- 1Department of Wildlife, Fish, and Environmental Studies, Swedish University of Agricultural Sciences, Umeå, Sweden
- 2Meat and Fish Unit, Department of Food Science, Swedish University of Agricultural Sciences, Uppsala, Sweden
- 3Department of Forest Biomaterials and Technology, Swedish University of Agricultural Sciences, Umeå, Sweden
Biofuel production using microalgae is a renewable and environmental-friendly alternative to the use of fossil fuels. Microalgae storage lipids are promising resources for biofuel production. In this study, pure strains of the microalgae Scenedesmus dimorphus and Selenastrum minutum were grown in untreated municipal wastewater for six days under mixotrophic conditions. The algae strains were subjected to different stresses such as nutrient deprivation, and 5% (w/v) salinity to trigger lipid production and to study effect on FAME composition. The highest lipid concentrations were found in S. dimorphus (35 and 34%) and in S. minutum (40 and 39%) under nutrient deprivation and 5% salinity, respectively. On the one hand, salt stress decreased biomass production; on the other hand in both S. dimorphus and S. minutum salt stress significantly increased the concentration of saturated fatty acid (SFA) and it decreased the concentration of poly-unsaturated fatty acid (PUFA) contents, which are desirable for the production of good quality biofuel such as biodiesel. Hence our findings show how salt stress could clearly affect FAME composition in short time 1–3 days, greatly improving the FAME quality as source of biofuel.
Introduction
In the last decades, many efforts have been made to investigate alternative energy sources. In the face of significant energy and environmental issues, renewable energy has been designated as a suitable and promising solution (Amin, 2009). Among the existing renewable alternatives to fossil fuels, algae have received increased interest as new biomass source for the production of renewable energy due to several factors, such as rapid growth rates, ubiquitous growth, high yields (7–31 times greater than the next best crop—palm oil). In addition, some algae can be harvested daily, algae biofuel contains no sulfur, it is non-toxic and highly bio-degradable, algal oil can be used as livestock feed, and residue can be processed into ethanol and can reduce carbon emissions (Demirbas and Demirbas, 2011).
Microalgal biomass can be used for the production of different types of renewable fuels such as biodiesel, methane, hydrogen, and ethanol (Velichkova et al., 2013). Microalgae are not only a source of fuel, but also the basis of a wide range of chemical compounds used in industry, food technology, and pharmaceuticals. They can be produced through autotrophic, mixotrophic or heterotrophic cultivation. Most of their essential nutrients can be supplied by wastewater and atmospheric CO2, leading to high productivity and an associated high lipid content, making them an attractive option.
For the commercial production oil-derived biodiesel from microalgae biomass, high lipid productivity of dominant, fast-growing algal strains is a major prerequisite. However, when large amounts of algal biomass are produced under optimal growth conditions, often the lipid content is relatively low; while slow-growing species have typically high lipid contents (Sharma et al., 2012). However, it seems clear that there are great potential benefits in using environmental stress as a tool to induce lipid biosynthesis. Different stresses can be used to improve lipid productivity in microalgae, and many studies have evaluated the effects of various stressors on algae, such as carbon dioxide, pH, UV treatment, temperature, higher salt content (Hanelt et al., 1997; Tchernov et al., 2004; Couee et al., 2006; Guschina and Harwood, 2006), and limited nitrogen availability (Mock and Kroon, 2002; Yang et al., 2013). In a previous study it has been shown that salinity stress increases neutral lipid content of algal cells (Ji et al., 2018). In the fresh water microalga Chlorella sorokiniana grown on BG11 salt stress produced a carbon redistribution from starch and protein to lipid (Kim et al., 2016; Zhang et al., 2018). However in another study dealing with salinity stress in Acutodesmus dimorphus grown on BG11 the carbon redistribution was from protein to lipid and carbohydrate (Chokshi et al., 2017). Other stresses, e.g., related to light, nutrients, and symbiotic relations induce changes in cellular composition (Lv et al., 2010), but have not been exploited much with regards to improving cell yields. Lipids extracted from algal biomass, particularly TAG (Triacylglycerol), are often composed of C16 and C18 series of long chain fatty acids, similar to those of vegetable oils suitable for biodiesel production (Ratledge and Wynn, 2002; Miao and Wu, 2006). Fresh water microalgae grown on BG11 under NaCl stress could change their FAMEs profile with a decrease in the saturated fatty acid content (Zhang et al., 2018).
Our aim was to investigate the effect of stresses induced by nutrient deprivation and high salt content on microalgal biomass and lipid production and FAMEs profile, using pure strains of the freshwater microalgae Scenedesmus dimorphus (S. dimorphus) and Selenastrum minutum (S. minutum) as potential biofuel producers.
Materials and Methods
Materials
Municipal untreated (raw) wastewater was obtained from the nearby wastewater treatment plant in Umeå (VAKIN, Umeå, Sweden). Initial pH of the wastewater was 7.5 ± 0.08. Pure strains of S. dimorphus (417) and S. minutum (326) were bought from UTEX, The Culture Collection of Algae at the University of Texas at Austin (in parenthesis is the strain UTEX id).
Microalgae Cultivation
The two algal strains were separately cultured in a 16 L working volume photobioreactor consisting of a cylindrical transparent glass flask with a top drive motor that stirs an impeller (Wheaton, USA). The algal strains grown in the photobioreactor received municipal untreated wastewater filtered with two layers of towel paper (100% cellulose) with a water filtration velocity of about 1.3 ml cm−2 min−1 to remove the largest particles. The algae were grown at room temperature (22 ± 2°C) under 16:8 h light-dark cycle with an irradiance of 150 μmol m−2 s−1 at the photobioreactor surface. The culture was grown up to six days with continuous agitation without CO2 addition. To investigate a possible role of bacterial growth on nutrients removal and biomass production a non-inoculated control experiment was performed in 1 L Erlenmeyer flask totally covered with aluminum foil to avoid exposing the wastewater to light. Non inoculated controls were kept for six days at room temperature (22 ± 2°C) similarly as above mentioned for growth in the photobioreactor.
Analyses of Ammonium and Total Phosphorus
Ammonium and total phosphorus were measured in untreated wastewater and after six days of algal growth in the photobioreactor and after six days for non-inoculated control. Analyses were performed in duplicate with a spectrophotometer (DR 3900 Hach Lange, Germany) following the manufacturer instructions (Hach Lange, Germany) as previously reported (Gentili, 2014).
Stress Treatments
Six-day-old S. dimorphus and S. minutum cultures grown in the photobioreactor (see above) were subjected to treatments such as nutrient deprivation and 5% (w/v) NaCl in 1–3 day time course. We have chosen 5% salt because it is the concentration found in some chemical industries wastewater effluent. According to unpublished results from previous studies of both S. dimorphus and S. minutum cultures grown in wastewater, the strains are in exponential phase after a cultivation period of six days. The samples were kept in the growth cabinet (Conviron, Canada) under 16 h of light at 22°C and 8 h of dark at 16°C for three days under the optimal light intensity of 150 μmol m−2 s−1 as PAR (photosynthetic active radiation).
Nutrient deprivation stress was created using tap water, pH 8.1, ammonium, nitrate, and phosphate concentrations were < 0.01 mg/l (below the instrument detection limit). For tap water treatment, sterile 50 ml centrifugal plastic tubes received 25 ml of six-day-old algal cultures from the photobioreactor; samples were centrifuged at 3,520 g for 5 min and supernatants were discarded. Subsequently, the remaining pelleted cells of algae were washed with distilled water, resuspended in 25 ml of tap water and kept for up to three days.
For the prolonged growth on original wastewater, sterile 50 ml centrifugal plastic tubes received 25 ml of six-day-old algal cultures from the photobioreactor. Then the algae were kept to grow in the same wastewater used in the photobioreactor. This treatment was used as reference treatment when compared to the nutrient deprivation or the salt stress treatment.
For the 5% salt treatment, NaCl was added to the required volume of six-day-old culture withdrawn from the photobioreactor. Fifteen replicates of six-day-old cultures of S. dimorphus and S. minutum grown in the photobioreactor were used as the day 0 reference to compare with the treated samples. Treated samples were collected daily in fifteen replicates at each time point. Each sample consisted of 25 ml of culture volume in a 50 ml tube.
Microalgae Biomass Harvest
All treated cultures were harvested by centrifugation at 3,520 g for 5 min and the cell pellets were washed with distilled water. The dry weight of algae biomass was determined gravimetrically after overnight oven drying at 65°C. Algae biomass was expressed as dry weight (mg/l). All biomass determination was performed in triplicate.
Total Lipid Extraction
Total lipids were extracted from fresh microalgae biomass using the simplified Folch method previously developed in our laboratory (Axelsson and Gentili, 2014). The lipids were extracted using a mixture of chloroform/methanol (2:1 v/v) and 0.73% NaCl water solution. The quantity of total lipids was measured gravimetrically and expressed as dry weight percentage. Total lipid extraction was performed in five replicates.
Extraction and Determination of Soluble Sugars and Starch
Samples (0.05–0.2 g fresh weight) were extracted as previously described (Tobias et al., 1992). Analyses of sucrose, fructose, and glucose were performed in accordance with (Guglielminetti and Perata, 1995). The pellets containing starch were extracted using 10% KOH, the neutralized supernatants were treated with 2.5 units amyloglucosidase (from Rhizopus niger) (Sigma Aldrich) for 3 h to release glucose. The glucose units released after amyloglucosidase treatment were used for starch quantification (Magneschi and Perata, 2009). All extractions were done in triplicate.
Total Protein Content
Total protein extraction was performed as follows: Samples (0.05–0.2 g fresh weight) were frozen with liquid nitrogen, ground, and extracted in 100–300 μl of test buffer. Test buffer was composed of 100 mM Tris-HCl pH 8.00, 10 mM EDTA, 5% SDS, and 100 mM NaCl. The samples suspended in the buffer were heated at 100°C for 10 min and centrifuged for 10 min at 4°C at a maximum speed of 10,000 n/min−1 (Hettich EBA 12). Supernatants were collected for the analysis of total protein. Total protein content was determined using PierceTM BCA Protein Assay kit. Bovine serum albumin (BSA) was used as standard to quantify the algal proteins. All extractions were done in triplicate.
Fatty Acid Determination
Algae were harvested by centrifugation at 3,520 g for 5 min and the cell pellets were washed with distilled water. Pelleted cells were boiled immediately in 1–2 ml of isopropanol at 80°C for 10 min and stored at −20°C. All samples were brought to room temperature before total lipid extraction. The extracted lipids were dried using a multievaporator under vacuum (Büchi, Switzerland). Once dry, the samples were flushed with N2 at 30°C. Transesterification of fatty acids (FA) into FA methyl esters (FAME) was carried out as follows: 1 ml of 5% H2SO4 in dry methanol was added to the dried samples and flushed briefly with N2 to completely remove air and boiled at 80°C for 2 h. We then added 1 ml of distilled water and 2 ml of pure petroleum ether, vigorously vortexed for 30 s and centrifuged at 1,250 g for 2 min. The upper phase was removed using a long Pasteur pipette and the supernatant was transferred into a clean glass vial. This step was repeated twice, adding only 2 ml of petroleum ether. The samples were completely dried using the multievaporator (Büchi, Switzerland). Dry samples were blown with N2 at room temperature. All the reagents used were of analytical grade (Sigma Aldrich). Dry samples were dissolved in 50–60 μl hexane and 1 ul was injected and analyzed using a gas chromatograph CP 3800 (Varian AB, Stockholm, Sweden) equipped with a flame ionization detector and a split injector and fitted with a 50 m length x 0.22 mm i.d. x 0.25 μm film thickness BPX 70 fused-silica capillary column (SGE, Austin, TX, USA) (Fredriksson Eriksson and Pickova, 2007). The temperatures of the injector and detector were 230 and 250°C, respectively. Fatty acids were identified by comparing their retention times with those of the standard mixture GLC-461 (Nu-check Prep, Elysian, USA). Peak areas were integrated using Galaxie chromatography data system software version 1.9 (Varian AB, Stockholm, Sweden).
Statistical Analysis
The differences were analyzed using one-way analysis of variance, setting the level of significance of < 0.05 (Minitab 18).
Results
Influence of Nutrient Deprivation and Salt Stress on Biochemical Composition of S. dimorphus
After six days, non-inoculated controls showed a limited ammonium and total phosphorus reduction of 12.8 and 9.4% respectively. After six days, control biomass had a mean±SE value of 7 ± 1 mg/L. There was a clear biomass reduction considering that the biomass at experiment start had a mean ± SE value of 16 ± 2.8 mg/L. While after six days of algal growth in the photobioreactor the two algae could reduce ammonium by 79% and total phosphorus by 93.7%.
Biochemical composition is shown in Figure 1; the contents of total lipids, proteins and carbohydrates were analyzed in the prolonged growth in the original wastewater, nutrient deprivation and 5% salt for 1–3 days' time course. Tap water had very limited amounts of nutrients, so it was regarded as a nutrient deprivation stress condition. In nutrient deprivation, the total carbohydrate content of S. dimorphus showed a dramatic reduction over time (Figure 1A). Total carbohydrate content was 56.5% of the dry weight at day 0, 42.2, 38.4, and 38.9% at day 1, day 2 and day 3, respectively (Figure 1) this reduction was statistically significant (Table 1). In nutrient deprivation stress treatment, total protein content had a limited reduction from 22.6% of the dry weight at day 0 to 21.7, 20.1, and 19.4% at day 1, day 2, and day 3, respectively. However, even total protein reduction was statistically significant (Table 1). In particular, it is interesting to observe that lipid content was significantly increased in nutrient deprivation from 17.4% of the dry weight at day 0 to about 24.3, 38.2, and 29.6 at day 1, day 2, and day 3, respectively (Figure 1A, Table 1). Most highly triggered lipid production was observed in nutrient deprivation at day 2.
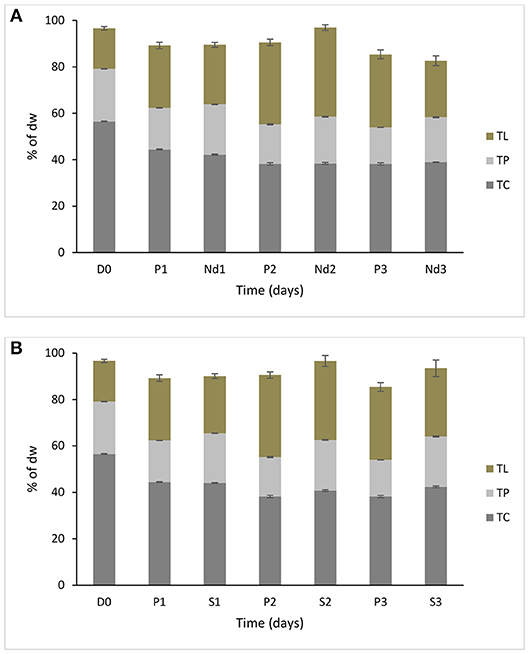
Figure 1. Biochemical composition of S. dimorphus over time (three days) (A) under nutrient deprivation stress and (B) 5% salt stress. Nutrient deprivation stress (Nd1, Nd2, and Nd3) was created by using tap water. Prolonged growth in original wastewater (P1, P2, and P3) algae were kept in municipal wastewater where previously have been grown for 6 days. Treatments of 5% salt stress were represented in S1, S2, and S3. D0 was grown for six days in municipal wastewater before treatment and it represents the start of the experiment. Total lipid (TL), total protein (TP) and total carbohydrate (TC) concentrations are reported as % of biomass dry weight. Bars represent the mean ± SE of five replicates for TL and three replicates for TP and TC.
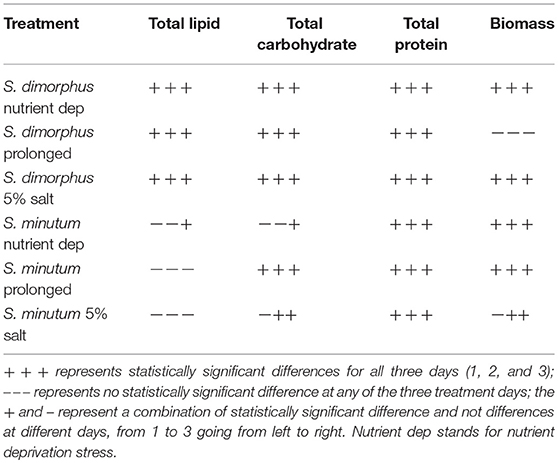
Table 1. Statistical analysis of the treatments compared to the samples at start of the treatments (D0).
In the prolonged growth in original wastewater samples of c1, c2, and c3, both total carbohydrate and total protein contents were decreased from day 0 to day 3 (Figure 1A, Table 1). Total carbohydrate content decreased from 56.5% at day 0 to 44.4% at day 1, 38.2% at day 2, and 37.7% at day 3, while total protein content dropped from 22.6% at day 0 to 17.9% at day 1, 17.0% at day 2, and 15.8 % at day 3. Total lipid content remarkably and significantly increased from day 0 to day 2 in both nutrient deprivation and prolonged growth in original wastewater samples from 17.4 to 38.2% and from 17.4 to 35.1%, respectively (Figure 1A).
During the 5% salt stress, the algae biomass pigmentation changed from green to yellow at day 1. Later, the color of the algal biomass turned to white or mostly colorless with progressing stress reaction in day 2 and 3. We observed an increased lipid content in day 1, day 2, and day 3 as 24.5, 33.8, and 28.9%, respectively, compared to17.4% in day zero (Figure 1B, Table 1). Total carbohydrate content showed a drastic reduction from 56.5% at day 0 to 44.0 % at day 1. We could observe a more limited reduction of total protein content of algal biomass during salt stress from 22.6% at day 0 to 21.4, 21.9, and 21.6 at day 1, 2, and day 3, respectively (Figure 1B); however this reduction was statistically significant (Table 1).
Salt stress clearly negatively affected S. dimorphus biomass production (Table 1). During salt stress, S. dimorphus had a biomass of 335.4 mg/L at day 0 to 251.9 mg/L at day 1, 253.6 mg/L at day 2, and 264.4 mg/L at day 3 (Figure 3A). As shown in Figure 4, S. dimorphus showed a significant reduction of biomass during salt stress of 22.7, 24.4, and 21.2% at day 1, 2, and 3, respectively. We could observe algal cells creating aggregates during the salt treatments as a result of cells excreting mucilage as a response to the unfavorable growth conditions caused by the high salinity.
Based on our results, S. dimorphus shows a reduction in algal biomass not only in 5% salt conditions, but also during nutrient deprivation as 271.4, 260.2, and 270.7 mg/L at day 1, 2, and 3, respectively (Figure 3A; Table 1). However, under prolonged growth in original wastewater conditions, the reduction was more limited and not significant (Table 1). As shown in Figure 3A, biomass production of c1, c2, and c3 was 307.9, 356.2, and 295.7 mg/L, respectively, while at day 0, biomass production was 335.4 mg/L.
Influence of Nutrient Deprivation and Salt Stress on Biochemical Composition of S. minutum
It was interesting to observe that under nutrient deprivation, S. minutum showed a reduction of total carbohydrate content statistically significant only at day 3 (Table 1) and a considerably higher reduction of total protein content compared to S. dimorphus (compared Figures 1A, 2A). Total carbohydrate content was less reduced than total protein, varying from 45.4% of the dry weight at day 0 to 45.0, 42.7, and 41.3% at day 1, day 2, and day 3, respectively (Figure 2A). Total protein content was significantly reduced from 22.9% of dry weight at day 0 to 17.3% at day 1 and 15.8% at day 2 in nutrient deprivation samples (Figure 2A, Table 1). Even though we could observe a substantial increment of total lipid content at day 1, 2, and 3 as 29.0, 32.6, and 35.5%, respectively (Figure 3), this increase was not statistically significant (Table 1). We found that biomass of S. minutum significantly increased over time with nutrient deprivation (Figure 3B, Table 1). Algal biomass increased from 78.4 mg/L at day 0 to 91.0 mg/L at day 1, 99.8 mg/L at day 2, and 92.0 mg/L at day 3 (Figure 3B). The behavior of S. minutum and S. dimorphus showed two different patterns of biomass accumulation during nutrient deprivation (Figures 3A,B).
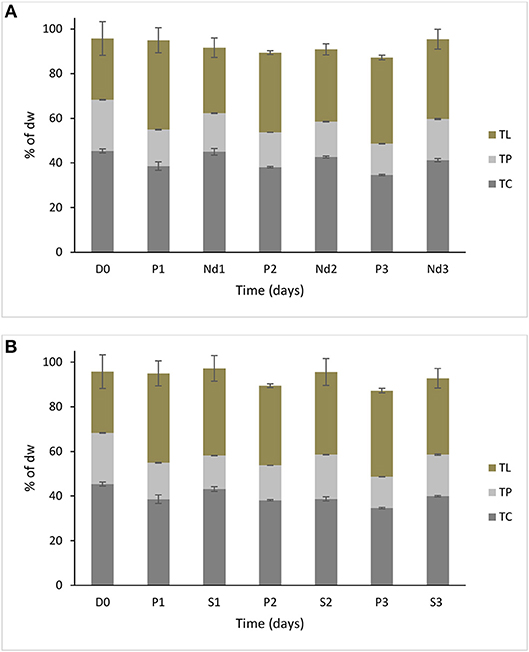
Figure 2. Biochemical composition of S. minutum over time (three days) (A) in nutrient deprivation stress and B) 5% salt stress. Nutrient deprivation stress (Nd1, Nd2, and Nd3) was created by using tap water. Prolonged growth in original wastewater (P1, P2, and P3) algae were kept in municipal wastewater were previously have been grown for 6 days. Treatments of 5% salt stress were represented in S1, S2, and S3.D0 was grown for six days in municipal wastewater before treatment and it represents the start of the experiment. Total lipid (TL), total protein (TP), and total carbohydrate (TC) concentrations are reported as % of biomass dry weight. Bars represent the mean ± SE of five replicates for TL and three replicates for TP and TC.
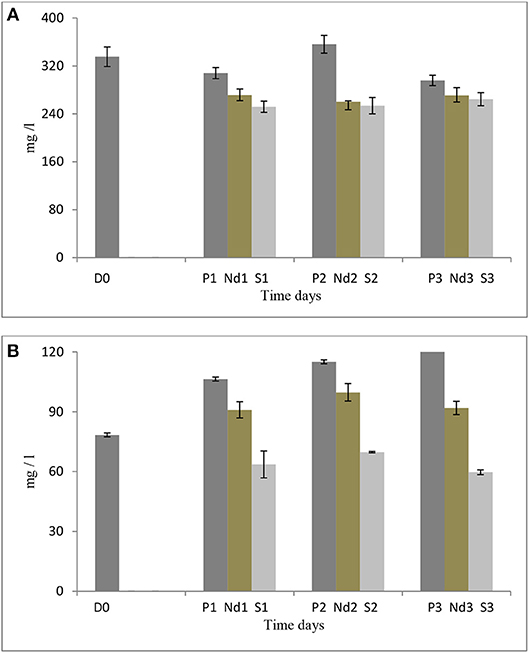
Figure 3. (A) Biomass production of S. dimorphus over time (three days) in prolonged growth, nutrient deprivation and 5% salt stress. (B) Biomass production of S. minutum over time (three days) in prolonged growth, nutrient deprivation, and 5% salt stress. Nutrient deprivation stress was created by using tap water while salt stress was created by using 5% NaCl. Prolonged growth in original wastewater P1, P2, and P3 algae were kept in municipal wastewater were previously have been grown for 6 days. D0 was grown for six days in municipal wastewater before treatment and it represents the start of the experiment. Biomass is reported as dry weight per liter (mg/L). Bars represent the mean ± SE of three replicates.
The prolonged growth in original wastewater treatments c1, c2, and c3 had a stimulating effect on total lipid content of S. minutum (Figure 2A). Total lipid contents increased at day 1, 2, and 3, respectively; however the increase was not statistically significant (Table 1). We clearly observed a statistically significant reduction of both total carbohydrate and total protein contents in the prolonged growth in original wastewater treatments (Figure 2A, Table 1). Hereby, total protein content was reduced from 22.9% of dry weight at day 0 to 16.3% at day 1, 15.6% at day 2, and 14.0% at day 3, while total carbohydrate content was reduced from 45.4% at day 0 to 38.6% at day 1, 37.4% at day 2, and 34.6% at day 3 (Figure 2A). As shown in Figure 3B, algal biomass of S. minutum in prolonged growth in original wastewater treatment significantly increased from 78.4 mg/L at day 0 to 106.4 mg/L at day 1, 115.0 mg/L at day 2, and 122.0 mg/L at day 3 (Table 1). We could confirm that in S. minutum, both nutrient deprivation and prolonged growth in original wastewater had a similar pattern in terms of lipid accumulation, biomass gain and total protein reduction during the 3 days' time course.
Total lipid content of S. minutum was influenced by the 5% salt stress (Figure 2B) at day 1, 2, and 3 but the differences were not statistically significant (Table 1). According to Figure 4, total carbohydrate content was reduced from 45.4% at day 0 to 43.1% at day 1, 38.7% at day 2, and 39.9% at day 3; while total protein content displayed the highest reduction from 22.9% at day 0 to 15.0% at day 1. It was clear that during the 5% salt stress, S. minutum biomass was reduced, from 78.4 mg/L at day 0 to 63.6 mg/L at day 1, 69.8 mg/L at day 2, and 59.7 mg/L at day 3 (Figure 3B). The biomass reduction of S. minutum was reported as 10.6, 11.0, and 23.9% in day 1, 2, and 3, respectively, in 5% salt stress (Figure 4) and it was statistically significant for day 2 and 3 (Table 1).
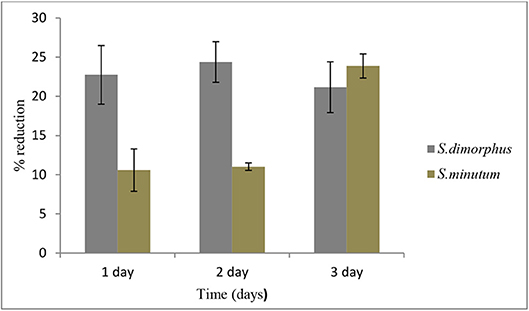
Figure 4. Biomass reduction of S. dimorphus and S. minutum over time (D1, D2, and D3) in 5% salt stress. Biomass reduction values are presented as % reduction with the reference to day 0. Bars represent mean ± SE of five replicates.
Relative Percentage of Various Fatty Acid Methyl Esters (FAMEs) Produced During Nutrient Deprivation and Salt Stress
The extracted lipids from both S. dimorphus and S. minutum in prolonged growth, nutrient deprivation and 5% salinity consisted of C16 and C18 groups (Table 2) that accounted approximately for 80–88% of total fatty acids. Relative percentage of saturated fatty acids (SFA) was higher than that of unsaturated fatty acids, such as monounsaturated fatty acids (MUFA) and poly-unsaturated fatty acids (PUFA), in both S. dimorphus and S. minutum under 5% salt stress (Table 2). Our results revealed that relative percentage of unsaturated fatty acids, such as MUFA and PUFA, was higher than that of SFA in both S. dimorphus and S. minutum in prolonged growth and nutrient deprivation (Tables 1, 2).
As shown in Table 2, in S. dimorphus, palmitic acid contents were 21.6, 20.6, and 40.5% in prolonged growth, nutrient deprivation and 5% salinity, respectively. Fatty acids belonging to the C18:1 group had a concentration of 23.3, 21.2, and 13.7% in S. dimorphus in prolonged growth, nutrient deprivation, and 5% salt stress samples for the 3 days' time period, respectively (Table 2). Polyunsaturated fatty acids, such as C18:3, were accumulated at 24.1% in prolonged growth, 29.7% in nutrient deprivation and 4.16 in 5% salt stress (Table 2). Under salt stress, stearic acid (C18:0) was similar in prolonged growth and nutrient deprivation at 2.5 and 2.7%, respectively, while at 11.2% under salt stress (Table 2). Hence, stearic acid content was remarkably higher in 5% salinity, which is 4.5 and 4.1 fold higher than in the prolonged growth and in nutrient deprivation samples. Extracted lipids from S. dimorphus were reported to have cis-9,12-linoleic acid at 5.3, 5.3, and 3.8%, respectively, in prolonged growth, nutrient deprivation and in 5% salt stress. However, the lipids extracted from both S. dimorphus and S. minutum in all three treatments had less polyunsaturated methyl esters than saturated and monounsaturated methyl esters (Table 2).
According to the lipid profile of S. minutum (Table 2), palmitic acid concentration was 29.3, 31.2, and 44.6% in prolonged growth, nutrient deprivation and 5% salinity, respectively. Stearic acid levels were 3.3% in prolonged growth, 4.5% in nutrient deprivation and 11.2% in salt 5% condition. As a consequence, stearic acid content in salt stress samples were 3.4 fold higher than in prolonged growth samples and 2.5 fold higher than in nutrient deprivation samples (Table 2). Based on Table 2, the fatty acid distribution of both S. dimorphus and S. minutum in all three treatments showed more palmitic acid (C16:0) and oleic acid (C18:1 group) and less linoleic (C18:2 n-6) and linolenic acid (C18:3 group). The ratio SFA/PUFA and MUFA/PUFA (Table 3) reveals a higher value of saturated fatty acids and monounsaturated fatty acids than of polyunsaturated fatty acids in S. minutum in all three treatments. However, for S. dimorphus, the ratios SFA/PUFA and MUFA/PUFA (Table 3) displayed the highest value in 5% salinity, while nutrient deprivation and prolonged growth treatment obtained lower ratio values.

Table 3. Fatty acid ratios of S. dimorphus and S. minutum in prolonged growth on original wastewater, nutrient deprivation (Nutrient dep), and 5% salt condition.
Discussion
Based on the literature, neutral lipids accumulation begins at the end of the log phase or in the stationary phase, when the nutrient content of the growth medium is -limited (Ratledge and Cohen, 2008). Our data of S. dimorphus find a confirmation in a study dealing with a similar algae strain such as Scenedesmus obliquus, where it was shown that NaCl salt could stimulate the accumulation of neutral lipids, decrease the content of chlorophyll a, b and carotenoids (Ji et al., 2018). In our study we found that in S. dimorphus nutrient and salt stress stimulated a reduction of carbohydrates and protein and an increase in lipids; these findings are in agree with previous studies dealing with Chlorella sorokiniana where salt stress induced a shift from proteins and starch to lipid synthesis (Kim et al., 2016; Zhang et al., 2018). According to the findings of (Yang et al., 2013), nitrogen deprivation triggers metabolic pathways for converting membrane lipids to TAG in P. tricornutum. Previous studies on Nannochloropsis sp. have reported that nitrogen limitation or deprivation can generate a significant accumulation of saturated and monounsaturated fatty acids (Hu and Guo, 2006) as well as decreased percentages of polyunsaturated fatty acids in the total fatty acids (Dong et al., 2013). These fatty acids are mainly associated to TAG storage, the preferred substrate for biodiesel production (Ratledge and Wynn, 2002; Miao and Wu, 2006).
The color change at 5% salinity was a good indicator of pigment degradation, followed by cell death. In a previous study has been shown that chlorophyll degradation occurred in Chlorella sorokiniana under salinity stress (Kim et al., 2016). In the prokaryotic blue-green alga Anabaena sp. (Ning et al., 2002) and in yeast was found that vacuolization occurs after salt stress (Huh et al., 2002). It has been considered as a cytological indicator of programmed cell death (Affenzeller et al., 2009). The 5% salt stress triggered lipid production in S. dimorphus, this is in agreement to what has previously been found in fresh water microalgae, where salt stress could stimulate lipid accumulation (Salama et al., 2013).
Under nutrient deprivation, total protein content was significantly reduced; this could be ascribed to the fact that under nitrogen starvation, the carbon dioxide fixed is converted into carbohydrates or lipids rather than proteins due to unavailability of nitrogen (Richardson et al., 1969). Another possible explanation could be that under nitrogen starvation, the consumption of NADPH decreased due to the scarcity of nitrogen, which inhibits the amino acid synthesis pathways, particularly the reaction from α-ketoglutarate to glutamate that generates an excessive accumulation of NADPH in the cells (Lee et al., 2001). Nutrient deprivation had a stimulating effect on total lipid content of S. minutum (Figure 2A). It is well known that for the synthesis of fatty acids, NADPH acts as a reducing agent and plays a crucial role in the two-step reduction process of fatty acid biosynthesis (Hutchings et al., 2005).
It has been shown that stress conditions produce lipid accumulation during photosynthesis (Courchesne et al., 2009) through an up-regulation of the enzyme Acetyl CoA carboxylase (ACCase) and lead to a possible increase of the carbon flux into the fatty acid synthesis pathway in photosynthetic organisms, including the green microalga C. vulgaris (Guarnieri et al., 2011).
Even though salt-induced osmotic stress can stimulate lipid accumulation, its effects on cell growth is largely unknown. According to (Rao et al., 2007), high salinity can stimulate accumulation of intracellular lipids in microalgae. In a recent study dealing with Neochloris oleoabundans under salt and nitrogen stress, it has been shown that proline and the ascorbate-glutathione cycle play a key role for successful osmoregulation under salt stress; furthermore two genes such as glycerol-3-phosphate acyltransferase and glycerol-3-phosphate dehydrogenase where identified as promising targets to enhance TAG production (de Jaeger et al., 2018). Hence a possible explanation of our results is that under the stresses studied in S. dimorphus glycerol-3-phosphate dehydrogenase and glycerol-3-phosphate dehydrogenase are regulated in a way that the carbohydrate accumulation is reduced while lipid accumulation is stimulated as shown in Figure 1. In other words the cellular carbon flow is conveyed to lipid production instead of carbohydrate.
In agreement with a previous study (Couee et al., 2006), lipid production of microalgae can be grouped into two categories: storage lipids (non-polar lipids) and structural lipids (polar lipids). However, during nutrient stress, 80% of the accumulated lipids are comprised of TAGs storage lipids (Tornabene et al., 1983; Kawata et al., 1998), which can be transesterified to produce biodiesel.
Our study shows that both under nutrient deprivation and prolonged growth in original wastewater, total lipid content especially of S. dimorphus was remarkably high (Figure 1A). Interestingly, lipid production increased even if related to biomass production (compare Figures 1, 2 with Figures 3A,B), similarly to what was previously shown for marine microalgal strains (Rodolfi et al., 2009). However, concerning the relationship between biomass and lipid production under salt stress the two algal strains behaved differently as underlined by the fact that S. dimorphus even with a clear reduction of biomass still accumulated lipid when compared to day 0; while in S. minutum the reduction of biomass was not balanced by a large lipid production when compared to day 0.
Lipids produced from microalgal strains usually consist of fatty acids comprising mainly C16 and C18 fatty acids, which are very similar to those of vegetable oils (Miao and Wu, 2006); C16 and C18 fatty acids are suitable for biodiesel production (Miao and Wu, 2006).
Our results show that the dominant fatty acids of S. dimorphus and S. minutum in prolonged growth, nutrient deprivation and 5% salinity were C16 and C18 fatty acids (Table 2) similarly to what previously shown (Salama et al., 2013). Therefore, the extracted oils can be used as basic material for the production of biodiesel (Miao and Wu, 2006). Particularly interesting was the effect of salinity stress on the fatty acids quality of both algal strains, as shown by the clear reduction of the unsaturated fatty acids 18:3 and 18:1 and the increase of the saturated 16:0 and 18:0 (Table 2). Hence the salinity stress could generate lipid suitable for warmer climates where cold-flow properties are less important.
However, our results concerning the salt stress effects on fatty acids quality are different to that previously for two freshwater microalgae Chlamydomonas mexicana and Scenedesmus obliquus where salt stress increased the concentration of 18:1 and 18:3 fatty acids (Salama et al., 2013).
The presence of polyunsaturated methyl esters in algal oil greatly affects the oxidative stability of biodiesel (Knothe, 2006). The susceptibility to oxidation of the double bonds during storage, reduces the acceptability of microalgal oil for production of biodiesel (Chisti, 2007).
The increased relative percentage of C16:0 and the decreased percentage of C18:3 group fatty acids will result in a fatty acid profile that is most likely to yield biodiesel with poor cold-flow properties and good oxidative stability (Knothe, 2009). The C 18:1 group of the fatty acid content of S. minutum was 25.2% in prolonged growth, 26.5% in nutrient deprivation, and 20.4% in 5% salinity (Table 2). A considerably higher level of oleic acid (C 18:1 group) is desirable for a good quality biodiesel (Singh and Mallick, 2014).
Both of the algal strains we studied had high values of SFA/PUFA and MUFA/PUFA ratios in 5% salinity (Table 3), which is desirable for good quality biodiesel as they guarantee oxidative stability of the fuel (Singh and Mallick, 2014). The extracted lipids from both S. dimorphus and S. minutum in in all three treatments showed satisfying overall properties suitable for biodiesel production.
From an application point of view we believe that salt water present in industrial effluent and of course in sea water can be successfully used to induce lipids accumulation but especially fatty acids saturation in fresh water microalgae. The induction of lipid accumulation and fatty acids saturation occurs in short time as shown in the present study; hence this treatment has potential industrial application.
Future studies should focus on the optimization of the salt stress at larger scale in industrial sites thus to investigate the feasibility of such process.
Author Contributions
NP and FG conceived and designed the experiments. NP performed the experiments and analyzed the data. JP performed part of the experiments and analyzed part of the data. FG performed part of the experiments and part of the data analysis. NP wrote the paper. FG wrote part of the paper. JP gave important input during the writing process.
Conflict of Interest Statement
The authors declare that the research was conducted in the absence of any commercial or financial relationships that could be construed as a potential conflict of interest.
Acknowledgments
We greatly appreciate the financial support of the Kempe Foundation (JCK-1512) and the Swedish Energy Agency (38239-1). The availability of the technical staff at Vakin was greatly appreciated. We thank Dr. John Ball for language editing.
References
Affenzeller, M. J., Darehshouri, A., Andosch, A., Lütz, C., and Lütz-Meindl, U. (2009). Salt stress-induced cell death in the unicellular green alga Micrasterias denticulaata. J. Exp. Bot. 60, 939–954. doi: 10.1093/jxb/ern348
Amin, S. (2009). Review on biofuel oil and gas production processes from microalgae. Energy Conv. Manage. 50, 1834–1840. doi: 10.1016/j.enconman.2009.03.001
Axelsson, M., and Gentili, F. (2014). A single –step method for rapid extraction of total lipids for green microalgae. PLoS ONE 9:e89643. doi: 10.1371/journal.pone.0089643
Chisti, Y. (2007). Biodiesel from microalgae. Biotechnol. Adv. 25, 294–306. doi: 10.1016/j.biotechadv.2007.02.001
Chokshi, K., Pancha, I., Ghosh, A., and Mishra, S. (2017). Salinity induced oxidative stress alters the physiological responses and improves the biofuel potential of green microalgae Acutodesmus dimorphus. Bioresour. Tech. 244, 1376–1383 doi: 10.1016/j.biortech.2017.05.003
Couee, I., Sulmon, C., Gouesbet, G., and Amrani, A. E. (2006). Involvement of soluble sugars in reactive oxygen species balance and responses to oxidative stress in plants. J. Exp. Bot. 57, 449–459. doi: 10.1093/jxb/erj027
Courchesne, N. M. D., Parisien, A., Wang, B., and Lan, C. Q. (2009). Enhancement of lipid production using biochemical, genetic and transcription factor engineering approaches. J. Biotech. 141, 31–41. doi: 10.1016/j.jbiotec.2009.02.018
de Jaeger, L., Carreres, B. M., Springer, J., Schaap, P. J., Eggink, G., Martins, Dos Santos, V. A. P., et al. (2018). Neochloris oleoabundans is worth its salt: transcriptomic analysis under salt and nitrogen stress. PLoS ONE 13:e0194834. doi: 10.1371/journal.pone.0194834
Demirbas, A., and Demirbas, A. F. (2011). Importance of algae oil as a source of biodiesel. Energy Conv. Manage. 52, 163–170. doi: 10.1016/j.enconman.2010.06.055
Dong, H. P., Williams, E., Wang, D. Z., Xie, Z. X., Hsia, R. C., Jenck, A., et al. (2013). Responses of Nannochloropsis oceanica IMETI to long-term nitrogen starvation and recovery. Plant Physiol. 162, 1110–1126. doi: 10.1104/pp.113.214320
Fredriksson Eriksson, S., and Pickova, J. (2007). Fatty acids and tocopherol levels in M. Longissimus dorsi of beef cattle in Sweden – a comparison between seasonal diets. Meat Science 76, 746–754. doi: 10.1016/j.meatsci.2007.02.021
Gentili, F. G. (2014). Microalgal biomass and lipid production in mixed municipal, dairy, pulp and paper wastewater together with added flue gases. Bioresou. Technol. 169, 27–32. doi: 10.1016/j.biortech.2014.06.061
Guarnieri, M. T., Nag, A., Smolinski, S. L., Darzins, A., Seibert, M., and Pienkos, P. T. (2011). Examination of triacylglycerol biosynthetic pathways via de novo transcriptomic and proteomic analyses in an unsequenced microalga. PLoS ONE 6:e25851. doi: 10.1371/journal.pone.0025851
Guglielminetti, L., and Perata, P. A. (1995). AEffect of anoxia on carbohydrate metabolism in rice seedlings. Plant Physiol. 108, 735–741. doi: 10.1104/pp.108.2.735
Guschina, I. A., and Harwood, L. J. (2006). Lipids and lipid metabolism in eukaryotic algae. Prog. Lipid Res. 45, 160–186. doi: 10.1016/j.plipres.2006.01.001
Hanelt, D., Melchersmann, B., Wiennke, C., and Nultsch, W. (1997). Effects of high light stress on photosynthesis of polar macroalgae in relation to depth distribution. Mar. Ecol. Prog. Ser. 149, 255–266. doi: 10.3354/meps149255
Hu, H., and Guo, K. (2006). Response of the growth and fatty acid composition of Nannochloropsis sp. To environmental factors under elevated CO2 concentration. Biotechnol. Lett. 28, 987–992. doi: 10.1007/s10529-006-9026-6
Huh, G. H., Damsz, B., Matsumoto, T. K., Reddy, M. P., Rus, A. M., Ibeas, J. I., et al. (2002). Salt causes ion disequilibrium-induced programmed cell death in yeast and plants. Plant J. 29, 649–659. doi: 10.1046/j.0960-7412.2001.01247.x
Hutchings, D., Rawsthorne, S., and Emes, M. (2005). Fatty acid synthesis and the oxidative pentose phosphate pathway in developing embryos of oilseed rape (Brassica napus L.). J. Exp. Bot. 56, 577–585. doi: 10.1093/jxb/eri046
Ji, X., Cheng, J., Gong, D., Zhao, X., Qi, Y., Su, Y., et al. (2018). The effect of NaCl stress on photosynthetic efficiency and lipid production in freshwater microalga—Scenedesmus obliquus XJ002. Sci. Total Environ. 633, 593–599. doi: 10.1016/j.scitotenv.2018.03.240
Kawata, M., Nanba, M., Matsukawa, R., Chihara, M., and Karube, I. (1998). Isolation and characterization of a green alga Neochloris sp. for CO2 fixation. Stud. Surf. Sci. Catal. 114, 637–640. doi: 10.1016/S0167-2991(98)80840-9
Kim, B. H., Ramanan, R., Kang, Z., Cho, D. H., Oh, H. M., and Kim, H. S. (2016). Chlorella sorokiniana HS1, a novel freshwater green algal strain, grows and hyperaccumulates lipid droplets in seawater salinity. Biomass Bioenergy 85, 300–305. doi: 10.1016/j.biombioe.2015.12.026
Knothe, G. H. (2006). Analyzing biodiesel: standards and other methods. J. Am. Oil Chem. Soc. 83, 823–833. doi: 10.1007/s11746-006-5033-y
Knothe, G. H. (2009). Improving biodiesel fuel properties by modifying fatty ester composition. Energy Environ. Sci. 2, 759–766. doi: 10.1039/b903941d
Lee, S. Y., Hong, S. H., Park, S. J., van Wegen, R., and Middelberg, A. P. J. (2001). “Metabolic flux analysis on the production of poly (3-hydroxybutyrate),” in Polyesters I: Biological systems and biotechnological production, eds, Y. Doi, and A. Steinbüchel (Wiley-VCH Pub: Weinheim), 249–261.
Lv, J. M., Cheng, L. H., Xu, X. H., Zhang, L., and Chen, H. L. (2010). Enhanced lipid production of Chlorella vulgaris by adjustment of cultivation conditions. Bioresou. Tech. 101, 6797–6804. doi: 10.1016/j.biortech.2010.03.120
Magneschi, L., and Perata, P. (2009). Rice germination and seedling growth in the absence of oxygen. Ann. Bot. 103, 181–196. doi: 10.1093/aob/mcn121
Miao, X. L., and Wu, Q. (2006). Biodiesel production from heterotrophic microalgal oil. Bioresour. Tech. 97, 841–846. doi: 10.1016/j.biortech.2005.04.008
Mock, T., and Kroon, B. M. A. (2002). Photosynthetic energy conversion under extreme conditions- 1: important role of lipids as structural modulators and energy sink under N-limited growth in Antarctic sea ice diatoms. Phytochemistry 61, 41–51. doi: 10.1016/S0031-9422(02)00216-9
Ning, S. B., Guo, H. L., Wang, L., and Song, Y. C. (2002). Salt stress induces programmed cell death in prokaryotic organism Anabaena. J. Appl. Microbiol. 93, 15–28. doi: 10.1046/j.1365-2672.2002.01651.x
Rao, A. R., Dayananda, C., Sarada, R., Shamala, T. R., and Ravishankar, G. A. (2007). Effect of salinity on growth of green alga Botryococcus braunii and its constituents. Bioresou. Tech. 98, 560–564. doi: 10.1016/j.biortech.2006.02.007
Ratledge, C., and Cohen, Z. (2008). Microbial and algal oils: do they have a future for biodiesel or as commodity oils? Lipid Tech. 20, 155–160. doi: 10.1002/lite.200800044
Ratledge, C., and Wynn, J. P. (2002). The biochemistry and molecular biology of lipid accumulation in oleaginous microorganisms. Adv. Appl. Microbiol. 51, 1–51. doi: 10.1016/S0065-2164(02)51000-5
Richardson, B., Orcutt, D. M., Schwertner, H. A., Martinez, C. L., and Wickline, H. E. (1969). Effects of nitrogen limitation on the growth and composition of unicellular algae in continuous culture. Appl. Microbiol. 18, 245–250.
Rodolfi, L., Chini Zittelli, G., Bassi, N., Padovani, G., Biondi, N., Bonini, G., et al. (2009). Microalgae for oil: strain selection, induction of lipid synthesis and outdoor mass cultivation in a low-cost photobioreactor. Biotech. Bioeng. 102, 100–112. doi: 10.1002/bit.22033
Salama, E. S., Kim, H. C., Abou-Shanab, R. A. I., Ji, M. K., Oh, Y. K., Kim, S. H., et al. (2013). Biomass, lipid content, and fatty acid composition of freshwater Chlamydomonas mexicana and Scenedesmus obliquus grown under salt stress. Bioproc. Biosyst. Eng. 36, 827–833. doi: 10.1007/s00449-013-0919-1
Sharma, K. K., Schuhmann, H., and Schenk, P. M. (2012). High lipid induction in microalgae for biodiesel production. Energies 5, 1532–1553. doi: 10.3390/en5051532
Singh, D. K., and Mallick, N. (2014). Accumulation potential of lipids and analysis of fatty acid profile of few microalgal species for biodiesel feedstock. J. Microbiol. Biotech. Res. 4, 37–44.
Tchernov, D., Gorbunov, M. Y., De Vargas, C., Yadev, S. N., Milligan, A. J., Häggblom, M., et al. (2004). Membrane lipids of symbiotic algae are diagnostic of sensitivity to thermal bleaching in corals. Proc. Natl. Acad. Sci. U.S.A. 101, 13531–13535. doi: 10.1073/pnas.0402907101
Tobias, R. B., Boyer, C. D., and Shannon, J. C. (1992). Alterations in carbohydrate intermediates in the endosperm of starch deficient maize (Zea mays L) genotypes. Plant Physiol. 99, 146–152. doi: 10.1104/pp.99.1.146
Tornabene, T. G., Holzer, G., Lien, S., and Burris, N. (1983). Lipid composition of the nitrogen starved green alga Neochloris oleoabundans. Enzy. Microb. Tech. 5, 435–440. doi: 10.1016/0141-0229(83)90026-1
Velichkova, K., Sirakov, I., and Georgiev, G. (2013). Cultivation of Scenedesmus dimorphus strain for biofuel production. Agri. Sci. Tech. 5, 181–185. doi: 10.1080/09593330.2014.966769
Yang, Z. K., Niu, Y. F., Ma, Y. H., Xue, J., Zhang, M. H., Yang, W. D., et al. (2013). Molecular and cellular mechanisms of neutral lipid accumulation in diatom following nitrogen deprivation. Biotech. Biofuels 6:67. doi: 10.1186/1754-6834-6-67
Keywords: biofuel, fatty acid methyl esters (FAMEs), municipal untreated wastewater, nutrient deprivation, salt stress, Scenedesmus dimorphus, Selenastrum minutum
Citation: Pushpakumari Kudahettige N, Pickova J and Gentili FG (2018) Stressing Algae for Biofuel Production: Biomass and Biochemical Composition of Scenedesmus dimorphus and Selenastrum minutum Grown in Municipal Untreated Wastewater. Front. Energy Res. 6:132. doi: 10.3389/fenrg.2018.00132
Received: 08 June 2018; Accepted: 19 November 2018;
Published: 03 December 2018.
Edited by:
Ao Xia, Chongqing University, ChinaReviewed by:
Thiyagarajan Sekar, Madurai Kamaraj University, IndiaPau Loke Show, University of Nottingham, United Kingdom
Copyright © 2018 Pushpakumari Kudahettige, Pickova and Gentili. This is an open-access article distributed under the terms of the Creative Commons Attribution License (CC BY). The use, distribution or reproduction in other forums is permitted, provided the original author(s) and the copyright owner(s) are credited and that the original publication in this journal is cited, in accordance with accepted academic practice. No use, distribution or reproduction is permitted which does not comply with these terms.
*Correspondence: Francesco G. Gentili, ZnJhbmNlc2NvLmdlbnRpbGlAc2x1LnNl