- 1State Key Laboratory on Marine Resource Utilization in South China Sea, Hainan Provincial Key Laboratory of Research on Utilization of Si-Zr-Ti Resources, College of Materials Science and Chemical Engineering, Hainan University, Haikou, China
- 2Key Laboratory of Advanced Energy Materials Chemistry (Ministry of Education), Nankai University, Tianjin, China
- 3National Laboratory of Solid State Microstructures, Nanjing University, Nanjing, China
In situ characterization techniques have made a significant progress in recent years, especially in the electrochemical field. For Li-ion batteries, in situ characterization techniques refer to using analytical equipment to directly characterize electrode materials during electrochemical measurements. At present, most in situ batteries are developed from commercial simulated batteries, of which the cost is very high and the cycle life is quite short. In this work, two kinds of coin-cell-based in situ batteries were designed as in situ X-ray diffraction (XRD) and Raman coin cells which exhibit many admirable advantages, such as low cost, long cycle life, easy to carry, and so on. In the designing process, in situ XRD and Raman coin cell have been tested with two electrode materials of Li4Ti5O12 and LiFePO4, and we solved many technical problems of assembling and measuring these two kinds of cells. Finally, in situ coin cells could be improved to investigate a variety of electrode materials, and this technique would arouse wide interests in the electrochemical field.
Introduction
Li-ion batteries as a recyclable power source have been used in a variety of electronic devices and energy storage equipment (Armand and Tarascon, 2008), which has aroused a lot of interests in the academic community, while the complicated electrochemical process is still mysterious within Li-ion batteries during the cycling (Qian et al., 2015; Ross, 2015; Wu et al., 2016; Yang et al., 2017). In situ characterization techniques are quite suitable to explore the structure-behavior relationship of Li-ion batteries, which can in situ observe the electronic structure, the crystal structure, the evolution of micro-morphology, etc. (Yang et al., 2017). In recent years, many in situ characterization techniques have been developed, such as in situ X-ray diffraction (XRD) (Hu et al., 2014; Liu et al., 2014; Sharma et al., 2015), in situ Raman spectroscopy (Gross et al., 2013; Lanz et al., 2013; Wu et al., 2013a), in situ Fourier transform infrared spectroscopy (Cheng et al., 2007), and in situ transmission electron microscopy (Chen et al., 2015).
Li-ion batteries work in a closed environment to protect the electrode materials from air atmosphere, so it is very difficult to be acquired the internal information of these batteries, except for some ex situ characterization techniques after deconstructing the batteries. In order to study the structure change and surface evolution of electrode materials during the electrochemical reactions, many researchers have made great efforts to develop in situ techniques for Li-ion batteries. Thurston et al. (1998) designed an early in situ XRD cell to measure electrode materials and intuitively observed lattice expansion and contraction, phase transition, and multi-phase formation. In next two decades, in situ XRD cells have been developed sufficiently with a typical construction as follows: a hole was created in a protective case or current collector and next sealed by an X-ray transparent material, such as a Kapton, Be, or Al foil. They were usually based on the simulated battery (Misra et al., 2012; He et al., 2013; Lin et al., 2013, 2014; Roberts et al., 2014; Stancovski and Badilescu, 2014; Villevieille et al., 2014) or the coin cell (Thorne et al., 2011; Fell et al., 2012; Zhu et al., 2013; Lowe and Gao, 2014). The first in situ Raman cell was designed by Inaba et al. in 1995, which was used to study the electrochemical Li intercalation into Graphite (Inaba et al., 1995). Shu et al. (2011) also manufactured an in situ Raman battery to investigate the electrode material of Li4Ti5O12. Most in situ Raman cells were based on the simulated battery (Long et al., 2011; Nakagawa et al., 2012; Gross et al., 2013; Lanz et al., 2013; Wu et al., 2013b; Hy et al., 2014). However, these in situ batteries have not been widely spread in the research community, due to their complicated construction, high cost, and short operation time.
In this work, coin-cell-based in situ batteries have been designed and tested for Li-ion batteries. By modifying the normal coin cells, we fabricated in situ XRD and Raman coin cells, with which some excellent measurement results are obtained for Li4Ti5O12 and LiFePO4. Compared with commercial simulated batteries, in situ coin cells have many advantages, such as low cost, simple assembly, and good sealing. Thereby, coin-cell-based in situ characterization techniques would arouse wide interests in the electrochemical field.
Experiment
Design of In Situ Coin Cells
As shown in Figure 1A, a normal coin cell was composed of a negative battery case, a battery shrapnel, a lithium anode, a separator, an electrode material, a current collector, and a positive battery case from top to bottom. Based on a normal coin cell, we designed in situ XRD and Raman coin cells (Figures 1B,C). For in situ XRD, a Be sheet as an X-ray windows was firmly attached to the bottom case with a thermoplastic film. Here, the Be sheet was chosen for its high X-ray transmittance and large electrochemical stability window (0–4 V vs. Li+/Li). In the construction, all components were dried in an oven of 80°C for 6 h and then assembled in a glove box filled with Ar gas (H2O, O2 < 1 PPM). Notably, the current collector is a metal mesh with two conductive tails, and the working electrode was toward the X-ray window, instead of the counter electrode in a normal coin cell. The in situ Raman coin cell is similar to the in situ XRD coin cell, except for an optical window of quartz.
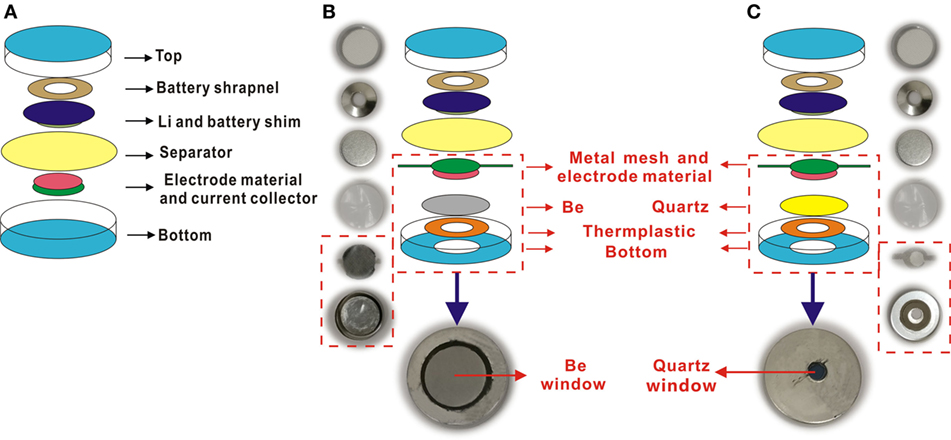
Figure 1. Structure diagrams and real photos of normal coin cell and in situ coin cell. (A) Normal coin cell. (B) In situ X-ray diffraction coin cell. (C) In situ Raman coin cell.
Preparation of Working Electrode
For in situ XRD, one working electrode was prepared as a mixture paste containing 42.5 wt.% Li4Ti5O12 (Ishihara Sangyo Kaisha, Ltd.), 42.5 wt.% acetylene black, and 15 wt.% polytetrafluoroethylene (PTFE), which was pressed on a stainless steel mesh. Another was prepared as a mixture paste containing 80 wt.% LiFePO4 (Sumitomo Osaka Cement Co. Ltd., Japan), 10 wt.% acetylene black, and 10 wt.% PTFE, which was pressed on an Al mesh (100 mesh) with a mass loading of approximately 5 mg cm−2. The counter electrode of lithium metal was separated from the working electrode by a Celgard film 2400 porous polypropylene film, and the electrolyte was 1 M LiClO4 in a mixture of ethylene carbonate (EC)/diethyl carbonate (DEC) (1:1 in volume) or 1 M LiPF6 in a mixture of EC and dimethyl carbonate (DMC) (1:1 in volume).
For in situ Raman, the working electrode was composed of 90 wt.% Li4Ti5O12 and 10 wt.% PTFE which was pressed onto a stainless steel mesh. For comparison, the carbon content was tailored as 10 and 42.5 wt.% in the working electrode. The counter electrode of lithium metal was separated from the working electrode by a Celgard film 2400 porous polypropylene film, and the electrolyte was 1 M LiClO4 in a mixture of EC/DEC (1:1 in volume).
In Situ Measurements
Electrochemical measurements were conducted on a Battery Testing System (BioLogic VSP-300) at the room temperature, with a potential range of 1.2–2.0 V for Li4Ti5O12 and 2.8–4.0 V for LiFePO4. Simultaneously, XRD patterns were acquired by using a Bruker D2 PHASER diffractometer with Cu kα radiation, or Raman spectra were collected on Thermo Scientific™ DXR Micro-Raman Spectrometer.
Results and Discussion
Our in situ XRD/Raman coin cells were tested with the working electrode materials of Li4Ti5O12 and LiFePO4, and their constructions were optimized according to the experimental results. For in situ XRD coin cell, we selected two Be sheets of 0.2 and 0.5 mm thick to in situ measure the LiFePO4 electrode. During the charge from 2.8 to 4.0 V with a rate of 0.1 C, in situ XRD measurements were conducted simultaneously. As shown in Figure 2A, the diffraction peaks of LiFePO4 can be observed for the Be sheet of 0.2 mm thick, but it is difficult for the Be sheet of 0.5 mm thick, as shown in Figure 2B, because the thick Be sheet would absorb the X-ray strongly. In the in situ XRD pattern of LiFePO4, an XRD peak of LiFePO4 (211) (labeled “T”) decreases during the charge process and two XRD peaks of FePO4 (020) and (211) (labeled “H”) emerges and increases, which was same as the literature reported in the past (Gibot et al., 2008; Meethong et al., 2012). Thereby, the peak intensity is dependent on the thickness of Be sheet, and the thickness of 0.2 mm is suitable for in situ XRD measurements.
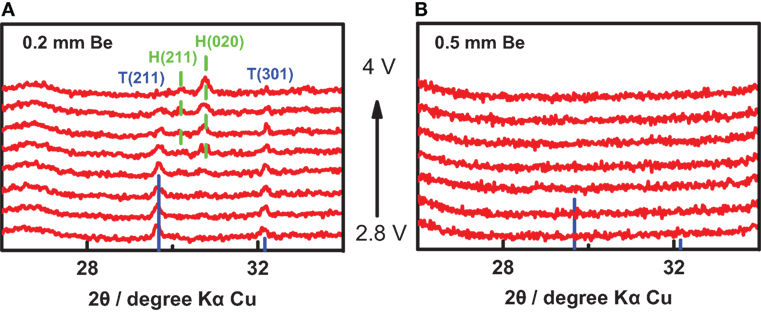
Figure 2. In situ X-ray diffraction (XRD) measurement with different Be sheets. The in situ XRD results of LiFePO4 with a Be sheet of (A) 0.2 mm and (B) 0.5 mm thick, respectively. “H” represents heterosite (lithium-poor phase, FePO4), “T” represents triphylite (lithium-rich phase, LiFePO4).
It is well known that the sample for XRD measurement must be placed in a fixed plane, and the measured peak would shift with the height of the plane. In our in situ XRD coin cell, the thin Be sheet would be bent under a big pressure, which is expected to influence the measured peak more of less. As shown in Figure 3A, we prepared two in situ XRD coin cells (A-battery and B-battery) with the battery shim of 0.8 and 1.0 mm, respectively. The Be sheet is very flat in A-battery while it is evidently curved in B-battery, owing to the different pressures from the top to the bottom. Figure 3B shows XRD patterns of Li4Ti5O12 measured with these two in situ coin cells. The XRD peak of B-battery is obviously broader than that of A-battery, with a quite big peak shoulder in the small angle. Actually, the bent Be sheet made different parts of the working electrode located at different heights, so the broad and asymmetrical peak of B-battery was the superposition of partial XRD peaks at different angles. Thereby, we chose the battery shim of 0.8 mm thick to obtain a flat X-ray window under a proper pressure.
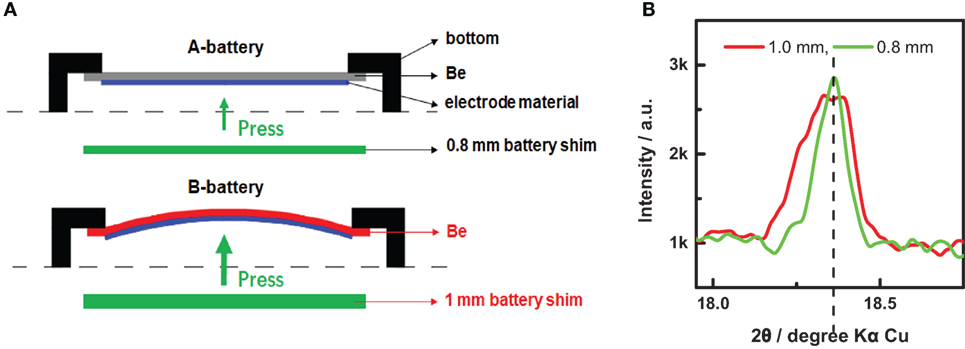
Figure 3. Influence of the electrode bending on X-ray diffraction (XRD) results. (A) Schematics of the flat and bent electrode films pressed on the Be sheets. (B) XRD patterns of Li4Ti5O12 measured with two different in situ coin cells in (A).
To check the in situ XRD coin cell with different electrolytes, the cathode material of LiFePO4 was measured in a potential range of 2.8–4.0 V with two common electrolytes as 1 M LiClO4 in EC/DEC (1:1 in volume) and 1 M LiPF6 in EC/DMC (1:1 in volume). As shown in Figure 4, the charge and discharge curves are quite good for LiPF6, while the charge curve for LiClO4 shows an abnormal plateau at the voltage of 3.8 V. Actually, the abnormal plateau should be attributed to a side reaction, as the LiClO4 with a strong oxidizability might react with the Be sheet at a high voltage. Thus, the electrolyte of LiPF6 (EC/DMC = 1:1 in volume) is proved to be a good choice for the cathode material in the in situ XRD coin cell. On the other hand, the anode material of Li4Ti5O12 can be in situ measured excellently for both the electrolytes with a potential range of 1.2–2.0 V.
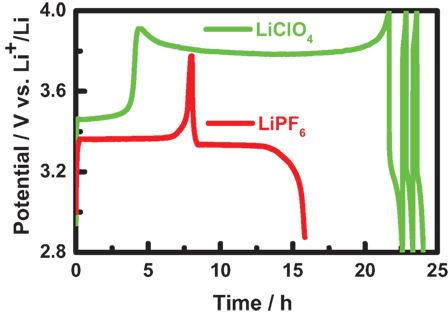
Figure 4. The charge and discharge curve of in situ X-ray diffraction coin cells with different electrolytes as 1 M LiClO4 in a mixture of ethylene carbonate (EC) and diethyl carbonate (1:1 in volume, green) or 1 M LiPF6 in a mixture of EC and dimethyl carbonate (1:1 in volume, red). Here, the charge–discharge measurements were conducted at a current rate of C/10 within a potential range of 2.8−4.0 V.
Using this in situ XRD coin cell, we successfully obtained the in situ XRD patterns during the electrochemical reaction, as shown in Figure 5, which can be deeply analyzed to reveal the structural evolution of electrode materials. For Li4Ti5O12, the electrochemical measurement was performed as: charge at C/10 to 2.0 V and then potentiostatic within 10 h; discharge at C/10 to 1.2 V and then potentiostatic within 10 h. Simultaneously, in situ XRD patterns were acquired every 1 h (including the measurement time of 42 min and the interval time of 18 min). As shown in Figure 5B, the red and blue curves were measured during the charge and discharge processes, respectively. The XRD peaks can be excellently fitted with the Lorentzian function to retrieve the peak position, peak intensity, peak area, and full width at half maximum. For Peak (111) of Li4Ti5O12, the peak position shifted to the small angle during the charge process and then the peak position gradually recovered during the discharge process, as shown in Figure 5C. Meanwhile, the peak intensity decreased and recovered during the charge and discharge processes, respectively, as shown in Figure 5D. However, the fitting results of peak position and intensity are not so smooth, which should be attributed to the small signal-noise ratio. Furthermore, the peak position and intensity can be used to evaluate the phase fraction and lattice constants, which is significant to study the structural evolution in Li-ion batteries.
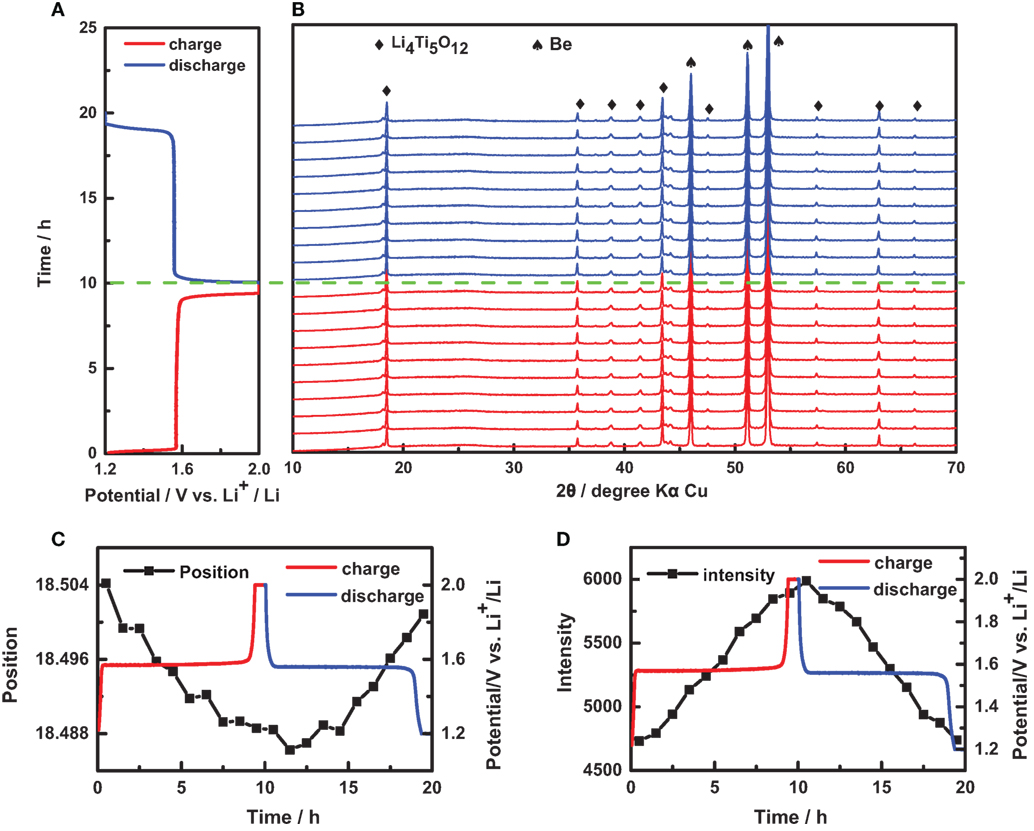
Figure 5. In situ characterization of Li4Ti5O12 electrode. (A) A charge/discharge curve of an in situ coin cell with Li4Ti5O12 electrode: charge at C/10 to 2.0 V and then potentiostatic within 10 h; discharge at C/10 to 1.2 V and then potentiostatic within 10 h. (B) In situ X-ray diffraction (XRD) patterns of this in situ coin cell during the charge and discharge processes. The variation of (C) position and (D) intensity of peak (111) retrieved from in situ XRD patterns in (B).
There also exist some limitations in our design. Although the Be sheet is very popular for in situ XRD, Be is very toxic in the form of powder and it can be oxidized easily at a high potential, thus, it was sometimes substituted by Al film or Al-plastic film. In the in situ XRD coin cell, the small detective windows limited the mass loading of the working electrode, the edge of coin cell blocked the small-angle diffraction, and the Be sheet absorbed the X-ray especially for the small diffraction angle, which result in a low signal-noise ratio. This problem can be resolved by lengthening the measurement time or enhancing the X-ray intensity. In our lab-based XRD instrument, the low X-ray intensity requires a long collection time to achieve a high signal-noise ratio. In comparison, the synchrotron XRD instrument has a strong X-ray beam with a short wavelength, which can penetrate through in situ XRD batteries easily, so the XRD signal is strong enough for the real-time monitoring of electrode materials. However, due to the high intensity, the X-ray of synchrotron might decompose the electrolyte of Li-ion batteries.
On the other hand, the in situ Raman coin cell was optimized by tailoring the carbon content in working electrode. We prepared three work electrodes of Li4Ti5O12 with different carbon contents as 0% C, 5% C, and 42.5% C. As shown in Figure 6A, the Raman spectrum of the Li4Ti5O12 is very close to those previously reported, in which three bands at 238, 433, and 680 cm−1 are attributed to O–Li–O bending in the octahedral unit LiO6, Li–O stretching in the tetrahedral unit LiO4, and Ti–O stretching in the octahedral unit TiO6, respectively (Liu et al., 1994; Leonidov et al., 2003; Julien and Zaghib, 2004). Evidently, the signal for 0% C is much stronger than those for 5% C and 42.5% C, and the Raman peaks of PTFE for 0% C were observed as three Raman bands at 1,200–1,400 cm−1 region, as shown in Figure 6B. However, the conductivity of Li4Ti5O12 is very poor, and no carbon additive will lead to a large polarization. Contrastively, the Raman D-band and G-band of carbon were observed for 5% C and 42.5% C, and the weak signal should be attributed to the strong optical absorption of carbon.
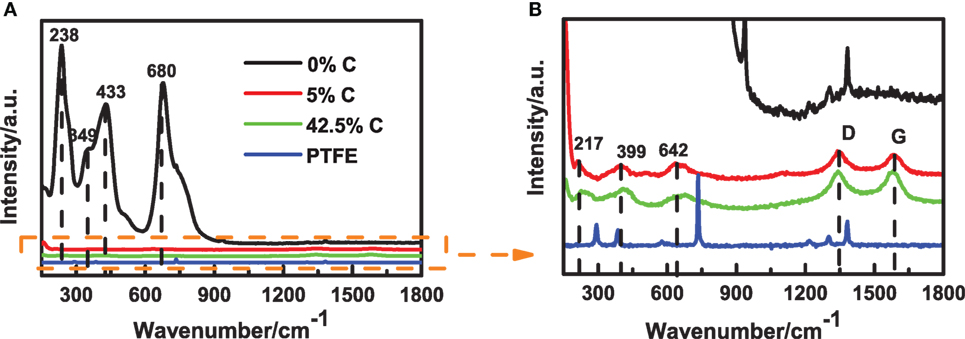
Figure 6. Raman spectra of Li4Ti5O12 electrodes with different carbon contents. (A) Raman spectra of three Li4Ti5O12 electrodes and (B) enlarged view of (A).
If the electrode material exhibits a good electrical conductivity, the in situ Raman measurement can be directly conducted without the carbon additive. Nevertheless, considering the poor electrical conductivity of some electrode materials, we suggest a potential solution that adopts Surface Enhanced Raman Scattering (SERS), which can be conducted by dropping gold-dielectric nanocomposites on a normal electrode with carbon. Even if the electrode material contains the carbon additive, the Raman signal can be enhanced significantly by the gold-dielectric nanoparticles. To achieve a non-destructive and ultrasensitive SERS, various nanoparticles have been synthesized. Li et al. first reported shell-isolated nanoparticle-enhanced Raman spectroscopy (SHINERS) (Li et al., 2010), and then Yu et al. used the SHINERS to investigate that the by-products and overpotential were reduced in Li–O batteries by water addition (Yu et al., 2017). Similarly, Huang et al. (2013) synthesized a novel Au−Pd bimetallic nanostructure as a platform for highly sensitive monitoring of catalytic reactions by SERS.
Through the quartz window of in situ Raman coin cell, the Raman laser is directly illuminated on the working electrode with electrolyte for a long time, so the laser source might influence the measurement. Thus, two identical in situ Raman coin cells are assembled with the working electrode of Li4Ti5O12 and the electrolyte of 1 M LiClO4 in EC/DEC (1:1 in volume), and we adopted two different Raman laser sources for comparison. As a result, the Raman spectrum of Li4Ti5O12 was very stable for the wavelength of 780 nm, while the Raman D-band and G-band of carbon emerged after 10 min for the wavelength of 532 nm, as shown in Figure 7. By further increasing the measuring time, only carbon signal can be observed in the spectrum for the wavelength of 532 nm. Obviously, the electrolyte was decomposed and carbonized seriously under the illumination of short-wavelength laser. Thereby, in situ measurement can be conducted for a long time by using the long-wavelength laser with a low photon energy.
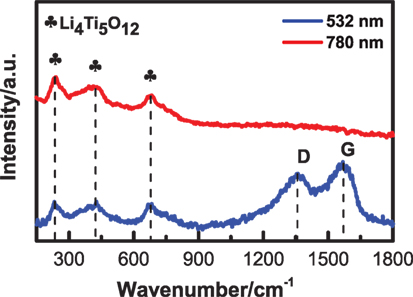
Figure 7. Raman spectra of Li4Ti5O12 electrodes measured by using different laser sources, with the laser wavelength of 532 and 780 nm for the blue and red curves, respectively.
By using our in situ Raman coin cell, the in situ Raman spectra were collected for the working electrode of Li4Ti5O12, as shown in Figure 8. At the charged state, we observed the typical Raman bands of Li4Ti5O12. After discharge, these Raman bands disappeared clearly, while they recovered after recharge. These phenomena are excellently consistent with early literatures (Schneider et al., 2011; Shu et al., 2011). Currently, it is still not clear about the disappearance of Raman signal in this case. Normally, it might be owing to structure change during phase transition (Schneider et al., 2011). In addition, Li4Ti5O12 material becomes electrically conductive after discharge, and then the optical skin depth of the laser will be reduced, which in turn will lead to the disappearance of Raman signals. Therefore, the in situ Raman signals can be used to study the local structures and variations, which is quite useful to reveal the structure degradation in Li-ion batteries.
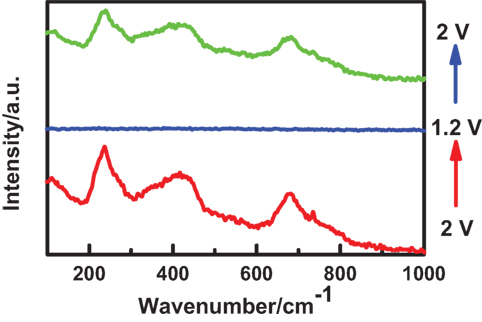
Figure 8. In situ Raman spectra of Li4Ti5O12 electrode. The Raman spectrum evolved as the charged electrode (bottom red curve) was discharged to 1.2 V (middle blue curve) and then charged to 2.0 V (top green curve).
However, in situ Raman was rarely adopted in Li-ion batteries for some problems. In the in situ Raman coin cell, the conductive agent of carbon would seriously suppress the Raman signals in the working electrode, which can be overcome by using the SERS technique as dropping gold-dielectric nanocomposites on a normal electrode with carbon. To prevent the decomposition of electrolyte, we can replace the short-wavelength laser with the long-wavelength laser, while some vibrational modes might be lost in the new Raman spectrum. During continuous charging and discharging, the fluorescent background would be increased with the electrolyte decomposition, resulting in a weak Raman signal considerably.
Conclusion
In this work, we designed the in situ XRD/Raman coin cells and optimized their configurations by testing the working electrodes of Li4Ti5O12 and LiFePO4. In the in situ XRD coin cell, the Be sheet of 0.2 mm thick was chosen to reduce the X-ray absorption, and the internal pressure was tailored to prevent bending the thin Be sheet. The electrolyte of LiPF6 was proved to be a good choice for both cathode and anode materials, while the electrolyte of LiClO4 could not be adopted for the cathode materials. From the in situ XRD results, the peak position and intensity can be used to evaluate the phase fraction and lattice constants, which is significant to study the structural evolution in Li-ion batteries. On the other hand, in the in situ Raman coin cell, the conductive agent of carbon would suppress the Raman signals in the working electrode, which can be resolved by reducing the carbon content for conductive electrode materials or adopting SERS for normal electrodes. The long-wavelength laser was better for in situ Raman measurements, for the electrolyte of Li-ion batteries would be decomposed and carbonized seriously under the illumination of short-wavelength laser. According to the in situ Raman results, the local structures and variations can be investigated to reveal the structure degradation in Li-ion batteries. Therefore, in situ coin cells could be improved to investigate a variety of electrode materials, and this technique would arouse wide interests in the electrochemical field.
Author Contributions
YC directed the project, DL took charge of this investigation, LZ optimized in situ cells, analyzed the data, and authored the manuscript, XG designed the original in situ cells, and all authors contributed to the discussion.
Conflict of Interest Statement
The authors declare that the research was conducted in the absence of any commercial or financial relationships that could be construed as a potential conflict of interest.
Funding
This work was financially supported by the NSFC (No. 21603048 and No. 51362009), Natural Science Foundation of Hainan (Grant No. 20165186), the Science and Technology Development Special Fund Project (ZY2016HN07), the International Science & Technology Cooperation Program of Hainan (KJHZ2015-02), and the Hainan University’s Scientific Research Foundation (kyqd1545).
References
Armand, M., and Tarascon, J. M. (2008). Building better batteries. Nature 451, 652–657. doi:10.1038/451652a
Chen, X., Li, C., and Cao, H. (2015). Recent developments of the in situ wet cell technology for transmission electron microscopies. Nanoscale 7, 4811–4819. doi:10.1039/c4nr07209j
Cheng, Y., Wang, G., Yan, M., and Jiang, Z. (2007). In situ analysis of interfacial reactions between negative MCMB, lithium electrodes, and gel polymer electrolyte. J. Sol. St. Electrochem. 11, 310–316. doi:10.1007/s10008-006-0122-2
Fell, C. R., Chi, M., Meng, Y. S., and Jones, J. L. (2012). In situ X-ray diffraction study of the lithium excess layered oxide compound Li[Li0.2Ni0.2Mn0.6]O2 during electrochemical cycling ☆. Solid State Ionics. 207, 44–49. doi:10.1016/j.ssi.2011.11.018
Gibot, P., Casascabanas, M., Laffont, L., Levasseur, S., Carlach, P., Hamelet, S., et al. (2008). Room-temperature single-phase Li insertion/extraction in nanoscale LixFePO4. Nat. Mater. 7, 741–747. doi:10.1038/nmat2245
Gross, T., Giebeler, L., and Hess, C. (2013). Novel in situ cell for Raman diagnostics of lithium-ion batteries. Rev. Sci. Instrum. 84, 1278–1319. doi:10.1063/1.4813263
He, H., Huang, C., Luo, C. W., Liu, J. J., and Chao, Z. S. (2013). Dynamic study of Li intercalation into graphite by in situ high energy synchrotron XRD. Electrochim. Acta 92, 148–152. doi:10.1016/j.electacta.2012.12.135
Hu, C. W., Chen, T. Y., Shih, K. S., Wu, P. J., Su, H. C., Chiang, C. Y., et al. (2014). Real-time investigation on the influences of vanadium additives to the structural and chemical state evolutions of LiFePO4 for enhancing the electrochemical performance of lithium-ion battery. J. Power Sources 270, 449–456. doi:10.1016/j.jpowsour.2014.07.138
Huang, J., Zhu, Y., Lin, M., Wang, Q., Zhao, L., Yang, Y., et al. (2013). Site-specific growth of Au-Pd alloy horns on Au nanorods: a platform for highly sensitive monitoring of catalytic reactions by surface enhancement Raman spectroscopy. J. Am. Chem. Soc. 135, 8552–8651. doi:10.1021/ja4004602
Hy, S., Felix, F., Chen, Y. H., Liu, J. Y., Rick, J., and Hwang, B. J. (2014). In situ surface enhanced Raman spectroscopic studies of solid electrolyte interphase formation in lithium ion battery electrodes. J. Power Sources 256, 324–328. doi:10.1016/j.jpowsour.2014.01.092
Inaba, M., Yoshida, H., Ogumi, Z., Abe, T., Mizutani, Y., and Asano, M. (1995). ChemInform abstract: in situ Raman study on electrochemical Li intercalation into graphite. Cheminform 26, 20–26. doi:10.1149/1.2043869
Julien, C. M., and Zaghib, K. (2004). Electrochemistry and local structure of nano-sized Li4/3Me5/3O4 (Me = Mn, Ti) spinels. Electrochimica Acta 50, 411–416. doi:10.1016/j.electacta.2004.03.052
Lanz, P., Villevieille, C., and Novák, P. (2013). Electrochemical activation of Li2MnO3 at elevated temperature investigated by in situ Raman microscopy. Electrochim. Acta. 109, 426–432. doi:10.1016/j.electacta.2013.07.130
Leonidov, I. A., Leonidova, O. N., Perelyaeva, L. A., Samigullina, R. F., Kovyazina, S. A., and Patrakeev, M. V. (2003). Structure, ionic conduction, and phase transformations in lithium titanate Li4Ti5O12. Phys. Solid State 45, 2183–2188. doi:10.1134/1.1626760
Li, J. F., Huang, Y. F., Ding, Y., Yang, Z. L., Li, S. B., Zhou, X. S., et al. (2010). Shell-isolated nanoparticle-enhanced Raman spectroscopy. Sci. Found. China 464, 392–395. doi:10.1038/nature08907
Lin, C. K., Ren, Y., Amine, K., Qin, Y., and Chen, Z. (2013). In situ high-energy X-ray diffraction to study overcharge abuse of 18650-size lithium-ion battery. J. Power Sources 230, 32–37. doi:10.1016/j.jpowsour.2012.12.032
Lin, X., Ma, R., Shao, L., Shui, M., Wu, K., Wang, D., et al. (2014). In-situ X-ray diffraction study on the structural evolutions of oxidized fluorophosphates as anode materials for lithium-ion batteries. Ceram. Int. 40, 9107–9120. doi:10.1016/j.ceramint.2014.01.125
Liu, D. Z., Hayes, W., Kurmoo, M., Dalton, M., and Chen, C. (1994). Raman scattering of the Li1+xTi2-xO4 superconducting system. Phys. C Supercond. 235, 1203–1204. doi:10.1016/0921-4534(94)91826-0
Liu, Q., He, H., Li, Z. F., Liu, Y., Ren, Y., Lu, W., et al. (2014). Rate-dependent, Li-ion insertion/deinsertion behavior of LiFePO4 cathodes in commercial 18650 LiFePO4 cells. ACS Appl. Mater. Interfaces 6, 3282. doi:10.1021/am405150c
Long, B. R., Chan, M. K. Y., Greeley, J. P., and Gewirth, A. A. (2011). Dopant modulated Li insertion in Si for battery anodes: theory and experiment. J. Phys. Chem. C 115, 18916–18921. doi:10.1021/jp2060602
Lowe, M. A., and Gao, J. (2014). Mechanistic insights into operational lithium-sulfur batteries by in situ X-ray diffraction and absorption spectroscopy. RSC Adv. 4, 18347–18353. doi:10.1039/c4ra01388c
Meethong, N., Kao, Y. H., Tang, M., Huang, H. Y., Carter, W. C., and Chiang, Y. M. (2012). Electrochemically induced phase transformation in nanoscale olivines Li1−xMPO4 (M = Fe, Mn). Chem. Mater. 20, 6189–6198. doi:10.1021/cm801722f
Misra, S., Liu, N., Nelson, J., Hong, S. S., Yi, C., and Toney, M. F. (2012). In situ X-ray diffraction studies of (De)lithiation mechanism in silicon nanowire anodes. ACS Nano 6, 5465. doi:10.1021/nn301339g
Nakagawa, H., Domi, Y., Doi, T., Ochida, M., Tsubouchi, S., Yamanaka, T., et al. (2012). In situ Raman study on degradation of edge plane graphite negative-electrodes and effects of film-forming additives. J. Power Sources 206, 320–324. doi:10.1016/j.jpowsour.2012.01.141
Qian, D., Ma, C., More, K. L., Meng, Y. S., and Chi, M. (2015). Advanced analytical electron microscopy for lithium-ion batteries. NPG Asia Mater. 7, e193. doi:10.1038/am.2015.50
Roberts, M. R., Madsen, A., Nicklin, C., Rawle, J., Palmer, M. G., Owen, J. R., et al. (2014). Direct observation of active material concentration gradients and crystallinity breakdown in LiFePO4 electrodes during charge/discharge cycling of lithium batteries. J. Phys. Chem. C Nanomater. Interfaces 118, 6548–6557. doi:10.1021/jp411152s
Ross, F. M. (2015). Opportunities and challenges in liquid cell electron microscopy. Science 350, aaa9886. doi:10.1126/science.aaa9886
Schneider, H., Maire, P., and Novák, P. (2011). Electrochemical and spectroscopic characterization of lithium titanate spinel Li4Ti5O12. Electrochim. Acta 56, 9324–9328. doi:10.1016/j.electacta.2011.08.008
Sharma, N., Tapiaruiz, N., Singh, G., Armstrong, A. R., Pramudita, J. C., Brand, H. E. A., et al. (2015). Rate dependent performance related to crystal structure evolution of Na0.67Mn0.8Mg0.2O2 in a sodium-ion battery. Chem. Mater. 27, 150928134805001. doi:10.1021/acs.chemmater.5b02142
Shu, J., Shui, M., Xu, D., Gao, S., Yi, T., Wang, D. J., et al. (2011). Design and comparison of ex situ and in situ devices for Raman characterization of lithium titanate anode material. Ionics 17, 503–509. doi:10.1007/s11581-011-0544-4
Stancovski, V., and Badilescu, S. (2014). ChemInform abstract: in situ Raman spectroscopic-electrochemical studies of lithium-ion battery materials: a historical overview. J. Appl. Electrochem. 44, 23–43. doi:10.1007/s10800-013-0628-0
Thorne, J. S., Dahn, J. R., Obrovac, M. N., and Dunlap, R. A. (2011). An in situ study of the electrochemical reaction of Li with amorphous/nanostructured Cu6Sn5 + C. J. Electrochem. Soc. 158, A1328. doi:10.1149/2.040112jes
Thurston, T. R., Jisrawi, N. M., Mukerjee, S., Yang, X. Q., Mcbreen, J., Daroux, M. L., et al. (1998). Synchrotron x-ray diffraction studies of the structural properties of electrode materials in operating battery cells. Appl. Phys. Lett. 69, 194–196. doi:10.1063/1.117369
Villevieille, C., Sasaki, T., and Novák, P. (2014). Novel electrochemical cell designed for operando techniques and impedance studies. RSC Adv. 4, 6782–6789. doi:10.1039/c3ra46184j
Wu, J., Dathar, G. K., Sun, C., Theivanayagam, M. G., Applestone, D., Dylla, A. G., et al. (2013a). In situ Raman spectroscopy of LiFePO4: size and morphology dependence during charge and self-discharge. Nanotechnology 24, 424009. doi:10.1088/0957-4484/24/42/424009
Wu, J., Leng, G., Xu, X., Li, K., Lao, X., and Zhou, C. (2013b). In-situ synthesis of a cordierite-andalusite composite for solar thermal storage. Sol. Energy Mater. Sol. Cells 108, 9–16. doi:10.1016/j.solmat.2012.08.010
Wu, J., Shan, H., Chen, W., Gu, X., Tao, P., Song, C., et al. (2016). In situ environmental TEM in imaging gas and liquid phase chemical reactions for materials research. Adv. Mater. Weinheim 28, 9686–9712. doi:10.1002/adma.201602519
Yang, Y., Liu, X., Dai, Z., Yuan, F., Bando, Y., Golberg, D., et al. (2017). In situ electrochemistry of rechargeable battery materials: status report and perspectives. Adv. Mater. 29, 1606922. doi:10.1002/adma.201606922
Yu, Q., Wu, S., Jin, Y., Yang, S., Guo, S., Yang, S., et al. (2017). From O2− to HO2−: reducing by-products and overpotential in Li-O2 batteries by water addition. Angew. Chem. Int. Ed. Engl. 56, 4960–4964. doi:10.1002/anie.201611122
Keywords: Li-ion battery, coin cell, in situ X-ray diffraction, in situ Raman, Li4Ti5O12
Citation: Zhang L, Guo X, Huang J, Qu Y, Niu C, Du Z, Li D and Chen Y (2018) Coin-Cell-Based In Situ Characterization Techniques for Li-Ion Batteries. Front. Energy Res. 6:16. doi: 10.3389/fenrg.2018.00016
Received: 16 January 2018; Accepted: 05 March 2018;
Published: 20 March 2018
Edited by:
Da-Wei Wang, University of New South Wales, AustraliaCopyright: © 2018 Zhang, Guo, Huang, Qu, Niu, Du, Li and Chen. This is an open-access article distributed under the terms of the Creative Commons Attribution License (CC BY). The use, distribution or reproduction in other forums is permitted, provided the original author(s) and the copyright owner are credited and that the original publication in this journal is cited, in accordance with accepted academic practice. No use, distribution or reproduction is permitted which does not comply with these terms.
*Correspondence: De Li, bGlkZW5qdUBzaW5hLmNvbQ==;
Yong Chen, eWNoZW4yMDAyQDE2My5jb20=
†These authors have contributed equally to this work.