The BECCS Implementation Gap–A Swedish Case Study
- 1Mercator Research Institute on Global Commons and Climate Change, Berlin, Germany
- 2Department of Space, Earth and Environment, Chalmers University of Technology, Göteborg, Sweden
- 3Geographisches Institut, Humboldt-Universität Zu Berlin, Berlin, Germany
The IPCC has assessed a variety of pathways that could still lead to achievement of the ambitious climate targets set in the Paris Agreement. However, the longer time that climate action is delayed, the more the achievement of this goal will depend on Carbon Dioxide Removal (CDR) technologies and practices. In the models behind these pathways, the main CDR technology is Bioenergy combined with Carbon Capture and Storage (BECCS). We review the role that BECCS could play in reaching net-zero targets based on the existing 1.5°C scenarios. Such scenarios presented in the literature typically have BECCS at a GtCO2 per year scale. We also assess the potentials and obstacles for BECCS implementation at the national level, applying Sweden as a case study. Given that BECCS deployment has scarcely started and, thus, is far from capturing 1 GtCO2 per year, with lead times on the scale of multiple years, we conclude that there will be a large implementation gap unless BECCS development is immediately intensified, emissions are reduced at a much faster pace or removals realized through other CDR measures. In the national case study, we show that Sweden has favorable conditions for BECCS in that it has large point sources of biogenic emissions, and that BECCS has been identified as one potential “supplementary measure” for reaching the Swedish target of net-zero emissions in 2045. Yet, work on planning for BECCS implementation has started only recently and would need to be accelerated to close the implementation gap between the present advancement and the targets for BECCS proposed in a recent public inquiry on the roles of supplementary measures. An assessment of two ramp-up scenarios for BECCS demonstrates that it should in principle be possible to reach the currently envisaged deployment scales, but this will require prompt introduction of political and economic incentives. The main barriers are thus not due to technological immaturity, but are rather of a socio-economic, political and institutional nature.
Introduction
An analysis of the 1.5°C pathways assessed in the recent IPCC Special Report on 1.5°C Global Warming reveals that CO2 capture and storage (CCS) can reach up to 460 GtCO2 cumulatively by mid-century, noting that this includes also CCS from coal and gas plants and that some pathways will still derive more than 20 EJ per year from coal in 2050 (Rogelj et al., 2018). In addition, Bioenergy with CCS (BECCS) remains as the major technology for removing CO2 in the vast majority of scenarios associated with a high likelihood of achieving ambitious climate targets, such as those laid out in the Paris Agreement (Rogelj et al., 2018). BECCS is a carbon removal technology that is considered as promising for the near future, as the technologies on which it is based–bioenergy as well as capture, transport and Storage of CO2–have all been demonstrated at scale. Other important CDR methods include Direct Air Carbon Capture and Storage (DACCS) and afforestation or reforestation. Application of BECCS serves two purposes in terms of mitigating emissions: 1) it can offset ‘hard-to-abate’ sector emissions (Davis et al., 2018; Royal Society and Royal Academy of Engineering, 2018; Tong et al., 2019); and 2) in the longer run, it can create net-negative emissions, which would be required to return from a likely overshoot of the target (Luderer et al., 2018; Minx et al., 2018).
It is of course indispensable that fossil fuel reserves stay in the ground or are only used in association with CCS (see e.g., Johnsson et al., 2019), and that the application of CDR is not used as an excuse to postpone other mitigation measures. Currently, far more fossil carbon is used (80% of the primary energy supply) than biogenic carbon (around 12%). Moreover, a substantial share of the biomass uses is from so-called ‘traditional’ biomass (International Energy Agency, 2017), which means that the actual share is associated with large uncertainties. Nevertheless, there are countries with large forests, some of which have well-developed forest management systems with high productivity and with a net growth of the carbon stock. In addition, there is significant potential for establishing new biomass production systems, although there are substantial variations in the estimates of their potential contributions (Creutzig et al., 2015).
Still, there has been little progress toward the implementation of CCS and even less so in the case of BECCS (Peters et al., 2017). This has been confirmed by Fridahl (2017) who, based on survey responses from 711 delegates at a UN climate change conference, has reported that BECCS is not prioritized compared to alternative mitigation technologies. Therefore, we conclude that there is a large implementation gap between the dramatic ramp-up of BECCS (or alternative CDR options) in most of the ambitious climate stabilization pathways that are mainly generated by Integrated Assessment Models (IAMs) (Clarke et al., 2014; Smith et al., 2016; Minx et al., 2018; Rogelj et al., 2018) and the current development of BECCS at both the global and regional scales. This gap is unlikely to be closed in the absence of strongly enhanced climate policies. Currently, the price signal for CO2 is too weak for CCS (e.g., in the EU emissions trading system; EU ETS) and no climate policy presently exists that provides incentives strong enough for BECCS to be deployed at scale.
In this work, we focus on BECCS and compare its timeframes in key global scenarios with the timeframe of implementation at the national scale, applying Sweden as a case study. We use Sweden because: 1) in theory, it holds favorable conditions for BECCS, having a well-developed forest industry and net growth of carbon stock in the forests; 2) it has strong ambitions to become a forerunner in climate action, having established a national target of achieving net-zero emissions by 2045, after which emissions should be net-negative; and 3) a public inquiry (SOU, 2020) recently conducted in Sweden has proposed actions to accelerate CDR upscaling (in particular, BECCS) and has suggested explicit targets for BECCS in 2030 and 2045. Bellamy and Geden (2019) cite Stockholm Exergi, which is the provider of district heating for the capital of Sweden and which is currently using biochar (a byproduct of heat generation from biogenic waste) to remove CO2 and is planning for BECCS (currently having a small test unit in operation) (Gustafsson, 2018) as an example of companies that are acting as early movers to comply with the Swedish net-zero greenhouse gas (GHG) target by 2045. Similarly, Honegger and Reiner (2018) have pointed out that it will be up to potentially progressive industrialized countries to take the first steps toward mobilizing CDR, including BECCS. Considering the above three points, and considering its national political rhetoric, Sweden appears to be well placed to take on such a role.
The present study addresses two important questions related to BECCS in the national context: Under what conditions can BECCS be ramped up in Sweden and can that knowledge help us understand other regions and focus attention on the leverage points needed for BECCS scale-up? In tackling these questions, we refer to the BECCS implementation gap as the difference between–on the one hand–the rates of BECCS deployment observed in the global 1.5°C pathways (see Bioenergy Combined With Carbon Capture and Storage in the 1.5°C pathways) and–on the other hand–the actual progress of implementation with respect to technology, policy and economics, governance and society (The potential role of BECCS in Sweden). Fridahl (2017) has stressed the need for studies of the sociopolitical preconditions for large-scale CDR deployment. The present paper also contributes to revealing the corresponding knowledge gaps, while also identifying areas for direct action. The latter is important, since there is an urgency related to developing concrete strategies for CDR implementation, and direct action is required in parallel with research if net-zero targets and, subsequently, net-negative levels of emissions are to be reached in time.
Methodology
To answer the research questions outlined above, we adopted a mixed-methods approach, starting with global climate stabilization pathways, primarily on the basis of the literature based on the use of IAMs. As already indicated, these models allow for assessments of the implications of and sensitivity to a wide range of parameters at global scales, and they complement national models that inform the implementation with greater granularity of the top-line policy requirements discovered by IAMs (Fuhrman et al., 2019). Thus, the resulting global pathways represent a natural point of departure for motivating our national case study, which represents a comprehensive exploration of the potential with higher granularity for policymaking. This may not only translate the acquired knowledge to countries with similar conditions, but may also serve to identify aspects that can be improved in global models, such as including hitherto unexplored BECCS potentials for industry. In this section, we outline the steps of our approach, following a schematic representation (Figure 1).
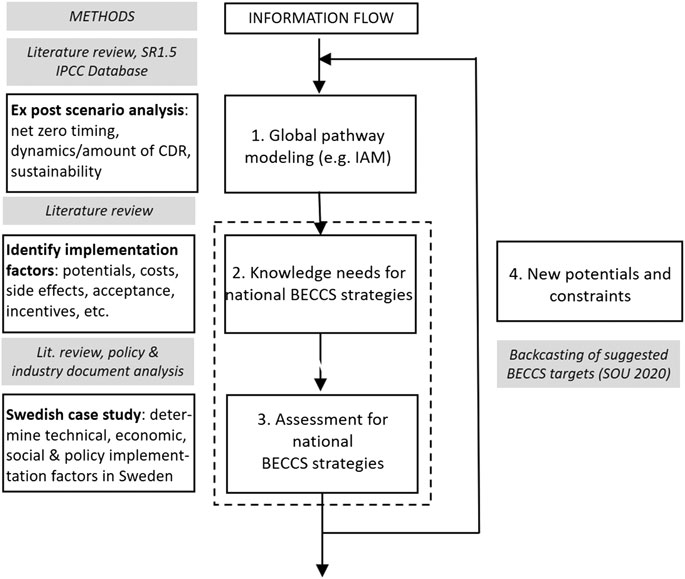
FIGURE 1. Schematic of the procedure for assessing the role of BECCS through linking national strategies to global pathway modeling systems such as Integrated Assessment Modeling (IAM).
First, we assess the timeline for the required ramp-up of BECCS on a global scale, as obtained from the global 1.5°C pathways, taking these dynamics as guidance for national CDR strategies (Step 1 in Figure 1). Subsequently, this is complemented with a review of the literature on the lack of connection between global pathways and national strategies, and identifying knowledge needs for the latter (Step 2 in Figure 1). We then examine Sweden as a regional BECCS case study (Step 3 in Figure 1) by using qualitative analysis, underpinned with quantitative data when possible: 1) analyzing the technical conditions for BECCS rollout in Sweden; 2) assessing the potentials and costs of Swedish BECCS based on the peer-reviewed literature and information obtained from industry; 3) distilling insights into Swedish policy planning for BECCS from the recently issued SOU 2020:4 report titled The road toward a climate positive future, which emerged from a public inquiry; and 4) eliciting potential barriers related to social acceptance based on the peer-reviewed literature. These steps allow us to characterize more definitively the BECCS implementation gap for the Swedish regional case and to identify the initial entry points for actions to close this gap. In particular, we develop two hypothetical roadmaps for upscaling BECCS in Sweden, based on observations of existing industry plans, and use these roadmaps to assess the BECCS targets suggested in the SOU 2020:4 report. Thus, the targets serve as a starting point and we backcast the pathways to reach these targets, in the process of which we attain a better understanding of the underlying conditions that need to be in place for the roadmaps to be realized. These underlying conditions are thereafter highlighted in the conclusion.
Finally, as depicted in the flow diagram in Figure 1, this chain of analyses does not need to culminate in informing national strategy. Instead, newly identified potentials and constraints can be fed back into the modeling of global pathways (Step 4 in Figure 1), so as to provide more realistic assumptions for IAMs concerned with the prospects of BECCS. In order for a comprehensive feedback loop to be established, the procedure obviously has to be carried out for all countries and regions for which BECCS can be identified as a potentially important technology. The latter is clearly beyond the scope of the present study and is to be understood as indicative of future research on this topic.
Bioenergy Combined With Carbon Capture and Storage in the 1.5°C Pathways
While this study focuses on BECCS, it is important to note that for a complete analysis, fossil fuel CCS has to be considered also, given that many of the inhibiting factors that lead to the BECCS implementation gap are inextricably linked to the development of fossil fuel CCS. There are also several emissions sources that use a mixture of fossil and biogenic feedstocks, such that if they are equipped with CCS they will to some extent be a BECCS application. In addition, as CCS and BECCS are typically based on the same technologies, their demonstration and scale-up should not be treated separately but in an integrated manner to accelerate the learning process toward large-scale implementation. The 1.5°C pathways that have been assessed in the IPCC Special Report on 1.5°C Global Warming feature quantities of CO2 stored through CCS until 2100 that range from zero to 1900 GtCO2 depending on the portfolio of mitigation measures (Rogelj et al., 2018). The 1.5°C pathways that exclude CCS completely–such as the one by Grubler et al. (2018)–foresee much higher potentials for demand-side mitigation measures than the previously described pathways and, in particular, much lower energy demand. All the 1.5 °C pathways obviously require a comprehensive phasing out of the use of fossil fuels, in particular coal. CCS could allow a smoother transition for countries that have large endowments of fossil fuel reserves, which represent an important component of their economies (Johnsson et al., 2019), while complying with strict emission reductions. Since CCS can also be applied to biogenic emission sources, it represents a versatile mitigation technology. It can, on the one hand, limit CO2 emissions from fossil fuels used for electricity generation, liquids production and industrial applications, and on the other hand, potentially remove CO2 from the atmosphere when combined with bioenergy (Kriegler et al., 2014).
Of the up to 460 GtCO2 captured and stored up to 2050 in the above-mentioned IPCC Special Report on 1.5°C Global Warming (IPCC, 2018a), up to 190 GtCO2 are derived from biogenic sources and that fraction will typically increase in the second half of the century (Rogelj et al., 2018). In particular, pathways that limit global warming to 1.5°C with limited or no overshoot feature BECCS deployment of up to 1, 8, and 16 GtCO2 per year in 2030, 2050, and 2100, respectively (IPCC, 2018b). BECCS that results in the removal of 16 GtCO2 per year would deliver ∼225 EJ per year of primary energy (assuming BECCS is used in electricity generation) in 2100 (Smith et al., 2016), as compared to the current level of about 50 EJ of biomass energy per year. In addition to scaling up BECCS, this would obviously require a substantial scaling up of the biomass supply from agricultural and forestry residues, as well as from dedicated bioenergy crops grown on abandoned agricultural land and expansion into grasslands (Vaughan et al., 2018). While the estimates vary widely, most of the literature indicates that biomass energy could be scaled to something between 100 and 300 EJ per year by mid-century (Smith et al., 2014; Vaughan et al., 2018). This level would be exceeded in pathways that stabilize at 1.5°C in 2100 but that overshoot the target during the century.
The nature of the side-effects from BECCS crucially depends on the mode of implementation and the scale. Thus, the planting of monocultures for increased use of biomass, for example, could indeed result in carbon removal but might also be at odds with other societal and environmental goals, e.g., other Sustainable Development Goals (SDGs) related to food security and biodiversity conservation (Smith et al., 2019). There is a vast body of literature testifying to an increased demand for land from large-scale BECCS, putting food production, biodiversity, etc. at risk (see Fuss et al., 2018a and references therein). Resorting to marginal land or using residues as well as dedicated crops, as shown by Vaughan et al. (2018), could mitigate some of this risk. Yet, at carbon removal levels in the double-digit Gigatonne range, as given above, fewer and fewer opportunities for careful implementation remain, resulting in increasingly severe trade-offs for different land uses. This comes on top of the uncertainties associated with the availability of marginal and degraded land globally. Obviously, the associated ramping up of the biomass supply would be a tremendous challenge.
A systematic literature review of more than 1,000 studies (Robledo-Abad et al., 2017) regarding the side-effects of bioenergy reveals that there can be both trade-offs and co-benefits. However, negative effects are more often reported in the literature that focuses on the social and environmental dimensions, mostly in relation to land use changes that have impacts on ecosystems and food security. Positive effects of bioenergy are more often observed in techno-economic studies, emphasizing the technological opportunities, such as yield increases, and economic benefits (e.g., employment opportunities). Similarly, the majority of the non-IAM literature on BECCS is more concerned with the negative side-effects that a large-scale rollout would have for different dimensions of sustainability, with most of the research being concerned with the land footprint, highlighting the detrimental impacts on the safeguarding of terrestrial ecosystems and the provision of food security for a growing population (Fuss et al., 2018b; Smith et al., 2019). Other CDR options, such as Enhanced Weathering or increasing ocean alkalinity, do not feature a large land footprint, although they are associated with other uncertainties and, thus, not widely included in IAMs to date, making an integrated assessment difficult. In fact, since model intercomparison of wider CDR portfolios in IAMs has only recently started (e.g., Realmonte et al., 2019) and many IAM experts themselves are critical of the notion that IAMs can be expanded to many CDR methods beyond BECCS (Rickels et al., 2019), the discussion is still very much focused on the environmental sustainability of BECCS.1 However, since IAMs have a rather crude representation of the supply chains associated with many BECCS technologies and lack regional detail, many of the low-hanging fruits in the area of BECCS are still not accounted for–in the same way that the lack of reconciliation between top-down and bottom-up approaches has been identified as leading to important tradeoffs being overlooked in the related context of bioenergy (Creutzig et al., 2012). IAMs thus may also overestimate realistic BECCS deployment rates due to a lack of realistic regional assumptions related to, for example, a sustainable and socially acceptable biomass supply. In general, the missing granularity of top-line policy requirements uncovered by IAMs (Fuhrman et al., 2019) reflects the difficulties associated with assessing geologic suitability and feasibility from the perspectives of other SDGs.
Another dimension of implementation that is not well-represented in global IAM modeling is the absence of good governance systems for the management of biomass systems across the globe. As Vaughan et al. (2018) have pointed out very clearly, only one-third of bioenergy crops are grown in regions associated with more-developed governance frameworks. This could impact negatively both the environmental and social sustainability aspects if even more biomass was to be needed in the future, as confirmed in IAM studies such as that carried out by Butnar et al. (2020). Global climate pathways might also create the impression that governance will have to be jurisdictional or even global, in particular when considering the removal of tens of Gigatonnes of CO2. Yet, while there may be aspects associated with, for example, the sustainability of large-scale land use change that would need at least global coordination, there is increasing recognition that CDR will primarily emerge as a bottom-up strategy that is governed by companies and cities, which means that it cannot be comprehensively coordinated in a top-down fashion globally (Bellamy and Geden, 2019). This indicates opportunities for nested governance approaches, which have often been recommended in the context of Reduced Emissions from Deforestation and Degradation (REDD+) (Kashwan and Holahan, 2014).
Finally (heterogeneous) societal preferences are not yet captured in IAMs (Forster et al., 2020). Whether and to what extent different types of CDR technologies and practices will be acceptable to society are issues that do not yet feature in IAMs. Nonetheless, the scenario space has recently started to widen in this respect, both in terms of constraining contested technologies and land use change and through the explicit integration of the mitigation potentials of hitherto largely unexploited opportunities associated with processes such as innovation and behavioral changes (Grubler et al., 2018; Rogelj et al., 2018; van Vuuren et al., 2018).
Given that BECCS deployment has scarcely started and, thus, is far from being capable of capturing 1 GtCO2 per year and that lead times are on the scale of multiple years (see also The potential role of Bioenergy Combined With Carbon Capture and Storage in Sweden), there will be a large implementation gap if emissions cannot be reduced at a much faster pace and removals cannot be realized through other CDR measures, such as afforestation. This implementation gap is further exacerbated by the paucity of knowledge regarding the efficient ramping up of BECCS (Nemet et al., 2018). As this will depend on local conditions, there is a great need to assess the national context for the upscaling of BECCS. Otherwise, there is a risk that BECCS will largely remain a hypothetical technology (e.g., in IAM models), resulting in an underestimation of the actual implementation gap and, consequently, too much faith being placed in BECCS as a straightforward solution for achieving national net-zero goals (Laude, 2020). To date few countries and states, e.g., Sweden, the United Kingdom, and California, are actively looking into the definitive CDR portfolios to be deployed in order to fulfill their national (or federal) climate neutrality goals, including the policy framework (Committee on Climate Change, 2019; Baker et al., 2020; SOU, 2020). Table 1 summarizes the key messages from the analysis of the global pathways for BECCS.
We take an initial step toward addressing this knowledge gap by focusing on the case of Sweden, which has many existing biogenic emission sources, access to a sustainable biomass supply chain, technological experience with carbon capture in research and demonstration, and ties to the Northern Lights Project on storage infrastructure. Thus, there should exist in principle good conditions for a relatively rapid ramping up of BECCS, which would significantly contribute to the national net-zero (or, in this case, even net-negative) emissions goals. It is important to emphasize the need for such national case studies to complement IAM policy pathways (for the reasons outlined above). The analysis conducted herein should, therefore, not be understood as a substitute for IAMs, in line with what has been concluded by others (Gambhir et al., 2019).
The Potential Role of Bioenergy Combined With Carbon Capture and Storage in Sweden
Entry Points for a National Bioenergy Combined With Carbon Capture and Storage Strategy: Technical Potentials
Sweden is a highly industrialized country with several basic-material industries, such as iron and steel, cement, and pulp and paper (P&P), all of which emit substantial amounts of CO2. At the same time, the electricity generation system is to a large extent CO2-free, since hydro power and nuclear power each provide around 40% of the electricity generation, with the remaining 20% being mainly from wind power and the power generated in combined heat and power (CHP) plants.
Sweden has a well-developed forest industry with high-level production of various biomass-based products, such as saw timber, P&P, and different wood products. The Swedish forest industry is established around large forestry resources and a well-developed forest management system with a growing carbon stock in the forests (Swedish Environmental Protection Agency, 2017). In addition, there is the potential to enhance forest productivity, so as to increase the output levels of forest products while enhancing carbon sequestration in the forests (Cintas et al., 2017). The waste from the forest industry (branches and stumps from the forests and wood waste from the timber industry) is used in the energy system, typically in heat only and CHP plants in district heating systems. Thus, current biomass use follows a cascading principle from long-lived products to paper products to the biomass waste fractions used for energy purposes. Since the 1990s, there has been an increase in the use of biomass in the transportation sector, with biofuels mainly produced from biogenic waste from the P&P industry in the form of tall oil, together with imported biofuels. The current share of biomass in road transportation is around 20%. However, the major share of biomass is used in industry, mainly for saw timber (46%) and pulpwood (42%), with the remaining share (including residues from industrial use) being used in the energy sector. Thus, there are large biogenic point sources from these biomass conversion processes, mainly P&P plants and CHP plants (Garðarsdóttir et al., 2018). This can be concluded from Figure 2, which shows a map of the Swedish large point sources of CO2 emissions (including both fossil and biogenic). Figure 2a shows the sources with emissions in the range of 100–500 ktCO2 per year and Figure 2B shows those sources with emissions that exceed 500 kt per year. As for the BECCS potential, there are around 70 facilities with biogenic CO2 emissions of >100 kt per year, which together exceed 30 MtCO2 per year.
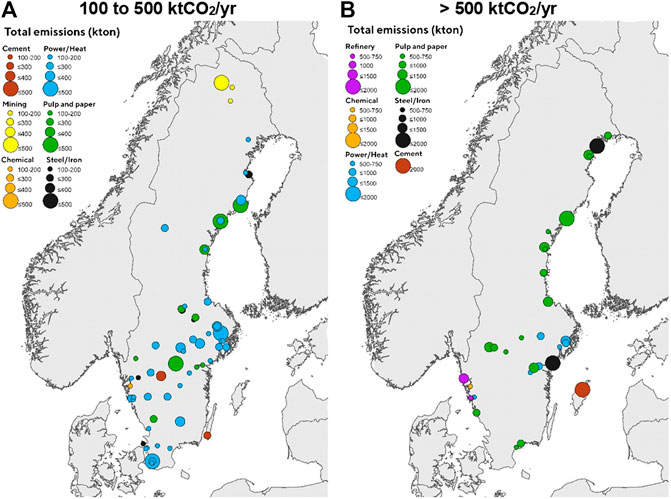
FIGURE 2. Swedish emissions sources from different types of plants with: (A) emissions in the range of 100–500 ktCO2/year; and (B) emissions >500 ktCO2/year. Reproduced from (Johnsson and Kjärstad, 2019).
If applying CO2 capture to these emission sources, storage can most likely be “purchased” from Norway, as that country has well-documented storage (Anthonsen et al., 2013; Lyng Anthonsen et al., 2016) and advanced plans for the establishment of a transboundary storage infrastructure, although this will result in a dependency on foreign infrastructure operators. Work on establishing the Norwegian storage infrastructure has already started (the Northern Lights Project for storage in the North Sea). This project should be favorable for reducing lead times once capture projects are initiated in Sweden. In particular, Northern Lights, which is being developed by Equinor (formerly Statoil), includes intermediate storage facilities for receiving CO2 transport by ships (Equinor, 2019; Furre et al., 2019). The realization of this infrastructure is linked to the execution of the first two Norwegian large-scale, on-shore CCS projects. These projects will each capture around 400 ktCO2 per year: from the Norcem cement plant in Brevik (south of Norway); and from the waste-fired CHP plant in Klemetsrud (south of Oslo). The Norwegian Oil and Gas Ministry has recently proposed (Det Kongelege Olje- og Energidepartementet, 2020) that the cement plant should receive State funding (with the Norwegian Government covering the major part of the cost over 10 years), and that the CHP plant should receive funding provided that 50% of the total can be raised from sources other than the State (a decision on this was taken in December 2020, forming the so-called “Langskip”-project).
Figure 2 clearly shows that a substantial proportion of the largest emission sources in Sweden is located along the coastline which, in a future CCS/BECCS system, will facilitate transport of the captured CO2 by ship to geologic storage sites located beneath the North Sea. It is not clear what the lower limit in terms of the size of flue gas flow will be for applying CO2 capture. This will depend on the concentration of CO2 in the flue gases, climate policy, the value of the product, and customers’ willingness to pay for climate-neutral or climate-positive products, which in turn will depend on which value chain is considered. It seems likely that the initial projects (beyond demonstration projects) will target large emissions sources, e.g., those exceeding 500 ktCO2 per year (Figure 2B). Once there is sufficient experience of using the CCS technology and a transport and storage infrastructure has been established that can be shared with additional capture plants, capture could be applied to smaller sources of emissions, perhaps those with emissions of 100-500 ktCO2 per year (Figure 2A).
The combination of the many existing biogenic emission sources and the access to well-established biomass markets, supply chains, and forest management system should provide favorable conditions for BECCS. Thus, Sweden can be expected to be an early driving force for implementing BECCS, in particular if it teams up with Norway for storage in connection with the Northern Lights Project (or possibly with other upcoming Dutch or UK storage projects in the North Sea). The challenge will be to establish policies that create incentives for mitigating biogenic emissions. Such policies are lacking at present but have been proposed, as discussed in Climate targets.
The Economic Potential
Johnsson et al. (2020) have generated a marginal abatement cost curve for CCS (post-combustion using amine scrubbing) applied to the largest industrial emissions sources in Sweden (excluding CHP plants in the energy sector), with emissions exceeding 500 ktCO2 per year (Figure 2B). These include 28 units, representing cement (1 plant), chemicals (1 plant), iron and steel (3 plants, including a CHP unit), refineries (3 plants), and P&P plants (20 plants). These plants constitute a mix of fossil fuel and biogenic emissions (several of them having multiple stacks). The total emissions captured from the 28 industrial units amount to 23 MtCO2 per year, which corresponds to more than half of Sweden’s total (fossil fuel) CO2 emissions from all sectors (around 43 MtCO2 per year).2 This level of capture is achieved at an average cost of 80–135 €/tCO2 if one includes the costs for transport and storage (Johnsson et al., 2020). If the same level of emissions was to be captured solely from fossil fuel emissions, capture would have to be included also for smaller sources of emissions, thereby driving up the cost significantly. This shows that BECCS should not be treated in isolation. Instead, it should be integrated with CCS in order to follow a cost-efficient ramping up of CO2 capture. In addition, there are opportunities to capture CO2 from CHP plants that are mainly burning biomass (branches and tops from forestry), including waste-fired units that typically burn a mixture of renewable and fossil (plastic waste) fuels. All in all, it can be concluded that Sweden has strong potential for BECCS with the already existing point sources of biogenic emissions. Thus, capturing CO2 from industrial emissions sources represents a powerful approach in that it can remove a substantial portion of CO2 emissions through implementing CCS at a limited number of plants. It should be noted that maintaining the outputs from plants when adding BECCS will require increased sources of biomass supply (to compensate for the loss of efficiency of the process), although the degree to which this supplementation is necessary depends on the type of process, including the availability of waste heat to power part of the capture process.
When it comes to the potential for storage, there is, on the one hand, a large storage potential in Norway and, as mentioned in the previous section, work has already been initiated to establish a storage infrastructure. On the other hand, Sweden has limited storage capacity within its own territory and there is little geologic information on the storage conditions. As a consequence, determining the actual storage capacity would require substantial geologic surveying (see Mortensen et al. (2016) and Mortensen et al. (2017) for overviews of the Swedish storage potential).
Climate Targets
In 2017, Sweden adopted a climate policy framework that consists of a climate act, climate targets, and a climate policy council. Sweden’s long-term target is to have net-zero GHG emissions by 2045 at the latest, after which the emissions should be at net-negative levels (Swedish Environmental Protection Agency, 2017). Net-zero emissions by 2045 are specified as at least an 85% reduction in domestic emissions, as compared to the corresponding levels in 1990. Thus, up to 15% of the reductions can be met by so-called ‘supplementary measures’, which can involve carbon removal through BECCS or other technical measures (e.g., DACCS3), increased carbon uptake by the terrestrial biosphere (e.g., afforestation), and the implementation of offsetting measures abroad. That is, these removals are designed to compensate for the residual emissions that are expected to occur in ‘hard-to-abate’ sectors (cf.Davis et al., 2018; Luderer et al., 2018 on compensation of residual emissions at the global level), such as agriculture and the use of fossil fuels in transport, most notably aviation. The requirement for measures to be taken in other countries (offsets, so to say) is that they must be above and beyond what would otherwise have been done in those countries. Concerning the ‘supplementary measures’, it is reasonable to assume that BECCS, owing to its favorable conditions, will be a suitable candidate technology.
The recently published inquiry conducted for the Government of Sweden (SOU 2020:4) regarding supplementary measures has proposed that such measures should be created to account for the equivalent of at least 3.7 MtCO2 of CDR by 2030. Up to 2045, the corresponding level is set at 10.7 MtCO2. The inquiry (SOU2020:4) has concluded that it will be much more costly to reach the target of net-zero emissions in the absence of such supplementary measures, since that would require a comprehensive transformation of the agricultural sector (e.g., to mitigate non-CO2 emissions such as methane and nitrous oxide). The SOU2020:4 inquiry report proposes that BECCS is critical for meeting the target of net-negative emissions after 2045. The report also indicates that the climate policy action plans, which the Government of Sweden submits to the Parliament every 4 years, should include how the work on the supplementary measures is progressing. The system for collecting data and reporting of the removals should be carried out by the Swedish Environmental Protection Agency, which is already monitoring progress toward reaching the national climate goals.
Incentives
From the work conducted by Johnsson et al. (2020), the average cost for capture, transport and storage can, for the sake of simplicity, be averaged at 100 €/tCO2–a cost level that is almost identical to the Swedish CO2 tax (somewhat exceeding 100 €/tCO2). However, only selected parts of the economy, such as the transportation sector and the heating sector, are in reality exposed to this tax. Incentives for mitigating the above-mentioned large point sources of fossil fuel emissions (Figure 2), for which CO2 capture is an option, are manifested through the EU ETS. However, the present allowance price of around 30 €/tCO2 is considerably lower than the cost of CCS, and previous research has shown that political uncertainty can have a detrimental impact on future price developments (Koch et al., 2016; Fuss et al., 2018a). For the biogenic emissions, there are as of yet no economic mitigation incentives. Thus, a ramp-up of BECCS will obviously require governmental intervention in order to establish incentives and, thereby, reduce the risks for the investor. As for CCS, there is a need to reform the EU ETS to increase allowance prices or to provide governmental intervention until allowance prices have reached a sufficiently high level. Examples of accompanying measures that foster CCS from other parts of the world include Low Carbon Fuel Standard (LCFS) policies and the 45Q tax credit for US industrial manufacturers that capture carbon from their operations. The latter would earn US$50 per metric tonne of CO2 stored permanently or $35 per metric tonne if the CO2 is put to use, which could mean that the balance does not remain negative (Hepburn et al., 2019). Finally, it is also important to devise incentive schemes that gain strong acceptance within society (see also the discussion of Bellamy et al. (2019) in Incentives), as well as from industry.
When it comes to Sweden creating incentives for CDR, the report proposes a reverse-auctioning process (one buyer–the Government–and many sellers–those who can offer carbon removal). It is proposed in the SOU2020:4 report that the reverse auctions would result in differentiated guarantee prices for actors who win the auctions for storing CO2 from biogenic sources. The auctioning system aims to serve as an initial support and it would by 2030 be limited to 2 MtCO2 per year, which would correspond to 3–5 BECCS plants. The system will thereafter be evaluated to decide what continued governance is required for BECCS. Furthermore, it is stated in SOU2020:4 that “the compensation paid out should be the difference between the agreed guarantee price and the value of any EU funding and national funding to promote bio-CCS that an actor receives. To have funds paid out, it is required that the project owner has applied for relevant support from the EU”. Thus, if no EU funding is secured, the system gives a contracted guarantee price that will be paid in full. If EU funding is secured, the difference between the EU funding and the guaranteed price will be paid by the Swedish State, i.e., corresponding to a contract-for-difference scheme. At present, it is not known when there will be any incentives to mitigate biogenic emissions within the EU ETS. Integration of CDR into the EU ETS would require an amendment to the EU ETS Directive (installations only using biomass are not covered by the ETS Directive); see Rickels et al. (2020) for a discussion of CDR and EU emissions trading.
While the details of the reverse-auctioning system are not yet established (and other incentive schemes are being evaluated), it seems likely that it will only apply as long as there is a positive difference between the guaranteed price from the auctioning and any EU funding (such as emission credits or support from the EU innovation fund or other investment or operational support schemes). If such funding is greater than the contracted guarantee price, the system can be designed so that the difference will not have to be paid to the State (corresponding to a price floor but not a price ceiling) or so that it has to be paid back to the State (in that case, corresponding to both a price floor and a price ceiling). There are obviously also other design issues that must still be outlined, e.g., when payments should be issued (SOU2020:4 suggests that partial payments be made in advance, to act as a form of investment aid and with a binding period of 10–20 years, thereby enabling long-term planning for the parties involved).
Assuming that the auctioning scheme proposed in SOU2020:4 will be implemented, there will be a level of predictability associated with the funding of BECCS, thereby compensating for the uncertainty emanating from the EU level, where there are still no incentives for CDR. It is also not clear–and probably not an obvious task–as to how to coordinate the national auctioning system with any EU measure for supporting CDR. Perhaps for that reason, it is (in SOU2020:4) proposed to develop a common, long-term instrument to promote BECCS either in a technology-neutral manner or by altering the EU ETS so that BECCS gives rise to emission credits that may be used within the EU ETS. However, this would require that measures are taken to adjust the number of emission allowances in the system, to avoid undermining incentives to reduce fossil-fuel emissions. In the SOU2020:4 report, it is concluded that the development of a common instrument to promote BECCS may be the easiest way forward, since it will not require renegotiation of the EU’s main legal provisions.
Although SOU2020:4 proposes that the reverse-auctioning system be limited to a maximum of 2 MtCO2 captured and stored annually by 2030, it expects a certain shortfall of this level and assumes instead that 1.8 MtCO2 can be reached. Assuming an average cost of 100 €/tCO24 and no other EU policy measures are in place to create incentives for BECCS or other CDR methods with lower cost significantly contributing to carbon removal, this would mean that the Government of Sweden would have to pay out up to 180 million € per year5. This can be compared to the annual intake from the Swedish CO2 tax, which amounts to 2.3 billion €. If capture was applied to all biogenic emissions from the above-mentioned industrial plants, around 10 Mt/year (cf.Johnsson et al., 2020), the associated governmental financing would amount to around 1 billion €/year. The latter seems unlikely, although it is a reasonable assumption that 10 Mt/year will only be reached in the longer term when other financing instruments have been found and costs have been decreased further. These figures can also be placed in the context of the substantial economic recovery packages being applied for restarting the economy after the COVID-19 pandemic, with one of the Swedish recovery packages being worth up to 10 billion €. Thus, it is not so obvious what constitutes a high or a low cost for climate mitigation, as this depends both on the value of the avoided externalities if climate targets can be met and the influence on the economy in a wider sense (such as job creation or job losses resulting from mitigation measures). In general, there are indications that the levels of governmental spending on recovery packages (globally) are much higher than the spending required to change course so to be in line with the Paris Agreement (Andrijevic et al., 2020).
Assuming that the auctioning (possibly together with other support measures) will cover the cost of BECCS (around 100€/tCO2, as mentioned above), this may result in a higher value of CDR compared to the cost of causing fossil fuel emissions, at least up to 2030, since the allowance price within the EU ETS is at present around 30€/tCO2. There is an obvious difference between fossil and biogenic carbon emissions in that removing carbon from the atmosphere is a benefit for society, although it confers no extra benefit on the BECCS operator, whereas fossil emissions generate economic benefits for the operator but result in external costs. One has to be conscious of the risk of making it more profitable to establish CDR by means of bioenergy with capture than to avoid fossil fuel emissions in the first place (i.e., the value of CDR would exceed the penalty of emitting fossil emissions). In this case, there would, for example, be incentives to install inefficient BECCS plants because more CO2 could be captured (cf.Fajardy et al., 2018), which would result in enhancement of undesirable side-effects from increasing the biomass demand for BECCS. This is important to avoid, since CCS and BECCS are still representing ‘linear’ systems, and are not necessarily promoting a circular economy. It will obviously always be beneficial for the climate that measures are taken to store carbon that would otherwise have been emitted to the atmosphere. However, with a scarcity of climate-neutral carbon atoms, combustion processes with heat losses are not favorable and, thus, losses should at least be minimized.
The SOU2020:4 inquiry also proposes that the reverse auction could be opened to other CDR technologies, although it is concluded that these are currently technically immature and, therefore, of lesser relevance. Nevertheless, there are already initiatives6 that attempt to stimulate interest in DACCS concepts, primarily for hard-to-abate sectors. Although DACCS is considered to be almost an order of magnitude more expensive than conventional CCS (and BECCS), see Gambhir and Tavoni (2019) and references therein, it has the advantage of being more flexible with respect to location and can be installed close to storage units (although to take advantage of storage in the vicinity of the DACCS plants, the storage needs to be onshore or by a coastline near the offshore storage unit). A recent review of DACCS technologies (Fasihi et al., 2019) points to that capture costs with DACCS can come down to 100 €/tCO2 or less, which seems surprisingly low considering that capture is performed at a concentration of little more than 400 ppm CO2, as opposed to CCS and BECCS which capture CO2 at percent levels (typically 5–20% depending on the type of emission source). In some locations, such as in Iceland, geothermal (free) heat is available for powering DACCS (of a low-temperature DACCS type, such as the technology proposed by Climeworks; Beuttler et al., 2019), which may reduce costs and, thereby, help to reach the above-mentioned cost. It may very well be that sectors with hard-to-abate emissions may find it worthwhile to invest in DACCS as an independent mitigation option to compensate for their costly residual emissions. In summary, DACCS can be a realistic complement to BECCS, although this would require the technology to be demonstrated at scale and to be shown to bring down the cost.
An alternative or complementary way to finance BECCS is based on the fact that an assumed CCS cost of 100 €/tCO2 will only marginally influence the prices of the end-products. Rootzén and Johnsson (2016) and Rootzén and Johnsson (2017) have estimated the increases in the price of a car and of a building made of CO2-neutral steel and cement and steel, respectively. These materials are rendered CO2-neutral through CCS (as part of a portfolio of measures within which CCS is a substantial component) at a total cost of around 100 €/tCO2. It is shown that this would result in price increases for the car and the building of less than 0.5% (Rootzén and Johnsson, 2016; Rootzén and Johnsson, 2017). This result may open an entry point for voluntarily initiated ‘climate clubs’, whereby companies along key value chains gather to fund CCS (and other abatement options) through some risk-sharing scheme that enables them to provide climate-neutral products. Considering the heated debate on climate change, it is likely that such climate-neutral products will have a role to play, particularly since they only need to be marginally more expensive. A similar approach can be taken for BECCS. The consumer price increase to compensate for hard-to-abate sector emissions or to provide climate-positive end-products, i.e., products for which the associated emissions are offset (or more than offset) by other mitigation actions, may only be small. Klement et al. (2021) have analyzed the value chain of the P&P industry and shown that the cost for BECCS in the production of a number of low-value products, such as oat milk in paper packaging, card boxes and books, would only marginally affect the selling price if they were made of climate-positive paper. It is worth noting that within the food industry, there are already products marketed as climate-positive (e.g., a Swedish hamburger chain and dairy products). Since the climate benefits of many offset programs–often in the form of tree-planting in developing countries - have been criticized, BECCS (and DACCS) may have future roles here, since the climate benefit accrued from the actual storage of CO2 would be hard to dispute in this sense (assuming that leakage can be ruled out).
Societal Acceptance
Concerning the levels of acceptability by the different groups in Sweden (the public, politicians, researchers, industry, etc.), domestic, sustainable biomass supply chains might imply lower barriers to public acceptance than are present in countries with concerns related to inducing indirect land use change, even though empirical evidence to support this hypothesis is still lacking. For the CCS part of the BECCS supply chain, there are also tentative indications that the societies in the Scandinavian countries are more open to deployment than other European countries (Haug and Stigson, 2016). At the same time, it has to be stressed that BECCS is not the preferred mitigation option and that renewables like solar and wind, for example, enjoy stronger public support (Fridahl, 2017). Some parts of the BECCS chain are also subject to conflicting views, especially the expansion of forest biomass production for bioenergy, even though this could change when it is combined with CCS (Fridahl and Lehtveer, 2018). One such view is that this type of strategy could substantially reduce forest carbon stocks due to an increase in clear-cut areas, which emit more CO2 in the decade after harvesting, thereby neutralizing the emissions savings gained from substituting biomass for fossil fuels, with further negative side-effects such as impacts on biodiversity and higher vulnerability to climate change, as the number of tree species is decreased. As a consequence, proponents of this view demand that priority be given to storing carbon in existing forests. Another view opposes this demand, pointing out that the capacity for carbon sequestration in forests declines as they age, and that the carbon could be re-emitted in the case of wildfires, pests and other disturbances, which are likely to occur more frequently as results of ongoing climate change. An additional argument is that it is unlikely that society can quickly transition from using hydrocarbons in different conversion processes, including combustion, which underlines the importance of using renewable carbon, including biomass.
Another argument against the increased use of bioenergy is that it emits CO2. While this is factually true, as long as there is net growth of carbon stocks and a need for carbon-based fuels and feedstocks (i.e., which cannot easily be replaced by renewable electricity), this argument is questionable (for a discussion, see Berndes et al., 2018). It is reasonable to assume that this argument will be further weakened for cases in which biomass is combined with CCS. An attempt to understand and reconcile these views of the role of forests in climate is elaborated by Berndes et al. (2018).
A recent study of public perception of BECCS carried out in the United Kingdom has also shown that the choice of policy instrument for incentivizing BECCS has an impact on the level of acceptance, in that payments for removal appear to be preferred over price guarantees for producers who are selling energy derived from BECCS (Bellamy et al., 2019). This fits with other studies that identify the framing as a critical factor in how society responds to BECCS technologies (Gough and Mander, 2019).
For a more comprehensive assessment of BECCS acceptance in Sweden, studies that directly target the Swedish public and other actors need to be conducted. There is a paucity of empirical evidence in this area, especially covering the full BECCS chain. Polling public attitudes to different mitigation technologies is difficult in terms of what the results from such polls will mean in an actual siting situation. However, since the application of BECCS at existing plants will not–in contrast to, for example, wind power–require new industrial sites to be developed, one may expect less public resistance than there would be to technologies requiring new siting. In particular, this will be true if applying BECCS to coastal emission sources in combination with ship transport, for which there will be no need to locate new pipelines. Acceptance will be inextricably linked also to how carbon removal will be governed.
Governance and Regulatory “Readiness”
As mentioned above, it still remains unclear as to how carbon removal originating from bioenergy generation with CCS will be accounted for, both at the national and EU levels. Monitoring, reporting and verification (MRV) would be transnational for some parts of the chain (e.g., as the storage will likely take place in Norway), giving rise to liability issues, as well as potentially raising geopolitical concerns due to the increased dependence on the foreign availability of storage space. Under scenarios of increased BECCS targets, good governance would also need to acknowledge the increased competition for biomass, which is also used for non-mitigation purposes. This connects to the need for a holistic governance that takes into account not only climate targets, but also a broader set of SDGs. Fuhrman and colleagues (Fuhrman et al., 2019) have identified overlaps with other SDGs in the context of CDR, which are not systematically assessed in the current IAM literature (Bioenergy Combined With Carbon Capture and Storage in the 1.5°C pathways).
As for the regulatory framework, there do not appear to be any major barriers to the storage of captured Swedish CO2 emissions in Norway, although a bilateral agreement must be set up within a provisional amendment (from 2009) to the London Protocol. A resolution that allows provisional application of the 2009 amendment has recently been set up jointly by Norway and the Netherlands (International Maritime Organization, 2019) and accepted, removing the barrier to cross-border export of CO2 for offshore storage. This allows the six countries that have accepted the amendment to use the 2009 amendment provisionally. This should hold for all countries, including Sweden, provided a Declaration is made.
Table 2 summarizes the assessment of opportunities and barriers for BECCS deployment in Sweden, along with the underlying evidence for the assessment.
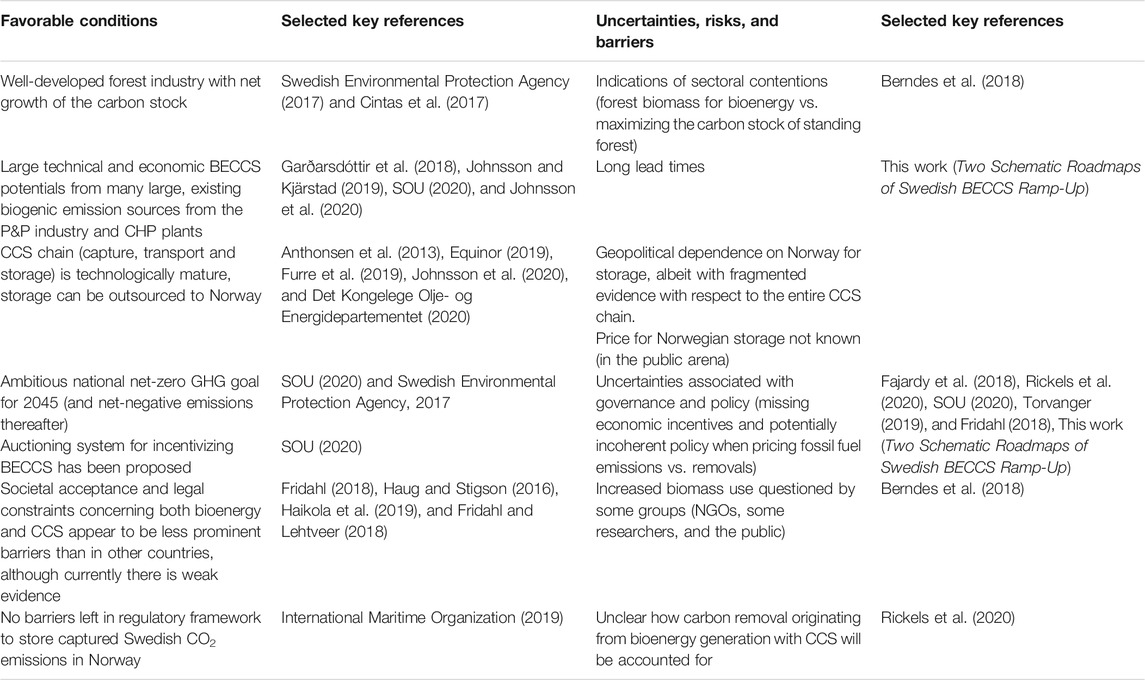
TABLE 2. Conclusions from case study–opportunities and uncertainties, risks and barriers for BECCS deployment in Sweden.
Two Schematic Roadmaps of Swedish BECCS Ramp-Up.
It is challenging to estimate a realistic timeline for the deployment of CCS and BECCS in Sweden and compare it to the ambition outlined in SOU2020:4. Therefore, we have resorted to designing two scenarios, which depend mainly on assumptions concerning the timeline of adding capture to plants, which are either existing or in the process of being built, while both assume sufficient incentives and no barriers to connecting to Norwegian geologic storage units. As mentioned previously, it can be argued that BECCS is based on proven technologies–if referring to post-combustion capture with amine-based capture, which is commercially available (Bui et al., 2018; Los Alamos National Laboratory, 2019). Post-combustion capture has been applied in the chemical industry for a long time, albeit for purposes other than CO2 storage. With respect to the use of CCS, in 2019 there were 19 large-scale (>400 ktCO2 yearly capture7) plants globally, with a further four plants under construction (The Global CCS Institute, 2019). Transport and storage have been proven at large scale and, for example, large-scale storage (around 1 MtCO2 per year) has been carried out by the Norwegian natural gas industry in the North Sea since 1996. Thus, based on practical experience gained from the above as well as the authors’ discussions with industry it can be argued that CCS is at a high technology readiness level (TRL), i.e., 8 or 9. Yet, applying CCS to new processes, such as those in the P&P industry, can be expected to be associated with long lead times, including the need for large-scale demonstration prior to commercial projects. In general, few studies in the literature have addressed the TRLs of CO2 capture, including post-combustion capture, and the works that are available were completed before most of the above-mentioned large-scale projects were put in operation and, thus, generally point to lower TRLs (see Rubin et al., 2012 and references therein).
Assuming that the two Norwegian projects discussed in The economic potential will be approved during 2020, it will take at least three years until they are fully operational (and possibly only the cement plant will be completed within this time frame, if the CHP plant fails to raise the required co-funding). In fact, the cement plant targets 2024 as the start-up year. When it comes to the cement industry, it seems reasonable to assume that the Norwegian capture project will need to be evaluated before capture will be installed at the Swedish cement plant. The main reason for this is that the Norwegian and Swedish cement plants have the same owner (Heidelberg Cement). As for CHP, there is also a small pilot project at Stockholm Exergi–the main utility for delivering heat within Stockholm–and this company has a target of having full-scale capture from their Stockholm Värtan plant by 2024 at the earliest (Levihn, 2020), although we assume that this is conditional on governmental support (such as that mentioned above). Thus, an optimistic scenario is that there will be capture at this plant by 2024 and at the Swedish cement plant some years later (assuming that the experiences from the Norwegian cement plant are in line with expectations). The Preem refinery on the West coast of Sweden has started to work with CCS, with the aim to apply CCS to their hydrogen production, which is part of the refinery process. Their level of hydrogen production will increase with their ambition to use more biogenic feedstock in the refinery. So far, they are–similar to Stockholm Exergi–operating a small pilot plant. Thus, the aspiration to have 3–5 full-scale BECCS plants (1.8 Mt per year) in operation by 2030, as is proposed in SOU2020:4, seems highly optimistic, albeit not impossible. It will require that at least two of these plants will be built in parallel and that there will be a more or less immediate “kick-start” of a coordinated BECCS program. However, considering the above-mentioned difference between the value of negative emissions required for a kick-start of BECCS and the lower cost of emitting fossil fuel CO2, an additional support scheme will most likely be required–probably for CCS and BECCS concomitantly (in addition to other measures to achieve rapid and deep reductions of large point source emissions).
There are, according to 2016 data from the Swedish Environmental Protection Agency, 36 energy plants and 27 P&P plants with biogenic emissions that individually exceed 100 kt/year.8 It should be mentioned that several of the CHP plants (the waste plants) burn a mix of biogenic and fossil fuels, increasing the total flue gas flow. The major share of the emissions from the P&P plants is biogenic, and these have generally large biogenic emissions, with 20 plants having emissions exceeding 500 kt/year (as mentioned above and analyzed by Johnsson et al., 2020). Other emissions sources, which in the future may contribute to negative emissions, are refineries and cement plants (three refineries and two cement plants), which are currently increasing their shares of biogenic feedstocks. Thus, their net emissions may become negative when applying CCS, depending on both the capture rate and biogenic feedstock share. In any case, there are sufficient numbers of biogenic emissions sources for BECCS to play a significant role, as also described in The economic potential.
The two roadmap scenarios focus on P&P plants and energy (CHP and heat-only) plants, since these constitute the major share of the current Swedish biogenic emissions sources. Both roadmaps assume that incentives for capturing biogenic emissions are in place, e.g., resulting from the proposed auctioning system proposed in SOU2020:4. In addition, as mentioned above, there are plans to apply capture to cement plants and refineries, although these currently use fossil feedstocks. At what rate and to what extent these plants can change to biogenic feedstocks is not known (and for the cement plant this will obviously only be possible for the fuel-related emissions). Nonetheless, in principle, these industries could also eventually attain net-negative carbon emissions.
At present, there are around 7–8 (chemical) P&P plants emitting more than 1,000 ktCO2/year and around 5 CHP plants with biogenic emissions exceeding 500 kt/year, and a similar number of plants emitting around 300–400 ktCO2/year. Since P&P plants have several stacks (with the major part of emissions originating at the recovery boiler), we assume an average capture of 600 ktCO2/year from each plant. Similarly, we assume that on average 400 ktCO2/year are captured from each CHP plant. This provides a rough estimate of BECCS ramp-up in Sweden. It is likely that this overestimates the initial development but, provided large-scale BECCS implementation takes off, it may underestimate the development toward 2045.
Based on current plans, it is assumed that it will be possible to start up the first CHP BECCS plant in 2024 (i.e., the Värtan Plant in Stockholm). For P&P power, there are fewer concrete plans and, thus, it is assumed that the first plant cannot be expected to be put in operation until late in this decade (here assuming 2027). For each industry category (P&P and CHP), it is assumed that it will take 5 years from when the first full-scale plant is put into operation until the second plant can be put into operation, owing to the time required to evaluate the operation of the first plant (i.e., the first plant is assumed to correspond to a large-scale demonstration plant for gaining experience of the whole BECCS chain: capture, transport and storage). In the optimistic scenario (Figure 3A), there is on average a 2.5-years lag between the projects that follow the first project, whereas in the less-optimistic scenario (Figure 3B) this lag is assumed to average 5 years Figure 3 gives the results from the roadmap analysis, and it can be concluded that compared to the targets proposed in SOU 2020:4, the 2030 BECCS target seems to be challenging given the above assumptions, whereas the 2045 target seems likely to be within reach in both scenarios if applying the lower boundary of 3 MtCO2/year, but obviously provided that initial ramp-up is successful.
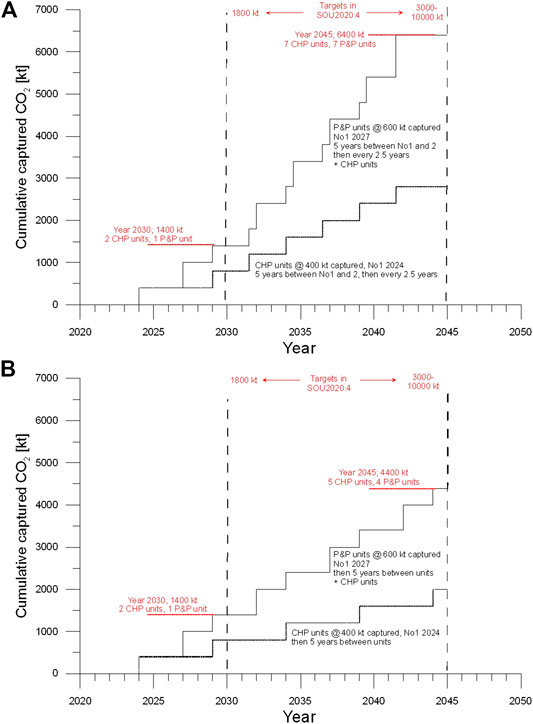
FIGURE 3. Two schematic roadmaps for the development of full-scale BECCS projects in Sweden. These roadmaps assume the application of capture in P&P and CHP plants. The roadmaps apply the target values proposed in SOU (2020):4; 1.8 MtCO2 captured is expected to result from the auctioning system by 2030, and the indicative target is to capture 3–10 MtCO2 by 2045. (A) An optimistic scenario; (B) a less-optimistic scenario.
Both scenarios obviously require a much stronger climate policy than what is currently in place, including incentives for capturing biogenic emissions (such as the reversed-auctioning system proposed in SOU2020:4). The above analysis is restricted to existing plants. Although it should be a fair assumption that most of the biogenic emission sources, which may be equipped with capture plants, will be existing plants, some biogenic fuel production processes may be added. The latter will, in turn, depend on the development of the refinery industry and on their ability to transform so as to provide advanced biofuels for road, aviation and maritime transportation.
If the proposed BECCS targets were to be met solely by capturing CO2 from the biogenic emissions from the present CHP units (Figure 3), this would require capture at three plants for the 2030 target (1.8 Mt/year). The 2045 target would require capture in at least five plants for the lower range given (3 Mt per year) and would basically require all large-scale CHP units to reach the upper level of removal, i.e., 10 Mt/year. If instead only P&P units are targeted, it would be sufficient to conduct capture at one or two of the largest plants for the 2030 target to be met and at three to nine plants for the range given for the 2045 target (3–10 Mt/year). These figures all assume 90% capture and cumulative addition of the captured CO2, starting from the largest unit. Capture would most likely be applied to a mix of CHP and P&P units, as assumed in the two scenarios depicted in Figure 3.
BOX It should be technically possible to scale up BECCS to reach the recently proposed targets for 2030 and 2045 provided that the following conditions are met:
1) Action is started immediately (i.e. in 2020) for first full-scale implementation before 2025;
2) Current small-scale demonstration projects are successful in terms of technology performance;
3) The necessary incentives are put in place, i.e. incentives for mitigating biogenic emissions at a level in line with the cost of BECCS (∼100 €/ton CO2)
4) The Northern Lights Project is successful during the next few years and the necessary permits are secured;
5) Capacity and knowledge building progresses to control costs and obtain reliable capture processes; and
6) Transparent communication channels are established to deal with issues concerning public and social acceptance.
In summary, the assessment of possible ramp-up of BECCS in Sweden shows that there is an urgent need to start large-scale implementation of BECCS if the targets proposed in the public inquiry (SOU 2020:4) are to be met. Most critical is to meet the 2030 target for BECCS, whereas the 2045 target should be attainable, provided that actions are instituted immediately for first implementation before 2025 and that implementation is thereafter continuously ramped up over the entire period up to 2045. These conditions and the other conditions identified as necessary in this paper are summarized in the box.
Conclusion
When the IPCC responded to the invitation to assess what we know and do not know about reaching a temperature goal of 1.5°C global warming above pre-industrial levels after the Paris Agreement, only a handful of 1.5°C scenarios were available, reflecting a heavy dependence on carbon removal through BECCS (Rogelj et al., 2015). During the course of the assessment, more scenarios became available–partially in response to concerns raised regarding the adverse side effects of a large-scale rollout of BECCS. On the one hand, this expanded the scenario space by excluding CCS from the mitigation mix (e.g., Grubler et al., 2018) and, on the other hand, it led to the exploration of CO2 mitigation through demand reductions and lifestyle changes (e.g., van Vuuren et al., 2018). Still, carbon removal plays an important role in all of the pathways assessed by the IPCC (2018b), and as countries move toward formulating their own net-zero emission goals, they look to global pathways for information that these pathways are not necessarily designed to provide.9 As key needs for national roadmaps and policy design, we identify greater granularity at the technology and supply chain levels, geologic storage suitability, and feasibility with respect to interactions with other SDGs. In addition, there needs to be consideration of the current and envisaged policy mixes, which are also influenced by the political economy and other non-climate factors. More scenarios to test the impacts of different governance approaches and legal constraints are required. Finally, co-design and participatory approaches should be considered to capture bottlenecks and opportunities associated with social acceptance. While such participatory approaches are often called for (e.g., Cox et al., 2018) in the context of BECCS deployment, there are doubts that this is compatible with expensive, technologically advanced CDR proposals, which are typically non-participatory and centralized (McLaren, 2016). With these caveats in mind, there are some experiences gained from other activities, such as participatory integrated assessments of the social acceptance of wind energy (Scherhaufer et al., 2018), which could at least conceptually serve as a template. Future research should look into possible analogues in the context of mitigation to allow for the development and refinement of such approaches.
To assess the extent to which the top-down expectations of BECCS can be fulfilled, there is an urgent need for national bottom-up assessments that include evaluations of the technical, economic, policy and social aspects. Such an analysis should as a first step focus on regions with well-developed biomass markets, such as those originating from forest industries. Here, Sweden is used as an example, on the basis that it has a well-developed forest industry and many large, existing biogenic emission sources from the P&P industry and CHP plants. These conditions, combined with Sweden’s ambitious goal of reaching net-zero GHG emissions by 2045 and becoming a net-negative emitter thereafter, clearly indicate a role for carbon removal in the Swedish climate change mitigation mix. In this study, we demonstrate that Sweden has considerable potential for removing CO2 by capturing biogenic emissions and storing them geologically. Despite the favorable conditions, we identify an implementation gap. An analysis of the factors that inhibit BECCS implementation along the dimensions of technology and systems, governance, economics and policy, and acceptability shows that–discrepant with commonly held beliefs–the most substantial barriers are not of a technological nature. Indeed, the components of BECCS are technologically mature, the forest management systems for producing Swedish biomass are long-established, and productive and sustainable post-combustion capture has entered higher TRLs, although experience from larger-scale application at Swedish emission sources is lacking. Moreover, the transport of CO2 is a known and commercially available technology, and storage is being outsourced to Norway, which has a long-time practical experience. Unlike other European countries, societal acceptance, legal constraints and political uneasiness with respect to both bioenergy and CCS seem to be less prominent barriers, even though the assessment in Incentives shows very clearly that knowledge remains too fragmented to draw robust conclusions on this issue. In addition, contentions can be identified when zooming into the different components of the BECCS chain, as exemplified by the controversy related to expanding biomass supply from forestry vs. maximizing the carbon stock of the standing forest or the geopolitical uncertainties associated with the dependence on Norway for geologic storage, as discussed in detail in Incentives. It has to be stressed that regarding social preference, the literature is sparse and there is a need for targeted Swedish surveys to attain a more refined understanding of whether any of these issues could be show-stoppers and to determine whether the impacts of other aspects have been underestimated.
Major uncertainties remain regarding the dimensions of governance, economics and policy that lead to disincentives for implementing BECCS. An important policy aspect is discovering a way to establish coherence between fossil fuel pricing and valuing carbon removal, while still offering enough support to close the implementation gap in a rapidly closing time-window. This is also important, since a criticism that has been directed toward BECCS is that it could postpone efforts to reduce fossil fuel emissions (Minx et al., 2018). Therefore, a coherent policy is needed for fostering the acceptability of BECCS in a more fundamental manner. Estimates of two Swedish roadmap scenarios for ramping up BECCS to meet recently proposed targets for 2030 and 2045 indicate that it should be possible to meet the targets, although this will require: 1) urgent action; 2) that the current small-scale demonstration projects will be successful; 3) that the necessary incentives are put in place; and 4) that the Norwegian plans to develop a storage infrastructure are successfully executed during the next few years (the Northern Lights Project) and that the necessary permits are secured. In addition, there will be a need for: 5) capacity and knowledge building to control costs and obtain reliable capture processes, which is likely to depend on the international diffusion of CCS and BECCS technologies; and 6) transparent communication to deal with emerging issues concerning acceptance of the technology.
In light of the prominent role of BECCS in global scenarios that limit warming to well below 2°C and the maturity of the BECCS technology, the main implications from this analysis are that–despite sustainability concerns about a large-scale, global BECCS rollout - there is a strong need for national bottom-up assessments of possible roadmaps for BECCS, which consider emission sources, transport and storage infrastructure, social acceptance, and economy-wide and environmental effects. From this follows the recommendation that, provided the national analysis identifies a reasonably strong potential for sustainable BECCS, economic incentives should be put in place, such as the reverse-auctioning system proposed for Sweden. Furthermore, comprehensive policy packages need to account for the high value of forestry-derived products and the potentially increased competition between sectors (for which biomass can be a mitigation option), most notably the transportation, chemicals and energy sectors. In addition, investments will be delayed if policy uncertainties are not resolved, so a clear commitment to BECCS implementation needs to be signaled to investors, who–under the current premise of first needing to seek EU support, the materialization of which is not clear–face significant uncertainty with respect to their planning. This will be the case unless the proposed auctioning system is indeed implemented, mitigating the difference between any EU support and the cost for BECCS. In the first place, this will require harmonization of definitions, accounting and governance. Finally, a pathway that involves transformative investments is vulnerable to unforeseen events, as experienced during the current economic crisis associated with the COVID-19 pandemic. Policy packages must be stress-tested for external shocks, to give robust signals to investors.
This study has taken the first step toward demonstrating that additional analyses at the national level are needed to close the implementation gap that we observe when comparing climate ambition with actual CDR deployment (in this case, BECCS). While the global pathways offer a sound basis for the UNFCCC debate and an understanding of the different avenues to reach ambitious global temperature goals, more analysis in the context of individual countries is needed, as they move toward their own net-zero emissions goals and the implementation thereof.
Whether or not the lessons learned from the Swedish case study can readily be generalized to other countries remains to be seen. Evidently, countries with similar conditions are more likely to learn from this case study. How similar the countries and their situations are will be difficult to determine without further research. Technical potentials might be more readily available and assessable than insights into current industry and policy processes, and societal acceptance (Nemet et al., 2018). With this caveat in mind, there are several insights that we want to flag as particularly useful for other countries’ net-zero considerations: 1) global studies can provide general guidance on the required ramp-up, although they may disregard local opportunities and tradeoffs and should, thus, be accompanied by national analyses without excluding options that are contentious (or not) at Gigatonne-scale; 2) while BECCS is based on comparatively mature technologies, it will require immediate political and economic incentives to be ramped up sufficiently quickly in countries that feature sustainable and socially acceptable BECCS pathways; 3) adopting a value chain perspective and looking more into new “climate-positive” products linked to BECCS can open up alternative or complementary economic entry points to technology rollout; 4) societal preference is as important as technical feasibility when moving to implementation, yet this is where the largest knowledge gaps arise in the context of BECCS and CDR, more generally; and 6) the rapidly closing time-window for reaching the ambitious Paris Agreement targets may call for hybrid approaches to governance, whereby local BECCS ramp-up could proceed more readily while cross-jurisdictional issues (e.g., related to the sustainability of biomass imports or accounting for emissions removal) could be governed at higher levels. This would also apply to other CDR approaches that might prove more amenable in other countries.
Data Availability Statement
Publicly available datasets were analyzed in this study. This data can be found here: https://data.ene.iiasa.ac.at/iamc-1.5c-explorer/; https://doi.org/10.1016/j.ijggc.2018.06.022; https://research.chalmers.se/en/publication/509912. The data on the IPCC SR1.5 pathways are available at IIASA’s scenario explorer.
Author Contributions
SF and FJ conceived the study together. SF led the scenario and implementation gap analysis, while FJ led the work on the Swedish case. Both authors discussed and interpreted the results and jointly wrote the paper.
Funding
SF has conducted this work in the frame of her Jubilee Professorship at Chalmers University and the project ‘Comparative assessment and region-specific optimization of GGR’ under grant reference NE/P019900/1 funded by the Natural Environment Research Council of the United Kingdom. Filip Johnsson conducted this work with co-funding from Vinnova and the Swedish Energy Agency.
Disclaimer
Frontiers Media SA remains neutral with regard to jurisdictional claims in published maps and institutional affiliations.
Conflict of Interest
The authors declare that the research was conducted in the absence of any commercial or financial relationships that could be construed as potential conflicts of interest.
The handling editor declared a past co-authorship with one of the authors SF.
Acknowledgments
The work has been carried out as part of Sabine Fuss’ Jubilee Professorship at Chalmers University and has benefitted greatly from discussions with colleagues at the department. We acknowledge the inspiring comments received from participants in the Mistra Carbon Exit Seminar “Bioenergy with Carbon Capture and Storage–opportunities and challenges” in Stockholm on October 23rd, 2019.
Footnotes
1Note, however, that recent work examining the impact of a larger roll-out of DACCS still finds an important role for BECCS in the mitigation portfolio (Fuhrman et al., 2020).
2Sweden’s total GHG emissions were 53 MtCO2 in 2018.
3In the Swedish Climate Political framework of 2017 only BECCS is mentioned as possible CDR avenue. In the Governmental Inquiry (SOU, 2020) it is proposed that negative emissions can also be obtained by means of other technical measures which are verifiable.
4Based on the 80–135 €/ton CO2 cost range given by Johnsson et al. (2020).
5The figure will depend strongly on parameters such as the relation between CAPEX and OPEX, depreciation rate and should only be seen as an approximative upper value.
6The Nordic DAC Group is an initiative that promotes Direct Air Capture in Sweden and the Nordic countries: www.nordicdacgroup.com/.
7Large scale: At least 400 ktCO2 annually for industrial capture and at least 800 ktCO2 for a coal power plant capture.
8100 ktCO2/year is arbitrarily chosen as the lower limit for CCS to limit the specific capture cost (€/tCO2), although, as mentioned above, the first projects would probably have to target larger emission sources.
9Looking into the future, greater sectoral detail is foreseen to emerge in the literature. This could facilitate progress on reconciling the top-down modeling with the bottom-up analysis.
References
Andrijevic, M., Schleussner, C.-F., Gidden, M. J., McCollum, D. L., and Rogelj, J. (2020). COVID-19 recovery funds dwarf clean energy investment needs. Science 370, 298–300. doi:10.1126/science.abc9697 | CrossRefFull Text
Anthonsen, K. L., Aagaard, P., Bergmo, P. E. S., Erlström, M., Fareide, J. I., Gislason, S. R., et al. (2013). CO2 storage potential in the Nordic region. Energy Procedia. 37, 5080–5092. doi:10.1016/j.egypro.2013.06.421 CrossRefFull Text
Baker, S. E., StolaroffPeridas, J. K. G., Pang, S. H., Goldstein, H. M., Lucci, F. R., Li, W., et al. (2020). Getting to neutral: options for negative emissions in California, Available at https://livermorelabfoundation.org/2019/12/19/getting-to-neutral/, accessed April 2020.CrossRefFull Text
Bellamy, R., and Geden, O. (2019). Govern CO2 removal from the ground up. Nat. Geosci. 12, 874–876. doi:10.1038/s41561-019-0475-7 CrossRefFull Text
Bellamy, R., Lezaun, J., and Palmer, J. (2019). Perceptions of bioenergy with carbon capture and storage in different policy scenarios. Nat. Commun. 10, 743. doi:10.1038/s41467-019-08592-5 | CrossRefFull Text
Berndes, G., Goldmann, M., Johnsson, F., Lindroth, A., Wijkman, A., Abt, B., et al. (2018). Forests and the climate, KSLA, Stockholm. CrossRefFull Text
Beuttler, C., Charles, L., and Wurzbacher, J. (2019). The role of direct air capture in mitigation of anthropogenic greenhouse gas emissions. Front. Clim. 1, 10. doi:10.3389/fclim.2019.00010 CrossRefFull Text
Bui, M., Adjiman, S., Bardow, A., Anthony, E. J., Boston, A., et al. (2018). Carbon capture and storage (CCS): the way forward. Energy Environ. Sci. 11, 1062–1176. doi:10.1039/c7ee02342a CrossRefFull Text
Butnar, I., Broad, O., Solano Rodriguez, B., and Dodds, P. E. (2020). The role of bioenergy for global deep decarbonization: CO2 removal or low-carbon energy?. GCB Bioenergy 12, 198–212. doi:10.1111/gcbb.12666 CrossRefFull Text
Cintas, O., Berndes, G., Hansson, J., Poudel, B. C., Bergh, J., Börjesson, P., et al. (2017). The potential role of forest management in Swedish scenarios towards climate neutrality by mid century. For. Ecol. Manage. 383, 73–84. doi:10.1016/j.foreco.2016.07.015 CrossRefFull Text
Clarke, L., Jiang, K., Akimoto, K., Babiker, M., Blanford, G., Fisher-Vanden, K., et al. (2014). Assessing transformation pathways. In “Climate Change 2014: Mitigation of Climate Change. Contribution of Working Group III to the Fifth Assessment Report of the Intergovernmental Panel on Climate Change,” (editors: Edenhofer, O. et al) (pp. 413–510). Cambridge University Press.CrossRefFull Text
Committee on Climate Change (2019). Net Zero: the UK’s contribution to stopping global warming. Available at: https://www.theccc.org.uk/publication/net-zero-the-uks-contribution-to-stopping-global-warming/%0Awww.theccc.org.uk/publications, accessed 1 April 2020. CrossRefFull Text
Cox, E. M., Pidgeon, N., Spence, E., and Thomas, G. (2018). Blurred lines: the ethics and policy of greenhouse gas removal at scale. Front. Environ. Sci. 6, 38. doi:10.3389/fenvs.2018.00038 CrossRefFull Text
Creutzig, F., Popp, A., Plevin, R., Luderer, G., Minx, J., and Edenhofer, O. (2012). Reconciling top-down and bottom-up modelling on future bioenergy deployment. Nat. Clim. Change, doi:10.1038/nclimate1416 CrossRefFull Text
Creutzig, F., Ravindranath, N. H., Berndes, G., Bolwig, S., Bright, R., Cherubini, F., et al. (2015). Bioenergy and climate change mitigation: an assessment. GCB Bioenergy 7, 916–944. doi:10.1111/gcbb.12205 CrossRefFull Text
Davis, S. J., Lewis, N. S., Shaner, M., Aggarwal, S., Arent, D., Azevedo, I. L., et al. (2018). Net-zero emissions energy systems. Science 360, eaas9793. doi:10.1126/science.aas9793 | CrossRefFull Text
de Coninck, H., Revi, A., Babiker, M., Bertoldi, P., Buckeridge, M., Cartwright, A., et al. (2018). “Strengthening and implementing the global response,” in Global Warming of 1.5°C: An IPCC Special Report on the impacts of global warming of 1.5°C above pre-industrial levels and related global greenhouse gas emission pathways, in the context of strengthening the global response to the threat of climate change, sustainable development, and efforts to eradicate poverty. Editors V. Masson-Delmotte, P. Zhai, H.-O. Pörtner, D. Roberts, J. Skea, P. R. Shukla, A. Pirani, W. Moufouma-Okia, C. Péan, R. Pidcock, S. Connors, J. B. R. Matthews, Y. Chen, X. Zhou, M. I. Gomis, E. Lonnoy, T. Maycock, M. Tignor, and T. Waterfield. In Press. CrossRefFull Text
Det Kongelege Olje- og Energidepartementet (2020). Meld. St. 33 langskip–fangst og lagring av CO2. 33. Available at: https://www.regjeringen.no/contentassets/943cb244091d4b2fb3782f395d69b05b/nn-no/pdfs/stm201920200033000dddpdfs.pdf. CrossRefFull Text
Equinor (2019). Northern lights project concept report. Available at: https://northernlightsccs.com/assets/documents/Northern-Lights-Project-Concept-report.pdf, (Accessed September 1, 2020). CrossRefFull Text
Fajardy, S. M., Dowell, N. M., Fajardy, M., and DowellMac, N. (2018). The energy return on investment of BECCS : is BECCS a threat to energy security ?. Energy Environ. Sci. 11, 1581–1594. doi:10.1039/C7EE03610H CrossRefFull Text
Fasihi, M., Efimova, O., and Breyer, C. (2019). Techno-economic assessment of CO2 direct air capture plants. J. Clean. Prod. 224, 957–980. doi:10.1016/j.jclepro.2019.03.086 CrossRefFull Text
Forster, J., Vaughan, N. E., Gough, C., Lorenzoni, I., and Chilvers, J. (2020). Mapping feasibilities of greenhouse gas removal: key issues, gaps and opening up assessments. Global Environ. Change. 63, 102073. doi:10.1016/j.gloenvcha.2020.102073 CrossRefFull Text
Fridahl, M. (2017). Socio-political prioritization of bioenergy with carbon capture and storage. Energy Pol. 104, 89–99. doi:10.1016/J.ENPOL.2017.01.050 CrossRefFull Text
Fridahl, M. (2018). Incitamentsstrukturer för bioenergi med koldioxidavskiljning och -lagring i Sverige och Europeiska Unionen linköping/stockholm. Available at: http://liu.diva-portal.org/smash/get/diva2:1335389/FULLTEXT01.pdf. CrossRefFull Text
Fridahl, M., and Lehtveer, M. (2018). Bioenergy with carbon capture and storage (BECCS): global potential, investment preferences, and deployment barriers. Energy Res. Soc. Sci. 42, 155–165. doi:10.1016/j.erss.2018.03.019 CrossRefFull Text
Fuhrman, J., McJeon, H., Doney, S. C., Shobe, W., and Clarens, A. F. (2019). From zero to hero?: why integrated assessment modeling of negative emissions technologies is hard and how we can do better. Front. Clim. 1, 11. doi:10.3389/fclim.2019.00011 CrossRefFull Text
Fuhrman, J., McJeon, H., Patel, P., Doney, S. C., Shobe, W. M., and Clarens, A. F. (2020). Food–energy–water implications of negative emissions technologies in a +1.5 °C future. Nat. Clim. Change. 10, 920–927. doi:10.1038/s41558-020-0876-z CrossRefFull Text
Furre, A. K., Meneguolo, R., Ringrose, P., and Kassold, S. (2019). Building confidence in CCS: from sleipner to the Northern Lights project. First Break. 37, 81–87. doi:10.3997/1365-2397.n0038 CrossRefFull Text
Fuss, S., Canadell, J. G., Ciais, P., Jackson, R. B., Jones, C. D., Lyngfelt, A., et al. (2020). Moving toward net-zero emissions requires new alliances for carbon dioxide removal. One Earth. 3, 145–149. doi:10.1016/j.oneear.2020.08.002 CrossRefFull Text
Fuss, S., Flachsland, C., Koch, N., Kornek, U., Knopf, B., and Edenhofer, O. (2018a). A framework for assessing the performance of cap-and-trade systems: insights from the European Union emissions trading system. Rev. Environ. Econ. Pol. 12, 220–241. doi:10.1093/reep/rey010 CrossRefFull Text
Fuss, S., Lamb, W. F., Callaghan, M. W., Hilaire, J., Creutzig, F., Amann, T., et al. (2018b). Negative emissions - Part 2: costs, potentials and side effects. Environ. Res. Lett. doi:10.1088/1748-9326/aabf9f CrossRefFull Text
Gambhir, A., Butnar, I., Li, P. H., Smith, P., and Strachan, N. (2019). A review of criticisms of integrated assessment models and proposed approaches to address these, through the lens of BECCs. Energies 12, 1–21. doi:10.3390/en12091747 CrossRefFull Text
Gambhir, A., and Tavoni, M. (2019). Direct air carbon capture and sequestration: how it works and how it could contribute to climate-change mitigation. One Earth. 1, 405–409. doi:10.1016/j.oneear.2019.11.006 CrossRefFull Text
Garðarsdóttir, S. Ó., Normann, F., Skagestad, R., and Johnsson, F. (2018). Investment costs and CO2 reduction potential of carbon capture from industrial plants – a Swedish case study. Int. J. Greenh. Gas Control. 76, 111–124. doi:10.1016/j.ijggc.2018.06.022 CrossRefFull Text
Gough, C., and Mander, S. (2019). Beyond social acceptability: applying lessons from CCS social science to support deployment of BECCS. Curr. Sustain. Energy Rep. 6, 116–123. doi:10.1007/s40518-019-00137-0 CrossRefFull Text
Grubler, A., Wilson, C., Bento, N., Boza-Kiss, B., Krey, V., McCollum, D. L., et al. (2018). A low energy demand scenario for meeting the 1.5 °C target and sustainable development goals without negative emission technologies. Nat. Energy 3, 515–527. doi:10.1038/s41560-018-0172-6 CrossRefFull Text
Gustafsson, K. (2018). “Spear heading negative emissions in stockholm’s multi-energy system,” in Bioenergy with carbon capture and storage: from global potentials to domestic realities. Editor M. Fridahl (Brussels:European Liberal Forum), 69–87. CrossRefFull Text
Haikola, S., Hansson, A., and Fridahl, M. (2019). Map-makers and navigators of politicised terrain: expert understandings of epistemological uncertainty in integrated assessment modelling of bioenergy with carbon capture and storage. Futures 114, 102472. doi:10.1016/j.futures.2019.102472 CrossRefFull Text
Haug, J. K., and Stigson, P. (2016). Local acceptance and communication as crucial elements for realizing CCS in the Nordic region. Energy Procedia. 86, 315–323. doi:10.1016/j.egypro.2016.01.032 CrossRefFull Text
Hepburn, C., Adlen, E., Beddington, J., Carter, E. A., Fuss, S., Mac Dowell, N., et al. (2019). The technological and economic prospects for CO2 utilization and removal. Nature 575, 87–97. doi:10.1038/s41586-019-1681-6 | CrossRefFull Text
Honegger, M., and Reiner, D. (2018). The political economy of negative emissions technologies: consequences for international policy design. Clim. Pol. 18, 306–321. doi:10.1080/14693062.2017.1413322 CrossRefFull Text
IEA (2017). World energy outlook 2020. Paris: IEA Available at: https://www.iea.org/reports/world-energy-outlook-2017 | CrossRefFull Text
International Maritime Organization (2019). The 41st consultative meeting of contracting parties to the London Convention and 14th meeting of contracting parties to the London Protocol. Available at: https://www.imo.org/en/MediaCentre/MeetingSummaries/Pages/LC-41-LP-14-.aspx, (Accessed September 1, 2020).CrossRefFull Text
IPCC (2018a). “Summary for policymakers,” in: Global Warming of 1.5°C: An IPCC Special Report on the impactsof global warming of 1.5°C above pre-industrial levels and related global greenhouse gas emission pathways, in the context of strengthening the global response to the threat of climate change, sustainable development, and efforts to eradicate poverty. Editors V. Masson-Delmotte, P. Zhai, H.-O. Pörtner, D. Roberts, J. Skea, P. R. Shukla, A. Pirani, W. Moufouma-Okia, C. Péan, R. Pidcock, S. Connors, J. B. R. Matthews, Y. Chen, X. Zhou, M. I. Gomis, E. Lonnoy, T. Maycock, M. Tignor, and T. Waterfield. In Press.CrossRefFull Text
IPCC (2018b). “Summary for policymakers,” in: Global Warming of 1.5°C: An IPCC Special Report on the impacts of global warming of 1.5°C above pre-industrial levels and related global greenhouse gas emission pathways, in the context of strengthening the global response to the threat of climate change, sustainable development, and efforts to eradicate poverty. Editors V. Masson-Delmotte, P. Zhai, H.-O. Pörtner, D. Roberts, J. Skea, P. R. Shukla, A. Pirani, W. Moufouma-Okia, C. Péan, R. Pidcock, S. Connors, J. B. R. Matthews, Y. Chen, X. Zhou, M. I. Gomis, E. Lonnoy, T. Maycock, M. Tignor, and T. Waterfield. In Press.CrossRefFull Text
Johnsson, F., and Kjärstad, J. (2019). Avskiljning, transport och lagring av koldioxid i Sverige–behov av forskning och demonstration, Report B1969, IVL Svenska Miljöinstitutet.CrossRefFull Text
Johnsson, F., Kjärstad, J., and Rootzén, J. (2019). The threat to climate change mitigation posed by the abundance of fossil fuels. Clim. Pol. 19, 258–274. doi:10.1080/14693062.2018.1483885 CrossRefFull Text
Johnsson, F., Normann, F., and Svensson, E. (2020). Marginal abatement cost curve of industrial CO2 capture and storage–a Swedish case study. Front. Energy Res. submitted [Epub ahead of print]. doi:10.3389/fenrg.2020.00175 CrossRefFull Text
Kashwan, P., and Holahan, R. (2014). Nested governance for effective REDD+: institutional and political arguments. Interntational J. Commons 8, 554–575. doi:10.18352/ijc.450 CrossRefFull Text
Klement, J., Rootzén, J., Johnsson, F., and Normann, F. (2021). Supply chain driven commercialisation of bio energy carbon capture and storage. Accepted for publication in Frontiers in Climate, section Negative Emission Technologies. CrossRefFull Text
Koch, N., Grosjean, G., Fuss, S., and Edenhofer, O. (2016). Politics matters: regulatory events as catalysts for price formation under cap-and-trade. J. Environ. Econ. Manag. 78, 121–139. doi:10.1016/j.jeem.2016.03.004 CrossRefFull Text
Kriegler, E., Weyant, J. P., Blanford, G. J., Krey, V., Clarke, L., Edmonds, J., et al. (2014). The role of technology for achieving climate policy objectives: overview of the EMF 27 Study on global technology and climate policy strategies. Climatic Change. 123, 353. doi:10.1007/s10584-013-0953-7 CrossRefFull Text
Laude, A. (2020). Bioenergy with carbon capture and storage: are short-term issues set aside? Mitig. Adapt. Strategies Glob. Change. 25, 185–203. doi:10.1007/s11027-019-09856-7 CrossRefFull Text
Levihn, F. (2020). Personal communication, Stockholm. CrossRefFull Text
Los Alamos National Laboratory (2019). Preliminary assessment of post-combustion capture of carbon dioxide at the San Juan generating station Los Alamos. Available at: https://www.lanl.gov/science-innovation/science-programs/applied-energy-programs/_assets/docs/preliminary-technical-assessment-december2019.pdf, (Accessed September 1, 2020)CrossRefFull Text
Luderer, G., Vrontisi, Z., Bertram, C., Edelenbosch, O. Y., Pietzcker, R. C., Rogelj, J., et al. (2018). Residual fossil CO 2 emissions in 1.5-2 °C pathways. Nat. Clim. Change [Epub ahead of print]. doi:10.1038/s41558-018-0198-6 CrossRefFull Text
Lyng Anthonsen, K., Frykman, P., and Møller Nielsen, C. (2016). Mapping of the CO2 storage potential in the Nordic region. GEUS Bull. 35, 87–90. doi:10.34194/geusb.v35.4946 CrossRefFull Text
McLaren, D. P. (2016). “Framing out justice: the post-politics of climate engineering discourses,” in Climate justice and geoengineering: ethics and policy in the atmospheric anthropocene. Editor C. Preston (London: Rowman & Littlefield Publishers), 139–160. CrossRefFull Text
Minx, J. C., Lamb, W. F., Callaghan, M. W., Fuss, S., Hilaire, J., Creutzig, F., et al. (2018). Negative emissions—Part 1: research landscape and synthesis. Environ. Res. Lett. 13, 063001. doi:10.1088/1748-9326/aabf9b CrossRefFull Text
Mortensen, G. M., Bergmo, P. E. S., and Emmel, B. U. (2016). Characterization and estimation of CO2 storage capacity for the most prospective aquifers in Sweden. Energy Procedia. 86, 352. doi:10.1016/j.egypro.2016.01.036 CrossRefFull Text
Mortensen, G. M., Erlström, M., Nordström, S., and Nyberg, J. (2017). Geologisk lagring av koldioxid i Sverige: lägesbeskrivning avseende förutsättningar, lagstiftning och forskning samt olje- och gasverksamhet i Östersjöregionen, Sveriges geologiska undersökning. 0349–2176; 142Uppsala.CrossRefFull Text
Nemet, G. F., Callaghan, M. W., Creutzig, F., Fuss, S., Hartmann, J., Hilaire, J., et al. (2018). Negative emissions - Part 3: innovation and upscaling. Environ. Res. Lett. [Epub ahead of print]. doi:10.1088/1748-9326/aabff4 CrossRefFull Text
Peters, G. P., Andrew, R. M., Canadell, J. G., Fuss, S., Jackson, R. B., Korsbakken, J. I., et al. (2017). Key indicators to track current progress and future ambition of the Paris Agreement. Nat. Clim. Change. 7. doi:10.1038/nclimate3202 CrossRefFull Text
Realmonte, G., Drouet, L., Gambhir, A., Glynn, J., Hawkes, A., Köberle, A. C., et al. (2019). An inter-model assessment of the role of direct air capture in deep mitigation pathways. Nat. Commun. 10, 3277. doi:10.1038/s41467-019-10842-5 | CrossRefFull Text
Rickels, W., Merk, C., Reith, F., Keller, D. P., and Oschlies, A. (2019). (Mis)conceptions about modeling of negative emissions technologies. Environ. Res. Lett. 14, 104004. doi:10.1088/1748-9326/ab3ab4 CrossRefFull Text
Rickels, W., Proelß, A., Geden, O., Burhenne, J., and Fridahl, M. (2020). The future of (negative) emissions trading in the European Union, Kiel, Germany. Kiel Working Paper 2164, Sept 2020. CrossRefFull Text
Robledo-Abad, C., Althaus, H. J., Berndes, G., Bolwig, S., Corbera, E., Creutzig, F., et al. (2017). Bioenergy production and sustainable development: science base for policymaking remains limited. GCB Bioenergy. 9, 541–556. doi:10.1111/gcbb.12338 | CrossRefFull Text
Rogelj, J., Popp, A., Calvin, K. V., Luderer, G., Emmerling, J., Gernaat, D., et al. (2015). Transition pathways towards limiting climate change below 1.5°C. Nat. Clim. Change. 5, 519–527. doi:10.1038/nclimate2572 CrossRefFull Text
Rogelj, J., Shindell, D., Jiang, K., Fifita, S., Forster, P., Ginzburg, V., et al. (2018). “Mitigation pathways compatible with 1.5°C in the context of sustainable development,” in Global Warming of 1.5 °C: An IPCC special report on the impacts of global warming of 1.5 °C above pre-industrial levels and related global greenhouse gas emission pathways, in the context of strengthening the global response to the threat of climate change, sustainable development, and efforts to eradicate poverty. Editors Masson-Delmotte, V., P. Zhai, H.-O. Pörtner, D. Roberts, J. Skea, P.R. Shukla, A. Pirani, W. Moufouma-Okia, C. Péan, R. Pidcock, S. Connors, J.B.R. Matthews, Y. Chen, X. Zhou, M.I. Gomis, E. Lonnoy, T. Maycock, M. Tignor, and T. Waterfield. In Press. Available at: http://www.ipcc.ch/report/sr15/. CrossRefFull Text
Rootzén, J., and Johnsson, F. (2016). Paying the full price of steel – perspectives on the cost of reducing carbon dioxide emissions from the steel industry. Energy Pol. 98, 459–469. doi:10.1016/j.enpol.2016.09.021 CrossRefFull Text
Rootzén, J., and Johnsson, F. (2017). Managing the costs of CO2 abatement in the cement industry. Clim. Pol. 17, 781–800. doi:10.1080/14693062.2016.1191007 CrossRefFull Text
Royal Society and Royal Academy of Engineering (2018). Greenhouse gas removal, London. Available at: royalsociety.org/greenhouse-gas-removal. CrossRefFull Text
Rubin, E. S., Mantripragada, H., Marks, A., Versteeg, P., and Kitchin, J. (2012). The outlook for improved carbon capture technology. Prog. Energy Combust. Sci. 38, 630–671. doi:10.1016/j.pecs.2012.03.003 CrossRefFull Text
Scherhaufer, P., Höltinger, S., Salak, B., Schauppenlehner, T., and Schmidt, J. (2018). A participatory integrated assessment of the social acceptance of wind energy. Energy Res. Soc. Sci. 45, 164–172. doi:10.1016/j.erss.2018.06.022 CrossRefFull Text
Smith, P., Adams, J., Beerling, D. J., Beringer, T., Calvin, K. V., Fuss, S., et al. (2019). Land-management options for greenhouse gas removal and their impacts on ecosystem services and the sustainable development goals. Annu. Rev. Environ. Resour. 44, 255–286. doi:10.1146/annurev-environ-101718-033129 CrossRefFull Text
Smith, P., Bustamante, M., Ahammad, H., Clark, H., Dong, H., Elsiddig, E. A., et al. (2014). “Agriculture, forestry and other land use (AFOLU),” in climate change 2014: mitigation of climate change. contribution of working group III to the fifth assessment report of the Intergovernmental Panel on Climate Change. Editors O. Edenhofer, R. Pichs-Madruga, Y. Sokona, E. Farahani, S. Kadner, K. Seyboth, A. Adler, I. Baum, S. Brunner, P. Eickemeier, B. Kriemann, J. Savolainen, S. Schlömer, C. von Stechow, T. Zwickel, and J. C. Minx. Cambridge, United Kingdom and New York, NY, USA: Cambridge University Press).CrossRefFull Text
Smith, P., Davis, S. J., Creutzig, F., Fuss, S., Minx, J., Gabrielle, B., et al. (2016). Biophysical and economic limits to negative CO2 emissions. Nat. Clim. Change. 6, 42–50. doi:10.1038/nclimate2870 CrossRefFull Text
SOU (2020). Vägen till en klimatpositiv framtid [The Pathway to a Climate-Positive Future]. Stockholm: Statens Offentliga Utredningar. CrossRefFull Text
Sveriges Geologiska Undersökning (2017). Geologisk lagring av koldioxid i Sverige–Lägesbeskrivning avseende förutsättningar, lagstiftning och forskning samt olje- och gasverksamhet i Östersjöregionen. CrossRefFull Text
Swedish Environmental Protection Agency (2017). Sweden’s climate act and climate policy framework. Available at: http://www.swedishepa.se/Environmental-objectives-and-cooperation/Swedish-environmental-work/Work-areas/Climate/Climate-Act-and-Climate-policy-framework-/. (Accessed April 1, 2020).CrossRefFull Text
The Global CCS Institute (2019). Facilities database. Available at: https://co2re.co/FacilityData. CrossRefFull Text
Tong, D., Zhang, Q., Zheng, Y., Caldeira, K., Shearer, C., Hong, C., et al. (2019). Committed emissions from existing energy infrastructure jeopardize 1.5 °C climate target. Nature doi:10.1038/s41586-019-1364-3 CrossRefFull Text
Torvanger, A. (2019). Governance of bioenergy with carbon capture and storage (BECCS): accounting, rewarding, and the Paris Agreement. Clim. Pol. 19, 329–341. doi:10.1080/14693062.2018.1509044 CrossRefFull Text
van Vuuren, D. P., Stehfest, E., Gernaat, D. E. H. J., van den Berg, M., Bijl, D. L., de Boer, H. S., et al. (2018). Alternative pathways to the 1.5 °C target reduce the need for negative emission technologies. Nat. Clim. Change. 8, 391–397. doi:10.1038/s41558-018-0119-8 CrossRefFull Text
Vaughan, N. E., Gough, C., Mander, S., Littleton, E. W., Welfle, A., Gernaat, D. E. H. J., et al. (2018). Evaluating the use of biomass energy with carbon capture and storage in low emission scenarios. Environ. Res. Lett. 13. doi:10.1088/1748-9326/aaaa02 CrossRefFull Text
Keywords: carbon capture and storage, bioenergy, paris climate targets, sweden, net-zero
Citation: Fuss S and Johnsson F (2021) The BECCS Implementation Gap–A Swedish Case Study. Front. Energy Res. 8:553400. doi: 10.3389/fenrg.2020.553400
Received: 18 April 2020; Accepted: 10 December 2020;
Published: 11 February 2021.
Edited by:
Mai Bui, Imperial College London, United KingdomReviewed by:
Andres Fernando Clarens, University of Virginia, United StatesNicolaas Vermeulen, Northwestern University, Philippines
Copyright © 2021 Fuss and Johnsson. This is an open-access article distributed under the terms of the Creative Commons Attribution License (CC BY). The use, distribution or reproduction in other forums is permitted, provided the original author(s) and the copyright owner(s) are credited and that the original publication in this journal is cited, in accordance with accepted academic practice. No use, distribution or reproduction is permitted which does not comply with these terms.
*Correspondence: Sabine Fuss, fuss@mcc-berlin.net