Economics of CO2 Utilization: A Critical Analysis
- 1Departments MIFT and ChiBioFarAm, University of Messina and ERIC Aisbl, Messina, Italy
- 2NEXTCHEM (Maire Tecnimont for Energy Transition), Rome, Italy
The economics of CO2 utilization are discussed from a critical perspective, with a concise analysis of the state-of-the-art of economics in power-to-X (methanol, methane). The main elements of the analysis of the economics are commented to provide guidelines on how to interpret the techno-economic results in the area of CO2 utilization. It remarks the need of a careful analysis of the specific context, and of the limits of the evaluation, in order to go beyond the just use of the results without a proper analysis of how the data support the conclusions, their limits and applicability. Case examples discussed in a more detail regard the CO2 to methanol or methane conversion, from the perspective of highlighting possible issues or limits rather than to indicate which results are more valuable, which is out of the scope of this contribution.
Introduction
The techno-economic feasibility of CO2 utilization routes is a crucial element for their industrial implementation, together with other relevant aspects, such as the impact on the reduction of CO2 emissions (Centi et al., 2019a; Centi et al., 2019b). This impact must be considered in terms of services provided using CO2 to substitute fossil resources rather than in terms of 1) amount of CO2 stored and 2) storage time (Schlögl et al., 2018). CO2 large-scale reduction is when its use is as medium to increase renewable energy utilization. This is the way to account the impact of CCU (CO2 utilization) routes (Lanzafame et al., 2017a).
Many reviews and papers have discussed the costs and techno-economic feasibility of CO2 utilization routes, a selection (not exhaustive) of which is the following: Centi and Perathoner (2010), Quadrelli et al. (2011), Aresta et al. (2013), Centi et al. (2013), Barbato et al. (2014), Centi and Perathoner (2014), Perathoner and Centi (2014), Laumb et al. (2013), Ampelli et al. (2015), Dimitriou et al. (2015), Pérez-Fortes et al. (2016), Naims (2016), Navarrete et al. (2017), Ordomsky et al. (2017), Senftle and Carter, (2017), Zheng et al. (2017), Iaquaniello et al. (2018), Koytsoumpa et al. (2018), González-Garay et al. (2019a), Hepburn et al. (2019), Jens et al. (2019), Zhang et al. (2019), Grim et al. (2020), Meunier et al. (2020), Mustafa et al. (2020), Zhang et al. (2020), and Zimmermann et al. (2020). Although the conclusions are often contradictory, it is commonly suggested that there still exist large barriers for the implementation and deployment of CCU.
There is, on the other hand, a fast-evolving scenario for CO2 utilization paths, in terms of both costs and technical feasibility. A number of industrial pilot plants or demo size units have been developed to demonstrate techno-economic feasibility. Examples include CO2 methanation (Schiebahn et al., 2015; Bailera et al., 2017; Chwola et al., 2020) or conversion to methanol (Son et al., 2018). Haldor Topsoe is building an around 50 t/yr methanol plant to demonstrate their eSMR Methanol™ technology for cost-competitive production of sustainable methanol from biogas by using an electrified reactor. Carbon Recycling International (CRI) and other members of the MefCO2 research consortium are building in Germany an about 300 t/yr CO2-to-methanol demo unit.
Carbon Recycling International was the first to construct a demo/semi-commercial plant for methanol production from CO2 in Iceland. In this case, CO2 and electricity derive from geothermal sources and thus it is not an easily replicable model. Carbon2Chem pilot plant at the Thyssenkrupp steel mill in Duisburg (Germany) is also designed to produce about 50 t/yr of methanol. Audi e-Gas pilot plant produces 1,000 tons/yr of methane from CO2. STORE and GO demo plant in Germany produces 1,400 cubic meters of synthetic methane from CO2 per day. These are few of the many examples of pilot and demo plants around the so-called power-to-liquid and power-to-gas technologies. Pilot/demo plants are present also for other CO2 utilization paths.
There is thus an apparent discrepancy between the many industrial initiatives in the area of CO2 utilization and the debate on CO2 economics, going from sceptical to optimistic, but often with an intrinsic difficulty to identify which is the more appropriate conclusion. Together with the discussion on CO2 economics, the environmental impact is often also made, typically through the use of Life Cycle Assessment (LCA) or derived methodologies. For environmental and greenhouse gas (GHG) impact, there is also often a spread of indications (Aresta et al., 2002; von der Assen et al., 2013, von der Assen et al., 2014; Garcia-Herrero et al., 2016; Thonemann, 2020). It may be thus questioned why different conclusions can be obtained for the same problem.
Both analyses of the process economics and environmental impact follow complex methodologies, and the final result depends on several parameters and the boundary limits applied on the analysis. It is thus not surprising that even with the same base of data, different conclusions can be present. Thus, particularly attention is necessary in using the results out of the proper context.
For this reason, it is good to provide some guidelines and general comments about CO2 economics. The aim is to give a background of knowledge allowing a better analysis of literature data, and of their eventual limits. This contribution aims to discuss these aspects, although avoiding to indicate which results should be considered valid or not. This could be made, in fact, only for specific contexts and situations, which may change with time. The aim here is instead to provide the bases for this analysis evidencing also the key factors determining economics. Although some example will be also discussed in a more detail, this contribution is not intended to be neither a review of the state-of-the-art on CO2 economics, neither a didactic presentation of the methodologies for this analysis.
The aim is to remark the need of a critical approach in evaluating CO2 economics, providing also the main background technical aspects necessary, in particular, for not-experts. A concise state-of-the-art, limited to CO2 economics in power-to-X (methanol, methane) solutions, is also presented, to provide the reader elements for a better understanding.
The Context and Approach for the Analysis of CO2 Utilization Routes
As a general statement, it may be indicated that CO2 utilization paths are already feasible routes to consider and thus to analyze in a more detail with reference to 1) the specific case of applications, 2) the synergies possible, and 3) the benefits and barriers. Scale-up is also a relevant aspect, and this is also the reason of the many pilot/demo projects. However, most of these studies are focused mainly at increasing the technology readiness level (TRL) up to a 6–7 value (TRL 7 is system prototype demonstration in operational environment), rather than to create the right value chain in relation to energy transition. Being present a full transition in energy, rather than the implementation of single novel technologies, the feasibility of CO2 utilization routes, which include economic assessment, must be analyzed in the context of the value chain. However, current approaches are lacking from this perspective. This is a crucial issue to solve also for CO2 economics and thus for the proper analysis of the feasibility of CO2 utilization routes.
It should be also remarked that rather than by economic only aspects, the industrial exploitability is often technology-limited today. In other words, the new generation technologies, which can enable a larger use of CO2 utilization paths, are still at a too early stage while those available and implemented at pilot/demo scale, still suffer of many limitations. There is thus an innovation gap for a wider spread of CO2 utilization solutions. Typically only the technological gap between fundamental research (up to TRL of three corresponding to the proof of the concept) and a TRL of 6–7 (technology demonstrated in industrially relevant) is considered as the key element.
The innovation gap is that existing when a proper pipeline between current and next generation technologies is missing. It is the innovation rather than the technological gap the crucial aspects to solve in a system change as that related to energy transition. This aspect will be further commented later.
Energy and chemistry systems are strongly linked even if their nexus is changing (Abate et al., 2015a). They define together the set of production, transformation, transport and distribution processes of raw materials and products. Thus, a proper analysis of CO2 economics cannot be made without properly considering this evolving nexus. Some elements on this aspect will be commented later.
Part of the innovation gap is a consequence also that a correct evaluation of the process economics is often difficult and complex, being these new generation technologies still at a not-sufficient level of development. This is also the problem for LCA and analogous methodologies, not well suited, or having a too high uncertainty, when applied to technologies at a too early stage of development. This is always valid in changing the technologies. However, this becomes a critical issue when this occurs simultaneously to a system change, as that on-going due to energy transition. This will be concisely discussed in the following section.
To remark that notwithstanding the large R&D effort the range of novel concepts and solutions explored is limited (Ampelli et al., 2015). In addition, often constrains in terms of industrial applicability and of the impact related to a system change are not properly considered, as commented later. There is a need of a broader-view approach, exploring new solutions, synergies and possibilities (Centi and Čejka, 2019a), but at the same time also strongly guided from an industrial vision on targets, issues, integration, solutions.
In addition, the synergies created from the system change, for example from the integration between solar- and bio-refineries (Abate et al., 2015b), offer new perspectives. However, these emerge only from a system analysis rather than from a classical techno-economic assessment (Centi et al., 2019b).
A Concise State-of-the-Art on Power-to-X (Methanol, Methane) Economics
As indicated in the introduction, this contribution is not a review on CO2 economics or on the methodologies for this analysis, but rather aims to provide elements for a critical analysis and how to put current results on CO2 economics in the right perspective. At the same time, the scope is also to remark the needs of a different approach, based on considering the elements remarked above (value chain, energy-chemistry nexus, innovation gap, system change). However, for a proper analysis, it is useful to provide concise background indications on the state-of-the-art in the area. This is limited to Power-to-X (methanol, methane) economics for conciseness.
Many studies have been reported in literature about the techno-economic assessment of Power-to-X (PtX) technologies. A not-exhaustive list is the following: Kim et al. (2011), Barbato et al. (2014), Hofstetter et al. (2014), De Saint Jean et al. (2015), Hannula (2015), Roh et al. (2015), Schiebahn et al. (2015), Tremel et al. (2015), Atsonios et al. (2016), Gutièrrez-Martin and Rodriguez-Anton (2016), Kourkoumpas et al. (2016), Pérez-Fortes et al. (2016), Räuchle et al. (2016), Rivera-Tinoco et al. (2016), Rivarolo et al. (2017), Gonzàlez-Aparicio et al. (2017), Parra et al. (2017), Asif et al. (2018), Hank et al. (2018), Hoppe et al. (2018), Michailos et al. (2018), Moellenbruck et al. (2018), Alsayegh et al. (2019), Do and Kim (2019), Gonzàlez-Garay et al. (2019a), Nami et al. (2019), Nguyen and Zondervan (2019), Nieminen et al. (2019), Zhang et al. (2019b), Bos et al. (2020), Khunathorncharoenwong et al. (2020), and Zhang and Desideri (2020).
In few cases, and limited essentially to methanol case, these technologies were evaluated out of the specific context of storage of local excess of renewable energy. Few studies have considered the impact on energy transition and sustainability of the transport of remote renewable energy sources by PtX technologies, and very few the impact on aspects such as value chain, energy-chemistry nexus, innovation gap, and system change. The impact on saving CO2 emissions, and in general on lowering impact on the environment, is often considered, but a broader view, able to predict the effective impact related to system change, is not typically present.
Figure 1 summarizes the results in terms of cost of production of methanol (€/h) or of methane (€/MWh) from CO2 by using renewable energy sources. The year indicated in Figure 1 is the year of publication of the related paper. When data were in US$ a conversion factor of 1.18 was used. Refence values for methanol and methane are also indicated. The results were not homogeneous in terms of database for costs of raw materials, technologies, approach, and boundary limits, and it is out of the scope to analyze here these specific aspects. However, this Figure 1 clearly remarks how the results are very spread, with estimated costs varying up to one order of magnitude, which is largely beyond the usual ±30‰ variation in costs assumed in preliminary techno-economic evaluations. There is thus an intrinsic basic issue in these estimations, and a clear trend, for example with year of publication, cannot also be evidenced.
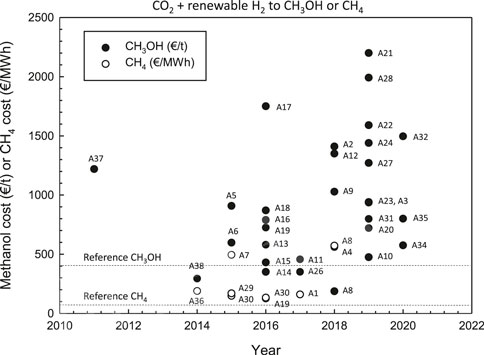
FIGURE 1. An overview of the results in terms of costs of methanol production (€/t) and of methane production (€/MWh) in CO2 conversion using H2 produced from renewable energy sources. References: A1, Parra et al., 2017; A2, Asif et al., 2018; A3, Nieminen et al., 2019; A4, Moellenbruck et al., 2018; A5, Hannula, 2015; A6, Roh et al., 2015; A7, De Saint Jean et al., 2015; A8, Hoppe et al., 2018; A9, Hank et al., 2018; A10, Zhang et al., 2019b; A11, Rivarloo et al., 2017; A12, Michailos et al., 2018; A13–A15, Kourkoumpas et al., 2016; A16–A17, Rivera-Tinoco et al., 2016; A18, Atsonios et al., 2016; A19, Räuchle et al., 2016; A20–A22, Nguyen Zondervan, 2019; A23, Alsayegh et al., 2019; A24, Nami et al., 2019; A25, Pérez-Fortes et al., 2016; A26, Gonzàlez-Aparicio et al., 2017; A27–A28, Gonzàlez-Garay et al., 2019b; A29, Schiebahn et al., 2015; A30, Gutièrrez-Martin and Rodriguez-Anton, 2016; A31, Do and Kim, 2019; A32, Khunathorncharoenwong et al., 2020; A33, Tremel et al., 2015; A34, Zhang and Desideri, 2020; A35, Bos et al., 2020; A36, Hofstetter et al., 2014; A37, Kim et al., 2011; A38, Barbato et al., 2014. Original Figure.
Figure 1 also remarks that the use of one or few of these results, is misleading, when used to assess techno-economic feasibility, but also to evaluate the impact. In fact, the impact of CCU technologies is clearly related to economics. For example, all estimations (about one third of those in Figure 1) for a methanol production cost above about 800 €/t, i.e., about twice the current average methanol value, indicate the not feasibility of this solution, and thus a close to zero impact. The area of estimations below this threshold, and up to reference methanol value, may be discussed in relation to incentives in CO2 reduction, carbon taxes and other opportunities. Thus, the impact may greatly depend on these aspects. The estimations below the reference methanol value (about 12‰ of the total) indicate instead that this technology may be ready to be applied commercially and potentially on large scale.
Although we discuss here only CO2 economics, it is evident from above considerations, the strong nexus also with impact on GHG reduction targets and transition on energy and chemical production.
Economics of CO2 Utilization in the Broader Context of Energy Transition
While current state-of-the-art CO2 utilization technologies are an important element to foster the transformation, the real opportunities and positive economics in CO2 utilization technologies will likely derive from the second generation processes. This term indicates those which both introduce new technological solutions, (for example, a more efficient direct use of renewable energy sources) and which are designed to effectively integrate within the new value chain deriving from energy transition. A consequence is the need of novel assessment approaches, which include new models for evaluation of CO2 economics (Centi et al., 2019b).
Applying conventional assessments and analysis methodologies to a system in transition leads to misleading analyses, In fact, the latter is characterized from high non-linearity between the components, while conventional assessment is based largely on linear relations between the elements (although often not explicitly stated). This was proved historically in the past transition occurring in chemical production around 70 years ago (Centi et al., 2009). This is a general aspect to consider in evaluating the economics of CO2 utilization. It is also emerging from the analysis of the past transitions, that companies not able to understand these aspects, and thus not well prepared to the transition, largely lost their core business. Thus, novel conceptual approaches to analyze business strategies in transition periods including advanced economical methodologies are a crucial industrial aspect. A proper analysis of the economics of CO2 utilization processes is thus a relevant element for an effective industrial strategy.
Cost of renewable energy is a determining factor in CO2 economics, often accounting more of 70% of the total costs (Centi and Perathoner, 2020). Power-to-X routes (Chehade et al., 2019; Rego de Vasconcelos and Lavoie, 2019) are largely based on the production of H2 by electrolysis, which is then used for the catalytic conversion of CO2. The cost of producing renewable electrical energy has been dramatically decreased in recent years. Part of the large spread in results (Figure 1) derives from the difference in renewable energy costs. On these bases, results are too spread to make reliable conclusions and predict a future trend. There are problems of accounting methodology, but especially on how to properly consider the evolution trend, which is not linear in transition economy.
Another related issue is the availability of renewable energy sources to account for the transition and to convert CO2. Often who is negative about the use of CO2 utilization technologies objects that there is not a sufficient excess renewable electrical energy for large-scale application. While in the past Power-to-X technologies were developed originally to store the excess available of renewable energy, there is no reason today to use this limited view. Dedicated production is feasible and preferable, but still not solving the issue of fluctuation and a constant supply of energy. If energy comes from the grid to bypass the discontinuity issue, it is on the average over 70‰ deriving from non-renewable sources even in the coming decade. The use of green certificates claiming that only energy from renewable sources is used, is a clear unproper sustainable approach.
However, the main point is that the products of CO2 conversion (methanol, methane) are chemical energy vectors, and particularly methanol can be easy transported and stored. Thus, this reaction can be used to store/transport renewable energy which can be produced in remote areas at low cost. These chemical energy vectors will thus allow to create a trading system of renewable energy substituting the current one based on fossil fuels (or derivates). This full renewable-energy economy will be at the basis of the system change related to energy transition, and it is thus important to analyze the enabling technologies, among which catalysis plays certainly a relevant role (Centi and Perathoner, 2009; Lanzafame et al., 2017b; Čejka et al., 2018). Strictly related to these aspects is also the concept of solar fuels and chemicals (Perathoner and Centi, 2014; Ampelli et al., 2015; Perathoner and Centi, 2019). Without including the evaluation of Power-to-X technologies in this broader context and sustainable future, all the economics fail to produce the relevant estimations. This is the question remarked before. A transition period requires to use new conceptual assessment models, while the application of the current one often fails in making reliable indications. The very spread estimations summarized in Figure 1, are a good indication of the basic problem which exists. As a side comment, it evidences also why just a review of the results has a scarce meaning, and thus why this contribution has a different approach.
In order to assess properly the impact and economics of CO2 utilization, it is thus necessary to put them in the general context of on-going system change, and its future implications. The analysis of the costs should take into account these aspects. CO2 economics cannot be thus disjointed from a proper contextualization in terms of energy transition.
Impact and Economics of CO2 Utilization
Relations and Misleading Concepts
Another general issue in the discussion of economics of CO2 utilization is the often assumption that the production of chemicals will lead to marginal impacts in terms of contribution to the reduction of GHG emissions. Different is the case of the production of fuels, but in this case the low impact is related to being not economically competitive. A recent Nature paper cites in the abstract that “Pathways that involve chemicals, fuels and microalgae might reduce emissions of carbon dioxide but have limited potential for its removal” (Hepburn et al., 2019). To analyze this conclusion, and in general the relation between scale and economics of CO2 utilization, it is necessary to enter in the details of this analysis. The technologies considered able to contribute significantly to GHG emission reduction (in the Gt scale), do not include typically CCU (or Power-to-X), but only land- or marine-based sequestration techniques. See, for example, Intergovernmental Panel on Climate Change (IPCC) reports (IPCC, 2014). We should comment that this conclusion is due to a too limited vision of how accounting the impact. An example will be given in the section on economics of CO2 to methanol to enable a worldwide economy based on renewable energy.
Hepburn et al. (2019) considers ten pathways: 1) CO2-based production of chemicals (urea, methanol, polyols and polymers); 2) CO2-based fuels (methanol, methane, dimethyl ether, and Fischer–Tropsch fuels); 3) microalgae fuels and other microalgae products; 4) concrete building materials; 5) CO2 enhanced oil recovery (CO2-EOR—Enhanced Oil Recovery); 6) bio-energy with carbon capture and storage (BECCS); 7) enhanced weathering; 8) forestry techniques, including afforestation/reforestation, forest management and wood products; 9) land management via soil carbon sequestration techniques; and 10) biochar. Among the parameters accounted, the time of storage and likelihood of release are considered as key elements. We remark, however, that this is not a correct way to evaluate the impact. It is not the amount of captured CO2 and for how long time it remains stored, but the amount of fossil fuels used that can be avoided (Schlögl et al., 2018). Thus, a short cycle such as for fuels could be beneficial rather than negative, because implies the possibility to substitute a larger amount of fossil fuels with renewable resources over a given period.
To clarity better this aspect, it should be remarked that it is the same concept of changing from a linear to a circular economy. In a linear economy resources are taken from the environment, and wastes are introduced in the environment. In a carbon circular economy, the use of carbon should be circular i.e. the waste reused to avoid, or minimize, both the use of resources and the production of waste. The storage of emitted CO2 will still maintain this linear model. It could reduce GHG, but remains a wrong approach. In some cases, differently from what considered by Hepburn et al. (2019), technologies like Enhanced Oil recovery (EOR) are even negative instead that positive, because lead to the higher exploitation of fossil resources.
In a circular model, a longer time of storage/sequestration is eventually a negative element, because reduces the circularity. In a carbon circular model, the GHG impact should be calculated as the reduction induced by substituting with renewable sources the current use of fossil fuels. Accounting CO2 utilization potential as the volume of product multiplied by the amount of CO2 required to make an equivalent amount of the product (Hepburn et al., 2019), is not correct and leads to underestimation (in the case of power-to-X) or overestimation (in the case of EOR). Note also that EOR impact is overestimated due to the many effective constrains in use. Hepburn et al. (2019) also not properly accounted the impact of urea production. CO2 derives from the process itself, i.e. from the fossil fuels (typically methane) used to produce the H2 necessary for ammonia synthesis. Thus, current urea production is not a way to capture CO2 in chemicals, if green or solar ammonia is not used. The difference between green and solar is in the separate production of green H2 and its use in the nearly conventional catalytic synthesis of ammonia, or instead the direct production of NH3 from N2, H2O and sunlight. This is, however, a technology still not commercial.
In the specific estimation of CO2 economics, the costs indicated by Hepburn et al. (2019) are based on an uncritical analysis of literature. It is not the aim here to criticize this paper, but to use as an example to remark how often in terms of economics of CO2 utilization there are not correct conclusions based on a not enough critical analysis of the problems. Figure 1 well remarks this issue, and how could influence the estimation of the potential impact. On the other hand, it is evident that the input given to the debate on the methodologies to reduce GHG by this and other papers may lead to misleading conclusions. In general, it thus contributes to the existing confusion regarding the effective potential and economics of CCU.
The SAPEA report “Novel carbon capture and utilization technologies: research and climate aspects” (Schlögl. et al., 2018) attempted this analysis to provide an independent scientific opinion. However, a further effort is necessary, because there are still too strong lobby interests in all the area of GHG emissions, being energy so pervasive for our life and thus being at its core also in terms of economical consequences. Thus, changing from a fossil fuel centric to a renewable energy-based vision has macro-economic and geo-political consequences affecting strong lobbies. Often, in addition, there is the tendency to advertise solutions to preserve these lobby interest as in favor of the environment (greenwashing).
Concepts and Elements of the Analysis
Using Hepburn et al. (2019) as a working example, some relevant concepts and elements of the analysis may be commented. Discussion is limited to economics for methanol and methane from CO2. For methanol, the paper indicates a cost of production of 510 $/t with respect to a market value they indicated in 400 $/t (quite large fluctuations were observed in this average methanol commercial price, reaching even a minimum of 160 $/t in May 2020 at Rotterdam). It should be also remarked that market value of a fuel/chemical is different from the cost of production, which is generally lower, but a series of clarifications are needed to explain the meaning of the term “generally.”
The cost of production is determined from the combination of CAPEX (Capital Expenses) and OPEX (Operative Expenses). There are many aspects which determine the cost of production, some discussed later. Note also that especially for new technologies, estimation of CAPEX is difficult and complex and thus requires significant specific professional experience, and estimations by just using simulation software (for example, Aspen or ChemCad, as commented later) may give not correct indications. Even for engineering societies, it is a common practice to include contingencies of 15–40‰ to consider the difficulties in the estimations.
One aspect to remark here is that the costs are determined assuming often a full capacity of production (100‰, excluding typical interruptions for maintenance and causalities), but typical operations are at lower capacity. This is due to several aspects, among the highly fluctuating market demand is relevant. Typically, to operate economically, chemical plants should operate at a minimum 90–95‰ of the full capacity, but in the actual highly volatile situation, operations below this threshold are common. Thus, a plant which is designed to operate for a given cost may effectively operates at a higher production cost and higher than market value. This in addition to the many other factors determining the costs.
The situation is also different if the product is for the market (for which often high competition is present) with respect to an internal use by the company itself. In addition, in CO2 utilization technologies, there are a series of additional relevant factors, for example all the incentives for the production of biofuels, to avoid CO2 emissions, or on the contrary taxation on CO2 emissions. Sustainability, in addition, is becoming an increasing driving factor to determine industrial strategies in this area (Basile et al., 2019).
Note furthermore that also market value depends on many factors and may change largely. Contract price are different from spot prices, and there are large differences on regional bases and by quarterly. In methanol, for example, these variations influence typically ±20 the average market value, in some cases even more. In 2019, EU methanol spot vs. contract price, had negative peaks up to −140 €/t, thus up to 30–35‰ of the average contract price. In addition, contract prices may depend on many factors, and can be different for internal use and when, for example, the target is a mandatory reduction of the CO2 emissions. Thus, what could be supposed to be not economic based on average values, could be instead quite different for specific situations. When there are large differences, it may be expected that may be difficult to overcome them. However, it is prudential not make this assumption when estimated costs are within the ±50 range, for which contextualization of the analysis is necessary (e.g., referred to a specific business case). This is often the actual case for various CO2 utilization cases, particularly for the Power-to-X area. This is also the motivation why many demo/pilot units are under evaluation.
Turning back to the discussion of the cost for methanol from CO2, this cost depends on several aspects, among which the two critical are the cost of electrical energy (if green H2 is produced using electrolyzers) and CAPEX amortization time. Amortization time defines the time needed to decrease, or account for, the investment cost. CAPEX is the capital expenditure, i.e. the fixed cost for construction of the plant. The amortization time, in the definition used here for Power-to-X technologies, considers that the fixed costs have to account also for how many hours per year the plant will operate, and not only for how many years the plant will be in operations. In using renewable energy, if only few hours per day of available electrical energy could be used (besides to all other problems related to start up/shut down operations) the CAPEX amortization will largely increase the costs of production. Costs can be significantly lower when full time operations are considered. Note also that in addition to amortization for CAPEX, the interest rate on the capital, usually in the form of weighted average cost of capital (WACC), must be considered. This could change significantly based on the economic period and may vary from about 3 to 8‰.
At the same time, the years of amortization of the plant can largely vary, depending on various considerations, going typically from 7 years for environmental technologies to 20 years or longer for mature technologies. Environmental technologies are supposed to be substituted with more efficient ones after short times. Considering that in CO2 utilization technologies, CAPEX account for up to 70‰ of the production cost, it is evident that the CO2 estimations can produce positive or negative indications without a proper analysis.
Note also that CAPEX in chemical processes has a much lower impact on the production costs, typically <40–50‰. On the other hand, an analysis of the specific CAPEX costs for CO2 to methanol, reveals that over 50‰ of these costs are related to the electrolyzer. Although in terms of efficiency this technology cannot be largely improved, the costs are instead largely related to the possibility of a larger-scale industrialization, besides that on the nominal power. Note finally that H2 production from electrolyzers is the commonly considered solution, because H2 (from solar splitting of water) is still at an initial stage of development. However, green H2 (e.g., not based on fossil fuels), can be produced in other ways, for example, from waste or from biomethane (i.e., methane in biogas). In the latter case, H2 derives from its catalytic decomposition to H2 and carbon. These alternative solutions are often not considered but could be part of a platform of technologies to guarantee continuous production of green H2 on a regional basis through pipeline distribution.
Dimensioning Cost to Scale of Production
Figure 2 reports an example of the specific investment costs (thus CAPEX normalized per kW of electrical energy) for producing H2 by three type of electrolyzers (PEMEC: Proton Exchange Membrane Electrolyzer; AEC: Alkaline Electrolyzer; SOEC: Solid Oxide). These costs are reported as a function of the scaling effects (size of the electrolyzer) and technological learning (e.g., expected progresses from year 2020 to 2050). This technology learning is often difficult to estimate. Often progresses in the reduction of costs can be even larger than optimistic expectations, as teaches well the case of decrease of costs of photovoltaic electrical energy production.
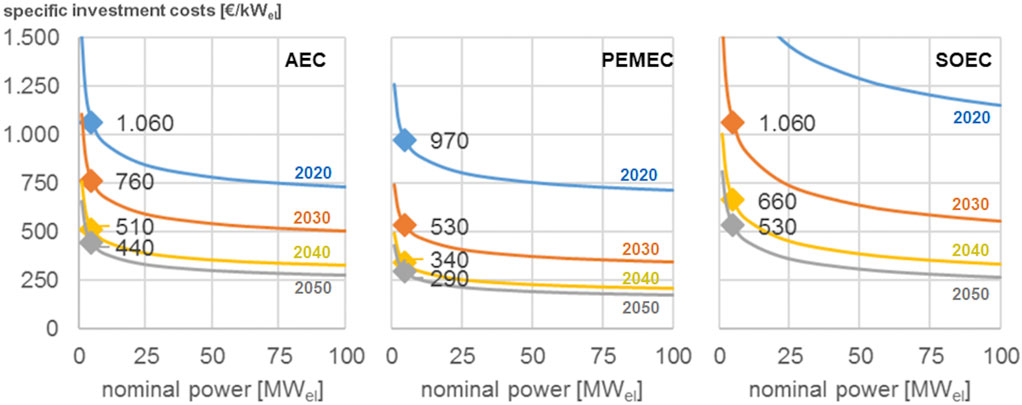
FIGURE 2. Cost development of H2 electrolyzers for three type of electrolyzers (PEMEC, Proton Exchange Membrane Electrolyzer; AEC, Alkaline Electrolyzer; SOEC, Solid Oxide) as function of the nominal power of the electrolyzers and estimated decrease in costs of production for different years in the 2020–2050 range. Elaborated from Zauner et al. (2019). No copyright.
These costs may be discussed, but this is not relevant here. The point evidenced from Figure 2 is that the costs (for example, for PEMEC electrolyzers) can change from less than 250 €/kWel to over 1,000 €/kWel (Zauner et al., 2019) which thus reflect in large differences in the production cost. Depending on which cost is chosen, the overall cost for the product of CO2 utilization can largely change. These aspects add to the other factors indicated before.
The scale factor in economic cost estimations is usually determined using the following relation:
The cost (cb) is related to a standard cost (ca) multiplied the ratio of the respective size (S) powered to a factor f, which typically is less than one and in the 0.6–0.9 typical range. This equation is used for single equipment, but it is practice the use also for plants, although may be not easy to find an appropriate reference, eg a plant with analogous characteristics. More correctly, although with a margin of error typically of ±30%, the process cost and thus CAPEX, is determined usually from the proper identification of the quantified process flowsheet and of the main equipment necessary and their size (Palo et al., 2019). Then, the cost for the various single equipment can be estimated as in Eq. 1.
Today, most of the engineering software for simulation already contain methods to calculate these costs (this is what typically made in many publications). The difference between estimations made by engineering companies experienced in the area is that they have an internal database of the effective costs typically not available for those at academic level using these software. Reliability of the results is thus largely different. From the cost of the equipment, the overall cost is estimated by adding typical percentages for all the other necessary elements (Palo et al., 2019). Again, there are large differences possible between estimations and their reliability based on this method, which can largely depend on the experience. Simulation software produce good results for relatively standard and established processes, but reliability in addressing new technologies in limited. Therefore, quantification of CAPEX costs can produce large differences. In CO2 utilization technologies, where CAPEX and its amortization procedure are crucial components for the final production cost, a proper analysis, not limited to the final number, is essential.
This should include a proper estimation of the degree of cost reduction by technological and industrial development, the first related to improvement in the technology efficiency, while the second, and often the more relevant, is the cost reduction related to larger-scale industrial manufacture. This could decrease by a factor up to 5–10, and thus a relevant aspect to consider, but difficult to predict.
Putting in the right context of the energy transition is another important element. It was introduced before the concept that if the time of utilization of electrolyzers is limited to the few hours of when surplus of renewable energy is available, cost can be high, being amortization charged on too few hours. On the other hand, if high full-load hours operations are considered (typically >5.000–6.000 h/h), a gray electricity mix should be used (today, on the average and in an optimistic view, 70‰ from fossil fuels and 30‰ from renewable sources). This will largely decrease the cost of production, depending on the type of renewable energy source.
Case Analysis
Zauner et al. (2019) estimated for year 2020 that methane cost (produced from CO2) is on the average 43 € cents/kWhLHV for electricity deriving from PV cells, around 27 € cents/kWhLHV for electricity produced from wind (which allow more extended times of operations, when low-cost electricity is available) and around 13 € cents/kWhLHV for electricity derived from the grid and 6,000 h/y operations. This time of operations is 68‰ of the full time theoretically possible and thus is a low prudential value. The usual time of operation for chemical processes is in the 86–90‰ of full time, depending on process reliability. Thus, further reduction of the cost is even possible. There is thus a factor four between the costs, depending on the time of operations.
Clearly, today a technology which uses CO2 and electricity deriving from fossil fuels has no meaning, but in the future, with a progressive increase of renewable energy in the energy mix, the use of electricity from the grid could be realistic. For this reason, Zauner et al. (2019) indicated that the costs of methane from CO2 could be in the 3–6 range (for year 2050) by combining technology development and cost reduction associated to this change in the energy mix. Thus, up to one order of magnitude less than the maximum estimated costs today. As a reference, the average cost of production of CH4 from CO2 given in the Nature paper of Hepburn et al. (2019) is 1739 $/t which translated to a unit as that indicated above is about 12 € cents/kWhLHV. The usual way to indicate costs for a gas fuel like methane is by volume, i.e. €/Nm3, a value currently around 0.2 (methane lower calorific power–PCI–i.e., the amount of energy released during the stoichiometric combustion of a fuel, is about 8,600 kcal/Nm3).
A correct reference to compare these values should be not fossil methane, which cost is largely associated to geo-political motivations, but rather biomethane. International Energy Agency (IEA) in a recent report (IEA, 2020) indicates biomethane production costs ranging from 3 to over 20 USD/MBtu (Btu is the British thermal unit) in 2018 depending on the sources (crops, manure, municipal solid waste or wastewater) and potential global biogas demand. Currently (year 2020) natural gas has a value around 2 USD/MBtu in US, 5–7 in Europe and 10 in Japan). The costs of biomethane tendentially decrease over time, narrowing the cost gap with projected natural gas prices. By considering as reference 19 USD/MBtu, this translate to about 50 € cents/kWhLHV. However, a fraction of potential biomethane (up to 30 Mtoe) has a cost of around 10–15 cents/kWhLHV, which is in the range of competition with the domestic price of natural gas.
IEA report indicates that the biomethane actual production (35 Mtoe) is only a fraction of the potential (730 Mtoe) and is expected to increase to 200 and 330 Mtoe for years 2030 and 2040, respectively, in a sustainable development scenario. Thus, these data well evidence that producing methane from biogenic CO2 sources (which can be also the fraction of CO2 present in the biogas) and green H2 is a route economically in line with biomethane (e.g., the methane fraction in biogas). It is a well reasonable approach to reduce carbon footprint of biogas production by utilization of the CO2 component of biogas and its methanation, thus integrating biogas with renewable energy sources (Abate et al., 2015b). Thus, putting in the right perspective of energy transition, economics of CO2 methanation indicates a potential large impact, in contradiction to the cited Nature paper of Hepburn et al. (2019).
The same remark could be applied to the case of CO2 to methanol. Here the difference with respect to methane is that methanol is a liquid and can be thus easily transported and stored, by ship for example. Methanol can be thus used to establish a world trading of renewable energy stored in a liquid (Barbato et al., 2014), e.g., the equivalent of current fossil-centric energy system, largely associated to the possibility of easy transport of oil. Methane could be liquefied, but this is an energy-intense operation. It can be transported via pipeline, but where the network exists, and also storage can be possible underground, but in specific geological situations. Thus, flexibility of use is strongly reduced.
With the concept of using methanol as chemical energy storage (CES) vector, it is possible to bypass the limit of the use of excess renewable energy sources, using instead sources around the world where can be produced at economic costs. There is still large potential, but untapped, for hydropower sources which cannot be exploited, being in geographical areas where the produced electrical energy cannot be exploited. This concept will be further discussed later, but it is worth to comment here that the main advantage of hydropower as renewable electrical source is that it allows a continuous production of energy, without the fluctuations present in other cases (PV, wind). This is, as commented before, a crucial factor for economics.
There are many areas, deserts for example, where a combined availability of PV and wind production exists, which together with available technologies for short-term storage of electrical energy, could guarantee a constant production. In terms of impact on the land use and on the population, these areas do not have specific issues, and instead their utilization can have a positive impact on precipitations (around doubled) and the land fraction of vegetation covered (Li et al., 2018). This is a combination of reduced albedo, creation of microclima, effect on wind, etc. Deserts make up to one third of the land’s surface area. Just 1/18 of Saharan Desert is enough to fuel (with PV at the current efficiency level) the world energy need. These are just indicative numbers, but remarking that there is not a potential limitation in terms of land use and potential impact on population. The current limit is the transport of renewable electrical energy, but CES technology could solve it. Thus, the potential impact is large, in Gton scale (as commented later), differently from what indicated in the cited Nature paper of Hepburn et al. (2019). In terms of economics, as discussed later, this is a potentially attractive solution, although it is not important here to demonstrate this point.
The relevant point here is that without putting well in the context of energy transition and future scenario, the economics of CO2 and the impact of CO2 utilization technologies cannot give the right indications. It is necessary not just use numbers but enter in detail and analyze how estimations have been made, which limits they have, which assumptions have been made, how these are valid out of the specific context. In terms of assessment, there is the need to also have a broader model of analysis, as commented later. Techno-economic estimation are and remain a very valuable instrument, but that should be used in the proper way. It is necessary to have well in mind the advantages and the limits. This is even more relevant when a system change is present, with thus also major changes in the full value chain, supply-demand, and technology requirements.
Extending the Method to Assess the Techno-Economic Impact
Zimmermann et al. (2020) recently discussed that there are no generally accepted techno-economic assessment (TEA) methods and often “apples vs. oranges” are compared with thus low transparency and readability, as remarked also in the introduction. They thus developed guideline for TEA and LCA technologies for the case of CO2 utilization technologies. These guidelines will be shortly summarized here for the main relevant aspects for the discussion. An initial recommendation they made is that TEA should start from the identification of the context, the intended use and the limitations, concepts which are well coincident with what discussed above.
Zimmermann et al. (2020) indicated that TEA should follow the concepts of LCA in terms of structuring the approach and different phases of the analysis: goal and scope, inventory, calculation of indicators, interpretation, and reporting. TEA should state clearly and unambiguously 1) the study context (location, time horizon, scale and partners, etc.), 2) the intended application and motivations (like decision support for R&D funding allocation, investment decisions or policy, etc.), and 3) the target audience (e.g.,, R&D experts, funding agencies, investors, etc.). In addition, being TEA studies functional for decision making having also long-term implications, scenario analysis should be adequately described. System boundaries should be clearly defined and be consistent with the TEA goal and perspective. Up- and down-stream units (for example, CO2 capture, separation and transport processes, hydrogen or electricity production, etc.) should be clearly defined if included or not, but consistently with parallel LCA studies. A proper benchmarking is necessary.
Criteria and assessment indicators should be also correctly defined. After the goal and scope phase, the inventory phase should follow. Data quality, what are the collected data, how a CO2 price is derived, how data for other inputs (hydrogen, electricity, and other materials) are obtained, what are the documents of the inventory data are among main elements proving the input for calculations. The latter should include best practices and economic indicators (including, but not limited, to CAPEX/OPEX). Finally, interpretation is the final phase, which should include uncertainty and sensitivity analysis, interpretation of indicators and multicriteria decision analysis. Reporting concludes the assessment.
This procedure formalizes the mechanism, such as the ISO 14044 norms formalize the LCA methodology. Thus, it guarantees a better transparency of the data, and their usability, but do not addresses the key of the remarks discussed before. They require a step-change in the procedure, and how to integrate in the strategy of development and in general in a novel engineering approach to assess processes based on the use of renewable energy and alternative carbon sources (Centi at al., 2019b). Suitable novel indicators for both economics and LCA have also to be developed.
We early remarked that the traditional economic models and concepts, such as scale-economy, does not well account for the analysis of emerging CO2 utilization technologies. New assessment tools are required. They need to include relevant elements, which are not currently present (or present in a too limited amount) in TEA methodologies, such as “the capability to analyze socio-economical macro-trends, market evolution, competitiveness related to entire ecosystems, sustainability and integration into territory (rather than globalization), non-linear dynamic of changes and costs evolution, extended life-cycle cost and social analysis” (Centi at al., 2019b).
Note, that also a trend in LCA is to expand the scope and a series of new LCA methodologies have been developed, for example Life Cycle Cost analysis (LCC), Social or Environmental LCA (S-LCA, E-LCA) which can then all be integrated in Life Cycle Sustainability Analysis (LCSA) (Heijungs et al., 2013). The application of these novel methods to CO2 utilization cases, however, is still limited (von der Assen et al., 2014). Although LCSA accounts better of climate change issues and biodiversity and offers a more holistic perspective, as all the LCA methods, they are implemented largely through the use of database, rather than on actual data and effective specific, process-linked, analyses. They could be thus not a substitution of TEA, but allow to integrate the specific process in its and its input/output life cycles, i.e. from “cradle-to-grave.”
A Case Example: Economics of CO2 to Methanol
Producing Methanol to Import Renewable Energy From Remote Areas
To complete the discussion of the economics of CO2 utilization, a specific example will be discussed in this section: the conversion of CO2 to methanol which is used to transport renewable energy from remote areas (the specific example, refers to hydropower produced in Chile, where a large potential production is present, but which cannot be exploited locally) (Barbato et al., 2014). CO2 is transported by ship, while methanol transported back in the same ship, a technology current feasible. This is thus an example of using untapped remote renewable energy sources. Hydropower allows to have a continuous production, here considering a capacity factor of 320 days per year.
What is the potential impact of this technology? This was estimated by considering the potential power available worldwide as untapped renewable energy, either of hydropower and of PV + wind sources in remote areas, such as deserts. Prudential estimation is of an amount of 10 PWh per year of additional renewable energy that could be exploited by enabling an effective route for the conversion of electrical to chemical energy to store and transport renewable energy. 10 PWh correspond to 7 Gt of CO2 equivalent emissions saved. There are some energy losses in the process, but the message is that the potential is in the Gt range, thus well equivalent or even superior than many alternatives.
Often it is questioned that the storage in fuels is not relevant, because CO2 is re-emitted. As commented before, the point is that the fuel provides a service (energy, heating etc.) than otherwise should be produced by using fossil fuels. Thus, the GHG impact is in terms of avoidance of use of fossil fuels at equivalent service, rather than in terms of sequestration of CO2. Nevertheless, the rational approach is to re-capture CO2. This is possible, for example by using methanol in turbines to generate electrical energy and heat, with integrated recapture of CO2 (an available technology, by using pressurized turbines and CO2 separation membranes, technologies already available). Thus, a closed-cycle could be realized, with an efficiency in storing renewable energy of the order of 50–60‰ (Barbato et al., 2014), which is well aligned to current large energy-storage technologies, for example, pumped hydroelectric energy storage (PHES). Note that for efficiencies >50‰ in a close-loop approach, the technology will act as a negative CO2 emission technology.
From the technology side, as indicated, there are already various pilot units that have developed the conversion of CO2 to methanol with green H2, generally produced using electrolyzers. Still some degrees of improvement are possible but may be considered that the technology is currently nearly mature for exploitation, also for remote and small-scale productions, requiring efficient and compact units. Electrolyzers are also commercially available. Improvements in terms of stability and pressure of operation could improve further the performances and operability, and the integration with the catalytic step, (for example, operations without the need to compress H2, a costly element). Even if simplified as analysis, it may be concluded that there are not significant current technical limitations to implement the technology, just a “normal” further technological progress as necessary in exploiting new technologies.
The question is thus the cost of producing methanol. The cited paper by Barbato et al. (2014) reports all the details. Figure 3 reports the simplified process flowsheet used to estimate, by process simulation, the production cost of methanol from CO2 using green H2 from electrolysis with electrical energy produced by hydropower. There are five major process blocks:
(1) Production of renewable electricity using a dedicated hydropower unit, which costs are integrated in the calculations;
(2) Production of green H2 using AEC electrolyzers (operating a 15 bar, as the current state of the art of commercial units);
(3) Capture of CO2 (from concentrated and rather pure sources, as some stream in refinery or in biogas units, after methane separation), and transport to a 1,500 km distance by ship after drying and compression at 75 bar (using Carbotube 75 technology proprietary of the Maire Tecnimont Group; transport costs are based on their commercial experience);
(4) Preparation of the feed mixture for the methanol step, by compressing and mixing CO2/H2 and then converting part of the CO2 in a RWGS reaction (using commercial copper-based catalysts) to produce a CO/CO2/H2 mixture suitable for the methanol synthesis; water, which is produced in RWGS step, is removed by a conventional drying technology;
(5) Catalytic synthesis of methanol (using commercial copper-zinc-oxide based catalysts), followed by purification and storage; methanol is then transported back using the same ships used to transport CO2.
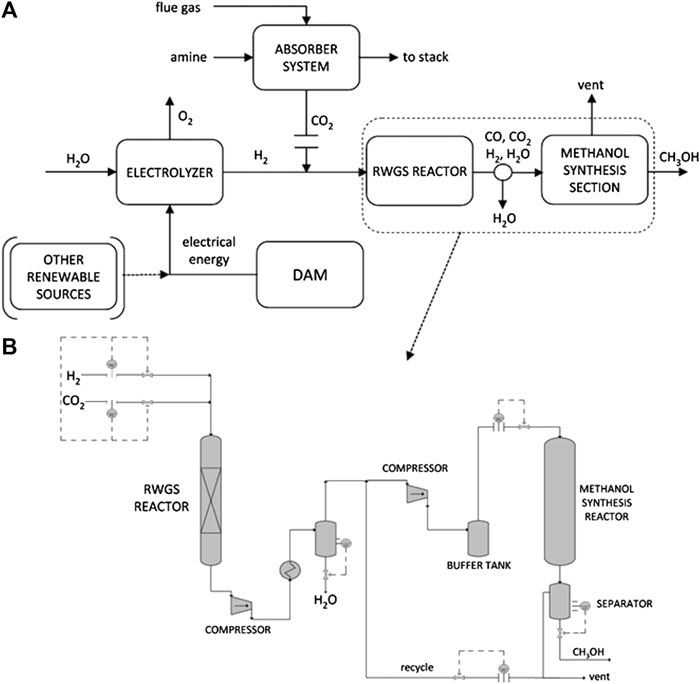
FIGURE 3. A) Simplified block diagram of different material flows participating in the process of methanol synthesis from CO2 and renewable H2. (B) Simplified flowsheet for the section of the RWGS reactor and methanol synthesis system. Reproduced with permission from Barbato et al. (2014).
Note that CO2 may be converted also directly to methanol, without a first RWGS reactor (the catalysts active for methanol synthesis are also active for RWGS). Separation in two stages, with intermediate water elimination, adds costs (although not very large), but avoid the inhibition by water on the reaction rate of methanol production. Developing hydrophobic methanol synthesis catalysts, thus less sensitive to inhibition by water allows thus to make more effective the direct CO2 to methanol conversion, thus saving on CAPEX costs.
Reliability of Estimations
The H2 production by hydropower is assumed €0.140 per Nm3 H2, which is the current cost of electrolysis for large-scale H2 production with an H2 efficiency in the range of 62–65‰, a currently feasible efficiency. This would correspond to 4.5–4.8 kWh per Nm3 of H2 produced. The cost of CO2 capture was estimated by considering a conventional CO2 absorption amine process, which is a robust and available technology. It uses an aqueous solution with 30‰ by weight monoethanolamine (MEA). Cost for CO2 capture are based on actual costs for this technology used in other processes, and thus are quite reliable values. There is a growing research on alternative and less expensive capture technologies, which can reduce the cost of CO2 capture by over 20‰. Margins to reduce further the costs are thus present.
Note that in general in all CO2 utilization technologies, the cost of capture/purification of CO2 can be significant, up to half of the overall cost (Schlögl et al., 2018). Thus, decreasing these costs are a crucial element for future developments. Note that, although not valid for this specific case but for other relevant cases as the direct conversion of CO2 by artificial-like photosynthetic devices, elimination of the step of a separated CO2 capture unit may be possible. For example, by including membrane-like systems able to capture CO2 directly and transfer to the machinery for its photoelectrocatalytic conversion (as occurs in leaves). In other cases, CO2 nearly pure (as in biogas after separation of methane, or in fermentation processes, but also in some refinery cases), could be available. Thus, cost of separation/purification of CO2 could strongly depend on the specific case analyzed. The main key economic parameters used to estimate the methanol production costs are summarized in Table 1 (Barbato et al., 2014).
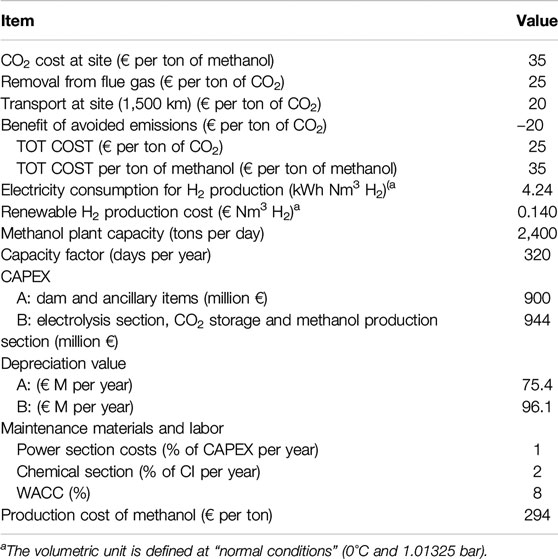
TABLE 1. Key economic parameters used to estimate the methanol production costs. Reproduced with permission from Barbato et al. (2014).
CO2 is captured in the examined case at refinery sources, where it is available with good concentration and purity. The recovered CO2 is first dehydrated (to prevent wet carbonate corrosion) and then compressed up to 75 bars before shipping. Shipping is made with CO2 cargos at a distance of 1,500 km. CO2 is stored in the ship in tanks filled with a carbon-based support (Carbolite). This is a commercially available solution, which allows to use the ships to transport back methanol. Shipping costs has a low impact on the overall costs, thus changing the distance has not a relevant impact.
While on-step direct CO2 conversion is possible, in this example it was preferred to have a first stage of reverse water gas shift–RWGS (to form thus CO from CO2) followed by catalytic CO2-rich syngas conversion. Water removal is realized with conventional techniques between these two stages. There are degrees of improvement in terms of 1) better (more productive) and more stable catalysts (less sensitive to higher water content and CO2 concentration in the feed with respect to commercial methanol catalysts), 2) use of microreactors to intensify the process and more efficient control of the reaction temperature profile (it is an exothermic reversible reaction), 3) optimized integration with the electrolyzer, and 4) efficient heat integration. There is thus also here the possibility to decrease further the costs by about 15–20‰. However, it is a prudential approach to evaluate, as base case, a reliable state-of-the-art technology.
The costs, although estimated with a simplified method based on the quantified flow sheet and cost for main equipment, with then estimation of final CAPEX by fixed percentages for other components, are reliable within the indicated ±30 range. Due to in-house available experience on analogous equipment and plants in building commercial units, the estimation results robust and reliable. OPEX are estimated by considering operating and maintenance (O&M) and variable operating costs (VOC). CAPEX is the predominant factor influencing the overall cost of production (about 75‰). CAPEX has been split in two main components. The first is related to produce the electrical energy by hydropower. This investment cost for hydropower unit construction was estimated in 1,000 € kW−1, a current realistic cost indicated by hydropower plant producers. For this part of the investment, the depreciation rate was calculated using a 40-year period. For the second part of the investment, related to the costs of the electrolysis and methanol synthesis units, the depreciation value was calculated using a 20-year period. It was then assumed to calculate the depreciation rate for a WACC of 8‰. Currently (year 2020), this value is significantly lower, i.e., < 4–5‰. This lowers the depreciation costs.
Cost of CO2 at site (thus including capture and delivery) was considered 35 € per ton of methanol, considering that one-ton CO2 produces 0.77 tons methanol. Cost is low but includes 20 € per ton CO2 of benefit for avoided emissions. This is a reasonable assumption, although may be different case to case. Costs were calculated for a methanol plant capacity of 2,400 tons per day. Note that as indicated before, the costs are calculated including those related to produce the hydropower unit. Often instead costs are estimated by using commercial values for renewable electrical energy and not including the CAPEX for dedicated renewable electrical energy production.
Thus, all the elements of the calculation are valid and reliable. Based on these indications, the cost of production of methanol was about 300 € per ton. The cost is largely lower than that reported in the cited Nature paper of Hepburn et al. (2019) and in many of other TEA studies (Figure 1). This cost makes competitive to use this technology to import untapped renewable energy sources. Contract price for methanol, as a general indication, may be considered around 300 €/t.
Impact and Market
Beginning year 2020 the methanol average market price was about 270 €/t, with European spot prices averaging around €205/t during the fourth quarter of 2019, but this year prices and forecasts are largely affected from coronavirus outbreak. New methanol productions started in Iran, Trinidad and the US, and MTO-based olefin production (MTO–methanol to olefin; a major process consuming methanol) is running near-peak levels, while conventional (from oil) olefin and olefin-derivative capacity in Asia-Pacific was growing.
Thus, short term forecasts for methanol are not positive, but the possibility to use methanol as biofuel (when produced from waste or biogenic CO2) in substitution of bioethanol, suffering of increasing costs and social concerns, opens new prospects and markets. These brief notes just to evidence the difficulty in predicting market value trends for chemicals and thus in making reliable conclusions about the use of new technologies.
A Comparison with Literature
How compare indications above with other literature results? Here the aim is not to provide an analysis with all published data. In the cited Nature paper of Hepburn et al. (2019) they reported to have analyzed 24 papers in total on methanol production case, arriving to the conclusion of a cost of methanol production of 510 $/t, i.e., about 465 €/t, about 40‰ higher with respect to that indicated in Table 1. This could make a significant difference between possibility of application of the technology, as indicated by Barbato et al. (2014) and consideration instead of a marginal possible impact, as indicated by Hepburn et al. (2019). It results thus interesting to analyze as exercise some other estimations on CO2 economics for methanol production.
Pérez-Fortes et al. (2016) reported a techno-economic and environmental assessment of the process of methanol synthesis using captured CO2. The two main conclusions were that 1) “production costs results too high for a financially attractive project”, and 2) “there is a net potential for CO2 emissions reduction of 2.71 MtCO2/yr in Europe.” Thus, quite in contrast with those discussed above, and it is thus interesting to analyze more in-depth the reasons of the different conclusions.
The conceptual design of the process of capture CO2 and catalytic conversion to methanol is made by Pérez-Fortes et al. (2016) using ChemCad commercial software, which is used for academic exercises, but typically not in engineering societies. Aspen is another simulation software often used in TEA studies, but not in engineering societies which use for estimation of the purchased equipment costs mainly in-house direct values on analogous systems. H2 generation and transport is not included within the boundary limits of calculations, but it is supposed will arrive to CO2 conversion plant through a pipeline. The price of H2 is 3,090 €/t. This is one of the critical estimations, because influences the conclusions of a negative gross margin for the methanol plant, because "the H2 cost surpasses the revenues obtained by selling the methanol" and determines that "the total cost of production is almost 1.7 times the expected revenue" (Pérez-Fortes et al., 2016).
Just as a reference, one ton of methanol requires 0.189 tons H2, thus for a CH3OH market value of 400 €/t, the cost of H2 results thus higher (580€ for a hydrogen price of 3,090 €/t). It is certain that with this H2 cost CO2 economics to methanol result negative. The problem is thus to analyze whether a lower H2 production cost is possible. Therefore, an integrated production of low-cost green H2 which guarantee continuous operations has to be considered, as made in the Barbato et al. (2014) paper. They include CAPEX for construction of a dedicated hydropower plant, but the result is a largely lower H2 production cost.
CO2 capture and transport, and further purification steps of the CO2 stream are also out of the boundary limits considered by Pérez-Fortes et al. (2016). Sensitivity with respect to this and H2 cost were calculated by these authors for their estimations. For base case price (€/t) of a methanol cost of 400 €/t, they reported that CO2 and H2 cost should be 0 and 3,090 €/t, respectively. This would correspond to a net present value NPV of −1,036.2 M€ for a project of 20 years. NPV represents the present value of a cash flow, thus a negative value indicates that the entrance due to selling the products are lower with respect to costs. To obtain a NPV of zero, they estimated that the CO2 cost should be negative (−222 €/t) and H2 cost also nearly half (1,453 €/t), with a market value for methanol of 724 €/t. From here the conclusion, that the process is not economic, having considered 400 €/t the commercial product value for methanol. As commented before, Barbato et al. (2014) estimated instead a cost of production of methanol of nearly 300 €/t (thus lower than the market value) by using an on-site (integrated) production of H2 by electrolysis using the electrical energy of a dedicated hydropower unit.
A closer look at the results of Pérez-Fortes et al. (2016) with respect to those in the Barbato et al. (2014) paper reveals also that CAPEX and OPEX costs are highly different, even if the process scheme is not so different to justify the difference. CAPEX indicated by Pérez-Fortes et al. (2016) is 496.5 €/(tCH3OH/y) for a methanol production is 1,320 t/day. CAPEX for Barbato et al. (2014) is 61 €/(tCH3OH/y) for a 2,400 t/day, excluding CAPEX for producing the electrical energy, in order to have comparable data. OPEX (assuming the same capacity factor) are 58% of the total costs for Pérez-Fortes et al. (2016), while 25% of the total costs for Barbato et al. (2014). It is thus evident that there are very different ways to estimate the costs, which produce these large differences and thus reflect also in the final costs and derived conclusion on competitiveness and impact of the technology.
It is not the scope here to comment which of these two estimations is the more reliable. The aim is to remark only that without entering in the details of the calculations and estimation, the final conclusions itself have limited value. The same analysis could be applied to other estimations of TEA for methanol productions or other CO2 economic estimations. The examples discussed represent thus only practical cases rather than be model for correct or not procedures. As a final comment, the focus here on only economics aspects, rather than also on technological one, does not intend to minimize the crucial role of technology development, including for example creating and mastering nano-objects to develop next-generation of catalytic materials (Centi and Perathoner, 2011). All these technological aspects have a decisive role in the progress and practical use of the CO2 utilization route. Limiting discussion to economics is just to focus the analysis.
Conclusions
The economic assessment of new processes for CO2 utilization is a crucial element to establish the impact and contribution of CCU to GHG reduction and climate mitigation. Discussion was focused on Power-to-X (methanol, methane), being that studied in a more detail from an economic perspective among CCU technologies. There are many areas of growing interest for CO2 utilization, for example that of electrocatalytic processes (Genovese et al., 2013; Perathoner and Centi, 2019), but they are at a much lower level of industrial development. Only the area of CO2 utilization for production of CO2-containing polymers can be considered at an equal level of development. However, in these cases, the interest is as low-cost carbon source, rather than as effective technologies to mitigate climate changes.
It is necessary to stress again that this is not a review of economics of CO2 utilization, neither a didactic presentation of how to estimate these economics. It is rather a conceptual perspective contribution around a key question: how reliable literature data on CO2 economics are, and related estimation of the impact on GHG emissions and climate change mitigation (Centi et al., 2013; Centi and Perathoner, 2014). The starting point is Figure 1, based on state-of-the-art analysis of literature indications on economics of Power-to-X technologies. Data show the very large spread of results in estimating the cost of production of methanol and methane from CO2 by using renewable energy source. This Figure clearly evidences that without a guide to analyze these results, it is not possible to use some or few of these results to make conclusions, whether or not these are technologies suitable to contribute significantly to GHG emission reduction.
In order to provide this guide for interpretation of literature results on CO2 economics, we have analyzed here some of the questions, and methods related to CO2 economics as a procedure to assess the feasibility of the CO2 utilization processes, and thus also their potential impact. Although with a focus on Power-to-X technologies for CO2 conversion to methanol and methane (Centi and Perathoner, 2020), the discussion has a more general relevance for all the area of the so-called CO2 utilization technologies. In fact, here the attention was to comment on the methodologies to analyze CO2 economics and their limit and validity, using some examples to clarify these aspects. Note also that the objective was not to indicate what is the effective costs, but to provide the basic elements to understand the values reported in literature and the limits related to the analysis.
The need of a proper contextualization of the results was remarked, particularly in relation to the impact which is strongly associated not only to economics, but also to a proper identification of the future value chain. A correct prospects analysis requires to go beyond the current often too limited approaches (as considering CO2 utilization as a storage option) and have a broader view in relation to the system change related to energy (and chemistry) transition.
Note as final remark that we have utilized here the term CCU only as utilization, and not as the full definition of CCU (carbon capture and utilization) or CCSU (carbon capture, storage and utilization). These acronyms indicate the categorial need to have a carbon capture step, and indirectly also that utilization is just a complementary option to storage of CO2. We have commented in the text that both indications are not correct, neither lead to a correct evaluation of the economics and impact. From a semantic definition, note also that the definition "perennial energy sources" is more correct with respect to that of "renewable energy sources", although the latter is more extensively used and thus utilized also here.
In conclusion, we hope that this critical perspective provides some useful elements for a better analysis of the economics of chemical processes in the area of CO2 utilization and providing also some guidelines on how to account for techno-economic results and their impact on GHG reduction.
Author Contributions
All authors contributed equally to the discussion and analysis, while CG wrote the text that was revised and approved by co-authors.
Funding
CG and SP thanks the ERC Synergy SCOPE (project 810,182) and PRIN 2017 “CO2 ONLY” project nr. 2017WR2LRS, respectively, for the financial support in the realization of this work.
Conflict of Interest
Authors GI and AS were employed by the company NEXTCHEM.
The remaining authors declare that the research was conducted in the absence of any commercial or financial relationships that could be construed as a potential conflict of interest.
Glossary
AEC, Alkaline Electrolyzer BECCS Bio-Energy with Carbon Capture and Storage; CAPEX, CAPital EXpenditure; CES, Chemical Energy Storage; CCSU, Carbon Capture, Storage and Utilization; CCU, Carbon Capture and Utilization; E-LCA, Environmental LCA; EOR, Enhanced Oil Recovery; GHG, GreenHouse Gas; IPCC, Intergovernmental Panel on Climate Change; LCA, Life Cycle Assessment; LCC, Life Cycle Cost; LCSA, Life Cycle Sustainability Analysis; LHV, Lower Heating Value; MEA, Mono Ethanol Amine; MTO, Methanol to Olefin; Mtoe, Million tons of oil equivalent; NPV, Net Present Value; O&M, Operating and Maintenance; OPEX, OPerative EXpenses; PEMEC, Proton Exchange Membrane Electrolyzer; PtX, Power-to-X, including Power-to-Gas (PtG or P2G) and Power-to-Liquid (PtL or P2L); PHES, Pumped Hydroelectric Energy Storage; PV, PhotoVoltaic; RWGS, Reverse Water Gas Shift; S-LCA, Social LCA; SOEC, Solid Oxide Electrolyzer; TEA, Techno-Economic Assessment; TRL, Technology Readiness Level; VOC, Variable Operating Costs; WACC, Weighted Average Cost of Capital.
REFERENCES
Abate, S., Centi, G., Lanzafame, P., and Perathoner, S. (2015a). The energy-chemistry nexus: a vision of the future from sustainability perspective. J. Energy Chem. 24, 535–547. doi: 10.1016/j.jechem.2015.08.005
Abate, S., Lanzafame, P., Perathoner, S., and Centi, G. (2015b). New sustainable model of biorefineries: biofactories and challenges of integrating bio- and solar refineries, Chem. Sus. Chem. 8, 2854–2866. doi: 10.1002/cssc.201500277
Alsayegh, S., Johnson, J. R., Ohs, B., and Wessling, M. (2019). Methanol production via direct carbon dioxide hydrogenation using hydrogen from photocatalytic water splitting: process development and techno-economic analysis. J. Clean. Prod. 208, 1446–1458. doi: 10.1016/j.jclepro.2018.10.132
Ampelli, C., Perathoner, S., and Centi, G. (2015). CO2 utilization: an enabling element to move to a resource- and energy-efficient chemical and fuel production. Phil. Trans. R. Soc. A 373, 1–35. doi: 10.1098/rsta.2014.0177
Aresta, M., Caroppo, A., Dibenedetto, A., and Narracci, M. (2002). “Life cycle assessment (LCA) applied to the synthesis of methanol. Comparison of the use of syngas with the use of CO2 and dihydrogen produced from renewables,” in Environmental challenges and greenhouse gas control for fossil fuel utilization in the 21st century. Editors M. M. Maroto-Valer, C. Song, and Y. Soong (New York, NY: Springer US), 329–347.
Aresta, M., Dibenedetto, A., and Angelini, A. (2013). The changing paradigm in CO2 utilization. J. CO2 Util. 3–4, 65–73. doi: 10.1016/j.jcou.2013.08.001
Asif, M., Gao, X., Lv, H., Xi, X., and Dong, P. (2018). Catalytic hydrogenation of CO+ from 600 MW supercritical coal power plant to produce methanol: a techno-economic analysis. Int. J. H2 Energy 43, 2726–2741. doi: 10.1016/j.ijhydene.2017.12.086
Atsonios, K., Panopoulos, K. D., and Kakaras, E. (2016). Investigation of technical and economic aspects for methanol production through CO2 hydrogenation. Int. J. H2 Energy 41, 2202–2214. doi: 10.1016/j.ijhydene.2015.12.074
Bailera, M., Lisbona, P., Romeo, L. M., and Espatolero, S. (2017). Power to gas projects review: lab, pilot and demo plants for storing renewable energy and CO2. Renew. Sustain. Energy Rev. 69, 292–312. doi: 10.1016/j.rser.2016.11.130
Barbato, L., Centi, G., Iaquaniello, G., Mangiapane, A., and Perathoner, S. (2014). Trading renewable energy by using CO2: an effective option to mitigate climate change and increase the use of renewable energy sources. Energy Technol. 2, 453–461. doi: 10.1002/ente.201300182
A. Basile, G. Centi, M. De Falco, and G. Iaquaniello (Editors) (2019). “The vision of future sustainable energy, catalyst, and chemistry: opportunities for innovation and business,” in Catalysis, green chemistry and sustainable energy. Studies in surface science and catalysis. Amsterdam, Netherlands: Elsevier B.V., Vol. 79, 7–20.
Bos, M. J., Kersten, S. R. A., and Brilman, W. F. D. (2020). Wind power to methanol: renewable methanol production using electricity, electrolysis of water and CO2 air capture. Appl. Energy 264, 114672. doi: 10.1016/j.apenergy.2020.114672
Čejka, J., Nachtigall, P., and Centi, G. (2018). New catalytic materials for energy and chemistry in transition. Chem. Soc. Rev. 47, 8066–8071. doi: 10.1039/C8CS90119H
Centi, G., and Čejka, J. (2019a). Needs and gaps for catalysis in addressing transitions in chemistry and energy from a sustainability perspective. ChemSusChem 12, 621–632. doi: 10.1002/cssc.201802637
Centi, G., Iaquaniello, G., and Perathoner, S. (2019b). Chemical engineering role in the use of renewable energy and alternative carbon sources in chemical production. BMC Chem. Eng. 1, 5. doi: 10.1186/s42480-019-0006-8
Centi, G., and Perathoner, S. (2009). Catalysis: role and challenges for a sustainable energy. Top. Catal. 52, 948–961. doi: 10.1007/s11244-009-9245-x
Centi, G., and Perathoner, S. (2020). Chemistry and energy beyond fossil fuels. A perspective view on the role of syngas from waste sources. Catal. Today 342, 4. doi: 10.1016/j.cattod.2019.04.003
Centi, G., and Perathoner, S. (2011). Creating and mastering nano-objects to design advanced catalytic materials. Coord. Chem. Rev. 255, 1480–1498. doi: 10.1016/j.ccr.2011.01.021
Centi, G., and Perathoner, S. (2014). Green carbon dioxide: advances in CO2 utilization. Hoboken, NJ: Wiley Publisher. ISBN-10: 9781118590881
Centi, G., Perathoner, S., and Rak, Z. S. (2003). Reduction of greenhouse gas emissions by catalytic processes. Appl. Catal. B Environ. 41, 143–155. doi: 10.1016/s0926-3373(02)00207-2
Centi, G., and Perathoner, S. (2010). Towards solar fuels from water and CO2. Chem. Sus. Chem. 3, 195–208. doi: 10.1002/cssc.200900289
Centi, G., Quadrelli, E. A., and Perathoner, S. (2013). Catalysis for CO2 conversion: a key technology for rapid introduction of renewable energy in the value chain of chemical industries. Energy Environ. Sci. 6, 1711–1731. doi: 10.1039/c3ee00056g
Centi, G., Trifiró, F., Perathoner, S., and Cavani, F. (2009). Sustainable industrial processes. Weinheim, Germany: Wiley VCH Publishers. ISBN 978-3-527-31552-9
Chehade, Z., Mansilla, C., Lucchese, P., Hilliard, S., and Proost, J. (2019). Review and analysis of demonstration projects on power-to-X pathways in the world. Int. J. Hydrogen Energy 44, 27637–27655. doi: 10.1016/j.ijhydene.2019.08.260
Chwola, T., Spietz, T., Wieclaw-Solny, L., Tatarczuk, A., Krotki, A., Dobras, S., et al. (2020). Pilot plant initial results for the methanation process using CO2 from amine scrubbing at the Laziska power plant in Poland. Fuel 263, 116804. doi: 10.1016/j.fuel.2019.116804
De Saint Jean, M., Baurens, P., Bouallou, C., and Couturier, K., (2015). Economic assessment of a power-to-substitute-natural-gas process including high-temperature steam electrolysis. Int. J. Hydrogen Energy 40, 6487–6500. doi: 10.1016/j.ijhydene.2015.03.066
Dimitriou, I., García-Gutiérrez, P., Elder, R. H., Cuéllar-Franca, R. M., Azapagic, A., and Allen, R. W. K. (2015). Carbon dioxide utilisation for production of transport fuels: process and economic analysis. Energy Environ. Sci. 8, 1775–1789. doi: 10.1039/c4ee04117h
Do, T. N., and Kim, J. (2019). Process development and techno-economic evaluation of methanol production by direct CO2 hydrogenation using solar-thermal energy. J. CO2 Util. 33, 461–472. doi: 10.1016/j.jcou.2019.07.003
IPCC Climate Change (2014). IPCC Climate Change 2014: mitigation of climate change. Editors Edenhofer, O. (Cambridge: Cambridge University Press).
Garcia-Herrero, I., Cuéllar-Franca, R. M., Enríquez-Gutiérrez, V. M., Alvarez-Guerra, M., Irabien, A., and Azapagic, A. (2016). Environmental assessment of dimethyl carbonate production: comparison of a novel electrosynthesis route utilizing CO2 with a commercial oxidative carbonylation process. ACS Sustain. Chem. Eng. 4, 2088–2097. doi: 10.1021/acssuschemeng.5b01515
Genovese, C., Ampelli, C., Perathoner, S., and Centi, G. (2013). Electrocatalytic conversion of CO2 on carbon nanotube-based electrodes for producing solar fuels. J. Catal. 308, 237–249. doi: 10.1016/j.jcat.2013.08.026
González-Aparicio, I., Pérez-Fortes, M., Zucker, A., and Tzimas, E. (2017). Opportunities of integrating CO2 utilization with RES-E: a power-to-methanol business model with wind power generation. Energy Proc. 114, 6905–6918. doi: 10.1016/j.egypro.2017.03.1833
González-Garay, A., Frei, M. S., Al-Qahtani, A., Mondelli, C., Guillén-Gosálbez, G., and Pérez-Ramírez, J. (2019). Plant-to-planet analysis of CO2-based methanol processes. Energy Environ. Sci. 12, 3425–3436. doi: 10.1039/c9ee01673b
Grim, R. G., Huang, Z., Guarnieri, M. T., Ferrell, J. R., Tao, L., and Schaidle, J. A. (2020). Transforming the carbon economy: challenges and opportunities in the convergence of low-cost electricity and reductive CO2 utilization. Energy Environ. Sci. 13, 472–494. doi: 10.1039/c9ee02410g
Gutiérrez-Martín, F., and Rodríguez-Antón, L. M. (2016). Power-to-SNG technology for energy storage at large scales. Int. J. Hydrogen Energy 41, 19290–19303. doi: 10.1016/j.ijhydene.2016.07.097
Hank, C., Gelpke, S., Schnabl, A., White, R. J., Full, J., Wiebe, N., et al. (2018). Economics and carbon dioxide avoidance cost of methanol production based on renewable hydrogen and recycled carbon dioxide: power-to-methanol. Sust. Energy Fuels 2, 1244–1261. doi: 10.1039/c8se00032h
Hannula, I. (2015). Co-production of synthetic fuels and district heat from biomass residues, carbon dioxide and electricity: performance and cost analysis. Biomass Bioenergy 74, 26–46. doi: 10.1016/j.biombioe.2015.01.006
Heijungs, R., Settanni, E., and Guinée, J. (2013). Towards a computational structure for life cycle sustainability analysis: unifying LCA and LCC. Int. J. Life Cycle Assess. 18, 1722–1733. doi: 10.1007/s11367-012-0461-4
Hepburn, C., Adlen, E., Beddington, J., Carter, E. A., Fuss, S., Mac Dowell, N., et al. (2019). The technological and economic prospects for CO2 utilization and removal. Nature 575, 87–97. doi: 10.1038/s41586-019-1681-6
Hofstetter, D., Battke, B., Cox, B., and Hughes, J. (2014). Power-to-Gas in Switzerland - demand, regulation, economics, technical potential. Zurich: Swiss Federal Institute of Technology.
Hoppe, W., Bringezu, S., and Wachter, N. (2018). Economic assessment of CO2-based methane, methanol and polyoxymethylene production. J. CO2 Util. 27, 170–178. doi: 10.1016/j.jcou.2018.06.019
Iaquaniello, G., Setini, S., Salladini, A., and De Falco, M. (2018). CO2 vaporization through direct methanation of flue gas and renewable hydrogen: a technical and economic assessment. Int. J. Hydrogen Energy 43, 17069–17081. doi: 10.1016/j.ijhydene.2018.07.099
International Energy Agency (IEA) (2020). Outlook for biogas and biomethane: prospects for organic growth. Paris: IEA Publishers. Available at: https://webstore.iea.org/download/direct/2970?fileName=Outlook_for_biogas_and_biomethane.pdf (Accessed May 27, 2020).
Jens, C. M., Mueller, L., Leonhard, K., and Bardow, A. (2019). To integrate or not to integrate-techno-economic and life cycle assessment of CO2 capture and conversion to methyl formate using methanol, ACS Sustain. Chem. Eng. 7, 12270–12280
Khunathorncharoenwong, N., Charoensuppanimit, P., Assabumrungrat, S., and Kim-Lohsoontorn, P. (2020). Techno-economic analysis of alternative processes for alcohol-assisted methanol synthesis from carbon dioxide and hydrogen, Int. J. Hydrogen Energy in press. doi: 10.1016/j.ijhydene.2020.01.230
Kim, J., Henao, C. A., Johnson, T. A., Dedrick, D. E., Miller, J. E., Stechel, E. B., et al. (2011). Methanol production from CO2 using solar-thermal energy: process development and techno-economic analysis. Energy Environ. Sci. 4, 3122e3132. doi: 10.1039/c1ee01311d
Kourkoumpas, D. S., Papadimou, E., Atsonios, K., Karellas, S., Grammelis, P., and Kakaras, E. (2016). Implementation of the power to methanol concept by using CO2 from lignite power plants: techno-economic investigation. Int. J. Hydrogen Energy 41, 16674–16687. doi: 10.1016/j.ijhydene.2016.07.100
Koytsoumpa, E. I., Bergins, C., and Kakaras, E. (2018). The CO2 economy: review of CO2 capture and reuse technologies. J. Supercrit. Fluids 132, 3–16. doi: 10.1016/j.supflu.2017.07.029
Lanzafame, P., Abate, S., Ampelli, C., Genovese, C., Passalacqua, R., Centi, G., et al. (2017a). Beyond solar fuels: renewable energy‐driven chemistry. Chem. Sus. Chem. 10, 4409–4419.
Lanzafame, P., Perathoner, S., Centi, G., Gross, S., and Hensen, E. J. M. (2017b). Grand challenges for catalysis in the Science and Technology Roadmap on Catalysis for Europe: moving ahead for a sustainable future. Catal. Sci. Technol. 7, 5182–5194. doi: 10.1039/c7cy01067b
Laumb, J. D., Kay, J. P., Holmes, M. J., Cowan, R. M., Azenkeng, A., Heebink, L. V., et al. (2013). Economic and market analysis of CO2 utilization technologies: focus on CO2 derived from north Dakota lignite. Energy Proc. 37, 6987–6998. doi: 10.1016/j.egypro.2013.06.632
Li, Y., Kalnay, E., Motesharrei, S., Rivas, J., Kucharski, F., Kirk-Davidoff, D., et al. (2018). Climate model shows large-scale wind and solar farms in the Sahara increase rain and vegetation. Science 361, 1019–1022. doi: 10.1126/science.aar5629
Meunier, N., Chauvy, R., Mouhoubi, S., Thomas, D., and De Weireld, G. (2020). Alternative production of methanol from industrial CO2. Renew. Energy 146, 1192–1203. doi: 10.1016/j.renene.2019.07.010
Michailos, S., Sanderson, P., Villa Zaragoza, A., McCord, S., Armstrong, K., Styring, P., et al. (2018). Methanol worked examples for the TEA and LCA guidelines for CO2 utilization. Available at: https://deepblue.lib.umich.edu/handle/2027.42/145723 (Accessed July 10, 2020).
Moellenbruck, F., Kempken, T., Dierks, M., Oeljeklaus, G., and Goerner, K. (2018). Cogeneration of power and methanol based on a conventional power plant in Germany. J. Energy Storage 19, 393–401. doi: 10.1016/j.est.2018.08.018
Mustafa, A., Lougou, B. G., Shuai, Y., Wang, Z., and Tan, H. (2020). Current technology development for CO2 utilization into solar fuels and chemicals: a review. J. Energy Chem. 49, 96–123. doi: 10.1016/j.jechem.2020.01.023
Naims, H. (2016). Economics of carbon dioxide capture and utilization-a supply and demand perspective. Environ. Sci. Pollut. Res. 23, 22226–22241. doi: 10.1007/s11356-016-6810-2
Nami, H., Ranjbar, F., and Yari, M. (2019). Methanol synthesis from renewable H2 and captured CO2 from S-Graz cycle: energy, exergy, exergoeconomic and exergoenvironmental (4E) analysis. Int. J. Hydrogen Energy 44, 26128–26147. doi: 10.1016/j.ijhydene.2019.08.079
Navarrete, A., Centi, G., Bogaerts, A., Martín, Á., York, A., and Stefanidis, G. D. (2017). Harvesting renewable energy for carbon dioxide catalysis. Energy Technol. 5, 796–811. doi: 10.1002/ente.201600609
Nguyen, T. B. H., and Zondervan, E. (2019). Methanol production from captured CO2 using hydrogenation and reforming technologies environmental and economic evaluation. J. CO2 Util. 34, 1–11. doi: 10.1016/j.jcou.2019.05.033
Nieminen, H., Laari, A., and Koiranen, T. (2019). CO2 hydrogenation to methanol by a liquid-phase process with alcoholic solvents: a techno-economic analysis. Processes 7, 405. doi: 10.3390/pr7070405
Ordomsky, V. V., Dros, A.-B., Schwiedernoch, R., and Khodakov, A. Y. (2017). “Challenges and role of catalysis in CO2 conversion to chemicals and fuels,” in Nanotechnology in catalysis. Editors Sels, B., Van de Voorde, M. (Hoboken, NJ: Wiley) Vol. 3, 803–850.
Pérez-Fortes, M., Schöneberger, J. C., Boulamanti, A., and Tzimas, E. (2016). Methanol synthesis using captured CO2 as raw material: techno-economic and environmental assessment. Appl. Energy 161, 718–732. doi: 10.1016/j.apenergy.2015.07.067
Palo, E., Iaquaniello, G., and Mosca, L. (2019). “Calculate the production costs of your own process,” in Catalysis, green chemistry and sustainable energy. Editors Basile, A., Centi, G., De Falco, M., Iaquaniello, G., Studies in surface science and catalysis (Amsterdam, Netherlands: Elsevier B.V.), Vol. 179, 141–157.
Parra, D., Zhang, X., Bauer, C., and Patel, M. K. (2017). An integrated techno-economic and life cycle environmental assessment of power-to-gas systems. Appl. Energy 193, 440–454.doi: 10.1016/j.apenergy.2017.02.063
Perathoner, S., and Centi, G. (2014). CO2 Recycling: a key strategy to introduce green energy in the chemical production chain. Chem. Sus. Chem. 7, 1274–1282. doi: 10.1002/cssc.201300926
Perathoner, S., and Centi, G. (2019). Catalysis for solar-driven chemistry: the role of electrocatalysis. Catal. Today 330, 157–170. doi: 10.1016/j.cattod.2018.03.005
Quadrelli, E. A., Centi, G., Duplan, J. L., and Perathoner, S. (2011). Carbon dioxide recycling: emerging large-scale technologies with industrial potential. Chem. Sus. Chem 4, 1194–1215. doi: 10.1002/cssc.201100473
Räuchle, K., Plass, L., Wernicke, H. J., and Bertau, M. (2016). Methanol for renewable energy storage and utilization. Energy techn. 4, 193–200. doi: 10.1002/ente.201500322
Rego de Vasconcelos, B., and Lavoie, J. M. (2019). Recent advances in power-to-X technology for the production of fuels and chemicals. Front. Chem 7, 392. doi: 10.3389/fchem.2019.00392
Rivarolo, M., Bellotti, D., Magistri, L., and Massardo, A. F. (2017). Feasibility study of methanol production from different renewable sources and thermo-economic analysis. J. CO2 Util. 21, 132–138.
Rivera-Tinoco, R., Farran, M., Bouallou, C., Auprêtre, F., Valentin, S., Millet, P., et al. (2016). Investigation of power-to-methanol processes coupling electrolytic hydrogen production and catalytic CO2 reduction. Int. J. Hydrogen Energy 41, 4546–4559. doi: 10.1016/j.ijhydene.2016.01.059
Roh, K., Nguyen, T. B. H., Suriyapraphadilok, U., Lee, J. H., and Gani, R. (2015). “Development of sustainable CO2 conversion processes for the methanol production,” in 12th international symposium on process systems engineering. Editors Gernaey, V., Huusom, J.K., Gani, R. (Amsterdam: Elsevier Publishing), 1145–1150.
Schiebahn, S., Grube, T., Robinius, M., Tietze, V., Kumar, B., and Stolten, D. (2015). Power to gas: technological overview, systems analysis and economic assessment for a case study in Germany, Int. J. Hydrogen Energy 40, 4285–4294. doi: 10.1016/j.ijhydene.2015.01.123
Schlögl, R., Abanades, C., Aresta, M., Azapagic, A., Blekkan, E. A., Cantat, T., et al. (2018). “Novel carbon capture and utilisation technologies: research and climate aspects, SAPEA (Novel carbon capture and utilisation technologies: research and climate aspects),” in Evidence review report No. 2 (Berlin, Germany:SAPEA Publishers). ISBN 978-3-9819415-5-5
Senftle, T. P., and Carter, E. A. (2017). The holy grail: chemistry enabling an economically viable CO2 capture, utilization, and storage strategy. Acc. Chem. Res. 50, 472–475. doi: 10.1021/acs.accounts.6b00479
Son, M., Park, M.-J., Kwak, G., Park, H. G., and Jun, K. W. (2018). Maximum production of methanol in a pilot-scale process, Kor. J. Chem. Eng., 35, 355–363. doi: 10.1007/s11814-017-0295-7
Thonemann, N. (2020). Environmental impacts of CO2-based chemical production: a systematic literature review and meta-analysis. Appl. Energy 263, 114599. doi: 10.1016/j.apenergy.2020.114599
Tremel, A., Wasserscheid, P., Baldauf, M., and Hammer, T. (2015). Techno-economic analysis for the synthesis of liquid and gaseous fuels based on hydrogen production via electrolysis. Int. J. Hydrogen Energy 40, 11457–11464. doi: 10.1016/j.ijhydene.2015.01.097
von der Assen, N., Jung, J., and Bardow, A. (2013). Life-cycle assessment of carbon dioxide capture and utilization: avoiding the pitfalls. Energy Environ. Sci. 6, 2721–2734. doi: 10.1039/c3ee41151f
von der Assen, N., Voll, P., Peters, M., and Bardow, A. (2014). Life cycle assessment of CO2capture and utilization: a tutorial review. Chem. Soc. Rev. 43, 7982–7994. doi: 10.1039/c3cs60373c
Zauner, A., Böhm, H., Rosenfeld, D. C., and Tichler, R. (2019). Analysis on future technology options and on techno-economic optimization (D7.7 of STORE&GO EU project no. 691797). Available at: https://www.storeandgo.info/fileadmin/downloads/deliverables_2019/20190801-STOREandGO-D7.7-EIL-Analysis_on_future_technology_options_and_on_techno-economic_optimization.pdf (Accessed May 26, 2020).
Zhang, C., Gao, R., Jun, K. W., Kim, S. K., Hwang, S. M., Park, H. G., et al. (2019). Direct conversion of carbon dioxide to liquid fuels and synthetic natural gas using renewable power: techno-economic analysis. J. CO2 Util. 34, 293–302. doi: 10.1016/j.jcou.2019.07.005
Zhang, H., and Desideri, U. (2020). Techno-economic optimization of power-to-methanol with co-electrolysis of CO2 and H2O in solid-oxide electrolyzers. Energy, 199, 117498. doi: 10.1016/j.energy.2020.117498
Zhang, H., Wang, L., Van herle, J., Maréchal, F., and Desideri, U. (2019). Techno-economic optimization of CO2-to-methanol with solid-oxide electrolyzer. Energies 12, 3742. doi: 10.3390/en12193742
Zhang, S., Zhuang, Y., Liu, L., Zhang, L., and Du, J. (2020). Optimization-based approach for CO2 utilization in carbon capture, utilization and storage supply chain. Comput. Chem. Eng. 139, 106885. doi: 10.1016/j.compchemeng.2020.106885
Zheng, Y., Zhang, W., Li, Y., Chen, J., Yu, B., Wang, J., et al. (2017). Energy related CO2 conversion and utilization: advanced materials/nanomaterials, reaction mechanisms and technologies. Nanomater. Energy 40, 512–539. doi: 10.1016/j.nanoen.2017.08.049
Keywords: CO2 economics, methanol, power-to-X, techno-economic analysis, CO2 impact, CO2 utilization
Citation: Centi G, Perathoner S, Salladini A and Iaquaniello G (2020) Economics of CO2 Utilization: A Critical Analysis. Front. Energy Res. 8:567986. doi: 10.3389/fenrg.2020.567986
Received: 31 May 2020; Accepted: 26 August 2020;
Published: 22 September 2020.
Edited by:
Angela Dibenedetto, University of Bari Aldo Moro, ItalyReviewed by:
Ludo Diels, Flemish Institute for Technological Research (VITO), BelgiumHasmukh A. Patel, Aramco Services Company, United States
Copyright © 2020 Centi, Perathoner, Salladini and Iaquaniello. This is an open-access article distributed under the terms of the Creative Commons Attribution License (CC BY). The use, distribution or reproduction in other forums is permitted, provided the original author(s) and the copyright owner(s) are credited and that the original publication in this journal is cited, in accordance with accepted academic practice. No use, distribution or reproduction is permitted which does not comply with these terms.
*Correspondence: Gabriele Centi, centi@unime.it