- 1Chemical Sciences Division, Physical Sciences Directorate, Oak Ridge National Laboratory, Oak Ridge, TN, United States
- 2Bredesen Center for Interdisciplinary Research and Graduate Education, University of Tennessee, Knoxville, Knoxville, TN, United States
- 3Department of Chemistry, Virginia Tech, Blacksburg, VA, United States
- 4Department of Chemical Engineering and Materials Science, Yuan Ze University, Taoyuan, Taiwan
- 5Department of Mechanical, Aerospace, and Biomedical Engineering, University of Tennessee, Knoxville, Knoxville, TN, United States
- 6Department of Chemistry, University of Tennessee, Knoxville, Knoxville, TN, United States
Lithium-ion batteries (LIBs) have attracted worldwide research interest due to their high energy density and long cycle life. Solid-state LIBs improve the safety of conventional liquid-based LIBs by replacing the flammable organic electrolytes with a solid electrolyte. Among the various types of solid electrolytes, hybrid solid electrolytes (HSEs) demonstrate great promise to achieve high ionic conductivity, reduced interfacial resistance between the electrolyte and electrodes, mechanical robustness, and excellent processability due to the combined advantages of both polymer and inorganic electrolyte. This article summarizes recent developments in HSEs for LIBs. Approaches for the preparation of hybrid electrolytes and current understanding of ion-transport mechanisms are discussed. The main challenges including unsatisfactory ionic conductivity and perspectives of HSEs for LIBs are highlighted for future development. The present review provides insights into HSE development to allow a more efficient and target-oriented future endeavor on achieving high-performance solid-state LIBs.
Introduction
Lithium-ion batteries (LIBs) have revolutionized battery technologies, serving as the key component in personal portable electronics, electric vehicles, and stationary energy storage (Ge et al., 2014; Yanilmaz et al., 2016; Zhu et al., 2016b, d; Luo et al., 2017; Famprikis et al., 2019; Lagadec et al., 2019; Lee et al., 2019; Liu et al., 2019; Zhao et al., 2020). Driven by the growing number of applications and ever increasing use of consumer electronics, significant research effort has been focused on developing improved battery systems and reducing production costs (Fu et al., 2014; Luo et al., 2016; Zhu et al., 2018b, c). Conventional LIBs suffer from potential fire hazards caused by short-circuiting of the battery that may cause thermal runaway (Varzi et al., 2016; Manthiram et al., 2017; Wang X. et al., 2020; Zhang C. et al., 2019). In addition, the high reactivity of liquid electrolytes with the electrodes leads to side reactions and capacity fade over time (Xiao et al., 2020). Solid-state electrolytes, on the other hand, are non-flammable, offering higher thermal stability, providing improved safety compared to liquid electrolytes batteries. Furthermore, their potential for enabling the use of a high capacity Li metal anode may greatly enhance the energy and power density and cycle life of current batteries (Pomerantseva et al., 2019). Therefore, the replacement of liquid electrolytes with high-performance solid electrolytes is imperative to overcome the related safety issues of conventional batteries, as well as to improve their energy density by enabling the use of high energy density electrodes (Janek and Zeier, 2016).
An ideal solid electrolyte should possess the characteristics of (1) high ionic conductivity, (2) high electronic resistance, (3) high cation-transference number, (4) wide electrochemical stability window, (5) outstanding thermal stability, (6) excellent mechanical strength, (7) reduced interfacial resistance, and (8) low cost and ease of synthesis (Manthiram et al., 2017). Since an alumina-based solid electrolyte was used in a high-temperature sodium-sulfur battery in the 1960s (Kummer and Neill, 1968; Hsueh and Bennion, 1971), tremendous effort has been made to develop advanced solid electrolytes with the above characteristics. Inorganic electrolytes exhibit high ionic conductivity and mechanical strength, while polymer electrolytes provide flexibility, processability and good contact with the electrodes, reducing the electrode/solid electrolyte interface.
Despite significant improvement in solid electrolytes, there are several remaining challenges (Wang et al., 2014; Lopez et al., 2019; Zhao et al., 2020). For inorganic electrolytes, issues with grain boundary resistance, chemical and electrochemical stability with the electrodes, high cost and lack of processability need to be addressed. Plus, inorganic electrolytes are typically brittle. As a result, they do not compensate for volume change of electrodes during charge and discharge. For polymer electrolytes, its practical application remains hindered by low ionic conductivity at ambient temperature (Agapov and Sokolov, 2011; Wang et al., 2014; Manthiram et al., 2017; Liu et al., 2019; Zhao et al., 2020). Their low ionic conductivity renders them incapable of achieving the charging and discharging rates required in practical utilization. Therefore, developing solid electrolytes that meet all the requirements for the realization of high-performance all-solid-state battery technologies is the key to revolutionize modern electrochemical energy storage devices (Liu et al., 2019).
An efficient way to address the performance issues of solid electrolytes is to hybridize two or more component for combined advantages of each component. Hybrid solid-state electrolytes (HSEs) may combine the advantages of inorganic and polymer electrolytes while overcoming the disadvantages of each component when used separately, as shown in Figure 1. The main strategy of HSEs is to disperse high-surface-area inorganic fillers into a polymer matrix, which is the focus of this review. Other morphologies include using ceramic as the matrix, fiber mat and so on. Compared to inorganic and polymer electrolytes, hybrid electrolytes exhibit a combined advantage of each component including high ionic conductivity, good mechanical properties from inorganic component as well as reduced interfacial resistance from polymer component. The up-to-date performance metrics of ionic conductivity, transference number, and electrochemical stability of recently developed HSEs are summarized in Table 1. To date, a number of inorganic fillers have been utilized, such as inert fillers (Al2O3, SiO2, TiO2, LiNbO3, and BaTiO3) and active fillers consisting of metal oxides or sulfides (Appetecchi and Passerini, 2000; Adebahr et al., 2003; Kumar, 2004; Zhao et al., 2016a). It is essential to summarize the state-of-the-art progress of HSEs based on the most recently available publications. A brief overview of solid-state electrolytes is provided, and are divided into three categories: inorganic electrolytes, polymer electrolytes, and hybrid electrolytes. Furthermore, the performance of various HSE systems is discussed in detail, along with ion transport mechanisms and fabrication methods. Despite many studies, current HSEs still have relatively low ambient ionic conductivity (<10–3 S/cm), poor interfacial stability, and high interfacial resistance, which greatly restrict the performance of the LIBs. In the end of this review, the current challenge and perspective of HSEs are discussed.
Hybrid Solid Electrolytes
Polymer Matrix
Polymers are widely used as solid electrolytes. Polymer electrolytes are attracting increasing interest because of their flexibility, processability, high safety, and scalability. Polymer electrolytes have been studied for use in a variety of high energy and power density batteries, including lithium-ion, lithium-sulfur, and lithium-air rechargeable batteries (Kim J. G. et al., 2015; Sun et al., 2017). With the incorporation of lithium salts, the ionic conductivity of solid-state polymer electrolytes can reach 10–6 to 10–5 S/cm at room temperature (Wang et al., 2016; Meabe et al., 2019). With the covalent attachment of anions to the polymer backbone, single lithium ion conducting polymer electrolytes show a lower ionic conductivity of 10–7 to 10–6 S/cm at room temperature (Cao et al., 2017, 2020; Zhang H. et al., 2017). Recent study demonstrated the remarkably high Li+ conductivity of 5.84 × 10–4 S/cm at 25°C (Ahmed et al., 2019). Due to its flexible nature and good adhesive properties, polymer electrolytes components can form good contact with the electrodes. These unique electrochemical properties highlight the promise of polymer electrolytes for solid-state battery applications. The polymers commonly used as electrolytes include poly(ethylene oxide) (PEO), poly(carbonate) (PC), poly(siloxane), poly(acrylonitrile) (PAN), and poly(vinylidene fluoride) (PVDF). For many polymer electrolytes, ion mobility is strongly coupled to the segmental motion of the polymer chains, limiting the achievable ambient conductivity (Wang et al., 2014; Porcarelli et al., 2016). In addition, the crystalline phase of polymers (e.g., PEO) generally hinders ion transport below the melting point (Tm∼ 65°C for PEO) (Xue et al., 2015). To reduce the degree of crystallinity and the glass-transition temperature (Tg) of polymers, a variety of methods have been explored, including polymer blends, copolymerization and crosslinking (Soo, 1999; Wen et al., 2000; Fullerton-Shirey and Maranas, 2009; Lopez et al., 2019; Cao et al., 2020).
Among polymer electrolytes, PEO-based polymer electrolytes are most extensively studied due to its faster polymer dynamics at ambient temperature and excellent capability to solvate various salts (Lopez et al., 2019). Salt-doped PEO exhibits reasonable ionic conductivity as an HSEs component due to its low glass transition temperature (∼-30°C with salts), (Senthil Kumar et al., 2014) remaining in a rubbery state at room temperature. Additionally, PEO demonstrates an outstanding ability to solvate large concentration of lithium salts (Berthier et al., 1983; Karan et al., 2008) and it may have favorable interactions with inorganic fillers (Croce et al., 1999; Malathi and Tamilarasan, 2014). The ether oxygen atoms in PEO act as a Lewis base to coordinate with the Li-ion (Meyer, 1998). The coordination of PEO with the Li-ion creates pseudo ionic crosslinks that restrict the movement of Li+ and slows down the segmental motion of the polymer chains when the concentration of salt becomes high (typically above EO:Li = 12) (Lehmann et al., 2019). The restricted segmental motion of the PEO chains results in an increase in Tg and a reduction in ionic conductivity. The low Li transference number in PEO also originates from the strong coordination of the Li-ion by the ether oxygens. As a result, large anions like trifluoromethanesulfonyl imide (TFSI–) moves faster than the small cation. Another weakness of PEO is its high crystallinity (>60%), originating from its linear structure. Due to these properties, the room temperature ionic conductivity of PEO with Li salts is low (10–6 S/cm), 3–4 orders of magnitude lower than that of commercial liquid electrolytes (Wright, 1975; Lee et al., 2005). To decrease the crystallinity and improve the mechanical properties of PEO, crosslinking has been adopted. The network structure with short PEO chains between crosslinking helps to decrease the crystallinity of PEO; however, the mobility of the polymer segments is also restricted, such that the ionic conductivity is often reduced (Maccallum et al., 1984). Another commonly used method to decrease the crystallinity of PEO, is to add inorganic or organic plasticizers to the polymer, which was first proposed in Liang (1973). PEO with organic plasticizers typically exhibits worse mechanical properties than neat PEO (Quartarone et al., 1998). On the other hand, PEO with inorganic fillers usually shows improved mechanical and thermal stability (Weston and Steele, 1982). In addition to fillers, polymer blending can improve the mechanical strength of a PEO electrolyte. PEO/P(VDF-HFP)/LLZTO electrolyte shows largely enhanced tensile strength of 3.5 MPa and Young’s modulus of 53.5 MPa (∼10 times higher than that of PEO/LLZTO SPE) (Wang T. et al., 2020). This approach of polymer–polymer hybrid solid electrolytes provides another avenue to enhance the mechanical property and overall electrolyte properties.
PCs have also drawn attention for use as solid electrolytes. The advantage of PCs includes their high dielectric constant and ability to dissolve lithium salts. Spectroscopic techniques and molecular dynamics simulations indicate that both of the oxygen atoms in PC (carbonyl group oxygen and alkoxy oxygen) can coordinate with Li+ (Matsumoto et al., 2013; Ong et al., 2015). As a commercially available polymeric material, PC made from bisphenol A (Scheme 1A) exhibits high strength, toughness, and good durability. However, bisphenol A PC without any modifications cannot be utilized as an HSE, due to its low ambient temperature ionic conductivity of 7 × 10–9 S/cm (Spiegel et al., 2000). The Tg of PCs made from bisphenol A is typically above 100°C (i.e., Tg of Lexan is 145°C) (Yang and Yetter, 1994) due to the rigid phenol group in the backbone. Therefore, the mobility of polymer chains is limited at room temperature, resulting in its low ionic conductivity. Matsumoto et al. (2013) synthesized a PC by utilizing hydroquinone in place of bisphenol A (Scheme 1B) and by adding plasticizers. Although the conductivity (1.5 × 10–6 S/cm at 30°C) is still not satisfactory, the transference number was improved. The Li-ion transference number was above 0.5, higher than that of PEO (transference number is only 0.2–0.5) (Evans et al., 1987; Pożyczka et al., 2017). The weaker coordinating interaction of both the carbonyl oxygen and alkoxy oxygen groups in PC with Li+ compared with that of the ether oxygen in PEO contributes to the higher Li-ion transference number. With the introduction of aliphatic groups into the PC backbone, a highly flexible PC can be fabricated. Meabe et al. (2017) synthesized a series of PCs with (CH2)x where x = 4, 5, 6, 7, 8, 9, 10, and 12 (Scheme 1C). The results showed that the solid electrolyte made from PCs with (CH2)x exhibited an optimal conductivity and lowest Tg when x = 7. With the addition of 80 wt% LiTFSI, the conductivity of low molecular weight poly(dodecamethylene carbonate) can reach 1 × 10–4 S/cm at room temperature (Meabe et al., 2017). An alternative method to incorporate a carbonate group into a polymer is via sidechain instead of in the backbone. For example, poly-1,1-di[2- (2,4-dioxa-3-pentanoyl)ethyl]silacyclobutane (polySBDC) (Scheme 1D), exhibited a significantly decreased Tg (−25°C) with the ionic conductivity reaching 1.2 × 10–4 S/cm at 30°C (Matsumoto et al., 2016). Copolymerization of PC with a polyester (carbonate: ester = 80: 20) achieved a room temperature ionic conductivity of 4.1 × 10–5 S/cm (Scheme 1E) (Mindemark et al., 2015).
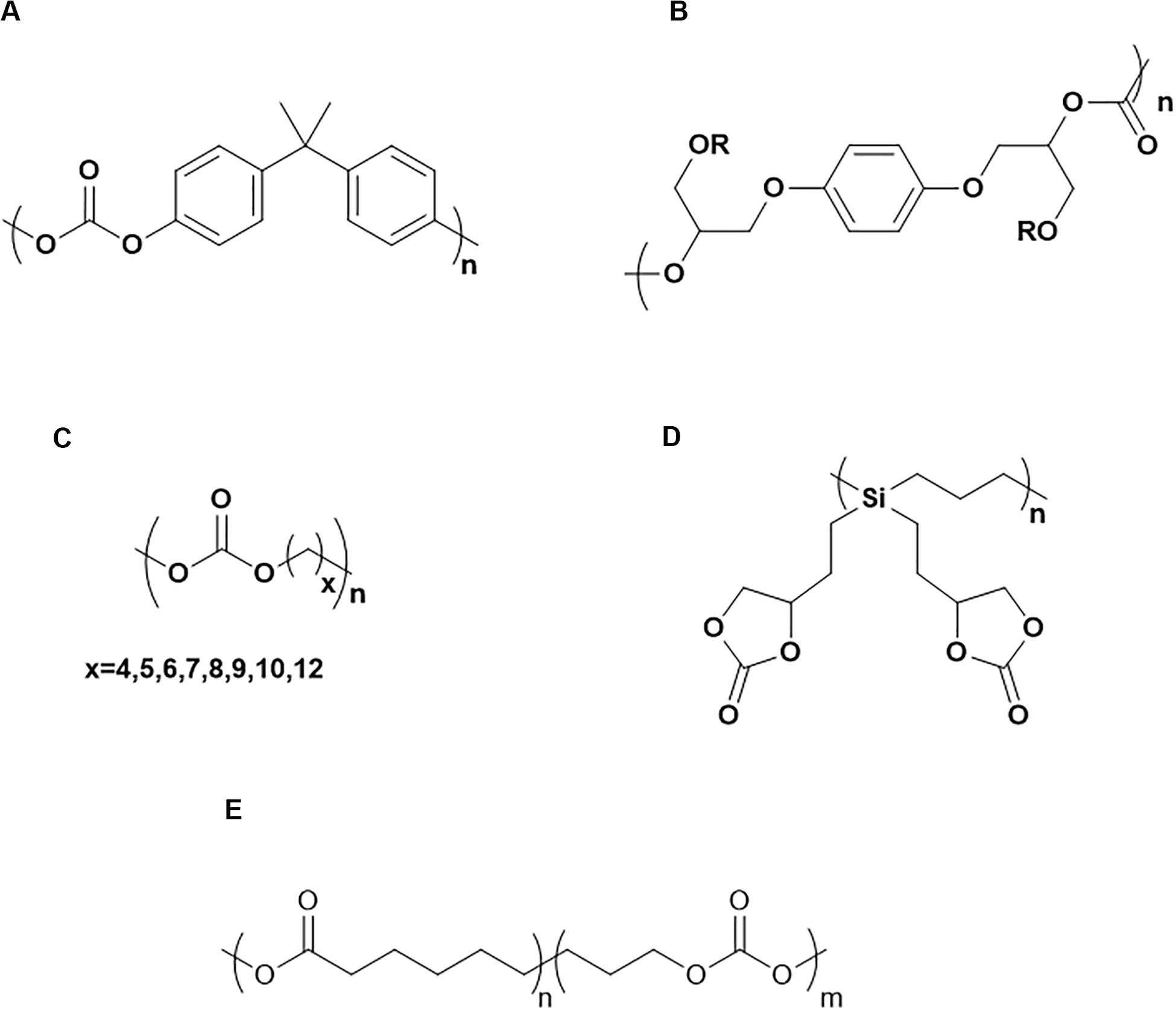
Scheme 1. Chemical structure of (A) PC made from Bisphenol A, (B) PC made from hydroquinone group, (C) APC with different length of CH2, (D) PolySBDC, (E) Poly(carbonate-co-ester).
PAN is the first polymer to be used as a solid electrolyte that contains the nitrile group. The nitrogen atom in PAN is considered to have the ability to coordinate with Li+ due to its strong electronegativity. In fact, nitrile groups can be found in liquid electrolytes and additives. PAN (Scheme 2A) is a semi-crystalline polymer at room temperature with a Tg of ∼80°C. Based on these thermal properties, PAN was not expected to have high ionic conductivity, because the PAN chains are less flexible than that of PEO. PAN exhibited an ionic conductivity of 6.5 × 10–7 S/cm with 20 wt% of LiCIO4 at room temperature (Yang et al., 1996). The main challenge for PAN is its limited solubility in organic solvents. Dimethylformamide (DMF) and dimethyl sulfoxide (DMSO) are commonly used to dissolve PAN. Since they are high boiling point solvents, it is very challenging to remove them from a solvent-cast polymer electrolyte. As a result, the residual solvent trapped in the polymer matrix significantly affects the Li+ transport and electrochemical stability of the solid electrolyte (Gutmann, 1976). Forsyth et al. (2000) utilized a hot-press method to prepare solvent-free PAN films. With 72 wt% LiCF3SO3, its conductivity reached 2 × 10–6 S/cm at 65°C (Forsyth et al., 2000). To make the nitrile-based polymer more soluble, a methyl group can be introduced into the backbone. Poly(methacrylonitrile) (PMAN) (Scheme 2B) can be dissolved in acetone. The film contained no residual solvent with the use of the low boiling point casting solvent. The reported conductivity of PMAN is ∼10–5 S/cm at 75°C. However, the PMAN film has a high Tg (110°C), which limits its performance at room temperature (Saunier et al., 2002). Another strategy to improve the solubility of PAN is to incorporate PAN with other polymer segments. Florjañczyk et al. reported a copolymer of PAN with butyl acrylate (Scheme 2C). The butyl acrylate group makes it possible to prepare the electrolyte using a solvent casting method with acetonitrile. In addition, the Tg of the copolymer decreases to 42–44°C and shows a high capability of dissolving lithium salts (Florjañczyk et al., 2004, 2005).
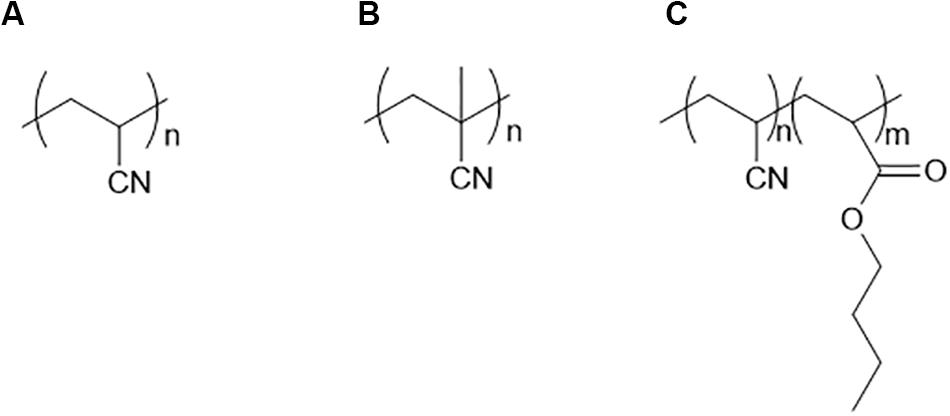
Scheme 2. Chemical structure of (A) PAN, (B) PMAN, (C) Copolymer of acrylonitrile and butyl acrylate.
Fillers
Many types of inorganic materials such as perovskite-type, garnet-type, sodium superionic conductor (NASICON)-type, amorphous oxides, and sulfide materials have been developed as a LIB electrolyte (Inaguma et al., 1993; Zheng et al., 2018). As reported, perovskite-type [e.g., Li0.34(1)La0.51(1)TiO2.94(2) (LLTO)] (Inaguma et al., 1993) and garnet-type [e.g., Li5La3M2O12 (M = Nb or Tb) and Li6ALa2M2O12 (A = Ca, Sr or Ba; M = Nb or Ta)] (Geiger et al., 2011; Murugan et al., 2011) solid electrolytes exhibit high ionic conductivity of > 10–3 S/cm at room temperature. NASICON-type materials generally have an AM2(PO4)3 formula (A = Li, Na, or K; M = Ge, Zr, or Ti) (Thangadurai and Weppner, 2006). For this type of solid electrolyte, their ionic conductivity can be improved by the substitution of the metal (Ge, Zr, or Ti) by Hf or Sn (Martinez-Juarez et al., 1995). Among all inorganic solid electrolytes, sulfide-type solid electrolytes (i.e., Li2S-SiS2) are another popular electrolyte and investigated due to exhibiting some of the highest reported ionic conductivities. Kamaya et al. reported that the ionic conductivity of a Li3PS4 crystal was significantly increased to 2.2 × 10–3 S/cm at room temperature by doping with phosphorus and germanium (Kamaya et al., 2011). In addition, their wide electrochemical stability window could be beneficial for use in high-voltage solid-state batteries (Chen et al., 2018b). However, the major drawback of inorganic materials, whether amorphous or crystalline, is their brittleness, making large scale production quite challenging. Furthermore, maintaining good contact between the electrolyte and electrodes is difficult because of their lack of flexibility and volume changes during charging and discharging (Muramatsu et al., 2011). Therefore, by using polymer as the matrix with inorganic fillers, the interfacial property between the electrolyte and electrode will be significantly improved, thus improving the overall battery performance.
In HSEs, inorganic fillers play an important role in determining the performance of the battery. In the following section, we divide the polymer-inorganic composite electrolyte into two categories based on the function of the filler; inert fillers, with no bulk Li-ion conduction, and active fillers usually with high bulk Li-ion conduction.
Inert Fillers
Inert fillers do not conduct Li+ themselves. Ceramic nanoparticles utilized in HSEs include Al2O3, TiO2, and SiO2 (Croce et al., 1998). Even though ceramic oxides do not participate in ion conduction directly, it was reported that ionic conductivity increased with the addition of the ceramic filler over a wide temperature range (30–100°C) (Croce et al., 1998). In hybrid electrolytes, the inorganic fillers not only act as solid plasticizers to inhibit the crystallization kinetics of the polymer segments but also promote ion mobility. Lin et al. (2016) reported that the crystallinity of PEO was highly decreased by SiO2 via the in situ hydrolysis method compared to the blending method, attributing to their much stronger chemical/mechanical interactions between monodispersed 12 nm diameter SiO2 nanospheres and PEO chains, and thus facilitates polymer segmental motion for ionic conduction. This led to good ionic conductivity (1.2 × 10–3 S/cm at 60°C, 4.4 × 10–5 S/cm at 30°C). Besides the ionic conductivity, incorporating inert fillers with the polymer electrolyte was also reported to widen the electrochemical stability window up to 5.5 V (Lin et al., 2016).
Weston and Steele (1982) firstly studied the effects of incorporating inert Al2O3 fillers to a LiClO4/PEO electrolyte in 1982. From then on, inert fillers with different sizes as well as surface functionalities have been extensively investigated attributing to the important role of the fillers surfaces/interfaces in improving conductivity. The interfacial area is strongly dependent on the size and content of inert fillers.
Ceramic nanoparticles influence not only the electrochemical properties but also the mechanical properties of polymer electrolytes. Ramesh et al. (2007) studied the effect of SiO2 on the mechanical properties of a poly(vinyl chloride)- PEO polymer electrolyte. The Young’s modulus increased from 500 to 2300 Pa with the addition of 10% SiO2 (Ramesh et al., 2007). Wen et al. investigated the effect of BaTiO3 on a PEO-poly(propylene oxide) crosslinked amorphous polymer. With 10% BaTiO3, the tensile strength improved from 1.42 to 2.12 MPa at 80°C (Wen et al., 2003).
Another interesting direction of the inert filler is the interaction between the functional group on the inert filler surface and the polymer chains. Liu et al. studied the interaction between the −OH/H functional group on the SiO2, Li+, and the PEO chains. It was concluded that with SiO2, the ionic conductivity of PEO was not improved. Instead, the transference number was highly increased up to 0.56. The results were ascribed to the hydrogen bond between the -OH/H functional group on the SiO2 surface and the PEO chain. The hydrogen bonding provided a free space around the SiO2 particle and the free space would favor an absorption-like process. The anion, which contains -F elements, was trapped in the free space. Thus, the mobility of the anion was hindered (Liu et al., 2004).
Active Fillers
Active fillers participate in ionic conductivity. Metal oxides or sulfides are promising lithium-ion conductors. Li-ion transport in an active filler composite system is complex, and the proposed mechanisms are discussed in Section “Hybrid Solid Electrolytes.” In this section, we provide an overview of several active fillers and their electrochemical performance.
Li1+xAlxTi2–x(PO4)3 (LATP) and Li1+xAlxGe2–x(PO4)3 (LAGP) are superionic conductors with the same structure as NASICON, but in Li-ion conducting form (Xu et al., 2004). When the Ti/Ge elements are partially substituted with Al, the ionic conductivity can be elevated from 10–4 S/cm to 10–3 S/cm for LATP (Aono, 1990; Xu et al., 2006) and 10–2 S/cm for LAGP (Kumar et al., 2009) at room temperature. The use of Li1.3Al0.3Ti1.7(PO4)3 as an active filler in a PEO matrix was first reported in Nairn et al. (1996). With a 66% LATP loading in PEO (w/w), the ionic conductivity was increased to 10–4 S/cm at 40°C, two orders of magnitude higher than pure PEO. Wang et al. further systemically studied Li1+xAlxTi2–x(PO4)3/PEO composites with varied Li/EO ratios, amount of x in LATP, and LATP/PEO weight ratios (Wang Y.-J. et al., 2006). At a fixed EO to Li ratio of EO/Li = 8, the ionic conductivity of Li1+xAlxTi2–x(PO4)3 reaches its maximum value when x = 0.5. For PEO-Li1.3Al0.3Ti1.7(PO4)3, (x = 0.3) composite, the maximum ionic conductivity (10–6 S/cm at room temperature) occurs when EO/Li = 16. The presence of LATP was found to suppresses the crystallinity of PEO at low loadings (5 wt%) but the degree of PEO crystallinity increases with increasing addition of LATP. In addition, compared to the randomly dispersed LATP, it was found that a vertically aligned structure of LATP in PEO can enhance the ionic conductivity to 5.2 × 10–5 S/cm (Zhai et al., 2017). A similar strategy was applied to LAGP with PEO. The room temperature ionic conductivity increased 6 times for a composite with an aligned ceramic structure (1.7 × 10–4 S/cm) compared to the randomly dispersed one (2.7 × 10–5 S/cm). The aligned composite electrolyte in a Li//LiFePO4 cell showed a capacity retention (above 85% after 400 cycles) at 0.6 C (Wang et al., 2019). Li et al. fabricated a 3-dimensional fiber network consisting of LATP and PAN, which they embedded into a PEO matrix. The LATP/PAN/PEO composite electrolyte exhibited an electrochemical stability window of 5 volts and a 10.7 MPa tensile strength (Li D. et al., 2018). LAGP has higher ionic conductivity than LATP because LAGP has a lower resistance at the interface with the polymer (Mariappan et al., 2012). Jung et al. (2015) studied a LAGP-PEO composite electrolyte with different weight ratios. It was found that with 60% (w/w) LAGP, the cells showed the lowest electrode-electrolyte interfacial resistance and best rate capability (Jung et al., 2015).
Perovskites are another family of inorganic ionic conductors, among which Li3xLa2/3–xTiO3 (LLTO) is the most commonly used. Li0.34La0.51TiO2.94 exhibits an ionic conductivity of 10–5 S/cm at room temperature, which was first reported in Inaguma et al. (1993). LLTO nanowire and nanoparticle PAN composite electrolytes were studied by Liu et al. (2015). An LLTO nanowire/PAN composite showed improved ionic conductivity compared to the one with dispersed nanoparticles at the same ceramic loading, due to the continuous conductive networks formed by the nanowires. The same strategy was also applied to PEO-based composite, resulting in an increased ionic conductivity of 2.4 × 10–4 S/cm at room temperature (Zhu et al., 2018a).
Garnet type inorganic ion conductors are also commonly used in composite electrolytes. Li7La3Zr2O12 (LLZO) is a promising material for use in Li-ion batteries. With an LLZO loading of 52.5% (w/w), the ionic conductivity of PEO-based HSE can achieve 10–4 S/cm at 55°C (Choi et al., 2015). Further studies have developed integrated all-solid-state batteries based on this composite electrolyte. The batteries exhibited stable performance in a Li//LiFePO4 cell. When LLZO was placed in a PVDF-HFP matrix, the electrochemical stability window was widened (Zhang et al., 2018). A derivative material of LLZO was fabricated by the partial substitution of Zr by Ta (LLZTO) and was also studied as a composite electrolyte. However, there was little improvement in the ionic conductivity of the LLZTO/PEO composite electrolyte (1.9 × 10–5 S/cm) compared to the LLZO/PEO composite (1.0 × 10–5 S/cm) at 30°C (Choi et al., 2015; Zha et al., 2018). With the utilization of a poly(propylene carbonate) matrix instead of PEO, the conductivity of an LLZTO/poly(propylene carbonate) composite was improved to 5.2 × 10–4 S/cm at 21°C (Zhang J. et al., 2017).
In addition to the oxides mentioned above, sulfides are another class of inorganic ionic conductors, and the various types are comprehensively reviewed by Chen et al. (2018c). Compared with oxides, S2– has a larger ionic radius and lower electronegativity, thus, providing a weaker association with Li+. The ionic conductivity of sulfides is generally higher than oxides. One of the highest reported ionic conductivities (10–2 S/cm at room temperature) of a sulfide-based inorganic electrolyte is for Li10GeP2S12. The high ionic conductivity is attributed to the formation of anisotropic Li+ diffusion pathway in the Li10GeP2S12 crystalline structure. The combination of sulfides with a polymer not only show improved ionic conductivity but also have an extended electrochemical stability window of 5.7 V (Zhao et al., 2016b). A study utilizing high loadings (95 wt%) of a 78Li2S–22P2S5 glass type ceramic compared the performance of HSEs with PVDF and PEO polymer matrices (Zhang et al., 2020). While PVDF provided a higher ionic conductivity (4.54 × 10–4 S/cm) than PEO (1.27 × 10–4 S/cm) at room temperature, the LiTFSI salt was found to be inhomogeneously distributed in the PVDF composite. The composites achieved a charge/discharge capacity of 566 mA h/g for the PVDF and 725 mA h/g for the PEO in a Li//sulfur-carbon nanotube cell after 100 cycles.
Ion Conduction Mechanisms in Hybrid Solid Electrolytes
Ion conduction mechanisms in inorganic solid electrolytes are quite straightforward and generally occurs by ion hopping. The ions diffuse through the connected available vacant and interstitial sites in the crystal. The ionic conductivity of the solid electrolytes is determined by the concentration and distribution of the defects. In polymer electrolytes, the current understanding of ion conduction is the combination of ion movement with local polymer segmental motion and ion jumping over some potential energy barrier if the segmental motion is slow (Gibbs and DiMarzio, 1958; Cohen and Turnbull, 1959; Fan et al., 2018; Bocharova and Sokolov, 2020). The addition of inorganic fillers to the polymer electrolytes has proven to be an effective way to increase its ionic conductivity (Chen and Vereecken, 2019; Dirican et al., 2019). Recent efforts on explaining how ceramic fillers positively enhance the ionic conductivity is described below.
Ionic Conduction With Inert Fillers
Current understanding of the effects of the inert fillers, such as micro/nanoparticles, Al2O3 (Weston and Steele, 1982; Croce et al., 1998, 1999, 2000; Appetecchi et al., 2000), SiO2 (Fan et al., 2003; Tominaga et al., 2005; Wang X.-L. et al., 2006; Tominaga and Endo, 2013), TiO2 (Croce et al., 1999, 2000; Appetecchi et al., 2000; Croce et al., 2000), ZrO2 (Croce et al., 2006), molecular sieves (Munichandraiah et al., 1995; Xi et al., 2004), and ferroelectric materials, has focused on two mechanisms: (1) the promotion of salt dissociation to create more free Li-ions by acting as centers of Lewis acid-base interactions; (2) the suppression of polymer crystallization.
The first hypothesis, the Lewis acid-base theory, was first proposed by Wieczorek in 1995 to explain the interactions between inorganic filler, polymer, and salt (Przyluski et al., 1995; Wieczorek et al., 1995, 1996). With the Lewis acid-base theory, the explanation is that in HSEs systems, the Li-ion is considered as a Lewis acid, and the solvating groups of polymers and ClO4– anions act as Lewis bases. The fillers can be considered a Lewis acid or base, depending on their surface chemistry (Figure 2). Hence, several acid-base interactions are occurring in these systems. An acidic surface can attract the polymer solvating groups or ClO4–, leading to free associated Li+ cations. The addition of a basic surface attracts Li-ions and polymer functional groups, improving the dissociation of Li+-ClO4– ion pairs. Croce et al. added superacid ZrO2 to a PEO/LiBF4 complex. The Li-ion transference number was greatly improved as the acidic surface of the ceramic filler demonstrated a higher affinity toward ClO4– than that of Li+, promoting the separation Li+-ClO4– ion pairs (Croce et al., 2001).
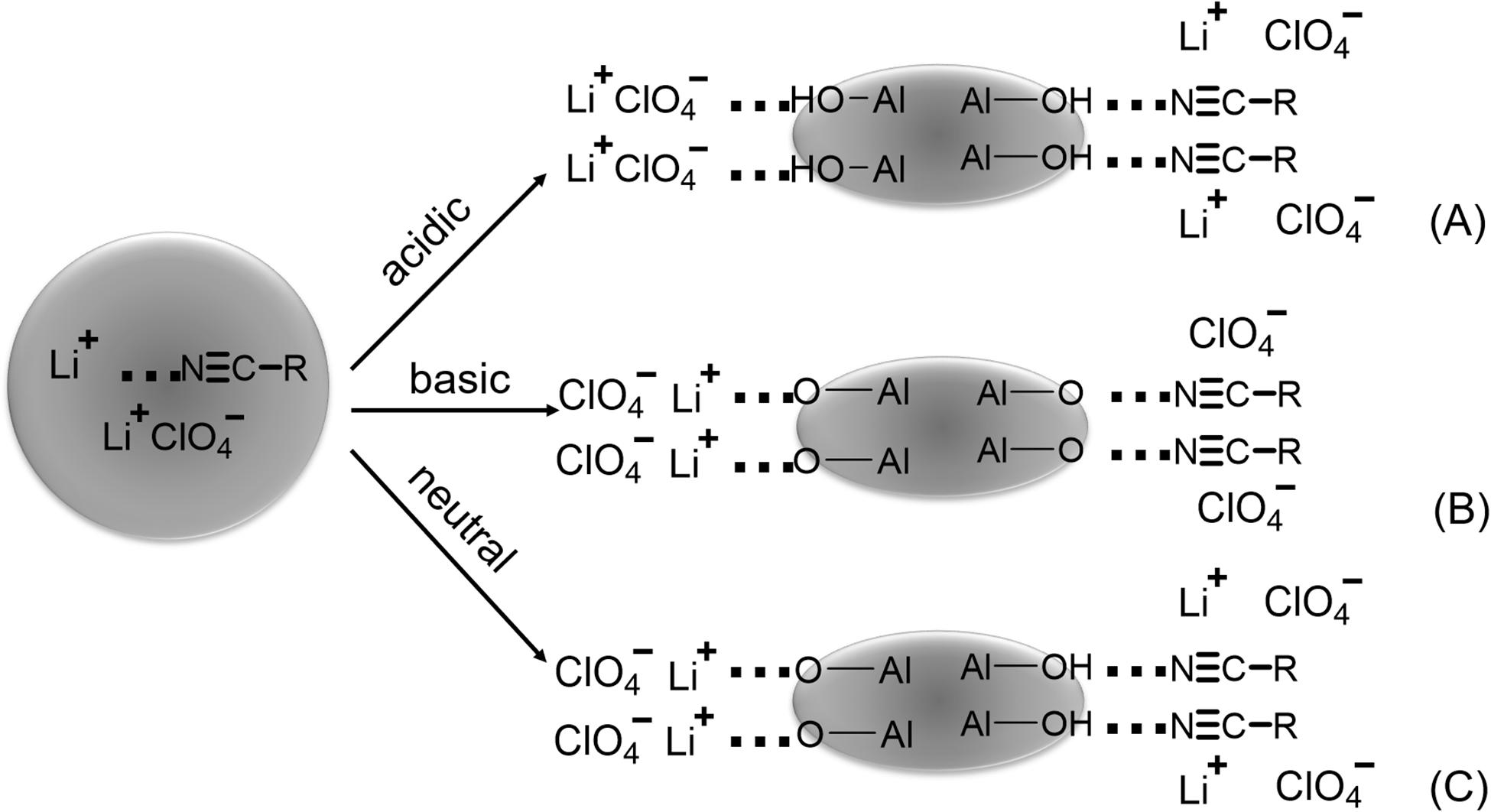
Figure 2. Schematic demonstration of the Lewis acid-base dissociation effects of (A) Lewis acidic, (B) Lewis basic, and (C) neutral surface groups on ionic conductivity. Reprinted with permission from reference Wang et al. (2003). Copyright 2003 The Electrochemical Society, Inc.
In PEO-based electrolyte systems, studies have shown that the crystallization of the polymer is suppressed with the addition of inert fillers, which further increases the ionic conductivity (Bouchet et al., 2013; Hanson et al., 2013; Khurana et al., 2014; Das and Ghosh, 2015; Zhu et al., 2018a). Ion transport in PEO is strongly coupled to segmental dynamics, and freezing of segmental motion in crystalline phase suppresses ionic conductivity. The addition of inert fillers suppresses the crystallinity of PEO, which leads to increased segmental dynamics, resulting in a higher conductivity. Researchers have shown that the addition of γ-LiAlO2 filler into PEO-based electrolyte greatly reduces the crystallization rate, increasing ionic conductivity, and the lithium/electrolyte interfacial stability (Hu et al., 2007; Tan et al., 2016).
However, Lewis interactions and polymer crystallinity are not the full picture of ion conduction mechanisms in HSEs with inert fillers. Marcinek et al. (2000) have shown that the ionic conductivity for a PEG-based electrolyte increases with the addition of neutral, acidic, or basic inert filler Al2O3 (Croce et al., 2001). The ionic conductivities of the electrolytes for acidic and basic surfaces are similar, and both are higher than the one with a neutral surface. However, the trend of the fraction of ion pairs measured for the electrolytes in the order of acidic > neutral > basic surface is inconsistent with the trend of the fraction of free ions. Fan et al. added fumed-silica particles into PEG-based electrolytes with lithium salts, leading to a better conductivity of the composite electrolyte. However, no conductivity differences were observed between the non-polar PEG with modified polar PEG electrolytes (Fan, 1997). Hanson et al. (2013) reported theoretical results of atomistic molecular dynamics simulations to study lithium ion diffusivities in PEO/TiO2 and observed that the lithium ion diffusivities decrease with increased particle loading. Those contradictions may indicate the involvement of other factors or ion conduction mechanisms functioning in parallel, which are not yet fully revealed.
Ionic Conduction With Active Fillers
The ion conduction mechanisms in HSEs with active fillers are complicated, as not only the active fillers can influence the polymer chain structure, but also it can create additional Li+ pathways within the composites. The pathways include active filler, polymer, and polymer/active filler interface. Many studies have shown the new Li+ pathways created by the active fillers such as LLZO (Murugan et al., 2007; Tao et al., 2017), LLTO (Le et al., 2015; Liu et al., 2015), and LATP (Goodenough et al., 1976; Aono, 1990; Chen X. C. et al., 2019; Peng et al., 2020), are the main reasons for the increase in ionic conductivity. These pathways, together with Li-ion concentration and ion mobility, determine the ionic conductivity of HSEs.
To understand the Li+ diffusion pathway, Zheng et al. (2016) took advantage of high-resolution 6Li NMR to investigate Li+ ion transportation in different phases of an HSE. The method builds on the basis that 6Li replaces 7Li during Li+ transport. Using a composite electrolyte with a mixture of PEO/LiClO4 polymer matrix and LLZO (50 wt%), the authors confirmed the presence of three ways of Li-ion transport (Li+ in the polymer phase, ceramic phase, and polymer-ceramic interface) within the material. Furthermore, by evaluating changes in the 6Li amount before and after electrochemical cycling tests revealed that Li+ diffusion in PEO-based electrolyte with LLZO (50 wt%) active filler preferred the pathway through the LLZO ceramic phase (Grey and Dupré, 2004; Bhattacharyya et al., 2010; Ilott et al., 2014). Yang et al. (2017) utilized a similar method to investigate the Li+ pathway in the electrolyte of PAN/LiClO4/LLZO nanowire. The results showed a strong interaction between LLZO nanowires and the PAN polymer chains. Cycle tests demonstrated that the Li+ preferred to diffuse through the PAN-nanowire interface instead of the polymer or ceramic phase in HSEs with a low concentration of LLZO filler. In another study of PEO/LiClO4/LATP HSEs, it has been shown that the ionic conductivity started to decrease beyond 4 vol% of LATP filler. The decrease was attributed to the agglomeration of nanoparticles that decreases volume ratio of the interface. Roman et al. studied the particle size effects on the ionic conductivity and found that the ionic conductivity increases as the particle size decreases, which is consistent with the prediction of the continuum percolation model (Roman, 1990). Zheng and coworkers did a systematic investigation on the compositional dependence of the three main factors for ionic conductivity, including ion mobility, ion transport pathways, and active ion concentration (Zheng and Hu, 2018). As shown in PEO (LiTFSI)/LLZO, with LLZO concentrations of 5 wt%, 20 wt%, and 50 wt% system in Figure 3, although active ion concentration increases with the addition of more ceramic filler, the actual fraction of Li+ participating in the conduction does not show the same trend. At low contents of LLZO (5 and 20 wt%), with the increase of LLZO content, ion mobility increases, and ion transport is primarily through the PEO phase. At high LLZO content (50 wt%), a percolated network is formed by LLZO, through which most of the ion transport occurs. The 50 wt% LLZO composite showed notably lower ionic conductivity compared to the other composites, due to LLZO aggregation blocking the ion transport pathway though PEO and the high activation energy required for a Li-ion to move from one discrete LLZO particle to another or to the PEO phase. In general, the battery performance increases with increasing the loading of the fillers, when the loading ratio is small. Once the filler loading reaches certain level, the performance will not improve any more or even deteriorates due to filler aggregation.
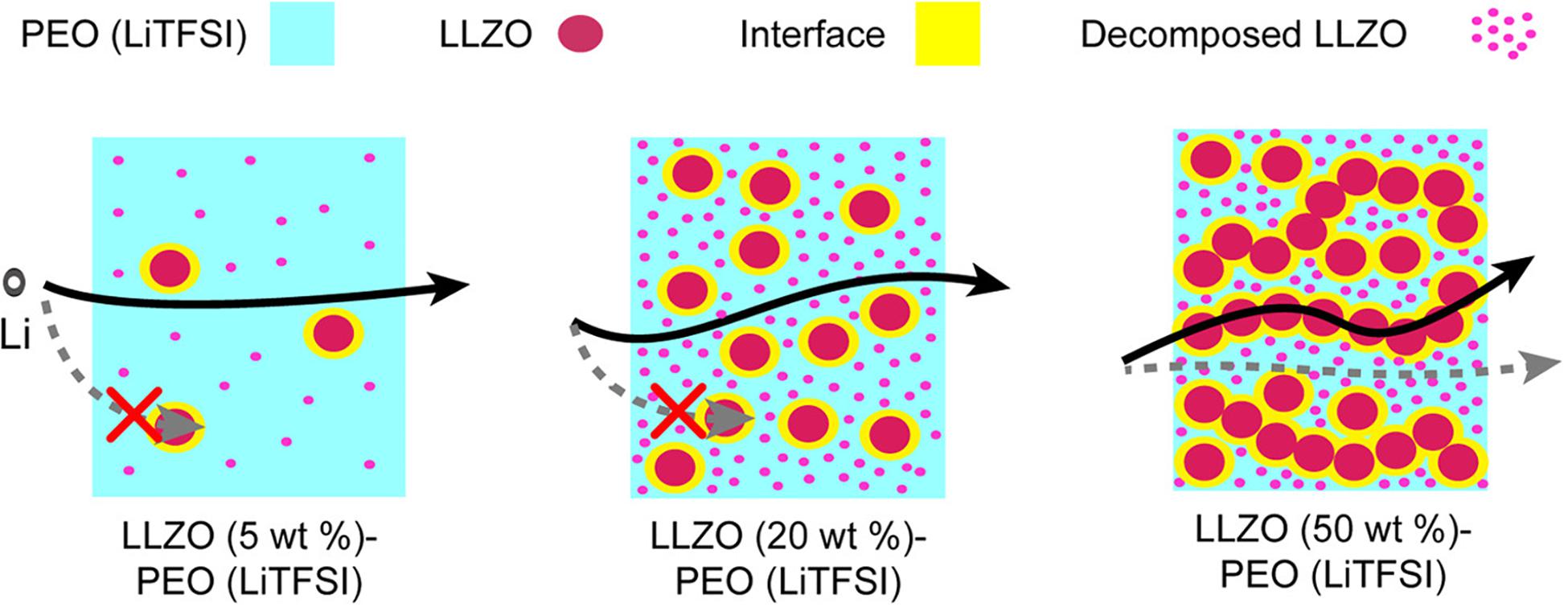
Figure 3. Schematic of Li-ion pathways for PEO (LiTFSI)/LLZO with LLZO concentrations of 5 wt%, 20 wt%, and 50 wt%. Reprinted with the permission from reference Zheng and Hu (2018). Copyright 2018 American Chemical Society.
Preparation of Hybrid Solid Electrolytes
Hybrid solid electrolytes have been widely studied as promising electrolytes systems due to the synergetic effects of each component, which provide new opportunities to develop high-performance batteries with improved safety (Shim et al., 2014; Yue et al., 2016; Zhang et al., 2018). For HSEs, various preparation methods including casting, electrospinning, and in situ polymerization are utilized. Optimization of the preparation method is critical for enabling HSEs to achieve high performance characteristics such as good interfacial contact and the formation of ion-conduction pathways (Kim J.-K. et al., 2015; Manthiram et al., 2017; Liu et al., 2018). As such, the various fabrication methods employed in the preparation of HSEs are discussed in the following sections.
Casting
Casting is one of the common techniques used to prepare polymer films and their composites, and is applied in a variety of fields (Zhu et al., 2012, 2014). In a typical preparation method, a polymer is dissolved in a suitable solvent together with a salt and inorganic material to prepare a slurry and is then cast on a substrate to fabricate the HSE film. The films can be obtained after drying the slurry in a (vacuum) oven under certain conditions. For example, a facile tape casting method was performed by Chen et al. to prepare a thin solid electrolyte with improved interfacial contact between the HSE and the electrode, achieving a high-performance solid-state battery. As a result, the cell with such a structural design could deliver an initial capacity of 125 mAh/g at a current density of 0.1 C for the fabricated Li//LiFePO4 cell at room temperature (Chen X. et al., 2019).
To further solve the interfacial issue, isostatic processing, in which equal pressure is applied in all directions on the substrate, has been recognized as a promising method. By using this approach, good mechanical contact, as well as the enhancement of ionic transference, could be assured. For example, Afyon et al. (2019) utilized garnet-type solid electrolytes of LLZO for assembling solid-state battery cells and composite electrodes as well. Generally, LLZO was prepared based on a modified sol-gel synthesis-combustion approach. Sub-micron-sized particles were obtained at 650°C (Afyon et al., 2019). The resultant LLZO electrolyte pellets had relative densities of ∼87% and ionic conductivities of ∼0.5 × 10–3 S/cm at room temperature. A composite of antimony nanoparticles, carbon black, LLZO electrolyte powder, and PVDF was tape-casted onto sintered thin LLZO pellets with a thickness of 300–400 μm. The dried solid-state electrodes were then pressed at a force of 1000 kN onto the solid electrolyte to ensure good contact at the interface of electrode/LLZO. Additionally, this method is scalable, as shown in Figure 4.
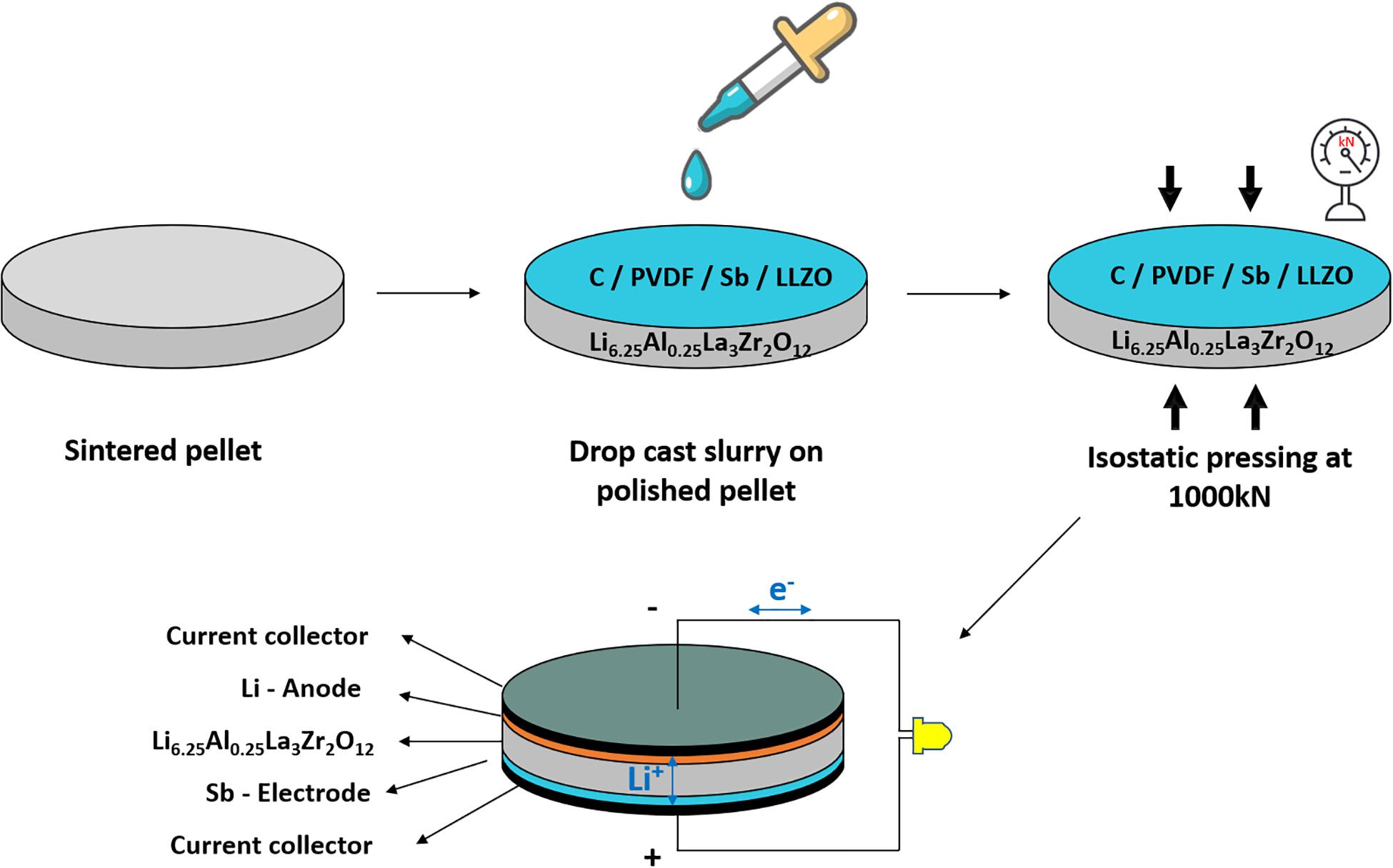
Figure 4. Schematic image showing the deposition of the electrode to prepare an all-solid-state battery. Reprinted with permission from reference Afyon et al. (2019). Copyright 2019 Royal Society of Chemistry.
Electrospinning
Electrospinning has been recognized as one of the promising approaches to produce functional one-dimensional (1D) nanomaterials, which provide unique properties including high surface-to-volume ratio, good flexibilities, and large porosity (Zhu et al., 2015, 2016a, e; Li et al., 2018a, b; Zhu J. et al., 2019). The open porous structure and nanosized fibers may provide good ion transport path, benefiting the electrochemical performance of the prepared cells. The basic setup of electrospinning is shown in Figure 5A, which includes a syringe, a syringe pump, a high-voltage supplier, and a collector (Zhu et al., 2016c). Zhu et al. designed and prepared a PEO-based HSE with 1D ceramic LLTO nanofibers, showing a good ionic conductivity of 2.4 × 10–4 S/cm at room temperature (Zhu P. et al., 2019). Briefly, LLTO precursor nanofibers were first fabricated via electrospinning using poly(vinylpyrrolidone) as the polymer matrix followed by heat-treatment. The effect of treatment temperature on the morphology of the prepared LLTO has also been studied. As can be seen from Figures 5B,C, the average diameter of the LLTO nanofibers treated at 800°C is around 110 nm with a good fibrous shape. Figure 5D shows the TEM images of the individual LLTO nanofibers. The high-resolution TEM image indicates a lattice spacing of ∼0.27 nm, as shown in Figure 5E, which is ascribed to the (110) plane of LLTO. The resultant LLTO calcined at 800°C was mixed with PEO to prepare the LLTO/PEO solid-state composite electrolyte using acetonitrile as the casting solvent. It exhibits a high ionic conductivity of 2.4 × 10–4 S/cm at room temperature and a large electrochemical stability window of up to 5.0 V vs. Li/Li+.
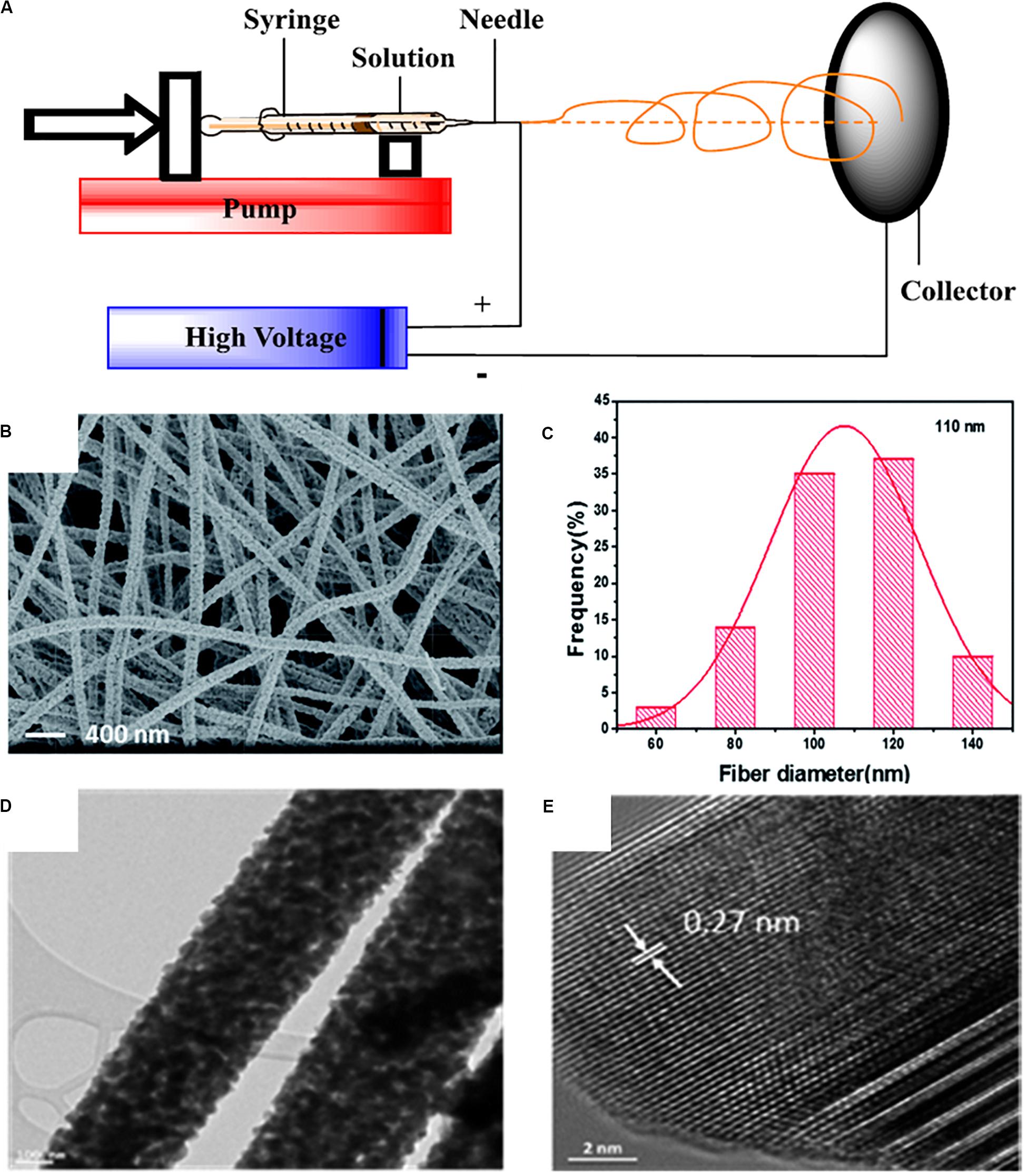
Figure 5. (A) Schematic illustration of the setup of electrospinning. Reprinted with permission from reference Zhu et al. (2016c). Copyright 2016 Elsevier. (B) SEM images of the prepared LLTO nanofibers and (C) the corresponding diameter distribution. (D) Low- and (E) high-resolution TEM images of the LLTO nanofibers. Reprinted with permission from reference Zhu et al. (2018a). Copyright 2018 Royal Society of Chemistry.
Spray Coating
Spray coating is a facile and scalable processing method to manufacture composite electrolyte films. Pandian et al. (2018) used aqueous spray coating to fabricate composite electrolyte films with high ceramic loadings (>50 vol% ceramic). In the spray coating process, slurry containing the polymer electrolyte and ceramic particles are delivered through the spray nozzle onto the substrate and forms a thin layer of composite. The thin layer is allowed to dry on the heated substrate before the next layer is delivered. The spraying-drying procedure is repeated until the desired thickness is reached. This method creates a film that is large, crack-free and three-dimensionally uniform, even with very high ceramic loadings. The thickness can be precisely controlled from a few micrometers to hundreds of micrometers. Comparatively conventional casting methods lead to crack formation when the ceramic loadings are high, due to segregation of the ceramics during drying. Furthermore, using aqueous slurry is a green process that is less expensive, more environmentally friendly which makes it attractive for commercialization.
In situ Polymerization
In situ polymerization to prepare HSEs provides several advantages including solvent-free preparation and improved contact with electrodes (Zhou et al., 2015, 2017; Suk et al., 2016; Chen et al., 2018a; Duan et al., 2018). Generally, a precursor containing a curable monomer, an initiator, lithium salts, and other additives is cured under certain conditions (i.e., thermal, UV irradiation, etc.) to synthesize HSEs. For example, Zhou et al. synthesized a solid-state electrolyte based on nitrile materials for achieving high-performance LIBs. Figure 6 exhibits the synthesis route. Briefly, in situ polymerizing the cyanoethyl polyvinyl alcohol (PVA-CN) was performed to prepare this hierarchical structure, which was dissolved in succinonitrile (SN) and then mixed with two lithium salts (LiTFSI and LiPF6) at a weight ratio of 5:1 to generate a slurry. The prepared slurry was further poured into a PAN nanofiber membrane and put into a cell. After heating the cell at 70°C for 6 h, a crosslinked 3D framework structure filled by SN−based solid electrolyte could be fabricated. In this work, LiPF6 was used as an initiator for the polymerization of PVA-CN, and LiTFSI could provide charge carrier due to its low dissociation and large anionic radius. A conductivity of 4.49 × 10–4 S/cm could be achieved. The assembled cells used LiFePO4 and lithium foil as the cathode and the anode, respectively, with the above-mentioned solid thin film as the electrolyte. An initial discharge capacity of 147 mAh/g for such cells achieved at a current density of 0.1 C. A capacity retention of 97% was obtained even at the 100th cycle, indicating a relatively stable electrochemical performance (Zhou et al., 2015).
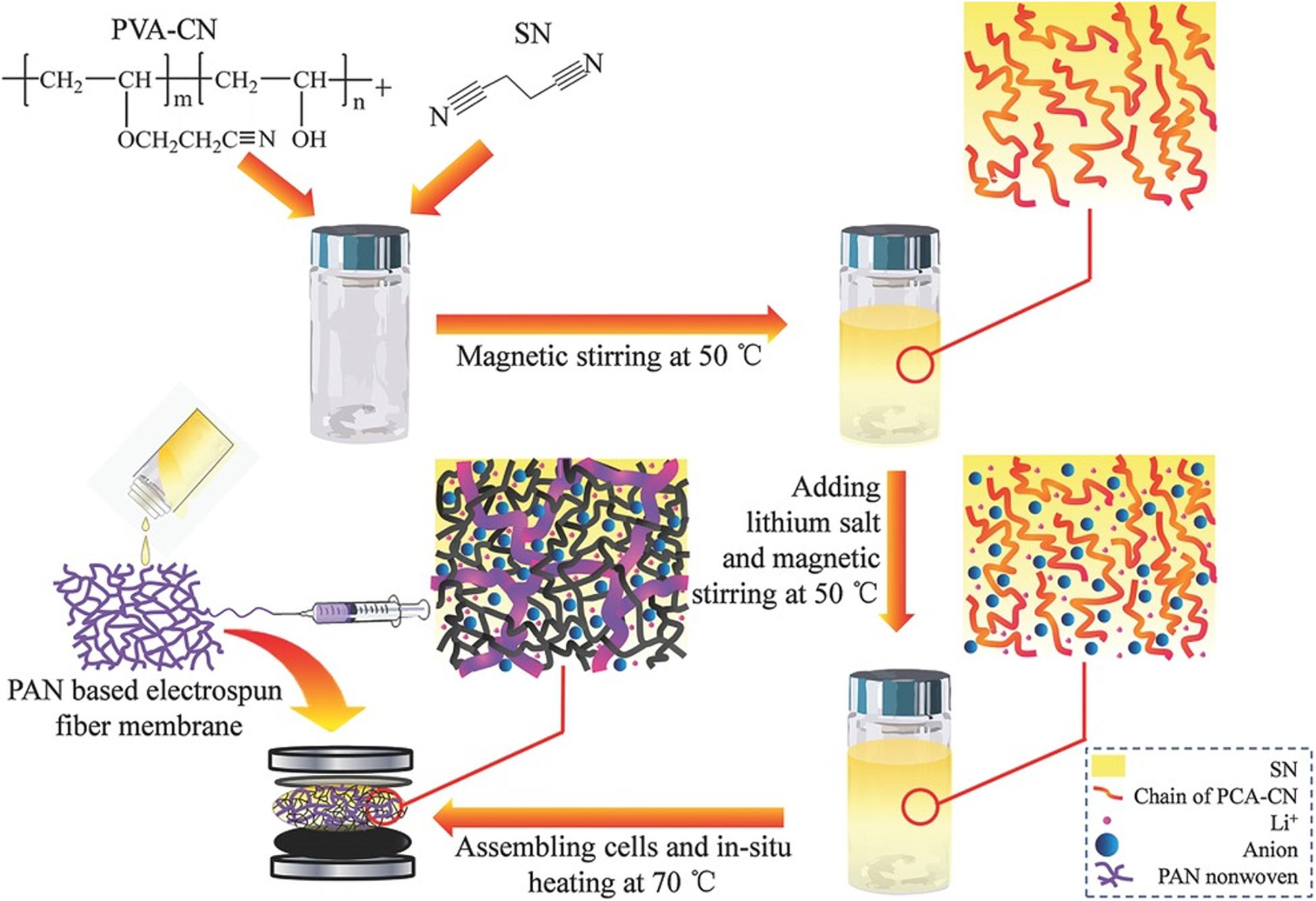
Figure 6. Schematic image showing the procedure for the in situ synthesis of solid-state electrolyte based on nitrile materials. Reference Zhou et al. (2015). Copyright 2015 John Wiley and Sons.
Hybrid Methods
The above approaches can be combined to prepare unique composite solid electrolytes that take advantage of the benefits of each technique (Yan et al., 2019, 2020). For instance, Yan et al. used electrospinning to manufacture Li6.28La3Al0.24Zr2O12 (LLAZO) nanofibers, which were then modified with silane functional groups (denoted as s@LLAZO) with different reaction times. The goal was to investigate the effect of silane functionalization on eliminating agglomeration of LLAZO during the casting process. In this study, a new type of hybrid solid electrolyte composed of s@LLAZO nanofibers and poly(ethylene glycol) diacrylate (PEGDA) was prepared and developed (Figure 7). As a result, the silane coupling agent could eliminate the agglomeration effect, which ensured high ionic conductivity and large lithium transference number of the s@LLZAO-PEGDA electrolyte, demonstrating a wider electrochemical stability window and better cycling stability for the cells with such electrolytes. LiNi1/3Mn1/3Co1/3O2 was also fabricated to be used as the cathode material for the cells. The high voltage cells with an HSE of s@LLZAO-PEGDA with LiNi1/3Mn1/3Co1/3O2 exhibited a stable capacity of 110 mAh/g after 250 cycles at a current density of 0.5 C at room temperature and with a high capacity retention of 97%. The excellent capacity retention was due to the good ionic conductivity of 3.9 × 10–4 S/cm and large transference number of 0.61 for the prepared hybrid electrolytes (Yan et al., 2020).
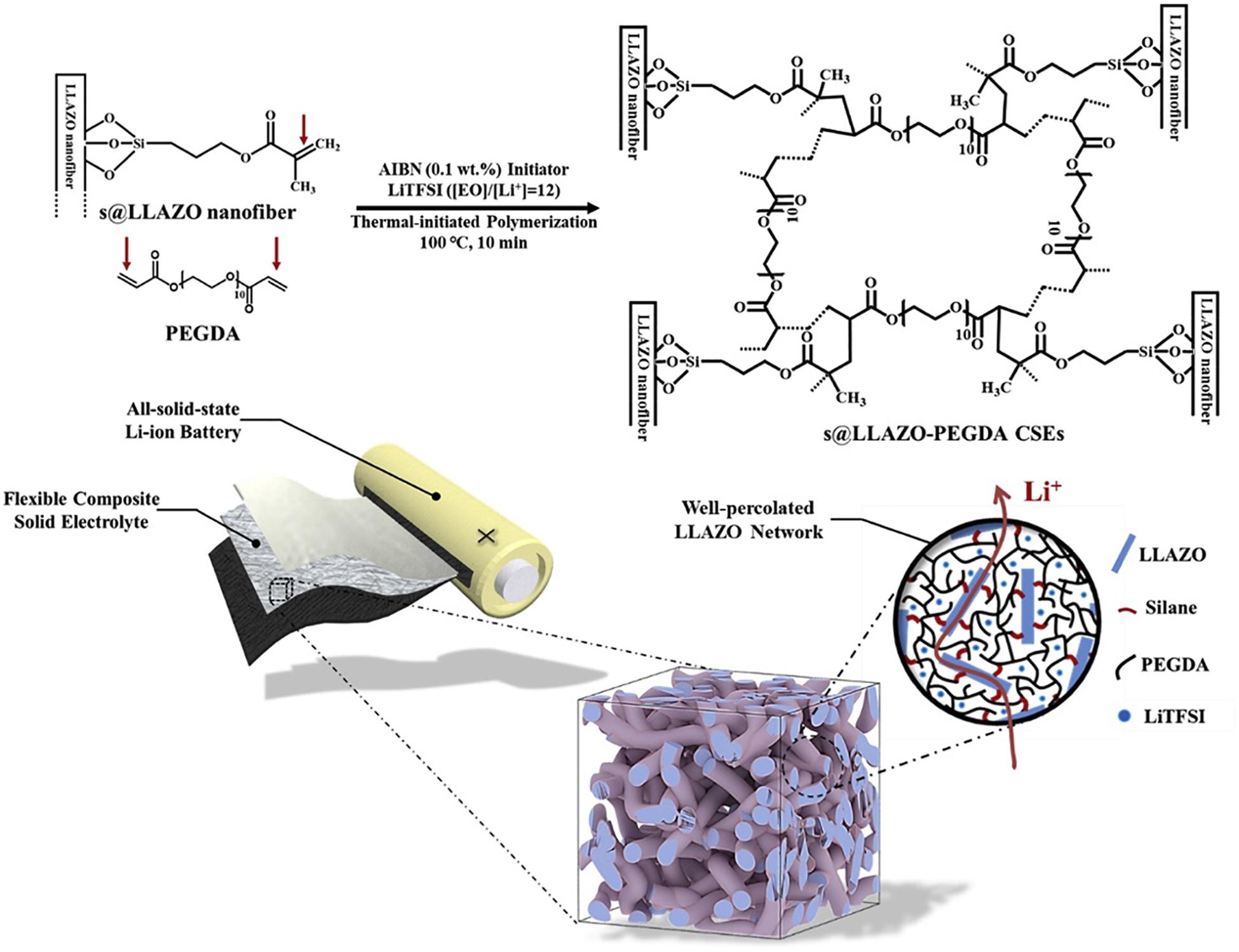
Figure 7. Schematic illustration of the synthesis process of s@LLAZO-PEGDA composite solid electrolytes with percolated s@LLAZO network within the composite electrolyte. Reprinted with permission from reference Yan et al. (2020). Copyright 2020 Elsevier.
In another example, Palmer et al. (2020) used a novel spray coating – partially sintering method to create a three-dimensionally interconnected ceramic network using a doped LATP ceramic (LIGCGTM). The ceramic network is then backfilled with a crosslinkable PEO-based polymer electrolyte to form the composite. The resulting composite has a very high ceramic loading (77 wt% or 61 vol%) and an ionic conductivity of 3.5 × 10–5 S/cm at 20°C with an activation energy of 0.43 eV. X-ray tomography verified that the composite is a bicontinuous structure with a connected ceramic phase and a connected polymer phase. The main ion transport pathway is through the ceramic network as the ionic conductivity scales proportionally with the volume fraction of the ceramic network. Owing to the interconnected structure of the ceramic and the polymer, the composite electrolyte exhibits much improved mechanical strength compared to the neat polymer electrolyte and decreased brittleness compared to the neat ceramic electrolyte.
Current Challenges and Perspectives
In this review, we have highlighted three types of solid electrolytes; inorganic, polymer, and HSEs. HSEs can be the most promising solid-state electrolytes for LIBs, attributed to their good mechanical properties, excellent processability, low flammability, wide electrochemical stability window, and ease of scalability. In particular, HSEs incorporating different matrix components and fillers, common processing methods, and ion transport mechanisms were discussed in details. In recent years, the performance of LIBs with composite solid electrolytes has been greatly enhanced. The improved properties of HSEs have come close to being adequate for their potential use in LIBs.
Looking to the future, solid-state LIBs may become even more important in personal portable electronics, electric vehicles, and stationary storage devices. To promote the practical application and commercialization of solid-state batteries, the performance of HSEs for LIBs still needs to be improved. Current issues of HSEs include unsatisfactory ionic conductivity (<10–3 S/cm at room temperature), poor interfacial stability, and high interfacial resistance, which greatly restrict the performance of these electrolytes.
Inorganic-type electrolytes allow for the realization of high power density LIBs, attributed to the high ionic conductivity of these electrolytes. However, inorganic-type electrolytes are brittle, have low adhesion to electrodes, and are difficult to manufacture, especially using current battery manufacturing processes, which limits their practical application. Polymer electrolytes are easy to process, are flexible and have good adhesion to electrodes, while their ionic conductivity is far from satisfactory. Therefore, HSEs developed by the mixing of an inorganic filler with a polymer matrix have attracted much attention. Many HSEs demonstrate better ionic conductivity than that of the polymer electrolyte matrix itself. Nonetheless, the mixing of filler and matrix still needs improvement to increase ionic conductivity of composite electrolytes. Constructing a good dispersion method and strengthening the interaction between filler and the polymer may contribute to the improvement of ionic conductivity. Another factor is that insufficient investigation into the ion conduction mechanisms in HSEs is, restricting well-articulated development of novel fillers, polymers, and their composite. Continuous Li-ion transport channels between interconnected polymer matrix and filler are also in need of an in-depth study. In addition, the electrolyte/electrode interface has a great impact on the internal resistance of a battery. Significant advancements need to be made to improve the interfacial contact between the electrolyte and electrodes in order to achieve high performance of solid-state batteries.
Our perspective on the future research directions of HSEs for solid-state LIBs is to develop safe, highly stable, and conductive composite polymer electrolytes. A fundamental understanding of the mechanisms of ion transport, the interfacial properties between the polymer and filler, and between the electrolyte and electrodes may facilitate further molecular design to address these issues.
The future focus of HSE development should include:
(i) Understand the underlying mechanisms of ion transport in HSEs. A variety of proposed mechanisms of ion transport through an HSE are discussed in section “Ion conduction mechanisms in hybrid solid electrolytes” in detail. As discussed, the dominant mechanism depends on several factors, including the type of filler (inert or active) and filler loading. By developing a deeper understanding of each mechanism and the molecular interactions that cause transition from one mechanism to another is instrumental in the rational design of new HSE materials. Designing HSE materials on the molecular level may allow for greater interactions between polymer and filler that may be essential in increasing the ionic conductivity of the HSEs. Computational simulations would assist in the investigation of ion transport mechanisms and the polymer-filler interactions.
(ii) Improve the interfacial property between the polymer matrix and fillers, which is pivotal in obtaining a solid-state electrolyte with high conductivity and processability. The polymer-filler interface can influence the crystallinity and morphology of the polymer chain and provide a path for ion transportation through the HSE. Furthermore, with active fillers, controlling the interface between the active ceramic particle and the polymer is crucial for marrying the high conductivity of conductive ceramics with the flexibility of a polymer. Treatment and functionalization of filler’s surfaces might provide significant enhancement of interfacial transport. A greater understanding of the structure-property relationships that control the polymer/filler interface may allow for the rational design of materials to create a stable interface. The nanostructure of HSEs could be investigated by TEM. In addition, typical polymer composite characterization techniques including, differential scanning calorimetry, rheology, broadband dielectric spectroscopy, and X-ray diffraction, would assist in gaining an in-depth understanding of the interfacial behavior between matrix and filler.
(iii) The design and synthesis of novel polymer matrices would open new avenues for improving the performance of HSEs. The optimal combination of matrix and filler would allow for high ionic conductivity and stability to be achieved. Novel polymer electrolytes designed specifically for a composite system is a field that researchers have rarely explored. Single ion conducting polymers, namely polyelectrolytes where the anions are covalently tethered to the polymer backbone and the lithium ions are the only mobile species, shows potential of increasing the lithium transference number. Considerable improvements in battery performance has been achieved by the introduction of single ion conducting polymers and the optimization of the macromolecular architecture via random and block copolymerization with various monomers. Therefore, a promising direction for HSE development is the use of a single ion conducting polymer as the matrix material.
(iv) Design of HSEs that are compatible with next-generation high energy density anodes and cathodes. In commercial LIBs, some commonly used cathode materials are lithium cobalt oxide, lithium iron phosphate (LFP), and lithium nickel manganese cobalt (NMC). The anode typically consists of graphite, but new anode materials of a significantly higher energy density are currently under investigation, including lithium metal. Both the anode and cathode require the solid electrolyte to exhibit a certain amount of flexibility and adhesion to ensure low interfacial resistance at the electrode/electrolyte interface. While the use of a lithium metal anode requires a strong solid electrolyte to resist puncture by lithium dendrites. HSEs can provide both requirements of flexibility and mechanical strength that opens the avenue for the use of a lithium metal anode that is not realizable with liquid electrolytes.
(v) Advanced simulations and computations would provide insight and a path to an in-depth understanding of ion transport, the interaction between polymer and filler, and interactions between electrode and electrolyte. By taking advantage of advanced computing simulations and calculations, researchers will be able to make informed decisions on new materials design and selection, and the potential combinations of fillers and polymers that may lead to improved performance of HSEs.
Author Contributions
LH wrote the manuscript with support from ML, JZ, TL, ZZ, XT, C-TH, PC, and XC. AS and TS helped supervise the project. All authors contributed to the article and approved the submitted version.
Funding
This research was supported by the U.S. Department of Energy, Office of Science, Basic Energy Sciences, Materials Sciences and Engineering Division.
Conflict of Interest
The authors declare that the research was conducted in the absence of any commercial or financial relationships that could be construed as a potential conflict of interest.
Acknowledgments
This manuscript has been authored by UT-Battelle, LLC under Contract No. DE-AC05-00OR22725 with the U.S. Department of Energy. The United States Government retains and the publisher, by accepting the article for publication, acknowledges that the United States Government retains a non-exclusive, paid-up, irrevocable, world-wide license to publish or reproduce the published form of this manuscript, or allow others to do so, for United States Government purposes. The Department of Energy will provide public access to these results of federally sponsored research in accordance with the DOE Public Access Plan (http://energy.gov/downloads/doe-public-access-plan). XC acknowledges support from U.S. Department of Energy (DOE), Office of Energy Efficiency and Renewable Energy for the Vehicle Technologies Office’s Advanced Battery Materials Research Program as well as Office of Science, Basic Energy Sciences, Materials Sciences and Engineering Division.
References
Adebahr, J., Byrne, N., Forsyth, M., MacFarlane, D. R., and Jacobsson, P. (2003). Enhancement of ion dynamics in PMMA-based gels with addition of TiO2 nano-particles. Electrochim. Acta 48, 2099–2103. doi: 10.1016/S0013-4686(03)00191-9
Afyon, S., Kravchyk, K. V., Wang, S., Broek, J. V. D., Hänsel, C., Kovalenko, M. V., et al. (2019). Building better all-solid-state batteries with Li-garnet solid electrolytes and metalloid anodes. J. Mater. Chem. A 7, 21299–21308.
Agapov, A. L., and Sokolov, A. P. (2011). Decoupling ionic conductivity from structural relaxation: a way to solid polymer electrolytes? Macromolecules 44, 4410–4414. doi: 10.1021/ma2001096
Ahmed, F., Choi, I., Rahman, M. M., Jang, H., Ryu, T., Yoon, S., et al. (2019). Remarkable conductivity of a self-healing single-Ion conducting polymer electrolyte, poly(ethylene-co-acrylic lithium (fluoro sulfonyl)imide), for all-solid-state li-Ion batteries. ACS Appl. Mater. Interf. 11, 34930–34938. doi: 10.1021/acsami.9b10474
Aono, H. (1990). Ionic conductivity of solid electrolytes based on lithium titanium phosphate. J. Electrochem. Soc. 137:1023. doi: 10.1149/1.2086597
Appetecchi, G. B., Croce, F., Persi, L., Ronci, F., and Scrosati, B. (2000). Transport and interfacial properties of composite polymer electrolytes. Electrochim. Acta 45, 1481–1490. doi: 10.1016/S0013-4686(99)00363-1
Appetecchi, G. B., and Passerini, S. (2000). PEO-carbon composite lithium polymer electrolyte. Electrochim. Acta 45, 2139–2145. doi: 10.1016/S0013-4686(99)00437-5
Berthier, C., Gorecki, W., Minier, M., Armand, M. B., Chabagno, J. M., and Rigaud, P. (1983). Microscopic investigation of ionic conductivity in alkali metal salts-poly(ethylene oxide) adducts. Solid State Ionics 11, 91–95. doi: 10.1016/0167-2738(83)90068-1
Bhattacharyya, R., Key, B., Chen, H., Best, A. S., Hollenkamp, A. F., and Grey, C. P. (2010). In situ NMR observation of the formation of metallic lithium microstructures in lithium batteries. Nat. Mater. 9, 504–510. doi: 10.1038/nmat2764
Bocharova, V., and Sokolov, A. P. (2020). Perspectives for polymer electrolytes: a view from fundamentals of ionic conductivity. Macromolecules 53, 4141–4157. doi: 10.1021/acs.macromol.9b02742
Bouchet, R., Maria, S., Meziane, R., Aboulaich, A., Lienafa, L., Bonnet, J.-P., et al. (2013). Single-ion BAB triblock copolymers as highly efficient electrolytes for lithium-metal batteries. Nat. Mater. 12, 452–457. doi: 10.1038/nmat3602
Cao, P.-F., Li, B., Yang, G., Zhao, S., Townsend, J., Xing, K., et al. (2020). Elastic Single-Ion conducting polymer electrolytes: toward a versatile approach for intrinsically stretchable functional polymers. Macromolecules 53, 3591–3601. doi: 10.1021/acs.macromol.9b02683
Cao, P.-F., Wojnarowska, Z., Hong, T., Carroll, B., Li, B., Feng, H., et al. (2017). A star-shaped single lithium-ion conducting copolymer by grafting a POSS nanoparticle. Polymer 124, 117–127. doi: 10.1016/j.polymer.2017.07.052
Chen, S., Wang, J., Zhang, Z., Wu, L., Yao, L., Wei, Z., et al. (2018a). In-situ preparation of poly(ethylene oxide)/Li3PS4 hybrid polymer electrolyte with good nanofiller distribution for rechargeable solid-state lithium batteries. J. Power Sources 387, 72–80. doi: 10.1016/j.jpowsour.2018.03.016
Chen, S., Wen, K., Fan, J., Bando, Y., and Golberg, D. (2018b). Progress and future prospects of high-voltage and high-safety electrolytes in advanced lithium batteries: from liquid to solid electrolytes. J. Mater. Chem. A 6, 11631–11663. doi: 10.1039/C8TA03358G
Chen, S., Xie, D., Liu, G., Mwizerwa, J. P., Zhang, Q., Zhao, Y., et al. (2018c). Sulfide solid electrolytes for all-solid-state lithium batteries: structure, conductivity, stability and application. Energy Storage Mater. 14, 58–74. doi: 10.1016/j.ensm.2018.02.020
Chen, X., He, W., Ding, L.-X., Wang, S., and Wang, H. (2019). Enhancing interfacial contact in all solid state batteries with a cathode-supported solid electrolyte membrane framework. Energy Environ. Sci. 12, 938–944. doi: 10.1039/C8EE02617C
Chen, X., and Vereecken, P. M. (2019). Solid and solid-like composite electrolyte for lithium ion batteries: engineering the Ion conductivity at interfaces. Adv. Mater. Interf. 6:1800899. doi: 10.1002/admi.201800899
Chen, X. C., Sacci, R. L., Osti, N. C., Tyagi, M., Wang, Y., Palmer, M. J., et al. (2019). Study of segmental dynamics and ion transport in polymer–ceramic composite electrolytes by quasi-elastic neutron scattering. Mol. Syst. Design Eng. 4, 379–385. doi: 10.1039/C8ME00113H
Choi, J.-H., Lee, C.-H., Yu, J.-H., Doh, C.-H., and Lee, S.-M. (2015). Enhancement of ionic conductivity of composite membranes for all-solid-state lithium rechargeable batteries incorporating tetragonal Li7La3Zr2O12 into a polyethylene oxide matrix. J. Power Sources 274, 458–463. doi: 10.1016/j.jpowsour.2014.10.078
Cohen, M. H., and Turnbull, D. (1959). Molecular transport in liquids and glasses. J. Chem. Phys. 31, 1164–1169. doi: 10.1063/1.1730566
Croce, F., Appetecchi, G. B., Persi, L., and Scrosati, B. (1998). Nanocomposite polymer electrolytes for lithium batteries. Nature 394, 456–458. doi: 10.1038/28818
Croce, F., Curini, R., Martinelli, A., Persi, L., Ronci, F., Scrosati, B., et al. (1999). Physical and chemical properties of nanocomposite polymer electrolytes. J. Phys. Chem. B 103, 10632–10638. doi: 10.1021/jp992307u
Croce, F., Persi, L., Ronci, F., and Scrosati, B. (2000). Nanocomposite polymer electrolytes and their impact on the lithium battery technology. Solid State Ionics 135, 47–52. doi: 10.1016/S0167-2738(00)00329-5
Croce, F., Persi, L., Scrosati, B., Serraino-Fiory, F., Plichta, E., and Hendrickson, M. A. (2001). Role of the ceramic fillers in enhancing the transport properties of composite polymer electrolytes. Electrochim. Acta 46, 2457–2461. doi: 10.1016/S0013-4686(01)00458-3
Croce, F., Settimi, L., and Scrosati, B. (2006). Superacid ZrO2-added, composite polymer electrolytes with improved transport properties. Electrochem. Commun. 8, 364–368. doi: 10.1016/j.elecom.2005.12.002
Das, S., and Ghosh, A. (2015). Ion conduction and relaxation in PEO-LiTFSI-Al2O3 polymer nanocomposite electrolytes. J. Appl. Phys. 117:174103. doi: 10.1063/1.4919721
Dirican, M., Yan, C., Zhu, P., and Zhang, X. (2019). Composite solid electrolytes for all-solid-state lithium batteries. Mater. Sci. Eng. R Rep. 136, 27–46. doi: 10.1016/j.mser.2018.10.004
Duan, H., Yin, Y.-X., Zeng, X.-X., Li, J.-Y., Shi, J.-L., Shi, Y., et al. (2018). In-situ plasticized polymer electrolyte with double-network for flexible solid-state lithium-metal batteries. Energy Storage Mater. 10, 85–91. doi: 10.1016/j.ensm.2017.06.017
Evans, J., Vincent, C. A., and Bruce, P. G. (1987). Electrochemical measurement of transference numbers in polymer electrolytes. Polymer 28, 2324–2328. doi: 10.1016/0032-3861(87)90394-6
Famprikis, T., Canepa, P., Dawson, J. A., Islam, M. S., and Masquelier, C. (2019). Fundamentals of inorganic solid-state electrolytes for batteries. Nat. Mater. 18, 1278–1291. doi: 10.1038/s41563-019-0431-3
Fan, J. (1997). Composite electrolytes prepared from fumed silica, polyethylene oxide oligomers, and lithium salts. J. Electrochem. Soc. 144:399. doi: 10.1149/1.1837423
Fan, L., Nan, C.-W., and Zhao, S. (2003). Effect of modified SiO2 on the properties of PEO-based polymer electrolytes. Solid State Ionics 164, 81–86. doi: 10.1016/j.ssi.2003.08.004
Fan, L., Wei, S., Li, S., Li, Q., and Lu, Y. (2018). Recent progress of the solid-state electrolytes for high-energy metal-based batteries. Adv. Energy Mater. 8:1702657. doi: 10.1002/aenm.201702657
Florjañczyk, Z., Zygadło-Monikowska, E., Affek, A., Tomaszewska, A., Łasińska, A., Marzantowicz, M., et al. (2005). Polymer electrolytes based on acrylonitrile–butyl acrylate copolymers and lithium bis(trifluoromethanesulfone)imide. Solid State Ionics 176, 2123–2128. doi: 10.1016/j.ssi.2004.08.046
Florjañczyk, Z., Zygadło-Monikowska, E., Wieczorek, W., Ryszawy, A., Tomaszewska, A., Fredman, K., et al. (2004). Polymer-in-salt electrolytes based on acrylonitrile/butyl acrylate copolymers and lithium salts. J. Phys. Chem. B 108, 14907–14914. doi: 10.1021/jp049195d
Forsyth, M., Sun, J., Macfarlane, D. R., and Hill, A. J. (2000). Compositional dependence of free volume in PAN/LiCF3SO3 polymer-in-salt electrolytes and the effect on ionic conductivity. J. Polym. Sci. Part B Polym. Phys. 38, 341–350. doi: 10.1002/(sici)1099-0488(20000115)38:2<341::Aid-polb6<3.0.Co;2-s
Fu, K., Li, Y., Dirican, M., Chen, C., Lu, Y., Zhu, J., et al. (2014). Sulfur gradient-distributed CNF composite: a self-inhibiting cathode for binder-free lithium–sulfur batteries. Chem. Commun. 50, 10277–10280. doi: 10.1039/C4CC04970E
Fullerton-Shirey, S. K., and Maranas, J. K. (2009). Effect of LiClO4 on the structure and mobility of PEO-based solid polymer electrolytes. Macromolecules 42, 2142–2156. doi: 10.1021/ma802502u
Ge, Y., Jiang, H., Fu, K., Zhang, C., Zhu, J., Chen, C., et al. (2014). Copper-doped Li4Ti5O12/carbon nanofiber composites as anode for high-performance sodium-ion batteries. J. Power Sources 272, 860–865. doi: 10.1016/j.jpowsour.2014.08.131
Geiger, C. A., Alekseev, E., Lazic, B., Fisch, M., Armbruster, T., Langner, R., et al. (2011). Crystal chemistry and stability of “Li7La3Zr2O12” garnet: a fast lithium-Ion conductor. Inorg. Chem. 50, 1089–1097. doi: 10.1021/ic101914e
Gibbs, J. H., and DiMarzio, E. A. (1958). Nature of the glass transition and the glassy state. J. Chem. Phys. 28, 373–383. doi: 10.1063/1.1744141
Goodenough, J. B., Hong, H. Y. P., and Kafalas, J. A. (1976). Fast Na+-ion transport in skeleton structures. Mater. Res. Bull. 11, 203–220. doi: 10.1016/0025-5408(76)90077-5
Grey, C. P., and Dupré, N. (2004). NMR studies of cathode materials for lithium-Ion rechargeable batteries. Chem. Rev. 104, 4493–4512. doi: 10.1021/cr020734p
Gutmann, V. (1976). Empirical parameters for donor and acceptor properties of solvents. Electrochim. Acta 21, 661–670. doi: 10.1016/0013-4686(76)85034-7
Hanson, B., Pryamitsyn, V., and Ganesan, V. (2013). Mechanisms underlying Ionic mobilities in nanocomposite polymer electrolytes. ACS Macro Lett. 2, 1001–1005. doi: 10.1021/mz400234m
Hsueh, L., and Bennion, D. N. (1971). EMF measurements of sodium activity in sodium amalgam with beta-alumina. J. Electrochem. Soc. 118:1128. doi: 10.1149/1.2408260
Hu, L., Tang, Z., and Zhang, Z. (2007). New composite polymer electrolyte comprising mesoporous lithium aluminate nanosheets and PEO/LiClO4. J. Power Sources 166, 226–232. doi: 10.1016/j.jpowsour.2007.01.028
Ilott, A. J., Trease, N. M., Grey, C. P., and Jerschow, A. (2014). Multinuclear in situ magnetic resonance imaging of electrochemical double-layer capacitors. Nat. Commun. 5:4536. doi: 10.1038/ncomms5536
Inaguma, Y., Liquan, C., Itoh, M., Nakamura, T., Uchida, T., Ikuta, H., et al. (1993). High ionic conductivity in lithium lanthanum titanate. Solid State Commun. 86, 689–693. doi: 10.1016/0038-1098(93)90841-A
Janek, J., and Zeier, W. G. (2016). A solid future for battery development. Nat. Energy 1:16141. doi: 10.1038/nenergy.2016.141
Ju, J., Wang, Y., Chen, B., Ma, J., Dong, S., Chai, J., et al. (2018). Integrated interface strategy toward room temperature solid-state lithium batteries. ACS Appl. Mater. Interf. 10, 13588–13597. doi: 10.1021/acsami.8b02240
Jung, Y.-C., Lee, S.-M., Choi, J.-H., Jang, S. S., and Kim, D.-W. (2015). All solid-state lithium batteries assembled with hybrid solid electrolytes. J. Electrochem. Soc. 162, A704–A710. doi: 10.1149/2.0731504jes
Kamaya, N., Homma, K., Yamakawa, Y., Hirayama, M., Kanno, R., Yonemura, M., et al. (2011). A lithium superionic conductor. Nat. Mater. 10, 682–686. doi: 10.1038/nmat3066
Karan, N. K., Pradhan, D. K., Thomas, R., Natesan, B., and Katiyar, R. S. (2008). Solid polymer electrolytes based on polyethylene oxide and lithium trifluoro- methane sulfonate (PEO–LiCF3SO3): ionic conductivity and dielectric relaxation. Solid State Ionics 179, 689–696. doi: 10.1016/j.ssi.2008.04.034
Khurana, R., Schaefer, J. L., Archer, L. A., and Coates, G. W. (2014). Suppression of lithium dendrite growth using cross-linked polyethylene/Poly(ethylene oxide) electrolytes: a new approach for practical lithium-metal polymer batteries. J. Am. Chem. Soc. 136, 7395–7402. doi: 10.1021/ja502133j
Kim, J. G., Son, B., Mukherjee, S., Schuppert, N., Bates, A., Kwon, O., et al. (2015). A review of lithium and non-lithium based solid state batteries. J. Power Sources 282, 299–322. doi: 10.1016/j.jpowsour.2015.02.054
Kim, J.-K., Scheers, J., Park, T. J., and Kim, Y. (2015). Superior Ion-conducting hybrid solid electrolyte for all-solid-state batteries. ChemSusChem 8, 636–641. doi: 10.1002/cssc.201402969
Kumar, B. (2004). From colloidal to composite electrolytes: properties, peculiarities, and possibilities. J. Power Sources 135, 215–231. doi: 10.1016/j.jpowsour.2004.04.038
Kumar, B., Thomas, D., and Kumar, J. (2009). Space-charge-mediated superionic transport in lithium Ion conducting glass–ceramics. J. Electrochem. Soc. 156:A506. doi: 10.1149/1.3122903
Kummer, J. T., and Neill, W. (1968). Secondary Battery Employing Molten Alkali Metal Reactant. Google Patents US3404035A.
Lagadec, M. F., Zahn, R., and Wood, V. (2019). Characterization and performance evaluation of lithium-ion battery separators. Nat. Energy 4, 16–25. doi: 10.1038/s41560-018-0295-9
Le, H. T. T., Kalubarme, R. S., Ngo, D. T., Jadhav, H. S., and Park, C.-J. (2015). Bi-layer lithium phosphorous oxynitride/aluminium substituted lithium lanthanum titanate as a promising solid electrolyte for long-life rechargeable lithium–oxygen batteries. J. Mater. Chem. A 3, 22421–22431. doi: 10.1039/C5TA06374D
Lee, H., Oh, P., Kim, J., Cha, H., Chae, S., Lee, S., et al. (2019). Advances and prospects of sulfide all-solid-state lithium batteries via one-to-one comparison with conventional liquid lithium Ion batteries. Adv. Mater. 31:1900376. doi: 10.1002/adma.201900376
Lee, Y. M., Seo, J. E., Choi, N.-S., and Park, J.-K. (2005). Influence of tris(pentafluorophenyl) borane as an anion receptor on ionic conductivity of LiClO4-based electrolyte for lithium batteries. Electrochim. Acta 50, 2843–2848. doi: 10.1016/j.electacta.2004.11.058
Lehmann, M. L., Yang, G., Gilmer, D., Han, K. S., Self, E. C., Ruther, R. E., et al. (2019). Tailored crosslinking of Poly(ethylene oxide) enables mechanical robustness and improved sodium-ion conductivity. Energy Storage Mater. 21, 85–96. doi: 10.1016/j.ensm.2019.06.028
Li, D., Chen, L., Wang, T., and Fan, L.-Z. (2018). 3D fiber-network-reinforced bicontinuous composite solid electrolyte for dendrite-free lithium metal batteries. ACS Appl. Mater. Interf. 10, 7069–7078. doi: 10.1021/acsami.7b18123
Li, Y., Zhu, J., Shi, R., Dirican, M., Zhu, P., Yan, C., et al. (2018a). Ultrafine and polar ZrO2-inlaid porous nitrogen-doped carbon nanofiber as efficient polysulfide absorbent for high-performance lithium-sulfur batteries with long lifespan. Chem. Eng. J. 349, 376–387. doi: 10.1016/j.cej.2018.05.074
Li, Y., Zhu, J., Zhu, P., Yan, C., Jia, H., Kiyak, Y., et al. (2018b). Glass fiber separator coated by porous carbon nanofiber derived from immiscible PAN/PMMA for high-performance lithium-sulfur batteries. J. Membr. Sci. 552, 31–42. doi: 10.1016/j.memsci.2018.01.062
Liang, C. C. (1973). Conduction characteristics of the lithium iodide-aluminum oxide solid electrolytes. J. Electrochem. Soc. 120:1289. doi: 10.1149/1.2403248
Lin, D., Liu, W., Liu, Y., Lee, H. R., Hsu, P.-C., Liu, K., et al. (2016). High ionic conductivity of composite solid polymer electrolyte via in situ synthesis of monodispersed SiO2 nanospheres in poly(ethylene oxide). Nano Lett. 16, 459–465. doi: 10.1021/acs.nanolett.5b04117
Liu, W., Liu, N., Sun, J., Hsu, P.-C., Li, Y., Lee, H.-W., et al. (2015). Ionic conductivity enhancement of polymer electrolytes with ceramic nanowire fillers. Nano Lett. 15, 2740–2745. doi: 10.1021/acs.nanolett.5b00600
Liu, X., Li, X., Li, H., and Wu, H. B. (2018). Recent progress of hybrid solid-state electrolytes for lithium batteries. Chem. Eur. J. 24, 18293–18306. doi: 10.1002/chem.201803616
Liu, Y., Lee, J. Y., and Hong, L. (2004). In situ preparation of poly(ethylene oxide)–SiO2 composite polymer electrolytes. J. Power Sources 129, 303–311. doi: 10.1016/j.jpowsour.2003.11.026
Liu, Y., Zhu, Y., and Cui, Y. (2019). Challenges and opportunities towards fast-charging battery materials. Nat. Energy 4, 540–550. doi: 10.1038/s41560-019-0405-3
Lopez, J., Mackanic, D. G., Cui, Y., and Bao, Z. (2019). Designing polymers for advanced battery chemistries. Nat. Rev. Mater. 4, 312–330. doi: 10.1038/s41578-019-0103-6
Luo, L., Li, D., Zang, J., Chen, C., Zhu, J., Qiao, H., et al. (2017). Carbon-coated magnesium ferrite nanofibers for lithium-Ion battery anodes with enhanced cycling performance. Energy Technol. 5, 1364–1372. doi: 10.1002/ente.201600686
Luo, L., Xu, W., Xia, Z., Fei, Y., Zhu, J., Chen, C., et al. (2016). Electrospun ZnO–SnO2 composite nanofibers with enhanced electrochemical performance as lithium-ion anodes. Ceram. Int. 42, 10826–10832. doi: 10.1016/j.ceramint.2016.03.211
Maccallum, J. R., Smith, M. J., and Vincent, C. A. (1984). The effects of radiation-induced crosslinking on the conductance of LiClO4⋅PEO electrolytes. Solid State Ionics 11, 307–312. doi: 10.1016/0167-2738(84)90022-5
Malathi, M. T., and Tamilarasan, K. (2014). Synthesis and characterization of polyethylene oxide based nano composite electrolyte. Sadhana 39, 999–1007.
Manthiram, A., Yu, X., and Wang, S. (2017). Lithium battery chemistries enabled by solid-state electrolytes. Nat. Rev. Mater. 2:16103. doi: 10.1038/natrevmats.2016.103
Marcinek, M., Bac, A., Lipka, P., Zalewska, A., Żukowska, G., Borkowska, R., et al. (2000). Effect of filler surface group on ionic interactions in PEG-LiClO4-Al2O3 composite polyether electrolytes. J. Phys. Chem. B 104, 11088–11093. doi: 10.1021/jp0021493
Mariappan, C. R., Gellert, M., Yada, C., Rosciano, F., and Roling, B. (2012). Grain boundary resistance of fast lithium ion conductors: comparison between a lithium-ion conductive Li–Al–Ti–P–O-type glass ceramic and a Li1.5Al0.5Ge1.5P3O12 ceramic. Electrochem. Commun. 14, 25–28. doi: 10.1016/j.elecom.2011.10.022
Martinez-Juarez, A., Rojo, J. M., Iglesias, J. E., and Sanz, J. (1995). Reversible monoclinic-rhombohedral transformation in LiSn2(PO4)3 with NASICON-type structure. Chem. Mater. 7, 1857–1862. doi: 10.1021/cm00058a016
Matsumoto, K., Kakehashi, M., Ouchi, H., Yuasa, M., and Endo, T. (2016). Synthesis and properties of polycarbosilanes having 5-membered cyclic carbonate groups as solid polymer electrolytes. Macromolecules 49, 9441–9448. doi: 10.1021/acs.macromol.6b01516
Matsumoto, M., Uno, T., Kubo, M., and Itoh, T. (2013). Polymer electrolytes based on polycarbonates and their electrochemical and thermal properties. Ionics 19, 615–622. doi: 10.1007/s11581-012-0796-7
Meabe, L., Huynh, T. V., Mantione, D., Porcarelli, L., Li, C., O’Dell, L. A., et al. (2019). UV-cross-linked poly(ethylene oxide carbonate) as free standing solid polymer electrolyte for lithium batteries. Electrochim. Acta 302, 414–421. doi: 10.1016/j.electacta.2019.02.058
Meabe, L., Lago, N., Rubatat, L., Li, C., Müller, A. J., Sardon, H., et al. (2017). Polycondensation as a versatile synthetic route to aliphatic polycarbonates for solid polymer electrolytes. Electrochim. Acta 237, 259–266. doi: 10.1016/j.electacta.2017.03.217
Meyer, W. H. (1998). Polymer electrolytes for lithium-Ion batteries. Adv. Mater. 10, 439–448. doi: 10.1002/(sici)1521-4095(199804)10:6<439::Aid-adma439<3.0.Co;2-i
Mindemark, J., Sun, B., Törmä, E., and Brandell, D. (2015). High-performance solid polymer electrolytes for lithium batteries operational at ambient temperature. J. Power Sources 298, 166–170. doi: 10.1016/j.jpowsour.2015.08.035
Munichandraiah, N., Scanlon, L. G., Marsh, R. A., Kumar, B., and Sircar, A. K. (1995). Influence of zeolite on electrochemical and physicochemical properties of polyethylene oxide solid electrolyte. J. Appl. Electrochem. 25, 857–863. doi: 10.1007/BF00233905
Muramatsu, H., Hayashi, A., Ohtomo, T., Hama, S., and Tatsumisago, M. (2011). Structural change of Li2S–P2S5 sulfide solid electrolytes in the atmosphere. Solid State Ionics 182, 116–119. doi: 10.1016/j.ssi.2010.10.013
Murugan, R., Ramakumar, S., and Janani, N. (2011). High conductive yttrium doped Li7La3Zr2O12 cubic lithium garnet. Electrochem. Commun. 13, 1373–1375. doi: 10.1016/j.elecom.2011.08.014
Murugan, R., Thangadurai, V., and Weppner, W. (2007). Fast lithium Ion conduction in garnet-Type Li7La3Zr2O12. Angewandte Chem. Int. Ed. 46, 7778–7781. doi: 10.1002/anie.200701144
Nairn, K., Forsyth, M., Every, H., Greville, M., and MacFarlane, D. R. (1996). Polymer-ceramic ion-conducting composites. Solid State Ionics 8, 589–593. doi: 10.1016/0167-2738(96)00212-3
Ong, M. T., Verners, O., Draeger, E. W., van Duin, A. C. T., Lordi, V., and Pask, J. E. (2015). Lithium Ion solvation and diffusion in bulk organic electrolytes from first-principles and classical reactive molecular dynamics. J. Phys. Chem. B 119, 1535–1545. doi: 10.1021/jp508184f
Palmer, M. J., Kalnaus, S., Dixit, M. B., Westover, A. S., Hatzell, K. B., Dudney, N. J., et al. (2020). A three-dimensional interconnected polymer/ceramic composite as a thin film solid electrolyte. Energy Storage Mater. 26, 242–249. doi: 10.1016/j.ensm.2019.12.031
Pandian, A. S., Chen, X. C., Chen, J., Lokitz, B. S., Ruther, R. E., Yang, G., et al. (2018). Facile and scalable fabrication of polymer-ceramic composite electrolyte with high ceramic loadings. J. Power Sources 390, 153–164. doi: 10.1016/j.jpowsour.2018.04.006
Peng, J., Xiao, Y., Clarkson, D. A., Greenbaum, S. G., Zawodzinski, T. A., and Chen, X. C. (2020). A Nuclear magnetic resonance study of cation and anion dynamics in polymer–ceramic composite solid electrolytes. ACS Appl. Polym. Mater. 2, 1180–1189. doi: 10.1021/acsapm.9b01068
Pomerantseva, E., Bonaccorso, F., Feng, X., Cui, Y., and Gogotsi, Y. (2019). Energy storage: the future enabled by nanomaterials. Science 366:eaan8285. doi: 10.1126/science.aan8285
Porcarelli, L., Gerbaldi, C., Bella, F., and Nair, J. R. (2016). Super soft all-ethylene oxide polymer electrolyte for safe all-solid lithium batteries. Sci. Rep. 6:19892. doi: 10.1038/srep19892
Pożyczka, K., Marzantowicz, M., Dygas, J. R., and Krok, F. (2017). IONIC CONDUCTIVITY AND LITHIUM TRANSFERENCE NUMBER OF POLY(ETHYLENE OXIDE):LiTFSI SYSTEM. Electrochim. Acta 227, 127–135. doi: 10.1016/j.electacta.2016.12.172
Przyluski, J., Siekierski, M., and Wieczorek, W. (1995). Effective medium theory in studies of conductivity of composite polymeric electrolytes. Electrochim. Acta 40, 2101–2108. doi: 10.1016/0013-4686(95)00147-7
Quartarone, E., Mustarelli, P., and Magistris, A. (1998). PEO-based composite polymer electrolytes. Solid State Ionics 110, 1–14. doi: 10.1016/S0167-2738(98)00114-3
Ramesh, S., Winie, T., and Arof, A. K. (2007). Investigation of mechanical properties of polyvinyl chloride–polyethylene oxide (PVC–PEO) based polymer electrolytes for lithium polymer cells. Eur. Polym. J. 43, 1963–1968. doi: 10.1016/j.eurpolymj.2007.02.006
Roman, H. E. (1990). A continuum percolation model for dispersed ionic conductors. J. Phys. Condensed Matter 2, 3909–3917. doi: 10.1088/0953-8984/2/17/002
Saunier, J., Chaix, N., Alloin, F., Belières, J. P., and Sanchez, J. Y. (2002). Electrochemical study of polymethacrylonitrile electrolytes: conductivity study of polymer/salt complexes and plasticized polymer electrolytes. Part I. Electrochim. Acta 47, 1321–1326. doi: 10.1016/S0013-4686(01)00852-0
Senthil Kumar, R., Raja, M., Anbu Kulandainathan, M., and Manuel Stephan, A. (2014). Metal organic framework-laden composite polymer electrolytes for efficient and durable all-solid-state-lithium batteries. RSC Adv. 4, 26171–26175. doi: 10.1039/C4RA03147D
Shim, J., Kim, D.-G., Lee, J. H., Baik, J. H., and Lee, J.-C. (2014). Synthesis and properties of organic/inorganic hybrid branched-graft copolymers and their application to solid-state electrolytes for high-temperature lithium-ion batteries. Polym. Chem. 5, 3432–3442. doi: 10.1039/C4PY00123K
Soo, P. P. (1999). Rubbery block copolymer electrolytes for solid-state rechargeable lithium batteries. J. Electrochem. Soc. 146:32. doi: 10.1149/1.1391560
Spiegel, E. F., Adamic, K. J., Williams, B. D., and Sammells, A. F. (2000). Solvation of lithium salts within single-phase dimethyl siloxane bisphenol-A carbonate block copolymer. Polymer 41, 3365–3369. doi: 10.1016/S0032-3861(99)00535-2
Suk, J., Lee, Y. H., Kim, D. Y., Kim, D. W., Cho, S. Y., Kim, J. M., et al. (2016). Semi-interpenetrating solid polymer electrolyte based on thiol-ene cross-linker for all-solid-state lithium batteries. J. Power Sources 334, 154–161. doi: 10.1016/j.jpowsour.2016.10.008
Sun, C., Liu, J., Gong, Y., Wilkinson, D. P., and Zhang, J. (2017). Recent advances in all-solid-state rechargeable lithium batteries. Nano Energy 33, 363–386. doi: 10.1016/j.nanoen.2017.01.028
Tan, R., Gao, R., Zhao, Y., Zhang, M., Xu, J., Yang, J., et al. (2016). Novel organic–inorganic hybrid electrolyte to enable LiFePO4 Quasi-solid-state Li-Ion batteries performed highly around room temperature. ACS Appl. Mater. Interf. 8, 31273–31280. doi: 10.1021/acsami.6b09008
Tao, X., Liu, Y., Liu, W., Zhou, G., Zhao, J., Lin, D., et al. (2017). Solid-state lithium–sulfur batteries operated at 37 °C with composites of nanostructured Li7La3Zr2O12/Carbon foam and polymer. Nano Lett. 17, 2967–2972. doi: 10.1021/acs.nanolett.7b00221
Thangadurai, V., and Weppner, W. (2006). Recent progress in solid oxide and lithium ion conducting electrolytes research. Ionics 12, 81–92. doi: 10.1007/s11581-006-0013-7
Tominaga, Y., Asai, S., Sumita, M., Panero, S., and Scrosati, B. (2005). Fast ionic conduction in PEO-based composite electrolyte filled with ionic liquid-modified mesoporous silica. Electrochem. Solid State Lett. 8:A22. doi: 10.1149/1.1828354
Tominaga, Y., and Endo, M. (2013). Ion-conductive properties of polyether-based composite electrolytes filled with mesoporous silica, alumina and titania. Electrochim. Acta 113, 361–365. doi: 10.1016/j.electacta.2013.09.117
Varzi, A., Raccichini, R., Passerini, S., and Scrosati, B. (2016). Challenges and prospects of the role of solid electrolytes in the revitalization of lithium metal batteries. J. Mater. Chem. A 4, 17251–17259. doi: 10.1039/C6TA07384K
Wang, A., Xu, H., Zhou, Q., Liu, X., Li, Z., Gao, R., et al. (2016). A new all-solid-state hyperbranched star polymer electrolyte for lithium Ion batteries: synthesis and electrochemical properties. Electrochim. Acta 212, 372–379. doi: 10.1016/j.electacta.2016.07.003
Wang, T., Zhang, R., Wu, Y., Zhu, G., Hu, C., Wen, J., et al. (2020). Engineering a flexible and mechanically strong composite electrolyte for solid-state lithium batteries. J. Energy Chem. 46, 187–190. doi: 10.1016/j.jechem.2019.10.010
Wang, X., Kerr, R., Chen, F., Goujon, N., Pringle, J. M., Mecerreyes, D., et al. (2020). Toward high-energy-density lithium metal batteries: opportunities and challenges for solid organic electrolytes. Adv. Mater. 32:e1905219. doi: 10.1002/adma.201905219
Wang, X., Zhai, H., Qie, B., Cheng, Q., Li, A., Borovilas, J., et al. (2019). Rechargeable solid-state lithium metal batteries with vertically aligned ceramic nanoparticle/polymer composite electrolyte. Nano Energy 60, 205–212. doi: 10.1016/j.nanoen.2019.03.051
Wang, X., Zhang, Y., Zhang, X., Liu, T., Lin, Y.-H., Li, L., et al. (2018). Lithium-salt-rich PEO/Li0.3La0.557TiO3 interpenetrating composite electrolyte with three-dimensional ceramic nano-backbone for all-solid-state lithium-Ion batteries. ACS Appl. Mater. Interf. 10, 24791–24798. doi: 10.1021/acsami.8b06658
Wang, X.-L., Mei, A., Li, M., Lin, Y., and Nan, C.-W. (2006). Effect of silane-functionalized mesoporous silica SBA-15 on performance of PEO-based composite polymer electrolytes. Solid State Ionics 177, 1287–1291. doi: 10.1016/j.ssi.2006.06.016
Wang, Y., Fan, F., Agapov, A. L., Saito, T., Yang, J., Yu, X., et al. (2014). Examination of the fundamental relation between ionic transport and segmental relaxation in polymer electrolytes. Polymer 55, 4067–4076. doi: 10.1016/j.polymer.2014.06.085
Wang, Y.-J., Pan, Y., and Kim, D. (2006). Conductivity studies on ceramic Li1.3Al0.3Ti1.7(PO4)3-filled PEO-based solid composite polymer electrolytes. J. Power Sources 159, 690–701. doi: 10.1016/j.jpowsour.2005.10.104
Wang, Z., Huang, X., and Chen, L. (2003). Understanding of effects of nano-Al2O3 particles on ionic conductivity of composite polymer electrolytes. Electrochem. Solid State Lett. 6:E40. doi: 10.1149/1.1615352
Wen, Z., Itoh, T., Ichikawa, Y., Kubo, M., and Yamamoto, O. (2000). Blend-based polymer electrolytes of poly(ethylene oxide) and hyperbranched poly[bis(triethylene glycol)benzoate] with terminal acetyl groups. Solid State Ionics 134, 281–289. doi: 10.1016/S0167-2738(00)00707-4
Wen, Z., Itoh, T., Uno, T., Kubo, M., and Yamamoto, O. (2003). Thermal, electrical, and mechanical properties of composite polymer electrolytes based on cross-linked poly(ethylene oxide-co-propylene oxide) and ceramic filler. Solid State Ionics 160, 141–148. doi: 10.1016/S0167-2738(03)00129-2
Weston, J. E., and Steele, B. C. H. (1982). Effects of inert fillers on the mechanical and electrochemical properties of lithium salt-poly(ethylene oxide) polymer electrolytes. Solid State Ionics 7, 75–79. doi: 10.1016/0167-2738(82)90072-8
Wieczorek, W., Florjanczyk, Z., and Stevens, J. R. (1995). Composite polyether based solid electrolytes. Electrochim. Acta 40, 2251–2258. doi: 10.1016/0013-4686(95)00172-B
Wieczorek, W., Stevens, J. R., and Florjañczyk, Z. (1996). Composite polyether based solid electrolytes. The Lewis acid-base approach. Solid State Ionics 85, 67–72. doi: 10.1016/0167-2738(96)00042-2
Wright, P. V. (1975). Electrical conductivity in ionic complexes of poly(ethylene oxide). Br. Polym. J. 7, 319–327. doi: 10.1002/pi.4980070505
Xi, J., Miao, S., and Tang, X. (2004). Selective transporting of lithium Ion by shape selective molecular sieves ZSM-5 in PEO-based composite polymer electrolyte. Macromolecules 37, 8592–8598. doi: 10.1021/ma048849d
Xiao, Y., Wang, Y., Bo, S.-H., Kim, J. C., Miara, L. J., and Ceder, G. (2020). Understanding interface stability in solid-state batteries. Nat. Rev. Mater. 5, 105–126. doi: 10.1038/s41578-019-0157-5
Xu, X., Wen, Z., Gu, Z., Xu, X., and Lin, Z. (2004). Lithium ion conductive glass ceramics in the system Li1.4Al0.4(Ge1-xTix)1.6(PO4)3 (x=0–1.0). Solid State Ionics 171, 207–213. doi: 10.1016/j.ssi.2004.05.009
Xu, X., Wen, Z., Yang, X., Zhang, J., and Gu, Z. (2006). High lithium ion conductivity glass-ceramics in Li2O–Al2O3–TiO2–P2O5 from nanoscaled glassy powders by mechanical milling. Solid State Ionics 177, 2611–2615. doi: 10.1016/j.ssi.2006.04.010
Xue, Z., He, D., and Xie, X. (2015). Poly(ethylene oxide)-based electrolytes for lithium-ion batteries. J. Mater. Chem. A 3, 19218–19253. doi: 10.1039/C5TA03471J
Yan, C., Zhu, P., Jia, H., Du, Z., Zhu, J., Orenstein, R., et al. (2020). Garnet-rich composite solid electrolytes for dendrite-free, high-rate, solid-state lithium-metal batteries. Energy Storage Mater. 26, 448–456. doi: 10.1016/j.ensm.2019.11.018
Yan, C., Zhu, P., Jia, H., Zhu, J., Selvan, R. K., Li, Y., et al. (2019). High-performance 3-D fiber network composite electrolyte enabled with Li-Ion conducting nanofibers and amorphous PEO-based cross-linked polymer for ambient all-solid-state lithium-metal batteries. Adv. Fiber Mater. 1, 46–60. doi: 10.1007/s42765-019-00006-x
Yang, C. R., Perng, J. T., Wang, Y. Y., and Wan, C. C. (1996). Conductive behaviour of lithium ions in polyacrylonitrile. J. Power Sources 62, 89–93. doi: 10.1016/S0378-7753(96)02414-7
Yang, H., and Yetter, W. (1994). Miscibility studies of high Tg polyester and polycarbonate blends. Polymer 35, 2417–2421. doi: 10.1016/0032-3861(94)90781-1
Yang, T., Zheng, J., Cheng, Q., Hu, Y.-Y., and Chan, C. K. (2017). Composite polymer electrolytes with Li7La3Zr2O12 garnet-type nanowires as ceramic fillers: mechanism of conductivity enhancement and role of doping and morphology. ACS Appl. Mater. Interf. 9, 21773–21780. doi: 10.1021/acsami.7b03806
Yanilmaz, M., Lu, Y., Zhu, J., and Zhang, X. (2016). Silica/polyacrylonitrile hybrid nanofiber membrane separators via sol-gel and electrospinning techniques for lithium-ion batteries. J. Power Sources 313, 205–212. doi: 10.1016/j.jpowsour.2016.02.089
Yue, L., Ma, J., Zhang, J., Zhao, J., Dong, S., Liu, Z., et al. (2016). All solid-state polymer electrolytes for high-performance lithium ion batteries. Energy Storage Mater. 5, 139–164. doi: 10.1016/j.ensm.2016.07.003
Zha, W., Chen, F., Yang, D., Shen, Q., and Zhang, L. (2018). High-performance Li6.4La3Zr1.4Ta0.6O12/Poly(ethylene oxide)/Succinonitrile composite electrolyte for solid-state lithium batteries. J. Power Sources 397, 87–94. doi: 10.1016/j.jpowsour.2018.07.005
Zhai, H., Xu, P., Ning, M., Cheng, Q., Mandal, J., and Yang, Y. (2017). A flexible solid composite electrolyte with vertically aligned and connected Ion-conducting nanoparticles for lithium batteries. Nano Lett. 17, 3182–3187. doi: 10.1021/acs.nanolett.7b00715
Zhang, C., Feng, Y., Han, Z., Gao, S., Wang, M., and Wang, P. (2019). Electrochemical and structural analysis in all-solid-state lithium batteries by analytical electron microscopy: progress and perspectives. Adv. Mater. 29:e1903747. doi: 10.1002/adma.201903747
Zhang, H., Li, C., Piszcz, M., Coya, E., Rojo, T., Rodriguez-Martinez, L. M., et al. (2017). Single lithium-ion conducting solid polymer electrolytes: advances and perspectives. Chem. Soc. Rev. 46, 797–815. doi: 10.1039/C6CS00491A
Zhang, J., Zang, X., Wen, H., Dong, T., Chai, J., Li, Y., et al. (2017). High-voltage and free-standing poly(propylene carbonate)/Li6.75La3Zr1.75Ta0.25O12 composite solid electrolyte for wide temperature range and flexible solid lithium ion battery. J. Mater. Chem. A 5, 4940–4948. doi: 10.1039/C6TA10066J
Zhang, J., Zheng, C., Lou, J., Xia, Y., Liang, C., Huang, H., et al. (2019). Poly(ethylene oxide) reinforced Li6PS5Cl composite solid electrolyte for all-solid-state lithium battery: enhanced electrochemical performance, mechanical property and interfacial stability. J. Power Sources 412, 78–85. doi: 10.1016/j.jpowsour.2018.11.036
Zhang, W., Nie, J., Li, F., Wang, Z. L., and Sun, C. (2018). A durable and safe solid-state lithium battery with a hybrid electrolyte membrane. Nano Energy 45, 413–419. doi: 10.1016/j.nanoen.2018.01.028
Zhang, Y., Chen, R., Wang, S., Liu, T., Xu, B., Zhang, X., et al. (2020). Free-standing sulfide/polymer composite solid electrolyte membranes with high conductance for all-solid-state lithium batteries. Energy Storage Mater. 25, 145–153. doi: 10.1016/j.ensm.2019.10.020
Zhao, Q., Stalin, S., Zhao, C.-Z., and Archer, L. A. (2020). Designing solid-state electrolytes for safe, energy-dense batteries. Nat. Rev. Mater. 5, 229–252. doi: 10.1038/s41578-019-0165-5
Zhao, Y., Huang, Z., Chen, S., Chen, B., Yang, J., Zhang, Q., et al. (2016a). A promising PEO/LAGP hybrid electrolyte prepared by a simple method for all-solid-state lithium batteries. Solid State Ionics 295, 65–71. doi: 10.1016/j.ssi.2016.07.013
Zhao, Y., Wu, C., Peng, G., Chen, X., Yao, X., Bai, Y., et al. (2016b). A new solid polymer electrolyte incorporating Li10GeP2S12 into a polyethylene oxide matrix for all-solid-state lithium batteries. J. Power Sources 301, 47–53. doi: 10.1016/j.jpowsour.2015.09.111
Zheng, F., Kotobuki, M., Song, S., Lai, M. O., and Lu, L. (2018). Review on solid electrolytes for all-solid-state lithium-ion batteries. J. Power Sources 389, 198–213. doi: 10.1016/j.jpowsour.2018.04.022
Zheng, J., and Hu, Y.-Y. (2018). New insights into the compositional dependence of Li-Ion transport in polymer–ceramic composite electrolytes. ACS Appl. Mater. Interf. 10, 4113–4120. doi: 10.1021/acsami.7b17301
Zheng, J., Tang, M., and Hu, Y. Y. (2016). Lithium ion pathway within Li7La3Zr2O12−polyethylene oxide composite electrolytes. Angewandte Chem. Int. Ed. 55, 12538–12542.
Zheng, J., Wang, P., Liu, H., and Hu, Y.-Y. (2019). Interface-enabled Ion conduction in Li10GeP2S12–Poly(ethylene Oxide) hybrid electrolytes. ACS Appl. Energy Mater. 2, 1452–1459. doi: 10.1021/acsaem.8b02008
Zhou, D., He, Y.-B., Liu, R., Liu, M., Du, H., Li, B., et al. (2015). In situ synthesis of a hierarchical all-solid-state electrolyte based on nitrile materials for high-performance lithium-Ion batteries. Adv. Energy Mater. 5:1500353. doi: 10.1002/aenm.201500353
Zhou, D., Liu, R., Zhang, J., Qi, X., He, Y.-B., Li, B., et al. (2017). In situ synthesis of hierarchical poly(ionic liquid)-based solid electrolytes for high-safety lithium-ion and sodium-ion batteries. Nano Energy 33, 45–54. doi: 10.1016/j.nanoen.2017.01.027
Zhu, J., Chen, C., Lu, Y., Ge, Y., Jiang, H., Fu, K., et al. (2015). Nitrogen-doped carbon nanofibers derived from polyacrylonitrile for use as anode material in sodium-ion batteries. Carbon 94, 189–195. doi: 10.1016/j.carbon.2015.06.076
Zhu, J., Chen, C., Lu, Y., Zang, J., Jiang, M., Kim, D., et al. (2016a). Highly porous polyacrylonitrile/graphene oxide membrane separator exhibiting excellent anti-self-discharge feature for high-performance lithium–sulfur batteries. Carbon 101, 272–280. doi: 10.1016/j.carbon.2016.02.007
Zhu, J., Ge, Y., Kim, D., Lu, Y., Chen, C., Jiang, M., et al. (2016b). A novel separator coated by carbon for achieving exceptional high performance lithium-sulfur batteries. Nano Energy 20, 176–184. doi: 10.1016/j.nanoen.2015.12.022
Zhu, J., Lee, C.-H., Joh, H.-I., Kim, H. C., and Lee, S. (2012). Synthesis and properties of polyimide composites containing graphene oxide via in-situ polymerization. Carbon Lett. 13, 230–235.
Zhu, J., Lim, J., Lee, C.-H., Joh, H.-I., Kim, H. C., Park, B., et al. (2014). Multifunctional polyimide/graphene oxide composites via in situ polymerization. J. Appl. Polymer Sci. 131:40177. doi: 10.1002/app.40177
Zhu, J., Lu, Y., Chen, C., Ge, Y., Jasper, S., Leary, J. D., et al. (2016c). Porous one-dimensional carbon/iron oxide composite for rechargeable lithium-ion batteries with high and stable capacity. J. Alloys Compounds 672, 79–85. doi: 10.1016/j.jallcom.2016.02.160
Zhu, J., Yanilmaz, M., Fu, K., Chen, C., Lu, Y., Ge, Y., et al. (2016d). Understanding glass fiber membrane used as a novel separator for lithium–sulfur batteries. J. Membr. Sci. 504, 89–96. doi: 10.1016/j.memsci.2016.01.020
Zhu, J., Yildirim, E., Aly, K., Shen, J., Chen, C., Lu, Y., et al. (2016e). Hierarchical multi-component nanofiber separators for lithium polysulfide capture in lithium–sulfur batteries: an experimental and molecular modeling study. J. Mater. Chem. A 4, 13572–13581. doi: 10.1039/C6TA04577D
Zhu, J., Zhu, P., Yan, C., Dong, X., and Zhang, X. (2019). Recent progress in polymer materials for advanced lithium-sulfur batteries. Prog. Polym. Sci. 90, 118–163. doi: 10.1016/j.progpolymsci.2018.12.002
Zhu, P., Yan, C., Dirican, M., Zhu, J., Zang, J., Selvan, R. K., et al. (2018a). Li0.33La0.557TiO3 ceramic nanofiber-enhanced polyethylene oxide-based composite polymer electrolytes for all-solid-state lithium batteries. J. Mater. Chem. A 6, 4279–4285. doi: 10.1039/C7TA10517G
Zhu, P., Yan, C., Zhu, J., Zang, J., Li, Y., Jia, H., et al. (2019). Flexible electrolyte-cathode bilayer framework with stabilized interface for room-temperature all-solid-state lithium-sulfur batteries. Energy Storage Mater. 17, 220–225. doi: 10.1016/j.ensm.2018.11.009
Zhu, P., Zang, J., Zhu, J., Lu, Y., Chen, C., Jiang, M., et al. (2018b). Effect of reduced graphene oxide reduction degree on the performance of polysulfide rejection in lithium-sulfur batteries. Carbon 126, 594–600. doi: 10.1016/j.carbon.2017.10.063
Keywords: lithium-ion batteries (LIBs), hybrid solid electrolytes (HSEs), solid electrolytes, polymer electrolytes, inorganic electrolytes, ionic conductivity, ion-transport mechanisms
Citation: Han L, Lehmann ML, Zhu J, Liu T, Zhou Z, Tang X, Heish C-T, Sokolov AP, Cao P, Chen XC and Saito T (2020) Recent Developments and Challenges in Hybrid Solid Electrolytes for Lithium-Ion Batteries. Front. Energy Res. 8:202. doi: 10.3389/fenrg.2020.00202
Received: 18 June 2020; Accepted: 31 July 2020;
Published: 02 September 2020.
Edited by:
Jian Liu, The University of British Columbia, CanadaReviewed by:
Wei Luo, Tongji University, ChinaYang Zhao, University of Western Ontario, Canada
Yuan Yang, Columbia University, United States
Copyright © 2020 Han, Lehmann, Zhu, Liu, Zhou, Tang, Heish, Sokolov, Cao, Chen and Saito. This is an open-access article distributed under the terms of the Creative Commons Attribution License (CC BY). The use, distribution or reproduction in other forums is permitted, provided the original author(s) and the copyright owner(s) are credited and that the original publication in this journal is cited, in accordance with accepted academic practice. No use, distribution or reproduction is permitted which does not comply with these terms.
*Correspondence: Lu Han, bHhoMzA2QGNhc2UuZWR1; Tomonori Saito, c2FpdG90QG9ybmwuZ292