Valorization of Wastewater Resources Into Biofuel and Value-Added Products Using Microalgal System
- 1Faculty of Applied Sciences and Biotechnology, Shoolini University of Biotechnology and Management Sciences, Solan, India
- 2Department of Bioinformatics, Mahila Mahavidyalaya, Banaras Hindu University, Varanasi, India
- 3Department of Chemical Engineering, Konkuk University, Seoul, South Korea
- 4Ministry of Education Key Laboratory of Cell Activities and Stress Adaptations, School of Life Sciences, Lanzhou University, Lanzhou, China
- 5Department of Biological Engineering, College of Engineering, Konkuk University, Seoul, South Korea
- 6Institute for Ubiquitous Information Technology and Application, Konkuk University, Seoul, South Korea
Wastewater is not a liability, instead considered as a resource for microbial fermentation and value-added products. Most of the wastewater contains various nutrients like nitrates and phosphates apart from the organic constituents that favor microbial growth. Microalgae are unicellular aquatic organisms and are widely used for wastewater treatment. Various cultivation methods such as open, closed, and integrated have been reported for microalgal cultivation to treat wastewater and resource recovery simultaneously. Microalgal growth is affected by various factors such as sunlight, temperature, pH, and nutrients that affect the growth rate of microalgae. Microalgae can consume urea, phosphates, and metals such as magnesium, zinc, lead, cadmium, arsenic, etc. for their growth and reduces the biochemical oxygen demand (BOD). The microalgal biomass produced during the wastewater treatment can be further used to produce carbon-neutral products such as biofuel, feed, bio-fertilizer, bioplastic, and exopolysaccharides. Integration of wastewater treatment with microalgal bio-refinery not only solves the wastewater treatment problem but also generates revenue and supports a sustainable and circular bio-economy. The present review will highlight the current and advanced methods used to integrate microalgae for the complete reclamation of nutrients from industrial wastewater sources and their utilization for value-added compound production. Furthermore, pertaining challenges are briefly discussed along with the techno-economic analysis of current pilot-scale projects worldwide.
Introduction
Water is a natural resource and considered the most essential component of all living creatures. Almost 70% of the earth’s surface is covered by water but only about 3% of it is fresh water (Cao et al., 2019). Water being such a scarce commodity, its integrity, and environmental perturbations should never be compromised. Every year about 3.4 million people die, and many suffer from a plethora of water-borne diseases due to polluted water being discharged recklessly from industries and wastewater treatment plants (Osiemo et al., 2019). Different sources of wastewater like municipal, agricultural, and industrial contain larger quantities of nutrients organic and inorganic contaminants (Bhatia et al., 2021d). Wastewater is released into the water bodies without proper treatment which affects the aquatic ecosystem adversely and results in eutrophication (Preisner et al., 2021). The root cause of this formidable situation is the lack of efficient, economical, and energy-saving methods for wastewater treatment. Conventional methods like electrochemical methods, precipitation, and membrane technologies are all non-sustainable (Rajasulochana and Preethy, 2016). They all are backed with instability, resource wasting, fouling, and exorbitant energy consumption. On the other hand, this wastewater offers a perfect niche for microalgae, unicellular photosynthetic organisms to grow and flourish synergistically with the prevailing bacterial colonies present in the sewer water. To save the environment and recycle the nutrients, wastewater treatment using microalgae is an imperative step. The use of microalgae for wastewater treatment is advantageous as it will help to reduce the biochemical oxygen demand (BOD) and chemical oxygen (COD) demand and remove the inorganic nutrients like nitrates and phosphates from the wastewater (Taziki et al., 2015). This could save a huge amount of cost as algae will assimilate all the nutrients along with bio fixing of inorganic carbon from the atmosphere (CO2 sequestration) (Bhatia et al., 2019a). Moreover, additional CO2 can be sourced from flue gas which is obtained from power plant emissions. Thereby, addressing air and water pollution simultaneously. Different wastewaters have different compositions based on their source. Microalgal growth is affected by nutrient components and other factors like temperature, pH, salinity, light intensity, duration, etc. (Cabello et al., 2015; Vuppaladadiyam et al., 2018). The selection of microalgal species is very important depending upon the characteristics of wastewater for efficient wastewater treatment. The optimum physiochemical conditions need to be maintained to obtain the maximum possible yield. It is reported that removal efficiency of 65-76% of COD, 68-81% of total nitrogen, and 90-100% of total phosphorous from piggery wastewater by freshwater microalgae Chlorella zofingiensis (Zhu et al., 2013). In another report, it was studied that COD removal of 50.9 and 56.5% from primary and secondary effluents, respectively, by green algae Chlorella spp. (Wang et al., 2010). Microalgae mediated wastewater treatment method can also produce biofuel (bioelectricity and biohydrogen) simultaneously or recovered biomass can be further used as feedstock to produce various commercially valuable biochemicals (Bhatia et al., 2021d). Along with nutrient recovery from waste streams, Maity et al. (2014) reported simultaneous production of 1068.383 mg g−1 dry wt. biomass of lipid with C16:0, C18:2n-6, C18:1, and C16:1 FAME content and 0.264 mV, 0.008 mW cm–2 of electric potential and bioelectricity using Leptolyngbya sp. JPMTW1 within 7 days. Microalgal biomass after wastewater treatment can be recovered using technologies such as centrifugation, flocculation, and electrochemical precipitation methods. Microalgal biomass is rich in carbohydrates, lipids, and proteins and is further used for extraction of commercially important products (lipids, carotenoids, polyhydroxyalkanoates, etc.) are biomass can be used as a feedstock for microbial fermentation to produce other commercial products (Bhatia et al., 2017; Goswami et al., 2020).
Despite the lucrative benefits of microalgae-based wastewater treatment, few limitations still persist. The current open and closed cultivation methods render contamination risks and huge operational costs, respectively. Further, harvesting and dewatering account for 20-30% of the total cost (Fasaei et al., 2018). Therefore, the concept of integrated systems is briefly discussed which can cut the major costs and make the method more economical. Besides, wastewater composition, culture collapse, salinity, etc., are some other pitfalls that could be addressed by subsequent research and development in this field. This review will discuss the current wastewater treatment technologies in action with microalgae dominance in the waste realm. It will address various cultivation systems and harvesting methods, integrating microalgae for wastewater treatment and sustainable product production. Furthermore, the present state-of-the-art review will also highlight the techno-economic analysis and status of nutrient recovery processes.
Composition of Wastewater
Wastewater consists of pollutants from different walks of life, reflecting the lifestyle and activities of people living in the city. It is a by-product of industrial, domestic, commercial, and agricultural chores and contains human excreta, washing wastes from houses, pesticides, nutrients from agricultural farms, organic biodegradable wastes from hospitals and abattoirs, non-biodegradable from pharmaceuticals manufacturing, toxic waste such as dyes and heavy metals from other industries, leachates from landfills and storm water during high precipitations (Rana et al., 2017). All these contaminants comprise only 0.1% of suspended and dissolved solids, while 99.9% is pure water (Samer, 2015). Most of it is organic debris, and only a small part consists of non-biodegradable inorganic wastes, which pose a significant problem in reclamation. Three-quarters of volatile (organic) solids in sewage are carbohydrates, proteins, and fats from discarded food waste, kitchen waste, dead organisms, etc. (Samer, 2015). The non-volatile part includes heavy metals salts, grit, and sand dust.
Microorganisms form a significant part of sewage treatment. They can range from moderate to high-risk biological pollutants like antibiotic resistant bacteria, viruses, protozoa, biotoxins, and helminths. Such infectious agents pose a substantial threat to human life and the environment (Jia and Zhang, 2019). On the other hand, some of the microbes like algae, pseudomonas, zoogloeal are environmental-friendly and aid in wastewater treatment. Sources of such microbes include faeces and other bodily fluids from several sick people and asymptomatic hosts leading to ailments like cholera, jaundice, typhoid from bacteria, hepatitis from viruses, and dysentery from protozoans. Coliform Escherichia coli are present in vitality, indicating other pathogens, and are therefore used to measure the infecting efficiency of sewage (Hendricks and Pool, 2012).
Among all, municipal wastewater is the most preferred wastewater for microalgae-based wastewater treatments because of the adequate amount of nutrients and a low number of toxic substances compared to industrial wastewater. It is generally categorized into (i) raw sewage, (ii) secondary effluent, and (iii) centrate, i.e., by-product of sludge dewatering. Studies have proved that centrate is the most suitable medium for microalgae cultivation with nutrient removal efficiencies of 89.1%, 90.8%, 93.9%, and 80.9% for TN, COD, NH4-N, and TP, respectively (Wang et al., 2010; Li et al., 2011). Moreover, additional CO2 (5-150%) along with municipal wastewater could enhance nutrient recovery and lipid production simultaneously (Ji et al., 2013). On the other hand, the agricultural waste contains an excessively high amount of ammonia, suspended solids (SS), nutrient load (due to animal manure), chroma, and turbidity which make it unfit for direct microalgae treatment. Therefore, it is generally applied after anaerobic digestion (AD) as much of the organic carbon gets converted to methane, leaving nitrate and phosphate in the digestate. The C/N ratio of the digested manure is comparatively low than centrate which makes the medium ideal for microalgae growth. Only a few algal strains support this type of medium and hence it is used after diluting for certain species (Zhou et al., 2012b; Zhu et al., 2013). Hence, a sequential two-stage cultivation process is preferred for animal manure wastewater and has great economic potential with multiple product production. In contrastingly, there is no standard treatment applicable for industrial wastewater due to its complex nature with added toxicity. Few microalgal species are adapted to exorbitant levels of heavy metals while most of the algal species are intolerable. Ankistrodesmus, Chlorella, and Scenedesmus are a few of the species competent for treating mill, olive oil, and paper industry wastewater (Rawat et al., 2011). The wastewater from the paper industry is characterized by 1720 mg L–1 and 2658 mg L–1 of suspended solids and dissolved solids with an average pH range of 8.5, respectively. The color value obtained is in the range of 735 Platinum Cobalt Unit while chemical oxygen demand and biochemical oxygen demand of 2420 mg L–1 and 778 mg L–1, simultaneously (Singh, 2015; Choi et al., 2017). Another study compares wastewater from three different sources like household, domestic, and offices, and found maximum phosphorus content in domestic waste as given in Table 1 (Vandith et al., 2018). Table 2 provides an elaborate picture of how microalgae can treat different sources of wastewater efficiently (Li et al., 2019; Al-Jabri et al., 2020). For about 38000 million liters per day of sewage generated, only 12000 million liters per day get treated in India, and among 35 metropolitan cities, only 51% of sewages, i.e., 8040 million liters per day of sewage treatment capacity exist for 15644 million liters per day of wastewater generated (National Status of Waste Water Generation and Treatment, 2020). According to The OPEC Fund for International Development wastewater report, the global scenario of sewage treatment is found to be 70% in high-income countries and is even depressing with only 8% in low-income countries (State of the Art Compendium Report on Resource Recovery from Water, 2018). Due to increased urbanization, industrialization, and leading water scarcity due to climate change, the global market related to resource recovery is tending to rise to $22.3 billion by 2021 from $12.2 Billion in 2016 (State of the Art Compendium Report on Resource Recovery from Water, 2018). Therefore, wastewater recovery and resource utilization are still untapped in many underdeveloped and few developing countries, and only a small portion is utilized in a safe and planned manner while the majority of partially treated or untreated wastewater is discharged unregulated into the water bodies.
Microalgae Cultivation Systems
Microalgae can be grown both in an open system and closed systems. An open system is present in nature as naturally occurring ponds that include lagoons, sea, and oceans. The photobioreactors are a closed system for algal production under controlled conditions of temperature, pH, and nutrients for higher yield of the biomass. The cultivation of microalgae can even be performed using wastewater such as domestic sewage water and palm oil milling effluents which can assist in the bioremediation of wastewater (Selmani et al., 1997; Posadas et al., 2017).
Open Systems
Open ponds are the simplest and easiest way to cultivate microalgae on large scale. The various types of open pond systems include lakes, ponds, artificial water bodies such as circular and raceway systems. The algae are cultivated in open pond systems (shallow ponds), and nutrients are added through runoff water from nearby land areas or by channeling the water from sewage/water treatment plants (Carlsson et al., 2007). Mixing is provided by paddle wheels in raceway ponds or rotating arms in circular ponds and mixing can even be provided at certain places in a large pond by the use of impeller blades (Yen et al., 2019). For example, Spirulina can grow at a very high pH (9-12) and therefore is dominant in soda lakes (Bindra et al., 2017). Dunaliella salina is emergent species in the shallow salt pan ponds (Borowitzka and Hallegraeff, 2007). There are various advantages of an open pond system as it provides a large surface area for growth, but it has lower cell concentration and requires efficient harvesting techniques (Tredici, 2004). The major limitation of the open and natural system includes the contamination by protozoa and bacteria and also it is difficult to control all the growth parameters in open systems (Lam et al., 2018). The harvesting of algae from a natural open system is one of the oldest methods and the oldest record of microalgae harvesting was done by Aztec peoples when they harvested Spirulina from Lake Texcoco located at Mexico (Hamed, 2016).
The first artificial ponds to be used are circular ponds and rotating agitators are used for efficient mixing and prevent sedimentation of algal biomass. The major limitation of this system is requirement of high energy usage and high construction cost (Hamed, 2016). This system is used in Japan and Taiwan for the cultivation of Chlorella (Lee, 1997; Shen et al., 2009). The high-rate algal ponds are used for the wastewater treatments pond is a shallow-depth which contains high loads of nitrogen and phosphorous content from wastewater, allows the microalgae to proliferate and produce high biomass (Craggs et al., 2012).
Raceway pond is the most feasible and cost-effective facility to treat large amounts of wastewater than other techniques because it has a low capital cost, more comfortable scale-up, and higher treatment volume (Zhou et al., 2013). The most used open culture system for growth is race-way pond design, which consists of long channels in loops or stirring devices, including paddlewheels. The various limitations of traditional raceway ponds with suspended algal culture are: (1) self-shadowing effect due to limited light penetration; (2) ineffective photosynthesis; (3) energy consumption and expenses for harvesting the suspended algal biomass and (4) reduced effluent quality due to inefficient harvest of the biomass (Chiaramonti et al., 2013).
Closed System (Photo-Bioreactors)
The photobioreactors are used to grow microalgae in a closed system where operating conditions and nutrients can be controlled and monitored using an automatic control system. An example of the closed system is a flat plate reactor with a complex mixer and the conditions required for the growth of Chlorella vulgaris is the optimum conditions of temperature (23.6-26.8°C) and dissolved oxygen (7.6-8.8 mg L–1) (Lakaniemi et al., 2012). The most commonly used photobioreactor are, (i) straight tubes which are arranged flat on the ground or in long vertical rows known as tubular bioreactors and (ii) helical bioreactors which are spirally wound tubes around a central support. The tubes used can be of glass, or perpex for the bioreactor system. The tubular bioreactors are mainly used outdoors and are arranged in different orientations such as vertical, horizontal, inclined, and helical to increase the mass production of algal biomass by capturing maximum sunlight, i.e., an increase in photosynthesis (Ting et al., 2017). The diameter of the tube can be from 10 to 60 mm (Ting et al., 2017). The specific limitation of tubular bioreactors is photosynthetic efficiency, and the energy consumption is higher. The major disadvantage of the tubular photobioreactor is the difference in the concentration along the long tubes that leads to poor mass transfer (Tan et al., 2020). The cell growth in the center is restricted due to lower photosynthesis in photobioreactor inhibiting concentration due to oxygen toxicity that can occur after only 1 minute in a tube without gas exchange (Posten, 2009). The uncontrolled growth of pathogenic microorganisms in the inner walls and the formation of biofilms, which influence the mass transfer of reagents due to external mass transfer resistance at the biofilm surface, is another drawback of the closed system (Skoneczny and Tabiś, 2015). These day’s plastic bag photobioreactors are in great demand, and they can be separated based on their volumes and are cost-effective and made up of polythene (Wang et al., 2012). The only limitation is that mixing the components and fragility of the bags can lead to a decrease in this system’s life. The major disadvantages of a closed system are high operating and construction costs.
Integrated Systems
Integrated systems are a complex mix of open and close culture systems in an advanced way to develop a hybrid system that overcomes their limitations collectively and produces an improved system with high pollutant removal efficiency and elevated biomass yield.
Immobilized Bioreactor
The immobilized system circumvents some major challenges in microalgae wastewater treatment processes. It eases some high-cost and energy-intensive steps of suspension systems, i.e., harvesting and separating algal biomass from the treated wastewater. Immobilization technology renders a fixed system where living cells are grown in a confined area and are prevented from moving independently in the system’s aqueous environment. There are six different immobilization techniques namely covalent coupling, affinity immobilization, adsorption, liquid-liquid emulsion, semipermeable membrane capture, and entrapment (Mallick, 2002). Among all, entrapment is the most exclusively used method for algae wastewater treatment and nutrient recovery. Algae cells are entrapped in a 3D gel matrix molded in the form of biocatalyst beads which are stabilized by cross-linking. For instance, widely used alginate and carrageenan beads in the case of algae are usually stabilized by Ca2+ and K+ respectively (Ting et al., 2017). This technology utilizes algae’s natural tendency to adhere to the natural or artificial substrate. The matrix consists of porous beads made of a polymeric material. This polymeric substrate must be hydrophilic to allow diffusion of wastewater into the bed and can be fabricated by various materials like synthetic acrylamide, polyvinyl, polyurethane, or naturally derived algal polysaccharides like alginate, agarose, and carrageenan. Chitosan also serves as an effective bead material individually and its durability gets enhanced when amalgamated with alginate (Castro and Ballesteros, 2020). A recent study reported faster growth rates and higher biomass production with successful nutrient removal capacity in C. vulgaris when grown in the alginate-chitosan matrix (Castro and Ballesteros, 2020). Such porous bead structures provide a protected environment for the selected species to thrive in a wastewater setting. Few studies used submerged Ca-alginate beads for the growth of microalgae-bacteria consortium in normal wastewater and revealed minimal contamination from other species and uninterrupted reduction in phosphorus and ammonium levels (Covarrubias et al., 2012; Kube et al., 2021). Apart from the material, cell density and gel thickness of the beads are also decisive characters for good results. If these are higher, it will lead to a light shading effect, and if lower, it renders low nutrient absorption (Ting et al., 2017). Few microalgae-based immobilized studies for wastewater treatment are listed in Table 3.
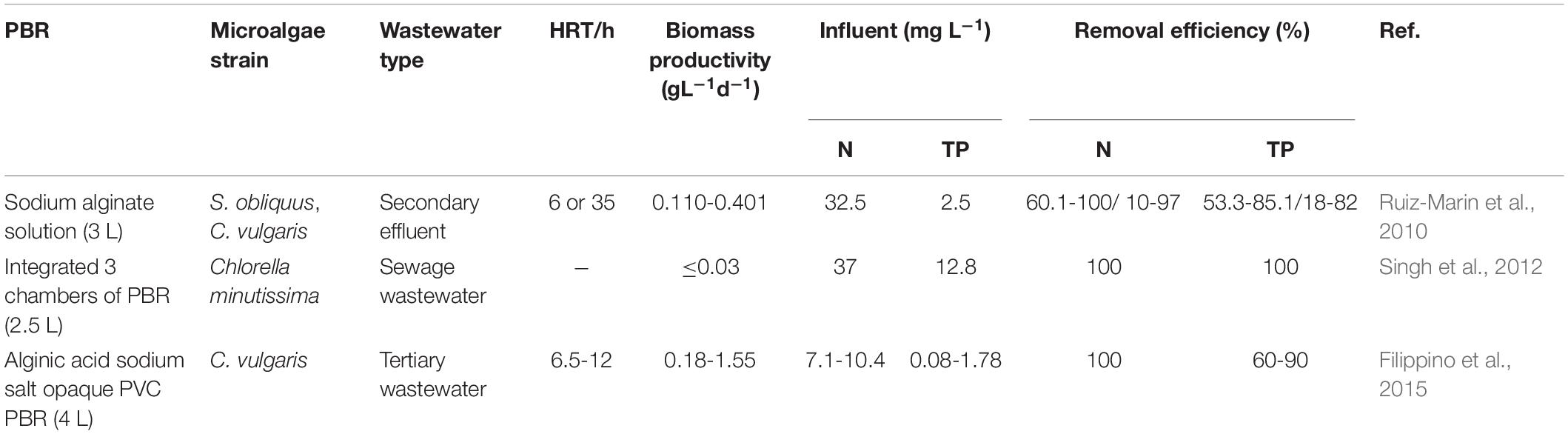
Table 3. Microalgae-based immobilized bead systems for wastewater treatment (Ting et al., 2017).
An ideal immobilized algal system should retain the viability of cells, shouldn’t interfere with photosynthetic ability, render low leakage of cells from the matrix and exhibit a high density of cells (Mallick, 2002). However, such systems have different metabolic effects on algal cultures. Only the surface algae can get optimum conditions (light intensity, nutrient availability) to grow while the microalgae inside the bead get deprived and show less activity due to the self-shading effect. Results are chiefly dependent on a higher surface to volume ratio to ensure maximum interaction between pollutants and microalgae surface (Wollmann et al., 2019). The current study on Scenedesmus obliquus showed 96.6 ± 0.1% removal in 50 mg/L NH4+-N and 300 mg L–1 COD by the immobilized system over cell-free mode in an artificial wastewater environment (Liu et al., 2019). Another study revealed 94% and 66% removal efficiency of NH4+-N and PO43-P, respectively, for agar-immobilized microalgae even after eight times recycling. This concludes that immobilized microalgae can be preserved for about 120 days and can be used repeatedly at room temperature (Hu et al., 2020).
There are five types of immobilized bioreactors for fixed microalgae-based sewage treatment, namely fluidized bed bioreactor, airlift bioreactor, parallel plate bioreactor, packed bed bioreactor, and hollow-fiber bioreactor briefly explained in Figure 1. Fluidization enables increased mass transfer, large surface area, finest mixing, homogenous temperature, and particle distribution (Mohd-Sahib et al., 2017; Nelson et al., 2017). There are two types of advanced fluidization: LS (liquid-solid) and GLS (gas-liquid-solid) fluidization. Both of them can operate on circulating fluidization in which particles are carried out to the top of the column and then returned to the bottom with high velocity via recycle line. In GLS, three phases are involved as both liquid and gas fluidizes the particles. An investigation on novel microalgae membrane bioreactor with internal circulating fluidized bed (ICFB) showed 52, 80, and 85% removal efficiency of NON, PO43–P, and NH4+–N in 40 days (Ding et al., 2020). A study modeled CO2 bio fixation using fluidized bed bioreactor onto polyurethane foam in nutrient rich wastewater setting and observed 97.7% (by wt. of lipid) of fatty acid methyl esters (FAMEs) mixture and found a high content of C16 and C18 FAME species for efficient combustion of biodiesel (Rosli et al., 2019). It was found that the wastewater removal efficiency also depends upon the flow pattern of the reactor besides cell density and gel thickness (Ting et al., 2017). Fluidized bed bioreactor showed better COD removal performance than parallel plate bioreactor rendered by a more extensive contact and continuous nutrient supply area (Ting et al., 2017). This system also possesses few limitations like difficulty in the evaluation of microalgae to count during treatment as it is packed in a matrix. Such constraints can be overcome by taking into account various other factors that influence the algal yield. Therefore, there is a need to broaden the understanding of immobilization on microalgae physiology and fill these lacunae of knowledge with further research.
Biofilm Packed Bed Bioreactor
Biofilm is a consortium of microorganisms embedded in a self-secreted extracellular polymeric substance (EPS) attached to a substratum. It can be an axenic (only consist of microalgae) or non-axenic systems (microalgae-bacteria consortium). Most of the systems are based on co-cultures of bacteria and microalgae as they share a stable symbiotic relationship in wastewater streams by maintaining a carbon dioxide-oxygen balance. Bacteria can feed on nutrients available in wastewater and also consumes oxygen to reduce the BOD while algae utilize CO2 released by bacteria and supplement the system with O2. This will make the whole system self-reliant in themselves (Boelee et al., 2014; Lee et al., 2014). Such biofilm reactor renders a tremendous advantage over the conventional suspended systems as they require a relatively minimal amount of water, i.e., for 1 ton of microalgae biomass, 17 tons of water is sufficient while for cell-free culture, 200 tons of water is needed (Podola et al., 2017). Moreover, any rough surface material can be used as a supporting material like nylon, stainless steel, and natural fibers, etc. (Garbowski et al., 2017). Rougher the surface, more easily microalgae can adhere to it. According to a study, nylon and stainless-steel mesh are the most effective supporting material for biofilm having Chlorella, Pediastrum, Scenedesmus, Nitzschia, and Cosmarium (Lee et al., 2014). The significant bottlenecks of suspended cultures like harvesting, separation, and dewatering are not required in biofilm-based packed bed reactor as microalgae can be easily removed by scraping the solid surface (Lee et al., 2014). Microalgae attached to the surface experience better light availability than in the suspended environment. Likewise, a study showed that 1.4-fold more biomass production and higher pollutant removal efficiency in C. vulgaris biofilm packed bed reactor comparing to the suspended bioreactor (Cheah et al., 2018). Many factors affect microalgal biofilms, such as selecting proper microalgal strain, nutrients, light, and carbon dioxide availability, temperature, pH, attaching material, flow velocity, and allelopathic microalgae interactions with other microorganisms in the case of consortium cultures (Mantzorou and Ververidis, 2019). Biofilm show high heavy metal removal efficiency as in the case of Chlorella with 10.55%, 24.8%, 26.4%, and 24.8% removal rate for Co, Mn, Sr, and Ni (Palma et al., 2017). This is due to the presence of a high number of functional groups for metal complexation in EPS.
Biofilm systems are majorly divided into three categories based on biofilm arrangement and liquid medium supply: (i) permanently submerged systems, i.e., those systems that are constantly submerged in the liquid culture medium, (ii) intermittently submerged systems, i.e., those systems that have repeated alternative exposures of liquid as well as gas phase (also called biofilms between two phases), and (iii) perfused systems, i.e., those systems in which substratum is directly provided with culture medium (Moreno Osorio et al., 2019). Various microalgae-based biofilm bioreactors are developed to integrate wastewater treatment with biofuel productions to obtain a high yield with high efficiencies than conventional techniques as depicted in Table 4. Different packed bed bioreactors like vertical, horizontal, parallel plate, flow cell, airlift, flexi-fiber, rotating biofilm reactors, and vertical-algal-biofilm enhanced raceway ponds are studied for reclamation potentials widely. However, a rotating biofilm packed bed bioreactor is more promising, with 31 gm–2d–1 growth in mixed culture conditions utilizing the municipal wastewater (Christenson and Sims, 2012). Furthermore, a recent study has also observed a formation of struvite within the microalgae biofilm matrix in an outdoor pilot-scale rotating algal biofilm reactor (Hillman and Sims, 2020). These systems are much more advanced than conventional packed bed reactors, but this technology is still primarily limited to laboratory conditions and needs to be further optimized in high pollutant loads to upscale it to industrial levels.
Factors Affecting Microalgal Growth
Microalgae cultivation requires various environmental conditions and biotic components such as temperature, light, pH, and nutrients for their optimum growth. The cultures require various nutrients, trace elements, vitamins, and growth regulators to grow. Redfield ratio suggested that the cultivation media could be flexible and adapted to microalgal metabolic needs and specific environmental conditions (Arrigo, 2005). The initial density directly affects the algal growth, i.e., higher algal density leads to better growth and ultimately results in higher efficiency for nutrient removal. The effect of various factors is explained below.
Temperature
Temperature is an important factor controlling microalgal growth and various metabolic activities, photosynthesis, and carbon fixation. The temperature of the pond varies on both seasonal and diurnal scales which affect the algal photosynthesis and respiration rates. The greater microalgal productivity was produced when the water temperature was controlled constantly compared to uncontrolled temperatures in high rate algal pond systems (Slegers et al., 2013). In a recent study, it was found that the higher the temperature, the lower the Rubisco’s affinity for carbon dioxide and eventually lower the carbon dioxide solubility in the media (Taziki et al., 2015). Henceforth, many species proliferate at the optimum temperature of 20-25°C as above 35°C; their growth becomes lethal due to increased photorespiration. Conversely, thermophiles can be utilized to serve the purpose and to reduce the cooling cost. Algae can be classified based on the temperature ranges as psychrophiles, mesophiles, and thermophiles. For instance, thermophilic microalgae Chlorogleopsis sp. can grow at a maximum temperature of 50°C (Morales et al., 2018). It experimented that different microalgae cultured at different temperatures showed a decrease in the growth rate, biomass, and lipid accumulation with an increase in temperature (Chaisutyakorn et al., 2018). Another study reported high carbon dioxide fixation and lipid accumulation up to 50% at 10°C in low nutrient availability in psychrotolerant species Acutodesmus obliquus (Lee et al., 2019). Galdieria sulphuraria show thermophilic growth behavior up to 56°C (Selvaratnam et al., 2014) while Chlorella sorokiniana has high photoautotrophic growth rates up to 43°C (Varshney et al., 2018). Hence, the growth rate of algae can be determined by the high rate of photosynthesis, which depends on light intensity and temperature (Béchet et al., 2013).
Light
Light plays an important role in the growth of microalgae. In a study, the relationship between light intensity, photoperiod, and production of biomass of microalgae Ankistrodesmus falcatus has been studied. The highest biomass (224.64 mg L–1) and biomass productivity (9.30 mg L–1 D–1) was observed under a light intensity of 150 μmol m–2 s–1, whereas the lowest biomass (183.48 mg L−1) and biomass productivity (7.58 mg L–1 D–1) was observed under the light intensity of 30 μmol m–2 s–1 (George et al., 2014). It was also reported that continuous light and 18 h dark cultures yielded lower biomass productivity. According to the literature, biomass production in many microalgae increased under high light conditions which generally causes an increase in reproduction until the saturation point intensity (Danesi et al., 2004). The wastewater quality directly affects the availability of light present in the culture medium. When at the beginning of the growth cycle, the light reaches a higher number of cells as the cell densities are low whereas at a higher density, the cells suffer from self-shading and sometimes the dissolved organic matters decrease the light availability to the culture for its growth (Novoveská et al., 2016). In a study, it was reported that three different types of lights red, blue, and white were studied on the algal species derived from the wastewater and the light spectrum influenced the cell size (Izadpanah et al., 2018). Light intensity directly affects the microalgal growth and the growth rate is directly proportional to Photon Flux density, but at the point of light saturation, the photon flux density is too high, which can lead to photoinhibition and photo-oxidation and ultimately the death of the cells (Bartosh and Banks, 2007). In a study, it was reported that the 19.4% of lipid content was increased when the amount of light intensity of 2700 Lux is provided to the culture (Rai et al., 2015). Microalgae can utilize the energy available in the wavelength range of 400–700 nm, which is referred to as photosynthetically active radiation (PAR) (Boyle, 2004). The optimum light intensity should be standardized for each species for maximum carbon dioxide assimilation and minimum photorespiration and photoinhibition. Scenedesmus almeriensis requires a light intensity of 100 μmol m–2 s–1 irradiance (Costache et al., 2013) whereas Scenedesmus obtusiusculus shows optimum growth at irradiation of 300 μmol m–2 s–1 irradiance (Cabello et al., 2015). The phenomenon of photoinhibition can be minimized by the usage of Light Emitting Diode lights instead of fluorescent tubes due to high electrical energy conversion efficiency, sufficient light intensity, and small volume and weight characteristics, and also decreased proton loss (Lee and Palsson, 1994). The optimum light intensity should be supplied to both sides of culture, i.e., material and watersides. The antioxidants present in microalgae can help in dealing with an excess of light and its effects.
Nutrients
The essential nutrients required for the optimum growth of algae include carbon, nitrogen, phosphorous, vitamins, and trace elements. The nutrient removal from wastewater by microalgae reduces the environmental impact of its discharge. Carbon is the essential nutrient for growth and is utilized by microalgae by the Calvin cycle. According to heterotrophic or mix-trophic metabolism, many species can grow on an organic substrate. The main organic compounds are monosaccharides, volatile fatty acids, glycerol, and urea utilized for growth. Organic carbon uptake into the cells occurs through diffusion, active transportation, and phosphorylation. A high concentration of carbon dioxide is required for the growth of microalgae C. vulgaris. The carbon dioxide fixation rate of C. vulgaris is 252 mg L–1D–1 and 86.7% of biomass was produced as shown in the investigation report (Bittencourt, 2009). C. vulgaris can uptake nitrogen, carbon dioxide, or phosphorous for its optimum growth (Gilles et al., 2008).
Nitrogen is required for the formation of genetic material, i.e., deoxyribonucleic acid and ribonucleic acid. It can be utilized in the form of nitrates, nitrites, urea, and ammonia. Removal of NH4+ is especially important as it is the more toxic form of nitrogen in wastewater. An increase in nitrogen available in the wastewater results in more uptake of nitrogen which leads to the production of more vital components for cellular activity further contributing to a greater amount of nitrogen being transported and assimilated by the cells (Kube et al., 2018). It is observed that C. vulgaris efficiently utilized and removed the high concentration of both nitrate and nitrite from the culture medium and simultaneously produces algal biomass for several wastewater treatments (Taziki et al., 2015). The other nutrients required are phosphorous, magnesium, iron, and calcium in trace amounts. Phosphorous is also utilized by microalgae for metabolic processes and several components such as nucleic acids and phospholipids. The uptake mechanisms as well as the use of phosphorous in algal cells depend on its concentration present in the wastewater (Solovchenko et al., 2016). The carbon assimilation is influenced by the phosphorous content present in the wastewater (Hu and Zhou, 2010), and wastewater carbon content has an impact on both nitrogen and phosphorous removal. The dissolved carbon dioxide is the most usable form of inorganic carbon for algae which leads to the maximum yield of algal biomass. For the nutrient removal and improvising algal growth, carbon dioxide is supplied to overcome low concentration of carbon in wastewater and reduces greenhouse gas emission by capturing carbon dioxide (Razzak et al., 2013). The pH also plays an important role in the optimum growth of algae. The maximum growth can be observed at optimum pH ranges from 7.9 to 8.3 (marine water), and 6.0 to 8.0 (freshwater). Most microalgae species are pH sensitive, and few of them can reach the range tolerated by C. vulgaris (Raize et al., 2004). In a closed system, the pH can increase up to pH 10. This rising pH value can be controlled by the amount of carbon dioxide or using inorganic or organic acids (Lam and Lee, 2012). Combustion of flue gas with high carbon dioxide concentrations can decrease the pH to 5, but, at higher acidic pH, the photosynthetic growth is limited. The pH value is affected by a decrease in metabolites and the release of several organic acids. The excess of OH reacts with CO2 to form HCO3–, resulting in a higher bicarbonate and carbonate alkalinity and higher total carbon availability. In wastewater treatment using algae, the pH rapidly increases and then remains at a relatively constant value (Kube et al., 2018). If wastewater is rich in NH4, a higher pH with less CO2 addition may promote volatilization, and if wastewater has a higher NO3– concentration a lower pH with more CO2 addition may encourage growth and improve nitrogen assimilation (Sutherland et al., 2015). High pH stress inhibits the cell cycle and triggers lipid accumulation (Vuppaladadiyam et al., 2018). Nutrients play an important role in the growth and productivity of microalgae such as iron, calcium, cobalt, manganese, zinc, molybdenum are vital for the growth of microalgae Dunaliella tertiolecta. The production of lipid in D. tertiolecta is increased when the culture was starved for nitrogen. For the production of oils and biofuels, it is economical to starve the culture for nitrogen content and will shorten production cycles and the waste content produced (Chen et al., 2011). Micronutrients such as iron, manganese are required in small amounts (2.5–30 ppm) and trace elements, such as cobalt, zinc, and molybdenum, are essential in very low concentrations (2.5–4.5 ppm) for efficient growth of microalgae. Micronutrients play an important role in algal metabolic functions such as coenzymes or energy carriers (Juneja et al., 2013). The high concentration of cobalt was previously considered toxic for algal species, but a controlled level of cobalt provided optimal lipid accumulation in T. suecica (Ghafari et al., 2016). The cobalt is mainly required for the synthesis of vitamin B12 (Li et al., 2007). Molybdenum in low concentration is optimal for Bacillus sudeticus, with 8% increase in lipid content; however, a high concentration showed improved lipid contents in C. vulgaris and T. suecica by 20% and 13% increases, respectively (Ghafari et al., 2016). Molybdenum is required for the assimilation of nitrates (Raven, 1988). Hence, it can be concluded that both macro and micronutrients are important for algal growth.
Harvesting of Algae
The production of microalgae biomass during wastewater treatment is considered as economic viability for algae-based product production. Microalgae-based wastewater treatment technology faces many challenges and microalgae harvesting is one of the major constraints due to the substantial cost and energy involved in these steps. Methods like centrifugation, flocculation, sedimentation, filtration, etc. have been reported to harvest biomass.
Chemical Flocculation
Flocculation is the process where microalgae are caused to clump together into a floc using various chemicals such as alum, or ferric chloride, etc. The critical factors of this process are cellular concentration, surface properties, net charge, and hydrophobicity, the concentration of the coagulant/flocculent species, pH, and ionic strength of the broth process (Papazi et al., 2010). The coagulation involves pH adjustment or electrolyte addition. The various biomass of microalgae recovered by flocculation includes C. vulgaris using Nano-aminoclays (Mg-APTES) as a coagulant (Farooq et al., 2013) whereas Chlorella minutissima is harvested by use of Fe2(SO4)3 as a coagulant (Papazi et al., 2010). C. vulgaris by auto flocculation can produce a recovery rate of 98% by using CaOH2. (Vandamme et al., 2012). In a recent study, the effect of pH variations and addition of flocculant such as calcium chloride on the harvesting of microalgae Arthrospira maxima was studied and observed that pH > 10 and CaCl2 0.2–2.0 gL–1 at a 1:30 ratio (v/v) of CaCl2/microalgae culture could be applied to harvest biomass efficiently (Caetano et al., 2020).
There are different ways to induce flocculation namely - (i) electrostatic patch, (ii) bridging, and (iii) sweep flocculation (Vandamme et al., 2013). Chemical coagulation/flocculation should - (i) result in no biomass contamination; (ii) lead to subsequent high-efficiency biomass settling; (iii) allow the reuse of the culture medium; (iv) environmental impact; and (v) cost-effective and non-toxic when applied in large scale (Molina Grima et al., 2003). The more electronegative the ion, the faster the coagulation (under 24 h) (Papazi et al., 2010). The negative charge is present on the surface of microalgae and The microalgae biomass can be contaminated by the used metals, declining its application in biofuel or animal feedstock. Coagulant addition (<10-fold) is required by the higher density cultures due to less charge on the surface of the cell wall leading to higher collision rates (Schlesinger et al., 2012). Chemical harvesting is an economical approach for the harvesting of microalgae from wastewater (Mohd Udaiyappan et al., 2017). The chemical flocculation method can be applied to large culture volumes without disrupting the cellular structure. The major disadvantage of this method is the presence of harmful chemicals that pose environmental risks.
Mechanical Methods
The most rapid and reliable method for harvesting algae is centrifugation and the algal biomass is separated based on density differences using centrifugal forces. A nozzle type disc centrifuges are suitable for all microalgae types as they are easily cleaned and sterilized, but the high investment and operating costs are their limitations. For harvesting filamentous algae, low-cost filtration methods are used. The strains grown in a high-rate algae pond system are selected, and filamentous algae are harvested by micro screening to retain larger cells, and smaller non-filamentous algae are washed away. For smaller suspended algae, tangential flow filtration is considered, but membrane fouling and replacement are not cost-effective (Uduman et al., 2010), and power requirements are high (Danquah et al., 2009). Use of membrane-based harvesting method has been reported for the recovery of Scenedesmus almeriensis biomass using polyvinylidene fluoride (PVDF) membrane which helps in separation by retaining biomass and growth media is passed through the membrane filter (Marino et al., 2019). Sedimentation is a low-cost harvesting method that can give concentrations of 1.5% solids (Danquah et al., 2009). Sedimentation is a slower process as settling rates of 0.1–2.6 cm h–1, and during the settling time, much of the biomass deteriorates (Greenwell et al., 2009). The various comparison of harvesting methods is depicted in Table 5, which explains their major benefits and limitations. In algae rich waters, dissolved air flotation is usually preferred over sedimentation methods (Teixeira and Rosa, 2006). Dissolved air flotation is a method commonly used in wastewater treatment sludge removal, i.e., for large scale setups but flocculants can cause a problem during downstream processing of algae (Greenwell et al., 2009). It was studied that pH-modulated dissolved air floatation harvesting method having optimized parameters such as pH, velocity gradient, mixing time, and floatation to harvest Chlorella sorokiniana from wastewater (de Leite et al., 2020). The major limitation of the mechanical method is the increased operational costs for algal production and is economically feasible only when a higher number of value-added products are produced (Park et al., 2011).

Table 5. Comparison of mechanical harvesting methods for algae (Adapted from Teixeira and Rosa, 2006; Greenwell et al., 2009; Uduman et al., 2010).
Biological Methods
Bio-flocculation is an environmentally friendly technique to harvest microalgae which involves cell aggregation using different microorganisms (bacteria, filamentous fungi) and autoflocculating microalgae. Use of microalgae-bacteria flocs in wastewater treatment results in increased microalgae recovery with a settling rate of 0.28-0.42 m h–1 (de Godos et al., 2014). The use of polymeric material produced by various microbes was also reported for the harvesting of microalgae. Ndikubwimana et al. (2016) studied pilot-scale bioflocculation of Desmodesmus brasiliensis using bioflocculant γ-PGA produced by Bacillus licheniformis CGMCC 2876 and reported flocculation efficiency >98%. Choi et al. (2020) evaluated the potential of activated sludge derived extracellular polymeric substance (ASD-EPS) for microalgae harvesting (C. vulgaris, Chlamydomonas asymmetrica, and Scenedesmus sp.) and C. vulgaris showed the highest bioflocculation efficiency. Microalgae flocculation is also achieved by using naturally available ions in brackish water, and a variety of precipitating ions, including Mg2+, Ca2+ can lead to auto flocculation of microalgae (Smith and Davis, 2012). A new process of pelletized cell cultivation was reported by Zhang et al., using coculture of filamentous fungal species with microalgae. Microalgae cells co-pelletized into fungal pellets and due to their large size, these can be easily harvested through a sieve (Zhang and Hu, 2012). For microalgae harvesting and wastewater treatment, microalgal bacterial flocs is a promising technology. In a study, it was reported that bioflocs formation enhances harvesting of C. vulgaris and removal of nutrients from seafood wastewater effluent (Nguyen et al., 2019b).
Reviewing all the reported harvesting techniques and emerging studies it seems none of the technology seems economically and ecologically viable at a large scale. Therefore, the development of a cost-effective, efficient, and eco-friendly harvesting process will continue to be a hot area of research.
Biofuel Production
In the coming decades, the world will require a vast amount of energy to support population and economic growth and to improve living standards. To protect the environment and meet increasing energy demand, renewable sources of energy need to be adopted (Bhatia et al., 2019b; Patel et al., 2020a, b, c). Due to rapid industrialization and subsequent improper waste handling, water pollution is increasing daily, especially in the Indian major cities, which has an incredibly harmful impact on human health and the environment (Bhatia R. K. et al., 2020). A biofuel is a fuel produced through contemporary biological processes such as dark-photo-fermentation, anaerobic digestion, and methanotrophs-based biotransformation of greenhouse gasses, etc. rather than geological processes involved in forming fossil fuels (Bindra et al., 2017; Patel et al., 2020d, e, f; Kumar et al., 2021). Microalgal biomass can be utilized to produce various types of biofuels including biodiesel, bioelectricity, biohydrogen, etc.
Biodiesel
Biodiesels are fatty acid methyl esters produced through the transesterification of oils with alcohols in the presence of catalysts (Bhatia S. K. et al., 2020). Microalgae can accumulate lipids that can be used as feedstock for biodiesel production (Anwar et al., 2017; Otari et al., 2020). The main steps in biodiesel production from microalgal biomass involve microalgae cultivation, drying of algal biomass, extraction of oil from algal biomass, and transesterification to fatty acid methyl esters (Bindra et al., 2017). Various methods are used to extract lipids from micro-algae such as mechanical extraction, solvent extraction, ultrasonic extraction, supercritical extraction, and enzymatic extraction. Transesterification is the process of conversion of oil to biodiesel (Demirbas, 2007). It involves reactions between triglycerides or fatty acids and alcohol in the presence of catalysts. Different alcohols such as methanol, ethanol, propanol, butanol, and amyl alcohols can be used in transesterification. However, methanol and ethanol are more commonly used in commercialized processes due to their low cost and physical and chemical advantage (Bhatia et al., 2015b; Patel et al., 2019). Various homogenous (acid or base) or heterogeneous catalysts (metallic nanoparticles) or enzymes (lipase) are used to carry transesterification reactions (Kumar et al., 2019; Otari et al., 2019).
Various methods of transesterification (supercritical, BIOX cosolvent) and bioreactor designs. (microtubular microreactors, membrane microreactors, microchannel reactors, microwave reactors, reactive distillation, and centrifugal contractor) have been reported for the conversion of oils into biodiesel (Bhatia et al., 2021a). In a recent study, it was found that microalgae Chlamydomonas reinhardtii produces 505 mg L–1 of biofuel from municipal waste (Kong et al., 2010). In a study, it was reported that in an effluent wastewater treatment plant under a continuous supply of carbon dioxide, C. vulgaris removed a higher concentration of phosphorous (Liu et al., 2020). Nanoparticles incorporation with microalgae is a new promising technology used for the high yield of biodiesel formation. According to the previous studies, a minimal amount of colloidal hydrous iron (III) oxide particles boosted almost 100% microalgae cell suspension; magnetic particles incorporated with aluminum sulfate were beneficial for cell separation from the mixed culture of Anabaena and Aphanizomenon microalgae species (Pattarkine and Pattarkine, 2012). The silver nano-particles applied on Chlamydomonas reinhardtii, and Cyanothece 51142 microalgae harvesting increased 30% higher biomass productivity, whereas calcium-oxide nano-particles increased the biodiesel conversion yield up to 91% via catalytic transesterification (Torkamani et al., 2010; Safarik et al., 2016).
The glycerol is produced as a by-product and is denser than biodiesel, which is continuously removed to increase biodiesel yield or used as feed to produce biohydrogen (Bindra et al., 2017; Prakash et al., 2018). Solvents are separated from methyl ester by evaporation. The efficacy of the produced biodiesel depends on the content of free fatty acids and the composition of the oil. Properties of produced biodiesel depend on, carbon-chain length, branching, and degree of unsaturation which affects the physicochemical properties of the biodiesel (cetane number, oxidation stability, iodine value, cold flow properties, density, and kinematic viscosity) (Bhatia et al., 2021a). For the use of transesterification, the biomass-derived heterogeneous catalysts produce 95% biodiesel yield and are cost-effective and eco-friendly. After the transesterification, the separation of produced biodiesel from catalysts, excess of alcohol, glycerol, and the remaining oil are important to ensure higher performance, protection of the engine, and to maintain the quality standards (Bindra and Kulshrestha, 2019). The wastewater from the food and beverage industries is suitable for the growth of microalgae as it has a low concentration of toxic chemicals and heavy metals (Bhatia S. K. et al., 2020). In a study, it was found that livestock waste contains a large amount of nitrogen and phosphorous which leads to an increase in the carbohydrate content of biomass and a decrease in lipids (Choudhary et al., 2020).
Biohydrogen
Hydrogen is the clean and more reliable energy alternative for non-renewable fossil fuels due to the high calorific value of ∼122 kJ g−1 and water molecule produced as end byproduct of their combustion (Patel et al., 2012, 2015; Bhatia et al., 2021b). Biohydrogen production from third-generation feedstock (microalgae) is the most sustainable and biorefinery approach towards energy crises and can be further improved using metabolic engineering (Kumar et al., 2020). Microalgal biomass harvested from wastewater treatment can produce biohydrogen via different methods like direct and indirect photolysis of water and dark fermentation, yielding various volatile fatty acids along with hydrogen production (Mishra et al., 2019; Rajesh Banu et al., 2021). Many factors affect the hydrogen production rate, namely, carbon and nitrogen sources and their relative ratios, pH, temperature, light/dark cycles, microalgal strain, culture set-up and, pre-treatment employed (Prabakar et al., 2018). Many microalgae species like Chlorella, Scenedesmus, and Saccharina are extensively used for biohydrogen production (Wang and Yin, 2018). Microalgae Scenedesmus obliquus showed 56.8 mL H2 g−1vs yield in urban wastewater (Batista et al., 2015). Another study revealed high biohydrogen production (204 mL H2 L–day–) in the immobilized culture of Scenedesmus obliquus under manipulated light conditions at 140 μE m–2s–1 of light intensity, 30°C and 7.5 pH with sulfur deprivation. Blue light elicits large algal biomass production in urban wastewater setup while purple light induces high biohydrogen yield (Ruiz-Marin et al., 2020). However, the low yield is a major challenge due to the oxygen sensitivity of enzyme, light, and CO2 fixation efficiency in the commercial setup. To deal with the O2-sensitivity issue, a study showed the significance of the addition of VFA (volatile fatty acid) in synthetic wastewater and pertaining to O2-deprivation. The study resulted in a significant increase in biohydrogen yield (65.4 ± 0.3 μmol H2 L–1 mM–1 acetate) in C. vulgaris culture grown in PBR (Hwang et al., 2018). Recent studies focused on improving the economy of the process through genetic engineering and deploying low-cost photoreactors (Sharma and Arya, 2017; Sharma et al., 2018; Schiano et al., 2019).
Microalgae-Microbial Fuel Cell (MMFC)
The production of electrons to the metabolism of microorganisms produces bioelectricity (Gurav et al., 2019). The microbial fuel cell is used to produce electricity from the hydrolysis and fermentation of algae. Microorganisms in microbial fuel cell biodegrade organic compounds into carbon dioxide, water, and energy through various metabolic pathways (Kondaveeti et al., 2019a; Bhatia et al., 2021c). The significant components of microbial fuel cells include an anode and cathode connected by a load. In the microorganism’s culture, the anode acts as a catalyst for decomposing the organic matter and produce electrons and protons (He and Angenent, 2006). In a microbial fuel cell, the production of electrons leads to carbon dioxide produced at the cathode which is utilized by microalgae for its growth and production of biomass. In MMFC, the microorganisms biodegrade the organic components into carbon dioxide, water, and energy by various metabolic cycles (Bhatia S. K. et al., 2020). Microbial fuel cells can simultaneously produce biofuel and bioelectricity and treat waste. The Figure 2 describes the production of bioelectricity in two-chambered microbial fuel cell using microalgae. Recent studies suggest that the C. vulgaris strain is efficient in producing bioelectricity as it contains more than 50% proteins (Becker, 2007). The microbial fuel cell chambers are constructed of glass, polycarbonate, and plexiglass (Rhoads et al., 2005). The anode is made up of materials such as carbon paper, graphite (Zhang et al., 2011), platinum and consists of organic substrates to be utilized for the production of electrons by microbes. The different types of microbial fuel cells are – (i) single-chamber MFC, (ii) two-chamber microbial fuel cell, and (iii) stacked microbial fuel cell (Kondaveeti et al., 2019a, b). A recent study reported 44,33 mWm–2 electricity production from tapioca wastewater via microbial fuel cell (Costa and Hadiyanto, 2018). In a recent study, it was reported that Chlamydomonas sp. TRC-1 produced for textile wastewater treatment produced biomass which further produced bioelectricity and produced power of 1.83 m–2 (Behl et al., 2020).
Other Value-Added Product Production
Microalgae are emerging to be one of the most promising long-term, sustainable sources of biomass and oils. Rapid growing and high lipid-content algae are selected for diverse applications in many sectors for the production of biofertilizers, biopolymers, and exopolysaccharides, etc.
Bioplastic
The increase in the production of plastic leads to environmental pollution (Bhatia et al., 2021e). The conventional plastic used is made up of petrol-based oils and cannot be disintegrated by microbes. Mostly these plastics are disposed of by using landfill, and incineration methods. In 2015, approximately 6300 Mt of plastic waste had been generated, around 9% of which had been recycled, 12% was incinerated, and 79% was accumulated in landfills or the natural environment and if the production and waste management trends continue at the same rate then roughly 12,000 Mt of plastic waste will be in landfills or in the natural environment by 2050 (Geyer et al., 2017). Incineration leads to the release of noxious harmful toxins into the environment (Zhang et al., 2003; Zeller et al., 2013). Therefore, bioplastic is the only alternative (Figure 3). It is a biodegradable material produced from biological sources like bacteria, microalgae, yeast, and transgenic plants (Park et al., 2020). Bio-derived plastics are of many types like polyhydroxyalkanoates, polylactic acid, starch, carbohydrate, and protein-based plastics (Bhatia et al., 2016). There are mainly three different methods for the production of bioplastics which include, (i) polymers extracted from biomass, (ii) polymers produced by microorganisms, (iii) polymers synthesized by bio-derivatives.
Various microalgae strains are cultivated for polyhydroxyalkanoates production and accumulation of polyhydroxyalkanoates occurs under specific culture conditions. Study have found that the microalgae Spirulina platensis produces 10% (dry cell weight) of polyhydroxyalkanoates under specific growth conditions of acetate and carbon dioxide in the medium (Laycock et al., 2013). The genetically engineered microalgal strains can be used in the future, by using clustered regularly interspaced short palindromic repeats clustered regularly interspaced short palindromic repeats and Cas9 (an RNA-guided cleaving enzyme) cloning systems for producing the increased amount of polyhydroxyalkanoates (Sternberg and Doudna, 2015). In a recent study, the microalgae were harvested from the effluent of wastewater treatment plant and created a liquid medium for the growth of recombinant E.coli for the production of polyhydroxybutyrate (Rahman et al., 2015). The pathway engineering in baker’s yeast Saccharomyces cerevisiae and then transferring the assembled pathway in the nucleus of C. reinhardtii (Noor-Mohammadi et al., 2014). Microcystis sp. produces 0.7 mg L–1 yield polyhydroxybutyrate product (Abdo and Ali, 2019).
Polyhydroxyalkanoates films are used to pack urea fertilizers and insecticides, which decomposes efficiently by the soil microbes used in the agricultural land (Singh et al., 2009; Aguilar and San Román, 2014). Polyhydroxyalkanoates is also used as scaffolds, bone plates, media for the slow release of drugs, and surgical sutures in the medical industry (Bhatia et al., 2019c). Polyhydroxyalkanoates have been used in bone renewal treatments and nerve injury due to their piezoelectric nature (Misra et al., 2006). A blending of polyhydroxyalkanoates with inorganic phases results in bioactive composites of higher properties that can be used in tissue engineering applications (Chen, 2009). Despite its imperative applications, there are few constraints like slow growth of algae, costly harvesting, extraction, and downstream processing steps which took a great toll on the global market demands of bioplastics. Due to this, many scientists are moving towards genetic engineering tools to circumvent the price barrier.
Biofertilizer
Biofertilizers are composite substances with live or latent microorganisms that colonize the rhizosphere of plants, fix atmospheric nitrogen, aid in nutrient availability of plants, phosphate solubilization, and harness the soil’s fertility for healthy crop production. These natural composites can rejuvenate the nutrient balance in soil deteriorated by overusing harmful chemical fertilizers, pesticides, and weedicides and catalyzes the decomposition kinetics of organic matter present in the soil. Moreover, algae are widely utilized for soil conditioning and integrated plant nutrition systems since they can recover excess nutrients from wastewater during cultivation and reclaim water for other uses (Han et al., 2019). The production of biofertilizers by microalgae when wastewater acts as a substrate for optimum growth and production is depicted in Figure 4. Among the various types of algal species studied, cyanobacteria are the simplest and one of the most efficient because it has a high water-holding capacity, ability to fix atmospheric nitrogen, short generation time, and ability to withstand extreme environments (Singh et al., 2016). According to the study on N15-labeled fertilizer, cyanobacteria assimilate more nitrogen than chemical fertilizer and are more reliable for rice plant cultivation (Fernández Valiente et al., 2000). Another exciting research on immobilized Chlorella pyrenoidosa on dairy waste effluent as a biofertilizer gives a 30% surge in paddy root and shoots length (Yadavalli and Heggers, 2013). A study also found a yield of 650 mg L–1 of Chlorella sp. biomass growing in municipal waste as a biofertilizer (Das et al., 2019).
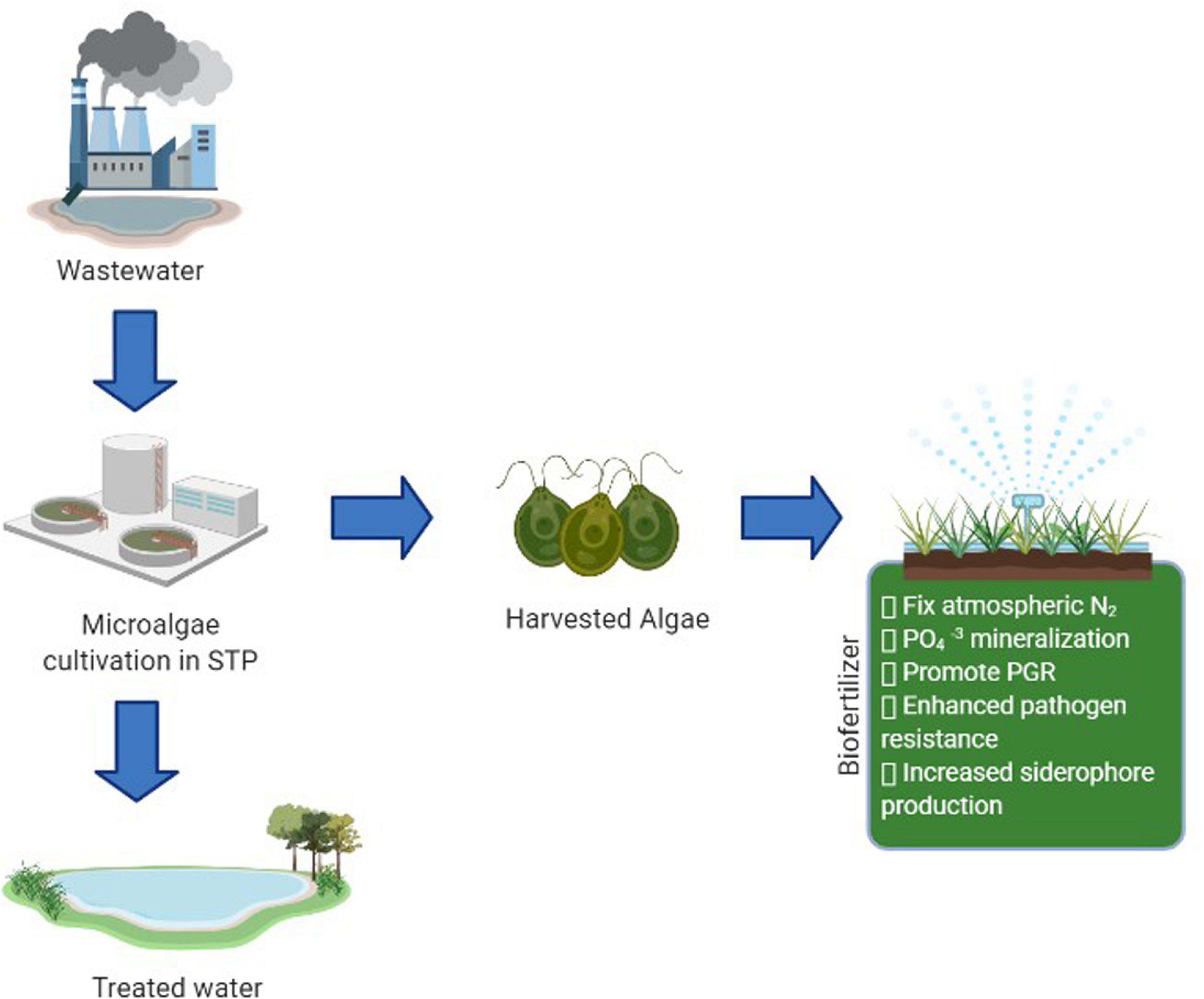
Figure 4. Schematic representation of reclamation of wastewater into a biofertilizer using microalgae (Created with BioRender.com).
Microalgae are also found to stimulate plant growth by producing essential plant growth promoters with plant-microbe symbiosis. Many studies are performed to validate the presence of extracellular products with growth regulators like activity in plants that aid in early germination. One such study on Lupinus termis grown in a consortium of cyanobacteria and bacteria revealed plant growth stimulating substances and showed improved germination compared to seeds untreated or treated with hormones like indole acetic acid, gibberellic acid, and cytokines (Win et al., 2018). Algae stimulate the production of siderophore, i.e., high-affinity Fe-chelating compounds secreted by bacteria by producing organic acids that photolyzes these siderophores and thereby triggers iron assimilation in very low iron and nitrogen conditions chiefly in the waterlogged or aquatic environment. A recent study valorized the freshwater microalgae Navicula pelliculosa induced siderophore biosynthesis in the bacterium Cupriavidus necator H16 (Kurth et al., 2019). Another experiment that cultivated microalgae in black water (toilet wastewater) concluded that microalgae biofertilizer works similar to inorganic fertilizer but emits more N2O and CO2 due to nitrification pathways. These increased emissions could be non-sustainable and need to be maintained (Suleiman et al., 2020). Despite the surfeit of advantages of microalgae-based biofertilizers, some bottlenecks of this technology hinder mass production. The primary constraints include the short shelf-life of cyanobacteria and the possible dangers of toxification of algae due to the outpouring of chemical pesticides, herbicides, and weedicides in cropland press a severe concern (Renuka et al., 2018). Shelf-life can be augmented to a certain extent by selecting translucent packing material, dry mixing, etc. (Jha and Prasad, 2006). Apart from this, light and temperature sensitivity also hurt a viable population count in storage conditions. Therefore, further research should be encouraged to prevail over these limitations and promote the integrated carbon-neutral, zero-waste biorefinery approach.
Exopolysaccharides
Industries have currently shifted their focus from polysaccharide inside the cell to exopolysaccharide, loosely attached to the cell wall surface (cell-bound exopolysaccharides) like sheaths, slime, and capsules or is secreted outside the cell to build a matrix as protection (Bhatia et al., 2015a; Sathiyanarayanan et al., 2015). It has gained much attention due to its facile extraction and isolation process from the media; it will prove to be an economical alternative and also saves a lot of time and energy. Exopolysaccharides are high-value compounds obtained from algal secretions with remarkable therapeutic properties associated with the compound’s conformations and molecular weight (Sathiyanarayanan et al., 2016). They are known to possess immunomodulatory, anticoagulant, antimutagenic, antibacterial, radioprotective, anticancer, antiulcer, and anti-inflammatory bioactivities (Bhatia et al., 2021b). Cyanobacteria and red algae are the chief producers of exopolysaccharides mucilages mainly to adapt to stress conditions like physio-chemical changes in the environment. The composition of exopolysaccharides varies from species to species and is conspicuously dependent upon culture conditions. Golgi apparatus and cytoplasm are the leading site for the formation of these exopolysaccharide molecules in microalgae and cyanobacteria, respectively. Exopolysaccharides prime sugar component includes glucose, fructose, galactose, xylose, arabinose, rhamnose, mannose, and fucose. Salt stress in halotolerant microalgae D. salina induces the release of a complex mixture of polyelectrolytes and polysaccharide content as a survival strategy (Mishra and Jha, 2009). Apart from the concentration of nitrogen, phosphorus, sulfates, temperature, pH, and intensity of the light also significantly impact the yield. In a study on microalgae Botryococcus braunii strain CCALA 220 (race A), a yield of 2 mg L–1 was obtained at optimum conditions of 950 μmol m–2 s–1 of light intensity and 6 mM of nitrate (Cepák and Přibyl, 2018). Another study gives a yield of 140 mg L–1 of EPS with Phaeodactylum tricornutum grown in palm oil mill effluent (Nur et al., 2019).
Other rationales include cell-to-cell interactions, biofilm formation, and adhesion mainly because of polyanionic and polycationic compounds like N-acetylglucosamine and xanthan that impart these properties (Bhunia et al., 2018). Exopolysaccharides are usually negatively charged, i.e., exhibit anionic properties of the biopolymer. Indeed, it can immobilize positively charged metal ions with positive charges in the effluent from wastewater due to uronic acid and sulfate’s presence on the surface (Freire-Nordi et al., 2005). The exopolysaccharides of Chlorella stigmatophora and Anabaena spiroides exhibit the metal-complexing capacity against Zn2+ and Cd2+ and Cu2+, Pb2+, and Hg2+, respectively (Freire-Nordi et al., 2005; Khangembam and Tiwari, 2016). This natural metal chelating property is very beneficial in biological water purification and to maintain ecological balance. Besides, the recent study has highlighted exopolysaccharides potentials as bio flocculant in freshwater Cyanothece sp. for the bioremediation of nano and microplastics from the waste streams and as a potential replacement of synthetic flocculants (Cunha et al., 2020). Many biochemical and rheological studies on exopolysaccharides can be expanded for mechanical and food engineering applications as drag-reducers in ships and thickening and gelling agents to increase food viscosity (Xiao and Zheng, 2016). A brief discussion about the major components of exopolysaccharides and their applications are depicted in Table 6.
Polysaccharides from Gyrodinium impudicum KG03 render excellent antiviral activity against encephalomyocarditis virus (Yim et al., 2003). Furthermore, strains like Nostoc flagelliforme, Porphyridium cruentum, and Aphanothece sacrum exhibit antibacterial and antiviral properties against Salmonella enteritidis and several viral species like Vaccinia virus, and African swine fever virus, etc. (Kanekiyo et al., 2005; Ngatu et al., 2012). Rhodella reticulata, Chlamydomonas reinhardtii, and Arthrospira platensis show impressive antioxidant and free radical scavenging pharmaceutical activities in a dose-dependent manner (Chen et al., 2010; Bafana, 2013; Pierucci et al., 2017). Moreover, S. platensis inhibits tumor invasion and metastasis by releasing sulfated polysaccharide calcium spinilan (El Baky et al., 2013). Microalgae-derived exopolysaccharides have enormous applications in biotechnological, food, pharmaceutical, and most importantly, in industrial wastewater treatment. This field is still in its infancy, and further studies and research are inevitable to disclose the understanding of complex structural and functional diversity and optimize exopolysaccharides yield with minimal cost further. Co-culturing and heterogeneity give good results with interspecies interactions and upsurge in exopolysaccharide production (Angelis et al., 2012). More research is required to scale-up this microalgae technology to exploit more lucrative resources supporting the biorefinery concept.
Techno-Economic Analysis and Global Status
Microalgae are utilized for wastewater treatment in many different ways all over the globe. New technologies are emerging with more efficient and feasible approaches to scale up from laboratory setup to industrial. Several industries have adopted phycoremediation to maintain their discharge standards and to perform well in corporate social responsibility. Besides, they gain huge profits by selling algae-based products like biofuel, feed, and fertilizer to the market. A study highlights the base case cost of about ∼1.6 € kg–1 to 1.8 € kg–1, and the projected case cost is p∼0.3 € kg–1 to 0.4 € kg–1 for microalgae cultivation in the case of raceway pond while in the case of packed bed bioreactor, its ∼9 € kg–1 to 10 € kg–1 and ∼3.8 € kg–1 respectively (Slade and Bauen, 2013). Another research valorizes the use of wastewater, and preferably blue light filter (480 nm) in the raceway system will significantly augment biomass production by 63% and 73%, respectively, i.e., decrease in cost from 2.71 to 0.73 $ kg−1 (Kang et al., 2015). A techno-economic analysis estimates a $2.23/gallon break-even selling price of biofuel with an 18.7% internal rate of returns if the cultivation, harvesting, and downstream cost are monitored. Figure 5 depicts the annual cost spent and income/revenue generated by utilizing wastewater for microalgae cultivation and simultaneous bio-oil production via pyrolysis. Therefore, it is anticipated that wastewater-based microalgae biofuel can become a powerful competitor for the crude oil industry shortly (Xin et al., 2016).
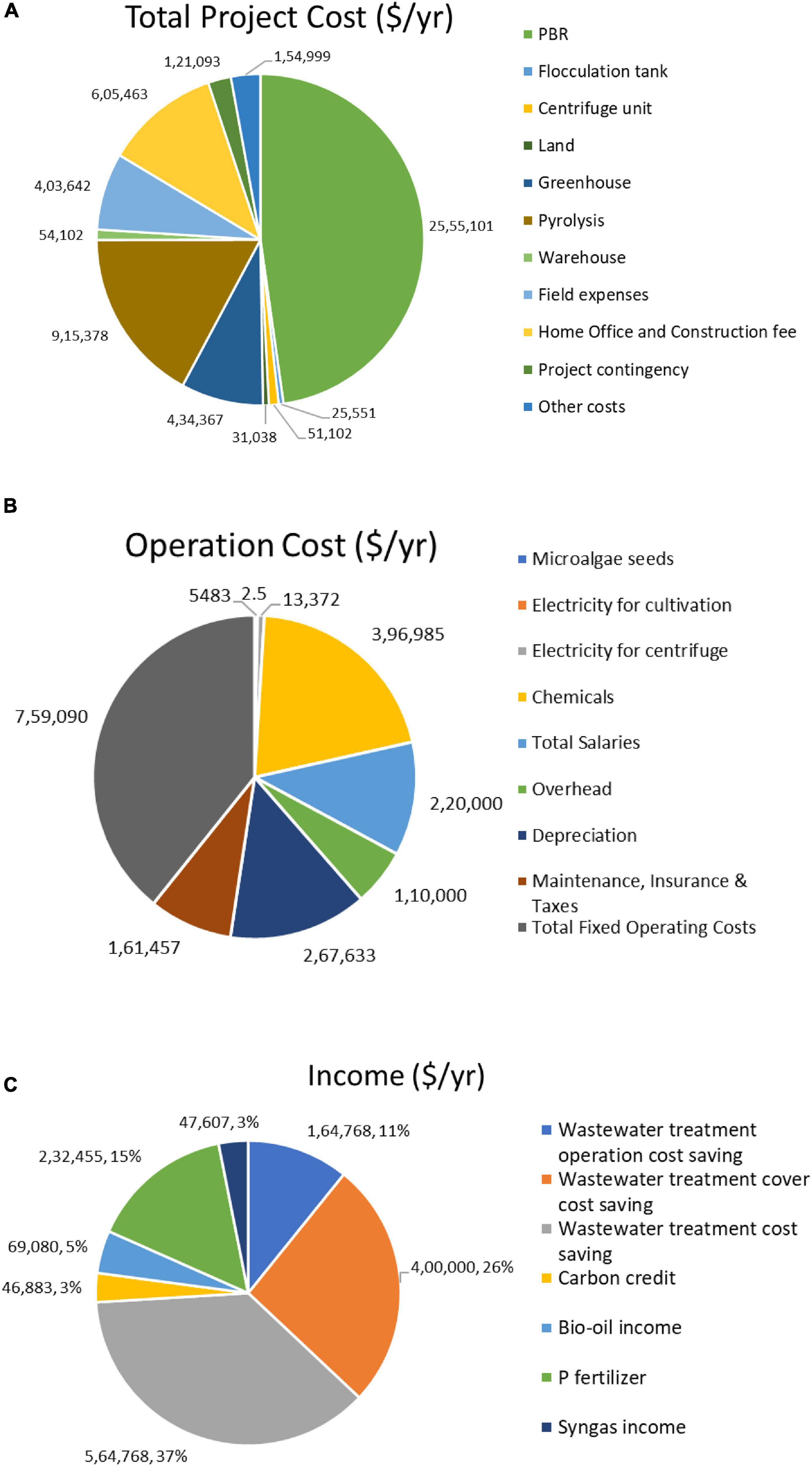
Figure 5. Distribution of costs and income per year (A) Distribution of capital costs; (B) Distribution of operation costs; (C) Distribution of income (Xin et al., 2016).
Lately, Bath University has collaborated with Wessex Waters sewage treatment works to pioneer the new project to deploy algae for recovering the phosphorus load in the United Kingdom. About 80-96% of phosphorus is treated from 3000 liters of wastewater per day in an installed pilot-scale High-Rate Algal Pond setup, resulting in polished wastewater fit for environmental discharge (Algae farms to offer a cheaper, renewable solution to cleaning wastewater, 2020). This seems a suitable replacement for the traditional chemical dosing of wastewater with iron as it can also produce nutrient-rich biomass that can be used for other purposes like biofuel, and bioplastic. Another successful example is phycospectrum that has revolutionized phycoremediation all over India with collaborations with Colombia wastewater treatment plant. It also introduces algal technology in SNAP Products PVT LTD to purify the acidic and toxic effluents high in total dissolved solutes using the naturally occurring Chroococcus turgidusstrain. A 1200 m2 reinforced cement concrete tank was set up to treat 1200 KL of volume at a time with an AC sheet roof to prevent rainwater from mixing. Earlier, 290 tons of sludge deposits per annum were buried in the landfill, which now is rich in nutrients and can be commercialized as valuable fertilizer, generating additional revenue. The industry saves 50 lakh p.a. spent on caustic soda to neutralize the wastewater and three lakhs for sludge disposal (Internet Achive Wayback Mechine, 2020). Similarly, it served about 32 mega industries in India by applying algal technologies to treat the polluted effluent sustainably and producer-waste products (Hanumantha et al., 2011). Currently, the company works on reducing oil slicks in wastewater to <2% using algal strain to transform sludge completely into a soil-like material with good water holding capacity (Sivasubramanian, 2020).
One recent project mainly meant to alleviate the toxic levels of wastewaters from the grassroots dyeing industry is Indus tiles. They are composed of clay material and are made into fan-shaped leaves with algae-laden venations. This cost-effective and reusable modular bioreactor wall system is designed by biochemical engineers in University College London using algae and hydrogel formulations for bioremediation of heavy metals and other pollutants from the wastewater disposed of by local artisans in India (The Indus Project, 2020). Phytochelatins produced by microalgae work as an adsorbent to capture the metals from the colored water and make it suitable for the environment. Another technology Algae wheel wastewater treatment process developed by One Water Inc. has virtually replaced the wetland system in Uniondale, New York, with an average daily flow of 22,300 gpd. This technology uses RAC^TM, which has a hybrid fixed-film rotator that integrates both algal biofilm and moving bed biofilm reactor systems for a significant reduction in pollutant loads (Johnson et al., 2018). Furthermore, an entirely similar approach by Gross-Wen Technologies that uses a vertical conveyer belt instead of a rotator is patented as the revolving algal biofilm treatment technology. A study on the revolving algal biofilm reactor obtained significant results showing 46% sulfates removing efficiency at the 0.56 g L–1d–1 rate on the treatment of low pH drainage from a mining industry. Also, it manifests a substantial reduction in chemical oxygen demand, phosphorus, and ammonia concentration compared with suspended cultures (Zhou et al., 2018). The biomass can be scrapped of quickly and deployed as a slow-releasing fertilizer, bioplastic, or biofuel. The different value-added products from different algal biomass are cultivated under optimum conditions for their highest yield and products such as for the production of biofertilizers, Chlorella sp. gives a maximum yield of 650 mg L–1 from MMT effluent wastewater source (biofertilizer) whereas Phaeodactylum sp. when grown in palm oil mills (wastewater source) yields 140 mg L–1 of exopolysaccharides (Das et al., 2019; Nur et al., 2019). The biofuel produced from municipal wastewater effluent by C. reinhardtii (bio-oil) produces 505 mg L–1 under the optimum conditions (Kong et al., 2010). Another value-added product such as polyhydroxyalkanoates is used for the development of biodegradable plastic obtained from Microcystis sp. cultivated in high-rate algal pond yields 0.7 mg L–1 of polyhydroxyalkanoates (Abdo and Ali, 2019).
Challenges and Perspectives
Microalgae are a promising feedstock for biorefineries due to their capability of resource recovery from wastewater. Microalgae cultivation in municipal wastewater requires nutrient supplements for the high yield of algal biomass, which is cost-effective. The (environmental) costs of synthetic nutrient additions could be replaced by co-fermentation of various wastewater, which provides all the essential nutrients and integration with other product production technologies may help to improve the overall production cost. Another challenge associated with wastewater is the presence of various contaminants (bacteria, protozoa, and fungi) which inhibit microbial growth. The pH and organic compounds such as tannins and lignin present in wastewater can be toxic for the growth of algae, and the presence of heavy metals absorbed by algae declines its usage for pharmaceutical products. Various pretreatment methods can be used to remove contaminants such as filtration and autoclaving but use of these methods is not feasible at a large scale, so there is need to find other methods that can be scaled up easily at lower cost. Wastewater treatment generally results in sludge production while using microalgal culture there is no requirement of chemicals used for the pretreatment and the sludge contains only algal biomass (Bhatia S. K. et al., 2020). For optimum pH and salinity, cultivation can be performed by mixing hypersaline water from industries or cultivating algae in seashore areas. Use of zeolite is also suggested to remove ammonia from wastewater and then use ammonia rich zeolite to release ammonia in algae culture (Markou et al., 2014). Microalgal biomass produced during wastewater treatment is not a waste, it can be used a material to prepare biochar and used as adsorbent for water pollutant removal. Furthermore, for lowering chemical oxygen demand and biological oxygen demand levels, the collected microalgae biomass can be subjected to anaerobic digestion for the production of methane and other energy source. Microalgae consortia (algae-algae, algae-bacteria) can be used to increase the productivity and coproduction of other valuable products (Leong et al., 2019; Nguyen et al., 2019a, b). The composition of wastewater depends on its source and affects microalgal growth, there is a need to find new microalgal species that are more robust, resistant to various environmental factors, withstand high organic load and fulfill most of the desired parameters (high nutrient removal and growth, accumulate high lipid content). Bioreactors and type of cultivation system also affect the growth and the biomass produced by microalgae to produce value-added products. Microalgae mostly grow on the surface of the water and at high culture density cells restrict light penetration and inhibit microalgae growth inside water. There is a need to design unique bioreactors to overcome these issues. Microalgal growth is also affected by other factors such as wastewater turbidity (inhibit light penetration), temperature variation which hinders the use of the same technology in all cases. Nowadays, the techniques used for harvesting are costly and also labor-intensive but in the coming future, with constant efforts and new technologies the new harvesting methods are being developed and produced microalgae can be used for animal feed, bioenergy, biochemical production, and other biotechnological applications. The use of microalgal based resource recovery from wastewater and its integration with other emerging technologies will definitely improve the overall economics of wastewater treatment and reduced the production cost of many commercially valuable compounds in near future.
Conclusion
Microalgae are the most promising candidate for the sustainable treatment of wastewater and the reclamation of nutrients for valuable product production. Microalgae outpace all the traditional wastewater treating techniques like electrochemical methods, precipitation, and membrane technologies by phycoremediation, which is more effective, economically stable, and produces less amount of waste. Microalgae significantly recover nitrogen and phosphorous, lowers biological oxygen demand, and chemical oxygen demand levels. However, many constraints limit the use of microalgae in industries such as, harvesting, and downstream purification of algal biomass for the production of high-value products. Special optimum conditions are needed to be maintained for a particular algal strain depending on the type of industry and desired product of interest. The field of phycology holds enormous opportunities for the purification of water, biofuel production, exopolysaccharides, and bioplastic industries. Sustainable processing of sewage waste and its complete utilization in terms of nutrients with microalgal aid and promoting a biorefinery approach is the need of an hour.
Author Contributions
KA and PaK did the conceptualization and wrote the original draft of the manuscript. SP, Y-HY, and XL carried out the formal analysis. SP, AS, and XL carried out the data curation. Y-HY, SP, PrK, SB, and SK wrote, reviewed, and edited the manuscript. All authors have read and agreed to the published version of the manuscript.
Conflict of Interest
The authors declare that the research was conducted in the absence of any commercial or financial relationships that could be construed as a potential conflict of interest.
Acknowledgments
We would like to thank Prof. Prem Kumar Khosla, Vice-Chancellor and Biotechnology & Bioengineering Department of Shoolini University to provide us with such an opportunity to gain an understanding in the field of phycology and to comprehend the enormous research and development to harness the full potentials of algae in every possible manner. We would also like to acknowledge Scientific Writing Cell, Shoolini University for their valuable inputs during the preparation of the manuscript. We would also like to acknowledge the KU Research Professor Program of Konkuk University, Seoul, South Korea.
References
Abdo, S. M., and Ali, G. H. (2019). Analysis of polyhydroxybutyrate and bioplastic production from microalgae. Bull. Natl. Res. Cent. 43:97. doi: 10.1186/s42269-019-0135-5
Aguilar, M. R., and San Román, J. (2014). Smart Polymers and their Applications. Amsterdam: Elsevier Ltd.
Algae farms to offer a cheaper, renewable solution to cleaning wastewater (2020). https://www.bath.ac.uk/case-studies/algae-farms-to-offer-a-cheaper-renewable-solution-to-cleaning-wastewater (accessed December 12, 2020).
Al-Jabri, H., Das, P., Khan, S., Thaher, M., and AbdulQuadir, M. (2020). Treatment of wastewaters by microalgae and the potential applications of the produced biomass—a review. Water 13:27. doi: 10.3390/w13010027
Angelis, S., Novak, A. C., Sydney, E. B., Soccol, V. T., Carvalho, J. C., Pandey, A., et al. (2012). Co-culture of microalgae, cyanobacteria, and macromycetes for exopolysaccharides production: process preliminary optimization and partial characterization. Appl. Biochem. Biotechnol. 167, 1092–1106. doi: 10.1007/s12010-012-9642-7
Anwar, M. Z., Kim, D. J., Kumar, A., Patel, S. K. S., Otari, S., Mardina, P., et al. (2017). SnO2 hollow nanotubes: a novel and efficient support matrix for enzyme immobilization. Sci. Rep. 7:15333. doi: 10.1038/s41598-017-15550-y
Bafana, A. (2013). Characterization and optimization of production of exopolysaccharide from Chlamydomonas reinhardtii. Carbohydr. Polym. 95, 746–752. doi: 10.1016/j.carbpol.2013.02.016
Bartosh, Y., and Banks, C. J. (2007). Algal growth response and survival in a range of light and temperature conditions: implications for non-steady-state conditions in waste stabilization ponds. Water Sci. Technol. 55, 211–218. doi: 10.2166/wst.2007.365
Batista, A. P., Ambrosano, L., Graça, S., Sousa, C., Marques, P. A. S. S., Ribeiro, B., et al. (2015). Combining urban wastewater treatment with biohydrogen production – an integrated microalgae-based approach. Bioresour. Technol. 184, 230–235. doi: 10.1016/j.biortech.2014.10.064
Béchet, Q., Shilton, A., and Guieysse, B. (2013). Modeling the effects of light and temperature on algae growth: state of the art and critical assessment for productivity prediction during outdoor cultivation. Biotechnol Adv. 31, 1648–1663. doi: 10.1016/j.biotechadv.2013.08.014
Becker, E. W. (2007). Micro-algae as a source of protein. Biotechnol. Adv. 25, 207–210. doi: 10.1016/j.biotechadv.2006.11.002
Behl, K., SeshaCharan, P., Joshi, M., Sharma, M., Mathur, A., Kareya, M. S., et al. (2020). Multifaceted applications of isolated microalgae Chlamydomonas sp. TRC-1 in wastewater remediation, lipid production and bioelectricity generation. Bioresour. Technol. 304:122993. doi: 10.1016/j.biortech.2020.122993
Bhatia, S. K., Kumar, N., and Bhatia, R. K. (2015a). Stepwise bioprocess for exopolysaccharide production using potato starch as carbon source. 3 Biotech. 5, 735–739. doi: 10.1007/s13205-014-0273-2
Bhatia, S. K., Yi, D.-H., Kim, Y.-H., Kim, H.-J., Seo, H.-M., Lee, J.-H., et al. (2015b). Development of semi-synthetic microbial consortia of Streptomyces coelicolor for increased production of biodiesel (fatty acid methyl esters). Fuel 159, 189–196. doi: 10.1016/j.fuel.2015.06.084
Bhatia, S. K., Bhatia, R. K., and Yang, Y.-H. (2016). Biosynthesis of polyesters and polyamide building blocks using microbial fermentation and biotransformation. Rev. Environ. Sci. Biotechnol. 15, 639–663. doi: 10.1007/s11157-016-9415-9
Bhatia, S. K., Bhatia, R. K., and Yang, Y. H. (2017). An overview of microdiesel - a sustainable future source of renewable energy. Renew. Sust. Energy. Rev. 79, 1078–1090. doi: 10.1016/j.rser.2017.05.138
Bhatia, S. K., Bhatia, R. K., Jeon, J.-M., Kumar, G., and Yang, Y.-H. (2019a). Carbon dioxide capture and bioenergy production using biological system – a review. Renew. Sust. Energy. Rev. 110, 143–158. doi: 10.1016/j.rser.2019.04.070
Bhatia, S. K., Gurav, R., Choi, T.-R., Han, Y. H., Park, Y.-L., Park, J. Y., et al. (2019b). Bioconversion of barley straw lignin into biodiesel using Rhodococcus sp. YHY01. Bioresour. Technol. 289:121704. doi: 10.1016/j.biortech.2019.121704
Bhatia, S. K., Wadhwa, P., Hong, J. W., Hong, Y. G., Jeon, J.-M., Lee, E. S., et al. (2019c). Lipase mediated functionalization of poly(3-hydroxybutyrate-co-3-hydroxyvalerate) with ascorbic acid into an antioxidant active biomaterial. Int. J. Biol. Macromole 123, 117–123. doi: 10.1016/j.ijbiomac.2018.11.052
Bhatia, S. K., Gurav, R., Choi, T.-R., Kim, H. J., Yang, S.-Y., Song, H.-S., et al. (2020). Conversion of waste cooking oil into biodiesel using heterogenous catalyst derived from cork biochar. Bioresour. Technol. 302:122872. doi: 10.1016/j.biortech.2020.122872
Bhatia, R. K., Ramadoss, G., Jain, A. K., Dhiman, R. K., Bhatia, S. K., and Bhatt, A. K. (2020). Conversion of waste biomass into gaseous fuel: present status and challenges in India. Bioenergy Res. 13, 1–23.
Bhatia, S. K., Bhatia, R. K., Jeon, J. M., Pugazhendhi, A., Kumar, A., Kumar, M., et al. (2021a). An overview on advancements in biobased transesterification methods for biodiesel production: oil resources, extraction, biocatalysts, and process intensification technologies. Fuel 285:119117. doi: 10.1016/j.fuel.2020.119117
Bhatia, S. K., Gurav, R., Choi, Y.-K., Choi, T.-R., Kim, H.-J., Song, H.-S., et al. (2021b). Bioprospecting of exopolysaccharide from marine Sphingobium yanoikuyae BBL01: Production, characterization, and metal chelation activity. Bioresour. Technol. 324:124674. doi: 10.1016/j.biortech.2021.124674
Bhatia, S. K., Jagtap, S. S., Bedekar, A. A., Bhatia, R. K., Rajendran, K., Pugazhendhi, A., et al. (2021c). Renewable biohydrogen production from lignocellulosic biomass using fermentation and integration of systems with other energy generation technologies. Sci. Total. Environ. 765:144429. doi: 10.1016/j.scitotenv.2020.144429
Bhatia, S. K., Mehariya, S., Bhatia, R. K., Kumar, M., Pugazhendhi, A., Awasthi, M. K., et al. (2021d). Wastewater based microalgal biorefinery for bioenergy production: progress and challenges. Sci. Total Environ. 751:141599. doi: 10.1016/j.scitotenv.2020.141599
Bhatia, S. K., Otari, S. V., Jeon, J.-M., Gurav, R., Choi, Y.-K., Bhatia, R. K., et al. (2021e). Biowaste-to-bioplastic (polyhydroxyalkanoates): conversion technologies, strategies, challenges, and perspective. Bioresour. Technol. 326:124733. doi: 10.1016/j.biortech.2021.124733
Bhunia, B., Prasad Uday, U. S., Oinam, G., Mondal, A., Bandyopadhyay, T. K., and Tiwari, O. N. (2018). Characterization, genetic regulation and production of cyanobacterial exopolysaccharides and its applicability for heavy metal removal. Carbohydr. Polym. 179, 228–243. doi: 10.1016/j.carbpol.2017.09.091
Bindra, S., and Kulshrestha, S. (2019). Converting waste to energy: production and characterization of biodiesel from Chlorella pyrenoidosa grown in a medium designed from waste. Renew. Energy 142, 415–425. doi: 10.1016/j.renene.2019.04.104
Bindra, S., Sharma, R., Khan, A., and Kulshrestha, S. (2017). Renewable energy sources in different generations of bio-fuels with special emphasis on microalgae derived biodiesel as sustainable industrial fuel model. Bioscience 14, 259–274. doi: 10.13005/bbra/2443
Bittencourt, S. E. (2009). Respirometric Balance and Analysis of Four Microalgae: Dunaliella Tertiolecta, Chlorella vulgaris, Spirulina platensis and Botryococcus braunii. thesis, University of Provence: Marseille. [dissertation].
Boelee, N. C., Temmink, H., Janssen, M., and Buisman, C. J. N. (2014). Balancing the organic load and light supply in symbiotic microalgal-bacterial biofilm reactors treating synthetic municipal wastewater. Ecol. Eng. 64, 213–221. doi: 10.1016/j.ecoleng.2013.12.035
Borowitzka, M., and Hallegraeff, G. (2007). Economic Importance of Algae. Hobart, TAS: University of Tasmania, 594–622.
Boyle, G. (2004). Renewable Energy: Power for a Sustainable Future, 2nd Edn. Oxford: Oxford University Press.
Cabello, J., Morales, M., and Revah, S. (2015). Effect of the temperature, pH and irradiance on the photosynthetic activity by Scenedesmus obtusiusculus under nitrogen replete and deplete conditions. Bioresour. Technol. 181, 128–135. doi: 10.1016/j.biortech.2015.01.034
Caetano, N. S., Martins, A. A., Gorgich, M., Gutiérrez, D. M., Ribeiro, T. J., and Mata, T. M. (2020). Flocculation of Arthrospira maxima for improved harvesting. Energy Rep. 6, 423–428. doi: 10.1016/j.egyr.2019.08.083
Cao, S., Jiang, Q., Wu, X., Ghim, D., Gholami Derami, H., Chou, P. I., et al. (2019). Advances in solar evaporator materials for freshwater generation. J. Mater. Chem. 7, 24092–24123. doi: 10.1039/c9ta06034k
Cardoso, L. G., Duarte, J. H., Costa, J. A. V., de Jesus Assis, D., Lemos, P. V. F., Druzian, J. I., et al. (2020). Spirulina sp. as a bioremediation agent for aquaculture wastewater: Production of high added value compounds and estimation of theoretical biodiesel. Bioenergy Res. 14, 254–264. doi: 10.1007/s12155-020-10153-4
Carlsson, S. A., Beilen, J. B., Moller, R., and Clayton, D. (2007). Micro-and Macro-algae: Utility for Industrial Applications Outputs from the EPOBIO Project. Newbury: University of York, CPL Press.
Castro, M. L. Y., and Ballesteros, F. C. (2020). “Nutrient removal and biomass production by immobilized chlorella vulgaris. frontiers,” in Water-Energy-Nexus—Nature-Based Solutions, Advanced Technologies and Best Practices for Environmental Sustainability, eds V. Naddeo, M. Balakrishnan, and K. H. Choo (Berlin: Springer), 187–190. doi: 10.1007/978-3-030-13068-8_46
Cepák, V., and Přibyl, P. (2018). Light intensity and nitrogen effectively control exopolysaccharide production by the green microalga Botryococcus braunii (Trebouxiophyceae). Genet. Plant Physiol. 8, 24–37.
Chaisutyakorn, P., Praiboon, J., and Kaewsuralikhit, C. (2018). The effect of temperature on growth and lipid and fatty acid composition on marine microalgae used for biodiesel production. J. Appl. Phycol. 30, 37–45.
Cheah, W. Y., Show, P. L., Juan, J. C., Chang, J. C., and Ling, T. C. (2018). Waste to energy: the effects of Pseudomonas sp. on Chlorella sorokiniana biomass and lipid productions in palm oil mill effluent. Clean. Technol. Environ. Policy 20, 2037–2045. doi: 10.1007/s10098-018-1505-7
Chen, B., You, W., Huang, J., Yu, Y., and Chen, W. (2010). Isolation and antioxidant property of the extracellular polysaccharide from Rhodella reticulata. J. Microbiol. Biotechn. 26, 833–840. doi: 10.1007/s11274-009-0240-y
Chen, G.-Q. (2009). A microbial polyhydroxyalkanoates (PHA) based bio- and materials industry. Chem. Soc. Rev. 38:2434. doi: 10.1039/b812677c
Chen, M., Tang, H., Ma, H., Holland, T. C., Ng, K. Y., and Salley, S. O. (2011). Effect of nutrients on growth and lipid accumulation in the green algae Dunaliella tertiolecta. Bioresour. Technol. 102, 1649–1655. doi: 10.1016/j.biortech.2010.09.062
Chiaramonti, D., Prussi, M., Casini, D., Tredici, M. R., Rodolfi, L., Bassi, N., et al. (2013). Review of energy balance in raceway ponds for microalgae cultivation: re-thinking a traditional system is possible. Appl. Energy 102, 101–111. doi: 10.1016/j.apenergy.2012.07.040
Choi, O. K., Hendren, Z., Kim, G. D., Dong, D., and Lee, J. W. (2020). Influence of activated sludge derived-extracellular polymeric substance (ASD-EPS) as bio-flocculation of microalgae for biofuel recovery. Algal Res. 45:101736. doi: 10.1016/j.algal.2019.101736
Choi, Y.-Y., Baek, S.-R., Kim, J.-I., Choi, J.-W., Hur, J., Lee, T.-U., et al. (2017). Characteristics and biodegradability of wastewater organic matter in municipal wastewater treatment plants collecting domestic wastewater and industrial discharge. Water 9:409. doi: 10.3390/w9060409
Choudhary, P., Assemany, P. P., Naaz, F., Bhattacharya, A., Castro, J., de, S., et al. (2020). A review of biochemical and thermochemical energy conversion routes of wastewater grown algal biomass. Sci. Total Environ. 726:137961. doi: 10.1016/j.scitotenv.2020.137961
Christenson, L. B., and Sims, R. C. (2012). Rotating algal biofilm reactor and spool harvester for wastewater treatment with biofuels by-products. Biotechnol. Bioeng. 109, 1674–1684. doi: 10.1002/bit.24451
Costa, C., and Hadiyanto, H. (2018). Bioelectricity production from microalgae-microbial fuel cell technology (MMFC). MATEC Web Conf. 156:1017. doi: 10.1051/matecconf/201815601017
Costache, T. A., Acien, F. G., and Morales, M. M. (2013). Comprehensive model of microalgae photosynthesis rate as a function of culture conditions in photobioreactor. Appl. Biotechnol. 97, 7627–7637.
Covarrubias, S. A., De-Bashan, Luz, E., Moreno, M., and Bashan, Y. (2012). Alginate beads provide a beneficial physical barrier against native microorganisms in wastewater treated with immobilized bacteria and microalgae. Appl. Microbiol. Biotechnol. 93, 2669–2680. doi: 10.1007/s00253-011-3585-8
Craggs, R., Sutherland, D., and Campbell, H. (2012). Hectare-scale demonstration of high-rate algal ponds for enhanced wastewater treatment and biofuel production. J. Appl. Phycol. 24, 329–337. doi: 10.1007/s10811-012-9810-8
Cunha, C., Silva, L., Paulo, J., Faria, M., Nogueira, N., and Cordeiro, N. (2020). Microalgal-based biopolymer for nano- and microplastic removal: a possible biosolution for wastewater treatment. Environ. Pollut. 263:114385. doi: 10.1016/j.envpol.2020.114385
Danesi, E. D. G., Rangel-Yagui, C. O., Carvalho, J. C. M., and Sato, S. (2004). Effect of reducing the light intensity on the growth and production of chlorophyll by Spirulina platensis. Biomass Bioenerg. 26, 329–335. doi: 10.1016/S0961-9534(03)00127-2
Danquah, M. K., Ang, L., Uduman, N., Moheimani, N., and Forde, G. M. (2009). Dewatering of microalgal culture for biodiesel production: exploring polymer flocculation and tangential flow filtration. J. Chem. Technol. Biotechnol. 84, 1078–1083. doi: 10.1002/jctb.2137
Das, P., AbdulQuadir, M., Thaher, M., Khan, S., Chaudhary, A. K., and Al-Jabri, H. (2020a). A feasibility study of utilizing hydrothermal liquefaction derived aqueous phase as nutrients for semi-continuous cultivation of Tetraselmis Sp. Bioresour. Technol. 295:122310. doi: 10.1016/j.biortech.2019.122310
Das, P., Khan, S., AbdulQuadir, M., Thaher, M., Waqas, M., Easa, A., et al. (2020b). Energy recovery and nutrients recycling from municipal sewage sludge. Sci. Total Environ. 715:136775. doi: 10.1016/j.scitotenv.2020.136775
Das, P., Quadir, M. A., Thaher, M. I., Alghasal, G. S. H. S., and Aljabri, H. M. S. J. (2019). Microalgal nutrients recycling from the primary effluent of municipal wastewater and use of the produced biomass as bio-fertilizer. Int. J. Environ. Sci. Technol. 16, 3355–3364. doi: 10.1007/s13762-018-1867-8
de Godos, I., Vargas, V. A., Guzmán, H. O., Soto, R., García, B., García, P. A., et al. (2014). Assessing carbon and nitrogen removal in a novel anoxic-aerobic cyanobacterial-bacterial photobioreactor configuration with enhanced biomass sedimentation. Water Res. 61, 77–85. doi: 10.1016/j.watres.2014.04.050
de Leite, L. S., dos Santos, P. R., and Daniel, L. A. (2020). Microalgae harvesting from wastewater by pH modulation and flotation: assessing and optimizing operational parameters. J. Environ. Manage. 254:109825. doi: 10.1016/j.jenvman.2019.109825
Demirbas, A. (2007). Progress and recent trends in biofuels. Prog. Energy Combust. Sci. 33, 1–18. doi: 10.1016/j.pecs.2006.06.001
Ding, Y., Guo, Z., Mei, J., Liang, Z., Li, Z., and Hou, X. (2020). Investigation into the novel microalgae membrane bioreactor with internal circulating fluidized bed for marine aquaculture wastewater treatment. Membranes 10, 1–10. doi: 10.3390/membranes10110353
El Baky, A. H., Hanaa, El Baz, K. F., and El-Latife, S. A. (2013). Induction of sulfated polysaccharides in Spirulina platensis as response to nitrogen concentration and its biological evaluation. J. Aquac. Res. Dev. 5, 1–8. doi: 10.4172/2155-9546.1000206
El-Naggar, N. E. A., Hussein, M. H., Shaaban-Dessuuki, S. A., and Dalal, S. R. (2020). Production, extraction and characterization of Chlorella vulgaris soluble polysaccharides and their applications in AgNPs biosynthesis and biostimulation of plant growth. Sci. Rep. 10:3011. doi: 10.1038/s41598-020-59945-w
Farooq, W., Lee, Y. C., Han, J. I., Darpito, C. H., Choi, M., and Yang, J. W. (2013). Efficient microalgae harvesting by organo-building blocks of nanoclays. Green. Chem. 15, 749–755. doi: 10.1039/c3gc36767c
Fasaei, F., Bitter, J. H., Slegers, P. M., and van Boxtel, A. J. B. (2018). Techno-economic evaluation of microalgae harvesting and dewatering systems. Algal Res. 31, 347–362. doi: 10.1016/j.algal.2017.11.038
Fernández Valiente, E., Ucha, A., Quesada, A., Leganés, F., and Carreres, R. (2000). Contribution of N2 fixing cyanobacteria to rice production: availability of nitrogen from 15N-labelled cyanobacteria and ammonium sulphate to rice. Plant Soil. 221, 107–112. doi: 10.1023/A:1004737422842
Filippino, K. C., Mulholland, M. R., and Bott, C. B. (2015). Phycoremediation strategies for rapid tertiary nutrient removal in a waste stream. Algal Res. 11, 125–133. doi: 10.1016/j.algal.2015.06.011
Freire-Nordi, C. S., Vieira, A. A. H., and Nascimento, O. R. (2005). The metal binding capacity of Anabaena spiroides extracellular polysaccharide: an EPR study. Process Biochem. 40, 2215–2224. doi: 10.1016/j.procbio.2004.09.003
Gao, F., Yang, Z. H., Li, C., Zeng, G. M., Ma, D. H., and Zhou, L. (2015). A novel algal biofilm membrane photobioreactor for attached microalgae growth and nutrients removal from secondary effluent. Bioresour. Technol. 179, 8–12. doi: 10.1016/j.biortech.2014.11.108
Garbowski, T., Bawiec, A., Pulikowski, K., and Wiercik, P. (2017). Algae proliferation on substrates immersed in biologically treated sewage. J. Eco. Eng. 18, 90–98. doi: 10.12911/22998993/66253
George, B., Pancha, I., Desai, C., Chokshi, K., Paliwal, C., Ghosh, T., et al. (2014). Effects of different media composition, light intensity and photoperiod on morphology and physiology of freshwater microalgae Ankistrodesmus falcatus – a potential strain for bio-fuel production. Bioresour Technol. 171, 367–374. doi: 10.1016/j.biortech.2014.08.086
Geyer, R., Jambeck, J. R., and Law, K. L. (2017). Production, use, and fate of all plastics ever made. Sci. Adv. 3:e1700782. doi: 10.1126/sciadv.1700782
Ghafari, M., Rashidi, B., and Haznedaroglu, B. Z. (2016). Effects of macro and micronutrients on neutral lipid accumulation in oleaginous microalgae. Biofuels 9, 147–156.
Gilles, S., Lacroix, G., Corbin, D., Ba, N., Ibriez, L. C., Nandjui, J., et al. (2008). Mutualism between euryhaline tilapia Sarotherodon melanotheron heudelotii and Chlorella sp. implications for nano-algal production in warmwater phytoplankton-based recirculating systems. Aquacult Eng. 39, 113–121. doi: 10.1016/j.aquaeng.2008.09.001
Goswami, R. K., Mehariya, S., Verma, P., Lavecchia, R., and Zuorro, A. (2020). Microalgae-based biorefineries for sustainable resource recovery from wastewater. J. Water Process Eng. 2020:101747. doi: 10.1016/j.jwpe.2020.101747
Greenwell, H. C., Laurens, L. M. L., Shields, R. J., Lovitt, R. W., and Flynn, K. J. (2009). Placing microalgae on the biofuels priority list: a review of the technological challenges. J. R. Soc. Interface 7, 703–726. doi: 10.1098/rsif.2009.0322
Gurav, R., Bhatia, S. K., Choi, T.-R., Jung, H.-R., Yang, S.-Y., Song, H.-S., et al. (2019). Chitin biomass powered microbial fuel cell for electricity production using halophilic Bacillus circulans BBL03 isolated from sea salt harvesting area. Bioelectrochem 130:107329. doi: 10.1016/j.bioelechem.2019.107329
Hamed, I. (2016). The evolution and versatility of microalgal biotechnology: a review. Compr. Rev. Food Sci. Food Saf. 15, 1104–1123. doi: 10.1111/1541-4337.12227
Han, P., Lu, Q., Fan, L., and Zhou, W. (2019). A review on the use of microalgae for sustainable aquaculture. Appl. Sci. 9:2377. doi: 10.3390/app9112377
Hanumantha, R. P., Ranjith Kumar, R., Raghavan, B., Subramanian, V., and Sivasubramanian, V. (2011). Application of phycoremediation technology in the treatment of wastewater from a leather-processing chemical manufacturing facility. Water SA 37, 7–14. doi: 10.4314/wsa.v37i1.64099
He, Z., and Angenent, L. T. (2006). Application of bacterial biocathodes in microbial fuel cells. Electroanalysis 18, 19–20. doi: 10.1002/elan.20060362
Hendricks, R., and Pool, E. J. (2012). The effectiveness of sewage treatment processes to remove faecal pathogens and antibiotic residues. J. Environ. Health 47, 289–297. doi: 10.1080/10934529.2012.637432
Hillman, K. M., and Sims, R. C. (2020). Struvite formation associated with the microalgae biofilm matrix of a rotating algal biofilm reactor (RABR) during nutrient removal from municipal wastewater. Water Sci. Technol. 81, 644–655. doi: 10.2166/wst.2020.133
Hu, H., and Zhou, Q. (2010). Regulation of inorganic carbon acquisition by nitrogen and phosphorus levels in the Nannochloropsis sp. World J. Microbiol. Biotechnol. 26, 957–961. doi: 10.1007/s11274-009-0253-6
Hu, J., Hao, L., Shukla, P., Lin, W., and Luo, J. (2020). Nitrogen and phosphorus removals by the agar-immobilized Chlorella sacchrarophila with long-term preservation at room temperature. Chemosphere 251:126406. doi: 10.1016/j.chemosphere.2020.126406
Hwang, J. H., Church, J., Lim, J., and Lee, W. H. (2018). Photosynthetic biohydrogen production in a wastewater environment and its potential as renewable energy. Energy 149, 222–229. doi: 10.1016/j.energy.2018.02.051
Internet Achive Wayback Mechine (2020). Phyco spectrum, algal technology, biofuels, biodiesel, phycoremediation, effluent treatment, dyeing effluent, CO2 mitigation, global warming, waste water treatment, world’s first phycoremediation plant, Dr V Sivasubramanian, Journal of Algal Biomass Utilization, JABU (2020). Available online at: https://web.archive.org/web/20191117081824/http://drvsiva.com/snap.html (accessed Aug 22, 2020).
Izadpanah, M., Gheshlaghi, R., Mahdavi, M. A., and Elkamel, A. (2018). Effect of light spectrum on isolation of microalgae from urban wastewater and growth characteristics of subsequent cultivation of the isolated species. Algal Res. 29, 154–158. doi: 10.1016/j.algal.2017.11.029
Jha, M. N., and Prasad, A. N. (2006). Efficacy of new inexpensive cyanobacterial biofertilizer including its shelf-life. World J. Microbiol. Biotechnol. 22, 73–79.
Ji, M. K., Abou-Shanab, R. A. I., Kim, S. H., Salama, E., Lee, S. H., Kabra, A. N., et al. (2013). Cultivation of microalgae species in tertiary municipal wastewater supplemented with CO2 for nutrient removal and biomass production. Ecol. Eng. 58, 142–148. doi: 10.1016/j.ecoleng.2013.06.020
Jia, S., and Zhang, X. (2019). Biological HRPs in wastewater. High-Risk Poll. Wastewater 2020, 41–78.
Johnson, D. B., Schideman, L. C., Canam, T., and Hudson, R. J. M. (2018). Pilot-scale demonstration of efficient ammonia removal from a high-strength municipal wastewater treatment side stream by algal-bacterial biofilms affixed to rotating contactors. Algal Res. 34, 143–153. doi: 10.1016/j.algal.2018.07.009
Juneja, A., Ceballos, R. M., and Murthy, G. S. (2013). Effects of environ- mental factors and nutrient availability on the biochemi- cal composition of algae for biofuels production: a review. Energies 6, 4607–4638. doi: 10.3390/en6094607
Kanekiyo, K., Lee, J. B., Hayashi, K., Takenaka, H., Hayakawa, Y., Endo, S., et al. (2005). T. Isolation of an antiviral polysaccharide, nostoflan, from a terrestrial cyanobacterium, Nostoc flagelliforme. J. Nat. Prod. 68, 1037–1041. doi: 10.1021/np050056c
Kang, Z., Kim, B. H., Ramanan, R., Choi, J. E., Yang, J. W., Oh, H. M., et al. (2015). A cost analysis of microalgal biomass and biodiesel production in open raceways treating municipal wastewater and under optimum light wavelength. J. Microbiol. Biotechnol. 25, 109–118. doi: 10.4014/jmb.1409.09019
Khangembam, R., and Tiwari, O. N. (2016). Production of exopolysaccharides by the cyanobacterium Anabaena sp. BTA992 and application as bioflocculants. J. Appl. Biol. 4, 008–011. doi: 10.7324/jabb.2016.40102
Kondaveeti, S., Kim, I. W., Otari, S., Patel, S. K. S., Pagolu, R., Losetty, V., et al. (2019a). Co-generation of hydrogen and electricity from biodiesel process effluents. Int. J. Hydrog. Energy 44, 27285–27296. doi: 10.1016/j.ijhydene.2019.08.258
Kondaveeti, S., Patel, S. K. S., Pagolu, R., Li, J., Kalia, V. C., Choi, M.-S., et al. (2019b). Conversion of simulated biogas to electricity: sequential operation of methanotrophic reactor effluents in microbial fuel cell. Energy 189:116309. doi: 10.1016/j.energy.2019.116309
Kong, Q.-X., Li, L., Martinez, B., Chen, P., Ruan, R., Kong, Q.-X., et al. (2010). Culture of microalgae Chlamydomonas reinhardtii in wastewater for biomass feedstock production. Appl. Biochem. Biotechnol. 160, 9–18. doi: 10.1007/s12010-009-8670-4
Kube, M., Fan, L., and Roddick, F. (2021). Alginate-immobilised algal wastewater treatment enhanced by species selection. Algal Res. 54:102219. doi: 10.1016/j.algal.2021.102219
Kube, M., Jefferson, B., Fan, L., and Roddick, F. (2018). The impact of wastewater characteristics, algal species selection and immobilisation on simultaneous nitrogen and phosphorus removal. Algal Res. 31, 478–488. doi: 10.1016/j.algal.2018.01.009
Kumar, A., Park, G. D., Patel, S. K. S., Kondaveeti, S., Otari, S., Anwar, M. Z., et al. (2019). SiO2 microparticles with carbon nanotube-derived mesopores as an efficient support for enzyme immobilization. Chem. Eng. J. 359, 1252–1264. doi: 10.1016/j.cej.2018.11.052
Kumar, G., Mathimani, T., Sivaramakrishnan, R., Shanmugam, S., Bhatia, S. K., and Pugazhendhi, A. (2020). Application of molecular techniques in biohydrogen production as a clean fuel. Sci. Total. Environ. 722:137795. doi: 10.1016/j.scitotenv.2020.137795
Kumar, M. D., Kavitha, S., Tyagi, V. K., Rajkumar, M., Bhatia, S. K., Kumar, G., et al. (2021). Macroalgae-derived biohydrogen production: biorefinery and circular bioeconomy. Biomass Convers. Biorefine 1–23. doi: 10.1007/s13399-020-01187-x
Kurth, C., Wasmuth, I., Wichard, T., Pohnert, G., and Nett, M. (2019). Algae induce siderophore biosynthesis in the freshwater bacterium Cupriavidus necator H16. Biometals 32, 77–88. doi: 10.1007/s10534-018-0159-6
Lakaniemi, A. M., Intihar, V. M., Tuovinen, O. H., and Puhakka, J. A. (2012). Growth of Chlorella vulgaris and associated bacteria in photobioreactors. Microb. Biotechnol. 5, 69–78. doi: 10.1111/j.1751-7915.2011.00298
Lam, M. K., and Lee, K. T. (2012). Potential of using organic fertilizer to cultivate Chlorella vulgaris for biodiesel production. Appl. Energy 94, 303–308. doi: 10.1016/j.apenergy.2012.01.075
Lam, T. P., Lee, T. M., Chen, C. Y., and Chang, J. S. (2018). Strategies to control biological contaminants during microalgal cultivation in open ponds. Bioresour. Technol. 252, 180–187. doi: 10.1016/j.biortech.2017.12.088
Laycock, B., Halley, P., Pratt, S., Werker, A., and Lant, P. (2013). The chemomechanical properties of microbial polyhydroxyalkanoates. Prog. Polym. Sci. 38, 536–583. doi: 10.1016/j.progpolymsci.2012.06.003
Lee, C.-J., and Palsson, B. (1994). High intensity algal bioreactors using light-emitting diodes. Biotechnol. Bioeng. 44, 1161–1167. doi: 10.1002/bit.260441002.48109
Lee, N., Oh, H. S., Oh, H. M., Kim, H. S., and Ahn, C. Y. (2019). Enhanced growth and lipid production in psychrotolerant acutodesmus by controlling temperature-dependent nitrogen concentration. Biomass Bioenergy 127:105267. doi: 10.1016/j.biombioe.2019.105267
Lee, S.-H., Oh, H.-M., Jo, B.-H., Lee, S.-A., Shin, S.-Y., Kim, H.-S., et al. (2014). Higher biomass productivity of microalgae in an attached growth system using wastewater. J. Microbiol. Biotechnol. 24, 1566–1573. doi: 10.4014/JMB.1406.06057
LeeY-K. (1997). Commercial production of microalgae in the Asia-Pacificrim. J Appl Phycol. 9, 403–411.
Leong, W. H., Azella Zaine, S. N., Ho, Y. C., Uemura, Y., Lam, M. K., Khoo, K. S., et al. (2019). Impact of various microalgal-bacterial populations on municipal wastewater bioremediation and its energy feasibility for lipid-based biofuel production. J. Environ. Manage. 249:13. doi: 10.1016/j.jenvman.2019.109384
Li, K., Liu, Q., Fang, F., Luo, R., Lu, Q., Zhou, W., et al. (2019). Microalgae-based wastewater treatment for nutrients recovery: a review. Bioresour. Technol. 291:121934. doi: 10.1016/j.biortech.2019.121934
Li, M., Zhu, Q., Hu, C.-W., Chen, L., Liu, Z.-L., and Kong, Z.-M. (2007). Cobalt and manganese stress in the microalga Pavlova viridis (prymnesiophyceae): effects on lipid peroxidation and antioxidant enzymes. J. Environ. Sci. 19, 1330–1335. doi: 10.1016/S1001-0742(07)60217-4
Li, Y., Chen, Y. F., Chen, P., Min, M., Zhou, W., Martinez, B., et al. (2011). Characterization of a microalga Chlorella sp. well adapted to highly concentrated municipal wastewater for nutrient removal and biodiesel production. Bioresour. Technol. 102, 5138–5144. doi: 10.1016/j.biortech.2011.01.091
Liu, X., Chen, G., Tao, Y., and Wang, J. (2020). Application of effluent from WWTP in cultivation of four microalgae for nutrients removal and lipid production under the supply of CO2. Renew. Energy 149, 708–715. doi: 10.1016/j.renene.2019.12.092
Liu, X., Wang, K., Zhang, J., Wang, J., Wu, J., and Peng, F. (2019). Ammonium removal potential and its conversion pathways by free and immobilized Scenedesmus obliquus from wastewater. Bioresour. Technol. 283, 184–190. doi: 10.1016/j.biortech.2019.03.038
Ma, C., Wen, H., Xing, D., Pei, X., Zhu, J., Ren, N., et al. (2017). Molasses wastewater treatment and lipid production at low temperature conditions by a microalgal mutant Scenedesmus sp. Z-4. Biotechnol. Biofuel. 10:111. doi: 10.1186/s13068-017-0797-x
Maity, J. P., Hou, C.-P., Majumder, D., Bundschuh, J., Thomas, R. K., Chen, C. P., et al. (2014). The production of biofuel and bioelectricity associated with wastewater treatment by green algae. Energy 78, 94–103. doi: 10.1016/j.energy.2014.06.023
Mallick, N. (2002). Biotechnological potential of immobilized algae for wastewater N, P and metal removal: a review. Biometals 15, 377–390. doi: 10.1023/A:1020238520948
Mantzorou, A., and Ververidis, F. (2019). Microalgal biofilms: a further step over current microalgal cultivation techniques. Sci. Total Environ. 651, 3187–3201. doi: 10.1016/j.scitotenv.2018.09.355
Marino, T., Figoli, A., Chianese, E., Rimauro, J., Mehariya, S., Musmarra, D., et al. (2019). Scenedesmus almeriensis solutions dewatering by using PVDF membrane. Chem. Eng. Trans. 74, 1411–1416. doi: 10.3303/CET1974236
Markou, G., Vandamme, D., and Muylaert, K. (2014). Using natural zeolite for ammonia sorption from wastewater and as nitrogen releaser for the cultivation of Arthrospira platensis. Bioresour. Technol. 155, 373–378. doi: 10.1016/j.biortech.2013.12.122
Mishra, A., and Jha, B. (2009). Isolation and characterization of extracellular polymeric substances from micro-algae Dunaliella salina under salt stress. Bioresour. Technol. 100, 3382–3386. doi: 10.1016/j.biortech.2009.02.006
Mishra, P. K., Rana, S., Singh, L., Sakinah, M., and Ab Wahid, Z. (2019). Outlook of fermentative hydrogen production techniques: an overview of dark, photo and integrated dark-photo fermentative approach to biomass. Energy Strategy Rev. 24, 27–37. doi: 10.1016/j.esr.2019.01.001
Misra, S. K., Valappil, S. P., Roy, I., and Boccaccini, A. R. (2006). Polyhydroxyalkanoate (PHA)/inorganic phase composites for tissue engineering applications. Biomacromolecules 7, 2249–2258. doi: 10.1021/bm060317c
Mohd Udaiyappan, A. F., Abu Hasan, H., Takriff, M. S., and Sheikh Abdullah, S. R. (2017). A review of the potentials, challenges and current status of microalgae biomass applications in industrial wastewater treatment. J. Water. Process. Eng. 20, 8–21. doi: 10.1016/j.jwpe.2017.09.006
Mohd-Sahib, A. A., Lim, J. W., Lam, M. K., Uemura, Y., Isa, M. H., Ho, C. D., et al. (2017). Lipid for biodiesel production from attached growth Chlorella vulgaris biomass cultivating in fluidized bed bioreactor packed with polyurethane foam material. Bioresour. Technol. 239, 127–136. doi: 10.1016/j.biortech.2017.04.118
Molina Grima, E., Belarbi, E.-H., Acién Fernández, F. G., Robles Medina, A., and Chisti, Y. (2003). Recovery of microalgal biomass and metabolites: process options and economics. Biotechnol. Adv. 20, 491–515. doi: 10.1016/S0734-9750(02)00050-52
Morales, M., Sánchez, L., and Revah, S. (2018). The impact of environmental factors on carbon dioxide fixation by microalgae. FEMS Microbiol. Lett. 365:262. doi: 10.1093/femsle/fnx262
Moreno Osorio, J. H., Pinto, G., Pollio, A., Frunzo, L., Lens, P. N. L., Esposito, G., et al. (2019). Start-up of a nutrient removal system using Scenedesmus vacuolatus and Chlorella vulgaris biofilms. Bioresour. Bioprocess. 6:27. doi: 10.1186/s40643-019-0259-3
National Status of Waste Water Generation and Treatment (2020). SulabhENVIS Centre. Available online at: http://www.sulabhenvis.nic.in/database/stst_wastewater_2090.aspx (accessed Dec 12, 2020).
Nelson, M. J., Nakhla, G., and Zhu, J. (2017). Fluidized-bed bioreactor applications for biological wastewater treatment: a review of research and developments. Eng 3, 330–342. doi: 10.1016/J.ENG.2017.03.021
Ndikubwimana, T., Zeng, X., Murwanashyaka, T., Manirafasha, E., He, N., Shao, W., et al. (2016). Harvesting of freshwater microalgae with microbial bioflocculant: a pilot-scale study. Biotechnol. Biofuels. 9:47. doi: 10.1186/s13068-016-0458-5
Ngatu, N. R., Okajima, M. K., Yokogawa, M., Hirota, R., Eitoku, M., Muzembo, B. A., et al. (2012). Anti-inflammatory effects of sacran, a novel polysaccharide from Aphanothece sacrum, on 2,4,6-trinitrochlorobenzene-induced allergic dermatitis in vivo. Ann. Allergy Asthma Immunol. 108, 117–122. doi: 10.1016/j.anai.2011.10.013
Nguyen, T. D. P., Le, T. V. A., Show, P. L., Nguyen, T. T., Tran, M. H., Tran, T. N. T., et al. (2019a). Bioflocculation formation of microalgae-bacteria in enhancing microalgae harvesting and nutrient removal from wastewater effluent. Bioresour. Technol. 272, 34–39. doi: 10.1016/j.biortech.2018.09.146
Nguyen, T. D. P., Nguyen, D. H., Lim, J. W., Chang, C.-K., Leong, H. Y., Tran, T. N. T., et al. (2019b). Investigation of the relationship between bacteria growth and lipid production cultivating of microalgae Chlorella Vulgaris in seafood wastewater. Energies 12:2282. doi: 10.3390/en12122282
Noor-Mohammadi, S., Pourmir, A., and Johannes, T. W. (2014). Method for assembling and expressing multiple genes in the nucleus of microalgae. Biotechnol. Lett. 36, 561–566. doi: 10.1007/s10529-013-1378-0
Novoveská, L., Zapata, A. K. M., Zabolotney, J. B., Atwood, M. C., and Sundstrom, E. R. (2016). Optimizing microalgae cultivation and wastewater treatment in large-scale offshore photobioreactors. Algal Res. 18, 86–94. doi: 10.1016/j.algal.2016.05.033
Nur, M. M. A., Swaminathan, M. K., Boelen, P., and Buma, A. G. J. (2019). Sulfated exopolysaccharide production and nutrient removal by the marine diatom Phaeodactylum tricornutum growing on palm oil mill effluent. J. Appl. Phycol. 31, 2335–2348. doi: 10.1007/s10811-019-01780-2
Osiemo, M. M., Ogendi, G. M., and M’Erimba, C. (2019). Microbial quality of drinking water and prevalence of water-related diseases in marigat urban centre. Kenya. Environ. Health Insights 13:1178630219836988. doi: 10.1177/1178630219836988
Otari, S. V., Patel, S. K. S., Kalia, V. C., and Lee, J.-K. (2020). One-step hydrothermal synthesis of magnetic rice straw for effective lipase immobilization and its application in esterification reaction. Bioresour. Technol. 302:122887. doi: 10.1016/j.biortech.2020.122887
Otari, S. V., Patel, S. K. S., Kim, S. Y., Haw, J. R., Kalia, V. C., et al. (2019). Copper ferrite magnetic nanoparticles for the immobilization of enzyme. Indian J. Microbiol. 59, 105–108. doi: 10.1007/s12088-018-0768-3
Palma, H., Killoran, E., Sheehan, M., Berner, F., and Heimann, K. (2017). Assessment of microalga biofilms for simultaneous remediation and biofuel generation in mine tailings water. Bioresour. Technol. 234, 327–335. doi: 10.1016/j.biortech.2017.03.063
Papazi, A., Makridis, P., and Divanach, P. (2010). Harvesting Chlorella minutissima using cell coagulants. J. Appl. Phycol. 22, 349–355. doi: 10.1007/s10811-009-9465-2
Park, J. B. K., Craggs, R. J., and Shilton, A. N. (2011). Wastewater treatment high rate algal ponds for biofuel production. Bioresour. Technol. 102, 35–42. doi: 10.1016/j.biortech.2010.06.158
Park, Y.-L., Bhatia, S. K., Gurav, R., Choi, T.-R., Kim, H. J., Song, H.-S., et al. (2020). Fructose based hyper production of poly-3-hydroxybutyrate from Halomonas sp. YLGW01 and impact of carbon sources on bacteria morphologies. Int. J. Biol. Macromol. 154, 929–936. doi: 10.1016/j.ijbiomac.2020.03.129
Patel, S. K. S., Gupta, R. K., Das, D., Lee, J.-K., and Kalia, V. C. (2020a). Continuous biohydrogen production from poplar biomass hydrolysate by a defined bacterial mixture immobilized on lignocellulosic materials under non-sterile conditions. J. Clean. Prod. 287:125037. doi: 10.1016/j.jclepro.2020.125037
Patel, S. K. S., Gupta, R. K., Kalia, V. C., and Lee, J.-K. (2020b). Integrating anaerobic digestion of potato peels to methanol production by methanotrophs immobilized on banana leaves. Bioresour. Technol. 323:124550. doi: 10.1016/j.biortech.2020.124550
Patel, S. K. S., Gupta, R. K., Kondaveeti, S., Otari, S. V., Kumar, A., Kalia, V. C., et al. (2020c). Conversion of biogas to methanol by methanotrophs immobilized on chemically modified chitosan. Bioresour. Technol. 315:12379. doi: 10.1016/j.biortech.2020.123791
Patel, S. K. S., Gupta, R. K., Kumar, V., Kondaveeti, S., Kumar, A., Das, D., et al. (2020d). Biomethanol production from methane by immobilized co-cultures of methanotrophs. Indian J. Microbiol. 60, 318–324. doi: 10.1007/s12088-020-00883-6
Patel, S. K. S., Kalia, V. C., Joo, J. B., Kang, Y. C., and Lee, J.-K. (2020e). Biotransformation of methane into methanol by methanotrophs immobilized on coconut coir. Bioresour. Technol. 297:122433. doi: 10.1016/j.biortech.2019.122433
Patel, S. K. S., Jeon, M. S., Gupta, R. K., Jeon, Y., Kalia, V. C., Kim, S. C., et al. (2019). Hierarchical macro-porous particles for efficient whole-cell immobilization: application in bioconversion of greenhouse gases to methanol. ACS Appl. Mater. Interfaces 11, 18968–18977. doi: 10.1021/acsami.9b03420
Patel, S. K. S., Kumar, P., and Kalia, V. C. (2012). Enhancing biological hydrogen production through complementary microbial metabolisms. Int. J. Hydrog. Energy 37, 10590–10603. doi: 10.1016/j.ijhydene.2012.04.045
Patel, S. K. S., Kumar, P., Singh, M., Lee, J.-K., and Kalia, V. C. (2015). Integrative approach to produce hydrogen and polyhydroxybutyrate from biowaste using defined bacterial cultures. Bioresour. Technol. 176, 136–141. doi: 10.1016/j.biortech.2014.11.029
Patel, S. K. S., Shanmugam, R., Kalia, V. C., and Lee, J.-K. (2020f). Methanol production by polymer-encapsulated methanotrophs from simulated biogas in the presence of methane vector. Bioresour. Technol. 304:123022. doi: 10.1016/j.biortech.2020.123022
Pattarkine, M. V., and Pattarkine, V. M. (2012). “Nanotechnology for algal biofuels,” in The Science of Algal Fuels, eds R. Gordon and J. Seckbach (Berlin: Springer), 149–160.
Pierucci, S., Klemeš, J. J., Piazza, L., Bakalis, S., Sed, G., Cicci, A., et al. (2017). Extraction and purification of exopolysaccharides from exhausted Arthrospira platensis (Spirulina) culture systems. Chem. Eng. Trans. 57, 211–216. doi: 10.3303/CET1757036
Podola, B., Li, T., and Melkonian, M. (2017). Porous substrate bioreactors: a paradigm shift in microalgal biotechnology? Trends Biotechnol. 35, 121–132. doi: 10.1016/j.tibtech.2016.06.004
Posadas, E., Alcántara, C., García-Encina, P. A., et al. (2017). “Microalgae-based biofuels and bioproducts,” in Microalgae Cultivation in Wastewater, eds C. Gonzalez-Fernandez and R. Muñoz (Sawston: Woodhead Publishing), 67–91. doi: 10.1016/B978-0-08-101023-5.00003-0
Posten, C. (2009). Design principles of photo-bioreactors for cultivation of microalgae. Eng. Life Sci. 9, 165–177. doi: 10.1002/elsc.200900003
Prabakar, D., Manimudi, V. T., Suvetha, K. S., Sampath, S., Mahapatra, D. M., Rajendran, K., et al. (2018). Advanced biohydrogen production using pretreated industrial waste: outlook and prospects. Renew. Sust. Energ. Rev. 96, 306–324. doi: 10.1016/j.rser.2018.08.006
Prakash, J., Sharma, R., Patel, S. K. S., Kim, I. W., and Kalia, V. C. (2018). Bio-hydrogen production by co-digestion of domestic wastewater and biodiesel industry effluent. PLoS One 13:e0199059. doi: 10.1371/journal.pone.0199059
Preisner, M., Neverova-Dziopak, E., and Kowalewski, Z. (2021). Mitigation of eutrophication caused by wastewater discharge: a simulation-based approach. Ambio 50, 413–424. doi: 10.1007/s13280-020-01346-4
Rahman, A., Putman, R. J., Inan, K., Sal, F. A., Sathish, A., Smith, T., et al. (2015). Polyhydroxybutyrate production using a wastewater microalgae-based media. Algal Res. 8, 95–98. doi: 10.1016/j.algal.2015.01.009
Rai, M. R., Gautom, T., and Sharma, N. (2015). Effect of salinity, pH, light intensity on growth and lipid production of microalgae for bioenergy application. Online J. Biol. Sci. 15, 260–267.
Raize, O., Argaman, Y., and Yannai, S. (2004). Mechanisms of biosorption of different heavy metals by brown marine macroalgae. Biotechnol. Bioeng. 87, 451–458. doi: 10.1002/bit.20136
Rajasulochana, P., and Preethy, V. (2016). Comparison on efficiency of various techniques in treatment of waste and sewage water – a comprehensive review. Resource-Eff. Technol. 2, 175–184. doi: 10.1016/j.reffit.2016.09.004
Rajesh Banu, J., Ginni, G., Kavitha, S., Yukesh Kannah, R., Adish Kumar, S., Bhatia, S. K., et al. (2021). Integrated biorefinery routes of biohydrogen: possible utilization of acidogenic fermentative effluent. Bioresour. Technol. 319:124241. doi: 10.1016/j.biortech.2020.124241
Rana, R. S., Singh, P., Kandari, V., Singh, R., Dobhal, R., and Gupta, S. (2017). A review on characterization and bioremediation of pharmaceutical industrieswastewater: an Indian perspective. Appl. Water. Sci. 7, 1–12. doi: 10.1007/s13201-014-0225-3
Raven, J. A. (1988). The iron and molybdenum use efficiencies of plant growth with different energy, carbon and nitrogen sources. New Phytol. 109, 279–287. doi: 10.1111/j.1469-8137.1988.tb04196.x
Rawat, I., Kumar, R., Mutanda, T., and Bux, F. (2011). Dual role of microalgae: phycoremediation of domestic wastewater and biomass production for sustainable biofuels production. Appl. Energy. 88, 3411–3424. doi: 10.1016/j.apenergy.2010.11.025
Razzak, S. A., Hossain, M. M., Lucky, R. A., Bassi, A. S., and de Lasa, H. (2013). Integrated CO2 capture, wastewater treatment and biofuel production by microalgae culturing - a review. Renew. Sust. Energ. Rev. 27, 622–653. doi: 10.1016/j.rser.2013.05.063
Renuka, N., Guldhe, A., Prasanna, R., Singh, P., and Bux, F. (2018). Microalgae as multi-functional options in modern agriculture: current trends, prospects and challenges. Biotechnol. Adv. Elsevier Inc. 36, 1255–1273. doi: 10.1016/j.biotechadv.2018.04.004
Rhoads, A., Beyenal, H., and Lewandowski, Z. (2005). Microbial fuel cell using anaerobic respiration as an anodic reaction and biomineralized manganese as a cathodic reactant. Environ. Sci. Technol. 39, 4666–4671. doi: 10.1021/es048386r
Rosli, S. S., Lim, J. W., Jumbri, K., Lam, K. M., Uemura, Y., Ho, C. D., et al. (2019). Modeling to enhance attached microalgal biomass growth onto fluidized beds packed in nutrients-rich wastewater whilst simultaneously biofixing CO2 into lipid for biodiesel production. Energy Convers. Manag. 185, 1–10. doi: 10.1016/j.enconman.2019.01.077
Ruiz-Marin, A., Canedo-López, Y., and Chávez-Fuentes, P. (2020). Biohydrogen production by Chlorella vulgaris and Scenedesmus obliquus immobilized cultivated in artificial wastewater under different light quality. AMB Expr. 10:191. doi: 10.1186/s13568-020-01129-w
Ruiz-Marin, A., Mendoza-Espinosa, L. G., and Stephenson, T. (2010). Growth and nutrient removal in free and immobilized green algae in batch and semi-continuous cultures treating real wastewater. Bioresour. Technol. 101, 58–64. doi: 10.1016/j.biortech.2009.02.076
Safarik, I., Prochazkova, G., Pospiskova, K., and Branyik, T. (2016). Magnetically modifed microalgae and their applications. Crit. Rev. Biotechnol. 36, 931–941. doi: 10.3109/07388551.2015.1064085
Samer, M. (2015). “Biological and chemical wastewater treatment processes,” in Wastewater Treatment Engineering, ed. M. Samer (Europe: InTech), doi: 10.5772/61250
Sathiyanarayanan, G., Bhatia, S. K., Kim, H. J., Kim, J.-H., Jeon, J.-M., Kim, Y.-G., et al. (2016). Metal removal and reduction potential of an exopolysaccharide produced by Arctic psychrotrophic bacterium Pseudomonas sp. PAMC 28620. RSC Adv. 6, 96870–96881. doi: 10.1039/C6RA17450G
Sathiyanarayanan, G., Yi, D.-H., Bhatia, S. K., Kim, J.-H., Seo, H. M., Kim, Y.-G., et al. (2015). Exopolysaccharide from psychrotrophic Arctic glacier soil bacterium Flavobacterium sp. ASB 3–3 and its potential applications. RSC Adv. 5, 84492–84502. doi: 10.1039/c5ra14978a
Schiano, V., Gino, A. S., Chuck, S. J., and Allen, M. J. (2019). The microalgae biorefinery: a perspective on the current status and future opportunities using genetic modification. App. Sci. 9:4793. doi: 10.3390/app9224793
Schlesinger, A., Eisenstadt, D., Bar-Gil, A., Carmely, H., Einbinder, S., and Gressel, J. (2012). Inexpensive non-toxic flocculation of microalgae contradicts theories; overcoming a major hurdle to bulk algal production. Biotechnol. Adv. 30, 1023–1030. doi: 10.1016/j.biotechadv.2012.01.011
Selmani, N., Mirghani, M. E., and Alam, M. Z. (1997). Study the Growth of Microalgae in Palm Oil Mill Effluent Wastewater. in IOP Conference series: Earth and Environmental Science. Putrajaya: IOP Publishing.
Selvaratnam, T., Pegallapati, A. K., Montelya, F., and Rodriguez, G. (2014). Evaluation of a thermo-tolerant acidophilic alga, Galdieria sulphuraria, for nutrient removal from urban wastewaters. Bioresour. Technol. 156, 395–399. doi: 10.1016/j.biortech.2014.01.075
Sharma, A., and Arya, S. K. (2017). Hydrogen from algal biomass: a review of production process. Biotechnol. Rep. 15, 63–69. doi: 10.1016/j.btre.2017.06.001
Sharma, P. K., Saharia, M., Srivstava, R., Kumar, S., and Sahoo, L. (2018). Tailoring microalgae for efficient biofuel production. Front. Mar. Sci. 5:382. doi: 10.3389/fmars.2018.00382
Shen, Y., Yuan, W., Pei, Z., Wu, Q., and Mao, M. (2009). Microalgae mass produc-tion methods. Trans ASABE 52, 1275–1287.
Silambarasan, S., Logeswari, P., Sivaramakrishnan, R., Incharoensakdi, A., Cornejo, P., Kamaraj, B., et al. (2021). Removal of nutrients from domestic wastewater by microalgae coupled to lipid augmentation for biodiesel production and influence of deoiled algal biomass as biofertilizer for Solanum lycopersicum cultivation. Chemosphere 268:129323. doi: 10.1016/j.chemosphere.2020.129323
Singh, J. S., Kumar, A., Rai, A. N., and Singh, D. P. (2016). Cyanobacteria: a precious bio-resource in agriculture, ecosystem, and environmental sustainability. Front. Microbiol. 7:529. doi: 10.3389/fmicb.2016.00529
Singh, K. S., Bansal, A., Jha, M. K., and Dey, A. (2012). An integrated approach to remove Cr(VI) using immobilized Chlorella minutissima grown in nutrient rich sewage wastewater. Bioresour. Technol. 104, 257–265. doi: 10.1016/j.biortech.2011.11.044
Singh, M., Patel, S. K. S., and Kalia, V. C. (2009). Bacillus subtilis as potential producer for polyhydroxyalkanoates. Microb. Cell Fact. 8:38. doi: 10.1186/1475-2859-8-38
Singh, S. (2015). Study of waste water effluent characteristics generated from paper industries. J. Basic Appl. Eng. Res. 2, 1505–1509.
Sivasubramanian V. (2020). http://www.algonauts.org/algonauts/4sivasubramanian.html (accessed Aug 22, 2020).
Skoneczny, S., and Tabiś, B. (2015). The method for steady states determination in tubular biofilm reactors. Chem. Eng. Sci. 137, 178–187. doi: 10.1016/j.ces.2015.06.024
Slade, R., and Bauen, A. (2013). Micro-algae cultivation for biofuels: cost, energy balance, environmental impacts and future prospects. Biomass. Bioenerg. 53, 29–38. doi: 10.1016/j.biombioe.2012.12.019
Slegers, P. M., Lösing, M. B., Wijffels, R. H., van Straten, G., and van Boxtel, A. J. B. (2013). Scenario evaluation of open pond microalgae production. Algal Res. 2, 358–368. doi: 10.1016/j.algal.2013.05.001
Smith, B. T., and Davis, R. H. (2012). Sedimentation of algae flocculated using naturally-available, magnesium-based flocculants. Algal Res. 1, 32–39. doi: 10.1016/j.algal.2011.12.002
Solovchenko, A., Verschoor, A. M., Jablonowski, N. D., and Nedbal, L. (2016). Phosphorus from wastewater to crops: an alternative path involving microalgae. Biotechnol. Adv. 34, 550–564. doi: 10.1016/j.biotechadv.2016.01.002
State of the Art Compendium Report on Resource Recovery from Water (2018). https://www.iwa-network.org/wp-content/uploads/2018/02/OFID-Wastewater-report-2018.pdf.
Sternberg, S. H., and Doudna, J. A. (2015). Expanding the biologist’s toolkit with CRISPR-Cas9. Mol. Cell. 58, 568–574. doi: 10.1016/j.molcel.2015.02.032
Suleiman, A. K. A., Lourenço, K. S., Clark, C., Luz, R. L., da Silva, G. H. R., Vet, L. E. M., et al. (2020). From toilet to agriculture: fertilization with microalgal biomass from wastewater impacts the soil and rhizosphere active microbiomes, greenhouse gas emissions and plant growth. Resour. Conserv. Recycl. 161:104924. doi: 10.1016/j.resconrec.2020.104924
Sutherland, D. L., Howard-Williams, C., Turnbull, M. H., Broady, P. A., and Craggs, R. J. (2015). The effects of CO2 addition along a pH gradient on wastewater microalgal photo-physiology, biomass production and nutrient removal. Water Res. 70, 9–26. doi: 10.1016/j.watres.2014.10.064
Tan, J. S., Leec, S. Y., Chewd, K. W., Lame, M. K., Lim, J. W., Hoh, S.-H., et al. (2020). A review on microalgae cultivation and harvesting, and their biomass extraction processing using ionic liquids. Bioengineered 11, 116–129. doi: 10.1080/21655979.2020.1711626
Tan, X. B., Wan, X. P., Yang, L. B., Wang, X., Meng, J., Jiang, M. J., et al. (2021). Nutrients recycling and biomass production from Chlorella pyrenoidosa culture using anaerobic food processing wastewater in a pilot-scale tubular photobioreactor. Chemosphere 270:129459. doi: 10.1016/j.chemosphere.2020.129459
Tao, Q., Gao, F., Qian, C. Y., Guo, X. Z., Zheng, Z., and Yang, Z. H. (2017). Enhanced biomass/biofuel production and nutrient removal in an algal biofilm airlift photobioreactor. Algal Res. 21, 9–15. doi: 10.1016/j.algal.2016.11.004
Taziki, M., Ahmadzadeh, H., and Murry, M. A. (2015). Growth of Chlorella vulgaris in high concentrations of nitrate and nitrite for wastewater treatment. Curr. Biotechnol. 4, 441–447. doi: 10.2174/2211550104666150930204835
Teixeira, M. R., and Rosa, M. J. (2006). Comparing dissolved air flotation and conventional sedimentation to remove cyanobacterial cells of Microcystis aeruginosa Part II. The effect of water background organics. Separat. Purif. Technol. 52, 84–94. doi: 10.1016/j.seppur.2006.03.017
The Indus Project (2020). Innovative Tile-based Bioremediation. Available online at: https://www.materialsource.co.uk/the-indus-project-tile-based-bioremediation/ (accessed Aug 22, 2020).
Ting, H., Haifeng, L., Shanshan, M., Zhang, Y., Zhidan, L., and Na, D. (2017). Progress in microalgae cultivation photobioreactors and applications in wastewater treatment: a review. International J. Agric. Biol. Eng. 10, 1–29.
Torkamani, S., Wani, S. N., Tang, Y. J., and Sureshkumar, R. (2010). Plasmon-enhanced microalgal growth in mini photobioreactors. Appl Phys Lett. 97:043703. doi: 10.1063/1.3467263
Tredici, R. (2004). Mass production of microalgae: photobioreactors. Handb. Microalgal Cul. 1, 178–214.
Uduman, N., Qi, Y., Danquah, M. K., Forde, G. M., and Hoadley, A. (2010). Dewatering of microalgal cultures: a major bottleneck to algae-based fuels. J. Renew. Sust. Energ. 2:012701. doi: 10.1063/1.3294480
Vadiveloo, A., Foster, L., Kwambai, C., Bahri, P. A., and Moheimani, N. R. (2021). Microalgae cultivation for the treatment of anaerobically digested municipal centrate (ADMC) and anaerobically digested abattoir effluent (ADAE). Sci. Total Environ. 775:145853. doi: 10.1016/j.scitotenv.2021.145853
Vandamme, D., Foubert, I., and Muylaert, K. (2013). Flocculation as a low-cost method for harvesting microalgae for bulk biomass production. Trends Biotechnol. 31, 233–239. doi: 10.1016/j.tibtech.2012.12.005
Vandamme, D., Foubert, I., Fraeye, I., Meesschaert, B., and Muylaert, K. (2012). Flocculation of Chlorella vulgaris induced by high pH: role of magnesium and calcium and practical implications. Bioresour. Technol. 105, 114–119. doi: 10.1016/j.biortech.2011.11.105
Vandith, V. A., Setiyawan, A. S., Soewondo, P., and Putri, D. W. (2018). The characteristics of domestic wastewater from office buildings in bandung, West Java, Indonesia. Indones. J. Urban Environ. Technol. 1:199. doi: 10.25105/urbanenvirotech
Varshney, P., Beardall, J., Bhattacharya, S., and Wangikar, P. P. (2018). Isolation and biochemical characterisation of two thermophilic green algal species- asterarcys quadricellulare and Chlorella sorokiniana, which are tolerant to high levels of carbon dioxide and nitric oxide. Algal Resource 30, 28–37. doi: 10.1016/j.algal.2017.12.006
Villar-Navarro, E., Baena-Nogueras, R. M., Paniw, M., Perales, J. A., and Lara-Martín, P. A. (2018). Removal of pharmaceuticals in urban wastewater: high rate algae pond (HRAP) based technologies as an alternative to activated sludge based processes. Water Res. 139, 19–29. doi: 10.1016/j.watres.2018.03.072
Vuppaladadiyam, A., Prinsen, P., Raheem, A., and Luque, R. (2018). Microalgae cultivation and metabolites production: a comprehensive review. Biofuels Bioproducts Biorefining 12, 304–324. doi: 10.1002/bbb.1864
Wang, B., Lan, C. Q., and Horsman, M. (2012). Closed photobioreactors for production of microalgal biomasses. Biotechnol. Adv. 30, 904–912. doi: 10.1016/j.biotechadv.2012.01.019
Wang, J., and Yin, Y. (2018). Fermentative hydrogen production using pretreated microalgal biomass as feedstock. Microb. Cell Fact. 17:22.
Wang, L., Min, M., Li, Y., Chen, P., Chen, Y., Liu, Y., et al. (2010). Cultivation of green algae Chlorella sp. in different wastewaters from municipal wastewater treatment plant. Appl. Biochem. Biotechnol. 162, 1174–1186. doi: 10.1007/s12010-009-8866-7
Win, T. T., Barone, G. D., Secundo, F., and Fu, P. (2018). Algal biofertilizers and plant growth stimulants for sustainable agriculture. Ind. Biotechnol. 14, 203–211. doi: 10.1089/ind.2018.0010
Wollmann, F., Dietze, S., Ackermann, J. U., Bley, T., Walther, T., Steingroewer, J., et al. (2019). Microalgae wastewater treatment: biological and technological approaches. Eng. Life Sci. 19, 860–871. doi: 10.1002/elsc.201900071
Xiao, R., and Zheng, Y. (2016). Overview of microalgal extracellular polymeric substances (EPS) and their applications. Biotechnol. Adv. 34, 1225–1244. doi: 10.1016/j.biotechadv.2016.08.004
Xin, C., Addy, M. M., Zhao, J., Cheng, Y., Cheng, S., Mu, D., et al. (2016). Comprehensive techno-economic analysis of wastewater-based algal biofuel production: a case study. Bioresour. Technol. 211, 584–593. doi: 10.1016/j.biortech.2016.03.102
Yadavalli, R., and Heggers, G. R. V. N. (2013). Two stage treatment of dairy effluent using immobilized Chlorella pyrenoidosa. J. Environ. Health Sci. Eng. 11, 1–6. doi: 10.1186/2052-336x-11-36
Yen, H. W., Hu, I. C., Chen, C. Y., Nagarajan, D., and Chang, J. S. (2019). “Design of photobioreactors for algal cultivation,” in Biofuels from Algae, 2nd Edn,. Amsterdam: Elsevier, 225–256. doi: 10.1016/B978-0-444-64192-2.00010-X
Yim, J. H., Kim, S. J., Ahn, S. H., Lee, C. K., Rhie, K. T., and Lee, H. K. (2003). Antiviral effects of sulfated exopolysaccharide from the marine microalga Gyrodinium impudicum strain KG03. Mar. Biotechnol. 2003, 17–25. doi: 10.1007/S10126-003-0002-Z
Zamalloa, C., Boon, N., and Verstraete, W. (2013). Decentralized two-stage sewage treatment by chemical-biological flocculation combined with microalgae biofilm for nutrient immobilization in a roof installed parallel plate reactor. Bioresour. Technol. 130, 152–160. doi: 10.1016/j.biortech.2012.11.128
Zeller, M. A., Hunt, R., Jones, A., and Sharma, S. (2013). Bioplastics and their thermoplastic blends from Spirulina and Chlorella microalgae. J. Appl. Polym. Sci. 130, 3263–3275. doi: 10.1002/app.39559
Zhang, J., and Hu, B. (2012). A novel method to harvest microalgae via co-culture of filamentous fungi to form cell pellets. Bioresour. Technol. 114, 529–535. doi: 10.1016/j.biortech.2012.03.054
Zhang, L., Chen, P., Huang, J., Yang, G., and Zheng, L. (2003). Ways of strengthening biodegradable soy-dreg plastics. J. Appl. Polym. Sci. 88, 422–427. doi: 10.1002/app.11718
Zhang, Q., Li, X., Guo, D., Ye, T., Xiong, M., Zhu, L., et al. (2018). Operation of a vertical algal biofilm enhanced raceway pond for nutrient removal and microalgae-based byproducts production under different wastewater loadings. Bioresour. Technol. 253, 323–332. doi: 10.1016/j.biortech.2018.01.014
Zhang, Y., Mo, G., Li, X., Zhang, W., Zhang, J., Ye, J., et al. (2011). A graphene modified anode to improve the performance of microbial fuel cells. J. Power Sources 196, 5402–5407. doi: 10.1016/j.jpowsour.2011.02.067
Zheng, H., Liu, M., Lu, Q., Wu, X., Ma, Y., Cheng, Y., et al. (2018). Balancing carbon/nitrogen ratio to improve nutrients removal and algal biomass production in piggery and brewery wastewaters. Bioresour. Technol. 249, 479–486. doi: 10.1016/j.biortech.2017.10.057
Zhou, H., Sheng, Y., Zhao, X., Gross, M., and Wen, Z. (2018). Treatment of acidic sulfate-containing wastewater using revolving algae biofilm reactors: sulfur removal performance and microbial community characterization. Bioresour. Technol. 264, 24–34. doi: 10.1016/j.biortech.2018.05.051
Zhou, W., Hu, B., Li, Y., Min, M., Mohr, M., Du, Z., et al. (2012b). Mass cultivation of microalgae on animal wastewater: a sequential two-stage cultivation process for energy crop and omega-3-rich animal feed production. Appl. Biochem. Biotechnol. 168, 348–363. doi: 10.1007/s12010-012-9779-4
Zhou, W., Li, Y., Gao, Y., and Zhao, H. (2017). Nutrients removal and recovery from saline wastewater by Spirulina platensis. Bioresour. Technol. 245, 10–17. doi: 10.1016/j.biortech.2017.08.160
Zhou, Y., Schideman, L., Yu, G., and Zhang, Y. (2013). A synergistic combination of algal wastewater treatment and hydrothermal biofuel production maximized by nutrient and carbon recycling. Energy Environ. Sci. 6, 3765–3779. doi: 10.1039/c3ee24241b
Keywords: microalgae, wastewater, biofuel, bio-economy, value-added products
Citation: Arora K, Kaur P, Kumar P, Singh A, Patel SKS, Li X, Yang Y-H, Bhatia SK and Kulshrestha S (2021) Valorization of Wastewater Resources Into Biofuel and Value-Added Products Using Microalgal System. Front. Energy Res. 9:646571. doi: 10.3389/fenrg.2021.646571
Received: 27 December 2020; Accepted: 11 March 2021;
Published: 01 April 2021.
Edited by:
Meisam Tabatabaei, MARA University of Technology, MalaysiaReviewed by:
Dillirani Nagarajan, National Cheng Kung University, TaiwanPau Loke Show, University of Nottingham Malaysia Campus, Malaysia
Lim Wei, Petronas University of Technology, Malaysia
Copyright © 2021 Arora, Kaur, Kumar, Singh, Patel, Li, Yang, Bhatia and Kulshrestha. This is an open-access article distributed under the terms of the Creative Commons Attribution License (CC BY). The use, distribution or reproduction in other forums is permitted, provided the original author(s) and the copyright owner(s) are credited and that the original publication in this journal is cited, in accordance with accepted academic practice. No use, distribution or reproduction is permitted which does not comply with these terms.
*Correspondence: Pradeep Kumar, pradeep.kumar@shooliniuniversity.com; Shashi Kant Bhatia, shashikonkukuni@konkuk.ac.kr; shashibiotechhpu@gmail.com; Saurabh Kulshrestha, sourabhkulshreshtha@shooliniuniversity.com
†These authors have contributed equally to this work