- 1Advanced Biofuels Process Development Unit, Lawrence Berkeley National Laboratory, Berkeley, CA, United States
- 2Department of Chemical and Biomolecular Engineering, University of California, Berkeley, Berkeley, CA, United States
- 3Bio-Manufacturing to Market Program at University of California, Berkeley, Berkeley, CA, United States
- 4Center for Environmental Research and Technology, University of California, Riverside, Riverside, CA, United States
- 5Department of Chemical and Environmental Engineering, Bourns College of Engineering, University of California, Riverside, Riverside, CA, United States
- 6BioEnergy Science Center (BESC), Oak Ridge National Laboratory, Oak Ridge, TN, United States
- 7Center for Bioenergy Innovation (CBI), Oak Ridge National Laboratory, Oak Ridge, TN, United States
Feedstock physical properties determine not only downstream flow behavior, but also downstream process yields. Enzymatic treatment of pretreated feedstocks is greatly dependent on upstream feedstock physical properties and choice of pre-processing Technologies. Currently available enzyme assays have been developed to study biomass slurries at low concentrations of ≤ 1% w/w. At commercially relevant biomass concentrations of ≥15% w/w, pretreated feedstocks have sludge-like properties, where low free water restricts movement of unattached enzymes. This work is an account of the various steps taken to develop a method that helps identify the time needed for solid-like biomass slurries transition into liquid-like states during enzymatic hydrolysis. A pre-processing technology that enables feedstocks in achieving this transition sooner will greatly benefit enzyme kinetics and thereby overall process economics. Through this in situ rheological properties determining method, we compared a model feedstock, Avicel®PH101 cellulose, with acid pretreated corn stover. Novozymes Cellic®CTec2 (80 mg protein/g glucan) can reduce 25% (w/w) Avicel from solid-like to liquid-like state in 5.5 h, as the phase angles rise beyond 45° at this time. The same slurry needed 5.3 h to achieve liquid-like state with Megazyme endoglucanase (40 mg protein/g glucan). After 10.8 h, CTec2 slurry reached a phase angle of 89° or complete liquid-like state but Megazyme slurry peaked only to 64.7°, possibly due to inhibition by cello-oligomers. Acid pretreated corn stover at 30% (w/w) with a CTec2 protein loading of 80 mg/g glucan exhibited a solid-like to liquid-like transition at 37.8 h, which reflects the combined inhibition of low water activity and presence of lignin. The acid pretreated slurry also never achieved complete liquid-like state due to the presence of biomass residue. This method is applicable in several scenarios comparing varying combinations of pre-processing technologies, feedstock types, pretreatment chemistries, and enzymes. Using this method, we can generate a process chain with optimal flow behavior at commercially-relevant conditions.
Introduction
Interest in alternative sources of sugar is increasing as novel approaches to biologically convert polysaccharides from renewable sources to fuels, chemicals, and materials are being devised rapidly. Lignocellulosic feedstocks have emerged as viable candidates but their biochemical deconstruction to simpler sugars requires the execution of several unit processes: harvest, storage, pre-processing, pretreatment, hydrolysis (or saccharification), conversion to fuels/chemicals (biological or chemical), and separation and purification to biofuel molecules (Satlewal et al., 2017). Enzymes employed in saccharification treatments cleave cellulose to individual long glucan chains and further hydrolyze them to simpler (monomeric and dimeric) sugars. A cocktail of synergistic cellulases is required to perform these multiple biochemical reactions. The synergy among three types of enzymes: (i) endoglucanases, (ii) exoglucanases, and (iii) β-glucosidases, produced by Trichoderma reesei, has often been used as a model to explain the mechanisms of cellulases (Agrawal et al., 2015a,b). Endoglucanase is instrumental in breaking down insoluble glucan strands to soluble oligomers (with degree of polymerization, DP < 6) and thereby reducing the viscosity of cellulose-rich solids. Exoglucanases breakdown the long chain soluble oligomers to cellobiose, which is further broken down to individual glucose units by β-glucosidase. This understanding of cellulolytic capabilities of cellulases has been developed based on research conducted for over a century, with cellulases uniquely identified in shipworms and molds in 1912. The application of cellulases in releasing lignocellulosic sugars was reckoned only in 1960 when alkali pretreated jute was found to be much more susceptible to enzymatic digestion than untreated jute, primarily due to the increased porosity of cellulose (Euler, 1912; Basu and Ghose, 1960; Selby and Maitland, 1967).
Dilute acid and ionic liquid pretreatments followed by enzymatic hydrolysis have enabled the release of >93 and 99% (of theoretical maximum, also referred to as TM) combined glucan and xylan yields from corn stover; however, these studies were conducted at low insoluble biomass concentrations (1 and 10% w/w, respectively) (Lloyd and Wyman, 2005; Li et al., 2010). Techno-economic analyses indicate that the use of higher insoluble biomass concentrations (>15% w/w) during deconstruction is critical for the economic success of commercial scale ethanol production from lignocellulosic sugars (Humbird et al., 2011; Agrawal et al., 2017). At these high insoluble concentrations (IC), with less water involved in the process, the size and thereby the capital and operating expenses of a refinery can be substantially reduced. However, high IC slurries pose significant processing problems, possibly resulting in substantial reductions of sugar yields and thereby defeating the economic cause altogether (Knutsen and Liberatore, 2010). The reduced presence of water exponentially increases viscosity of biomass, which poses a number of mass transfer challenges, especially reducing efficacy of enzymes (Geng et al., 2015). Figure S1 in Supplementary Information illustrates the impact of increasing IC on the ratio of available water to insolubles in the slurry. Video S1 was prepared to demonstrate the drastic change in rheology due to the reduced water availability on Avicel, a form of microcrystalline cellulose. Early on, Reese et al. stated that three main parameters influence enzymatic hydrolysis of cellulose: (1) hydratability, (2) particle size, and (3) pore size (Reese et al., 1950). Pretreated lignocellulosic biomass can be more hygroscopic than crystalline cellulose and can absorb water readily. However, at low water availability, pretreated biomass can be particulate in nature that can lead to the inhomogeneous application of enzymes at high IC, see Video S2. Pre-processing technologies are being devised to make feedstocks amenable to low water activity environments in pretreatment and enzymatic hydrolysis, to minimize particulate behavior and increase hydratability (Li et al., 2014). Biomass variability is another issue that pre-processing technologies can also address (Williams et al., 2016). However, such pre-processing technologies cannot be screened for performance in downstream operations with available analytical methods that are currently applicable for low concentration slurries alone (Hu et al., 2009; Goacher et al., 2012; Opitz et al., 2013; Malinowska et al., 2015).
Reese et al., as a first, correlated a drop in intrinsic viscosity with a drop in DP and progress of enzymatic hydrolysis of cellulose with enzymes from Trichoderma viride QM 6a (Reese et al., 1957). While viscosity provides useful information, rheological characterization can further this understanding through parameters, such as yield stress and viscoelastic properties (Funk and Dinger, 1994). Yield stress, the minimum stress required to induce flow in a material, has been studied substantially for both pretreated (Pimenova and Hanley, 2003; Knutsen and Liberatore, 2009; Stickel et al., 2009; Ehrhardt et al., 2010; Lavenson et al., 2011; Senturk-Ozer et al., 2011; Wiman et al., 2011; Samaniuk et al., 2012; Zhang et al., 2014) and enzymatically hydrolyzed (Roche et al., 2009; Viamajala et al., 2009; Geddes et al., 2010; Knutsen and Liberatore, 2010) feedstocks. But this parameter can be measured only on offline samples and not always in real time. While a few other authors (Knutsen and Liberatore, 2009; Stickel et al., 2009; Wiman et al., 2011) reported substantial studies on viscoelastic properties of biomass, which are ascertained through oscillatory testing, there have been no reports of real-time viscoelastic property measurements of feedstock hydrolysis. As such, no information on changes in physical properties of the high IC feedstock slurry occurring throughout an enzymatic treatment time is available. Viscoelastic parameters can differentiate solid-like and liquid-like behavior of a material and measuring them for enzymatic hydrolysis slurries gives an opportunity to quickly compare pre-processing technologies for viscosity reduction and faster transition to liquid-like behaviors in high IC slurries and thereby potential for improving biomass feed and overall economics. At the Advanced Biofuels Process Demonstration Unit (ABPDU), we developed an in situ viscoelastic property determining method that displays, in real time, the transition of high IC microcrystalline cellulose and other cellulose-rich materials from solid-like to liquid-like state during enzymatic hydrolysis with various cellulases.
Materials and Methods
Rheology, Viscoelastic Properties, and Rheometer
Rheology can be used to classify materials as: elastic (ideal solids), viscous (ideal fluids), or viscoelastic, see Figure S2. Typically, most materials lie between the ideal scenarios of elastic and viscous states and are called viscoelastic materials, characterized through three interrelated viscoelastic parameters: elastic modulus, viscous modulus, and phase angle (G′, G″, δ), see Equation 1. If stress and strain are plotted against a time period, the lag observed between these vectors is called phase angle, see Figure S2E. In simpler words, viscoelastic materials that are more viscous, or liquid-like, exhibit a phase angle above 45°, where the lag is higher and can rise up to 90°. For completely Newtonian-type fluids. This concept is applied throughout this study to depict changing viscoelastic properties of the high IC biomass slurries (Funk and Dinger, 1994).
where, G*, G′, and G″ are complex, elastic, and viscous moduli; σ and ε are stress and strain, and δ is phase angle.
In this study, rheological characterization of all samples was conducted on a Kinexus Pro+ oscillatory stress controlled rheometer (Malvern Instruments Ltd., Worcestershire, UK) with Peltier cartridges, both cylinder and plate type. The cartridges are able to reach 200°C with a stability of ±0.1°C controlled by feedback from PT100 sensors and since cellulases are mostly active at 50°C, all studies were conducted at this temperature in situ the rheometer. Rheometers are generally designed to test homogenous material where particle-edge effects, settling, and other such issues from insolubles in slurries are not expected to pose a problem. This study was focused on developing a novel method to study rheology of particulate pretreated biomass samples in real-time.
Substrates, Sample Preparation, and Enzymatic Hydrolysis
Preliminary study was performed on switchgrass (Panicum virgatum), generously provided by Dr. Putnam from UC-Davis, pretreated with an ionic liquid (IL), EmimAcetate (purity ≥90%, BASF, Ludwigshafen, Germany) (Li et al., 2013). Biomass was stored at 4°C prior to milling and sieving to 20-20 mesh size. Later, Avicel®PH101 cellulose (11363 Fluka, Sigma Aldrich, Milwaukee, WI), with a particle size of 200 μm, was chosen as a model material for this study. Two mixtures of (i) Avicel with xylan from beechwood (TCX0064, VWR International, TCI America, Portland, OR) at a 2:1 mass ratio and (ii) Avicel with xylan and alkali treated lignin (VWR International, TCI, Japan) at 5:3:2 mass ratio were also tested. Avicel®PH101 (11365 Fluka, Sigma Aldrich, Milwaukee, WI) with particle size of 50 μm were tested and compared with the model material. Finally, corn stover, kindly provided by Idaho National Laboratory, pretreated in dilute acid at UC-Riverside, and analyzed for composition at the ABPDU was used to test this method for application on lignocellulosic biomass, see Table S1 for composition (Sluiter et al., 2008a,b). Dilute acid pretreatment was carried out at 5% (w/w) biomass concentration on a dry basis at a temperature of 140°C for 40 min. Corn stover was soaked overnight at room temperature in 1% (w/w) sulfuric acid and then pretreated in a 1 L Parr® (Parr Instruments, Moline, IL) batch reactor at an impeller speed of 200 rpm. The reactor was heated in a fluidized sand bath (SBL-2D, Techne Corp., Princeton, NJ) maintained at 350°C for rapid heating. To stop the pretreatment, the reactor was quickly removed from the sand bath and quenched in water at room temperature. The pretreated corn stover was recovered over Whatman® No. 1 filter paper after washing with about 15 reaction volumes of deionized water.
Cellic®CTec2 and Cellic®Htec2 (Novozymes, Davis, CA), and endoglucanase derived from Aspergillus niger (EC 3.2.1.4, Megazyme International Ireland, Ireland) were tested at either 40 or 80 mg/g glucan. CTec2 protein concentration, per Pierce BCA protocol, was observed to be 190 mg protein/ml enzyme (Smith et al., 1985). Each rheological test was performed on a batch of sample prepared to be a total weight of 100 g. Solid components were analyzed for moisture content and weighed to achieve final predetermined dry weight. A liquid mixture containing 50 mL of 0.1 M citrate buffer (pH 5.4), 1 ml of 2% (w/w) sodium azide solution, and pre-determined enzyme loading was prepared prior to addition to solid fraction (Selig et al., 2008). The liquid mixture and solid components were added to a beaker and topped off with water to achieve the total weight of 100 g and a pH of 5.5. This batch was thoroughly mixed for homogeneity and equally divided into two portions, one was added to the cup of the rheometer for viscoelastic property measurements only. The other portion was added to a shake flask that mimicked the enzymatic hydrolysis treatment conditions (50°C and corresponding mixing) in the rheometer. Samples for sugar analysis were drawn from the shake flask.
Method Development on the Rheometer
The geometric attachments on the rheometer tested in this study included: parallel plate and vane-in-cup geometries, see Figure 1. Linear viscoelastic regions were determined for pretreated biomass before enzyme addition. Stress and frequency sweeps were conducted to identify the linear viscoelastic region to be below 10 Hz and 10 Pa. It is essential for us to perform all rheological property measurements within the linear viscoelastic range as no universal rheological constitutive equation can predict the behavior of the material in the non-linear range. These parameters were used as a starting point for the method. Method development was particularly challenging due to bulk density of material, settling issues, sample weeping, evaporation, and choice of oscillation frequency and amplitude throughout the treatment process. Since the sample was enclosed inside the cup of the rheometer, most often, we were unable to detect a failure due to aforementioned issues until after the tests were complete, usually after 24 h, making the method development process very time consuming. Trials conducted in developing the method are described in sections “Preliminary Study” and “Avicel as Model Material” through “The Challenge of Prolonged Viscoelastic Property Measurements” and Table 1 is a summary of some key test results that led to go/no-go decisions through the method development process. While the progress of method development was protracted, much of the information from the failed trials could be applicable in comprehending viscoelastic properties of insolubles in several other scenarios.
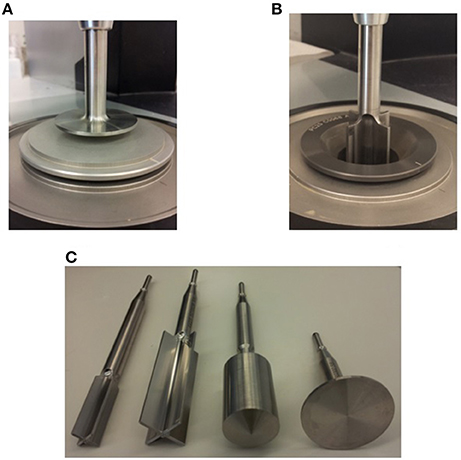
Figure 1. Various geometries and attachments used in this study; (A) Plate-Plate Geometry, (B) 25 mm Vane-in-Cup Geometry, and (C) 14 mm Vane, 25 mm Vane, 25 mm Bob, and 40 mm Plate Attachments.
Shake-Flask Internal Mixing and Sampling
The shake flask in the incubator with the biomass sample was initially set at 150 rpm. Since the mixing in this system is only external, a slower viscosity drop of the biomass sample was observed. On the other hand, faster viscosity drop was observed in the cup of the rheometer due to internal mixing from the vane geometry, internal mixing and a faster viscosity drop of the biomass were observed in the cup of the rheometer. To rectify this incoherence, up to 4 ball bearings were tested in the flask (see section Harmonizing with Shake Flask Studies). Every 3 h, approximately 0.5 g of sample was removed from the shake flask and exactly 0.25 g of this sample was weighed and diluted with water to 2.5 g on the balance, a 10 times mass dilution required to obtain enough liquid sample that could be filtered through 0.2 μm PTFE centrifugal filters. This mass to mass sampling was essential for the initial high viscosity samples that did not yield any free water with the sugars. Even so, any sugar-rich free water would be more concentrated with the soluble sugars and thereby not being representative of the entire batch (Kristensen et al., 2009). Samples were prepared in triplicate and stored at −20°C until necessary for High Performance Anion Exchange Chromatography (HPAEC) analysis (Tanjore et al., 2009). Further dilutions were performed when required to fit the range of HPAEC standards and detection.
HPAEC Sugar Measurement
Glucose (G1), cellobiose (G2), cellotriose (G3), cellotetraose (G4), cellopentaose (G5), cellohexaose (G6), and xylose (X1) were measured in this study. Determination of mono- and oligo-saccharide content was performed on Dionex ICS-3000® system (Thermo Scientific, Waltham, MA) equipped with a Dionex ED40® electrochemical detector. Separation was obtained using CarboPac® PA200 analytical (3 mm × 250 mm) and guard (2 mm × 50 mm) columns heated to 30°C (Thermo Scientific, Waltham, MA). Elution was performed using a gradient program (0–14% eluent B) over 18 min with 0.1 M NaOH (eluent A) and 0.5 M NaOAc containing 0.1 M NaOH (eluent B). Analytes were measured via pulsed amperometric detection (PAD) using a standard quadruple waveform.
Samples containing xylan required separation of glucose and xylose, which co-elute on the PA200 column. These samples were concurrently run on an Ultimate 3000 HPLC® system (Thermo Scientific, Waltham, MA) equipped with a Shodex Refractive Index® detector (Shoko Scientific Co., Ltd., Yokohama, Japan). Separation was obtained using an Aminex HPX-87H® analytical column (7.8 mm × 300 mm) (Bio-Rad, Hercules, CA) heated to 65°C. Isocratic elution was performed using freshly prepared 0.005 M sulfuric acid over 10 min with a 4-min wash step with 0.025 M sulfuric acid and re-equilibration between injections. Integration and analysis of samples was performed using Dionex Chromeleon® software (Thermo Scientific, Waltham, MA). Identification of mono- and oligo-saccharide content was determined relative to known standards (Megazyme International Ireland, Wicklow, Ireland). All monomeric and oligomeric yields were calculated as a ratio to their polysaccharides, either glucan or xylan, see Table S2 in Supplementary for stoichiometric equations.
Results and Discussion
Preliminary Study and Avicel as Model Material
With the availability of IL pretreated switchgrass (Li et al., 2013), we started rheological testing at 18% IC using the long 25 mm Vane-in-cup geometry at a 5 mm gap. In this preliminary study, only oscillation measurements were performed to determine the changing viscoelastic properties of the IL pretreated biomass. At the beginning, the material had a very low bulk density, 100 kg/m3, which gradually increased as the material was liquefied through enzymatic hydrolysis leading to a drop in the overall volume of the slurry. Although this test provided promising results during the initial stages of the experiment, the drop in the volume and thereby the level of biomass within the cup inevitably invalidated the use of long vane for reliable measurements. The vane geometry should be completely covered throughout the course of the study to avoid edge effects and provide repeatable and reliable data, see Figure S3 in Supplementary. Accordingly, a short vane (14 mm vane size) was purchased to develop the method without the risk of sample dropping below the vane's height in the cup. However, even the shorter vane was too long for these studies.
IL pretreatment renders biomass to be highly porous and hygroscopic. Even though the regenerated IL pretreated biomass had a large particle size (2–5 mm), the solid phase absorbed the aqueous phase almost instantly and remained suspended through enzymatic hydrolysis. However, crystalline cellulose, which represents the majority of commercially-available pretreated biomass, separates from the aqueous phase and settles. While an increase in bulk density of biomass through enzymatic hydrolysis could continue to be an issue with crystalline cellulose, several more variables exist that could influence establishing the applicable method to determine viscoelastic properties of all types of pretreated biomasses during enzymatic hydrolysis. As we have found and others reported (Palmqvist and Lidén, 2012; Tanjore et al., 2013), variations in feedstock type, pre-processing methods, pretreatment catalyst, and pretreatment severity all have a strong influence on the progress of enzymatic hydrolysis and cannot be underestimated while measuring viscoelastic properties. To develop a method that can be applied on samples of biomass from such a diverse set of unit operations, we chose Avicel as our model material. Without the influences of a particular pretreatment and/or choice of feedstock, a method based on Avicel, a more “pure” substrate, allowed us to closely examine enzymatic activity and its influence on viscoelastic properties and was more likely to yield reliable data from repeatable and consistent measurements.
Vane-In-Cup Geometry Was Most Suitable
We started with Avicel at a high IC of 35% (w/w), treated with 40 mg protein of CTec2/g glucan, and tested in 14 mm Vane-in-cup geometry with a 5 mm gap (z2). At 35% IC, Avicel slurry was not very amenable and would not uniformly spread in the cup of the reactor, see Video S1B in Supplementary. Several air gaps and drastic drop of slurry volume led us to lower insoluble concentrations. At 30% IC, even though Avicel had some free water in its system, it was much lower than in hydrolyzed IL pretreated biomass. This led to a lower drop in bulk density and thereby slurry height. However, a settling issue that we did not observe in IL pretreated switchgrass emerged during the test with 30% IC of Avicel. The high hygroscopicity and porosity of IL pretreated switchgrass allowed it to absorb and remain suspended uniformly in the slurry, which was not the case with the more granular and crystalline Avicel. Later, we found this suspension of biomass in slurries to be true for acid pretreated biomass as well. While IL pretreated switchgrass-water suspensions are apposite to consistent viscoelastic property measurements, we wanted to develop a method that was applicable to a broad array of feedstock-preprocessing combinations and thereby were bound to tackle the settling issue.
Initially, we attempted to manually mix the sample every hour, but settling reoccurred within 10 min of the mixing process. This mixing method was further erratic because of differences in manual mixing hour by hour, each time causing a small but noticeable change in phase angle. Since the initial consistency of sample was very thick at 30% IC, plate-on-plate geometry seemed to be a better choice to help overcome the settling issues and eliminate the effect of internal mixing occurring from the vane geometry in the cup. The very narrow gap between the plates (2 mm) on the rheometer allow for oscillation, but do not cause any internal mixing, much like in the shake flask. This method seemed to work very well for the first 12 h, but after this time period, as hydrolysis proceeded, the soluble and insoluble components of the mixture would separate. The solubles along with the water would leak out the sides of the plates. To be able to perform viscoelastic measurements longer than 12 h, we reverted to the vane-in-cup geometry. This time, to maintain the insolubles suspended in the mixture, continuous rotational viscosity measurements were incorporated into the sequence between intermittent oscillatory measurements. By combining both viscosity and viscoelastic measurements (30 s of oscillation followed by 5 min of rotational mixing), we created a continuous method that mitigated the settling issues. Though we obtained substantial viscosity data at a constant shear rate of 150 s−1, the primary objective of incorporating rotational measurements was to maintain continuous mixing in the rheometer cup and keep the insolubles suspended. However, this improved internal mixing of the biomass led to a poor correlation between rheology of the samples in the cup and shake flask.
Harmonizing With Shake Flask Studies
To ensure that glucose measurements from shake flask would be representative of those from the rheometer cup, it was necessary that there was no discord between progresses of enzymatic hydrolysis in both vessels. Initially, Avicel samples in the rheometer cup exhibited a higher phase angle than the material in shake flask, indicating that it was more liquid-like, primarily due to internal mixing from the viscosity measurements. Even when operating at 200 rpm, the laboratory shaker could not deliver the increase in phase angle as the rheometer set up as the shake flask can only provide external mixing. This external mixing was detrimental toward drop in sample viscosity, especially when at high IC of 30%. The addition of 1–4 steel ball bearings in each shake-flask along with external shaking was tested to create a mechanism for internal mixing. But, even with four ball bearings, there was very little drop in viscosity as 30% IC would not allow the ball bearings to move, well up to 12 h. At this IC, the samples were very thick and had a very paste-like quality, so the ball bearings were simply trapped inside the sample and did not rotate until hydrolysis proceeded enough to release the liquid phase.
After performing several tests at the 28% IC, we reduced the insolubles in the sample to 25% IC. Only at 25% IC or less, the ball bearings were moving appropriately in the shake flask from the start of the experiment, enforcing this concentration as our final choice of IC. However, more than one ball bearing was leading to shear in the shake flask samples, now leading to a faster than preferable drop in viscosity and, once again, lack of correspondence with the behavior of the sample in rheometer. To avoid any shear related influences, we proceeded with a single ball bearing at 25% IC that matches the rheological profile in the rheometer. We proceeded with this set up to find out that our sample in the rheometer began to settle at the low initial IC even when the rotational sequence was set up as high as 200 s−1. The short vane was not effective in suspending the insolubles at 25% concentration. Luckily, the bulk density of Avicel at this concentration was much higher and the drop in slurry height was minimal. Consequently, we returned back to the long (25 mm) vane, which was adequate in preventing settling when used with the combined sequence of oscillatory and rotational parts at 150 s−1, as described earlier.
Despite the advantages of reduced settling and enhanced mixing, the long vane presented a new set of challenges. Applying the parameters optimized for the short vane on method with the long vane would consistently push the slurry phase angle to 90° (completely Newtonian) before the shake flask appeared liquid-like. With the short vane, our oscillatory measurements were conducted at oscillatory frequency and amplitude of 10 Hz and 10 Pa. After a few optimization experiments, we found that oscillation at 5 Hz and 5 Pa led to a reliable and replicable rheological method with the long vane, but only up to 24 h, beyond which, evaporation of water from the slurry in the rheometer cup annulled our efforts.
The Challenge of Prolonged Viscoelastic Property Measurements
Most viscoelastic property measurements are performed off-line, within a time period of a few min. These offline measurements can still be conducted real time for a few predetermined samples, if the timeframe of test completion is within a few hours, e.g., hydrolysis of Solka Floc with cellulases. With the rate limiting action of endoglucanase, real time and in-line measurement of viscoelastic properties of crystalline cellulose during enzymatic hydrolysis for about 72 h was very challenging. Although very little volume loss was observed during the hydrolysis of Avicel at 25% IC, much of it was noticeably lost to evaporation after 24 h. Initially, to contain the water in the slurry, a layer of lighter density oil was added after setting the vane in the cup with the slurry (Klinkesorn et al., 2004). But this oil would gradually mix into the entire sample during rotational viscosity measurements. A heavier vacuum grease was the next alternative tested to create a seal sufficient to prevent evaporation (Sun and Gunasekaran, 2009). Although the grease worked a few times, ultimately the system was not replicable leaving us to seek out a different alternative. After several trials, we learned that, to obtain a replicable and reliable method and alleviate much of the difficulties we had previously experienced with moisture loss, we will have to curtail our measurements to a maximum of 24 h.
A time frame of 24 h was fast enough to avoid any uncertainty around evaporation yet slow enough to generate useful data needed to compare feedstocks, processing technologies, and enzymes. We doubled the enzyme loading to 80 mg protein/g glucan to evade any enzyme inhibition related issues and achieve the fastest possible hydrolysis for the substrate. Although this number represents a much higher enzyme loading than would be used in industrial applications, it provided a means to replicate the studies in the absence of evaporation issues. Oscillation frequency and amplitude of 5 Hz and 5 Pa were, once again, too vigorous for this higher enzyme loading where hydrolysis was complete within 2 h, while measurements using 1 Hz and 1 Pa required more than 12 h to reach a phase angle of ~45°. Oscillation frequency and amplitude for this system was optimized down to 3 Hz and 3 Pa. Finally, we established a rheological test that included continuous 5 min of rotational viscosity measurement at 150 s−1 followed by oscillatory test at 3 Hz and 3 Pa on 25% IC of Avicel at 50°C with an 80 mg protein/g glucan CTec2 loading.
With a defined process for replicable hydrolysis occurring in similitude in both the shake flask and in the rheometer, we were now ready to apply it for the viscoelastic property characterization of different enzyme cocktails. As a first step, we simply triplicated our test using 25% IC of Avicel with 80 mg protein/g glucan enzyme loading using Novozymes CTec2. CTec2 was selected because it is a complete enzyme complex (endoglucanases, exoglucanases, and β-glucosidases) for cellulose breakdown. We found that the material transitioned into liquid-like state within 5.5 h and achieved near Newtonian-behavior at roughly 10 h, when the phase angle of the sample surpassed 45° and reached 90°, respectively, see Figure 2A with glucose concentrations a little lower than 31 and 44.8% (of TM), respectively. Another approach to find this point of transition is to follow the profile of elastic (G′) and viscous (G″) moduli, see Figure 2B. G′ and G″ represent the solid and liquid-like behaviors of the material. As long as G′ was higher than G″, the material was still in the solid-like state. Once G″ crossed over the profile of G′ and observed to be stronger than G′, then the material had transitioned into the liquid-like state. Also, during this transition, complex modulus (G*), shifts from shadowing the profile of G′ to that of G″. Throughout the entire hydrolysis process, this substrate-enzyme combination exhibited minimal detection of gluco-oligomers; cellobiose was the only quantifiable oligomer and was only detected during the first 3 h of hydrolysis. Total yield, as calculated by the sum of glucose and cellobiose at 96 h, was 85% (of TM).
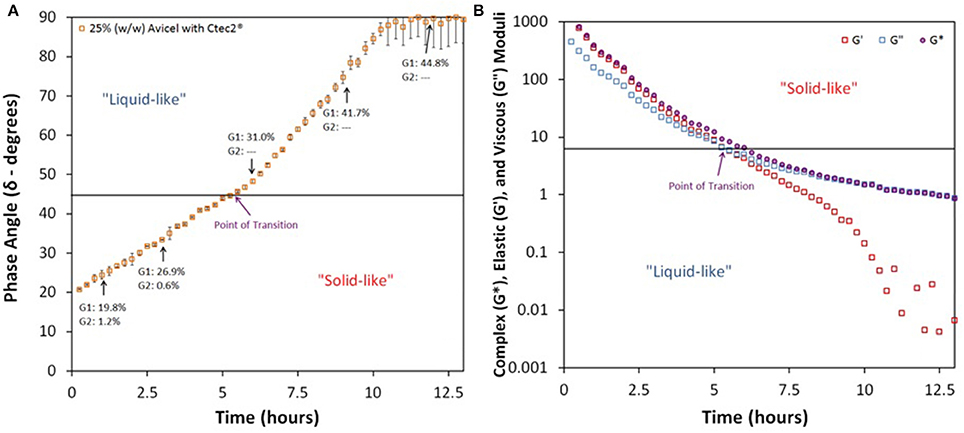
Figure 2. Profiles of (A) Phase Angle and (B) Complex (G*), Elastic (G′), and Viscous Moduli (G″) during enzymatic hydrolysis of Avicel; 25% (w/w) Avicel was treated with Novozymes Cellic® CTec2 at a loading of 80 mg protein/g glucan; G1, Glucose and G2, Cellobiose.
Rheological Testing Was Applicable With Other Enzymes and Substrates
In contrast to our method-establishing test, when Avicel at 25% IC was treated with only Megazyme's endoglucanase at 40 mg protein/ g glucan, a complete Newtonian-like behavior or 90° phase angle was never achieved, see Figure 3A. Since endoglucanase intrinsically hydrolyzes long chained polysaccharides only to cellobiose and not to glucose, we expected a slower transition from solid to liquid and a lower total yield. Surprisingly, as shown in the figure, we observed that endoglucanase alone can also surpass a phase angle of 45° in roughly 5.3 h. However, as expected, the final sugar yield (calculated by the sum of quantifiable oligomers) after 96 h of treatment was only 61% (of TM). Furthermore, the rise in phase angle begins to significantly taper off at roughly 7.5 h and never reaches complete Newtonian state. Again, this result was expected, reaffirming that endoglucanase alone is not sufficient for complete transition to Newtonian liquid-like behavior, let alone complete hydrolysis. The presence of a high concentration of oligomers (G2 = 13.8, G3 = 16.5, G4 = 1.1%, of TM) could have inhibited the activity of endoglucanase (Xue et al., 2015). Previously performed wet chemistry tests (Xiao et al., 2004; Hodge et al., 2009) and molecular simulations (Payne et al., 2011) have established such inhibition of enzyme activity, but through our method we were able to obtain this information in real time and potentially identify the onset of inhibition by comparing with CTec2 profile.
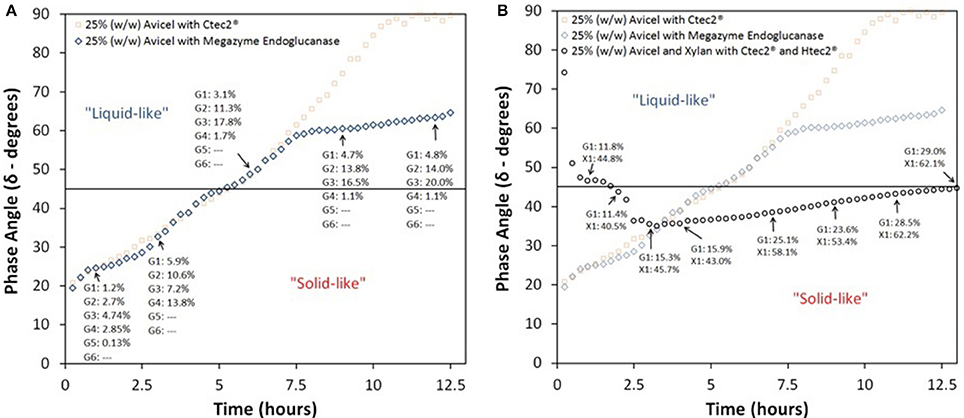
Figure 3. Phase angle profile of enzymatic hydrolysis of Avicel and Avicel-Xylan mixtures treated with various enzymes; (A) 25% (w/w) Avicel treated with only Megazyme endoglucanase at 40 mg protein/glucan, and (B) 25% (w/w) Avicel and Xylan (2:1) with Novozymes Cellic® CTec2 and HTec2 at protein loadings of 80 and 8 mg protein/g glucan, respectively; G1, Glucose; G2, Cellobiose; G3, Cellotriose; G4, Cellotetraose; G5, Cellopentaose; G6, Cellohexaose; and X1, Xylose.
Another inhibition type detected was the effect of xylan and xylooligomers on enzymatic hydrolysis of cellulose. It is known that hemicellulose polysaccharides, their oligomers, and xylose inhibit cellulase enzyme (Qing et al., 2010; Kumar and Wyman, 2014). When Avicel was mixed with xylan in a composition similar to what is expected in plant cell-wall (2:1 ratio) and treated with HTec2 along with CTec2, we initially observed a very high phase angle, shown in Figure 3B. This is a surprising find as hemicellulose is most hygroscopic among the three constituents of the plant cell wall (Acharjee et al., 2011). We expect that the lack of hydrogen bonding between xylan and glucan in our slurry mixtures makes it substantially different from pretreated biomass. Interestingly, from the start of the experiment, the phase angle of Avicel together with xylan mixture started to drop and stayed low for a 12 h time period and does not reach liquid-like state until after this time period. Xylose yields reached 62.1% (of TM) at 13 h but glucose yields were low at 29% (of TM). Glucose yields followed the expectation, per phase angle, i.e., glucose yield from Avicel at phase angle of 45° was about 30% (of TM). As expected, due to the enzyme complex, we did not see any oligomers in the samples, not even those from xylan. This finding bolstered our concept that viscoelastic properties of high IC slurries are good indicators of ongoing kinetics.
To further test this concept, we chose to vary composition of slurry by including lignin into the mix. Interestingly, Avicel with xylan and lignin at 28% IC had a profile somewhere between Avicel with xylan and Avicel alone, see Figure S4 in Supplementary. At initiation, the phase angle of this mixture was high but soon fell to near solid-like state, similar to that of the Avicel with xylan mixture, but then started to increase at a rate similar to that of Avicel, i.e., the hydrolysis process was more rapid in the presence of lignin. At low ICs, the addition of extracted lignin to an enzymatic hydrolysis slurry had shown to increase sugar yield from enzymatic hydrolysis (Nakagame et al., 2011). At high IC, improved rheology can be another motive for such additions. Apart from chemical composition, we also tested two separate particle sizes (200 and 50 μm) of Avicel and as expected, the slurry with smaller particle sized Avicel reached liquid-like state in much lesser time. The 50 μm Avicel required only half the time (0.67 h) of that of 200 μm Avicel (1.42 h) to achieve liquid-like state.
To further our understanding of rheological changes of cellulose-rich lignocellulosic materials during enzymatic hydrolysis, we tested acid pretreated corn stover at 30% IC. Biomass itself is much more hygroscopic than Avicel, compare Videos S1, S3 in Supplementary. Even though acid pretreatment renders biomass less hygroscopic than IL pretreatment does, acid pretreated biomass is still more hygroscopic than Avicel. Therefore, interestingly, at 30% IC of acid treated corn stover, evaporation issues were substantially mitigated as water was very much trapped in the insolubles, see Figure 4. We were able to test the high IC corn stover hydrolysis for a prolonged time but due to low water activity along with presence of insoluble cellulose, we were not able to achieve near Newtonian behavior, see Figure 5. To estimate the maximum corn stover insolubles concentration that can be administered at the initiation of enzymatic hydrolysis, without creating mass transfer issues and affecting the kinetics of the process, it will be necessary to perform rheological tests at several high IC levels. But once such a concentration level that is conducive for enzymatic hydrolysis is established, we can continue to add untreated biomass into a partially hydrolyzed slurry to re-use the released cellulase enzymes and increase the concentration of sugars in the recovered aqueous phase.
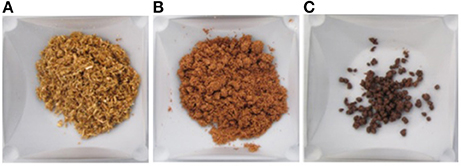
Figure 4. Corn stover at various stages of treatment; (A) Untreated (89% w/w lnsolubles Concentration), (B) Dilute acid pretreated (36% w/w lnsolubles Concentration), and (C) Enzymatically hydrolyzed (Final Insolubles Concentration after 24 h was 28% w/w; Initial Insolubles Concentration was 30% w/w).
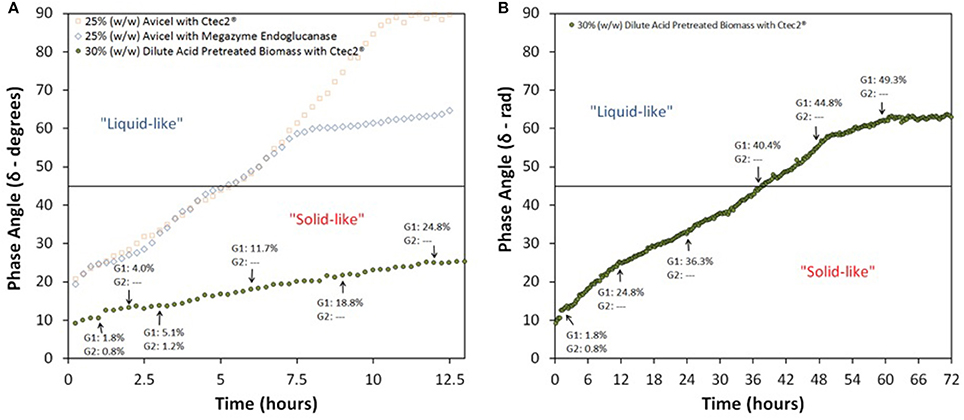
Figure 5. Phase Angle profile of enzymatic hydrolysis of 30% (w/w) dilute acid pretreated corn stover; Enzymatic hydrolysis was conducted with 80 mg protein/g glucan of Novozymes Cellic® CTec2 up to (A) 12.5 h and (B) and 72 h; G1, Glucose and G2, Cellobiose.
Perspective
Our understanding of pre-processing technologies and pretreatments of biomass and their influence on enzymatic hydrolysis of the recovered cellulose-rich materials has increased substantially in the past decade. The effects of lignin modification, lignin re-deposition, xylooligomers concentration, and several other factors have been studied extensively, but typically only at low ICs (Selig et al., 2007; Qing et al., 2010; Sannigrahi et al., 2011). In this study, we have been able to show that viscoelastic property measurements of high IC slurries can potentially provide several useful insights that can improve the prospects of commercial cellulosic sugar production.
Techno-economic analyses indicate that, apart from IC, enzyme production and purification are some of the top contributors to cost of lignocellulosic sugars. Achieving high sugar yields during saccharification, and recycling enzyme after, can tip the scales for economic success of lignocellulosic sugar-based products. As biomass is hydrolyzed, viscosity drop follows and the enzyme attached to a binding site on glucan can be freed back into the aqueous phase. The released enzyme can re-operate on remaining biomass by binding to a new site, possibly at a much faster rate due to a higher percentage of free water (Hodge et al., 2009; Geng et al., 2015). By comparing several pre-processed and pretreated biomass samples, we can identify the upstream technology that can enable a higher release of enzymes. Furthermore, after a certain level of hydrolysis is achieved, a pulsed feed of new pretreated biomass can be administered to the reactor. Such a fed-batch process can be effective only when care is taken that the new batch of hygroscopic biomass does not create enzyme mass transfer issues (Liu et al., 2015). With phase angle data from our rheological testing, along with further optimization, it will be possible to identify a suitable time for administering the new batch. Once again, if a pre-processing technology leads to faster addition of batches of pretreated biomass, it will be advantageous in providing higher concentrations of sugar in the liquid phase. Instead of measuring sugar release in real time, which is possible through already available in situ methods such as FT-NIR (Gierlinger et al., 2008), we developed a method to identify the time required to achieve liquid-like behavior, without performing any wet chemistry based analysis. This method is not mitigated by background effects that are inevitable when varying feedstock type and impact spectroscopic methods. Rheological behavior and hygroscopicity of biomass most definitely vary with feedstock type, harvest time, pre-processing methods, pretreatment chemistry, and other upstream events (Palmqvist and Lidén, 2012). However, feedstock or even enzyme variation is not a limiting factor for the method presented in this study as phase angle is a unit-less measurement of the behavior of a slurry. Real-time rheological testing can potentially provide unpredicted information that is not easily obtained through wet chemistry tests on samples that are taken on a predetermined schedule.
Conclusions
We developed widely applicable real-time rheological assessment of cellulases in high IC slurries, which are particularly challenging to test through wet chemistry methods. Transitioning viscoelastic properties of cellulose-rich insolubles at high concentrations correlated well with kinetics expected from previously established theories around enzyme inhibitions due to lack of water activity and presence of oligomers, monomers, and lignin. Through our method, it is possible to test multiple pre-processing and pretreatment technologies and cellulases such as endoglucanases for their ability to quickly convert a high IC solid-like slurry to liquid-like state. Such a faster transition can lead to higher availability of free water, reduced mass transfer limitations, and improved kinetics.
Author Contributions
PC and JG designed analytical studies and PC performed them; DT and NM devised and performed the rheology experiments and performed them; RK, SB, CW devised pretreatment studies and SB performed them; PC, DT, RK, and JG wrote the manuscript. All authors read and approved the final manuscript.
Funding
This report is based upon work supported by the U.S. Department of Energy. We would like to thank Energy Efficiency and Renewable Energy (EERE) Division's BioEnergy Technologies Office (BETO) for the funding required to pursue this work. This work was also supported, in part, by the BioEnergy Science Center (BESC) (Contract DE-PS02-06ER64304) and Center for Bioenergy Innovation (CBI) administered by Oak Ridge National Laboratory (ORNL) from DOE BER Office of Science. Another form of support came from the Bio-Manufacturing to Market student internship program at UC Berkeley, which as funded by the DOE EERE under Award No. DE-EE0006026 as a member of The Advanced Manufacturing Jobs and Innovation Accelerator Challenge. The views and opinions of the authors expressed herein do not necessarily state or reflect those of the United States Government or any agency thereof. Neither the United States Government nor any agency thereof, nor any of their employees, makes any warranty, expressed or implied, or assumes any legal liability or responsibility for the accuracy, completeness, or usefulness of any information, apparatus, product, or process disclosed, or represents that its use would not infringe privately owned rights.
Conflict of Interest Statement
The handling Editor declared a past co-authorship with one of the authors, DT.
The remaining authors declare that the research was conducted in the absence of any commercial or financial relationships that could be construed as a potential conflict of interest.
Acknowledgments
We thank Drs. Vicki Thompson, Kevin Kenney, and Chenlin Li from the Idaho National Laboratory (INL) for providing biomass feedstocks tested in this project. We appreciate the generous gift of Cellic CTec2 and HTec2 from Novozymes.
Supplementary Material
The Supplementary Material for this article can be found online at: https://www.frontiersin.org/articles/10.3389/fenrg.2018.00053/full#supplementary-material
References
Acharjee, T. C., Coronella, C. J., and Vasquez, V. R. (2011). Effect of thermal pretreatment on equilibrium moisture content of lignocellulosic biomass. Bioresour. Technol. 102, 4849–4854. doi: 10.1016/j.biortech.2011.01.018
Agrawal, R., Gaur, R., Mathur, A., Kumar, R., Gupta, R. P., Tuli, D. K., et al. (2015a). Improved saccharification of pilot-scale acid pretreated wheat straw by exploiting the synergistic behavior of lignocellulose degrading enzymes. RSC Adv. 5, 71462–71471. doi: 10.1039/C5RA13360B
Agrawal, R., Satlewal, A., Gaur, R., Mathur, A., Kumar, R., Gupta, R. P., et al. (2015b). Pilot scale pretreatment of wheat straw and comparative evaluation of commercial enzyme preparations for biomass saccharification and fermentation. Biochem. Eng. J. 102, 54–61. doi: 10.1016/j.bej.2015.02.018
Agrawal, R., Satlewal, A., Kapoor, M., Mondal, S., and Basu, B. (2017). Investigating the enzyme-lignin binding with surfactants for improved saccharification of pilot scale pretreated wheat straw. Bioresour. Technol. 224, 411–418. doi: 10.1016/j.biortech.2016.11.026
Basu, S., and Ghose, S. (1960). The production of cellulase by fungi on mixed cellulosic substrates. Can. J. Microbiol. 6, 265–282.
Ehrhardt, M., Monz, T., Root, T., Connelly, R., Scott, C., and Klingenberg, D. (2010). Rheology of dilute acid hydrolyzed corn stover at high solids concentration. App. Biochem. Biotech. 160, 1102–1115. doi: 10.1007/s12010-009-8606-z
Funk, J., and Dinger, D. (1994). “Viscosity and rheology,” in Predictive Process Control of Crowded Particulate Suspensions (New York, NY: Springer Science +Business Media, LLC), 235–252. doi: 10.1007/978-1-4615-3118-0_20
Geddes, C. C., Peterson, J. J., Mullinnix, M. T., Svoronos, S. A., Shanmugam, K., and Ingram, L. O. (2010). Optimizing cellulase usage for improved mixing and rheological properties of acid-pretreated sugarcane bagasse. Bioresour. Technol. 101, 9128–9136. doi: 10.1016/j.biortech.2010.07.040
Geng, W., Jin, Y., Jameel, H., and Park, S. (2015). Strategies to achieve high-solids enzymatic hydrolysis of dilute-acid pretreated corn stover. Bioresour. Technol. 187, 43–48. doi: 10.1016/j.biortech.2015.03.067
Gierlinger, N., Goswami, L., Schmidt, M., Burgert, I., Coutand, C., Rogge, T., et al. (2008). In Situ FT-IR microscopic study on enzymatic treatment of poplar wood cross-sections. Biomacromolecules 9, 2194–2201. doi: 10.1021/bm800300b
Goacher, R. E., Edwards, E. A., Yakunin, A. F., Mims, C. A., and Master, E. R. (2012). Application of time-of-flight-secondary ion mass spectrometry for the detection of enzyme activity on solid wood substrates. Anal. Chem. 84, 4443–4451. doi: 10.1021/ac3005346
Hodge, D. B., Karim, M. N., Schell, D. J., and McMillan, J. D. (2009). Model-based fed-batch for high-solids enzymatic cellulose hydrolysis. App. Biochem. Biotech. 152, 88–107. doi: 10.1007/s12010-008-8217-0
Hu, G., Heitmann, J. A., and Rojas, O. J. (2009). Quantification of cellulase activity using the quartz crystal microbalance technique. Anal. Chem. 81, 1872–1880. doi: 10.1021/ac802318t
Humbird, D., Davis, R., Tao, L., Kinchin, C., Hsu, D., Aden, A., et al. (2011). Process Design and Economics for Biochemical Conversion of Lignocellulosic Biomass to Ethanol. Technical Report, National Renewable Energy Laboratory.
Klinkesorn, U., Sophanodora, P., Chinachoti, P., and McClements, D. J. (2004). Stability and rheology of corn oil-in-water emulsions containing maltodextrin. Food Res. Int. 37, 851–859. doi: 10.1016/j.foodres.2004.05.001
Knutsen, J. S., and Liberatore, M. W. (2009). Rheology of high-solids biomass slurries for biorefinery applications. J. Rheol. 53, 877–892. doi: 10.1122/1.3143878
Knutsen, J. S., and Liberatore, M. W. (2010). Rheology modification and enzyme kinetics of high-solids cellulosic slurries: an economic analysis. Energy Fuels 24, 6506–6512. doi: 10.1021/ef100746q
Kristensen, J., Felby, C., and Jørgensen, H. (2009). Determining yields in high solids enzymatic hydrolysis of biomass. App. Biochem. Biotech. 156, 127–132. doi: 10.1007/s12010-008-8375-0
Kumar, R., and Wyman, C. E. (2014). Strong cellulase inhibition by Mannan polysaccharides in cellulose conversion to sugars. Biotech. Bioeng. 111, 1341–1353. doi: 10.1002/bit.25218
Lavenson, D. M., Tozzi, E. J., McCarthy, M. J., and Powell, R. L. (2011). Yield stress of pretreated corn stover suspensions using magnetic resonance imaging. Biotech. Bioeng. 108, 2312–2319. doi: 10.1002/bit.23197
Li, C., Knierim, B., Manisseri, C., Arora, R., Scheller, H. V., Auer, M., et al. (2010). Comparison of dilute acid and ionic liquid pretreatment of switchgrass: biomass recalcitrance, delignification and enzymatic saccharification. Bioresour. Technol. 101, 4900–4906. doi: 10.1016/j.biortech.2009.10.066
Li, C., Tanjore, D., He, W., Wong, J., Gardner, J. L., Sale, K. L., et al. (2013). Scale-up and evaluation of high solid ionic liquid pretreatment and enzymatic hydrolysis of switchgrass. Biotechnol. Biofuels 6, 1–13. doi: 10.1186/1754-6834-6-154
Li, Y., Li, X., Shen, F., Wang, Z., Yang, G., Lin, L., et al. (2014). Responses of biomass briquetting and pelleting to water-involved pretreatments and subsequent enzymatic hydrolysis. Bioresour. Technol. 151, 54–62. doi: 10.1016/j.biortech.2013.10.044
Liu, Y., Xu, J., Zhang, Y., Yuan, Z., and Xie, J. (2015). Optimization of high solids fed-batch saccharification of sugarcane bagasse based on system viscosity changes. J. Biotechnol. 211, 5–9 doi: 10.1016/j.jbiotec.2015.06.422
Lloyd, T. A., and Wyman, C. E. (2005). Combined sugar yields for dilute sulfuric acid pretreatment of corn stover followed by enzymatic hydrolysis of the remaining solids. Bioresour. Technol. 96, 1967–1977. doi: 10.1016/j.biortech.2005.01.011
Malinowska, K. H., Rind, T., Verdorfer, T., Gaub, H. E., and Nash, M. A. (2015). Quantifying synergy, thermostability, and targeting of cellulolytic enzymes and cellulosomes with polymerization-based amplification. Anal. Chem. 87, 7133–7140. doi: 10.1021/acs.analchem.5b00936
Nakagame, S., Chandra, R. P., Kadla, J. F., and Saddler, J. N. (2011). Enhancing the enzymatic hydrolysis of lignocellulosic biomass by increasing the carboxylic acid content of the associated lignin. Biotechnol. Bioeng. 108, 538–548. doi: 10.1002/bit.22981
Opitz, B., Prediger, A., Lüder, C., Eckstein, M., Hilterhaus, L., Lindner, P., et al. (2013). In situ microscopy for in-line monitoring of the enzymatic hydrolysis of cellulose. Anal. Chem. 85, 8121–8126. doi: 10.1021/ac4008495
Palmqvist, B., and Lidén, G. (2012). Torque measurements reveal large process differences between materials during high solid enzymatic hydrolysis of pretreated lignocellulose. Biotechnol. Biofuels 5:57. doi: 10.1186/1754-6834-5-57
Payne, C. M., Himmel, M. E., Crowley, M. F., and Beckham, G. T. (2011). Decrystallization of oligosaccharides from the cellulose Iβ surface with molecular simulation. J. Phys. Chem. Lett. 2, 1546–1550. doi: 10.1021/jz2005122
Pimenova, N. V., and Hanley, T. R. (2003). “Measurement of rheological properties of corn stover suspensions,” in Biotech for Fuels and Chemicals (New York, NY: Springer Science +Business Media, LLC), 383–392. doi: 10.1007/978-1-59259-837-3_30
Qing, Q., Yang, B., and Wyman, C. E. (2010). Xylooligomers are strong inhibitors of cellulose hydrolysis by enzymes. Bioresour. Technol. 101, 9624–9630. doi: 10.1016/j.biortech.2010.06.137
Reese, E. T., Segal, L., and Tripp, V. W. (1957). The effect of cellulase on the degree of polymerization of cellulose and hydrocellulose. Textile Res. J. 27, 626–632.
Reese, E. T., Siu, R. G. H., and Levinson, H. S. (1950). The biological degration of the soluble cellulose derivatives and its relationship to the mechanism of cellulose hydrolysis. J. Bacteriol. 59, 485–497.
Roche, C. M., Dibble, C. J., Knutsen, J. S., Stickel, J. J., and Liberatore, M. W. (2009). Particle concentration and yield stress of biomass slurries during enzymatic hydrolysis at high-solids loadings. Biotechnol. Bioeng. 104, 290–300. doi: 10.1002/bit.22381
Samaniuk, J. R., Scott, C. T., Root, T. W., and Klingenberg, D. J. (2012). Rheological modification of corn stover biomass at high solids concentrations. J. Rheol. 56, 649–665. doi: 10.1122/1.3702101
Sannigrahi, P., Kim, D. H., Jung, S., and Ragauskas, A. (2011). Pseudo-lignin and pretreatment chemistry. Energy Environ. Sci. 4, 1306–1310. doi: 10.1039/C0EE00378F
Satlewal, A., Agrawal, R., Bhagia, S., Das, P., and Ragauskas, A. J. (2017). Rice straw as a feedstock for biofuels: availability, recalcitrance, and chemical properties. Biofpr Biofuels Bioproducts Biorefining 12, 83–107. doi: 10.1002/bbb.1818
Selig, M. J., Viamajala, S., Decker, S. R., Tucker, M. P., Himmel, M. E., and Vinzant, T. B. (2007). Deposition of lignin droplets produced during dilute acid pretreatment of maize stems retards enzymatic hydrolysis of cellulose. Biotechnol. Prog. 23, 1333–1339. doi: 10.1021/bp0702018
Selig, M. J., Weiss, N., and Ji, Y. (2008). Enzymatic Saccharification of Lignocellulosic Biomass. Golden, CO: National Renewable Energy Laboratory.
Senturk-Ozer, S., Gevgilili, H., and Kalyon, D. M. (2011). Biomass pretreatment strategies via control of rheological behavior of biomass suspensions and reactive twin screw extrusion processing. Bioresour. Technol. 102, 9068–9075. doi: 10.1016/j.biortech.2011.07.018
Sluiter, A., Hames, B., Hyman, D., Payne, C., Ruiz, R., Scarlata, C., et al. (2008a). Determination of Total Solids in Biomass and Total Dissolved Solids in Liquid Process Samples. Golden, CO: National Renewable Energy Laboratory.
Sluiter, A., Hames, B., Ruiz, R., Scarlata, C., Sluiter, J., Templeton, D., et al. (2008b). “Determination of structural carbohydrates and lignin in biomass,” in Laboratory Analytical Procedure (Golden, CO: National Renewable Energy Laboratory).
Smith, P. K., Krohn, R. I., Hermanson, G. T., Mallia, A. K., Gartner, F. H., Provenzano, M. D., et al. (1985). Measurement of protein using bicinchoninic acid. Anal. Biochem. 150, 76–85. doi: 10.1016/0003-2697(85)90442-7
Stickel, J. J., Knutsen, J. S., Liberatore, M. W., Luu, W., Bousfield, D. W., Klingenberg, D. J., et al. (2009). Rheology measurements of a biomass slurry: an inter-laboratory study. Rheol. Acta 48, 1005–1015. doi: 10.1007/s00397-009-0382-8
Sun, C., and Gunasekaran, S. (2009). Effects of protein concentration and oil-phase volume fraction on the stability and rheology of menhaden oil-in-water emulsions stabilized by whey protein isolate with xanthan gum. Food Hydrocol. 23, 165–174. doi: 10.1016/j.foodhyd.2007.12.006
Tanjore, D., Gardner, J., Li, C., Wong, J., He, W., Sale, K., et al. (2013). “Rheological characterization of 1-ethyl-3-methylimidazolium acetate and lignocellulosic biomass mixtures,” in Joint BioEnergy Annual Retreat (Sonoma, CA).
Tanjore, D., Marshall, M. N., and Richard, T. L. (2009). “Biological pretreatments of corn stover with filamentous fungi,” in The 31st Symposium on Biotechnology for Fuels and Chemicals.
Viamajala, S., McMillan, J. D., Schell, D. J., and Elander, R. T. (2009). Rheology of corn stover slurries at high solids concentrations–effects of saccharification and particle size. Bioresour. Technol. 100, 925–934. doi: 10.1016/j.biortech.2008.06.070
Williams, C. L., Westover, T. L., Emerson, R. M., Tumuluru, J. S., and Li, C. (2016). Sources of biomass feedstock variability and the potential impact on biofuels production. BioEnergy Res. 9, 1–14. doi: 10.1007/s12155-015-9694-y
Wiman, M., Palmqvist, B., Tornberg, E., and Lidén, G. (2011). Rheological characterization of dilute acid pretreated softwood. Biotech. Bioeng. 108, 1031–1041. doi: 10.1002/bit.23020
Xiao, Z., Zhang, X., Gregg, D., and Saddler, J. (2004). “Effects of sugar inhibition on cellulases and β-glucosidase during enzymatic hydrolysis of softwood substrates,” in Proceedings of the Twenty-Fifth Symposium on Biotechnology for Fuels and Chemicals Held May 4–7, 2003, eds M. Finkelstein, J. McMillan, B. Davison, and B. Evans (Breckenridge, CO: Humana Press), 1115–1126.
Xue, S., Uppugundla, N., Bowman, M. J., Cavalier, D., Da Costa Sousa, L., E Dale, B., et al. (2015). Sugar loss and enzyme inhibition due to oligosaccharide accumulation during high solids-loading enzymatic hydrolysis. Biotechnol. Biofuels 8:195. doi: 10.1186/s13068-015-0378-9
Keywords: biomass, pre-processing, pretreatment, enzymatic hydrolysis, rheology, phase angle
Citation: Coffman P, McCaffrey N, Gardner J, Bhagia S, Kumar R, Wyman CE and Tanjore D (2018) In Situ Rheological Method to Evaluate Feedstock Physical Properties Throughout Enzymatic Deconstruction. Front. Energy Res. 6:53. doi: 10.3389/fenrg.2018.00053
Received: 04 April 2018; Accepted: 30 May 2018;
Published: 02 July 2018.
Edited by:
Allison E. Ray, Idaho National Laboratory (DOE), United StatesReviewed by:
Suyin Gan, University of Nottingham Malaysia Campus, MalaysiaRuchi Agrawal, Indian Oil Corporation, India
Copyright © 2018 Coffman, McCaffrey, Gardner, Bhagia, Kumar, Wyman and Tanjore. This is an open-access article distributed under the terms of the Creative Commons Attribution License (CC BY). The use, distribution or reproduction in other forums is permitted, provided the original author(s) and the copyright owner(s) are credited and that the original publication in this journal is cited, in accordance with accepted academic practice. No use, distribution or reproduction is permitted which does not comply with these terms.
*Correspondence: Deepti Tanjore, ZHRhbmpvcmVAbGJsLmdvdg==