- DBT-IOC Centre for Advanced Bioenergy Research, Indian Oil R&D Centre, Faridabad, India
Enzymatic hydrolysis at high solids loading has the potential to reduce both capital and operational expenditures. Here, pretreatment of rice straw (PRS) with dilute acid was carried out at a pilot scale (250 kg per day) at 162°C for 10 min and 0.35% acid concentration, followed by enzymatic hydrolysis at different total solids loadings. It showed that although the total sugar concentration increased from 48 to 132 g/l, glucan conversion reduced by 27% (84–66.2%) with increasing solids from 5 to 20% in batch mode. Therefore, two different fed-batch approaches were evaluated to improve the glucan conversion by the sequential addition of a substrate and/or enzyme. At 20% solid loadings and a 3 filter paper units/g enzyme dosage, the highest glucan conversion obtained was 66% after 30 h of hydrolysis in batch mode. However, in an optimized fed-batch approach, the glucan yield was improved to 70% by simply dividing the substrate feeding into three batches, that is, 50% at 0 h, 25% each after 4 h, and 8 h of hydrolysis reaction. The addition of surfactant (Ecosurf E6) further improved the conversion to 72% after 30 h. The role of critical factors, that is, inhibitors, enzyme–lignin binding, and viscosity, was investigated during the course of hydrolysis in the batch and fed-batch approaches. This study suggests a sustainable approach for improved hydrolysis at high solids loadings by fine-tuning a simple process.
Introduction
Global energy demand is increasing with an average growth rate of 1.3% per year, and it is expected to increase by 30% by 2035, with the highest energy consumption rate (4.2% per year) in India (Satlewal et al., 2017, 2018a,b). Bioethanol from lignocellulosic biomass (LB) plays a critical role in meeting this energy demand as a renewable and sustainable source of energy (Agrawal et al., 2015a). One of the primary challenges in producing second-generation biofuels is the recalcitrant nature of the LB, chiefly imposed by the complex lignin-carbohydrate linkages (LCCs). The LCCs enhance the integrity and rigidity of the plant cell wall, yielding a complex macromolecular assembly and rendering the recalcitrance for biorefining (Li et al., 2016). Thus, thermochemical pretreatment is essential to improve enzyme accessibility to carbohydrates, embedded within lignin, to produce fermentable sugars efficiently (Adsul et al., 2014; Agrawal et al., 2015a; Gaur et al., 2015; Saini et al., 2015; Antonopoulou et al., 2016; Skiba et al., 2017).
High total solids loadings [>15% (w/w)] are desired for scaling up of the process to produce high ethanol titer at reduced capital and operational expenditure (Modenbach and Nokes, 2013; Hou et al., 2016a). However, the high solids reaction faces challenges due to poor mass and heat transfer, leading to inefficient mixing and pumping, sugars accumulation during hydrolysis leading to feedback inhibition, and a high inhibitor concentration that lowers the hydrolysis yield (Cannella and Jørgensen, 2014). Specialized types of reactors with scrappers and impellers are thus required to overcome these challenges in mixing (Larsen et al., 2012; Zhang et al., 2014; Agrawal et al., 2015c; Hou et al., 2016a,b).
Efforts have been made in the last few years to reduce the saccharification cost by mutagenesis of cellulase-producing organisms for hyperproduction of cellulolytic enzymes (Agrawal et al., 2013b, 2017b). This has been achieved by using a cheaper carbon source to induce cellulase production (Agrawal et al., 2013a,c; Sharma et al., 2015), enzyme immobilization (Agrawal et al., 2016a,b), enzyme blending with high synergy (Gottschalk et al., 2010; Agrawal et al., 2015a,b; Sharma et al., 2015), and the addition of surfactants or proteins that reduce nonspecific binding of enzymes on lignin (Agrawal et al., 2017a), and simultaneous saccharification and fermentation (2015). Further, Wenjian et al. (2014) have reported that supplementing the polyelectrolytes, such as cationic polyacrylamide and poly(diallyldimethylammonium chloride) during enzymatic hydrolysis, has significantly improved the glucan and xylan yield.
Another strategy to resolve these challenges is the fed-batch approach, where only a process adjustment is required, by optimizing the dosage and timings of substrate and/or enzyme addition. This approach will benefit from the low viscosity by the optimization of the substrate-to-enzyme ratio in the reactor. Low solids provide enzymes with the opportunity to efficiently liquefy the available substrates in minimal time (Modenbach and Nokes, 2013). The free liquid is then available to accommodate more of the substrate (the next batch). High solids are thus easily accommodated in the fed-batch mode without affecting the rheology of the reaction. Timed additions of fixed amounts of substrate may help in minimizing the energy required for proper mixing and completion of the reaction. Timing of enzyme addition was also investigated as it has been reported to impact the overall liquefaction process and efficiency of the saccharification process (Gao et al., 2014; Cardona et al., 2015).
In this study, pretreatment of rice straw (RS) with dilute acid was carried out at a pilot scale (250 kg/day capacity). Further, enzymatic hydrolysis of pretreated rice straw (PRS) was carried out by batch and fed-batch approaches through substrate and enzyme splitting, to improve the hydrolysis yield. This study deciphered the critical roles of enzyme inhibitors (phenols and cellobiose), lignin, and viscosity affecting the fermentable sugar yield. The role of the surfactant in reducing the nonproductive enzyme-lignin binding is also elucidated.
Materials and Methods
Chemicals and Substrate
All chemicals of analytical grade were procured from Merck, India. Cellulase with a 220 filter paper unit (FPU)/g activity (Agrawal et al., 2014) (SacchariSEB-C6) was procured from Advanced Enzymes, Mumbai (India) and surfactant (Ecosurf E6) was kindly provided by the Dow Chemical Company (India) as a gift. Rice straw (Oryza sativa) with 9% moisture was procured locally, and it was milled using a knife mill to the particle size of 5–10 mm.
Pilot Scale Pretreatment
The RS pretreatment was carried out in a 250 kg/day-capacity continuous pilot scale horizontal pretreatment reactor as described by Kapoor et al. (2017). For this, 50 kg of dried RS was soaked in 500 L of dilute sulfuric acid (1% w/w) for 45 min at ambient temperature. To improve the total solids loading, excess acidified water was removed by using a hydraulic press with a 100 bar pressure. Pretreatment of the pressed RS (SPRS) with a moisture content of 35% was carried out at 162°C, 5 bar pressure, and a residence period of 10 min at a rate of 10 kg/h. Final acid concentration in the reactor was 0.35 wt% of the substrate. Pretreated RS was neutralized by adding a 1 N sodium hydroxide solution to bring the initial 1.8 pH up to 5 pH.
Enzymatic Hydrolysis
Batch Mode Hydrolysis
Enzymatic hydrolysis of acid pretreated RS was carried out at an enzyme dosage of 1–5 FPU/g TS at 5–20% total solids (TS) and at 50°C. The pH of the reaction was maintained at pH 5 using a 0.1 M sodium citrate buffer. Saccharification yield was calculated as described by Agrawal et al. (2017a) using the following equation:
Fed-Batch Mode Hydrolysis
The fed-batch experiments were carried out under the same reaction conditions as the batch mode, except that the substrate and the enzyme were added in multiple batches. Hydrolysis was performed at 3 FPU/g TS at an initial low dry matter. In all experiments, final TS concentration was 20%. The fed-batch was optimized by a one variable at a time (OVAT) approach as depicted in Table 1. The following were the different variables studied:
• Time of substrate feeding: The timing of the substrate portions was optimized, while the proportions of the substrate and the enzyme added were kept constant. Substrate was added in 3 portions of 50, 25, and 25% at 0, 4, and 8 h, respectively. In total, 100% of the enzyme was added at 0 h.
• Substrate Splitting (SS): The size of the substrate proportions was optimized here, while enzyme proportion and time were kept constant at 0, 4, and 8 h. In total, 100% of the enzyme was added at 0 h.
• Substrate plus enzyme splitting (SES): Portions of the enzyme were optimized here, while the substrate proportion and time were kept constant. The substrate was added in 3 portions of 50, 25, and 25% at 0, 4, and 8 h, respectively.
Effect of Ecosurf E6 on Fed-Batch Hydrolysis
The effect of the surfactant (Ecosurf E6) at 0.05% of TS was studied during the substrate-splitting approach and the optimized fed-batch hydrolysis condition. The substrate was added in 3 portions of 50, 25, and 25% at 0, 4, and 8 h, respectively. In total, 100% of the enzyme was added at 0 h. Hydrolyses and sample collection were carried out under the same reaction conditions as described in the Enzymatic Hydrolysis section.
Comparison of Different Modes of Hydrolysis
To understand the differences in hydrolysis among the batch mode and fed-batch mode substrate splitting, the substrate plus enzyme splitting, and the substrate splitting plus Ecosurf E6, various parameters were determined as follows:
Cellobiose and Total Phenols Estimations
Hydrolysate samples were taken periodically and analyzed for cellobiose and total phenols using Waters' HPLC with Aminex HPX-87P column (Bio-Rad, United States) coupled with refractive index detector (Agrawal et al., 2018). Milli-Q water was used as a mobile phase at a flow rate of 0.6 ml/min, with a column temperature of 75°C (Agrawal et al., 2015b). The total phenols were estimated by Folin-Ciocalteu method (Sanchez-Rangel et al., 2013).
Protein Adsorption and Lignin Content Measurements
To monitor the protein adsorption on the substrate, free protein was measured at various time intervals during the hydrolysis experiments by the Bradford method (Bradford, 1976). The total absorbed protein (%) was calculated as follows:
The lignin content was calculated directly based on the chemical composition of the pretreated RS by the NREL LAP protocol as described in the Pilot Scale Pretreatment section.
Viscosity Changes During Hydrolysis in Different Modes
The viscosity during hydrolysis was measured at specific time points during the enzymatic hydrolysis in different modes by means of a DV-III Ultra Rheometer as described by Rosgaard et al. (2007).
Analytical Methods
The moisture content of samples was determined using an infrared drier from Sartorius MA-150C (Model No. 000230V1), Germany (Kapoor et al., 2017). The compositional analysis of native- and pretreated- RS was carried out by a two-stage acid hydrolysis following the NREL LAP (Sluiter et al., 2011). The content of insoluble solids in the pretreated slurry was determined by following the NREL LAP (Sluiter et al., 2008b). The sugar analysis was performed using an Aminex HPX-87P column (Bio-Rad, USA) coupled with a refractive index detector in HPLC (Waters Gesellschaft Mbh, Austria) (Agrawal et al., 2017a; Kapoor et al., 2017).
Results and Discussion
Pretreatment and Chemical Composition of Rice Straw
During the dilute acid pretreatment, hemicellulose is hydrolyzed into monomeric sugars, that is, xylose, arabinose, galactose, glucose, and mannose, with alternations in the lignin structure (Agrawal et al., 2015b, 2017a; Skiba et al., 2017). This, in turn, increases the pore volume and surface area of the substrate, improving the enzyme accessibility. Chemical composition of native and acid PRS is depicted in Figure 1, which represents a hemicelluloses content decrease from 23.5 to 3.4%, with an increase in glucan (from 37 to 53%) and xylan content (from 13.4 to 27.8%). Similarly, Agrawal et al. (2015a) reported that after acid pretreatment of wheat straw, the cellulose content increased from 36.6 to 69.8% and the hemicellulose was reduced to 3.8%, while the lignin content increased from 22.2 to 26.4%. The solid content of the pretreated slurry was 26% and glucose and xylose concentrations in the slurry liquid were 12.1 and 45 g/L, respectively. The concentration of 5-hyxymethyfurfural (HMF), furfural, and acetic acid was 0.2, 0.4, and 1.4 g/L, respectively. Our previous study showed that optimized pretreatment conditions might lead to higher sugar recoveries with a meager amount of inhibitors, so that this slurry could be directly used for enzymatic hydrolysis and fermentation without any detoxification process (Satlewal et al., 2018b). The ash content in the PRS increased from 7.3 to 15.8%, while all of the extractives were removed from the solids and recovered in the liquid stream.
The mass closure for the sugars present in the 15 kg batch of RS with a 9% moisture content was carried out and showed that the native RS had 5.59 kg of glucose and 3.13 kg of xylose. After pretreatment, 89% or 4.97 kg of the glucan was recovered along with the solid residue, while only 0.38 kg of the glucose was found in the liquids. Therefore, a total of 95.7% glucan recovery was made after pretreatment, with only 6.8% glucose yield as monomeric sugar after the pretreatment. In contrast to this, 1.5 kg of the monomeric xylose sugars were recovered in the liquid stream accounting for 48% of the xylose, while 0.35 kg was obtained with solid residue. Thus, a total of 59% xylan recovery could be made during this pretreatment. Further, details of mass balance, sugars recovery, and calculation particulars could be referred from our previously published article (Kapoor et al., 2017).
Batch Mode Hydrolysis
The effect of the enzyme dosage (1–5 FPU/g TS) was studied in the enzymatic hydrolysis in the batch mode, where both the substrate and the enzyme were added at the beginning only. About 60% of the glucan conversion and 128.35 g/L total sugars were achieved after 24 h using 3 FPU/g TS at 20% solid loadings (Figure 2). However, a maximum of 68% conversion with a total 135 g/L sugars was achieved using 5 FPU/g of the enzyme. It was found that at low dose (1 and 2 FPU/g) of the enzyme, the sugar release was not significant. Based on the results that showed only an 8% increase when an extra 2 FPUs were added, the enzyme dose of 3 FPU/g TS was used for further studies.
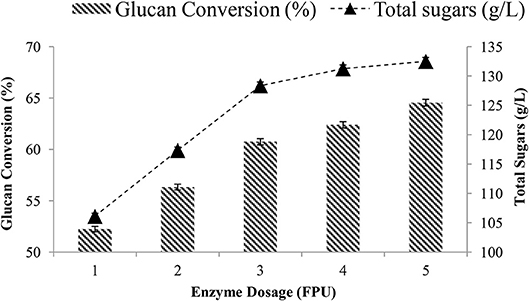
Figure 2. Glucan conversion and total sugars obtained at various enzyme dosages (1–5 FPU/g TS) at 20% TS after 24 h.
The effect of total solids loadings (5–20%) on the glucan conversion and total sugars was examined at 3 FPU/g TS. Although the total sugars concentration increased from 48 g/L at 5% TS to 132 g/L at 20% TS, the glucan conversion was found to drop by 20% (Figure 3). With the purpose to obtain high conversions along with high concentrations of fermentable sugars, a further study was carried out by the fed-batch approach at 20% total solids loadings. The fed-batch was optimized using the one-variable-at-a-time (OVAT) approach.
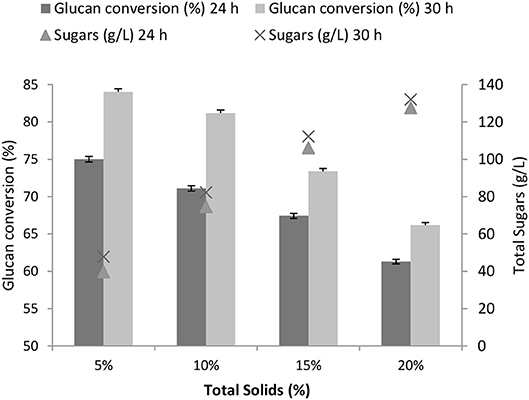
Figure 3. Glucan conversion and total sugars obtained at different total solids loadings (5–20%) at 3 FPU/g TS after 24 and 30 h.
Substrate Splitting: Optimization of the Time Interval and Sizes for Substrates Feeding During Fed-Batch Hydrolysis
The substrate was added in batches (50, 25, and 25%) at varying time periods, while all the enzyme (100%) was added at 0 h. The maximum conversion was achieved when the substrate was added in three batches at 0, 4, and 8 h (Figure 4). About 65% glucan conversion was achieved after 24 h and 70% glucan conversion after 30 h using 3 FPU/g TS. While maximum conversion achieved in the control (batch mode) was only 61% after 24 h and 66% after 30 h under the same conditions.
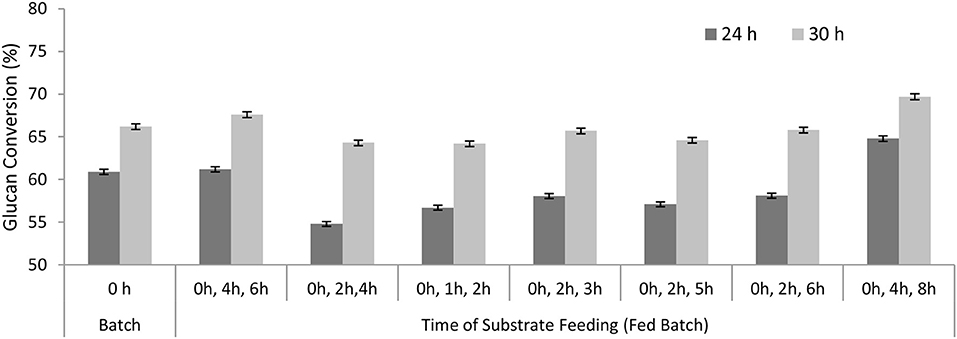
Figure 4. Glucan conversion during substrate additions in fed-batch approach. Size of substrate additions was constant (50, 25, and 25%) while the entire enzyme (100%) was added at the beginning.
Further, the sizes of the substrates to be added at an interval of 0, 4, and 8 h were optimized. Whole enzyme (100%) was added at the beginning, that is, at 0 h. The maximum conversion was achieved when the substrate was added in three batches of 50, 25, and 25% (Figure 5). About 64% glucan conversion was achieved after 24 h and 68% conversion after 30 h using 3 FPU/g TS, while the maximum conversion achieved in the batch mode was only 61% after 24 h and 65% after 30 h under similar conditions. Thus, in further experiments, the substrate was added proportionally in three batches (50, 25, and 25%) and at an interval of 0, 4, and 8 h, respectively.
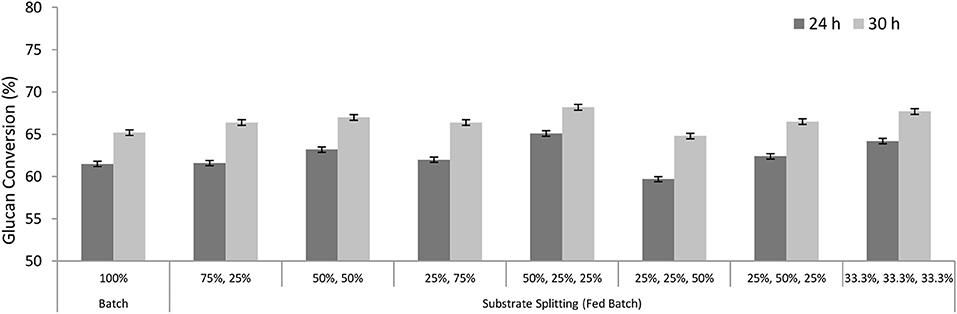
Figure 5. Glucan conversion during substrate splitting in fed-batch experiments. Time of substrates addition was constant (0, 4, and 8 h) and whole enzyme (100%) was added at the beginning.
Similarly, Zhao et al. (2013) compared the wet pulp hydrolysis at 30% total solids loadings by the batch and fed-batch approaches. More than a 10% increase in glucan and 20 g/L high glucose yield was obtained with the addition of the substrate at “0, 12, 36, and 48 h.” Gupta et al. (2012) also obtained a 50% increase in the sugar release with a 23% increase in the cellulose conversion by the intermittent addition of solids in a repeated fed-batch mode (substrate and enzyme thrice after 24, 56, and 80 h) at 20% total solids loadings as compared with the single-batch addition. Yang et al. (2011) showed an increase of 34–37% in the hydrolysis yield conversion at 30% total solids loadings in the three-stage (9, 9, and 12 h) hydrolysis as compared with the one-stage hydrolysis for 72 h, but at 20 FPU/g cellulose. Wang et al. (2012) charged the reactors with 1/2 of the final total solids loadings, followed by two additional feedings of 1/4th of the final total solids loadings at 24 and 48 h. The fed-batch hydrolysis method adopted in this study produced total sugar of 17.06 g/L more than the batch hydrolysis and raised the substrate consistency to 30%.
Contrary to this, Chandra et al. (2011) reported that the batch mode at 10% total solids loadings at 3 FPU/g cellulose gives a better sugar yield after 72 h of incubation as compared with the fed-batch reaction. This might be because of the irreversible enzyme-substrate binding and/or the inability of the remaining unabsorbed enzymes to find accessible cellulose on the freshly added substrate. Free protein measurements after 72 h indicated that while the cellulose conversion ceased, 50–70% of the cellulase was still adsorbed to the substrate. According to Chandra et al. (2011), the fed-batch reaction with a low total solids loadings, leads to a rapid glucose accumulation, which causes strong feedback inhibition especially at a low enzyme dosage and inefficient mixing.
Substrate Plus Enzyme Splitting: Optimization of the Sizes for Enzyme Feeding During Fed-Batch Hydrolysis
The strategy of enzyme addition might also affect the hydrolysis; therefore, the fed-batch approach was investigated with all of the enzyme added either at the beginning or proportionally in multiple batches along with the substrate. Enzyme sizes or dosages were optimized at different time intervals (0, 4, and 8 h). The effect of enzyme splitting was observed only after 48 h. When the enzyme was added in three batches of 50, 25, and 25%, the glucan conversion was 76.7% using a 3 FPU/g TS, while the control (batch mode) showed only 70.5% of the conversion after 48 h (Figure 6). When the whole enzyme (100%) was added at the beginning, that is, at 0 h, about 74.8% of the glucan conversion was obtained after 48 h in the fed-batch. These results are similar to Hodge et al. (2008), where a high yield (80%) was achieved by the enzyme-splitting approach in comparison with adding all the enzyme at the start of the reaction. Quite high enzyme dosages of 10.7 FPU/g of the cellulose (or 22 FPU/g TS considering 50% glucan in the pretreated substrate) were used in comparison with this study (Hodge et al., 2008). The major reasons for this dissimilarity might be the high solid loadings of 25% TS and the type, variety, and efficiency of the enzyme formulation, biomass, and pretreatment. A similar study by Ma et al. (2011) showed that at 25% (w/v) total solids loadings, the batch reaction reached about 50% conversion, whereas the fed-batches with a single-enzyme addition and multiple = –enzyme additions achieved about 5 and 84% conversions, respectively. The commercial cellulolytic enzyme cocktail used here is composed of endoglucanase, beta-glucosidase, exoglucanase, and other enzyme-stabilizing components. Although we have not evaluated the half-life of the enzyme in this study, previous studies have reported that commercial enzymes might have a half-life of 25–120 h (Saddler et al., 1985). In this study, 168 h was reported for the enzyme-splitting approach, where the fresh enzyme was added after every few hours, and this might have further extended the half-life of the enzyme and resulted into a high yield.
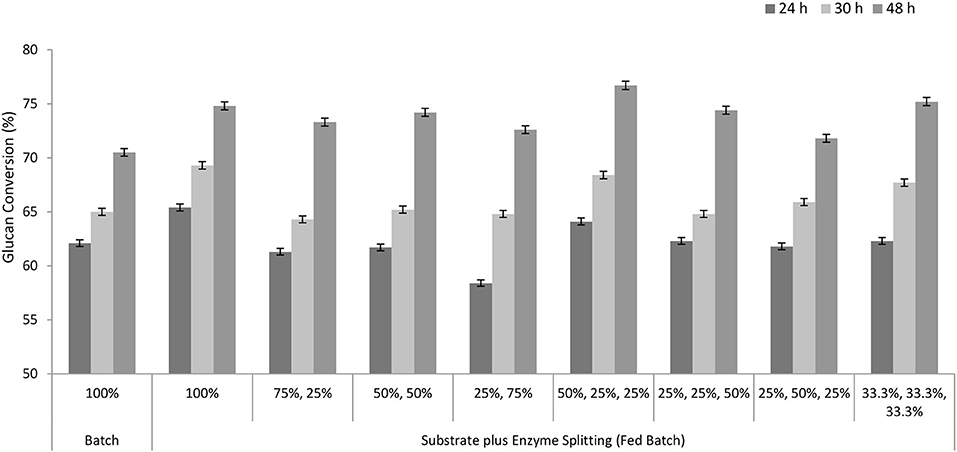
Figure 6. Glucan conversion during substrate plus enzyme splitting in fed-batch experiments. Time (0, 4, and 8 h) and size of the substrates (50, 25, and 25%) were constant.
Another set of studies claimed that the fed-batch process with multiple feeds of the enzyme do not perform as well as a single-stage feeding approach. Rosgaard et al. (2007) elucidated that the hydrolysis yield was not affected by the mode of enzyme application and the final glucose concentrations were similar in both the batch and fed-batch reactions. Geng et al. (2015) reported that adding the whole amount of enzymes at the beginning is more effective than adding the enzymes proportionally with the solid materials. Zhao et al. (2013) investigated three cellulase-feeding strategies: (3/3 of the enzymes at beginning; 2/3 at beginning, and 1/3 at the 12 h; 1/3 at the beginning, 1/3 at the 12 h, and the rest 1/3 at the 36 h). Quick liquefaction within 4 h was observed with strategy I (all of the enzyme addition at initial stage of saccharification (0 h), while only a fraction of the substrate was present during the fed-batch); however, similar glucose concentrations and glucan conversions were obtained for all three strategies after 216 h of hydrolysis. Similarly, according to a recent study by Cardona et al. (2015), adding all the enzyme at the start of the hydrolysis reaction was beneficial for process efficiency, as rapid liquefaction allowed earlier batch additions of the substrate (4.5 h instead of 6.5 h), thus achieving higher total solids loadings faster. Cardona et al. (2015) and Zhao et al. (2013) observed that while a higher ratio of the enzyme and substrate (E/S) may account for initial improvements, additional factors are at play to make liquefaction more efficient in the later additions. The longer exposure to the substrate added earlier in the reaction might allow for more constrained water to be released, thus decreasing viscosities in the soluble phase and facilitating the mass transfer of the enzymes to the substrate in subsequent additions (Roberts et al., 2011). Therefore, adding all the enzymes at the beginning may counteract the inhibitory effects of new substrate additions by more thoroughly liquefying the first batches of the substrate.
Yet another set of studies claimed that enzyme splitting was more significant than whole enzyme feeding in late hours of the process. Sugiharto et al. (2016) showed that the glucose concentration was higher for the first 40 h (25% total solids loadings was operated) when the whole enzyme was added at the beginning as compared with the concentration in the enzyme splitting. The glucose concentration was higher for the hydrolysis between 40 and 120 h with the enzyme splitting, as compared with the former one. Enzyme splitting could increase the enzymatic digestibility to 24–26% and the glucose concentration to 6–12% after 40 h, when compared with the whole enzyme feeding. Wanderley et al. (2013) also found a similar trend with a drop in the hydrolysis rate after 30 h of the process, which is supposed to be caused by the lignin presence in the bagasse. Cui et al. (2014) also reported that the enzyme splitting was more significant than the whole enzyme feeding after 20 h of the process. Cui et al. (2014) started the fed-batch experiments with an initial 5% TS, another 25% TS was added in 5 portions at 2, 5, 8, 12, and 17 h after beginning, giving a final TS concentration of 30%. The enzyme was added to the reactor either at the beginning or in 5 portions divided according to the TS content. While a decreasing trend was observed in the initial hours of the process, mainly due to the lower hydrolysis time for added fresh substrate and a higher concentration of the inhibitor (such as lignin, glucose) in enzyme splitting, the higher cellulose conversion was achieved in the later stage of the reaction (>27 h) and was attributed to the reduced glucose inhibition and adsorption onto the lignin. Similar work was carried out by Gao et al. (2014), who also evaluated these two kinds of enzyme-feeding strategies (namely whole enzyme and enzyme splitting). According to Gao et al. (2014), sugars were more in the former, until the final stage of 72 h.
In case of the enzyme splitting, the E/S ratio during the hydrolysis remains constant (Park et al., 2013). However, the E/S reduces considerably when the whole enzyme is added initially, while the fresh substrate is added stepwise. In addition to this, the enzyme also binds non-productively with the lignin and might also get deactivated in the presence of inhibitors generated after pretreatment and a high operational temperature (50°C) (Agrawal et al., 2015b). Thus, there is a high probability of prolonged enzyme exposure with inhibitors when the entire enzyme was added initially as compared with the enzyme splitting (Ganesh et al., 2000).
Effect of Ecosurf E6 on Fed-Batch Hydrolysis
Ecosurf E6 is a nonionic, alcohol ethoxylate type of surfactant. It is biodegradable and eco-friendly with a very low aquatic toxicity. The mechanism of the action of nonionic surfactants during enzymatic hydrolysis has been reported earlier. It has been proposed that nonionic surfactants improved the cellulose accessibility with their high lubricating ability and reduce the nonproductive enzyme-lignin binding to improve the hydrolysis yield (Agrawal et al., 2017a; Wang et al., 2018). The effect of the surfactant (Ecosurf E6) was compared in the batch and fed-batch hydrolysis. For the fed-batch, the substrate (50, 25, and 25%) was added at (0, 4, and 8 h) intervals. The enzyme was added as a whole at 0 h. It was found that when Ecosurf E6 was added, the glucan conversion increased from 65.3 to 68.8% in the batch mode. The conversion increased from 68.6 to 71.8% after 30 h when Ecosurf E6 was added under the fed-batch hydrolysis conditions (Figure 7). It was observed that the glucan conversion increased up to 3.5% when Ecosurf E6 was added during the batch hydrolysis. The increase was 4.7% when Ecosurf E6 was added under optimized fed-batch hydrolysis conditions. The synergism among Ecosurf E6 and the fed-batch is promising for improving the lignocellulose hydrolysis.
Cellobiose and Total Phenols Estimations
One of the disadvantages of dilute acid pretreatment was the formation of phenols, furfurals, and acids, which acted as inhibitors for the subsequent enzymatic hydrolysis and fermentation. Most of the furfurals and acids were generated from carbohydrate degradation during acid pretreatment and usually determined with chromatographic techniques as reported in the NREL protocols (Sluiter et al., 2008a). Unlike other inhibitors, phenols were generated by the lignin degradation during a high severe pretreatment and only a few reports have discussed the effect of phenolics on enzymatic hydrolysis. Folin-Ciocalteu's reagent-based method is considered as a convenient and reliable approach for phenols estimation in the hydrolysate (Jönsson et al., 2013). In this study, the concentrations of the cellobiose and the total phenols were monitored during both the batch and fed-batch approaches (Agrawal et al., 2015b). As shown in Figure 8, the concentration of the cellobiose ranged between 20 and 31 ppm at 0 h (I stage) of hydrolysis in different modes, but a significant difference was observed in the cellobiose concentration among the batch mode and fed-batch modes during 4 h (II stage) and 8 h (III stage). In the batch experiment, the cellobiose increased to 83.24 ppm after 4 h and up to 92.11 ppm after 8 h, while the concentrations were 28.14 and 31.11 ppm for the substrate splitting, 42.33 and 58.15 ppm for the substrate and enzyme splitting, and 22.46 and 20.44 ppm for the substrate splitting with Ecosurf E6 (0.5%). After 24 h of hydrolysis, the cellobiose ranged between 42 and 58 ppm for the four modes.
The total phenolic compounds present in the hydrolysis reaction depend directly upon the amount of substrate added in the reactor. About 700 ppm of the total phenolics was present in the batch process since the start of the reaction because all of the substrate was added at 0 h. While in the substrate-splitting approach, the phenolics concentration was found to be low (34.06 ppm) during the initial hours (0 h or I stage). It increased gradually from 516 ppm at 4 h (II stage) to 700 ppm after 8 h (III stage) when all of the substrate was added (Figure 9).
As shown in Figure 8, the fed-batch approaches had a benefit of low inhibition by the cellobiose and the phenols over the batch mode in the initial 8 h of hydrolysis, yielding a higher cellulose hydrolysis and conversion. A high enzyme-to-substrate ratio in the fed-batch experiments resulted in a low cellobiose formation in the initial hours; however, a low enzyme-to-substrate ratio may be attributed to the lower inactivation of the enzyme by the cellobiose.
Protein Adsorption and Lignin Content Measurements
To understand the nonspecific enzyme-lignin binding, protein adsorption was monitored during hydrolysis under four different modes viz., batch, substrate splitting, substrate plus enzyme splitting, and substrate splitting plus Ecosurf E6.
Similar to the total phenols, the lignin content is also subject to the amount of the substrate that has been added during the hydrolysis. Since all the substrate was added at the 0 h in the batch mode, the lignin was constant (55.6 mg/mL) throughout the batch process. While in the other three fed-batch approaches, the concentrations of the lignin were low until the initial 8 h (III stage) and until all the substrate was added. It ranged between about 27.8 mg/mL during 0 h (I stage), 41.7 mg/mL after 4 h (II stage), and 55.6 mg/mL after 8 h (III stage) of hydrolysis in the fed-batch modes (Figure 9).
It was observed that at 0 h, protein adsorption was 48% for the batch process, 32% for the substrate splitting, 23% for the substrate plus enzyme splitting, and 18% for the substrate splitting plus Ecosurf E6. After 4 h, protein adsorption was 75% for the batch process, 56% for the substrate splitting, 44% for the substrate plus enzyme splitting, and 32% for the substrate splitting plus Ecosurf E6. After 48 h, protein adsorption was 54% for the batch process, 41% for the substrate splitting, 32% for the substrate plus enzyme splitting, and 49% for the substrate splitting plus Ecosurf E6 (Figure 9). Though protein adsorption is a consequence of binding to the cellulose (specific) and lignin (nonspecific), it is difficult to exactly estimate the proportion bound independently on the cellulose and on the lignin. The separation of the cellulose and the lignin and studying the adsorption again, do not lead to the results of an actual substrate. But, the data show that the protein adsorption varies among the batch and fed-batch modes of hydrolysis, the latter causing reduced adsorption of the enzyme or the protein, which may cause better hydrolysis in the fed-batch mode. The lower lignin content in the initial hours of hydrolysis in the three fed-batch modes could be directly related to the observations.
Viscosity Changes During Hydrolysis in Different Modes
The specific aims of using the fed-batch mode, whether it is substrate splitting or the substrate plus enzyme splitting, were to achieve hydrolysis of high substrate levels, low viscosity during hydrolysis, and high glucose concentrations. Viscosity changes during hydrolysis were measured at specific time points during the enzymatic hydrolysis. Inadequate mixing, which imposes the diffusion limitations causing product inhibition and hindrance in the enzyme to substrate accessibility, has been significantly improved using different fed-batch modes. A high viscosity (1,438 mPa s−1) of the substrate was observed in the case of the batch operation, which dropped to 980 mPa s−1 after 8 h of hydrolysis. For the other three fed-batch modes, the viscosity ranged from 487 to 732 mPa/s during the initial 8 h of hydrolysis (Figure 10). This is again indicative of better hydrolysis in the fed-batch modes. Similar to the observations made by Rosgaard et al. (2007), the decrease in viscosity was found to be most pronounced within the first 8 h of reactions with all the modes of hydrolysis. The effect was more prominent among the batch (E/S ≤ 1) and fed-batch (E/S ≥ 1). Beyond 48 h, all the reactions reached a low viscosity level (40–70 mPa/s).
Conclusions
In this study, we have investigated the effect of multiple factors viz. high viscosity, inappropriate heat and mass transfer, and high inhibitor concentrations responsible for a substantial reduction in the enzymatic hydrolysis yield at high total solids loadings. Here, we proposed a modified fed-batch approach with the intermittent or split addition of the substrate and enzyme to improve the hydrolysis yield by reducing the viscosity (substrate-splitting approach) and the enzyme exposure to inhibitors and lignin (enzyme-splitting approach). This study further elucidated that the surfactant (Ecosurf E6) with an ability to reduce enzyme-lignin binding worked in a synergistic manner along with the optimized fed-batch approach to substantially improve the hydrolysis yield. However, a detailed pilot scale techno-economic analyses of the batch and fed-batch approaches need to be carried out to further evaluate the commercial feasibility of this concept.
Author Contributions
RA was responsible for the experimental work as well as writing the manuscript, creating the figures and tables and compiling all results. BB helped in writing the manuscript. AM, RK, and RG reviewed the manuscript and improved the content. AS is the corresponding author and the project leader for this manuscript.
Conflict of Interest Statement
RA, BB, AM, RK, RG, and AS work at the DBT-IOC Centre for Advanced Bioenergy Research, Indian Oil Corporation Ltd., Haryana, India.
Acknowledgments
We are thankful to Indian Oil Corporation Ltd., R&D Centre, Faridabad and Department of Biotechnology (DBT), Govt. of India for providing all infrastructural, analytical, and financial assistance. Dow Chemicals, India is duly acknowledged for providing surfactants.
References
Adsul, M., Sharma, B., Singhania, R. R., Saini, J. K., Sharma, A., Mathur, A., et al. (2014). Blending of cellulolytic enzyme preparations from different fungal sources for improved cellulose hydrolysis by increasing synergism. RSC Adv. 4, 44726–44732. doi: 10.1039/C4RA08129C
Agrawal, R., Satlewal, A., Gaur, R., Anshu, M., Kumar, R., Gupta, R. P., et al. (2015a). Improved saccharification of pilot-scale acid pretreated wheat straw by exploiting the synergistic behavior of lignocellulose degrading enzymes. RSC Adv. 5, 71462–71471. doi: 10.1039/C5RA13360B
Agrawal, R., Satlewal, A., Gaur, R., Mathur, A., Kumar, R., Gupta, R. P., et al. (2015b). Pilot scale pretreatment of wheat straw and comparative evaluation of commercial enzyme preparations for biomass saccharification and fermentation. Biochem. Eng. J. 102, 54–61. doi: 10.1016/j.bej.2015.02.018
Agrawal, R., Satlewal, A., Kapoor, M., Mondal, S., and Basu, B. (2017a). Investigating the enzyme-lignin binding with surfactants for improved saccharification of pilot scale pretreated wheat straw. Bioresour. Technol. 224, 411–418. doi: 10.1016/j.biortech.2016.11.026
Agrawal, R., Satlewal, A., Mathur, A. S., Gupta, R. P., Raj, T., Kumar, R., et al. (2014). Kinetic and enzyme recycling studies of immobilized β-glucosidase for lignocellulosic biomass hydrolysis. Environ. Eng. Manag. J. 1–6.
Agrawal, R., Satlewal, A., Sharma, B., Mathur, A., Gupta, R., Tuli, D., et al. (2017b). Induction of cellulases by disaccharides or their derivatives in Penicillium janthinellum EMS-UV-8 mutant. Biofuels 8, 615–622. doi: 10.1080/17597269.2016.1242692
Agrawal, R., Satlewal, A., and Varma, A. (2015c). “Characterization of Plant Growth-Promoting Rhizobacteria (PGPR): a perspective of conventional versus recent techniques,” in Heavy Metal Contamination of Soils: Monitoring and Remediation, eds I. Sherameti and A. Varma (Basel: Springer International Publishing), 471–485. doi: 10.1007/978-3-319-14526-6_23
Agrawal, R., Satlewal, A., and Verma, A. (2013c). Utilization of citrus sinensis waste for the production of β-glucosidase by solid-state fermentation using a bacillus subtilis mutant. Environ. Eng. Manag. J.
Agrawal, R., Satlewal, A., and Verma, A. K. (2013a). Production of an extracellular cellobiase in solid state fermentation. J. Microbiol. Biotechnol. Food Sci. 2, 2339–2350. doi: 10.1016/j.ejbt.2015.05.007
Agrawal, R., Satlewal, A., and Verma, A. K. (2013b). Development of a β-glucosidase hyperproducing mutant by combined chemical and UV mutagenesis. 3 Biotech 3, 381–388. doi: 10.1007/s13205-012-0095-z
Agrawal, R., Srivastava, A., and Verma, A. K. (2016a). Immobilization of β-glucosidase onto silicon oxide nanoparticles and augment of phenolics in sugarcane juice. J. Food Sci. Technol. 53, 3002–3012. doi: 10.1007/s13197-016-2269-x
Agrawal, R., Verma, A., and Satlewal, A. (2018). “Chapter 24 - Bioprospecting PGPR microflora by novel immunobased techniques,” in Crop Improvement Through Microbial Biotechnology, eds P. Ram, S. Sarvajeet Gill, and T. Narendra (Oxford: Elsevier), 465–478. doi: 10.1016/B978-0-444-63987-5.00024-4
Agrawal, R., Verma, A. K., and Satlewal, A. (2016b). Application of nanoparticle-immobilized thermostable β-glucosidase for improving the sugarcane juice properties. Innov. Food Sci. Emerg. Technol. 33, 472–482. doi: 10.1016/j.ifset.2015.11.024
Antonopoulou, G., Vayenas, D., and Lyberatos, G. (2016). Ethanol and hydrogen production from sunflower straw: the effect of pretreatment on the whole slurry fermentation. Biochem. Eng. J. 116, 65–74. doi: 10.1016/j.bej.2016.06.014
Bradford, M. M. (1976). A rapid and sensitive method for the quantitation of microgram quantities of protein utilizing the principle of protein-dye binding. Anal. Biochem. 72, 248–254. doi: 10.1016/0003-2697(76)90527-3
Cannella, D., and Jørgensen, H. (2014). Do new cellulolytic enzyme preparations affect the industrial strategies for high solids lignocellulosic ethanol production? Biotechnol. Bioeng. 111, 59–68. doi: 10.1002/bit.25098
Cardona, M. J., Tozzi, E. J., Karuna, N., Jeoh, T., Powell, R. L., and McCarthy, M. J. (2015). A process for energy-efficient high-solids fed-batch enzymatic liquefaction of cellulosic biomass. Bioresour. Technol. 198, 488–496. doi: 10.1016/j.biortech.2015.09.042
Chandra, R. P., Au-Yeung, K., Chanis, C., Roos, A. A., Mabee, W., Chung, P. A., et al. (2011). The influence of pretreatment and enzyme loading on the effectiveness of batch and fed-batch hydrolysis of corn stover. Biotechnol. Prog. 27, 77–85. doi: 10.1002/btpr.508
Cui, M., Zhang, Y., Huang, R., Su, R., Qi, W., and He, Z. (2014). Enhanced enzymatic hydrolysis of lignocellulose by integrated decrystallization and fed-batch operation. RSC Adv. 4, 44659–44665. doi: 10.1039/C4RA08891C
Ganesh, K., Joshi, J. B., and Sawant, S. B. (2000). Cellulase deactivation in a stirred reactor. Biochem. Eng. J. 4, 137–141. doi: 10.1016/S1369-703X(99)00045-5
Gao, Y., Xu, J., Yuan, Z., Zhang, Y., Liu, Y., and Liang, C. (2014). Optimization of fed-batch enzymatic hydrolysis from alkali-pretreated sugarcane bagasse for high-concentration sugar production. Bioresour. Technol. 167, 41–45. doi: 10.1016/j.biortech.2014.05.034
Gaur, R., Agrawal, R., Kumar, R., Ramu, E., Bansal, V. R., Gupta, R. P., et al. (2015). Evaluation of recalcitrant features impacting enzymatic saccharification of diverse agricultural residues treated by steam explosion and dilute acid. RSC Adv. 5, 60754–60762. doi: 10.1039/C5RA12475A
Geng, W., Jin, Y., Jameel, H., and Park, S. (2015). Strategies to achieve high-solids enzymatic hydrolysis of dilute-acid pretreated corn stover. Bioresour. Technol. 187, 43–48. doi: 10.1016/j.biortech.2015.03.067
Gottschalk, L. M. F., Oliveira, R. A., and da Silva Bon, E. P. (2010). Cellulases, xylanases, β-glucosidase and ferulic acid esterase produced by Trichoderma and Aspergillus act synergistically in the hydrolysis of sugarcane bagasse. Biochem. Eng. J. 51, 72–78. doi: 10.1016/j.bej.2010.05.003
Gupta, R., Kumar, S., Gomes, J., and Kuhad, R. C. (2012). Kinetic study of batch and fed-batch enzymatic saccharification of pretreated substrate and subsequent fermentation to ethanol. Biotechnol. Biofuels 5, 16. doi: 10.1186/1754-6834-5-16
Hodge, D. B., Nazmul Karim, M., Schell, D. J., and McMillan, J. D. (2008). Soluble and insoluble solids contributions to high-solids enzymatic hydrolysis of lignocellulose. Bioresour. Technol. 99, 8940–8948. doi: 10.1016/j.biortech.2008.05.015
Hou, W., An, R., Zhang, J., and Bao, J. (2016a). On-site measurement and modeling of rheological property of corn stover hydrolysate at high solids content. Biochem. Eng. J. 107, 61–65. doi: 10.1016/j.bej.2015.12.004
Hou, W., Zhang, L., Zhang, J., and Bao, J. (2016b). Rheology evolution and CFD modeling of lignocellulose biomass during extremely high solids content pretreatment. Biochem. Eng. J. 105, 412–419. doi: 10.1016/j.bej.2015.10.021
Jönsson, L. J., Alriksson, B., and Nilvebrant, N.-O. (2013). Bioconversion of lignocellulose: inhibitors and detoxification. Biotechnol. Biofuels 6, 16–16. doi: 10.1186/1754-6834-6-16
Kapoor, M., Soam, S., Agrawal, R., Gupta, R. P., Tuli, D. K., and Kumar, R. (2017). Pilot scale dilute acid pretreatment of rice straw and fermentable sugar recovery at high solid loadings. Bioresour. Technol. 224, 688–693. doi: 10.1016/j.biortech.2016.11.032
Larsen, J., Haven, M. Ø., and Thirup, L. (2012). Inbicon makes lignocellulosic ethanol a commercial reality. Biomass Bioenergy 46, 36–45. doi: 10.1016/j.biombioe.2012.03.033
Li, M., Pu, Y., and Ragauskas, A. J. (2016). Current understanding of the correlation of lignin structure with biomass recalcitrance. Front. Chem. 4:45. doi: 10.3389/fchem.2016.00045
Ma, X., Yue, G., Yu, J., Zhang, X., and Tan, T. (2011). Enzymatic hydrolysis of cassava bagasse with high solid loading. J. Biobased Mater. Bioenergy 5, 275–281. doi: 10.1166/jbmb.2011.1138
Modenbach, A. A., and Nokes, S. E. (2013). Enzymatic hydrolysis of biomass at high-solids loadings–A review. Biomass Bioenergy 56, 526–544. doi: 10.1016/j.biombioe.2013.05.031
Park, J. M., Oh, B.-R., Seo, J.-W., Hong, W.-K., Yu, A., Sohn, J.-H., et al. (2013). Efficient production of ethanol from empty palm fruit bunch fibers by fed-batch simultaneous saccharification and fermentation using saccharomyces cerevisiae. Appl. Biochem. Biotechnol. 170, 1807–1814. doi: 10.1007/s12010-013-0314-z
Roberts, K. M., Lavenson, D. M., Tozzi, E. J., McCarthy, M. J., and Jeoh, T. (2011). The effects of water interactions in cellulose suspensions on mass transfer and saccharification efficiency at high solids loadings. Cellulose 18, 759–773. doi: 10.1007/s10570-011-9509-z
Rosgaard, L., Andric, P., Dam-Johansen, K., Pedersen, S., and Meyer, A. S. (2007). Effects of substrate loading on enzymatic hydrolysis and viscosity of pretreated barley straw. Appl. Biochem. Biotechnol. 143, 27–40. doi: 10.1007/s12010-007-0028-1
Saddler, J. N., Hogan, C. M., and Louis-Seize, G. (1985). A comparison between the cellulase systems of Trichoderma harzianum E58 and Trichoderma reesei C30. Appl. Microbiol. Biotechnol. 22, 139–145. doi: 10.1007/BF00250034
Saini, J. K., Agrawal, R., Satlewal, A., Saini, R., Gupta, R., Mathur, A., et al. (2015). Second generation bioethanol production at high gravity of pilot-scale pretreated wheat straw employing newly isolated thermotolerant yeast Kluyveromyces marxianus DBTIOC-35. RSC Adv. 5, 37485–37494. doi: 10.1039/C5RA05792B
Sanchez-Rangel, J. C., Benavides, J., Heredia, B. J., Cisneros-Zevallos, L., and Jacobo-Velazquez, D. A. (2013). The Folin-Ciocalteu assay revisited: improvement of its specificity for total phenolic content determination. Anal. Methods 5, 5990–5999. doi: 10.1039/c3ay41125g
Satlewal, A., Agrawal, R., Bhagia, S., Das, P., and Ragauskas, A. J. (2018a). Rice straw as a feedstock for biofuels: availability, recalcitrance, and chemical properties. Biofuels Bioproducts Biorefining 12, 83–107. doi: 10.1002/bbb.1818
Satlewal, A., Agrawal, R., Bhagia, S., Sangoro, J., and Ragauskas, A. J. (2018b). Natural deep eutectic solvents for lignocellulosic biomass pretreatment: recent developments, challenges and novel opportunities. Biotechnol. Adv. doi: 10.1016/j.biotechadv.2018.08.009. [Epub ahead of print].
Satlewal, A., Saini, J. K., Agrawal, R., Mathur, A., Tuli, D. K., and Adsul, M. (2017). “Indian Biofuel Progress, GHG Emission and GHG Savings by Biofuels: Comparative Assessment with World,” in Sustainable Biofuels Development in India, eds C. K. Anuj and S. K. Rajeev (Cham: Springer International Publishing), 541–557. doi: 10.1007/978-3-319-50219-9_22
Sharma, B., Agrawal, R., Singhania, R. R., Satlewal, A., Mathur, A., Tuli, D., et al. (2015). Untreated wheat straw: potential source for diverse cellulolytic enzyme secretion by Penicillium janthinellum EMS-UV-8 mutant. Bioresour. Technol. 196, 518–524. doi: 10.1016/j.biortech.2015.08.012
Skiba, E. A., Budaeva, V. V., Baibakova, O. V., Zolotukhin, V. N., and Sakovich, G. V. (2017). Dilute nitric-acid pretreatment of oat hulls for ethanol production. Biochem. Eng. J. 126, 118–125. doi: 10.1016/j.bej.2016.09.003
Sluiter, A., Hames, B., Ruiz, R., Scarlata, C., Sluiter, J., Templeton, D., et al. (2008a). Determination of Structural Carbohydrates and Lignin in Biomass. Golden, CO: National Renewable Energy Laboratory; NREL Laboratory Analytical Procedures.
Sluiter, A., Hames, B., Ruiz, R., Scarlata, C., Sluiter, J., Templeton, D., et al. (2011). Determination of Structural Carbohydrates and Lignin in Biomass. Golden, CO: National Renewable Energy Laboratory.
Sluiter, A., Hyman, D., Payne, C., and Wolfe, J. (2008b). Determination of Insoluble Solids in Pretreated Biomass Material. Golden, CO: Laboratory Analytical Procedure (LAP) USA: National Renewable Energy Laboratory.
Sugiharto, Y. E., Harimawan, A., Kresnowati, M. T., Purwadi, R., Mariyana, R., Andry Fitriana, H. N., et al. (2016). Enzyme feeding strategies for better fed-batch enzymatic hydrolysis of empty fruit bunch. Bioresour. Technol. 207, 175–179. doi: 10.1016/j.biortech.2016.01.113
Wanderley, M. C., Martin, C., Rocha, G. J., and Gouveia, E. R. (2013). Increase in ethanol production from sugarcane bagasse based on combined pretreatments and fed-batch enzymatic hydrolysis. Bioresour. Technol. 128, 448–453. doi: 10.1016/j.biortech.2012.10.131
Wang, W., Zhuang, X., Tan, X., Wang, Q., Chen, X., Yu, Q., et al. (2018). Dual effect of nonionic surfactants on improving the enzymatic hydrolysis of lignocellulose. Energy Fuels 32, 5951–5959. doi: 10.1021/acs.energyfuels.8b00225
Wang, W., Zhuang, X., Yuan, Z., Yu, Q., Qi, W., Wang, Q., et al. (2012). High consistency enzymatic saccharification of sweet sorghum bagasse pretreated with liquid hot water. Bioresour. Technol. 108, 252–257. doi: 10.1016/j.biortech.2011.12.092
Wenjian, G., Haynes, D. R., and Lee, Y. Y. (2014). Effects of cationic polyelectrolytes on enzymatic hydrolysis of pulp mill sludge under high solid loading. J. Sci. Technol. Forest Products Process. 4, 36–43.
Yang, J., Zhang, X., Yong, Q., and Yu, S. (2011). Three-stage enzymatic hydrolysis of steam-exploded corn stover at high substrate concentration. Bioresour. Technol. 102, 4905–4908. doi: 10.1016/j.biortech.2010.12.047
Zhang, L.-P., Zhang, J., Li, C.-H., and Bao, J. (2014). Rheological characterization and CFD modeling of corn stover–water mixing system at high solids loading for dilute acid pretreatment. Biochem. Eng. J. 90, 324–332. doi: 10.1016/j.bej.2014.06.018
Keywords: pretreatment, hydrolysis, rice straw, surfactant, fed-batch, high solid
Citation: Agrawal R, Bhadana B, Mathur AS, Kumar R, Gupta RP and Satlewal A (2018) Improved Enzymatic Hydrolysis of Pilot Scale Pretreated Rice Straw at High Total Solids Loading. Front. Energy Res. 6:115. doi: 10.3389/fenrg.2018.00115
Received: 19 May 2018; Accepted: 15 October 2018;
Published: 15 November 2018.
Edited by:
Sachin Kumar, Sardar Swaran Singh National Institute of Renewable Energy, IndiaReviewed by:
Pratibha Dheeran, Maharaj Singh College, Saharanpur, IndiaWenjian Guan, Harvard University, United States
Copyright © 2018 Agrawal, Bhadana, Mathur, Kumar, Gupta and Satlewal. This is an open-access article distributed under the terms of the Creative Commons Attribution License (CC BY). The use, distribution or reproduction in other forums is permitted, provided the original author(s) and the copyright owner(s) are credited and that the original publication in this journal is cited, in accordance with accepted academic practice. No use, distribution or reproduction is permitted which does not comply with these terms.
*Correspondence: Alok Satlewal, c2F0bGV3YWxhQGluZGlhbm9pbC5pbg==; YWxvay5zYXRsZXdhbEBnbWFpbC5jb20=
†Present Address: Bharti Bhadana, Etudiante de Recherche, Local 3335A, Doctrante en Science de L'eau, L'Institut national de la recherche scientifique - Eau Terre Evironnement, Ville de Quebec, QC, Canada