- 1Carbon Upcycling Technologies, Calgary, AB, Canada
- 2Department of Chemistry, University of Calgary, Calgary, AB, Canada
Carbon dioxide removal (CDR) is an essential feature of climate mitigation. With current techniques falling short in terms of performance, cost, and environmental integrity, novel CO2 utilization has the potential to create a multi-billion dollar commodity market that is capable of sequestering large amounts of CO2 from the atmosphere. To achieve this, significant development in terms of government funding, policy change, and research is needed. This review highlights the current state of novel CO2 utilization technologies. The paper evaluates their future prospects by drawing parallels with two successfully developed hardware-heavy technologies of the last 50 years, namely the solar and the automobile industries. Both technologies have had radically different commercialization pathways, and offer important insights on facilitating and accelerating the development of novel CO2 utilization technologies to meet critically relevant climate targets.
Introduction
Scientific and historical evidence considered by the Intergovernmental Panel on Climate Change (IPCC) (IPCC, 2014) and the National Aeronautics and Space Administration (NASA) (NASA, 2008a) has illustrated a perpetual increase in atmospheric carbon dioxide (CO2) levels from the pre-industrial times to the present day. With high confidence, this anomalous occurrence has been related to anthropogenic activities (IPCC, 2014; Prentice et al., 2019). The result has been an increase in global temperatures by 0.8°C since the inception of the industrial revolution (IPCC, 2014; NASA, 2008b). Continual global warming is expected to change rainfall patterns and cause extreme weather events (Wheeler and Von Braun, 2013), thereby adversely impacting the distribution of crop yields (Wheeler and Von Braun, 2013), fish populations (Sharma et al., 2011), and freshwater resources (Arnelf and Reynard, 1996).
The Paris Agreement (Paris Agreement, 2015) was signed by the Conference of Parties in 2015 to prevent further climate effects by designing and implementing strategies to limit global temperature rise to less than 1.5°C by the end of this century. This objective implies reaching net zero CO2 emissions on the global scale by the year 2050 (Rogelji et al., 2018). Achieving this target would entail significant reductions in global CO2 emissions associated with the energy and agricultural sectors, as well as major industrial segments such as cement and steel production (Rogelji et al., 2018). The decarbonization of electricity supply at an industrial level and the use of electric appliances at the consumer level are also required in reducing CO2 emissions (Rogelji et al., 2018). Another element imperative to achieving net zero CO2 emissions is the institution of Carbon Dioxide Removal (CDR) techniques (Rogelji et al., 2018).
CDR is an umbrella term used to describe a range of techniques that serve to remove/sequester CO2 from the atmosphere in order to maintain or reduce its levels and ultimately prevent or reverse the impact of CO2 associated with climate change (National Research Council, 2015; Mach et al., 2014; Masson-Delmotte et al., 2018). Such techniques fall along a spectrum of approaches that remove CO2 by i) enhancing carbon utilization sinks already present in nature such as reforestation or afforestation; ii) creating advanced natural carbon utilization sinks in the soil (e.g., sequestration and biochar) and the ocean (e.g., enhanced weathering and marine algae farming (Chung et al., 2011)); and iii) engineering technologies such as Carbon Capture and Storage (CCS) or Direct Air Capture (DAC) that serve to remove and artificially store away atmospheric CO2 in geological reservoirs (National Research Council, 2015; Mach et al., 2014) (Table 1). Additionally, techniques such as Biomass Energy with Carbon Capture and Storage (BECCS) remove CO2 through the creation of biomass which can then be utilized to generate carbon-based forms of energy such as biofuels (National Research Council, 2015; Mach et al., 2014; Consoli, 2019).
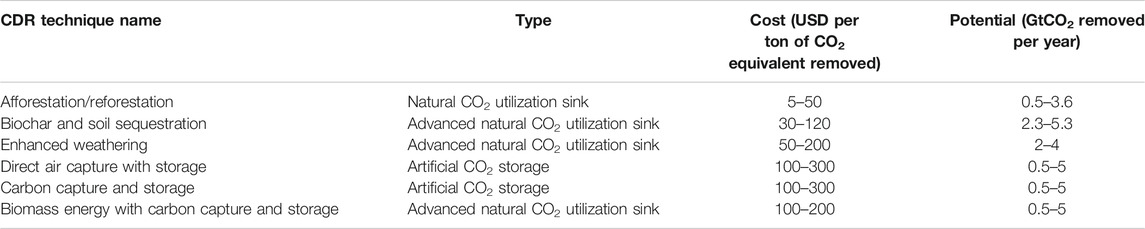
TABLE 1. An abridged list of CDR techniques analyzed by the IPCC for their CO2 removal potential in gigatons and the associated costs on an annual basis. Several studies providing a range of values for cost and potential for each technique were considered, however values reported by Fuss et al. were deemed the most realistic estimations by the IPCC.
CDR techniques have been an essential part of the climate mitigation-focused Integrated Assessment Models (IAMs) designed by the IPCC, which show a range of possible mitigation pathways that limit global warming to 1.5°C (Masson-Delmotte et al., 2018). More stringent pathways assume a significant reduction in energy demand and approximate a complete switch to renewable energy, whilst less stringent pathways are decidedly dependent on CDR techniques. Importantly, the realistic pathways are characterized by reduced stringency on energy and agricultural demand curtailment alongside increased adoption of CDR techniques (Masson-Delmotte et al., 2018). As the scenarios decrease in stringency, the cumulative requirement for CO2 removal increases from 0 Gt to 1,218 Gt by 2100 (Masson-Delmotte et al., 2018).
Widespread adoption and further development of CDR technologies as an inevitable part of the prospective global pathway of reducing CO2 levels is persuasive, as the International Energy Agency’s (IEA) business-as-usual scenario projects energy demand rising by an average of 35% by 2040 (IEA, 2018), whilst requirement for agricultural goods is estimated to rise by 50% in the year 2030 (Wheeler and Von Braun, 2013). As population growth and a consumption-focused lifestyle increases (Rogelji et al., 2018), CDR techniques are being recognized as more prominent climate change mitigation solutions. At the forefront are technologies such as CCS, DAC, and BECCS due to their potential for scalability and long term CO2 storage (Page et al., 2019).
However, several challenges ranging from high capital and operating costs; low practical carbon reduction/sequestration capacities; ecological side effects; performance uncertainties; low Technology Readiness Levels (TRL, is a measurement of the maturity of a technology, the levels range from 1 to 9 and technology maturity increases with the level numbers (Beck, 2013)); a lack of globally enforced carbon regulation, as well as a lack of feasible scale of deployment, have plagued the development of CDR technologies (National Research Council, 2015; Ciais et al., 2013; Field and Mach, 2017; de Coninck and Revi, 2018). For example, afforestation/reforestation and fertilization of land plants have limited carbon sequestration capacities and short timescales of CO2 storage that range from decades to centuries (Read and Royal, 2001; Ciais et al., 2013; Field and Mach, 2017). Whilst CCS and DAC offer long timescales of CO2 storage ranging in millennia, they are expensive techniques causing limitations to large scale deployment (Ciais et al., 2013; de Coninck and Revi, 2018). Enhanced weathering can cause permanent pH changes (Ciais et al., 2013) to the ocean and soil, whilst BECCS requires extensive land use that can negatively affect biodiversity (Allen et al., 2018).
Hence, a new family of CDR technologies that utilize atmospheric CO2 in a feedstock mix for producing value-added, industry-sought commodities are gaining traction as a cost-effective and economically driven CDR pathway (Sandalow et al., 2017; Norhasyima and Mahlia, 2018; Hepburn et al., 2019; Zhu, 2019). In recent literature, these technologies have been defined under the broad spectrum of CO2 utilization, which also includes techniques such as forestation, biochar production and Enhanced Oil Recovery (National Research Council, 2015; Norhasyima and Mahlia, 2018; Hepburn et al., 2019; Zhang et al., 2020; Baena-Moreno et al., 2019). We propose the term “novel CO2 utilization technologies” to classify this family of CDR technologies and define it as the utilization of atmospheric CO2 in a feedstock mix for producing commodities such as materials and chemicals for commercial and industrial use. By fabricating value added, industry-sought commodities from a feedstock of CO2 emissions, large scale deployment that can have the potential of causing a dent in the CO2 emissions is made feasible.
In recent years, several institutions and programs such as the US Department of Energy; the NRG COSIA Carbon X-PRIZE; Natural Resources Canada; and Emissions Reduction Alberta have expressed interest in the development of new technologies that utilize CO2 emissions through the allocation of funding and grants. Independent companies and start-ups that incorporate captured CO2 emissions into their feedstock for polymers and concrete, such as Covestro and Carbon Upcycling, are also emerging. The importance of such technologies in the context of climate mitigation is being increasingly recognized, however their full-scale impact is still under investigation.
In this paper we move beyond the range of popular CO2 utilization techniques found in contemporary literature such as forestation, biochar, and BECCS to discuss novel CO2 utilization in the context of the CO2 industrial commodity market. The purpose is to provide a discussion for facilitating rapidly scalable, scale-relevant, and market-driven pathways for the CO2 commodity market.
This is done by studying the conditions required and the associated challenges for the development and scaling of novel CO2 utilization technologies for the purpose of determining their prospects in the context of climate mitigation. Through an overview of the examples of successful commercialization and global scale achieved by the solar and automobile industry, we present a set of guidelines that will aid the growth and wide-spread implementation of novel CO2 utilization technologies. The intent is to elucidate contrasting evolutionary pathways of two other hardware-intensive industries from the industrial age as a way to provide a perspective to policy makers, innovators, corporations, and other entities in the ecosystem seeking to devise or contribute to a pathway to develop, scale, and commercially deploy novel CO2 utilization technologies today.
Carbon Dioxide Removal Techniques in Contemporary Literature
As previously mentioned, CDR techniques ranging from the simple forestation methods to the more progressive carbon capture technologies have been developed and studied in the literature for their cost and capacity to mitigate climate change (Mach et al., 2014; de Coninck and Revi, 2018). Their associated ecological side effects and performance abilities have also been studied in depth (Russell et al., 2012; Keller et al., 2018; McCormack et al., 2016). Table 1 summarizes the costs and CO2 removal capacities for a range of CDR techniques found by Fuss et al. (2018) and published by the IPCC.
In order to achieve net zero CO2 emissions by 2050 and consequently maintain global warming at 1.5°C, CDR techniques need to remove 37–40 GtCO2 per year starting in 2020; with rates only increasing annually from there onwards (Bui et al., 2018; Haszeldine et al., 2018; Masson-Delmotte et al., 2018). Evaluating the global estimated land available for afforestation/reforestation, a 0.5–3.6 GtCO2 per year removal capacity could be expected, which is insufficient to offset the required CO2 emissions in time for 2050. Additionally, a large water footprint is predicted for afforestation/reforestation techniques, with CO2 eventually being released back into the atmosphere after a few decades to centuries (de Coninck and Revi, 2018). In the discussion for CO2 sequestration through soil, the production of biochar is well documented. Biochar is a stable alternate form of biomass that has a slower rate of decay and can be added to soils to enhance their carbon sequestration capacity (National Research Council, 2015; de Coninck and Revi, 2018). Although soil sequestration is inexpensive, biochar production comes with heavy costs and potential impacts on the carbon cycle (Ciais et al., 2013).
Traditional techniques that involve reformed land practices for increased CO2 utilization through fertilization of land plants are important in decarbonizing the agriculture sector. However, these methods along with micro/macro algae farming are not a permanent source of CO2 storage as decomposition will eventually lead to CO2 returning back into the atmosphere (Read and Royal, 2001; de Coninck and Revi, 2018). Another technique that utilizes CO2 is the enhanced weathering process of rocks in the oceans that converts atmospheric CO2 to solid carbonates. Given optimum rock type, weathering rate and available area, this technique exhibits a high potential for CO2 storage (Strefler et al., 2018). However, enhanced weathering of rocks can cause several unintended ecological side effects such as the aforementioned changes in pH and the release of heavy metals into the environment (de Coninck and Revi, 2018; Strefler et al., 2018).
Advanced technologies that extract anthropogenic CO2 have been widely studied and explored for their role in the climate mitigation discourse. The CCS system removes CO2 emissions from point sources, such as combustion power plants and industrial facilities (Metz et al., 2005). Once the CO2 is separated from the gaseous matrices, it is compressed to high densities for ease of transportation and subsequent geological or ocean storage (Metz et al., 2005). The DAC technology focuses on removing CO2 gas directly from the ambient atmosphere (National Research Council, 2015; de Coninck and Revi, 2018; Haszeldine et al., 2018). Large scale deployment of both techniques is limited by the availability of safe CO2 storage as well as high installation and running cost (de Coninck and Revi, 2018). Studies conducted to evaluate the costs and CO2 removal/storage capacities of CCS and DAC show large variations in their findings, depending on the constraints assumed by the estimation models (Bui et al., 2018). The IPCC has analyzed several such studies and has settled with Fuss and colleagues’ work (Fuss et al., 2018), which estimates CO2 removal/storage capacity of 0.5–5 GtCO2 per year and a cost ranging from 100 to 300 USD per ton of CO2-equivalent removed (de Coninck and Revi, 2018). DAC ranks higher than CCS in terms of cost, due to the higher energy costs incurred through CO2 removal from the dilute atmosphere as compared to a concentrated flue gas source (de Coninck and Revi, 2018).
With regards to BECCS, estimated potentials from the literature range from 0–50 GtCO2 per year (de Coninck and Revi, 2018). Such a range suggests that BECCS can either provide no contribution in removing CO2 from the atmosphere or single-handedly mitigate it to acceptable levels by 2050. With carbon budgets rapidly on the decline, the latter scenario is plausible only with deep decarbonization (Gough et al., 2018). Fuss et al. has again incorporated restraints associated with the technique, such as the availability of safe CO2 storage, to calculate a more practical estimated potential of 0.5–5 GtCO2 per year (de Coninck and Revi, 2018; Haszeldine et al., 2018). Such a capacity range for BECCS merits further consideration in any comprehensive climate change mitigation policy framework. Additionally, high installation costs are characteristic of BECCS, which are estimated to be several hundred billion USD per year by 2050 at a rate of 3.3 GtCO2 equivalent per year (Smith et al., 2016; de Coninck and Revi, 2018). Concerns regarding sustainability are not far-fetched as a similar carbon reduction potential can be reached by forestation techniques which are much less costly, albeit coming with their own environmental impact, vide supra.
Since the spectrum of CCS technologies is susceptible to cost-effectiveness in consequence to technological advances (Metz et al., 2005), significant investments by global entities have been made in the field of CCS as a means of meeting CO2 mitigation targets. In their recent 2018 report, the European Union assessed the status of CCS technologies that had received nearly 1 billion euros of public tax funding in 2008 for commercial large scale deployment (Special Report, 2018). The report found the endeavor to be unsuccessful in commercializing CCS technologies due to low carbon prices set by regulations, wavering political interests of the EU states and the associated financial insecurities. Such a conclusion is not improbable as the opportunity to cultivate revenue with the geological storage of captured CO2 is scarce, especially with CCS deployment being concurrent with increased energy consumption at point sources (Siirola, 2014). Whilst the levelized costs for coal and natural gas power plants have increased in the past decade due to market factors (Rubin et al., 2015), the deployment of CCS technologies has raised these costs by an additional 45–70% in the power generation sector (Irlam, 2017). It is, however, important to recognize that the long-term (end-of-century) cost savings associated with the deployment of CCS technologies in the context of CO2 mitigation is in the US$ billion–US$ trillion range (Metz et al., 2005). As a result of the significant cost savings and the permanency of CO2 storage achieved through the deployment of CCS, it has been established as a primary CDR technique (Page et al., 2019). Furthermore, it is widely understood that integration with some form of utilization is favorable for improving CCS economics (Zhu, 2019; Sandalow et al., 2017; Jones et al., 2017; Center for Climate and Energy Solutions, 2020).
Commercializing CO2 removal through novel utilization can catalyze large scale implementation by creating a return on investment for financial input. An integrated process flow combining CO2 removal and novel utilization in lieu of geological storage can improve CDR sustainability in terms of land use, cost, and environmental impact; whilst providing an economic value proposition to justify accelerated deployment. Novel CO2 utilization has the potential to be market-viable and can be driven by free market factors, offering it an important advantage over conventional CCS methods in terms of potential traction and rate of adoption and growth. Accelerating CDR growth in the form of novel CO2 utilization is particularly necessary as the collection of current CDR techniques with their associated advances are operating on only a few MtCO2 per year removal scale (Bui et al., 2018; Malischek, 2020).
Current Status of Novel CO2 Utilization Challenges
Since the 1920s, CO2 has been used for a variety of industrial applications such as the carbonation of beverages, preservation of food items, and the enhanced recovery of oil (Kaliyan et al., 2007). Commercial grade CO2 has generally been obtained as a by-product from other industrial processes such as the manufacture of ammonia gas and hydrogen fuel, as well as the burning of coke and coal (Kaliyan et al., 2007). As anthropogenic CO2 levels continue to rise, the limited applications of CO2 that is retrieved from industrial waste are currently insufficient as a significant component in the CO2 utilization discourse. This further necessitates innovation in applications that employ CO2, such as novel CO2 utilization.
In the discussion regarding the utilization of captured CO2, common applications that have been investigated since the 1970s are limited to pathways associated with enhanced oil/methane recovery and urea production (Global CCS Institute, 2011). However, since the late 2000s, institutions such as the Center for Climate and Energy Solutions (C2ES) (Bobeck et al., 2019), the Innovation for Cool Earth Forum (ICEF) (Sandalow et al., 2017), the U.S Department of Energy (U.S. Department of Energy, 2016) the Global CO2 Initiative (CO2 Sciences Inc., 2016) and the Global CCS Institute (2011) have published comprehensive reports on the practical pathways that can be undertaken for producing high quality products through novel CO2 utilization. Table 2 provides a non-exhaustive summary of novel CO2 utilization products with their respective phases of development and potentials for CO2 removal (Gt).

TABLE 2. An abridged list of Novel CO2 Utilization products with their associated status and potentials for CO2 removal in gigatons. This list has been compiled from reports published by the Center for Climate and Energy Solutions (Bobeck et al., 2019), the Innovation for Cool Earth Forum (Sandalow et al., 2017), the U.S Department of Energy (U.S. Department of Energy, 2016) the Global CO2 Initiative (Global CO2 Initiative, CO2 Sciences Inc, 2016) and the Global Carbon Capture and Storage Institute (Global CCS Institute, 2011).
Construction materials such as aggregates and concrete are at the forefront of novel CO2 utilization with several commercial projects already underway (Zhu, 2019). Manufacturers that have been able to successfully design high quality products that incorporate a percentage of CO2 gas are currently few, and those that have actively commercialized such products at a high Technology Readiness Level are further scarce. Due to the novelty of these technologies, knowledge about costs and CDR capacities are institution- and process-specific. As such, the collective scale of operation in terms of CO2 capture and novel utilization is not yet known. In addition to the obscurity of CO2 conversion pathways for creating value-added products, this lack of knowledge has prevented bodies like the IPCC from incorporating novel CO2 utilization in their IAMs for climate mitigation (de Coninck and Revi, 2018). Whilst the demand of the current combination of novel CO2 utilization technologies is enough to offset ∼10% of anthropogenic CO2 emissions (Zhang et al., 2020), several research avenues are being developed to further identify and create industrially relevant products that can replace conventional materials (Peters et al., 2011).
To date, novel CO2 utilization technologies face a duality of challenges: firstly, with producing a net reduction of CO2 emissions throughout their lifecycle; and secondly, providing good quality carbon commodities at an economically viable price point. To ensure that the process of novel CO2 utilization remains carbon negative, it is pertinent to consider the amount of CO2 removed from the atmosphere, the source of energy being utilized, and the CO2 emissions incurred in the conversion and incorporation processes (de Coninck and Revi, 2018; Zimmermann et al., 2018; Müller et al., 2020). As is the case with all manufacturing processes, establishing cost-competitiveness for novel CO2 utilization products in the first few years of operation can, and is proving to be challenging, particularly if cheaper alternatives already exist. For example, in an article by Bloomberg magazine, industry leaders have stated that customers are reluctant to purchase environmentally-friendly types of cement with low carbon footprint, as traditional cement offers a better price (Dezem, 2019). Additionally, software technologies provide a safer domain of investment, where the possibility of sunk costs are low, which further makes financiers reluctant to invest in comparatively high-risk hardware technologies. Should intermittent changes become necessary, as is often the case with innovation, redesigning codes in software can be a lot simpler than rebuilding hardware. Although novel CO2 utilization technologies still require substantial capital for the installment of infrastructure relevant to CO2 capture and associated conversion facilities, investment in this field is highly likely as governmental bodies are fiercely trying to meet their share of goals outlined by the Paris Agreement. Additionally, the US Department of Energy has compiled several reports discussing the financial feasibility and chemical processes of CCS with a keen interest in utilization as well (U.S. Department of Energy, 2016; Mission Innovation, 2017). In September 2019, the Department announced an allocation of USD 110 million for the development and research of CCS technologies (US Department of Energy, 2019). The utilization of CO2 is also included in this fund, which is currently limited to enhanced oil recovery (EOR).
Literature that has focused on assessing the viability of using captured CO2 for chemical, plastic, or material production has found low TRL levels (Kätelhön et al., 2019) and low carbon prices (Smit et al., 2014) to be sources of hesitation in the employment of novel CO2 utilization. In order for CO2 to become a viable feedstock for chemical synthesis, carbon prices need to exceed the price of oil, which is the current major raw material for chemicals (Smit et al., 2014).
In addition to technical challenges, a lack of understanding and awareness regarding the concept of employing CO2 emissions for a commodity market instills a fear of uncertainty and a greater reliance on fossil fuels, which perturbs public investments and political support (Bui et al., 2018). However, novel CO2 utilization has the potential of becoming a multibillion dollar industry, in addition to reducing climate mitigation costs by 138% (Bui et al., 2018; U.S. Department of Energy, 2016). Cost savings are achieved by reduced expenditure on adaptation costs such as maintaining air quality and fresh water availability, as well as reduced economic loss due to damage to natural resources (IPCC, 2014). It is important to note that short-term (2030–2050) and long-term (2050–2100) mitigation costs are expected to increase by 44 and 37%, respectively, if mitigation measures employing CO2 removal such as novel CO2 utilization are delayed until 2030 (IPCC, 2014). Moreover, current energy intensive industrial processes that cannot be replaced with greener alternatives can be decarbonized via CO2 capture and novel utilization, which furthers the importance of developing such technologies (Bui et al., 2018; U.S. Department of Energy, 2016).
In the production of manufactured goods through novel CO2 utilization, several other unforeseen barriers to operations and upscaling may occur. For example, policy specifications by city or state bodies can cite limitations to the amount of novel CO2 materials that can be incorporated in chemicals, construction materials, and fuels (Carey, 2017; Sandalow et al., 2017). It is also conceivable that the scales of CO2 capture and novel CO2 utilization may not coincide, resulting in either a lack of supply to keep operations running or a large requirement of geological storage for a surplus of captured CO2. Companies that have successfully propelled their CO2 utilization technologies in the commercialization stages, such as Carbon8, have sustained considerable years of R&D before enjoying a high TRL level (Carey, 2017). Such companies still face further commercialization challenges such as high CO2 prices and strict standards preventing the incorporation of their materials (Carey, 2017). However, free market forces and directed policy incentives will enable these interfacial tensions to stabilize over time and reach market-driven, policy-nudged equilibriums similar to how the solar industry gained a foothold under Obama’s green building initiative in 2008–2010.
Potential Future Pathways
In order to assess the plausible pathways that the novel CO2 utilization market may take, it is important to extrapolate lessons from other hardware-heavy technology industries that have scaled successfully across the world, and to analyze their historical genesis, evolution, and development cycles. This section studies the development of two different successful technology industries: solar and automobile. These particular industries were chosen for analysis as their growth and deployment has occurred under vastly different circumstances and scales, leveraging different resources and trends to sustain accelerated growth. Moving forward, novel CO2 utilization at an industrial level may follow either, or a combination of these paths to commercialization and upscale.
The Solar Industry
The roots of the solar industry can be traced back to the discovery of the photovoltaic effect in 1839 (Harvey et al., 2017) which illustrated the production of an electrical current in a material upon its exposure to sunlight (Bube and Fahrenbruch, 1981). The following four decades were spent verifying and studying this discovery before a device capable of converting solar energy into electricity at a rate of 1% efficiency was invented in 1882 (Harvey et al., 2017; The History of Solar, 2019). The next 70 years saw periods of small improvements, dictated by a series of solar cell patents, experimentation with photoelectric materials, and academic studies (Harvey et al., 2017; The History of Solar, 2019). The industry took a leap when a fully functional solar cell was constructed by Bell Laboratories in 1954 which was capable of powering electrical equipment while operating at a conversion efficiency of 6% (Harvey et al., 2017; The History of Solar, 2019; Perlin, 2004). This device was well-funded and created in an attempt to find an alternate source of power for the company’s telephone system, as their conventional batteries were inefficient in tropical climates (Perlin, 2004). Interestingly, from concept to fabrication, the Bell solar cell entertained a mere 2 years of development, as compared to the preceding 70 years spotted with instances of advancements for solar cells (Perlin, 2004). In the case of novel CO2 utilization, growth has already begun to accelerate in a likewise manner, as the urgency of climate mitigation has pushed several institutions and investors, like the U.S Department of Energy, to seek a range of possible solutions.
With the invention of Bell Laboratories’ solar cell, licensing and further efforts to increase the efficiency of solar cells continued throughout the 50–60s (The History of Solar, 2019). Bell Laboratories was joined by the RCA Corporation and Hoffman Electric in simultaneous experimentation with different materials and electronic junctions to increase the solar-to-current conversion rate in increments until a 14% efficiency rate was achieved by 1960 (The History of Solar, 2019). This increase in efficiency was incentivized by inter-company competition and power supply requirements for space exploration shuttles and satellites during the latter decade when space programs were fueled by government support (The History of Solar, 2019; Perlin, 2004). Similarly, today in the novel CO2 utilization market, competition is increasing as numerous start-ups, especially under the Carbon X-PRIZE competition, are developing their pathways for utilization toward commercialization. It is important to note that the demand for CO2 utilization stems from a mix of private and government sectors, as opposed to the solely government-led projects in the case of solar cells.
In the 1970s, the solar industry was fueled by the United States’ government in an effort to relieve the energy crisis (Pinner and Rogers, 2015). This agenda prompted big corporations as well as governmental branches to provide regulatory support and investments for the development of solar energy. In 1972, Exxon Corporation funded research into the production of a solar cell that could reduce cost from 100 to 20 USD/Watt (The History of Solar, 2019). By the late 70s, the United States’ government dedicated entire facilities for the improvement of solar energy, which was followed by the deployment of several photovoltaic systems for public use (The History of Solar, 2019). In 1978, a 3.5 kW photovoltaic system was built to power a small village in Arizona and by 1982, a 1 MW scale power station was built in California (The History of Solar, 2019). In the same year, the US Department of Energy built a 10 MW scale solar demonstration facility with the help of industrial partners (The History of Solar, 2019). Global use of solar cells for electricity generation continued to increase on the MW scale and by 1993, a photovoltaic system which was grid-supported was built in California (The History of Solar, 2019). By the year 2000, photovoltaics had reached a capacity of 0.3 GW and were ready for larger scale deployment (Pinner and Rogers, 2015; Harvey et al., 2017).
The discovery of the photovoltaic effect was followed by two centuries worth of investigations and improvements before any scale of commercialization was reached. In order to establish the novel CO2 utilization industry as a significant force in the climate mitigation dialogue, it is necessary to avoid the hiatuses in terms of efficiency and capacity that the solar industry faced. The scale of development achieved in the two centuries must be condensed in mere decades for the CO2 commodity market to cause a sizable dent in emissions by 2050 (IPCC, 2014). The involvement of the US Department of Energy assisted in the demonstration and popularization of the solar industry, which parallels and shows promise for the development of the novel CO2 utilization market as well. Regulatory support by the United States government has administered improvements to the 45Q policy, which provides a tax credit for every metric ton of CO2 captured or utilized in enhanced oil recovery (Bobeck et al., 2019; US Department of Energy, 2019).
In addition to policy regulation, the United States government offered subsidies to manufacturers of solar cells and users alike, in order to create a demand for solar power. Homeowners were given $15,000–$20,000 to alleviate the one-time installation costs alongside feed-in-tariffs that provided a guaranteed price per kW of solar power for energy providers (Pinner and Rogers, 2015). Despite the collapse of the solar energy market in the early 2000s, state and government subsidies allowed for it to rise once again. By 2014, the market for solar energy was fostered enough to create solar photovoltaic panels with a capacity of 45 GW (Pinner and Rogers, 2015).
From the case of the solar industry, it can be determined that in order to increase the frequency of large-scale projects, escape market failures, and ensure that the CO2 commodity market stays competitive, investments, subsidies, and grants are needed to be instituted by governing bodies. Moreover, current policies that provide incentive for CO2 utilization such as the 45Q Tax Credit policy should lower the bar of eligibility from 25,000 mt (0.025 Mt) of CO2 utilized to enable small-scale projects (Smith et al., 2016). Additionally, business-consumer relationships need to be created for the CO2 commodity market to be widely accepted and for fears regarding fossil fuel reliance to be discredited.
Figure 1 shows the growth curves of the automobile and solar industries. Although developments in both solar and automobile industries escalated exponentially, their respective impacts on the energy and transportation sectors have been vastly different. Whilst the number of automobiles produced quickly superseded the production of horse-carriages and wagons (Figure 2) (Griffin, 1925; Steuart and Hawes, 1918), the contribution to power generation from solar energy has not yet reached the level of fossil fuel generated power (Figure 3) (Smil, 2019; Our World in Data, 2018; bp, 2019). Additionally, literature shows that large scale adoption and diffusion of new technologies not only require cost-competitiveness but an actual increase in profit (Hall, 2016). In the context of CDR technologies, this means that simply being environmentally friendly is not a realistic economically perceived criteria for large scale usage (Popp, 2010). The results of following this scenario are currently observed in the case of traditional CCS technologies that incur an additional cost for implementation without any real benefit to the industry, vide supra (Bui et al., 2018).
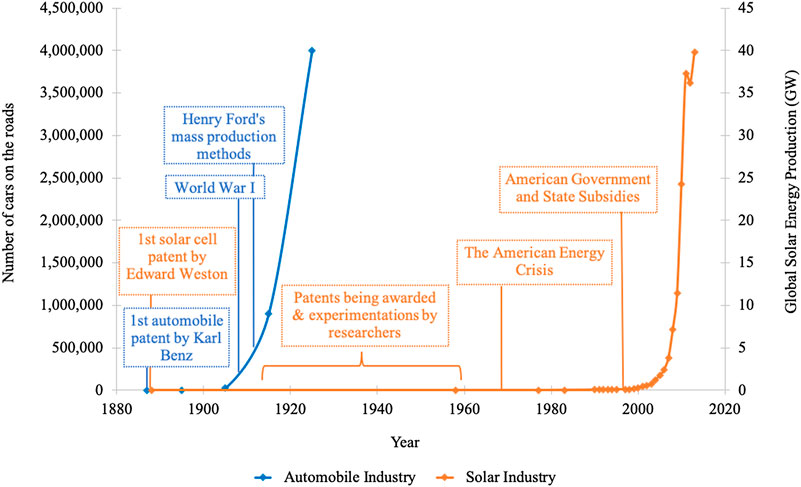
FIGURE 1. A comparison of the trajectories of the American solar and automobile industries with respect to output in terms of the number of cars present on roads for the automobile industry and the amount of energy produced (GW) for the solar industry.
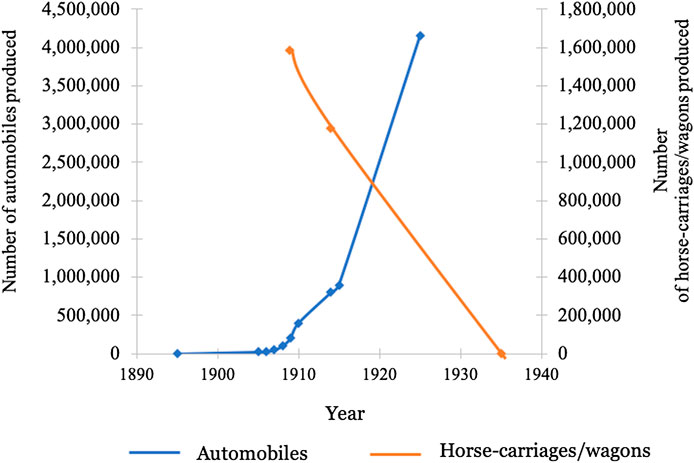
FIGURE 2. A comparison of the American production statistics of automobiles and horse-carriages/wagons. Values of automobile production obtained from (Griffin, 1925). Values of horse-carriages/wagon production units obtained from the US. Department of Commerce archives (Steuart and Hawes, 1918).
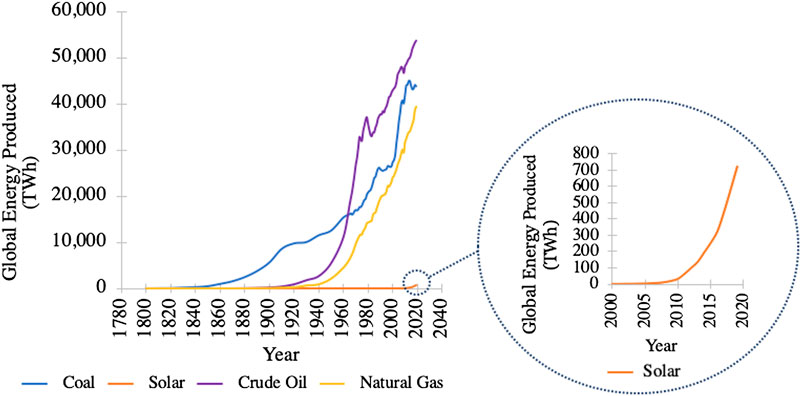
FIGURE 3. A comparison of different forms of global energy produced in terms of TWh from 1800 to 2019. Values are obtained as CVS files from ourworldindata.org (Our World in Data, 2018).
The Automobile Industry
Another industry with a successful commercialization history is the automobile industry. The globally ubiquitous automobile underwent several important developmental phases since its inception which are discussed herein. Given that automobiles are a standard commodity in most North American households, it may be easy to forget that this situation did not come to be overnight. It was not until the early 1900s that automobiles surpassed the use of horse-drawn vehicles, and several factors spanning over five decades from the late 1800s to the early 1900s were instrumental in facilitating this irreversible change (Cromer et al., 2018).
There are differing opinions as to when and by whom the first automobile was invented, as several attempts were made in the late 18th century to replace horse-drawn carriages. Notably, in the 1760s, after the advent of the steam engine, Frenchman Nicolas-Joseph Cugnot (Cromer et al., 2018) built a steam-powered artillery vehicle which could also be used for transportation purposes (autoTRADER.ca, 2019). The design features of this vehicle caused an uneven distribution of weight and deemed its usage impractical. However, the development of automobiles was not perturbed and in the following decades several more attempts were made to create practical, self-propelled, steam-powered vehicles.
An important leap for automobiles occurred in 1871 when Dr. John W. Carhart invented a steam-powered carriage able to feasibly tread on the already present wagon roads of Wisconsin (McBride, 1999). The resulting spark of interest was exemplified in state-wide competitions for building practical alternatives to animal-based vehicles. From there onwards, the most noteworthy milestone occurred in 1886: the patenting of The Benz Motorwagen by Karl Benz (Benz, 1886; Sovacool, 2009). The novelty of this automobile arose from the petroleum-based internal combustion engine used as the propellant of the vehicle (Cromer et al., 2018). From concept to fabrication, Benz dedicated two decades in order to counter the monetary challenges that had plagued the progress of his automobile (Seltzer, 1928). His project came to fruition when the automobile experiment was financed by his wife’s dowry and the investment of two private Mannheim investors, who were well aware of Benz’s talent and expertise (Dickmann et al., 2014). In comparison, a similar magnitude milestone from the solar industry, the Bell solar cell, took just 2 years to be constructed, as the project was funded by a well-established company.
Benz’s project was popularized once Bertha Benz demonstrated the practical use of this automobile for long-distance travel, which entered the realm of commercial production when Benz demonstrated it during the Paris Exhibition of 1887. Simultaneous to the invention of the Benz Motorwagen was the production of the gasoline-driven automobile by Gottlieb Daimler (Cromer et al., 2018). Due to the efficiency of Daimler’s engine, this vehicle won the first Paris-Rouen international automobile race in 1894, and secured a sizable price which was further used to develop the vehicle according to the increasing demand of higher horsepower (Seltzer, 1928). By 1895, 300 motor vehicles had been sold on the market (Griffin, 1925). These initial sales were made to wealthy folk to be exclusively used for recreational activities such as touring and cross-country racing (American Automobile Production, 1928). Since Benz and Daimler’s internal combustion engine-based vehicles met the elite community’s requirement of long-distance travel and enjoyed the advantage of a short engine lag time, their sales flourished and a “car culture” was born (American Automobile Production, 1928). In contrast, electric vehicles remained operational for a short period of time before the batteries required charging, making them unusable for long-distance travel, while steam-engine based vehicles suffered a long and inconvenient delay for steam formation before people could embark on a journey (American Automobile Production, 1928). It is important to note that no government support is evident in the first 30 years of automobile development, which had been driven entirely by private, independently rich investors, and sold as a luxury product. Additionally, advancements made while serving this high margin clientele steadily allowed for the higher scale of production, economies of scale, and significant cost reductions that ultimately made cars ubiquitous, as was with the case of many 20th Century innovations (Hall, 2016).
By 1905, the yearly production of automobiles approximated 25,000 in numbers (Griffin, 1925). Monumental to this advancement was the dissatisfaction incurred from the waste generated on roads from the use of horses as a means of transportation (Thompson, 2019). As city populations grew and industrialization progressed, the environmental dilemma of disease-ridden horse dung overgrew the advantage of the self-subsisting and economical animal. The automobile was deemed a greener alternative by urban developers and was heavily promoted by publications such as “The Horseless Age” as a definitive solution to the road sanitation (Nikiforuk, 2013). To enhance citizens’ comprehension of automobile benefits, the unit of horsepower was employed to define the working power of automobiles (Thompson, 2019). Further marketing strategies by motorists, car manufacturers and wealthy car owners helped to ultimately relieve some of the concerns that came with automobile use, such as safety. The appearance of asphalt paved roads in the 1870s granted easy assimilation for the use of petrol-fueled automobiles (Thompson, 2019). In an effort to improve city sanitation in the late 19th Century, engineers re-designed roads to accommodate sewage systems and asphalt became the preferred material to rebuild roads as it allowed for a more comfortable travel experience (Thompson, 2019). In the early 1900s, automobiles in the United States and Europe were handmade by several small companies that went out of business just as easily as they first started (Seltzer, 1928). This was attributed to high competition and the inability to reach economies of scale. With the advent of assembly lines and synchronized manufacturing in the 1910s, mass production became prevalent thanks to the efforts of Henry Ford and Ransom E. Olds (American Automobile Production, 1928). In 1915, 900,000 automobiles were produced and sold, in contrast to the 300 cars present in the market just under 20 years ago in 1895 (Griffin, 1925). This stage was characterized by the early industrialists as the end of the demonstration period in automobile development, as this technology was no longer perceived as novel. At the onset of World War 1, the advantage of mass-produced internal combustion automobiles was established, which were used for long-range transportation and military activities. By 1925, ∼4 million automobiles were present on roads and the industry entered into the phase of global expansion (Griffin, 1925).
Through the example of the automobile industry, it is understood that initial attempts at developing novel CO2 utilization technologies may serve a less commoditized, higher margin market before turning to commodities. Additionally, leveraging niche product lines that buy the technology time to iterate and improve its economic metrics and support infrastructure over time may be required. Trial and error in the industry is a natural course to upscaling with demonstration environments such as the Paris-Rouen competition in the case of automobiles, and the Carbon X-PRIZE competition in the case of novel CO2 utilization, allowing for increased investments. With the solar industry, the initial solar cells were a company venture which transitioned into a country-wide necessity in later years. Both turning points were heavily financed by interested parties, whereas automobiles being personal ventures did not receive governmental or corporate funding until much later into their lifecycle. Currently, novel CO2 utilization resembles solar industry, with multiple organizations and governments interested in its deployment (Strefler et al., 2018). The demand of automobiles grew rapidly in a short amount of time as this invention surpassed the performance ability of traditional transportation for commercial, personal, and military uses. Solar cells in contrast have not seen a complete displacement of traditional sources of energy. Moving forward, the ideal scenario for novel CO2 utilization is to design products and materials that out-perform traditional materials such as cements, plastics, and biofuels. Figure 1 shows similar exponential growths for both industries considered herein, with the solar energy curve being shifted almost a century forward.
Recommendations and Conclusion
The field of CDR operates in a nexus of industrial energy systems and consumer behavior. Conventional fossil fuel energy sources and the use of automobiles contribute to a significant proportion of CO2 emissions globally (Hall, 2016), and technologies in both these sectors offer important insights into challenges and opportunities in deploying novel CO2 utilization at a meaningful scale to reduce carbon emissions.
Whilst the solar industry received government support at virtually all TRL levels, the automobile industry, due to its inherently superior value proposition relative to the status quo, relied solely on small, private investments in its first 30 years of development. Both technologies, however, consistently improved their offerings through iterative and breakthrough advances.
Combining an effective market value proposition with targeted policy incentives, whilst facilitating the development and optimal operation of fundamental and empirical research and development in the field of novel CO2 utilization, is critical to enabling and accelerating the global deployment of this suite of CDR technology.
Based on the development trajectories of two commercially relevant and successfully scaled industries, the following factors are recommended for consideration in order to accelerate the development of novel CO2 utilization technologies:
(1) A principles-driven framework to address the systemic challenges associated with hardware-heavy technology sectors, particularly novel CO2 utilization technologies, needs to be established and adhered to. The principles could include:
a. Technological analysis on scope, impact potential, scalability challenges, and current state of supplementary technologies required for scaled deployment.
b. Economic analysis considering commercial viability, status of target markets, short- and long-term trends, and business models (evaluated differently for Business-to-Business and Business-to-Consumer ventures).
c. A standardized CO2 utilization-wide definition of TRL (Technology Readiness Level) and IRL (Investment Readiness Level) adjudicated by an independent board of experts to ensure consistent evaluation of technologies across the industry.
d. Transparent feedback on all pathways considered to ensure an efficient dissemination of technology and market-related information.
(2) A fundamentals-driven exercise to identify and investigate potential pathways that can meaningfully reduce global CO2 emissions using realistic technical and economic assumptions must be conceived and deployed by the stakeholders and decision makers in the field.
(3) An organized access to public and private capital for the scale-up and commercial delivery of pertinent technologies need to be established. This can be achieved by:
a. Development of clear pathways for funding at various TRL and IRL levels.
b. Commissioning of a Carbon Reduction program that follows the setup of Advanced Research Projects Agency–Energy (ARPA-E), focused on utilizing start-ups to combine fundamental research with industry partners.
c. Regular technical and investment workshops to ensure consistent communication between academia, entrepreneurs, public and private investment entities.
d. Formulation of 1- and 5-year plans to establish key performance metrics associated with research, development, and commercialization aspects of the industry.
(4) Policy incentives provided by individual governments need to be revised for accelerating the deployment of novel CO2 utilization technologies at a holistic global level.
a. The Canadian government should provide a comprehensive guideline on the eligibility criteria for its Carbon Capture Tax Credit (CCTC) program to incentivize the standardization of CO2 utilization technologies. A framework for grant eligibility should be established with funding options covering all aspects of the CO2 utilization process from R&D to operations and commercialization.
b. The US Department of Energy should extend and specify tax benefits regarding the 45Q policy, while reducing the threshold for projects to be eligible, thereby enabling synthesis of vast processes and products pertaining to CO2 utilization technologies.
c. The European Green Deal should include investment policies and initiatives regarding CO2 utilization technologies to catalyze the adoption of CCS and improve its commercial viability.
To achieve carbon emission reductions of scale, significant behavior change is required from customers to facilitate successful industrial deployment of novel CO2 utilization technologies. Importantly, a transparent and standardized methodology on all proposed CDR pathways needs to be developed and shared across the system to create a motive for behavioral change and adoption.
To effectively address these challenges, the deployment capacity, economic feasibility, and the permanence of carbon fixation in the new products and materials are fundamentally important considerations for any CO2 utilization technology and associated business. Tackling the collection and the deconvolution of this information requires scientific and engineering innovation as well as cross-disciplinary collaborations to replace traditional methods. As with many other hardware technologies, the concert of public and private support of vetted technology projects is vital for scale up and commercialization of novel CO2 utilization technologies, in order to overcome the hampering inertia of high cost, low production quality and small-scale.
Due diligence is needed on part of scientific experts and policy makers in order to substantiate claims to avoid unjust romanticizing of the fruits of the CO2 utilization market. CDR is a portfolio of techniques and should be treated as such. Enhanced oil recovery and BECCS are but threads in the grand fabric of CDR, and not one technology or technique is the crux of climate mitigation. As such, enabling novel CO2 utilization technologies to mitigate CO2 emission trends is critical if COP21 commitments are to be met.
Author Contributions
YW is the primary author of the paper, she compiled the review materials and wrote the manuscript. VK and PZ are co-authors of the paper, they both contributed to the editing of the manuscript and content organization. AS is the primary investigator for the manuscript, he provided the direction for the review and aided in the editing process.
Conflict of Interest
AS is the CEO and Founder of Carbon Upcycling Technologies Inc. Authors YW and PZ were employed by the company Carbon Upcycling Technologies Inc.
The remaining authors declare that the research was conducted in the absence of any commercial or financial relationships that could be construed as a potential conflict of interest.
Acknowledgments
The authors would like to express our gratitude to Alberta Innovates, and the University of Calgary nanotechnology group (UofC nanoGroup), respectively, for funding and organizing the 2019 Calgary nanoNexus networking event which ultimately enabled an industry-academia collaboration leading to the completion of this manuscript.
References
Allen, M. R., Pauline Dube, O., Solecki, W., Aragón-Durand, F., Cramer France, W., Humphreys, S., et al. (2018). “Framing and context,” in Global warming of 1.5°C. An IPCC Special Report on the impacts of global warming of 1.5°C above pre-industrial levels and related global greenhouse gas emission pathways, in the context of strengthening the global response to the Australia. Available at: https://www.ipcc.ch/site/assets/uploads/sites/2/2019/05/SR15_Chapter1_Low_Res.pdf (Accessed September 9, 2019).
American Automobile Production (1928). The Finance of American Automobile production. Econ. Hist. Arch. 107 (4428), 21. doi:10.1192/bjp.111.479.1009-a
Arnelf, N. W., and Reynard, N. S. (1996). The effects of climate change due to global warming on river flows in Great Britain. J. Hydrol. 183 (3–4), 397–424. doi:10.1016/0022-1694(95)02950-8
autoTRADER.ca. (2019). A brief history of early electric cars. Available at: https://www.autotrader.ca/newsfeatures/20190404/a-brief-history-of-early-electric-cars/. (Accessed September 7, 2019).
Baena-Moreno, F. M., Rodríguez-Galán, M., Vega, F., Alonso-Fariñas, B., Vilches Arenas, L. F., and Navarrete, B. (2019). Carbon capture and utilization technologies: a literature review and recent advances. Energy Sour. Part A Recover Util. Environ. Eff. 41 (12), 1403–1433. doi:10.1080/15567036.2018.1548518
Beck, D. F. (2013). Technology development life cycle processes, Albuquerque. Available at: http://prod.sandia.gov/techlib/access-control.cgi/2013/133933.pdf
Bobeck, J., Peace, J., Ahmad, F. M., and Munson, R. (2019). Carbon utilization—a vital and effective pathway for decarbonization, Arlington. Available at: https://www.c2es.org/site/assets/uploads/2019/09/carbon-utilization-a-vital-and-effective-pathway-for-decarbonization.pdf (Accessed December 8, 2019).
bp. (2019). Renewable energy. Available at: https://www.bp.com/en/global/corporate/energy-economics/statistical-review-of-world-energy/renewable-energy.html.html#solar-energy. (Accessed December 10, 2019).
Bube, R. H., and Fahrenbruch, A. L. (1981). Photovoltaic effect. Adv. Electron. Electron. Phys. 56 (C):163–217. doi:10.1007/s10811-010-9604-9
Bui, M., Adjiman, C. S., Bardow, A., Anthony, E. J., Boston, A., Brown, S., et al. (2018). Carbon capture and storage (CCS): the way forward. Energy Environ. Sci. 11 (5):1062–1176. doi:10.1039/C7EE02342A
Carey, P. (2017). Carbon8 Systems: developing an innovative and profitable process that combines waste CO2 and thermal residues, to create a carbon negative aggregate for construction and lock CO2 in for good within our built environment. Available at: http://nas-sites.org/dels/files/2018/02/1-5-CAREY-Carbon8-Systems-NAS.pdf (Accessed August 14, 2020).
Center for Climate and Energy Solutions. (2020). Global emissions. Available at: https://www.c2es.org/content/international-emissions/ (Accessed April 23, 2020).
Chung, I. K., Beardall, J., Mehta, S., Sahoo, D., and Stojkovic, S. (2011). Using marine macroalgae for carbon sequestration: a critical appraisal. J. Appl. Phycol. 23(5):877–886. doi:10.1007/s10811-010-9604-9
Ciais, P., Sabine, C., Bala, G., Bopp, L., Brovkin, V., Canadell, J., et al. (2013). “Carbon and other biogeochemical cycles,” in Climate change 2013: the physical science basis. Contribution of working group I to the fifth assessment report of the intergovernmental panel on climate change. New York. Available at: https://www.ipcc.ch/site/assets/uploads/2018/02/WG1AR5_Chapter06_FINAL.pdf (Accessed September 15, 2019).
Consoli, C. (2019). Bioenergy and carbon capture and storage. Available at: https://www.globalccsinstitute.com/wp-content/uploads/2019/03/BECCS-Perspective_FINAL_18-March.pdf (Accessed July 26, 2020).
Cromer, G. C., Cromer, O. C., Foster, C. G., and Purdy, K. W. (2018). “Automobile,” in Encyclopædia Britannica. Chicago, IL: Encyclopædia Britannica, inc. Available at: https://www.britannica.com/technology/automobile (Accessed September 7, 2019).
de Coninck, H., and Revi, A. (2018). “Strengthening and implementing the global response,” in Global warming of 1.5°C. An IPCC special report on the impacts of global warming of 1.5°C above pre-industrial levels and related global greenhouse gas emission pathways, in the context of strengthen. Available at: https://www.ipcc.ch/site/assets/uploads/sites/2/2019/05/SR15_Chapter4_High_Res.pdf (Accessed November 17, 2019).
Dezem, V. (2019). Cement produces more pollution than all the trucks in the world. Bloomberg. Available at: https://www.bloomberg.com/news/articles/2019-06-23/green-cement-struggles-to-expand-market-as-pollution-focus-grows. (Accessed December 8, 2019).
Dickmann, J., Appenrodt, N., and Brenk, C. (2014). Making Bertha: radar is the key to Mercedes-Benz’s robotic car. IEEE Spectr. 51 (8), 44–49. doi:10.1109/MSPEC.2014.6866437
Field, C. B., and Mach, K. J. (2017). Rightsizing carbon dioxide removal. Science 356 (6339), 706–707. doi:10.1126/science.aam9726
Fuss, S., Lamb, W. F., Callaghan, M. W., Hilaire, J., Creutzig, F., Amann, T., et al. (2018). Negative emissions—Part 2: costs, potentials and side effects. Environ. Res. Lett. 13 (6), 1–47. doi:10.1088/1748-9326/aabf9f
Global CCS Institute. (2011). Accelerating the uptake of Ccs : industrial use of captured carbon dioxide. New York City, NY: Parsons Brinckerhoff Technology.
Global CO2 Initiative, CO2 Sciences Inc. (2016). Global roadmap for implementing CO2 utilization, 1–61. Available at: https://assets.ctfassets.net/xg0gv1arhdr3/27vQZEvrxaQiQEAsGyoSQu/44ee0b72ceb9231ec53ed180cb759614/CO2U_ICEF_Roadmap_FINAL_2016_12_07.pdf
Gough, C., Garcia-Freites, S., Jones, C., Mander, S., Moore, B., Pereira, C., et al. (2018). Challenges to the use of BECCS as a keystone technology in pursuit of 1.5°C. Glob Sustain. 1, 1–9. doi:10.1017/sus.2018.3
Griffin, C. E. (1925). The evolution of the Automobile market. Harvard Bus Rev. 4, 407–416. doi:10.2307/1883944
Hall, B. H. (2016). Univerisity of CB, Khan B (Univerisity of CB. New economy handbook. Adopt. New Technol. 24 (5), 381. doi:10.1097/MOO.0000000000000298
Harvey, A., Larson, A., and Patel, S. (2017). History of power: the evolution of the electric generation industry. Power 161 (10), 32–47. Available at : https://www.powermag.com/history-of-power-the-evolution-of-the-electric-generation-industry/ (Accessed October 19, 2020)
Haszeldine, R. S., Flude, S., Johnson, G., and Scott, V. (2018). Negative emissions technologies and carbon capture and storage to Achieve the Paris agreement commitments. Philos. Trans. R. Soc. A Math. Phys. Eng. Sci. 376 (2119), 1–23. doi:10.1098/rsta.2016.0447
Hepburn, C., Adlen, E., Beddington, J., Carter, E. A., Fuss, S., Mac Dowell, N., et al. (2019). The technological and economic prospects for CO2 utilization and removal. Nature 575 (7781), 87–97. doi:10.1038/s41586-019-1681-6
IEA. (2018). World Energy Outlook 2019. Paris. Available at: https://www.iea.org/weo2019/ (Accessed November 13, 2019).
IPCC. (2014). Climate change 2014 synthesis report summary for Policymakers. Available at: https://www.ipcc.ch/site/assets/uploads/2018/02/AR5_SYR_FINAL_SPM.pdf (Accessed August 27, 2019).
Irlam, L. (2017). Global costs of carbon capture and storage. Available at: https://www.globalccsinstitute.com/archive/hub/publications/201688/global-ccs-cost-updatev4.pdf (Accessed August 10, 2020).
Jones, C. R., Olfe-Kräutlein, B., Naims, H., and Armstrong, K. (2017). The social acceptance of carbon dioxide utilisation: A review and research Agenda. Front Energy Res. 5, 1–13. doi:10.3389/fenrg.2017.00011
Kaliyan, N., Morey, R. V., Wilcke, W. F., Alagusundaram, K., and Gayathri, P. (2007). “Applications of carbon dioxide in food and processing industries: current status and future thrusts,” in 2007 ASABE annual international meeting. Minneapolis: American Society of Agricultural and Biological Engineers.
Kätelhön, A., Meys, R., Deutz, S., Suh, S., and Bardow, A. (2019). Climate change mitigation potential of carbon capture and utilization in the chemical industry. Proc. Natl. Acad. Sci. U. S. A. 166 (23), 11187–11194. doi:10.1073/pnas.1821029116
Keller, D. P., Lenton, A., Littleton, E. W., Oschlies, A., Scott, V., and Vaughan, N. E. (2018). The effects of carbon dioxide removal on the carbon cycle. Curr. Clim. Change Rep. 4 (3), 250–265. doi:10.1007/s40641-018-0104-3
Mach, K. J., Planton, S., and von Stechow, C. (2014). “Annex II: Glossary,” in: Climate change 2014: synthesis report. Contribution of Working Groups I, II and III to the Fifth Assessment Report of the Intergovernmental Panel on Climate Change. Available at: https://www.ipcc.ch/site/assets/uploads/2019/01/SYRAR5-Glossary_en.pdf (Accessed November 16, 2019).
Malischek, R. (2020). CCUS in Power. Available at: https://www.iea.org/reports/ccus-in-power (Accessed September 6, 2020).
Masson-Delmotte, V., Zhai, P., Portner, H. O., Roberts, D., Skea, J., Shukla, P., et al. (2018). “Summary for policymakers,” in Global Warming of 1.5°C. An IPCC Special Report on the impacts of global warming of 1.5°C above pre-industrial levels and related global greenhouse gas emission pathways, in the context of strengthening the global response to 2018. Available at: https://www.ipcc.ch/site/assets/uploads/sites/2/2019/05/SR15_SPM_version_report_LR.pdf (Accessed September 15, 2019).
McBride, S. D. (1999). History Just Ahead. Madison, WI: State Historical Society of Wisconsin. Available at: https://www.wisconsinhistory.org/Records/Article/CS13641 (Accessed September 7, 2019).
McCormack, C. G., Born, W., Irvine, P. J., Achterberg, E. P., Amano, T., Ardron, J., et al. (2016). Key impacts of climate engineering on biodiversity and ecosystems, with priorities for future research. J. Integr. Environ. Sci. 13 (2–4), 103–128. doi:10.1080/1943815X.2016.1159578
Metz, B., Davidson, O., de Coninck, H., Loos, M., and Meyer, L. I. P. C. C. (2005). IPCC special report on carbon dioxide capture and storage. prepared by working group III of the intergovernmental panel on climate change.
Mission Innovation. (2017). Report of the carbon capture, utilization and storage experts’ workshop. Houston. Available at: https://www.energy.gov/sites/prod/files/2018/05/f51/Accelerating Breakthrough Innovation in Carbon Capture%2C Utilization%2C and Storage_0.pdf (Accessed December 9, 2019).
Müller, L. J., Kätelhön, A., Bachmann, M., Zimmermann, A., Sternberg, A., and Bardow, A. (2020). A guideline for Life cycle assessment of carbon capture and utilization. Front Energy Res. 8, 1–20. doi:10.14279/depositonce-9904
NASA. (2008a). NASA global climate change and global warming: vital Signs of the Planet–carbon dioxide. Available at: https://climate.nasa.gov/vital-signs/carbon-dioxide/ (Accessed September 8, 2019).
NASA. (2008b). NASA global climate change and global warming: vital Signs of the Planet–global temperatures. Available at: https://climate.nasa.gov/vital-signs/global-temperature/ (Accessed October 7, 2019).
National Research Council, Division on Earth and Life Studies, Ocean Studies Board, Board on Atmospheric Sciences and Climate, Committee on Geoengineering Climate: Technical Evaluation and Discussion of Impacts. (2015). Climate intervention: carbon dioxide removal and reliable sequestration. London, UK: National Academies Press, 1–140.
Nikiforuk, A. (2013). The Big shift last time: from horse dung to car smog|The Tyee. Available at: https://thetyee.ca/News/2013/03/06/Horse-Dung-Big-Shift/ (Accessed September 7, 2019).
Norhasyima, R. S., and Mahlia, T. M. I. (2018). Advances in CO2 utilization technology: a patent landscape review. J CO2 Util. 26, 323–335. doi:10.1016/j.jcou.2018.05.022
Our World in Data (2018). Energy. Available at: https://ourworldindata.org/energy (Accessed October 19, 2020).
Paris Agreement. (2015). In Paris; 2015, 1–25. Available at: https://unfccc.int/sites/default/files/english_paris_agreement.pdf (Accessed September 14, 2019).
Perlin, J (2004). National REL. the silicon solar cell turns 50, Golden. Available at: https://www.nrel.gov/docs/fy04osti/33947.pdf (Accessed September 7, 2019).
Peters, M., Köhler, B., Kuckshinrichs, W., Leitner, W., Markewitz, P., and Müller, T. E. (2011). Chemical technologies for exploiting and recycling carbon dioxide into the value chain. ChemSusChem. 4 (9), 1216–1240. doi:10.1002/cssc.201000447
Pinner, D., and Rogers, M. (2015). Solar power comes of age how harnessing the sun got cheap and practical. Foreign Aff. 94(2), 19–33. doi:10.1002/cssc.201000447
Popp, D. (2010). Innovation and climate policy. Annu. Rev. Resour. Econ. 2, 275–298. doi:10.1146/annurev.resource.012809.103929
Prentice, I., Farquhar, G. D., Fasham, M. J. R., Goulden, M. L., Heimann, M., Jaramillo, V. J., et al. (2019). The carbon cycle and atmospheric carbon dioxide. Available at: https://www.ipcc.ch/site/assets/uploads/2018/02/TAR-03.pdf (Accessed September 8, 2019).
Read, D., and Royal, S. (2001). The role of land carbon sinks in mitigating GlobalCclimate change. London. Available at: www.royalsoc.ac.uk (Accessed September 9, 2019).
Rogelji, J., Shindell, D., Jiang, K., Fifita, S., Forster, P., Ginzburg, V., et al. (2018). “Mitigation pathways compatible with 1.5°C in the context of sustainable development,” in Global warming of 1.5°C. An IPCC special report on the impacts of global warming of 1.5°C above pre-industrial levels and related global greenhouse gas emission pathway. Available at: https://www.ipcc.ch/site/assets/uploads/sites/2/2019/02/SR15_Chapter2_Low_Res.pdf (Accessed September 15, 2019).
Rubin, E. S., Davison, J. E., and Herzog, H. J. (2015). The cost of CO2 capture and storage. Int. J. Greenh. Gas Control. 40, 378–400. doi:10.1016/j.ijggc.2015.05.018
Russell, L. M., Rasch, P. J., MacE, G. M., Jackson, R. B., Shepherd, J., Liss, P., et al. (2012). Ecosystem impacts of geoengineering: A review for developing a science plan. Ambio 41 (4), 350–369. doi:10.1007/s13280-012-0258-5
Sandalow, D., Aines, R., Friedmann, J., McCormick, C., and McCoy, S. (2017). Carbon dioxide utilization (CO2U) ICEF roadmap 2.0. Available at: https://www.icef-forum.org/pdf/2018/roadmap/CO2U_Roadmap_ICEF2017.pdf (Accessed August 6, 2020).
Seltzer, L. H. (1928). A financial history of the American automobile industry. Detroit: The Riverside Press Cambridge, 3–285. Available at: https://hdl.handle.net/2027/mdp.39015071115698 (Accessed September 7, 2019).
Sharma, S., Vander Zanden, M. J., Magnuson, J. J., and Lyons, J. (2011). Comparing climate change and species invasions as drivers of coldwater fish population extirpations. PLoS ONE. 6 (8), e22906. doi:10.1371/journal.pone.0022906
Siirola, J. J. (2014). Speculations on global energy demand and supply going forward. Curr. Opin. Chem. Eng. 5, 96–100 10.1016/j.coche.2014.07.002.
Smil, V. (2019). Energy transitions : global and national perspectives, 282. Available at: http://vaclavsmil.com/2016/12/14/energy-transitions-global-and-national-perspectives-second-expanded-and-updated-edition/ (Accessed December 10, 2019).
Smit, B., Park, A. H. A., and Gadikota, G. (2014). The grand challenges in carbon capture, utilization, and storage. Front. Energy Res. 2, 2013–2015. doi:10.3389/fenrg.2014.00055
Smith, P., Davis, S. J., Creutzig, F., Fuss, S., Minx, J., Gabrielle, B., et al. (2016). Biophysical and economic limits to negative CO2 emissions. Nat. Clim. Chang. 6 (1), 42–50. doi:10.1038/nclimate2870
Sovacool, B. K. (2009). Early modes of transport in the United States: lessons for modern energy policymakers. Policy Soc. 27 (4), 411–427. doi:10.1016/j.polsoc.2009.01.006
Special Report (2018). Demonstrating carbon capture and storage and innovative renewables at commercial scale in the EU: intended progress not achieved in the past decade. Available at: https://www.eca.europa.eu/Lists/ECADocuments/SR18_24/SR_CCS_EN.pdf (Accessed December 7, 2019).
Steuart, W. M., and Hawes, J. G. (1918). Census of manufactures: 1914. Washington, D.C.: Govt. Print.Off., 2–14.
Strefler, J., Amann, T., Bauer, N., Kriegler, E., and Hartmann, J. (2018). Potential and costs of carbon dioxide removal by enhanced weathering of rocks. Environ. Res. Lett. 13(3), 13–27. doi:10.1088/1748-9326/aaa9c4
The History of Solar. (2019). The History of Solar. Available at: https://www1.eere.energy.gov/solar/pdfs/solar_timeline.pdf (Accessed September 7, 2019).
Thompson, A. F. M. L. (2019). Nineteenth-century horse sense. Econ. Hist. Soc. 29 (1), 60–81. doi:10.2307/2594507
US Department of Energy. (2019). U.S. Department of Energy Announces $110M for Carbon Capture, Utilization, and Storage. Available at: https://www.energy.gov/articles/us-department-energy-announces-110m-carbon-capture-utilization-and-storage (Accessed December 9, 2019).
U.S. Department of Energy. (2016). Carbon capture, utilization and storage: climate change, Economic Competitiveness, and Energy Security. Available at:https://www.energy.gov/sites/prod/files/2017/01/f34/CarbonCapture%2C Utilization%2CandStorage-Climate Change%2CEconomicCompetitiveness%2CandEnergySecurity_0.pdf (Accessed November 28, 2019).
Wheeler, T., and Von Braun, J. (2013). Climate change impacts on global food security. Science 341 (6145), 508–513. doi:10.1126/science.1239402
Zhang, Z., Pan, S. Y., Li, H., Cai, J., Olabi, A. G., Anthony, E. J., et al. (2020). Recent advances in carbon dioxide utilization. Renew Sustain Energy Rev. 125, 109799. doi:10.1016/j.rser.2020.109799
Keywords: carbon dioxide removal techniques, carbon capture, carbon storage, carbon utilization, climate change mitigation
Citation: Warsi Y, Kabanov V, Zhou P and Sinha A (2020) Novel Carbon Dioxide Utilization Technologies: A Means to an End. Front. Energy Res. 8:574147. doi: 10.3389/fenrg.2020.574147
Received: 19 June 2020; Accepted: 22 September 2020;
Published: 28 October 2020.
Edited by:
Rafael Mattos Dos Santos, University of Guelph, CanadaReviewed by:
Pol Knops, Green Minerals BV, NetherlandsEric Miles Kennedy, The University of Newcastle, Australia
Stefano Stendardo, Italian National Agency for New Technologies, Energy and Sustainable Economic Development (ENEA), Italy
Copyright © 2020 Warsi, Kabanov, Zhou and Sinha. This is an open-access article distributed under the terms of the Creative Commons Attribution License (CC BY). The use, distribution or reproduction in other forums is permitted, provided the original author(s) and the copyright owner(s) are credited and that the original publication in this journal is cited, in accordance with accepted academic practice. No use, distribution or reproduction is permitted which does not comply with these terms.
*Correspondence: Apoorv Sinha, YS5zaW5oYUBjYXJib251cGN5Y2xpbmcuY29t