- Institute of Environmental and Sustainable Chemistry, Technische Universität Braunschweig, Braunschweig, Germany
The performance of primary and secondary electroactive biofilms grown on layered corrugated carbon (LCC) electrodes was studied over a period of several months. With an average projected current density of 6.7 mA cm−2, the studied secondary electroactive biofilms outperformed the primary biofilms (3.0 mA cm−2) over the entire experimental period. At the same time, both, primary and secondary biofilms, exhibited a constant Coulomb efficiency of about 89%. The study further illustrates that three-dimensional electrodes such as LCC allow a sustained long-term performance without significant decrease in electrode performance.
Introduction
The performance of bioelectrochemical systems (BES) in general and of microbial fuel cells (MFC) in particular has increased remarkably over the last decade (Schroeder, 2011; Oliveira et al., 2013). The defining characteristic of biofilms formed by electrochemically active bacteria is their ability to perform anode-respiration (Lovley, 2006; Logan, 2008; Torres et al., 2008). The activity of electroactive bacteria biofilms plays a major role in the context of this study. In the development of high-performance biofilm electrodes, two different approaches – a biological and a materials approach – have been followed. The biological approach is based on an improvement of the biofilm performance by means of sophisticated biofilm growth procedures. Therefore, electroactive biofilms can easily be grown using inoculi such as wastewater, soil, compost, or sediments (Liu et al., 2008; Cercado et al., 2013; Ketep et al., 2013), without any bacterial extraction steps. Using these inoculi, primary electroactive biofilms are produced, which already exhibit a good electrocatalytic activity. The bacteria of these biofilms can again serve as an inoculum for the generation of secondary biofilms (see Figure 1), which usually have a strongly enhanced electrocatalytic performance (Liu et al., 2008).
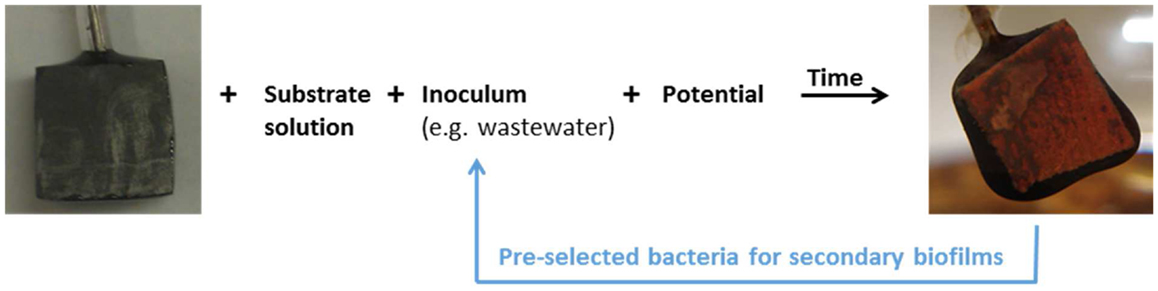
Figure 1. Schematic illustration of the electrochemical cultivation of primary and subsequent secondary electroactive biofilms.
A major materials approach is the surface modification (Cheng and Logan, 2007; Scott et al., 2007), which focuses on improving colonization potential for bacteria at the electrode surface. It has been suggested that surface modification can significantly reduce the formation time of an electroactive biofilm; however, the long-term performance of the biofilm remains unaffected by the surface treatment (Kumar et al., 2013). The second materials approach focuses on the development of tailored 3D electrode structures. With strongly increased surface area, available for the biofilm formation, these electrodes aim to increase biocatalyst density resulting in an increased electrocatalytic current. Numerous 3D structures (Wei et al., 2011) – based on graphite granule electrodes (Sell et al., 1989), carbon felt/paper electrodes (Srikanth et al., 2008; Liu et al., 2010), carbon brush electrodes (Cheng and Logan, 2007), carbon fibers (Liu et al., 2010), foams (Zhao et al., 2010), electrospun carbon fibers (Chen et al., 2011a,b; He et al., 2011), or natural templates like carbonized plant stems (Chen et al., 2012a) – have been proposed. With layered corrugated carbon (LCC), a product from the carbonization of corrugated cardboard, Chen et al. (2012b) proposed a promising 3D structure, providing surface area for biofilm growth and sufficiently large channels for substrate supply and product removal. With a projected current density of about 7 mA cm−2 per layer, this stackable electrode material outperformed previous 3D electrode structures.
Due to limited lab capacities, most authors (including ourselves) focus their experimental studies on relatively short time spans, i.e., over a few weeks (or a few semibatch cycles) of biofilm operation. In this study, we investigated the behavior of biofilm electrodes during a prolonged experimental period. The following questions were studied in detail: Will a primary biofilm increase its performance to eventually reach the current densities of a secondary biofilm after a longer period of time? Here, contradicting results of previous studies show, depending on the experimental conditions, either leveling effects (Santoro et al., 2012) or a sustained performance gain (Soussan et al., 2013).
Furthermore, the question will be addressed if 3D electrodes such as corrugated carbon sustain their high performance during long-term applications, or if a continuous biofilm growth will lead to clogging of the macrostructures, i.e., channels, layers, and will therefore decrease the performance of the 3D electrode materials. Thereby, in this study, we will focus on a purely electrochemical approach. A detailed biological investigation is currently in progress.
Materials and Methods
Electrochemical Setup and Conditions
All chemicals used in this study were purchased from Sigma Aldrich or Roth and were of analytical grade. All measurements (including biofilm growth) were performed under strictly anaerobic conditions at a temperature of 35°C. The electrochemical measurements were carried out in a half cell setup under potentiostatic control (VMP2 or MPG 2, BioLogic, France). Five-necked round-bottom flasks (250 mL) were used as electrochemical cells, containing a working electrode, a counter electrode (graphite rod, CP Graphite GmbH, Germany), and a Ag/AgCl (sat. KCl, 0.197 V vs. SHE) reference electrode (Sensortechnik Meinsberg GmbH, Germany) in a conventional three-electrode arrangement. For the sake of an easier handling in the long-term experiments, we did not shield the counter electrode from the bacterial solution by means of an ion exchange membrane. However, we performed reference measurements with shielded counter electrodes to exclude the potential impact of hydrogen, formed in the counter electrode reaction. The flasks were sealed with silicone stoppers and Parafilm® strips in order to assure anaerobic conditions.
The working electrodes were composed of LCC (Chen et al., 2012b), each consisting of three corrugate layers (see Figure 2). The projected surface area was in the range of 4–5 cm2 (for a precise overview, see Table 1). The electrode performance was studied at a constant applied potential (chronoamperometry, CA) of 0.2 V.

Figure 2. Schematic illustration of the electrode preparation starting from the raw cardboard material (left), via carbonization (middle) to the mounted electrode (right). The electrodes used in the experiments (right) consisted of a graphite current collector (the back and the sides being isolated with epoxy glue), stainless steel wire (covered with a silicone tube), and the layered corrugated carbon material.

Table 1. Summary of the geometric dimensions and the performance data of the LCC electrodes used for primary and secondary biofilm experiments.
All electrode potentials in this article refer to the silver/silver chloride reference electrode.
Electrode Preparation
The corrugated cardboard material was recycling paper based and was sampled from the cardboard packaging of a BioLogic potentiostat. It already consisted of three flute layers (flute height of first layer 2.4 mm, and second and third 3.0 mm). The material was carbonized at a temperature of 800°C in a batch type furnace (N 11/H, Nabertherm, Germany) with an integrated gas chamber. For this purpose, the head space of the gas chamber was purged with nitrogen for 30 min at room temperature prior to carbonization. After that, the temperature was quickly increased up to the final carbonization temperature (still under nitrogen atmosphere), which was held for at least 2 h.
After carbonization, the corrugated carbon was glued onto a graphite plate (polycrystalline graphite, 5 mm thickness, CP graphite), serving as current collector. To produce a conductive glue, a two-component epoxy resin (“5 min Epoxy”, R & G Faserverbundwerkstoffe, Germany) was mixed with graphite fibers (Toolcraft, Germany). The glue was processed within 5 min and was allowed to fully harden overnight. The total internal resistance of the electrodes was less than 10 Ω.
Cultivation Medium and Inoculum
This study is based on the use of “artificial wastewater,” consisting of 10 mM acetate as the carbon source and a standard cultivation medium as reported by Kim et al. (2005). It contained NH4Cl (0.31 gL−1), KCl (0.13 g L−1), NaH2PO4⋅H2O (2.69 g L−1), Na2HPO4 (4.33 g L−1), trace metal (12.5 mL L−1), and vitamin (12.5 mL L−1) solutions (Balch et al., 1979).
Real wastewater, i.e., effluent after pre-treatment (EAPT) taken from the wastewater treatment plant (WWTP) Steinhof (Braunschweig, Germany), served as the microbial source/inoculum.
Cultivation of Primary and Secondary Electroactive Biofilms
To ensure anaerobic conditions, the cultivation medium was purged with nitrogen for at least 20 min before inoculation. For primary biofilm growth, 10 mL of inoculum was added to 190 mL artificial wastewater to yield a total volume of 200 mL. After purging with nitrogen for 20 min, the cell was operated at a constant temperature of 35°C, with a constant working electrode potential of 0.2 V. The biofilm growth was monitored by measuring the current resulting from bioelectrocatalytic acetate oxidation. At least 25 batch cycles were performed, changing the whole substrate solution after a complete substrate consumption (indicated by zero current). Addition of microbial inoculum was limited to the first three cycles.
The method for the secondary biofilm cultivation was identical with that for primary biofilms, except for the used bacterial source. For secondary biofilm cultivation, primary electroactive biofilms (5 mL suspension of primary biofilm per 200 mL substrate solution) served as the bacterial inocula (the blue path shown in Figure 1). Primary biofilms grown on graphite rods (CP Graphite GmbH, Germany) were scratched off with a sterile spatula into a falcon tube filled with 5 mL buffer solution and dispersed with a vortex mixer (Vortex Genie 2, Scientific Industries, NY, USA) for 2 min. Afterward, these suspensions were used as inocula for fresh media solutions.
All experiments were carried out in duplicates.
Results and Discussion
Biofilm Cultivation
Figure 3 illustrates the electrocatalytic activity of a primary and a secondary biofilm at a layered corrugate carbon during the initial three semibatch cycles after inoculation of the system. The current is gained from the complete electrocatalytic oxidation of acetate by the electroactive biofilms (as shown in Eq. 1).
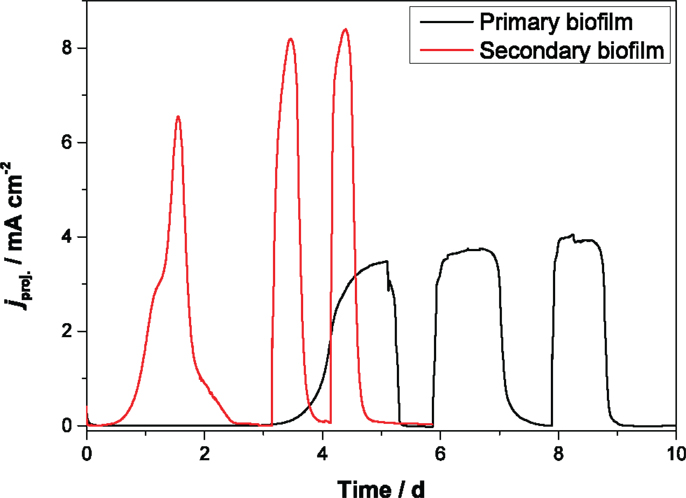
Figure 3. Current generation of a primary (black) and a secondary (red) biofilm at LCC electrodes during the first three semibatches after inoculation. The applied potential was 0.2 V, the temperature was 35°C.
After reaching the stationary phase of the biofilm growth, a steady maximum current density level of 3.9 mA cm−2 is achieved for primary biofilm and 8.3 mA cm−2 for secondary biofilm. The average current densities for the entire study are presented in Table 1. With 89.6 ± 3.2% (primary biofilm electrodes) and 88.3 ± 7.3% (secondary biofilm electrodes), the Coulomb efficiency (CE) for both biofilm generations was virtually identical. The data is in accordance with previous publications illustrating the doubling of the performance from primary to secondary biofilm (Liu et al., 2008).
Beside the high performance of secondary biofilms, their growth seems to be considerably faster than that of primary biofilms. As can be seen in Figure 3 the primary biofilm formation shows a lag phase of about 4 days. This time is required for the adaptation and the selection of electroactive bacteria from the original bacterial source (wastewater). After this lag phase, a constant current production can be observed in the following batch cycles. In contrast, secondary biofilm formation commences at a significantly reduced lag phase about 1 day. The reasons for the short lag time of the secondary biofilm formation as opposed to the primary biofilm formation are not fully understood. Yet, one explanation is the different abundance of electrochemically active bacteria in the different inocula. As shown in our group’s previous paper (Harnisch et al., 2011) for the example of acetate grown biofilms, Geobacter spec. are the clearly predominant species in the electroactive biofilms, although in the primary inoculum they are below detection limit for the used analytical method, flow cytometry. Second, it can be speculated that in wastewater bacteria like Geobacter are not adapted to extracellular electron transfer but rather utilize dissolved electron acceptors. This requires an adaptation toward electrode respiration. Once adapted, any colonization of a new electrode may proceed rapidly. Thus, using primary biofilm biomass as inoculum is much more efficient than using wastewater.
Due to the high performance (twice as high current density) of secondary biofilms in comparison to primary biofilms, the time necessary for complete substrate conversion decreases significantly. This becomes visible in the decreased duration of the individual batch cycles at identical gained electric charge (Figure 4). This performance gain would lead to a significant reduction in the required hydraulic retention time (HRT) of a bioelectrochemical reactor, which is an important parameter for a technical process. In this case, a reduction of HRT by 33% was achieved.
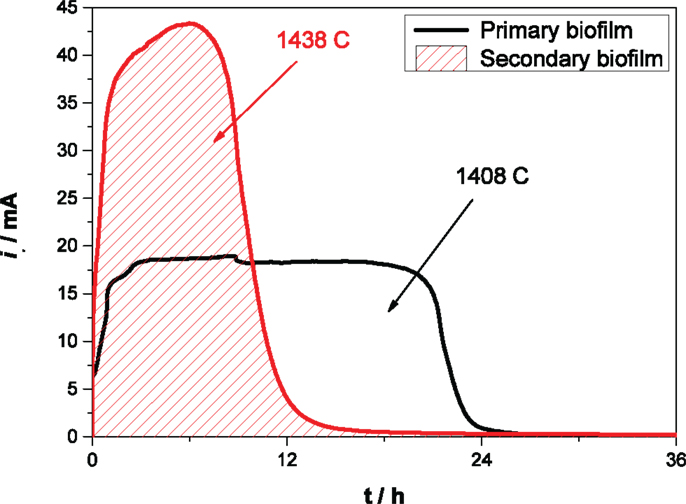
Figure 4. An example showing the comparison of the cycle duration of a primary (black) and secondary biofilm (red). The amount of charge transferred during the bioelectrocatalytic substrate oxidation is indicated by the curves’ areas.
Compared to our previous study (Chen et al., 2012b), the overall current densities (Table 1) in this study are significantly lower. This can be attributed to the lowered substrate concentration (10 mM, instead of 20 mM in our previous study), which we chose to better reflect the soluble COD fraction found in real wastewaters. Further, the electrode size in this study is larger than in the previous study (projected. surface areas of 4–5 cm2 vs. 1 cm2), at the same batch reactor volume. Combined with the lower initial substrate level, the faster substrate consumption does not allow significantly higher current densities.
Long-Term Studies
The major intent of this study was to explore the effect of the biofilm-generation on the produced current over a period of at least 25 batch cycles (primary biofilm ≈ 75 days, secondary biofilm ≈ 50 days). Since it is known that secondary biofilms generally produce higher current densities than primary biofilms (Rabaey et al., 2003; Kim et al., 2005; Liu et al., 2008), the question was if the difference in performance would sustain in the long term, or if the performance of primary biofilms would slowly increase to finally reach the performance of secondary biofilms.
The second question concerns LCC as a high-performance 3D electrode material. LCC electrodes outperformed previous electrode systems but is the performance sustainable over a longer period of operation?
Figure 5A shows the average maximum current density for two duplicates during 25 cycles of operation of primary and secondary biofilms and the corresponding Coulomb efficiencies (Figure 5B).
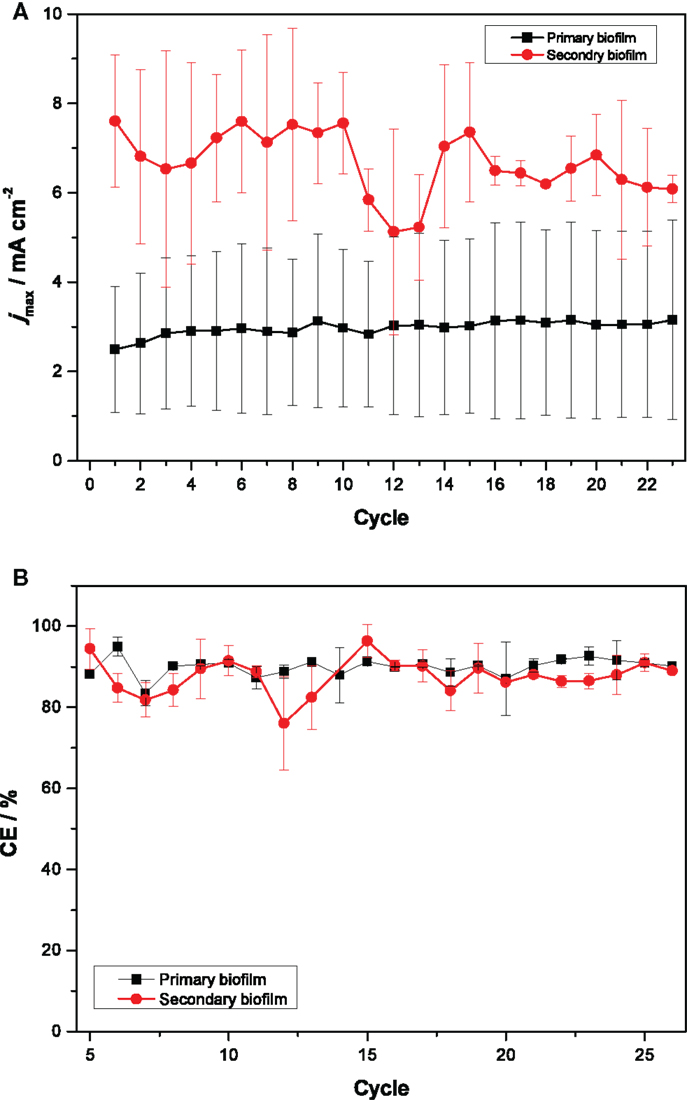
Figure 5. Maximum peak current density (A) and Coulombic efficiency (B) data from long-term experiments generated by primary (black) and secondary biofilms (red). The data shown represents the arithmetic mean of two duplicates. The error bars indicate the standard deviation.
The performance of the secondary biofilms (Figure 5, red trace) was – in average – at least two times higher than that of the primary biofilms (black trace). Even after the presented time segment, no significant increase in current production was observed for primary biofilms. On the other hand, even after 40 cycles, the performance of the secondary biofilms decreased only slightly, after about 100 days of operation (Figure 6). This decrease may be attributed to the fact that after the initial inoculation, the system was fed with sterile substrate solution, which may lead to a slow genetic deterioration of the electroactive bacteria biofilm. Under non-sterile conditions in a MFC, e.g., treating real wastewater, where the biofilm is in steady contact with wastewater bacteria would promote self-regeneration and therefore improve the long-term stability.
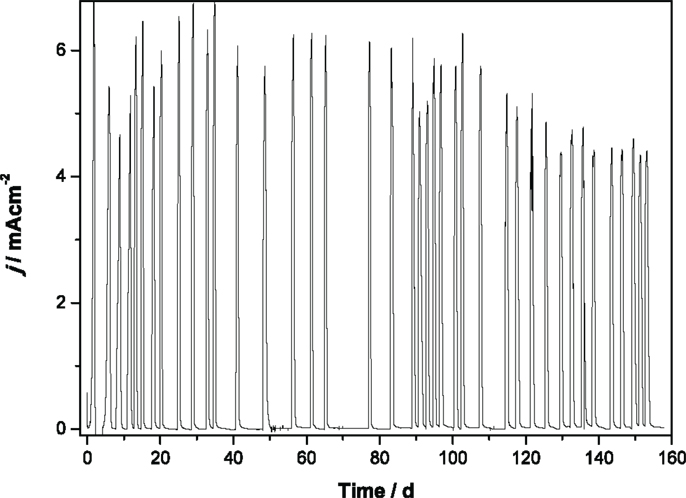
Figure 6. 40 batch cycles (160 days) of a continuously monitored secondary biofilm experiment. The applied potential was 0.2 V, the temperature was 35°C.
Over the entire experimental period, the CE of both biofilms is in the order of about 90% on average. This situation can be explained by the fact that in the used artificial wastewater (with acetate as the substrate), there are no competing (scavenging) biological processes that may lower the CE.
Although accidental oxygen intrusion occurred in one of the secondary biofilm experiments during cycle 11 (depicted by the temporary current decrease between cycle 11 and 13 in Figure 5), the performance recovered to its previous level.
It is also noteworthy that the carbonized cardboard material was mechanically stable under the chosen experimental conditions. Further, the electrode channels did not clog due to biomass accumulation (see Figure 7). After at least 25 batch cycles, the secondary biofilms (Figure 7B) were denser than the primary biofilms (Figure 7A); however, in both cases, the channels of the LCC electrode were clearly not blocked.
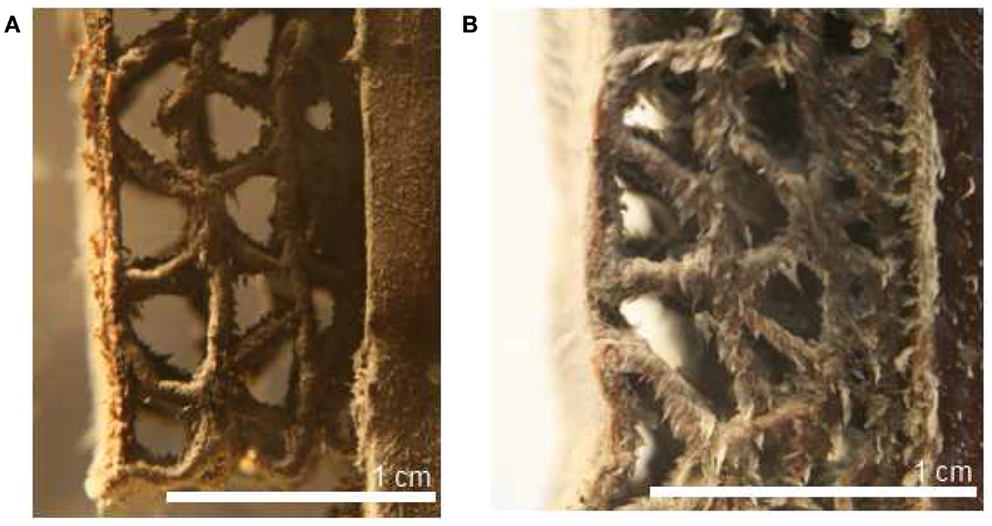
Figure 7. Digital photographs of primary (A) and secondary biofilms (B) grown on LCC electrodes for at least 25 batch cycles.
Conclusion
In this study, we have investigated the performance of primary and secondary electroactive biofilms grown on LCC electrodes. The study illustrates that corrugated layered carbon allows a sustained long-term performance without significant decrease in electrode performance. Furthermore, the study showed that the bioelectrocatalytic activity of secondary biofilms is about two times higher than of primary biofilms. This performance ratio is still sustainable after a long-time span, indicating a high stability of the biofilm properties after the initial biofilm formation. The biological background of the long-lasting performance increase of secondary biofilms has to be clarified in a respective biologic study.
Conflict of Interest Statement
The authors declare that the research was conducted in the absence of any commercial or financial relationships that could be construed as a potential conflict of interest.
Acknowledgments
The authors gratefully thank the Deutsche Forschungsgemeinschaft (DFG) for support (DFG grant SCHR 753/10-1).
References
Balch, W. E., Fox, G. E., Magrum, L. J., Woese, C. R., and Wolfe, R. S. (1979). Methanogens: reevaluation of a unique biological group. Microbiol. Rev. 43, 260–296.
Cercado, B., Byrne, N., Bertrand, M., Pocaznoi, D., Rimboud, M., Achouak, W., et al. (2013). Garden compost inoculum leads to microbial bioanodes with potential-independent characteristics. Bioresour. Technol. 134, 276–284. doi:10.1016/j.biortech.2013.01.123
Chen, S., He, G., Carmona-Martinez, A. A., Agarwal, S., Greiner, A., Hou, H., et al. (2011a). Electrospun carbon fiber mat with layered architecture for anode in microbial fuel cells. Electrochem. Commun. 13, 1026–1029. doi:10.1016/j.elecom.2011.06.009
Chen, S., Hou, H., Harnisch, F., Patil, S. A., Carmona-Martinez, A. A., Agarwal, S., et al. (2011b). Electrospun and solution blown three-dimensional carbon fiber nonwovens for application as electrodes in microbial fuel cells. Energy Environ. Sci. 4, 1417–1421. doi:10.1039/c0ee00446d
Chen, S., He, G., Hu, X., Xie, M., Liu, Q., Zhou, Y., et al. (2012a). Three-dimensional ordered macroporous carbon derived from a natural plant resource as anode for microbial bioelectrochemical systems. ChemSusChem 5, 1059–1063. doi:10.1002/cssc.201100783
Chen, S., He, G., Liu, Q., Harnisch, F., Zhou, Y., Chen, Y., et al. (2012b). Layered corrugated electrode macrostructures boost microbial bioelectrocatalysis. Energy Environ. Sci. 5, 9769–9772. doi:10.1039/c2ee23344d
Cheng, S., and Logan, B. E. (2007). Ammonia treatment of carbon cloth anodes to enhance power generation of microbial fuel cells. Electrochem. Commun. 9, 492–496. doi:10.1016/j.elecom.2006.10.023
Harnisch, F., Koch, C. H., Patil, S. A., Hübschmann, T., Müller, S., and Schröder, U. (2011). Revealing the electrochemically driven selection in natural community derived microbial biofilms using flow-cytometry. Energy Environ. Sci. 4, 1265–1267. doi:10.1039/c0ee00605j
He, G., Gu, Y., He, S., Schroeder, U., Chen, S., and Hou, H. (2011). Effect of fiber diameter on the behavior of biofilm and anodic performance of fiber electrodes in microbial fuel cells. Bioresour. Technol. 102, 10763–10766. doi:10.1016/j.biortech.2011.09.006
Ketep, S. F., Bergel, A., Bertrand, M., Achouak, W., and Fourest, E. (2013). Sampling location of the inoculum is crucial in designing anodes for microbial fuel cells. Biochem. Eng. J. 73, 12–16. doi:10.1016/j.bej.2013.01.001
Kim, J. R., Min, B., and Logan, B. E. (2005). Evaluation of procedures to acclimate a microbial fuel cell for electricity production. Appl. Microbiol. Biotechnol. 68, 23–30. doi:10.1007/s00253-004-1845-6
Kumar, A., Conghaile, P. Ó, Katuri, K., Lens, P., and Leech, D. (2013). Arylamine functionalization of carbon anodes for improved microbial electrocatalysis. RSC Adv. 3, 18759. doi:10.1039/c3ra42953a
Liu, Y., Harnisch, F., Fricke, K., Sietmann, R., and Schroeder, U. (2008). Improvement of the anodic bioelectrocatalytic activity of mixed culture biofilms by a simple consecutive electrochemical selection procedure. Biosens. Bioelectron. 24, 1012–1017. doi:10.1016/j.bios.2008.08.001
Liu, Y., Harnisch, F., Schroeder, U., Fricke, K., Climent, V., and Feliu, J. M. (2010). The study of electrochemically active mixed culture microbial biofilms on different carbon-based anode materials. Biosens. Bioelectron. 25, 2167–2171. doi:10.1016/j.bios.2010.01.016
Lovley, D. R. (2006). Bug juice harvesting electricity with microorganisms. Nat. Rev. Microbiol. 4, 497–508. doi:10.1038/nrmicro1506
Oliveira, V. B., Simões, M., Melo, L. F., and Pinto, A. (2013). Overview on the developments of microbial fuel cells. Biochem. Eng. J. 73, 53–64. doi:10.1016/j.bej.2013.01.012
Rabaey, K., Lissens, G., Siciliano, S. D., and Verstraete, W. (2003). A microbial fuel cell capable of converting glucose to electricity at high rate and efficiency. Biotechnol. Lett. 25, 1531–1535. doi:10.1023/A:1025484009367
Santoro, C., Lei, Y., Li, B., and Cristiani, P. (2012). Power generation from wastewater using single chamber microbial fuel cells (MFCs) with platinum-free cathodes and pre-colonized anodes. Biochem. Eng. J. 62, 8–16. doi:10.1016/j.bej.2011.12.006
Schroeder, U. (2011). Discover the possibilities. microbial bioelectrochemical systems and the revival of a 100-year-old discovery. J. Solid State Electrochem. 15, 1481–1486. doi:10.1007/s10008-011-1395-7
Scott, K., Rimbu, G. A., Katuri, K. P., Prasad, K. K., and Head, I. M. (2007). Application of modified carbon anodes in microbial fuel cells. IChemE Part B 85, 481–488. doi:10.1205/psep07018
Sell, D., Kraemer, P., and Kreysa, G. (1989). Use of an oxygen gas diffusion cathode and a three-dimensional packed bed anode in a bioelectrochemical fuel cell. Appl. Microbiol. Biotechnol. 31, 211–213. doi:10.1007/BF00262465
Soussan, L., Erable, B., Delia, M., and Bergel, A. (2013). The open circuit potential of Geobacter sulfurreducens bioanodes depends on the electrochemical adaptation of the strain. Electrochem. Commun. 33, 35–38. doi:10.1016/j.elecom.2013.04.013
Srikanth, S., Marsili, E., Flickinger, M. C., and Bond, D. R. (2008). Electrochemical characterization of Geobacter sulfurreducens cells immobilized on graphite paper electrodes. Biotechnol. Bioeng. 99, 1065–1073. doi:10.1002/bit.21671
Torres, C. I., Marcus, A. K., and Rittmann, B. E. (2008). Proton transport inside the biofilm limits electrical current generation by anode-respiring bacteria. Biotechnol. Bioeng. 100, 872–881. doi:10.1002/bit.21821
Wei, J., Liang, P., and Huang, X. (2011). Recent progress in electrodes for microbial fuel cells. Bioresour. Technol. 102, 9335–9344. doi:10.1016/j.biortech.2011.07.019
Keywords: microbial fuel cell, electroactive bacteria, electroactive biofilm, 3D electrode, layered corrugated carbon
Citation: Baudler A, Riedl S and Schröder U (2014) Long-term performance of primary and secondary electroactive biofilms using layered corrugated carbon electrodes. Front. Energy Res. 2:30. doi: 10.3389/fenrg.2014.00030
Received: 04 February 2014; Accepted: 16 July 2014;
Published online: 30 July 2014.
Edited by:
Jacob N. Chung, University of Florida, USAReviewed by:
Y.-H. Percival Zhang, Virginia Polytechnic Institute and State University, USASarah Glaven, Naval Research Laboratory, USA
Copyright: © 2014 Baudler, Riedl and Schröder. This is an open-access article distributed under the terms of the Creative Commons Attribution License (CC BY). The use, distribution or reproduction in other forums is permitted, provided the original author(s) or licensor are credited and that the original publication in this journal is cited, in accordance with accepted academic practice. No use, distribution or reproduction is permitted which does not comply with these terms.
*Correspondence: Uwe Schröder, Institute of Environmental and Sustainable Chemistry, Technische Universität Braunschweig, Hagenring 30, 38106 Braunschweig, Germany e-mail:dXdlLnNjaHJvZWRlckB0dS1icy5kZQ==
†André Baudler and Sebastian Riedl have contributed equally to this work.