- 1Central Department of Biotechnology, Tribhuvan University, Kirtipur, Nepal
- 2Central Department of Chemistry, Tribhuvan University, Kirtipur, Nepal
- 3Department of Chemistry and Earth Sciences, Qatar University, Doha, Qatar
Bioethanol (a renewable resource), blended with gasoline, is used as liquid transportation fuel worldwide and produced from either starch or lignocellulose. Local production and use of bioethanol supports local economies, decreases country's carbon footprint and promotes self-sufficiency. The latter is especially important for bio-resource-rich land-locked countries like Nepal that are seeking alternative transportation fuels and technologies to produce them. In that regard, in the present study, we have used two highly efficient ethanol producing yeast strains, viz., Saccharomyces cerevisiae (CDBT2) and Wickerhamomyces anomalous (CDBT7), in an electrochemical cell to enhance ethanol production. Ethanol production by CDBT2 (anodic chamber) and CDBT7 (cathodic chamber) control cultures, using 5% glucose as substrate, were 12.6 ± 0.42 and 10.1 ± 0.17 mg·mL−1 respectively. These cultures in the electrochemical cell, when externally supplied with 4V, the ethanol production was enhanced by 19.8 ± 0.50% and 23.7 ± 0.51%, respectively, as compared to the control cultures. On the other hand, co-culturing of those two yeast strains in both electrode compartments resulted only 3.96 ± 0.83% enhancement in ethanol production. Immobilization of CDBT7 in the graphite cathode resulted in lower enhancement of ethanol production (5.30 ± 0.82%), less than free cell culture of CDBT7. CDBT2 and CDBT7 when cultured in platinum nano particle coated platinum anode and neutral red-coated graphite cathode, respectively, ethanol production was substantially enhanced (52.8 ± 0.44%). The above experiments when repeated using lignocellulosic biomass hydrolysate (reducing sugar content was 3.3%) as substrate, resulted in even better enhancement in ethanol production (61.5 ± 0.12%) as compared to glucose. The results concluded that CDBT2 and CDBT7 yeast strains produced ethanol efficiently from both glucose and lignocellulosic biomass hydrolysate. Ethanol production was enhanced in the presence of low levels of externally applied voltage. Ethanol production was further enhanced with the better electron transport provision i.e., when neutral red was deposited on cathode and fine platinum nanoparticles were coated on the platinum anode.
Introduction
Dependence on petroleum-based transportation fuels is a major challenge for developing countries which don't have fossil fuel reserves. The challenge is severe in the landlocked countries such as Nepal (Joshi et al., 2011). In fact, landlocked countries spend a major share of their GDP to import fossil fuels via other countries. Given the above, serious attempts are being made to develop alternative energy sources that are expected to alleviate the above challenge. Bioethanol is one of those renewable and eco-friendly fuels (Khatiwada and Silveira, 2011). Besides being used as a fuel, bioethanol can also be used for other purposes such as making bioplastics (Rose and Palkovits, 2011) and development of ethanol fuel cells for electricity generation (Saisirirat and Joommanee, 2017).
Second generation biofuels such as bioethanol (obtained from lignocellulose) can be produced in rural as well as urban areas using easily available lignocellulosic biomass. Lignocellulose is the most abundant biomass worldwide with annual production of about 1,000 giga-metric tons. The biomass is a mixture of cellulose, hemicellulose, lignin and other contents such as pectin. Cellulose and hemicelluloses upon degradation produces fermentable sugars, e.g., glucose and xylose. These sugars can be further converted into important products, including ethanol, by fermentation. The extent of ethanol that can be produced by lignocellulosic biomass depends on composition of sugars, which varies in plants and the habitat in which they grow (Hermosilla et al., 2017; Joshi et al., 2018). To date the cost of ethanol production from lignocellulosic biomass depends on technique used. The better the technique, the lower is the cost of production (Haque and Epplin, 2012).
Yeasts such as S. cerevisiae are widely used for ethanol fermentation. Yeasts with high salt and ethanol tolerance are most valuable in this process. Further to produce ethanol efficiently from lignocellulosic hydrolysates, yeasts should be able to utilize both glucose as well as pentoses such as xylose and arabinose. This is because lignocellulosic biomass is rich in both glucose and xylose (a pentose) (Joshi et al., 2018). This is the main drawback of using S. cerevisiae alone as it cannot ferment both glucose and xylose. Accordingly, identifying yeast strains that can ferment both glucose and xylose is critical, if not, at the least use two different strains of yeast that are capable of utilizing these sugars independently. In this study, we have identified and used highly efficient, salt and ethanol tolerant, yeast strains, viz., S. cerevisiae (CDBT2) (Joshi et al., 2014) and xylose utilizing W. anomalous (CDBT7) for ethanol production using lignocellulosic biomass.
For enhancing ethanol production, electro-fermentation technology that merges traditional fermentation with electrochemistry can be adopted. Electro-fermentation is a novel process in which the microbial fermentative metabolism may be controlled electrochemically. The benefits of this process are that for the process (i) is selective, (ii) increases sugar (carbon) utilization efficiency, (iii) minimizes the use of additives for redox balance or pH control, (iv) enhance cell growth and (v) in some cases enhance product recovery (Schievano et al., 2016). The electrodes used in the electrochemical cell can act as electron sinks, as source of electrons or polarize ions present in microbes that allow an unbalanced growth. Such electrochemical modifications also exert significant effects on not only metabolism and cellular regulation, but also on interspecies interactions as well as the selection of microbial populations (Moscoviz et al., 2016). The novel yeast strains of S. cerevisiae (CDBT2) and W. anomalous (CDBT7), identified in our laboratory, are used in this study to determine better utilization of glucose and xylose from lignocellulosic biomass and enhancement in ethanol production in an electrochemical cell. The lignocellulosic biomass used in the study was obtained from Saccharum spontaneum pretreated with hot water at 100°C for 2 h followed by 0.5 M hydrochloric acid hydrolysis (Joshi et al., 2018).
Materials and Methods
Materials
Woven graphite felt (10 mm thickness) was purchased from Nippon Co., Nippon, Japan. Platinum wire (0.2 mm diameter), high grade neutral red, ethanol, and thionyl chloride were purchased from Sigma Chemical Co., St. Louis, MO, USA. Electrochemical cell (ECC) vessels made up of Pyrex glass were purchased from Adams and Chittenden Scientific Glass Co., California, USA. Nafion 117 membrane was purchased from DuPont Co., Wilmington, DE, USA. Hydrogen hexachloroplatinate (IV) hexahydrate (Kanto Chemicals Company, Japan) was kindly provided by Prof. Dr. Amar Prasad Yadav, Central Department of Chemistry, Tribhuvan University, Nepal. D-Glucose, dinitro salicylic acid (DNSA), sodium potassium tartrate, yeast extract, peptone, ammonium sulfate and sodium alginate were purchased from Hi-Media Company, Bangalore, India. All other chemical were of analytical grade and were available locally.
Biomass samples of S. spontaneum was harvested from the premises of Tribhuvan University Campus, Kirtipur, Kathmandu, Nepal during the month of August. The sampling location was 27.6818°N and 85.2865°S. The aerial portion of the collected sample was air dried for 24 h and cut into about 2 cm pieces. It was further dried in oven at 60°C for 24 h. The dry samples were ground using a blender. The blended biomass was sieved using 250–500 μm sieves and packed into plastic pouches for further use in fermentation studies.
Methods
Ethanol Production in Electrochemical Cell
Development of CDBT2 and CDBT7 inoculums
Preserved yeast strains CDBT2 (Gene Bank accession # MK910215) and CDBT7 (Gene Bank accession # MK910216) were used to develop inoculums. Inoculums were prepared by inoculating a loop-full of agar cultures of CDBT2 and CDBT7 strains into PYN (Peptone, Yeast extract and Nutrient) media (Peptone: 3.5 gm·L−1, yeast extract: 3 gm·L−1, KH2PO4: 2 gm·L−1, MgSO4:1 gm·L−1, and (NH4)2SO4: 1 gm·L−1) (Balakumar and Arasaratnam, 2012) supplemented with 5% glucose. They were cultured in orbital shaker for 18 h at 30°C and pH 5.0.
Construction of an electrochemical cell (ECC)
The anodic and cathodic compartment of ECC was assembled tightly using a rubber gasket inserted with nafion membrane that separates the two chambers (Figure 1). Working volume of the cathodic and anodic compartments were ~300 mL each. Normal graphite felt (11 cm × 3 cm × 1 cm) was used as cathode and platinum wire (0.2 mm diameter, 1 m length) was used as anode. Each time 1.2 mL of respective inoculum, prepared above, was added with 300 mL PYN media in each case.
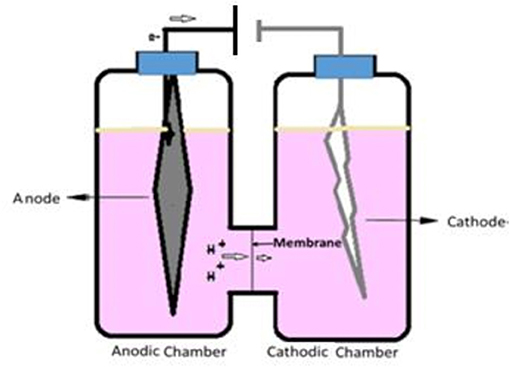
Figure 1. The electrochemical cell (ECC): The ECC was assembled using the Pyrex glass fuel cell assembly as shown in the figure, separated by a nafion membrane using a leak-proof rubber gasket. The electrodes were inserted through small openings in each of the chamber caps and then connected to a power pack to supply external voltage.
Optimization of ethanol production in an ECC
The yeast strains CDBT2 and CDBT7 were cultured in ECC (Figure 1) and evaluated for ethanol production in the presence of carbohydrate sources, i.e., glucose (5%) and lignocellulosic biomass hydrolysate (with 3.3% reducing sugar). Lignocellulosic biomass hydrolysate was prepared by collecting the hydrolysate that was formed after pretreating Saccharum spontaneum biomass (250–500 μm size) with hot water at 100°C for 2 h followed by hydrolyzing with 0.5M HCl for 24 h. The 5% glucose or biomass hydrolysate acted as carbohydrate sources (Joshi et al., 2018). PYN media composition was added as supplements. The fermentation media in ECC without culture and the external source of voltage served as control. The two compartments of the ECC were filled with PYN media supplemented with glucose or lignocellulosic biomass hydrolysate, inoculated with yeast strains and allowed to produce ethanol in the presence and absence of applied voltage at an optimized pH 5.5 and 30°C (Joshi et al., 2014).
Ethanol production by yeast strains CDBT2 and CDBT7 under applied voltage
In the first fermentation reaction, the yeast strains CDBT2 and CDBT7 were cultured in cathodic and anodic chambers, respectively. In a second fermentation reaction, the yeast strains CDBT2 and CDBT7 were cultured in anodic and cathodic chambers, respectively. In each of the above reactions, the ECC was supplied with 4V constantly. Ethanol production by yeast strains CDBT2 and CDBT7 in ECC was monitored at intervals of 12 h as described by Seo et al. (2009). The sample broth (1 mL) collected from ECC was initially centrifuged at 4000 × g for 15 min to remove cell debris. The supernatant was then mixed with 1 mL tri n-butyl phosphate (TBP), vortexed for 15 min and centrifuged at 4000 × g for 15 min to separate layers. About 750 μL of upper TBP layer was transferred to another tube and mixed with equal amount of acidified 5% potassium dichromate reagent. The mixture was vortexed, centrifuged as above and lower green layer (potassium dichromate reagent layer) was separated and its absorbance was measured at 595 nm using spectrophotometer against blank. Cell growth was monitored by measuring turbidity of culture broth at 600 nm. The reduction in reducing sugar concentrations in the broth samples were measured by DNSA method (Miller, 1959).
Formation of film in graphite electrode by yeast
Biofilm formation was analyzed by Scanning Electron Microscopy (SEM). After completion of experiment in ECC, graphite electrodes kept as cathode with cultures of CDBT2 and CDBT7 were vacuum evaporated and packed in sterile plastic pouch. The samples were sent to Advanced Instrumental Lab, Jawaharlal Nehru University, New Delhi, India for SEM. The biofilm formation was confirmed from the micrograph.
Ethanol production by CDBT2 and CDBT7 at different voltage
To determine optimum supply of external voltage for ethanol production, the ECC voltage was varied between 0 and 5V (± 0.1 V), at an interval of 1V and ethanol production was measured as above.
Comparison of ethanol production by CDBT7 vs. several strains of S. cerevisiae
To compare the ethanol production efficiency of CDBT2 with other S. cerevisiae, ethanol production in the anodic compartment of ECC was monitored by culturing S. cerevisiae strains obtained from different sources. Yeast strains MKY09 (a laboratory yeast strain) and Ethanol Red (an industrial yeast strain) were kindly provided by Prof. Eckhard Bole, University of Frankfurt, Germany. Yeast strain MKY09 transformed with pGPD2 plasmid inserted with laccase gene (pGPD2/lac) was developed at Central Department of Biotechnology, Tribhuvan University, Nepal (Bishwakarma, 2017). The plasmid pGPD2/lac was constructed by cloning synthetic laccase gene of Ganoderma lucidum of size 1,576 bp in pGPD2 expression vector purchased from Addgene Company. The constructed plasmid was transformed into MKY09 strain. Yeast strain CDBT7 was cultured in cathodic compartment in all cases. The two chambers were separated by nafion membrane. The ethanol production was measured as described above.
Effect of different electron transport enhancing system in ECC
Production of ethanol was monitored in cathodic and anodic compartments by culturing yeast strains CDBT2 and CDBT7 in various combinations as follows. In reaction 1, yeast strain CDBT2 was cultured in both the compartments. In reaction 2, yeast strains CDBT2 and CDBT7 were cultured in anodic and cathodic compartments, respectively. In reaction 3, yeast strains CDBT2 and CDBT7 were co-cultured in both cathodic and anodic compartments. In all reactions, ethanol production was monitored as above. In the next set of reactions, the graphite felt (cathode) and platinum wire (anode) were coated with different electron transport enhancers and used as cathode and anode in ECC. In first case, the graphite electrode was immobilized with yeast cells using calcium alginate gel and used as cathode. Briefly, about 2.4 mL of 18 h culture of yeast strains were first centrifuged, the pellet mixed with 10 mL of 25 mM phosphate buffer (pH 7.0) containing 4% sodium alginate, and allowed to absorb into the graphite electrode for 30 min. The graphite electrode containing alginate and yeast cells was then soaked in a chilled 100 mM CaCl2 solution for 30 min to induce calcium alginate coagulation and finally washed with 25 mM phosphate buffer. In the second case, the platinum electrode was coated with platinum nanoparticle electrochemically in anode. For this platinum wire was dipped in 10% hydrogen hexachloroplatinate (IV) hexahydrate solution in distilled water (Kanto Chemical Co.) with constant supply of 0.2 V using Hokuto Denko-151 potentiostat (Hokuto Denko Corporation, Japan) for 30 min. In the third case, the cathode was coated with neutral red as described by Jeon and Park (2010). Briefly, the graphite felt was first soaked in methanol, then dipped in 1% polyvinyl alcohol solution for 3 to 4 h and was dried in an oven at about 80°C for 24 h. The completely dried graphite felt was then soaked in pure chloroform containing 10% thionylchloride and 0.01% neutral red for 6 h. The graphite felt was then left for 12 h to air dry. It was then autoclaved and washed in running distilled water till color persists. Finally, it was dried at 60°C for 24 h and used as cathode. Best combination of electron transport system amongst was determined on the basis of higher ethanol production.
Ethanol Production by Lignocellulosic Biomass Hydrolysate as Carbohydrate Source
Ethanol production from hydrolysate
Lignocellulose biomass hydrolysate supplemented with PYN media was used for further fermentation to produce ethanol. The hydrolysates were kept in ECC chambers. After autoclaving, the chambers were inoculated with CDBT2 and CDBT7 yeast strains in anodic and cathodic chambers, respectively. The two chambers were separated by a nafion membrane. Ethanol production was measured as described above.
Comparison of ethanol production by nafion and cellulose acetate membrane
The experiments above were repeated in an ECC fitted with a cellulose acetate membrane to separate the two chambers. Ethanol production was measured as above and compared to those determined when the separating membrane was nafion membrane.
Statistical Analysis
All the data presented were the average of three redundant data. All graphs and statistical calculations were performed with Graph Pad Prism 8.0.1. Standard errors were represented in terms of ± standard deviation (±SD).
Results
Optimization of Ethanol Production in an Electrochemical Cell (ECC)
The standard conditions under which fermentation was performed in the ECC (schematic of ECC assembly shown in Figure 1) using various yeast strains were pH 5.5, 30°C, PYN broth supplemented with 5% glucose and an external supply of 4V. The fermentation system in which CDBT2 strain was cultured in anodic compartment and CDBT7 strain in cathodic compartment was found to be most efficient for ethanol production than culturing CDBT2 strain in cathodic compartment and CDBT7 strain in anodic compartment (Figures 2A,B, Table 1). Scanning electron microscopic imaging showed the formation of a film of CDBT7 in graphite felt electrode (Figure 3). The fermentation efficiency was enhanced in both cathodic and anodic chamber cultures as compared to fermentation reactions carried out in the absence of applied voltage. There was observed faster and enhanced growth of CDBT2 in anodic compartment (Figure 2C). On the other hand, growth of CDBT2 strain when cultured in cathodic compartment was relatively limited. The CDBT7 strain when cultured in anodic compartment, showed relatively lower enhancement in ethanol production (Table 1). On application of external voltage in the range of 0 to 5V (± 0.1V), while CDBT2 was cultured in the anodic compartment and CDBT7 cultured in cathodic compartment, application of 4V produced the highest amount of ethanol (Figure 2D). The enhancement of ethanol production observed was 19.4 ± 0.18% when CDBT2 was cultured in anodic compartment and 23.7 ± 0.51% when CDBT7 was cultured in cathodic compartment. Supply of external voltage through ECC in the absence of yeast inoculums (negative control) did not produce detectable levels of ethanol.
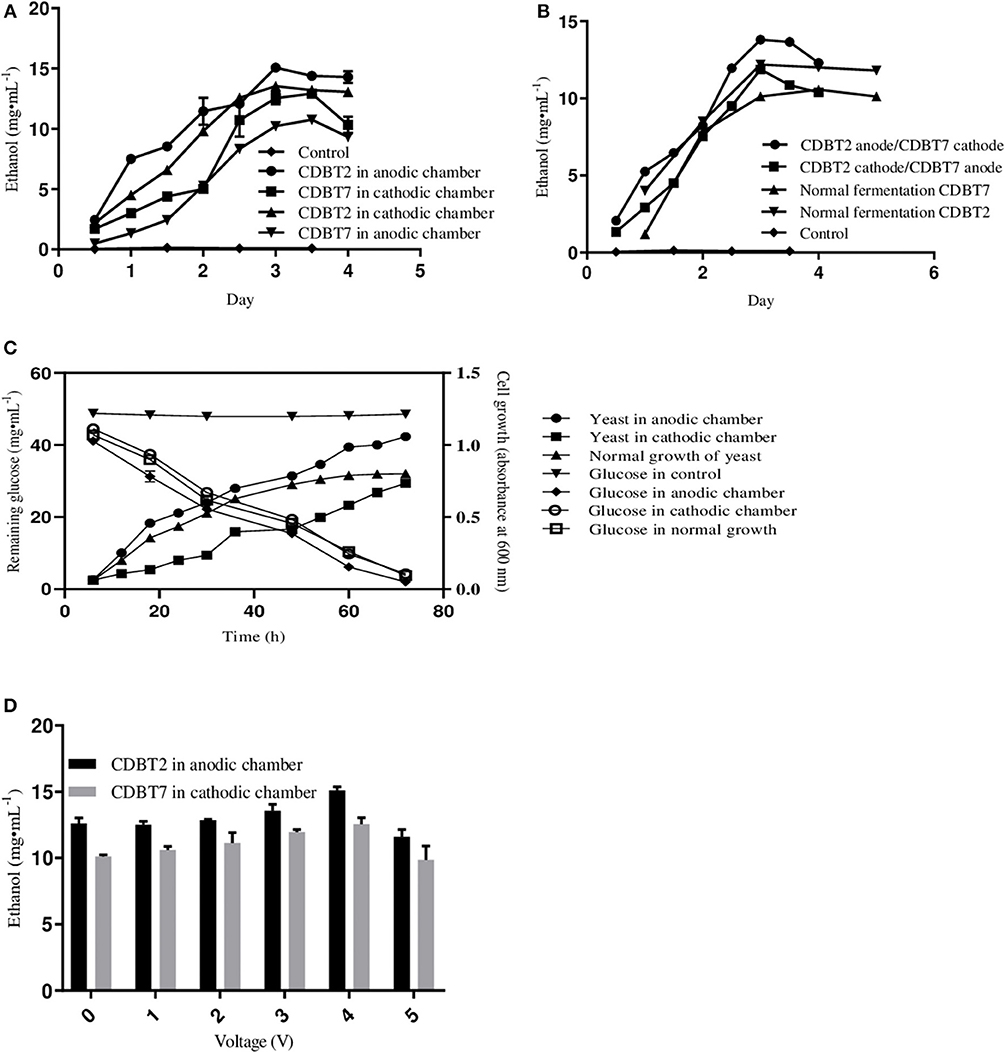
Figure 2. Ethanol production in ECC by yeast strains CDBT2/CDBT7 with the supply of 4V externally. (A) Ethanol production in cathodic and anodic chambers by CDBT2 and CDBT7 individually. (B) Average of ethanol production in both chambers. (C) Cell growth and glucose utilization when CDBT2 cultured in anodic and cathodic chambers with and without applied external voltage (normal growth—without the supply of external voltage). (D) Effect of applied electrical current on ethanol production by CDBT2 and CDBT7. (Control: ECC w/o/yeast culture and w/o voltage supply; normal fermentation: ECC w/o voltage supply).
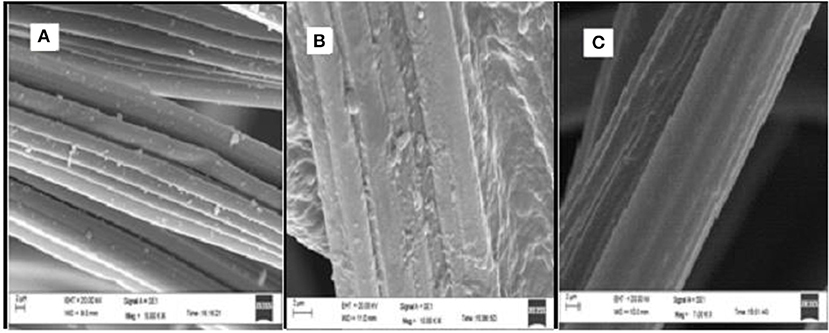
Figure 3. Scanning electron microscopic images of graphite felt electrodes. (A) Plane graphite felt. (B) Graphite felt cathode post CDBT7 growth and ethanol production in the cathodic chamber. (C) Graphite felt cathode post CDBT2 growth and ethanol production in the cathodic chamber.
Comparison of Ethanol Production by Various S. cerevisiae Strains Cultured in Anodic Compartment Coupled With CDBT 7 Strain Cultured in Cathodic Compartment
To determine the efficiency of ethanol production using different S. cerevisiae strains in ECC, ethanol production was monitored by culturing CDBT2, ethanol red, MKY09, and MKY09-pGPD2/lac in anodic compartment against CDBT7 in cathodic compartment. Once again, CDBT2 produced the highest amount of ethanol (Figure 4A) as compared to other S. cerevisiae strains. Further, genetic modification of MKY09 strain by transforming it with a laccase gene (MKY09-pGPD2/lac) did not affect ethanol production in ECC, rather both MKY09 and MKY09-pGPD2/lac strains produced similar amounts of ethanol. In addition, yeast strain CDBT2 cultured in anodic chamber, coupled to CDBT7 strain cultured in cathodic chamber showed enhancement of the ethanol production by CDBT7 (Figure 4B).
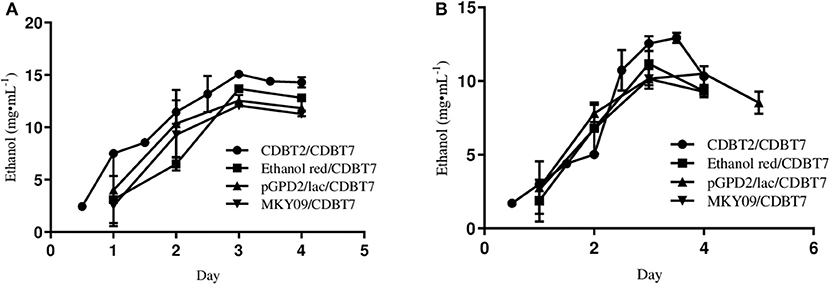
Figure 4. Ethanol production by various S. cerevisiae strains cultured in anodic compartment of electrochemical cell with CDBT7 cultured in cathodic compartment: a comparative study. (A) Ethanol production in anodic compartment. (B) Ethanol production in cathodic compartment.
Effect of Different Electron Transport Systems
Effect of Yeast Cultures
To determine the effect of yeast cultures on electron transport systems, yeast strains CDBT2 and CDBT7 were cultured in ECC in the following combinations; (i) CDBT2/CDBT2 in anodic/cathodic compartments; (ii) CDBT2/CDBT7 in anodic and cathodic compartments and (iii) co-culture of CDBT2 and CDBT7 in both compartments. In combination of CDBT2/CDBT2, it was found that CDBT2 yeast strain produced more ethanol in the anodic compartment (15.5 ± 0.14 mg·mL−1) as compared to cathodic compartment (13.4 ± 0.07 mg·mL−1). The average ethanol production in this combination was 14.4 ± 0.15 mg·mL−1 (Table 2) and the increase in ethanol production was 27.5 ± 0.44%. In combination of CDBT2/CDBT7, CDBT2 strain again produced more ethanol in anodic chamber (15.10 ± 0.28 mg·mL−1) as compared to CDBT7 strain in cathodic compartment (12.5 ± 0.50 mg·mL−1). The average ethanol production in this combination was 13.8 ± 0.56 mg·mL−1 (Figure 5A, Table 2) and the increase in ethanol production was 21.5 ± 0.71%. When CDBT2 and CDBT7 strains were co-cultured in both compartments, ethanol production was significantly lower as compared to individual cultures.
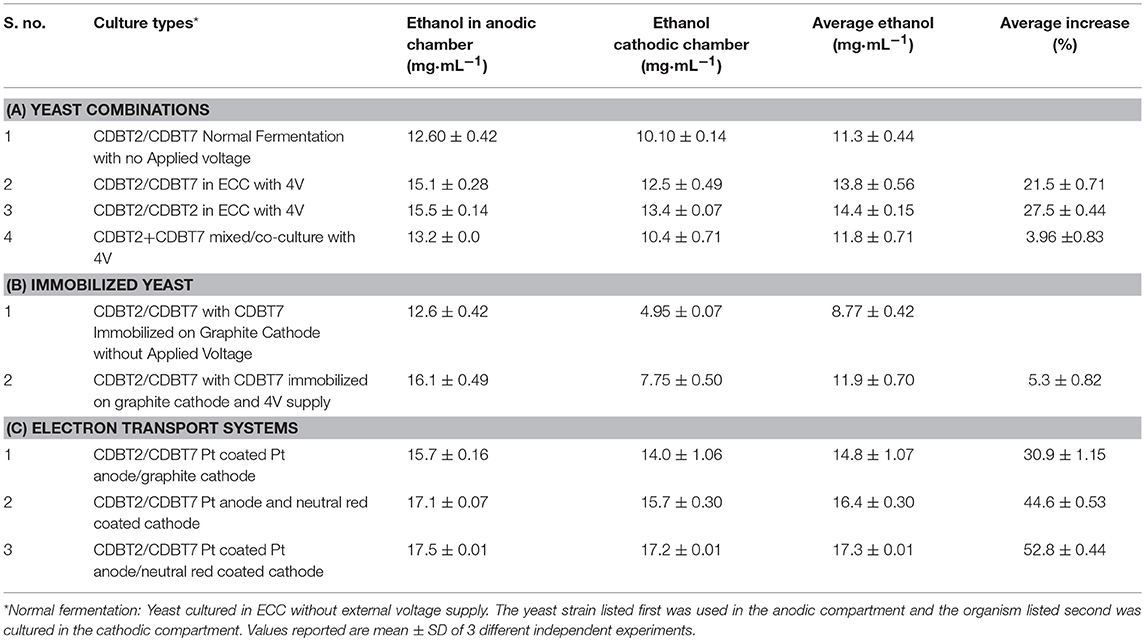
Table 2. Ethanol production in an ECC with various combinations of anodic and cathodic systems for electron transport enhancement: summary.
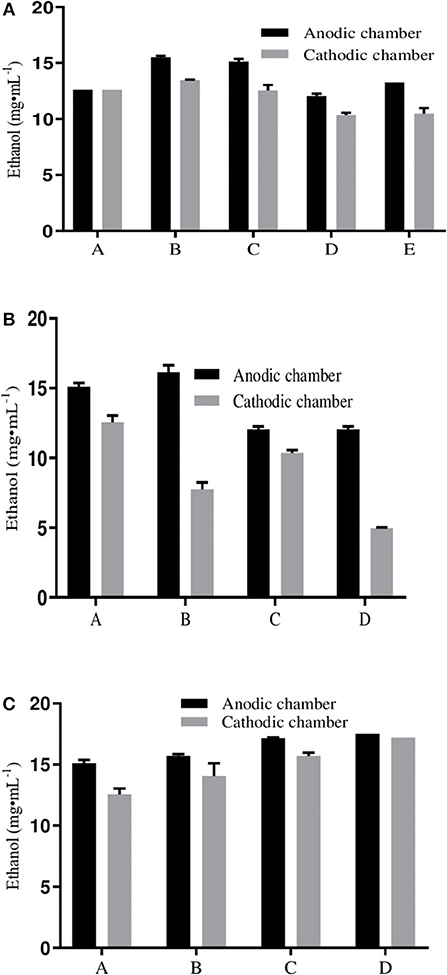
Figure 5. Ethanol production with different electron transport enhancement systems. (A) Use of yeasts in various combinations in ECC (A: CDBT2 normal fermentation; B: CDBT2/CDBT2 in Electrochemical cell; C: CDBT2/CDBT7 in Electrochemical cell, D: CDBT2/CDBT7 normal fermentation, E: CDBT2/CDBT7 mixed culture). (B) Use of graphite electrode immobilized with CDBT7 yeast strain [A: Graphite cathode/Pt anode; B: Graphite cathode (immobilized with CDBT7)/Pt anode; C: Normal fermentation CDBT2/CDBT7; D: Normal fermentation CDBT2/CDBT7 immobilized in graphite]. (C) Use of electrodes coated with different electron transporters (A: Normal Platinum anode/graphite cathode; B: Platinum coated Platinum anode/graphite cathode; C: Neutral red coated graphite cathode/Platinum anode; D: Neutral red coated cathode/Pt coated anode).
Effect of Immobilization of Yeast
Yeast strain CDBT7 immobilized in presence of calcium alginate on cathode, when used for ethanol production in an ECC with CDBT2 strain in anodic compartment, produced 12.6 ± 0.42 mg·mL−1 and 4.95 ± 0.07 mg·mL−1 ethanol by CDBT2 and CDBT7 strain respectively without the applied voltage i.e., there was a significant decrease in ethanol production (Figure 5B). The same system when used in ECC with the application external voltage input (4V), the ethanol production was significantly enhanced. The ethanol production in the latter case were 16.1 ± 0.49 mg·mL−1 (CDBT2 in anodic compartment) and 7.75 ± 0.5 mg·mL−1 (CDBT7 strain in the cathodic compartment), respectively. The average ethanol production was 11.5 ± 0.70 mg·mL−1 and the increase in ethanol production was 36.2 ± 0.54% than without voltage supply. However, the average ethanol production in ECC was less than without immobilization of CDBT7 in cathode. In summary, immobilization did not show the better enhancement in ethanol production.
Effect of Electron Transport Enhancing Materials
Coating of platinum electrode with fine particles (nanoparticles) of platinum using hexachloroplatinate (IV) and graphite cathode with neutral red, and culturing CDBT2 strain in anodic compartment and CDBT7 strain in cathodic compartment produced 17.5 ± 0.01 mg·mL−1 and 17.2 ± 0.01 mg·mL−1 ethanol, respectively (Figure 5C). A significant increase in ethanol production (52.8 ± 0.44%) was observed than normal fermentation without voltage supply (Table 2).
Electrochemical Ethanol Production From Saccharum spontaneum Hydrolysate
When lignocellulosic (Saccharum spontaneum) hydrolysate with 3.3% reducing sugar was used as substrate for fermentation in ECC, there was significant enhancement in ethanol production in anodic (9.0 ± 0.1 mg·mL−1; increase = 60.8 ± 0.10%) as well as in cathodic (11.9 ± 0.05 mg·mL−1; increase = 63.0 ± 0.07%) compartments, with an average enhancement of 61.9 ± 0.12% than normal fermentation without voltage supply. Ethanol production by CDBT2 and CDBT7 without external voltage supply were 5.6 ± 0.03 and 7.3 ± 0.06 mg·mL−1, respectively (Figure 6, Table 3). The increase in amount of ethanol produced, when the fermentation substrate was lignocellulosic hydrolysate, was much higher when compared to fermentation with glucose as substrate under identical conditions. In addition, when the membrane barrier was changed to cellulose acetate in place of nafion membrane, there was a further increase (6.30 ± 0.22%) in ethanol production (Figure 7, Table 3).
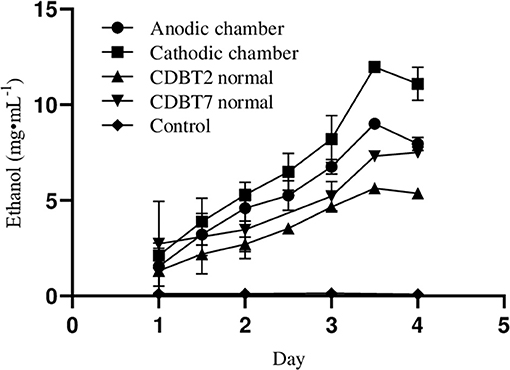
Figure 6. Ethanol production from lignocellulosic hydrolysate (S. spontaneum pretreated with hot water for 2 h followed by 0.5M acid hydrolysis) in ECC with CDBT2 and CDBT7 strains cultured in anodic and cathodic compartments, respectively (Control: ECC without yeast culture and without external voltage supply. Normal: ECC with yeast culture and without external voltage supply).
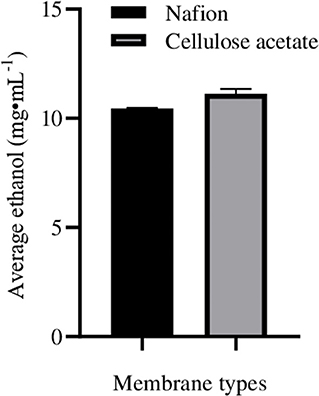
Figure 7. Ethanol production from lignocellulosic hydrolysate (S. spontaneum pretreated hot water for 2 h followed by 0.5M acid hydrolysis) in ECC with CDBT2 and CDBT7 in anodic and cathodic compartments separated by nafion membrane or cellulose acetate membrane. Ethanol measurements in the cultures were determined after 3.5 days of fermentation.
Discussion
S. cerevisiae CDBT2 and W. anomalus CDBT7 yeast strains were selected for the study because both are good ethanol producers and the later has been shown to be capable of converting xylose into ethanol. The pH optima for ethanol production using yeast strains CDBT2 and CDBT7 were pH 5.5 and temperature 30°C, respectively. The conditions were consistent with our previous experiments with optimization of yeast strains CDBT2 (Joshi et al., 2014). The ECC system with CDBT2 in anodic compartment and CDBT7 in cathodic compartment, produced the highest amount of ethanol as compared to reverse system tested. This may be because CDBT7 strain forms film on graphite cathode resulting in fast electron transport through cathode. This was further supported by the fact that (i) SEM images of cathode surface clearly show CDBT7 biofilm formation (Figure 3), and (ii) use of CDBT7 immobilized cathode resulted in significant fold increase in ethanol production when cultured in ECC (Table 2) (Toit and Pretorius, 2000). Further, the study by Mohamoud (2014) suggests that supply of external voltage enhances film redox potential and thus results in enhancing more electron transport and more ethanol production. According to that study there was 40% enhancement in film redox potential when electricity was passed to electrode with polyaniline/polyvinyl composite. Canelas et al. (2008) observed that ethanol formation requires the maintenance of NAD+/NADH ratios. In healthy eukaryotes, NAD+/NADH ratios usually are relatively high and the range varies widely (60–700). Canelas et al. (2008) also observed that the cytosolic free NAD+/NADH ratio in S. cerevisiae under steady and highly dynamic state ranges between 101 ± 14 and 320 ± 45 where as whole cell NAD+/NADH ratio was 7.5 ± 2.5. Further it was observed that in S. cerevisiae NAD+/NADH ratio was reduced when there is presence of electron donor and the ratio was increased in the presence of electron acceptor. In our case, when yeast strain was cultured in cathodic compartment, there was increment in ethanol production because the cathode was the electron donor, thus resulting more conversion of NAD+ to NADH which has directed the conversion of pyruvate to ethanol. Reversibly when yeast strain cultured in anodic compartment which is electron acceptor, converted NADH to NAD+ which had directed fast conversion of glucose to pyruvate so that we could see enhancement in growth and ethanol formation as well. In spite of the ratios reported above, eukaryotes could survive even when the NAD+/NADH ratio was as low as 7–10 (Veech et al., 1972). The supplied external voltage input polarizes ions present in cytosol and as a result positively charged NAD+ ion bound to cell membrane make the transfer of electrons from cathode easier and faster (Gunawardena et al., 2008; McGillivray and Gow, 2009) thus makes it easy to access for reduction of NAD+ to NADH in cathode resulting more ethanol production. According to Yau et al. (2013), the applied voltage was believed to polarize ionic charges in yeast cells, which may lower the tunnel barrier for transferring electrons during glucose oxidation resulting more pyruvate and hence more cell growth and ethanol production.
The pyruvate formed during glycolysis is converted to (i) acetyl CoA under aerobic conditions in eukaryotes, (ii) lactate during homolactic fermentation in mammals, or (iii) ethanol during anaerobic fermentation in yeast and bacteria. When an external voltage was supplied, NAD+ was directly converted to NADH, increasing the level of NADH. This is known to cause an imbalance in the growth of yeasts that favors production of ethanol by forcing the yeast to convert pyruvate to acetaldehyde and then to ethanol by consuming NADH. It has also been shown that increased NADH allosterically inhibits pyruvate dehydrogenase (Harris et al., 2002) and prevents conversion of pyruvate to acetyl coenzyme A. Accordingly, pyruvate can be diverted to ethanol formation. Song et al. (2014) have shown that external input of voltage can be used to control the kinetics of glucose metabolism in S. cerevisiae under both aerobic and anaerobic conditions. Here, intracellular electron carriers such as NAD+, NADH and the transplasma membrane electron transfer (tPMET) system located in the plasma membrane plays important role for direct transport of electrons through cell membrane. tPMET system consists of cytochromes and various redox enzymes such as NADH oxidase which provides redox activity to the membrane at specific sites.
In reality, for reduction of NAD+/NADH ratio, applied voltage requirement is around −0.33V (Veech et al., 1972). In our experiments, we can see that optimal ethanol production was obtained at 4V ± 0.1V. The higher voltage, we believe, was because of ohmic drop exerted by the designed ECC. Mathew et al. (2015) have reported the gradual increase in growth by 1.1 fold and ethanol production by 2 fold when S. cerevisiae was cultured with external voltage supply till 15V. In our study, increasing voltage beyond 4V reduces ethanol production. This may be because, increasing voltage might oxidize Platinum to Platinum dioxide which acted as insulator and decreased the activity of electrode (Wang et al., 2006). Similarly in cathode, there might be overproduction of hydrogen gas which might decrease the activity of graphite electrode (Hsu et al., 2008). Further, when mixed culture of yeast strains CDBT2 and CDBT7 were used, ethanol production was relatively low. This may be because when S. cerevisiae and W. anomalous were cultured together, growth of W. anomalous may have suppressed the growth of S. cerevisiae thus decreased the overall ethanol production (Ruyters et al., 2015).
Steinbusch et al. (2010) have reported an increase in more than 2 fold enhancement of ethanol production in the presence of an external source of electrical current when acetate was used as substrate for fermentation. He et al. (2016), have reported 60.3% enhancement in butanol production by Clostridium spp. when neutral red was used as electron transporter. He et al. have also demonstrated that neutral red increased the butanol production better than other electron transporters such as viologen dyes (dyes containing pyridine groups). Further neutral red can strongly bind to cell membranes (Park et al., 1999). It has a redox potential of about −0.325V which is similar to that of the redox potential of NADH (−0.32 V). Accordingly neutral red could interact with NADH and, thus, increase the level of NAD+/NADH giving rise to more ethanol. The decrease in ethanol production in immobilized culture was because immobilization reduces substrate diffusion and hence ethanol production was less than in normal growth whereas it enhances fast electron transport at the intersection of the electrode.
The increase in ethanol production due to applied external source of voltage was further more when acid hydrolyzed Saccharum spontaneum hydrolysate was used as substrate. This may be because; the NaCl present in the neutralized hydrolysate may help in enhancement of ethanol production by decreasing resistance. In fact, Yang et al. (2015) have shown that when the concentrations of NaCl was increased from 5 to 30 g·L−1 in the fermentation media, the internal resistances of the system decreases from 2432.0 to 2328.4 Ω. Similarly, Kamcev et al. (2018) also observed that increasing salt concentration increases electrical conductivity hence increases electron flow and reduces resistance. Accordingly, the increased conductivity is likely to promote ethanol production. The increased production of ethanol from lignocellulosic biomass could also be partly due to the presence of various natural products/substance present in the mixture that could promote growth of yeast strains. Alteration of cellulose acetate membrane in place of nafion membrane enhanced ethanol production. The latter could be due to transport of xylose across cellulose acetate membrane from anodic chamber to cathodic chamber, as well as decrease in internal resistance due to ion accumulation on the membrane surface (Tang et al., 2010).
Conclusion
In summary, a combination of yeast strains S. cerevisiae CDBT2 and W. anomalous CDBT7 effectively and efficiently produce ethanol from both glucose and lignocellulosic biomass hydrolysate. Use of CDBT2/CDBT7 strains in an ECC efficiently utilize reducing sugars as indicated by near complete utilization of reducing sugars. Ethanol production by CDBT2 and CDBT7 yeast strains can be enhanced by supplying low levels of external voltage. S. cerevisiae CDBT2 was most efficient at ethanol production in the anodic compartment, whereas W. anomalous CDBT7 yeast strain was most efficient in ethanol production in the cathodic compartment. Further enhancement of ethanol production was observed when ECC was operated with fine platinum nanoparticles coated on the platinum anode, and neutral red was deposited on graphite cathode. Hot water pretreated and mineral acid hydrolyzed lignocellulosic biomass (an economic method) can be used as substrate for fermentation in ECC with CDBT2 and CDBT7 strains. An additional advantage of using hydrolyzed lignocellulosic biomass was that it further enhances ethanol production in ECC. Given the data reported herein, yeast strain CDBT2 could serve as a potent industrial strain for genetic modification and ethanol production. One of the limitation of this study is to go for further scaled up. It is strongly believed that the scale up of this study is entirely feasible with the availability of large electrochemical fermentation cell and is next phase of our study.
Data Availability
All datasets generated for this study are included in the manuscript/supplementary files.
Author Contributions
JJ performed laboratory work and prepared manuscript draft. TB, LS, and AY edited manuscript. PD, BP, PP, MY, MM, and RM helped in laboratory works. All the authors revised the manuscript and approved the submitted version.
Conflict of Interest Statement
The authors declare that the research was conducted in the absence of any commercial or financial relationships that could be construed as a potential conflict of interest.
Acknowledgments
Mr. Sujan Bishwokarma, Central department of biotechnology, Tribhuvan University, Renewable Nepal funding program are highly acknowledged for their support.
References
Balakumar, S., and Arasaratnam, V. (2012). Osmo-, thermo- and ethanol- tolerances of. Braz. J. Microbiol. 43, 157–166. doi: 10.1590/S1517-838220120001000017
Bishwakarma, S. (2017). Heterologous expression of laccase gene of Ganoderma lucidum in Saccharomyces cerevisiae for degradation of lignocellulosic biomass (M. Sc. Thesis). Tribhuvan University, Registration No.:5-1-48-301-2007.
Canelas, A. B., Van Gulik, W. M., and Heijnen, J. J. (2008). Determination of the cytosolic free NAD/NADH ratio in Saccharomyces cerevisiae under steady-state and highly dynamic conditions. Biotechnol. Bioeng. 100, 734–743. doi: 10.1002/bit.21813
Gunawardena, A., Fernando, S., and To, F. (2008). Performance of a yeast-mediated biological fuel cell. Int. J. Mol. Sci. 9, 1893–1907. doi: 10.3390/ijms9101893
Haque, M., and Epplin, F. M. (2012). Cost to produce switchgrass and cost to produce ethanol from switchgrass for several levels of biorefinery investment cost and biomass to ethanol conversion rates. Biomass Bioenergy 46, 517–530. doi: 10.1016/j.biombioe.2012.07.008
Harris, R. A., Bowker-kinley, M. M., and Huang, B. (2002). Regulation of the activity of the pyruvate dehydrogenase complex. Adv. Enzyme Regul. 42, 249–259.
He, A. Y., Yin, C. Y., Xu, H., Kong, X. P., Xue, J. W., Zhu, J., et al. (2016). Enhanced butanol production in a microbial electrolysis cell by Clostridium beijerinckii IB4. Bioprocess Biosyst. Eng. 39, 245–254. doi: 10.1007/s00449-015-1508-2
Hermosilla, E., Schalchli, H., Mutis, A., and Diez, M. C. (2017). Combined effect of enzyme inducers and nitrate on selective lignin degradation in wheat straw by Ganoderma lobatum. Environ. Sci. Pollut. Res. 24, 21984–21996. doi: 10.1007/s11356-017-9841-4
Hsu, P. C., Seol, S. K., Lo, T. N., Liu, C. J., Wang, C. L., Lin, C. S., et al. (2008). Hydrogen bubbles and the growth morphology of ramified zinc by electrodeposition. J. Electrochem. Soc. 155:D400. doi: 10.1149/1.2894189
Jeon, B. Y., and Park, D. H. (2010). Improvement of ethanol production by electrochemical redox combination of Zymomonas mobilis and Saccharomyces cerevisiae. J. Microbiol. Biotechnol. 20, 94–100. doi: 10.4014/jmb.0904.04029
Joshi, B., Bhatt, M. R., Sharma, D., Joshi, J., Malla, R., and Sreerama, L. (2011). Lignocellulosic ethanol production: current practices and recent developments. Biotechnol. Mol. Biol. Rev. 6, 172–182. doi: 10.1111/j.1540-8159.2011.03289.x
Joshi, J., Bhattarai, T., and Sreerama, L. (2018). Efficient methods of pretreatment for the release of reducing sugars from lignocellulosic biomass native to Nepal and characterization of pretreated lignocellulosic biomass. Int. J. Adv. Biotechnol. Res. 9, 9–23.
Joshi, J., Shrestha, R., Manandhar, R., Manandhar, K., Sreerama, L., and Bhattarai, T. (2014). Improvement of ethanol production by electrochemical redox combination of yeast cells. Int. J. Biol. Sci. Appl. 1, 46–54. doi: 10.13140/RG.2.2.19735.06564
Kamcev, J., Sujanani, R., Jang, E. S., Yan, N., Moe, N., Paul, D. R., et al. (2018). Salt concentration dependence of ionic conductivity in ion exchange membranes. J. Membrane Sci. 547, 123–133. doi: 10.1016/j.memsci.2017.10.024
Khatiwada, D., and Silveira, S. (2011). Greenhouse gas balances of molasses based ethanol in Nepal. J. Clean. Prod. 19, 1471–1485. doi: 10.1016/j.jclepro.2011.04.012
Mathew, A. S., Wang, J., Luo, J., and Yau, S. T. (2015). Enhanced ethanol production via electrostatically accelerated fermentation of glucose using Saccharomyces cerevisiae. Sci. Rep. 5, 1–6. doi: 10.1038/srep15713
McGillivray, A. M., and Gow, N. A. R. (2009). Applied electrical fields polarize the growth of mycelial fungi. Microbiology 132, 2515–2525. doi: 10.1099/00221287-132-9-2515
Miller, G. L. (1959). Use of dinitrosalicylic acid reagent for determination of reducing sugar. Anal. Chem. 31, 426–428.
Mohamoud, M. A. (2014). Unexpected redox enhancement and electrochemical pseudo-capacitance performance of polyaniline/poly(vinyl alcohol) (PAn/PVA) composite films. Electrochim. Acta 139, 201–208. doi: 10.1016/j.electacta.2014.06.174
Moscoviz, R., Toledo-Alarcón, J., Trably, E., and Bernet, N. (2016). Electro-fermentation: how to drive fermentation using electrochemical systems. Trends Biotechnol. 34, 856–865. doi: 10.1016/j.tibtech.2016.04.009
Park, D. H., Laivenieks, M., Guettler, M. V., Jain, M. K., and Zeikus, J. G. (1999). Microbial utilization of electrically reduced neutral red as the sole electron donor for growth and metabolite production. Appl. Environ. Microbiol. 65, 2912–2917.
Rose, M., and Palkovits, R. (2011). Cellulose-based sustainable polymers: state of the art and future trends. Macromol. Rapid Commun. 32, 1299–1311. doi: 10.1002/marc.201100230
Ruyters, S., Mukherjee, V., Verstrepen, K. J., Thevelein, J. M., Willems, K. A., and Lievens, B. (2015). Assessing the potential of wild yeasts for bioethanol production. J. Ind. Microbiol. Biotechnol. 42, 39–48. doi: 10.1007/s10295-014-1544-y
Saisirirat, P., and Joommanee, B. (2017). Study on the performance of the micro direct ethanol fuel cell (Micro-DEFC) for applying with the portable electronic devices. Energy Proc. 138, 187–192. doi: 10.1016/j.egypro.2017.10.148
Schievano, A., Pepé Sciarria, T., Vanbroekhoven, K., De Wever, H., Puig, S., Andersen, S. J., et al. (2016). Electro-fermentation – merging electrochemistry with fermentation in industrial applications. Trends Biotechnol. 34, 866–878. doi: 10.1016/j.tibtech.2016.04.007
Seo, H. B., Kim, H. J., Lee, O. K., Ha, J. H., Lee, H. Y., and Jung, K. H. (2009). Measurement of ethanol concentration using solvent extraction and dichromate oxidation and its application to bioethanol production process. J. Ind. Microbiol. Biotechnol. 36, 285–292. doi: 10.1007/s10295-008-0497-4
Song, Y., Wang, J., and Yau, S. T. (2014). Controlled glucose consumption in yeast using a transistor-like device. Sci. Rep. 4, 1–7. doi: 10.1038/srep05429
Steinbusch, K. J., Hamelers, H. V., Schaap, J. D., Kampman, C., and Buisman, C. J. (2010). Bioelectrochemical ethanol production through mediated acetate reduction by mixed cultures. Environ. Sci. Technol. 44, 513–517. doi: 10.1021/es902371e
Tang, X., Guo, K., Li, H., Du, Z., and Tian, J. (2010). Microfiltration membrane performance in two-chamber microbial fuel cells. Biochem. Eng. J. 52, 194–198. doi: 10.1016/j.bej.2010.08.007
Toit, D. M., and Pretorius, I. S. (2000). Microbial spoilage and preservation of wine: using weapons from nature's own arsenal - a review. S. Afr. J. Enol. Vitic. 21, 74–96.
Veech, R. L., Guynn, R., and Veloso, D. (1972). The time-course of the effects of ethanol on the redox and phosphorylation states of rat liver. Biochem. J. 127, 387–397. doi: 10.1042/bj1270387
Wang, X., Kumar, R., and Myers, D. J. (2006). Effect of voltage on platinum dissolution. Electrochem. Sol. State Lett. 9, A225–A227. doi: 10.1149/1.2180536
Yang, E., Choi, M. J., Kim, K. Y., Chae, K. J., and Kim, I. S. (2015). Effect of initial salt concentrations on cell performance and distribution of internal resistance in microbial desalination cells. Environ. Technol. 36, 852–860. doi: 10.1080/09593330.2014.964333
Keywords: electrochemical cell, lignocellulosic biomass, Saccharomyces cerevisiae, Wickerhamomyces anomalous, Saccharum spontaneum, bioethanol
Citation: Joshi J, Dhungana P, Prajapati B, Maharjan R, Poudyal P, Yadav M, Mainali M, Yadav AP, Bhattarai T and Sreerama L (2019) Enhancement of Ethanol Production in Electrochemical Cell by Saccharomyces cerevisiae (CDBT2) and Wickerhamomyces anomalus (CDBT7). Front. Energy Res. 7:70. doi: 10.3389/fenrg.2019.00070
Received: 28 May 2019; Accepted: 11 July 2019;
Published: 31 July 2019.
Edited by:
Sarah Glaven, United States Naval Research Laboratory, United StatesReviewed by:
Darren Greetham, University of Huddersfield, United KingdomRicha Arora, Lovely Professional University, India
Copyright © 2019 Joshi, Dhungana, Prajapati, Maharjan, Poudyal, Yadav, Mainali, Yadav, Bhattarai and Sreerama. This is an open-access article distributed under the terms of the Creative Commons Attribution License (CC BY). The use, distribution or reproduction in other forums is permitted, provided the original author(s) and the copyright owner(s) are credited and that the original publication in this journal is cited, in accordance with accepted academic practice. No use, distribution or reproduction is permitted which does not comply with these terms.
*Correspondence: Jarina Joshi, amFyaW5hcmpvc2hpQGdtYWlsLmNvbQ==; amFyaW5hQGJpb3RlY2h0dS5lZHUubnA=