SpAN, we asked whether SpLox regulates SpAN gene expression. We used a morpholino approach to knock-down SpLox and quantified the number of neurons that expressed SpAN in larval stages. As previously published (37, 38), SpLox morphants are distinguished by a straight gut that lacks the pyloric sphincter. We found that in SpLox MO injected embryos there was an overall significant reduction of SpAN expression. For instance, control larvae had 3–4 SpAN+ apical organ neurons, while SpLox morphants had zero or only 1 SpAN+ apical organ neurons (Figures 5A,B). Similarly, SpLox morphants had fewer SpAN+ cells than control larvae on both the left and right sides (Figures 5A,C,D). We also observed a consistent number of larvae where there was no SpAN expression in at least one of the ectodermal domains (Figures 5B,C,D graphs). These results suggest that SpLox regulates SpAN expression in the cells where they are co-expressed: the apical organ neurons, the left and right lateral ganglia.
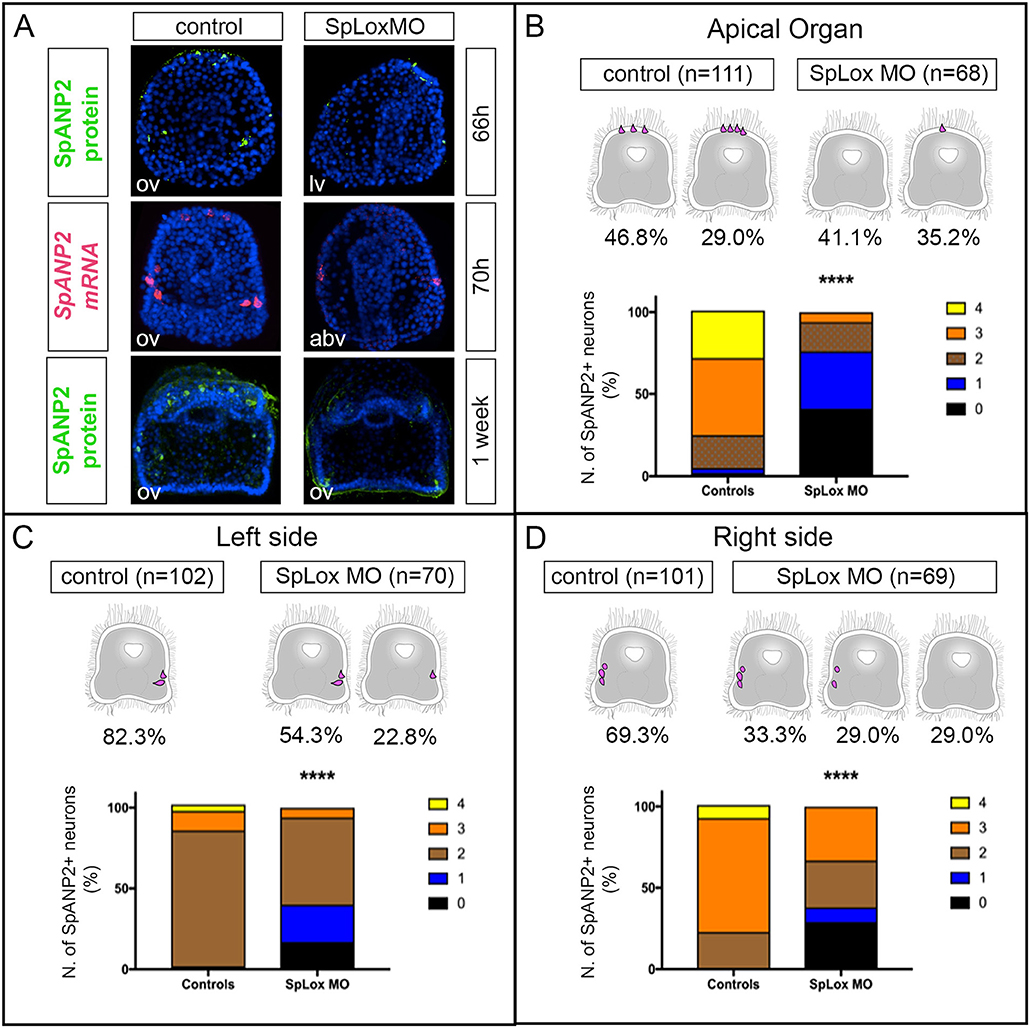
Figure 5. SpLox controls SpANP2 expression. (A) SpAN mRNA detected by single-color in situ hybridization or SpANP2 protein detected by immunofluorescence localization in controls and in larvae injected with SpLox MOs directed against the translation of SpLox RNA. Note that injected embryos/larvae show the typical SpLox MO phenotype of a straight gut that does not have the pyloric sphincter (38). All images are obtained as stacks of merged confocal Z sections. Nuclei are labeled blue with DAPI. (B–D) Quantification of the number of SpANP2 cells in the SpLox morphants shows a ****p < 0.0001 by Chi squared test. Cartoons of early larvae on top of the graphs summarize the most frequent phenotypes. In the graph we put together data form SpANP2+ cells at prism stage (66 h), early larvae (70 h), and 1-week old larvae. For (C,D) we show percentages of lateral ganglia and post-oral neurons together. abv, aboral view; lv, lateral view; ov, oral view.
Discussion
SpLox Marks a New Population of Neurons With a “Pre-pancreatic” Signature
In this study we discovered a new heterogeneous population of sea urchin neurons that is marked by the ParaHox gene SpLox. It has been recently found that neuronal precursors sequentially express the transcription factors SoxB2, SoxC, and Brn1/2/4 before differentiating into neurons (34, 50, 56). Our data indicate that a pre-pancreatic regulatory state marked by SpLox is active in selected neuronal precursors (summary in Figure 6A cartoon). SpLox neurons specifically express neuronal genes that the vertebrate pancreas co-opted. These “pre-pancreatic” genes are SpSoxC, SpPtf1a, and SpBrn1/2/4. First, SpLox is turned on in neural precursors that are SpSoxC positive. The vertebrate ortholog of SpSoxC is also expressed in the pancreatic endocrine cells during development (57). Second, SpLox neural precursors in the apical plate and the ciliary band transiently express SpPtf1a. In vertebrates, the SpPtf1a ortholog gives rise to all pancreatic progenitors (58, 59). Third, SpLox neurons also express SpBrn1/2/4, another marker of neuronal precursors (50) that is also expressed in the developing pancreas (29). The regulatory state of the SpLox neurons is dynamic. For instance, SpPtf1a might activate a pre-pancreatic program, but it is not necessary for maintaining it, while SpBrn1/2/4 remains on until larval stages.
It is intriguing that the SpLox neurons have a similar molecular signature but a distinct spatial distribution. One hypothesis is that perhaps the apical organ neurons represent the brain component of a pancreatic circuit, while the lateral ganglia are the peripheral component. It is known that the brain is an important target of the insulin that is produced by the pancreatic endocrine β-cells (60). The sea urchin has a tyrosine kinase receptor (SpInsr) that is ortholog of the vertebrate insulin receptor (INSR) and the insulin-like growth factor 1 receptor (IGF1R). An interesting comparison is that just as INSR and IGF1R are expressed in the vertebrate brain (61), SpInsr is also expressed in the apical organ of sea urchin larvae (24). This expression pattern suggests that apical organ neurons could respond to endocrine signals from the gut as the vertebrate brain does. The most significant difference between the two SpLox neuronal populations is that the apical organ neurons are serotoninergic (62), while lateral ganglia are dopaminergic (63). The relationship between these two types of neurons that have a similar signature but express also different neurotransmitters merits further investigation.
Sea Urchin Neurons Are a Heterogeneous Population
SpBrn1/2/4 has been shown to be a neuronal marker (50). In this study, we found that subsets of SpBrn1/2/4 neurons express distinct genes. For instance, SpBrn1/2/4 ciliary band neurons express SpNgn, while the apical neurons do not always express SpNgn. In the sea urchin L. variegatus, LvNgn has been shown to have a similar expression pattern to SpNgn (64), but no co-expression with other transcription factors is known. SpBrn1/2/4 neurons also express SpNeuroD and SpIsl in scattered cells. As it has been previously shown, waves of transcription factors are transiently expressed in neurogenesis (34, 50, 64). Therefore, one possibility is that cells lacking SpBrn1/2/4 expression at a given stage might express it a few hours later. Lateral ganglia appear like symmetrical neurons localized on the left and right side of the sea urchin larva. Despite this anatomical symmetry, these neurons do not express exactly the same genes. For instance, only the SpLox left lateral ganglion neurons express also SpIsl. The facts that not all the lateral ganglion neurons share the same molecular signature, and that right and left lateral ganglion neurons are not symmetrical, emphasizes the complexity and diversity of neuronal types in sea urchins (summarized in Figure 6A). Altogether, our findings demonstrate that in the sea urchin larva there is a huge diversity of neuronal subtypes that has not been completely characterized.
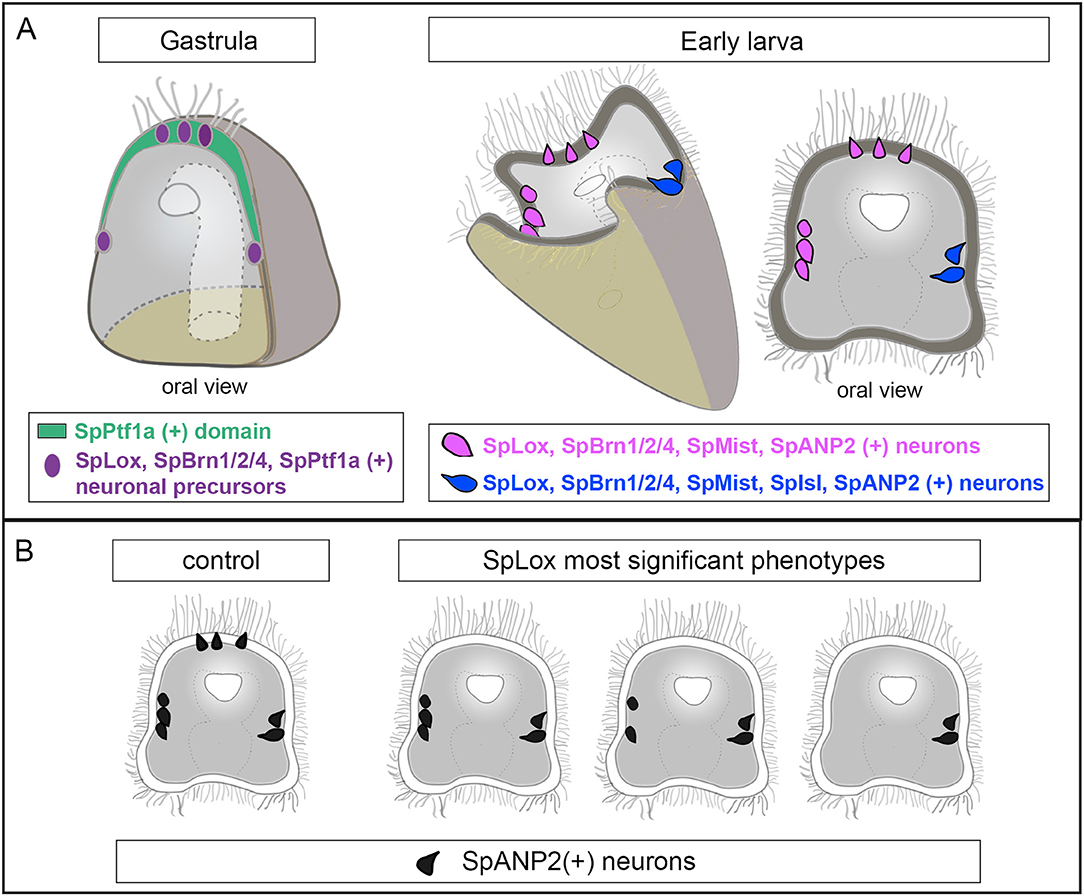
Figure 6. Summary of the regulatory state of the SpLox+ neurons. (A) Schematic representation of a sea urchin gastrula (left) and early larva (right) showing the neurons identified in this study. Neurons with the same pancreatic signature have the same color. (B) Cartoon showing decrease in SpANP2+ neurons in SpLox morphants. The three most frequent phenotypes are shown.
SpLox Regulates the Expression of SpANP2
We found that the novel echinoderm neuropeptide SpANP2 (Wood et al. 2018) (54) is expressed in several neurons, including the SpLox “pre-pancreatic” neurons. SpANP2 is expressed in the adult S. purpuratus nerve cords (65), but so far no sequence similarities with neuropeptides from other phyla have been identified (54). Its cellular localization suggests that SpANP2 is released in vesicles, in line with its role as a neurohormone. The fact that SpANP2 is expressed not only in SpLox neurons, but also in other cells, leads to two possible hypotheses. First, neurons with different molecular signatures could use the same mechanisms to regulate SpANP2 expression. Alternatively, different regulatory networks could control SpANP2 expression in distinct cells. In SpLox knocked down larvae, SpANP2 transcripts and protein were significantly reduced from the apical organ and in the peripheral neurons, but not all neurons were equally affected (a summary of these data is reported in Figure 6B). Therefore, our perturbation data suggest that SpLox is a general regulator of SpANP expression, but different gene regulatory networks could control SpANP expression in specific domains.
Conclusions
Neurons and pancreatic β-cells share many remarkable features, including similar gene expression, function and physiology (1–7). It has been shown that human neural progenitors can be induced to differentiate into pancreatic cells (66), suggesting that these two cell types use a very similar gene regulatory network. Previous authors (15, 67) have discussed the idea that a multipotent pancreatic progenitor co-opted a neural genetic program. Insulin-like peptides are expressed in the nervous system and the gut of non-vertebrate chordate, like echinoderms, tunicates and cephalochordates (17, 24, 68). These findings support the idea that pancreatic cells co-opted a neural genetic program. Our results expand this idea and suggest that pancreatic cells co-opted a neuronal program from a distinct neuronal subtype, rather than a generic one. Hence, we provide additional evidence that neurons with a pancreatic signature pre-dated the appearance of the vertebrate pancreatic regulatory state. Therefore, we propose that gut cells co-opted a pre-existing pre-pancreatic program from ancestral neurons already present in a deuterostome ancestor.
Materials and Methods
Animals
Adult Strongylocentrotus purpuratus were obtained from Patrick Leahy (Kerchoff Marine Laboratory, California Institute of Technology, Pasadena, CA, USA) and housed in circulating seawater aquaria at the Stazione Zoologica Anton Dohrn of Naples. Gametes were obtained by vigorous shaking of animals or by intracoelomic injection of 0.5 M KCl. Embryos were cultured at 15°C in filtered Mediterranean sea water diluted 9:1 with de-ionized water.
RNA Whole Mount in situ Hybridization
For fluorescent whole mount in situ hybridization (FISH), we followed the protocol outlined in (69). Triple FISH was performed as described in (70). Signal was developed with fluorophore-conjugated tyramide (1:400 reagent diluents, Perkin Elmer). Labeled probes were transcribed from linearized DNA using digoxigenin-11-UTP, fluorescein-12-UTP (Roche, Indianapolis, IN, USA), or labeled with DNP (Mirus, Madison, WI, USA) following kit instructions. SpLox, SpBrn1/2/4, SpSoxC, SpPtf1a, and SpMist probes were made as previously published [SpLox (71), SpBrn1/2/4 (25), SpSoxC (69) SpPtf1a, and SpMist (53)]. SpIsl, SpNgn and SpNeuroD probes were synthetized using the following primers: SpIsl-F: 5′-CGTGGACCAGACAGACTTGA-3′; SpIsl-R: 5′-AGTCGCTGAGTGCTTTCCAT-3′; SpNgn-F: 5′-TACGACAATGATGCCCAAGA-3′; SpNgn-R: 5′-CCGTTTCACAAAGCCATTTT-3′; SpNeuroD-F: 5′-CTCGCCACCTGATCTCTAC-3′; SpNeuroD-R: 5′-TTCCCGCCTTTCAAAATATG-3′. SpANP2 probe was made as published in Woods et al. 2018. Templates of all the probes were sequenced prior to probe generation and cloned in the pGEM®-T Easy Vector (Promega, Madison, WI, USA). Samples were imaged with a Zeiss 510 Meta confocal microscope.
Immunochemistry
Larvae were fixed in 4% PFA in FSW for 15 min at room temperature, washed multiple times in phosphate-buffered saline with 0.1% Tween-20 (PBST), and incubated overnight at 4°C with either the SpLox antibody (1:500) or the SpANP2 antibody (1:250) in 1 mg/ml BSA and 4% sheep serum in PBST. To mark the nervous system, the anti-Syn antibody (1:100) was added (39). Larvae were then washed three times with PBST and incubated for 1 h at room temperature with the secondary antibodies anti-rabbit-AlexaFluor 555 (Invitrogen) or anti-mouse 488 (Invitrogen) diluted 1/100 in 1 mg/ml BSA in PBST. After being washed in PBST, larvae were mounted for imaging with a confocal microscope (Zeiss 510Meta).
Perturbation Experiments With MO Injection
Translation-blocking antisense MO against SpLox was used at a concentration of 2 mM as published in (37) and (38). For each experiment 300 eggs were injected with ~2–4 pl of oligonucleotide injection solution. Each experiment was repeated at least three times. Note that the SpLox morpholino exhibits an unique phenotype that lacks the pyloric sphincter, as it has been previously shown (38). As a negative control, fertilized eggs were injected with the standard control morpholino (GeneTools, Pilomath, OR) and compared side-by-side with knockdown embryos.
Author Contributions
MP conception and design, acquisition of data, analysis and interpretation of data, drafting and revising the article, PP acquisition of data; analysis and interpretation of data, TM and MC: acquisition of data, PO analysis and interpretation of data, revising the article, contributed unpublished essential reagents, MA conception and design, analysis and interpretation of data, drafting or revising the article.
Conflict of Interest Statement
The authors declare that the research was conducted in the absence of any commercial or financial relationships that could be construed as a potential conflict of interest.
Acknowledgments
We would like to thank Pat Leahy (KML, Caltech, Pasadena, CA) for providing adult S. purpuratus, Giovanna Benvenuto (SZN) for microscopy assistance and Davide Caramiello (SZN) for animal maintenance. We are thankful to Robert Burke for teaching us - and the whole echinodern community- everything we know on the sea urchin neurvous system and for sharing antibodies. MP would like to thank Zak Swartz for stimulating discussions and paper revisions. This work was supported by MIUR (premiale PANTRAC) to MA. MP has been supported by a SZN PhD fellowship and by a fellowship from POR Campania FSE 2007–2013 Project-MODO, Model Organism. TM work was supported by FEBS short term fellowship. PP has been supported by the Marie Curie ITN EvoCELL (grant number: 766053, H2020 PEOPLE Work Programme of the European Commission, PI: MIA).
Supplementary Material
The Supplementary Material for this article can be found online at: https://www.frontiersin.org/articles/10.3389/fendo.2018.00650/full#supplementary-material
Supplementary Figure 1. SpANP2 is localized in serotoninergic apical organ neurons. Serotonin, Synaptotagmin B and SpANP2 immunofluorescence. The picture is a full projection of merged confocal stacks. Nuclei are stained with DAPI and depicted in blue.
Supplementary Table 1. Summary of the genes discussed in the present paper.
References
1. Pearse AGE, Polak JM. Neural crest origin of the endocrine polypeptide (APUD) cells of the gastrointestinal tract and pancreas. Gut (1971) 12:783–8. doi: 10.1136/gut.12.10.783
2. Fujita T, Kobayashi S, Yui R. Paraneuron concept and its current implications. Adv Biochem Psychopharmacol. (1980) 25:321–5.
3. Maechler P, Wollheim CB. Mitochondrial glutamate acts as a messenger in glucose-induced insulin exocytosis. Nature (1999) 402:685–9. doi: 10.1038/45280
4. Reetz A, Solimena M, Matteoli M, Folli F, Takei K, De Camilli P. GABA and pancreatic beta-cells: colocalization of glutamic acid decarboxylase (GAD) and GABA with synaptic-like microvesicles suggests their role in GABA storage and secretion. EMBO J. (1991) 10:1275–84. doi: 10.1002/j.1460-2075.1991.tb08069.x
5. van Arensbergen J, García-Hurtado J, Moran I, Maestro MA, Xu X, Van de Casteele M, et al. Derepression of Polycomb targets during pancreatic organogenesis allows insulin-producing beta-cells to adopt a neural gene activity program. Genome Res. (2010) 20:722–32. doi: 10.1101/gr.101709.109
6. Le Douarin NM. On the origin of pancreatic endocrine cells. Cell (1988) 53:169–71. doi: 10.1016/0092-8674(88)90375-3
7. Alpert S, Hanahan D, Teitelman G. Hybrid insulin genes reveal a developmental lineage for pancreatic endocrine cells and imply a relationship with neurons. Cell (1988) 53:295–308. doi: 10.1016/0092-8674(88)90391-1
8. Gonoi T, Mizuno N, Inagaki N, Kuromi H, Seino Y, Miyazaki J, et al. Functional neuronal ionotropic glutamate receptors are expressed in the non-neuronal cell line MIN6. J Biol Chem. (1994) 269:16989–92.
9. Thor S, Ericson J, Brännström T, Edlund T. The homeodomain LIM protein Isl-1 is expressed in subsets of neurons and endocrine cells in the adult rat. Neuron (1991) 7:881–9.
10. Jensen J. Gene regulatory factors in pancreatic development. Dev Dyn. (2004) 229:176–200. doi: 10.1002/dvdy.10460
11. Arda HE, Benitez CM, Kim SK. Gene regulatory networks governing pancreas development. Dev Cell (2013) 25:5–13. doi: 10.1016/j.devcel.2013.03.016
12. Schuurmans C, Guillemot F. Molecular mechanisms underlying cell fate specification in the developing telencephalon. Curr Opin Neurobiol. (2002) 12:26–34. doi: 10.1016/S0959-4388(02)00286-6
13. Afelik S, Chen Y, Pieler T. Combined ectopic expression of Pdx1 and Ptf1a/p48 results in the stable conversion of posterior endoderm into endocrine and exocrine pancreatic tissue. Genes Dev. (2006) 20:1441–6. doi: 10.1101/gad.378706
14. Song J, Xu Y, Hu X, Choi B, Tong Q. Brain expression of Cre recombinase driven by pancreas-specific promoters. Genesis (2010) 48:628–34. doi: 10.1002/dvg.20672
15. Arntfield ME, van der Kooy D. β-Cell evolution: how the pancreas borrowed from the brain. Bioessays (2011) 33:582–7. doi: 10.1002/bies.201100015
17. Lecroisey C, Le Pétillon Y, Escriva H, Lammert E, Laudet V. Identification, evolution and expression of an insulin-like peptide in the cephalochordate Branchiostoma lanceolatum. PLoS ONE (2015) 10:e0119461. doi: 10.1371/journal.pone.0119461
18. Holland LZ, Albalat R, Azumi K, Benito-Gutiérrez E, Blow MJ, Bronner-Fraser M, et al. The amphioxus genome illuminates vertebrate origins and cephalochordate biology. Genome Res (2008) 18:1100–11. doi: 10.1101/gr.073676.107
19. Galloway SM, Cutfield JF. Insulin-like material from the digestive tract of the tunicate Pyura pachydermatina (sea tulip). Gen Comp Endocrinol. (1988) 69:106–13. doi: 10.1016/0016-6480(88)90058-5
20. Reinecke M, Collet C. The phylogeny of the insulin-like growth factors. Int Rev Cytol. (1998) 183:1–94. doi: 10.1016/S0074-7696(08)60142-4
21. Olinski RP, Dahlberg C, Thorndyke M, Hallböök F. Three insulin-relaxin-like genes in Ciona intestinalis. Peptides (2006) 27:2535–46. doi: 10.1016/j.peptides.2006.06.008
22. Annunziata R, Perillo M, Andrikou C, Cole AG, Martinez P, Arnone MI. Pattern and process during sea urchin gut morphogenesis: the regulatory landscape. Genesis (2014) 52:251–68. doi: 10.1002/dvg.22738
23. Lowe EK, Cuomo C, Arnone MI. Omics approaches to study gene regulatory networks for development in echinoderms. Brief Funct Genomics (2017) 16:299–308. doi: 10.1093/bfgp/elx012
24. Perillo M, Arnone MI. Characterization of insulin-like peptides (ILPs) in the sea urchin Strongylocentrotus purpuratus: insights on the evolution of the insulin family. Gen Comp Endocrinol. (2014) 205:68–79. doi: 10.1016/j.ygcen.2014.06.014
25. Cole AG, Arnone MI. Fluorescent in situ hybridization reveals multiple expression domains for SpBrn1/2/4 and identifies a unique ectodermal cell type that co-expresses the ParaHox gene SpLox. Gene Expr Patterns (2009) 9:324–8. doi: 10.1016/j.gep.2009.02.005
26. Oliver-Krasinski JM, Stoffers DA. On the origin of the β cell. Genes Dev. (2008) 22:1998–2021. doi: 10.1101/gad.1670808
27. Perez-Villamil B, Schwartz PT, Vallejo M. The pancreatic homeodomain transcription factor IDX1/IPF1 is expressed in neural cells during brain development. Endocrinology (1999) 140:3857–60. doi: 10.1210/endo.140.8.7048
28. Howard-Ashby M, Materna SC, Brown CT, Chen L, Cameron RA, Davidson EH. Identification and characterization of homeobox transcription factor genes in Strongylocentrotus purpuratus, and their expression in embryonic development. Dev Biol. (2006) 300:74–89. doi: 10.1016/j.ydbio.2006.08.039
29. Hussain MA, Miller CP, Habener JF. Brn-4 transcription factor expression targeted to the early developing mouse pancreas induces ectopic glucagon gene expression in insulin-producing beta cells. J Biol Chem. (2002) 277:16028–32. doi: 10.1074/jbc.M107124200
30. He X, Treacy MN, Simmons DM, Ingraham HA, Swanson LW, Rosenfeld MG. Expression of a large family of POU-domain regulatory genes in mammalian brain development. Nature (1989) 340:35–41. doi: 10.1038/340035a0
31. Suzuki N, Rohdewohld H, Neuman T, Gruss P, Schöler HR. Oct-6: a POU transcription factor expressed in embryonal stem cells and in the developing brain. EMBO J. (1990) 9:3723–32.
32. Burke RD, Angerer LM, Elphick MR, Humphrey GW, Yaguchi S, Kiyama T, et al. A genomic view of the sea urchin nervous system. Dev Biol. (2006) 300:434–60. doi: 10.1016/j.ydbio.2006.08.007
33. Hinman VF, Burke RD. Embryonic neurogenesis in echinoderms. Wiley Interdiscip Rev Dev Biol. (2018) 7:e316. doi: 10.1002/wdev.316
34. Wei Z, Angerer LM, Angerer RC. Neurogenic gene regulatory pathways in the sea urchin embryo. Development (2016) 143:298–305. doi: 10.1242/dev.125989
35. Mellott DO, Thisdelle J, Burke RD. Notch signaling patterns neurogenic ectoderm and regulates the asymmetric division of neural progenitors in sea urchin embryos. Development (2017) 144:3602–11. doi: 10.1242/dev.151720
36. Burke RD, Moller DJ, Krupke OA, Taylor VJ. Sea urchin neural development and the metazoan paradigm of neurogenesis. Genesis (2014) 52:208–21. doi: 10.1002/dvg.22750
37. Cole AG, Rizzo F, Martinez P, Fernandez-Serra M, Arnone MI. Two ParaHox genes, SpLox and SpCdx, interact to partition the posterior endoderm in the formation of a functional gut. Development (2009) 136:541–9. doi: 10.1242/dev.029959
38. Annunziata R, Arnone MI. A dynamic regulatory network explains ParaHox gene control of gut patterning in the sea urchin. Development (2014) 141:2462–72. doi: 10.1242/dev.105775
39. Burke RD, Osborne L, Wang D, Murabe N, Yaguchi S, Nakajima Y. Neuron-specific expression of a synaptotagmin gene in the sea urchin Strongylocentrotus purpuratus. J Comp Neurol. (2006) 496:244–51. doi: 10.1002/cne.20939
40. Apelqvist A, Li H, Sommer L, Beatus P, Anderson DJ, Honjo T, et al. Notch signalling controls pancreatic cell differentiation. Nature (1999) 400:877–81. doi: 10.1038/23716
41. Gradwohl G, Dierich A, LeMeur M, Guillemot F. neurogenin3 is required for the development of the four endocrine cell lineages of the pancreas. Proc Natl Acad Sci USA. (2000) 97:1607–11. doi: 10.1073/pnas.97.4.1607
42. Ma Q, Kintner C, Anderson DJ. Identification of neurogenin, a vertebrate neuronal determination gene. Cell (1996) 87:43–52. doi: 10.1016/S0092-8674(00)81321-5
43. Quiñones HI, Savage TK, Battiste J, Johnson JE. Neurogenin 1 (Neurog1) expression in the ventral neural tube is mediated by a distinct enhancer and preferentially marks ventral interneuron lineages. Dev Biol. (2010) 340:283–92. doi: 10.1016/j.ydbio.2010.02.012
44. Dykes IM, Tempest L, Lee S-I, Turner EE. Brn3a and Islet1 act epistatically to regulate the gene expression program of sensory differentiation. J Neurosci. (2011) 31:9789–99. doi: 10.1523/JNEUROSCI.0901-11.2011
45. Gu C, Stein GH, Pan N, Goebbels S, Hörnberg H, Nave K-A, et al. Pancreatic beta cells require NeuroD to achieve and maintain functional maturity. Cell Metab. (2010) 11:298–310. doi: 10.1016/j.cmet.2010.03.006
46. Gu G, Dubauskaite J, Melton DA. Direct evidence for the pancreatic lineage: NGN3+ cells are islet progenitors and are distinct from duct progenitors. Development (2002) 129:2447–57.
47. Lee JK, Cho JH, Hwang WS, Lee YD, Reu DS, Suh-Kim H. Expression of neuroD/BETA2 in mitotic and postmitotic neuronal cells during the development of nervous system. Dev Dyn. (2000) 217:361–7. doi: 10.1002/(SICI)1097-0177(200004)217:4<361::AID-DVDY3>3.0.CO;2-8
48. Naya FJ, Huang HP, Qiu Y, Mutoh H, DeMayo FJ, Leiter AB, et al. Diabetes, defective pancreatic morphogenesis, and abnormal enteroendocrine differentiation in BETA2/neuroD-deficient mice. Genes Dev. (1997) 11:2323–34. doi: 10.1101/gad.11.18.2323
49. Naya FJ, Stellrecht CM, Tsai MJ. Tissue-specific regulation of the insulin gene by a novel basic helix-loop-helix transcription factor. Genes Dev. (1995) 9:1009–19. doi: 10.1101/gad.9.8.1009
50. Garner S, Zysk I, Byrne G, Kramer M, Moller D, Taylor V, et al. Neurogenesis in sea urchin embryos and the diversity of deuterostome neurogenic mechanisms. Development (2016) 143:286–97. doi: 10.1242/dev.124503
51. Howard-Ashby M, Materna SC, Brown CT, Chen L, Cameron RA, Davidson EH. Gene families encoding transcription factors expressed in early development of Strongylocentrotus purpuratus. Dev Biol. (2006) 300:90–107. doi: 10.1016/j.ydbio.2006.08.033
52. Zhou Q, Law AC, Rajagopal J, Anderson WJ, Gray PA, Melton DA. A multipotent progenitor domain guides pancreatic organogenesis. Dev Cell (2007) 13:103–14. doi: 10.1016/j.devcel.2007.06.001
53. Perillo M, Wang YJ, Leach SD, Arnone MI. A pancreatic exocrine-like cell regulatory circuit operating in the upper stomach of the sea urchin Strongylocentrotus purpuratus larva. BMC Evol Biol. (2016) 16:117. doi: 10.1186/s12862-016-0686-0
54. Rowe ML, Elphick MR. The neuropeptide transcriptome of a model echinoderm, the sea urchin Strongylocentrotus purpuratus. Gen Comp Endocrinol. (2012) 179:331–44. doi: 10.1016/j.ygcen.2012.09.009
55. Pin CL, Lemercier C, Konieczny SF. Cloning of the murine Mist1 gene and assignment to mouse chromosome band 5G2-5G3. Cytogenet Cell Genet. (1999) 86:219–22. doi: 10.1159/000015342
56. Anishchenko E, Arnone MI, D'Aniello S. SoxB2 in sea urchin development: implications in neurogenesis, ciliogenesis and skeletal patterning. Evodevo (2018) 9:5. doi: 10.1186/s13227-018-0094-1
57. Lioubinski O, Müller M, Wegner M, Sander M. Expression of Sox transcription factors in the developing mouse pancreas. Dev Dyn. (2003) 227:402–8. doi: 10.1002/dvdy.10311
58. Kawaguchi Y, Cooper B, Gannon M, Ray M, MacDonald RJ, Wright CVE. The role of the transcriptional regulator Ptf1a in converting intestinal to pancreatic progenitors. Nat Genet. (2002) 32:128–34. doi: 10.1038/ng959
59. Thompson N, Gésina E, Scheinert P, Bucher P, Grapin-Botton A. RNA profiling and chromatin immunoprecipitation-sequencing reveal that PTF1a stabilizes pancreas progenitor identity via the control of MNX1/HLXB9 and a network of other transcription factors. Mol Cell Biol. (2012) 32:1189–99. doi: 10.1128/MCB.06318-11
60. Gray SM, Meijer RI, Barrett EJ. Insulin regulates brain function, but how does it get there? Diabetes (2014) 63:3992–7. doi: 10.2337/db14-0340
61. Plum L, Schubert M, Brüning JC. The role of insulin receptor signaling in the brain. Trends Endocrinol Metab. (2005) 16:59–65. doi: 10.1016/j.tem.2005.01.008
62. Yaguchi S, Katow H. Expression of tryptophan 5-hydroxylase gene during sea urchin neurogenesis and role of serotonergic nervous system in larval behavior. J Comp Neurol. (2003) 466:219–29. doi: 10.1002/cne.10865
63. Bisgrove BW, Burke RD. Development of the nervous system of the pluteus larva of Strongylocentrotus droebachiensis. Cell Tissue Res. (1987) 248:335–43. doi: 10.1007/BF00218200
64. Slota LA, McClay DR. Identification of neural transcription factors required for the differentiation of three neuronal subtypes in the sea urchin embryo. Dev Biol. (2018) 435:138–49. doi: 10.1016/j.ydbio.2017.12.015
65. Menschaert G, Vandekerckhove TTM, Baggerman G, Landuyt B, Sweedler JV, Schoofs L, et al. A hybrid, de novo based, genome-wide database search approach applied to the sea urchin neuropeptidome. J Proteome Res. (2010) 9:990–6. doi: 10.1021/pr900885k
66. Hori Y, Gu X, Xie X, Kim SK. Differentiation of insulin-producing cells from human neural progenitor cells. PLoS Med. (2005) 2:e103. doi: 10.1371/journal.pmed.0020103
67. Eberhard D. Neuron and beta-cell evolution: learning about neurons is learning about beta-cells. Bioessays (2013) 35:584. doi: 10.1002/bies.201300035
68. Thompson JM, Di Gregorio A. Insulin-like genes in ascidians: findings in Ciona and hypotheses on the evolutionary origins of the pancreas. Genesis (2015) 53:82–104. doi: 10.1002/dvg.22832
69. Andrikou C, Iovene E, Rizzo F, Oliveri P, Arnone MI. Myogenesis in the sea urchin embryo: the molecular fingerprint of the myoblast precursors. Evodevo (2013) 4:33. doi: 10.1186/2041-9139-4-33
70. Andrikou C, Pai C-Y, Su Y-H, Arnone MI. Logics and properties of a genetic regulatory program that drives embryonic muscle development in an echinoderm. Elife (2015) 4:e07343. doi: 10.7554/eLife.07343
71. Arnone MI, Rizzo F, Annunciata R, Cameron RA, Peterson KJ, Martinez P. Genetic organization and embryonic expression of the ParaHox genes in the sea urchin S. purpuratus: insights into the relationship between clustering and colinearity. Dev Biol. (2006) 300:63–73. doi: 10.1016/j.ydbio.2006.07.037
72. Kim DK, Han SB, Hong ST, Choi YJ, Sun W, Geum D, et al. Expression of Sox11 and Brn transcription factors during development and following transient forebrain ischemia in the rat. Neurosci Lett. (2008) 433:259–64. doi: 10.1016/j.neulet.2008.01.016
73. Fode C, Gradwohl G, Morin X, Dierich A, LeMeur M, Goridis C, et al. The bHLH protein NEUROGENIN 2 is a determination factor for epibranchial placode-derived sensory neurons. Neuron (1998) 20:483–94. doi: 10.1016/S0896-6273(00)80989-7
74. Eng SR, Dykes IM, Lanier J, Fedtsova N, Turner EE. POU-domain factor Brn3a regulates both distinct and common programs of gene expression in the spinal and trigeminal sensory ganglia. Neural Dev. (2007) 2:3. doi: 10.1186/1749-8104-2-3
75. Lin JW, Biankin AV, Horb ME, Ghosh B, Prasad NB, Yee NS, et al. Differential requirement for ptf1a in endocrine and exocrine lineages of developing zebrafish pancreas. Dev Biol. (2004) 270:474–86. doi: 10.1016/j.ydbio.2004.02.023
76. Phippard D, Boyd Y, Reed V, Fisher G, Masson WK, Evans EP, et al. The sex-linked fidget mutation abolishes Brn4/Pou3f4 gene expression in the embryonic inner ear. Hum Mol Genet. (2000) 9:79–85. doi: 10.1093/hmg/9.1.79
77. Lemercier C, To RQ, Swanson BJ, Lyons GE, Konieczny SF. Mist1: a novel basic helix-loop-helix transcription factor exhibits a developmentally regulated expression pattern. Dev Biol. (1997) 182:101–13. doi: 10.1006/dbio.1996.8454
78. Furlong RF, Graham A. Vertebrate neurogenin evolution: long-term maintenance of redundant duplicates. Dev Genes Evol. (2005) 215:639–44. doi: 10.1007/s00427-005-0023-x
79. Hoshino M, Nakamura S, Mori K, Kawauchi T, Terao M, Nishimura YV, et al. Ptf1a, a bHLH transcriptional gene, defines GABAergic neuronal fates in cerebellum. Neuron (2005) 47:201–13. doi: 10.1016/j.neuron.2005.06.007
80. Poy MN, Eliasson L, Krutzfeldt J, Kuwajima S, Ma X, Macdonald PE, et al. A pancreatic islet-specific microRNA regulates insulin secretion. Nature (2004) 432:226–30. doi: 10.1038/nature03076
81. Cheung M, Abu-Elmagd M, Clevers H, Scotting PJ. Roles of Sox4 in central nervous system development. Brain Res Mol Brain Res. (2000) 79:180–91. doi: 10.1016/S0169-328X(00)00109-1
82. Smith SB, Watada H, German MS. Neurogenin3 activates the islet differentiation program while repressing its own expression. Mol Endocrinol. (2004) 18:142–9. doi: 10.1210/me.2003-0037
Keywords: Brn, islet, neurogenin, NeuroD, neuropeptide, Ptf1a
Citation: Perillo M, Paganos P, Mattiello T, Cocurullo M, Oliveri P and Arnone MI (2018) New Neuronal Subtypes With a “Pre-Pancreatic” Signature in the Sea Urchin Stongylocentrotus purpuratus. Front. Endocrinol. 9:650. doi: 10.3389/fendo.2018.00650
Received: 06 July 2018; Accepted: 16 October 2018;
Published: 02 November 2018.
Edited by:
Dan Larhammar, Uppsala University, SwedenReviewed by:
Linda Z. Holland, University of California, San Diego, United StatesTsubasa Sakai, Suntory Foundation for Life Sciences, Japan
Copyright © 2018 Perillo, Paganos, Mattiello, Cocurullo, Oliveri and Arnone. This is an open-access article distributed under the terms of the Creative Commons Attribution License (CC BY). The use, distribution or reproduction in other forums is permitted, provided the original author(s) and the copyright owner(s) are credited and that the original publication in this journal is cited, in accordance with accepted academic practice. No use, distribution or reproduction is permitted which does not comply with these terms.
*Correspondence: Maria I. Arnone, bWlhcm5vbmVAc3puLml0