- 1Department of Soil Sciences and Agricultural Chemistry Institute of Agricultural Sciences Banaras Hindu University, Varanasi, India
- 2Department of Microbiology (Centre of Excellence), Dr. Rammanohar Lohia Avadh University, Ayodhya, India
- 3Department of Botany, Institute of Sciences Banaras Hindu Uinversity, Varanasi, India
- 4Department of Biochemistry, Faculty of Science, University of Allahabad, Prayagraj, India
- 5Genotoxicity lab, Division of Toxicology and Experimental Medicine, CSIR-Central Drug Research Institute, Lucknow, India
The enzymatic saccharification of sugarcane bagasse was significantly increased by alkali pretreatment under mild conditions. The effectiveness of different concentrations of alkali and acid pretreatment of sugarcane bagasse for improving the enzymatic saccharification of lignocellulose has been evaluated. The sugarcane bagasse was characterized to contain 39.52% celluloses, 25.63% hemicelluloses, and 30.36% lignin. After that, sugarcane bagasse was pretreated with 5 and 10% of H2SO4 and NaOH at 121°C for 60 min. FTIR, XRD, and SEM analyses also showed significant molecular and surface structure changes of the sugarcane bagasse with 10% NaOH. Maximum saccharification was 489.5 mg/g from 10% NaOH pretreatment followed by 322.75, 301.25, and 276.6 mg/g from 10% H2SO4, 5% NaOH, and 5% H2SO4, respectively, which were 55.1, 32.0, 27.1, and 20.6 times higher than the that of the control. Cellulase and xylanase produced by Pseudomonas sp. CVB-10 (MK443365) and Bacillus paramycoides T4 (MN370035) were used to hydrolyze the pretreated bagasse, and the optimal condition was determined to be 30 h of the enzymatic reaction with the 3:1 ratio of enzymes under the temperature of 55°C, pH 5.0, and substrate concentration of 3%, leading to celluloses and hemicelluloses conversion in the enzymatic hydrolysis/saccharification that is more proficient.
Highlights
• Alkali pretreatment improves the saccharification rate of sugarcane bagasse.
• A high percent of lignin and hemicellulose was removed from sugarcane bagasse after alkali pretreatment.
• XRD, FTIR, and SEM analyses confirmed efficient structural changes occurred in pretreated samples.
• The enzymatic hydrolysis was affected by different concentrations of acid and alkali pretreatment conditions.
• Alkali soaking enhanced hydrolysis and glucose yield.
1 Introduction
Sugarcane is one of the most popular crops in India with more than 5 million hectares of land under cultivation. The average yield os sugarcan is more than 75000 kg/hectare with the total production exeeding 360 million tonnes and 110 million ton of bagasse, a solid waste resulting from juice extraction in 2019. Uttar Pradesh has the largest cultivable land of around 21lakh hectares with an annual output of 133.3 million tonnes. Uttar Pradesh stands proudly at the top of the list, second and third largest states are Maharashtra and Karnataka. India hold the second rank in the world after Brazil as far as sugarcane production is concerned.
Sugar industries generated a bulk amount of sugarcane bagasse from sugarcane as a by-product (Hofsetz and Silva 2012; Romero et al., 2020), during glucose, xylose, ethanol, and methane production as an alternate energy to gasoline has been widely practiced in the industry, by virtue of the pronounced fluctuation and increase in oil value, greenhouse gas emissions, global warming, and big demand of petroleum from some developing countries (Quintero et al., 2008; Romero et al., 2020). A part of sugarcane bagasse was used for electricity production, and the remaining 16 million tonnes of dry bagasse have no direct application. The generation of bioethanol can decrease the import of petroleum, and thereby increase the autonomy of energy growth in a country (energy security), such as the United States and Brazil (Demirbas 2011).
The sugarcane bagasse is, as any lignocellulosic material, mostly constituted by cellulose, hemicellulose, and lignin. These three components amount to more than 90% of the dry weight of the fiber. The predominant component of sugarcane bagasse biomass is cellulose, derived from D-glucose units, which condenses through β(1→4)-glycosidic bonds. It is nontoxic, renewable, biodegradable, modifiable, and has great potential as an excellent industrial material (Richardson and Gorton 2003; Nogales and Webber, 2017; Ponce et al., 2021). Cellulose consists of fibrils with crystalline and amorphous regions. Hemicelluloses can include the five-carbon sugars xylose and arabinose, the six-carbon sugars mannose and galactose, and the six-carbon deoxy sugar rhamnose. Lignin comprises about 1/3 of the mass of lignocellulose after cellulose and hemicellulose.
Various technologies have been adopted to improve the bioconversion of these substrates into bioethanol (Gupta et al., 2011; Ko et al., 2016; Costa et al., 2017). Enzymatic saccharification is one of the prominent approaches to alter cellulosic biomass into sugars because of low-energy constraint and less pollution. Due to the recalcitrant structure of lignocelluloses, a pretreatment step is required prior to enzymatic saccharification in order to make the cellulose more accessible to the enzymes (Zhao et al., 2008; Ko et al., 2016). The main aim of various pretreatment methods is to eliminate the lignin content and to reduce the cellulose crystallinity (Mosier et al., 2005). Various physical (comminution and hydro-thermolysis), chemical (acid, alkali, solvents, and ozone), and biological pretreatment methods have been examined over the years by several researchers (Chandel et al., 2014; Lai and Idris 2016; Fasanella et al., 2018).
In acid-catalyzed pretreatment, the major part of the hemicellulose is degraded, and the cellulose has to be hydrolyzed by the use of cellulases. Alkaline pretreatment is basically a delignification process, in which a significant amount of hemicellulose is solubilized as well. The action mechanism is believed to be saponification of intermolecular ester bonds cross-linking xylan hemicelluloses and other components, for example, lignin and other hemicellulose. Alkaline pretreatment also removes acetyl and various uronic acid substitutions on hemicellulose that reduce the accessibility of hemicellulose and cellulose to enzymes (Chang et al., 1997).
Single enzyme saccharification (either cellulase or xylanase) of pretreated sugarcane bagasse has previously been reported by several research workers. However, the application of the mixed cellulose and xylanase enzymatic reaction on pretreated sugarcane bagasse has not been reported elsewhere. The novelty of our work is that we used a cheapest source for pretreatment and mixed enzyme system for saccharification, which enhances the productivity. One another thing is that mixed culture treatment reduced the inhibitory by-product generated during acid and alkali treatments. To gain a greater insight into alkali pretreatment with mixed enzyme saccharification, the effect of alkali pretreatment with mixed enzyme saccharification on the structural changes of sugarcane bagasse was investigated.
Different physical and chemical properties of pretreated sugarcane bagasse (solid part) were studied by several research workers. The crystallinity of the cellulose segment (X-ray diffraction), the organic groups that comprise the biomass (Fourier transform infrared spectroscopy, FTIR), and exterior morphology (scanning electron microscopy, SEM) has been observed. Different morphological studies with different biomass such as soybean straw, wheat bran, and rice hulls have also been approved after different pretreatments (Zhao et al., 2010; Camargo et al., 2012; Nogales and Webber, 2017; Ponce et al., 2021; Ummalyma et al., 2021).
The main aim of this study was to apply strong acid and alkali pretreatments of sugarcane bagasse and analyze the chemical composition, CrI (crystallinity index), SEM and FTIR analyses of pretreated bagasse. Afterthat, enzymatic saccharification/hydrolysis of pretreated sugarcane bagasse by mix-culture was carried out for higher glucose production/generation.
2 Materials and Methods
2.1 Preparation of Raw Materials
The raw substrate sugarcane bagasse was collected locally, dried in a hot air oven at 50°C, and then cut into small pieces. The dried material was ground and passed through a 20–40 mesh size screen using a laboratory knife mill (Metrex Scientific Instrumentation, Delhi, India). The processed substrate was thoroughly washed, dried at 60°C, and stored in sealed plastic bags at room temperature for further experiments.
2.2 Microorganism
The strains of Pseudomonas sp. CVB-10 and Bacillus paramycoides T4, isolated from the soil sample of different sites of Varanasi, were used in this study. The Pseudomonas sp. CVB-10 and Bacillus paramycoides T4 culture was maintained on carboxymethyl cellulose (CMC) and xylan agar slants at 4°C and subcultured monthly.
2.3 Inoculum Preparation
The mother culture was prepared by inoculating one full loop of the 24-h grown culture of Pseudomonas sp. CVB-10 (MK443365) and Bacillus paramycoides T4 (MN370035) on the CMC and xylan agar plate in 50 ml CMC broth and xylan broth and incubated at 40°C for overnight to achieve the active exponential phase. A suitable amount of cell suspension was used to inoculate the test flasks.
2.4 Enzyme Production
The culture was grown in a 150-ml Erlenmeyer flask that contains 50 ml basal medium containing 2.0% untreated sugarcane bagasse and 0.5% ammonium sulfate for cellulase production and 1% birch wood xylan and 0.05% ammonium sulfate for xylanase production. The pH of the medium was adjusted to 5.5 prior to sterilization. The flasks were inoculated and incubated at 40°C for 48 h. The crude enzyme was filtered and centrifuged at 10,000 rpm for 10 min, and enzyme assay was carried out. Enzyme activity was measured by the Nelson–Somogyi method (Nelson 1944; Somogyi 1952). One unit of enzyme activity is defined as 1 mg of the reducing end group (glucose) released per min at 40°C.
2.5 Sugarcane Bagasse Composition
Cellulose, hemicellulose, lignin, ash, organic solvent extractive, and hot water extractive (100°C) contents were quantified in the raw material and in the solid fraction of the pretreated bagasse. The amounts of cellulose, hemicellulose, lignin and ashes were determined according to the methods described by Gouveia et al. (2009). Determinations of organic solvent and hot water extractives were carried out according to the NREL procedure (National Renewable Energy Laboratory, Golden, Colorado, USA) (Sluiter et al., 2008) with some modifications; quantification of hot water extractives (sugars, HMF, furfural, and organic acids) was carried out by high-performance liquid chromatography (HPLC). All characterizations were assayed in triplicate.
2.6 Pretreatments
2.6.1 Acid Pretreatment
The dilute H2SO4 (98% purity) pretreatment of the sugarcane bagasse substrate (10.0 g) was carried out using varied acid concentration (5 and 10%, w/v) and incubation time (30 and 60 min) at 121°C, using a ratio of 1/10 between the bagasse mass and the volume of acid solution. The hydrolysates after treatment were separated by filtering the contents through a double-layered muslin cloth. The residual biomass (cellulignin) was washed with tap water until neutral pH and dried in a hot air oven at 65°C.
2.6.2 Alkali Pretreatment
The sugarcane bagasse (10.0 g) was presoaked in two different concentrations of NaOH (5 and 10%, w/v) for 2 h and thereafter thermally pretreated at 121°C, using a ratio of 1/10 between the sugarcane bagasse mass and the volume of alkali solution for 30 and 60 min. The pretreated samples were filtered through a double-layered muslin cloth, washed extensively with tap water until neutral pH, and dried at 65°C.
2.7 Structural Characterization
2.7.1 Fourier Transform Infrared Spectroscopy Analysis
The chemical structures of untreated and pretreated sugarcane bagasse (acid and alkali) were characterized by FTIR (Thermo Electron Scientific Instruments LLC, United States). All solid samples were dried and then pressed into a disc with KBr. The samples (KBr pellets) for analyses were prepared by mixing the 2 mg material powder with 200 mg KBr. The discs used in this work were thin enough to obey the Beer–Lambert law. Infrared spectra were obtained using a Nicolet iS5 FTIR spectrometer with thirty-two scans along with a resolution of 4 cm−1 in the range of 400 and 4000 cm−1. Thus, it was possible to detect the changes caused by the pretreatments in relation to the content of lignin and hemicellulose.
2.7.2 X-Ray Diffraction
The crystalline nature of untreated and pretreated sugarcane bagasse samples (Acid and Alkali) was analyzed by using a Rigaku SmartLab 9 kW Powder type (without χ cradle) HR-XRD using monochromatic CuKa radiation (1.54 Å) set at 40 kV, 30 mA. The goniometer scanned a 2θ range between 5° and 70° at a 5°/min scanning rate. Samples were scanned over the range of 100 <2θ < 500 with a step size of 0.05°, and the CrI was determined using the empirical method proposed by Segal et al. (1959). Samples were measured in duplicate, and the average values of the CrI were obtained from the relationship between the intensity of the 002 peak for cellulose I (I002) and the minimum dip (Iam) between the 002 and the 101 peaks, following the formula:
in which I002 is the intensity for the crystalline portion of biomass at about 2 h = 22.5°, and Iam is the peak for the amorphous portion (i.e., cellulose, hemicelluloses, and lignin) at about 2 h = 16.6°. The second highest peak after 2 h = 22.5° was 2 h = 16.6° and was assumed to correspond to the amorphous region (Kumar et al., 2009).
2.7.3 Scanning Electron Microscopy
Scanning electron microscopy (SEM) was used to observe the morphology and to evaluate the changes in the external structure of the raw and pretreated sugarcane bagasse caused by the pretreatments (EVO 18 Research ZEISS, United Kingdom). SEM was carried out using a voltage of 10 kV and working distance of 10 mm, spot size of 4.0, SE detector, and metallizer (EVO 18 Research ZEISS, United Kingdom). Before determination, samples were mounted with conductive glue and coated with a thin layer of gold to improve the conductivity and the quality of the SEM images. Finally, many spots (at least five) were considered for each sample under different magnifications.
2.8 Enzymatic Saccharification
The pretreated sugarcane bagasse samples were hydrolyzed using the condensed enzyme. The hydrolysis reaction was performed in 0.1 M citrate buffer (pH 5.0) at 50°C for 96 h under a shaking condition (150 rpm). The substrate with the buffer was pre-incubated at 50°C on an orbit shaker incubator (RC5100 SELEC, NEOLAB, orbit shaker incubator, Germany) at 150 rpm for 2 h, and thereafter the slurry was added with cellulase and xylanase enzymes produced by the isolated bacterial culture Pseudomonas sp. CVB-10 and Bacillus paramycoides T4. Tween-20 (0.1%, v/v) was also added to the reaction mixture, and the reaction continued up to 48 h. Samples of enzymatic hydrolysate were withdrawn at regular intervals and analyzed for the amount of glucose released by Nelson (1944) and Somogyi (1952) methods. The effects of different factors, for e.g., pretreatment reaction time (6–48 h), substrate concentration (1–10% w/v), temperature (40–60°C), pH (4.0–6.0), substrate enzyme ratio (1:1, 1:2, 1:3, 2:1, and 3:1), and Tween-20 concentration (0.1–1.0%) on the enzymatic hydrolysis were determined by maintaining the enzyme/substrate ratio at 25 FPU/g. All enzyme saccharification experiments were performed in triplicate. All the experiments were performed in triplicate, and the results are presented as mean ± SD.
3 Results and Discussion
3.1 Chemical Analysis
Indigenous sugarcane bagasse was applied for chemical composition analysis and found that sugarcane bagasse contains cellulose (39.52%), hemicellulose (25.63%), total lignin (30.36%), ash (1.44%), and extractives (2.90%) (Table 1). Similar results were reported by several other research workers (Chandel et al., 2014; Ladeira-Ázar et al., 2018; Shi et al., 2019; Ummalyma et al., 2021). The composition of sugarcane bagasse fluctuates with variety, origin, cultivation type of sugarcane, and the analytical method used for the characterization (Canilha et al., 2012; Chandel et al., 2014). In contrast to our result, Moretti et al. (2014) observed that 46.9% cellulose, 16.3% hemicellulose, 27.1% lignin, and 2.0% ash were found in sugarcane bagasse. Lamounier et al. (2018) also reported that 54.4% cellulose, 13.5% hemicellulose, 26.1% total lignin, and 0.6% ash were present in sugarcane bagasse.
In the chemical analysis, it was found that after alkaline pretreatment, the proportion of cellulose and hemicellulose increased by 33 and 27%, respectively, while lignin decreased by 44%. Lamounier et al. (2018) also reported that after alkali pretreatment, the lignin content of sugarcane bagasse was decreased by 43%. These results were previously predictable because alkali works primarily on lignin, promoting its degradation. Lignin is considered a barrier that confines the access of essential enzymes for saccharification (Nakagame et al., 2011; Ladeira-Ázar et al., 2018). Hence, degradation of lignin may assist the action of cellulase and hemicellulase enzymes on cellulose and hemicellulose, respectively. Hydrolysis of hemicellulose and cellulose in alkaline pretreatment is less than that in the acid-treated samples (Carvalheiro et al., 2008; Lamounier et al., 2018). From the above results, it is clear that observed data are in agreement with text, which reports that alkaline pretreatment preferentially removes lignin (Falkoski et al., 2013; Lamounier et al., 2018), and acid pretreatment degrades the hemicellulose fraction (Harrison et al., 2013; Ladeira-Ázar et al., 2018). Hence, the pretreatment process is necessary for enzymatic competence during the saccharification process.
3.2 Structural Characterization
3.2.1 Fourier Transform Infrared Spectroscopy
The chemical structure of untreated and pretreated bagasse samples was analyzed by using FTIR. From Figure 1, it is clear that the spectra produced by samples pretreated with acid (5 and 10% H2SO4 at 121°C for 60 min) and alkali (5 and 10% NaOH at 121°C for 60 min) were highly different from untreated sugarcane bagasse. In this experiment, the absorbency of 897 cm−1 was observed, which is more intense in cases of acid-pretreated sugarcane bagasse than alkali-pretreated sugarcane bagasse and untreated sample. The results indicate that the presence of amorphous cellulose, the band at 897 cm−1, which distinguishes the C–O–C stretching at the β-1,4-glycosidic linkage, is burly and sharp (Pandey and Pitman 2003; Zhang et al., 2019). The intensity of the regenerated cellulose band is relatively stronger than that of the original cellulose. It has been reported that the intensity of this peak increases with a decrease in the crystallinity of the cellulose sample and a change in the crystal lattice from cellulose I to cellulose II (Nelson and O’Connor 1964; Wiercigroch et al., 2017; Ummalyma et al., 2021). These observations indicated that the regenerated cellulose has lower crystallinity, and the pretreatment led to the conversion of the crystalline structure of the original cellulose from cellulose I to cellulose II.
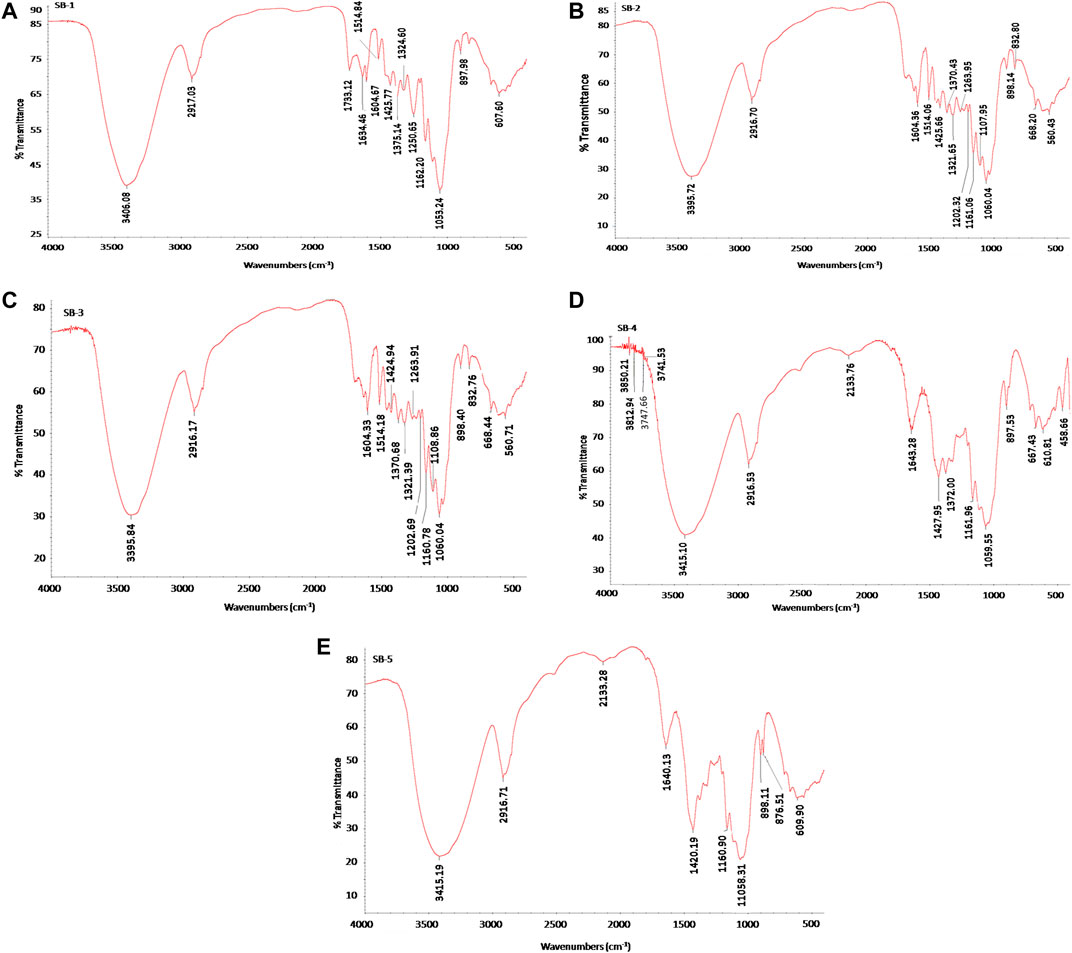
FIGURE 1. Fourier transform infrared (FTIR) spectra of untreated, acid-, and alkali- pretreated sugarcane bagasse (SB). (A) Untreated sugarcane bagasse, (B) 5% sulfuric acid-pretreated bagasse at 121°C for 60 min, (C) 10% sulfuric acid-pretreated bagasse at 121°C for 60 min, (D) 5% sodium hydroxide-pretreated bagasse at 121°C for 60 min, and (E) 10% sodium hydroxide-pretreated bagasse at 121°C for 60 min.
The band at 1,053 to 1,060 cm-1 shows the disordered crystalline region of untreated and pretreated sugarcane bagasse samples. These peaks represent the shattering of the hydrogen bond in pretreated sugarcane bagasse samples (Guo et al., 2009; Lai and Idris 2016). The absorbency of 1,250–1,263 cm−1 (C–C) was more intense in the acid-pretreated (5 and 10% H2SO4 at 121°C for 60 min) and untreated sugarcane bagasse and departs in the alkali-pretreated (5 and 10% NaOH at 121°C for 60 min) sample, although the peak at 1,202 cm−1 (C–O and C=O stretching) was stronger in the acid pretreatment. Disappearance of these bands represents that lignin was partially or effectively removed after alkali pretreatment (Lai and Idris 2016). Guilherme et al. (2015) also reported a similar observation regarding those peaks after pretreatment of bagasse.
The bands at 1,375, 1,162, and 1,055 cm−1 were weaker for acid and alkali pretreated samples than those for the raw sample. In addition, the broad band at 1,375 cm−1 was due to the phenolic hydroxyl group (Labbe et al., 2005; Remli et al., 2014). The mean value for the relative absorbance of phenolic hydroxyl groups was reduced for pretreated bagasse (Remli et al., 2014). The peaks at 1,375, 1,162, and 1,055 cm−1 are specifically attributed to C–H bending vibration, C–O–C asymmetric bridge stretching vibration, and C–O stretching vibration in cellulose and hemicellulose, respectively (Karthika et al., 2013; Qing et al., 2014; Wiercigroch et al., 2017; Ponce et al., 2021).
The band at 1,425 cm−1 is intense in crystalline cellulose and fragile in amorphous cellulose. Therefore, elevated crystalline cellulose was found in 5 and 10% H2SO4, 5% NaOH, and untreated sugarcane bagasse than in the 10% NaOH-treated sample. The peak 1,425 cm−1 can be assigned to bending vibration of CH2 (Han et al., 2012; Mood et al., 2013). This band is strong in crystalline cellulose and weak in amorphous cellulose (Bian et al., 2014). So, higher crystalline cellulose was obtained in 5 and 10% H2SO4, 5% NaOH, and untreated sugarcane bagasse than the 10% NaOH-treated sample. On the other hand, cellulose in sugarcane bagasse became more amorphous after pretreatment using strong alkali treatment. It could be concluded that the amount of amorphous cellulose was the highest in the sugarcane bagasse sample pretreated by 10% NaOH followed by 5% and 10% H2SO4 and 5% NaOH.
The peaks at 1,324, 1,514, and 1,604 cm−1 were indicators of hemicelluloses and lignin characteristics (Mood et al., 2013; Lv et al., 2018). More specifically, the 1,324 cm−1 peak reveals the aromatic hydroxyl groups generated by the cleavage of ether bonds within lignin and 1,514 cm−1 is associated with the aromatic skeletal modes of lignin, whereas 1,604 cm−1 is stated to be stretching of the C = C and C =O lignin aromatic ring (Karthika et al., 2013; Chandel et al., 2014; Lv et al., 2018; Ummalyma et al., 2021). As observed in Figure 1, bagasse samples subjected to acid pretreatment were delignified slightly for the peaks generated at 1,324, 1,514, and 1,604 cm−1, which were identical and that there was a subtle difference between the acid-pretreated samples and the untreated one. However, the peak disappears at 1,324, 1,514, and 1,604 cm−1 when bagasse subjected to alkali pretreatment was delignified more efficiently than the acid-pretreated and untreated sugarcane bagasse. Chandel et al. (2014), and Zhang et al. (2019) also reported similar results for sugarcane bagasse.
The FTIR analysis of bagasse further showed an aldehyde group absorption peak that was clearly present at 1733 cm−1. This absorbance has been suggested to be due to acetyl groups in the lignin or hemicellulose structure (Yoon et al., 2011; Ponce et al., 2021; Ummalyma et al., 2021). It was observed that the absorption peak at 1733 cm−1 disappeared when the sugarcane bagasse was pretreated with acid and alkali. The relative absorbance of these two kinds of CO groups was reduced in the pretreated solid residues (Chandel et al., 2014; Ummalyma et al., 2021). This reduction in the ketone and aldehyde groups may be due to degradation of the aliphatic chain of phenyl propane units in the lignin molecules. The absorbance by hydroxyl groups occurs as a number of different bands.
The peak at 3,395 cm−1 (O-H) was stronger in the acid pretreatment (5 and 10% H2SO4 at 121°C for 60 min) than that in the alkaline pretreatment (5 and 10% NaOH at 121°C for 60 min) and in untreated sugarcane bagasse. A similarity in the bands at 2,917 cm−1 could be observed for the raw material, acid, and alkaline pretreatment but was more intense for the acid pretreatments. The 2,917 cm−1 band represents C–H and CH2 stretching, which is unaffected by changes in crystallinity (Nelson and O’Connor 1964; Wiercigroch et al., 2017; Ponce et al., 2021). The results indicated that the highly crystalline cellulose in sugarcane bagasse was transformed to an amorphous form after pretreatment. Overall, as could be concluded from Figure 1, using alkali pretreatment is a suitable method for removing lignin.
3.2.2 X-Ray Diffraction
X-ray diffraction (XRD) investigation was carried out to assess the crystallinity degree of the untreated and pretreated bagasse. The XRD analysis of untreated bagasse, acid-pretreated (5 and 10% H2SO4 at 121°C for 60 min) bagasse (cellulignin), and alkali-pretreated (5 and 10% NaOH at 121°C for 60 min) cellulignin substrate is presented in Figures 2A–E. Crystallinity is strongly influenced by the biomass composition. The intensities (I002) of the amorphous cellulose peak and crystalline cellulose peak were considered to calculate the crystallinity index (CrI) of all five samples of bagasse. The CrI of untreated bagasse was 49.67%, which was close to a previously available report (Table 2) (Bi et al., 2016; Lv et al., 2018). The CrI of acid- and alkali-pretreated sugarcane bagasse was comparatively lower than that of untreated sugarcane bagasse showing the sequential increment in the cellulose content in these samples (Figures 2B–E). Acid pretreatment of bagasse (5 and 10% H2SO4 at 121°C for 60min) removed the hemicellulose and thus increased the cellulose amount in samples eventually and showed lower CrI (35.7 and 33.97%). Further, cellulignin when pretreated with alkali (5 and 10% NaOH at 121°C for 60 min) showed lower CrI (41.1 and 11.2%) because of the removal of lignin and thus increased the cellulose concentration in bagasse than that of untreated sugarcane bagasse and cellulignin. In other words, this sharp decrease in crystallinity due to the alkali pretreatment confirmed that the regenerated products were highly amorphous and thus cellulose surface accessibility and consequently the efficiency of enzymatic hydrolysis were considerably increased (Mosier et al., 2005; Yoon et al., 2011; Mood et al., 2013; Lv et al., 2018; Bartos et al., 2020; Ponce et al., 2021).
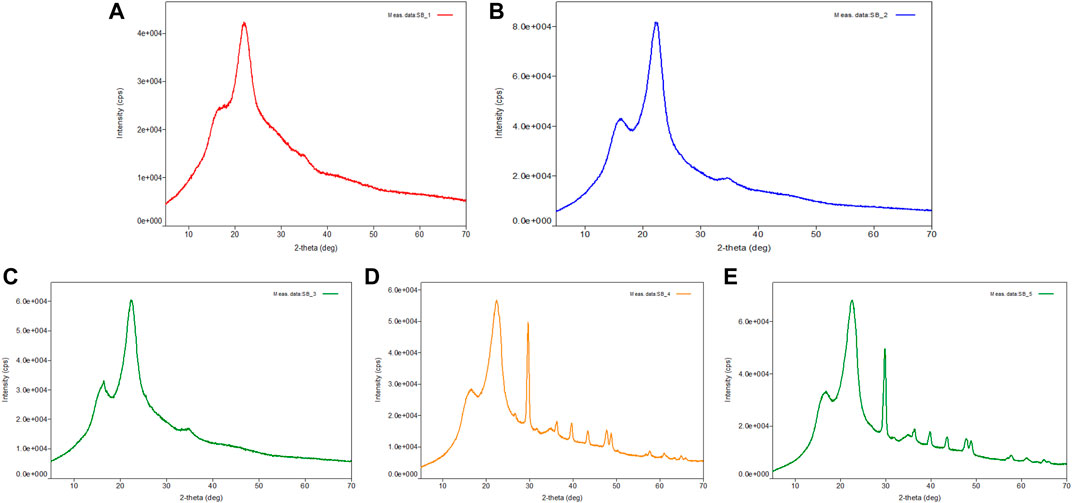
FIGURE 2. X-ray diffraction (XRD) pattern of native, acid-, and alkali-pretreated sugarcane bagasse (SB). The crystallinity index (CrI) was found to be increased in cellulignin and NaOH-pretreated bagasse. Enzymatic hydrolyzed SB showed the CrI value of cellulignin and NaOH-treated bagasse. (A) Untreated sugarcane bagasse, (B) 5% sulfuric acid-pretreated bagasse at 121°C for 60 min, (C) 10% sulfuric acid-pretreated bagasse at 121°C for 60 min, (D) 5% sodium hydroxide-pretreated bagasse at 121°C for 60 min, and (E) 10% sodium hydroxide-pretreated bagasse at 121°C for 60 min. CrI, crystallinity index; SB, sugarcane bagasse; XRD, X-ray diffraction.
3.2.3 Scanning Electron Microscopy
Scanning electron microscopy analyzed external structural alterations that occurred in pretreated sugarcane bagasse by different concentrations of acid (5 and 10% H2SO4 at 121°C for 60 min) and alkali (5 and 10% NaOH at 121°C for 60 min). SEM images of untreated, acid, and alkali-pretreated sugarcane bagasse samples were taken at different magnifications. Figure 3A clearly indicates that the untreated bagasse had highly compact, ordered, and rigid fibril morphology (Zhang et al., 2019; Ponce et al., 2021) when compared with that of acid- and alkali-pretreated bagasse samples (Figures 3B–E). Several research workers have reported similar observation for untreated and treated bagasse (Bi et al., 2016; Lv et al., 2018; Bartos et al., 2020). The alkali-pretreated bagasse sample (10% NaOH at 121°C for 60 min) was harshly disrupted followed by 5% NaOH, 10% H2SO4, and 5% H2SO4 pretreatments. On the other hand, acid (5 and 10% H2SO4 at 121°C for 60 min) and alkali (5% NaOH at 121°C for 60 min) pretreatments had some little dissimilar effects on sugarcane bagasse (Figures 3B–E). The disruption of the residue surface might have been caused by the solvating action of the acid and alkali pretreatment (Ang et al., 2012; Bartos et al., 2020; Ponce et al., 2021). Due to the partial removal of hemicelluloses and lignin, the surface of the bagasse with NaOH pretreatment became soft, loosened, and contained some micro-pores on the surface of the sugarcane bagasse (Figures 3D,E). From the figure, it revealed that the texture of the surface has become rough, puffy, loose, and conglomerate, and the native fibrous structure has been wholly distorted after the pretreatment by 10% NaOH at 121°C for 60 min. Acid (5 and 10% H2SO4 at 121°C for 60 min) and alkali (5% NaOH at 121°C for 60 min) pretreatments had similar effects on the bagasse sample (Figures 3B–E) and led to the highest modifications in the bagasse structure after 10% alkali pretreatment. Similarly, Fasanella et al. (2018) also reported that when bagasse treated with NaOH, it not only breaks the lignin structure but also hydrates and swells the cellulose fibers, reducing crystallinity. Accessibility of the substrate to the cellulolytic enzymes is one of the major factors influencing the hydrolysis process (Bian et al., 2014; Sun et al., 2016; Lv et al., 2018). The previous study has illustrated that the cellulases can get trapped in the pores, if the internal area is much larger than the external area (Cao et al., 2012). Thus, one of the objectives of the pretreatment is to increase the porosity and available surface area for the enzymatic attack (Lv et al., 2018; Zhang et al., 2019). The morphological investigation in the present study showed a significant increase in the porosity and surface area after the pretreatment, thus contributing to the enhancement of subsequent enzymatic hydrolysis (Remli et al., 2014; Lv et al., 2018; Zhang et al., 2019).
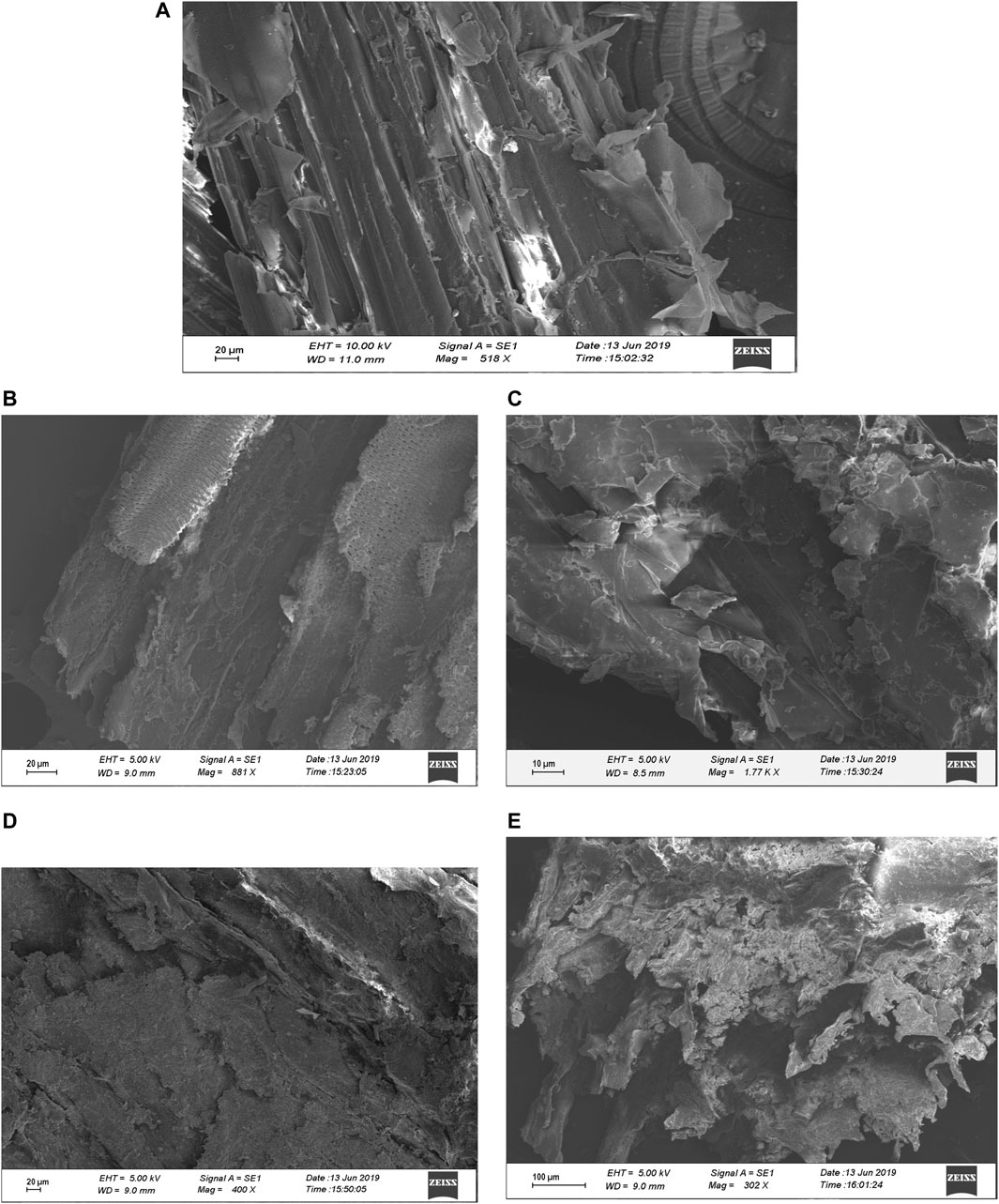
FIGURE 3. Scanning electron microscopic (SEM) analysis of sugarcane bagasse (SB). (A) Showing the surface image of untreated sugarcane bagasse, (B) 10% sulfuric acid-pretreated bagasse at 121°C for 30 min, (C) 10% sulfuric acid-pretreated bagasse at 121°C for 60 min, (D) 10% sodium hydroxide-pretreated bagasse at 121°C for 30 min, and (E) 10% sodium hydroxide-pretreated bagasse at 121°C for 60 min. SB, sugarcane bagasse; SEM, scanning electron microscopy.
3.3 Enzymatic Hydrolysis
Enzymatic hydrolysis of pretreated sugarcane bagasse was carried out by using cellulase and xylanase filtrates of Pseudomonas sp. CVB-10 and Bacillus paramycoides T4. Five different types of processed bagasse samples (untreated sugarcane bagasse, bagasse pretreated with 5 and 10% NaOH at 121°C for 60 min, and bagasse pretreated with 5% and 10% H2SO4 at 121°C for 60 min) were used for enzymatic saccharification. The various parameters such as hydrolysis time, substrate concentration, temperature, pH, enzyme ratio, and different concentrations of Tween-20 were optimized to achieve maximum saccharification of bagasse. All data are graphically represented in Figures 4A–F. A maximum of 489.50 mg/g glucose was obtained from the alkali-pretreated sugarcane bagasse after 30 h of enzymatic hydrolysis. Acid-pretreated bagasse (cellulignin) showed only 322.75 g/L sugar recovery proving the requirement of alkali-mediated delignification (Figures 4A–F). Chandel et al. (2014) also reported that the alkali-pretreated substrate showed maximum saccharification and reducing sugar production.
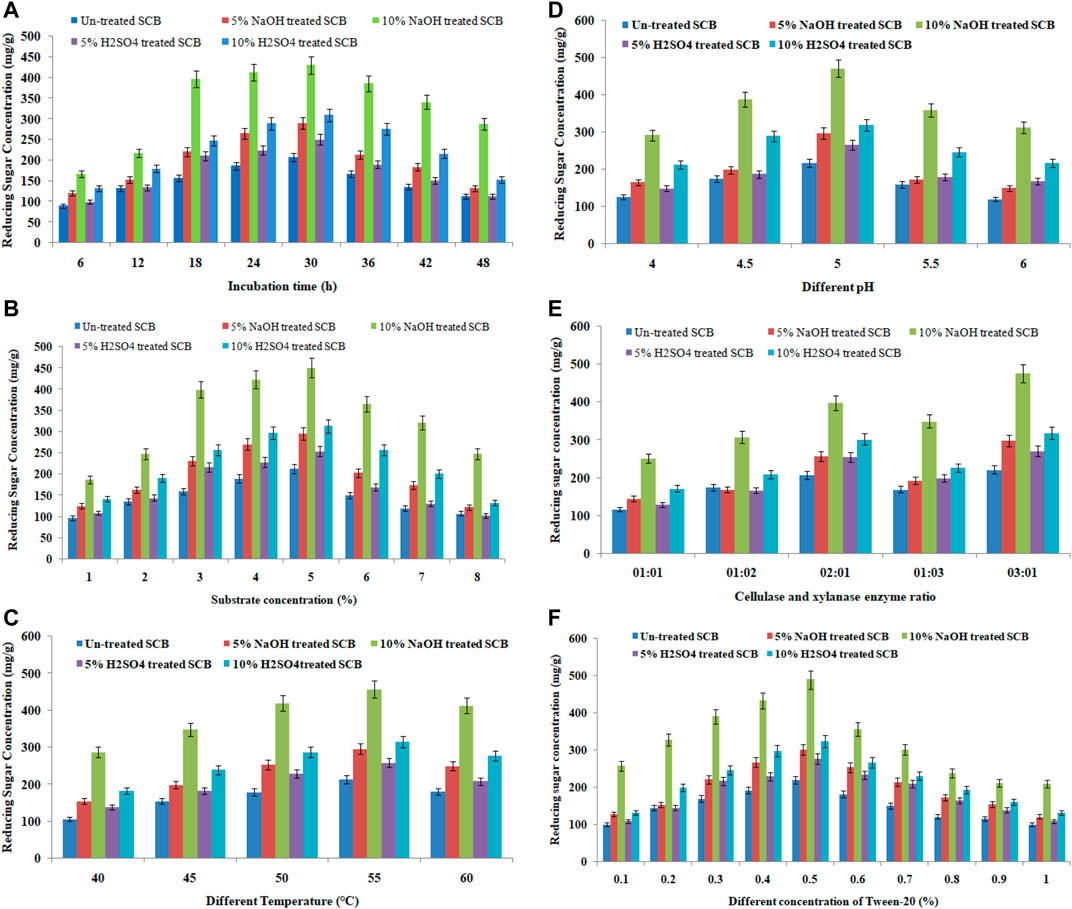
FIGURE 4. Reducing sugar yield of untreated, acid- (5 and 10% H2SO4 at 121°C for 60 min), and alkali-pretreated (5 and 10% NaOH at 121°C for 60 min) SB (sugarcane bagasse) after enzymatic hydrolysis. The hydrolysis was carried out using Pseudomonas sp. CVB-10 and Bacillus paramycoides T4 enzymes (cellulase and xylanase) with an enzyme load of 25 FPU/g. (A) Effect of different incubation time on enzymatic hydrolysis at 55°C, pH 5.0, and 2% substrate concentration. (B) Effect of different substrate concentration on enzymatic hydrolysis at 55°C, pH 5.0 for 30 h. (C) Effect of different temperature on enzymatic hydrolysis at pH 5.0, 5% substrate concentration for 30 h (D) The effect of different pH on enzymatic hydrolysis at 55°C and 5% substrate concentration for 30 h. (E) Effect of the different enzyme ratio on enzymatic hydrolysis at 55°C, pH 5.0, and 5% substrate concentration for 30 h. (F) Effect of different concentration of Tween-20 on enzymatic hydrolysis at 55°C, pH 5.0, 5% substrate concentration, and 3:1 enzyme concentration for 30 h.
Incubation time also influences the saccharification/hydrolysis rate of the untreated and pretreated sugarcane bagasse. From the result, it is clear that the concentration of the released reducing sugar was increased as the reaction time was increased (Figure 4A). A maximum of 430.95 mg/g reducing sugar with a maximal saccharification was obtained from pretreatment with 10% NaOH at 121°C followed by 10% H2SO4 (309.9 mg/g), 5% NaOH (289.6 mg/g), and 5% H2SO4 (250.67 mg/g) at 121°C within 30 h of enzymatic hydrolysis. The content of reducing sugar was gradually decreased after 30 h of incubation. This might be due to the inhibition of the enzyme activity by the accumulated hydrolysis products.
Enzyme saccharification/hydrolysis of samples was also affected by different concentrations (1.0–8.0%) of the substrate. The results showed that the maximum of 450.78 mg/g reducing sugar with maximum saccharification was achieved at 5% substrate concentration (10% alkali-pretreated sugarcane bagasse) within 30 h (Figure 4B). Above and below of this substrate concentration, the enzymatic saccharification rate and hydrolysis rate were decreased gradually. Similarly, Gupta et al. (2011) also reported that maximum reducing sugar production/saccharification was reported at 5% substrate concentration.
Temperature is an important factor, which influences not only the enzymatic reaction but also the activity of the cellulase and xylanase. Generally, as the temperature is raised to a certain range, the enzymatic activity is accelerated. The enzyme-catalyzed reaction, like most chemical reactions, proceeds at a faster velocity as the temperature is increased. The optimal reaction temperature for cellulase and xylanase is between 45°C–55°C. In this experiment, the maximum reducing sugar (456.87 mg/g substrate) with the maximum saccharification rate was observed at 55°C from alkali-pretreated sugarcane bagasse (10%) (Figure 4C). Above and below to the optimal temperature (55°C), the saccharification rate and reducing sugar concentration were reduced. Thus, the optimal temperature for enzymatic saccharification/hydrolysis was 55°C. Lamounier et al. (2018) also reported that maximum reducing sugar production was during saccharification at 55°C. Further increased temperature beyond 55°C, the concentration of reducing sugar and the saccharification rate were reduced. Thus, the optimal temperature for enzymatic saccharification/hydrolysis was 55°C.
The initial pH condition also influences enzymatic saccharification/hydrolysis. Maximum reducing sugar/saccharification was achieved at pH 5.0 from alkali-pretreated bagasse. When pH was increased or decreased than 5.0, the enzymatic reaction was reduced (Lai and Idris 2016). The initial pH change may result in the failure of cellulase and xylanase activity, or dissociation may occur between the substrate and active site of the enzyme, thus making the enzyme-catalyzed hydrolysis reaction to achieve maximal activity of the enzyme (Shuler and Kargi 1992; Lai and Idris 2016). For these reasons, enzymes are only active over a certain pH range.
The enzyme ratio also influences enzymatic saccharification/hydrolysis of pretreated sugarcane bagasse. Different enzyme ratios (cellulase: xylanase) 1:1, 1:2, 1:3, 2:1, and 3:1 were used for enzymatic saccharification/hydrolysis of pretreated samples. The highest amount of 476.9 mg/g of reducing sugar with maximum saccharification was obtained after 30-h reaction when the enzyme ratio was at 3:1. It was then followed by the enzyme ratios 2:1, 1:3, 1:2, and 1:1. The findings illustrated that the enzyme ratios of 2:1 and 3:1 produced higher amounts of reducing sugar than others. Similarly, Lai and Idris (2016) also reported that the 5:1 ratio of cellulase: β-glucosidase showed maximum glucose production.
The surfactant also influences the enzymatic hydrolysis at different concentrations by increasing the surface area of the substrate. In this experiment, different concentrations (0.1–1.0%) of Tween-20 were optimized for maximum saccharification under all optimized conditions. Figure 4F depicted that the maximum 489.50 mg/g reducing sugar with the maximum saccharification rate was achieved at 0.5% Tween-20 concentration. Above and below this concentration, there was no significant result reported from the surfactant. Surfactants generally enhance the surface area of lignocellulosic substrates to improve the extent of enzymatic hydrolysis. Non-ionic surfactant-like Tween 20 is more effective due to its adsorption on hydrophobic surfaces mainly composed of lignin fragments (Santos et al., 2011; Chandel et al., 2014).
4 Conclusion
Several things such as the lignin and hemicellulose composition, surface areas, crystallinity, and degree of polymerization influenced the sugarcane bagasse decomposition by cellulases and xylanases. The main aim of acid and alkali pretreatment is to remove the lignin and hemicellulose content, reduce cellulose crystallinity, and enhance the porosity. Hence, a suitable pretreatment method plays a key role in enhancing the competence of cellulose and hemicellulose hydrolysis. The acid and alkali pretreatment methods were applied in this study effort to raise the substrate’s surface area, reduce the lignin and hemicellulose content, and disorder the polymerization of sugarcane bagasse. Two different concentrations of acid and alkali were applied for pretreatment of sugarcane bagasse. Among different concentrations of acid and alkali pretreatments studied, the 10% NaOH pretreatment was found to be the most competent in lignin removal and led to the enhancement of the cellulose and hemicellulose content in pretreated sugarcane bagasse. This treatment technique recommends the opportunity of producing a cellulosic material largely free from lignin, which ultimately would be a good substrate for bioethanol generation. However, there is a need to build up proficient, biological, and delignification techniques to formulate the eco-friendly process. The FTIR, XRD, and SEM analyses showed the best results with 10% NaOH pretreatment followed by 10% H2SO4, 5% NaOH, and 5% H2SO4 pretreatment as the most efficient in terms of altering the morphology of sugarcane bagasse. Overall, maximum saccharification/hydrolysis of 10% NaOH-pretreated sugarcane bagasse generated 498.5 mg/g reducing sugar after 30 h, whereas hydrolysis of untreated sugarcane bagasse generated only 219.4 mg/g reducing sugar.
Data Availability Statement
The datasets presented in this study can be found in online repositories. The names of the repository/repositories and accession number(s) can be found below: https://www.ncbi.nlm.nih.gov/nucleotide/MK443365.1 and https://www.ncbi.nlm.nih.gov/nuccore/MN370035.1.
Author Contributions
ST carried out the research work and drafted the manuscript. JY and RG designed the experiment, contributed substantially to analysis and interpretation of data, and gave final approval of the version to be published. JSY performed the molecular characterization of the isolates and analysed the data. TV and RS improved the manuscript language. PKP and SKR critically reviewed and revised the manuscript. All the authors read and approved the final version of the manuscript.
Funding
ST and PKP express their gratitude to the University Grants Commission, New Delhi (UGC-DSKPDF No.F.4-2/2006 BSR/BL/17-18/0049 and UGC-DSKPDF No.F.4-2/2006 BSR/BL/17-18/0442) for providing financial assistance. RS would like to thank the department of Higher Education, Government of Uttar Pradesh, India for the financial support (47-2021/606/77-4-2021-4/56/2020) under the scheme of Research and Development of State Universities of UP.
Conflict of Interest
The authors declare that the research was conducted in the absence of any commercial or financial relationships that could be construed as a potential conflict of interest.
Publisher’s Note
All claims expressed in this article are solely those of the authors and do not necessarily represent those of their affiliated organizations, or those of the publisher, the editors, and the reviewers. Any product that may be evaluated in this article, or claim that may be made by its manufacturer, is not guaranteed or endorsed by the publisher.
Acknowledgments
Dr. D. S. Kothari PDF is greatly acknowledged by all authors.
Abbreviations
CMC, carboxymethyl cellulose; CrI, crystalline index; FTIR, fourier transform infrared; HMF, hydroxymethylfurfural; HPLC, high-performance liquid chromatography; KBr, potassium bromide; SEM, scanning electron microscopy; XRD, X-ray diffraction.
References
Ang, T. N., Ngoh, G. C., Chua, A. S. M., and Lee, M. G. (2012). Elucidation of the Effect of Ionic Liquid Pretreatment on rice Husk via Structural Analyses. Biotechnol. Biofuels. 5, 67. doi:10.1186/1754-6834-5-67
Bartos, A., Anggono, J., Farkas, Á. E., Kun, D., Soetaredjo, F. E., Móczó, J., et al. (2020). Alkali Treatment of Lignocellulosic Fibers Extracted from Sugarcane Bagasse: Composition, Structure, Properties. Polym. Test. 88, 106549. doi:10.1016/j.polymertesting.2020.106549
Bi, S., Peng, L., Chen, K., and Zhu, Z. (2016). Enhanced Enzymatic Saccharification of Sugarcane Bagasse Pretreated by Combining O2 and NaOH. Bioresour. Tech. 214, 692–699. doi:10.1016/j.biortech.2016.05.041
Bian, J., Peng, F., Peng, X.-P., Xiao, X., Peng, P., Xu, F., et al. (2014). Effect of [Emim]Ac Pretreatment on the Structure and Enzymatic Hydrolysis of Sugarcane Bagasse Cellulose. Carbohydr. Polym. 100, 211–217. doi:10.1016/j.carbpol.2013.02.059
Camargo, F. A., Innocentini-Mei, L. H., Lemes, A. P., Moraes, S. G., and Durán, N. (2012). Processing and Characterization of Composites of Poly(3-Hydroxybutyrate-Co-Hydroxyvalerate) and Lignin from Sugar Cane Bagasse. J. Compos. Mater. 46, 417–425. doi:10.1177/0021998311418389
Canilha, L., Chandel, A. K., Suzane dos Santos Milessi, T., Antunes, F. A. F., Luiz da Costa Freitas, W., das Graças Almeida Felipe, M., et al. (2012). Bioconversion of Sugarcane Biomass into Ethanol: An Overview about Composition, Pretreatment Methods, Detoxification of Hydrolysates, Enzymatic Saccharification, and Ethanol Fermentation. J. Biomed. Biotechnol. 2012, 1–15. doi:10.1155/2012/989572
Cao, W., Sun, C., Liu, R., Yin, R., and Wu, X. (2012). Comparison of the Effects of Five Pretreatment Methods on Enhancing the Enzymatic Digestibility and Ethanol Production from Sweet Sorghum Bagasse. Bioresour. Tech. 111, 215–221. doi:10.1016/j.biortech.2012.02.034
Carvalheiro, F., Duarte, L. C., and Girio, F. M. (2008). Hemicellulose Biorefineries: a Review on Biomass Pretreatments. J. Sci. Indus. Res. 67, 849–864. Available at: https://citeseerx.ist.psu.edu/viewdoc/download?doi=10.1.1.461.8516&rep=rep1&type=pdf.
Chandel, A. K., Antunes, F. A., Anjos, V., Bell, M. J., Rodrigues, L. N., Polikarpov, I., et al. (2014). Multi-scale Structural and Chemical Analysis of Sugarcane Bagasse in the Process of Sequential Acid-Base Pretreatment and Ethanol Production by Scheffersomyces Shehatae and Saccharomyces cerevisiae. Biotechnol. Biofuels 7, 63. doi:10.1186/1754-6834-7-63
Chang, V. S., Burr, B., and Holtzapple, M. T. (1997). Lime Pretreatment of Switchgrass. Appl. Biochem. Biotechnol. 63-65, 3–19. doi:10.1007/bf02920408
Costa, C. E., Romaní, A., Cunha, J. T., Johansson, B., and Domingues, L. (2017). Integrated Approach for Selecting Efficient Saccharomyces cerevisiae for Industrial Lignocellulosic Fermentations: Importance of Yeast Chassis Linked to Process Conditions. Bioresour. Tech. 227, 24–34. doi:10.1016/j.biortech.2016.12.016
Demirbas, A. (2011). Competitive Liquid Biofuels from Biomass. Appl. Energ. 88, 17–28. doi:10.1016/j.apenergy.2010.07.016
Falkoski, D. L., Guimarães, V. M., de Almeida, M. N., Alfenas, A. C., Colodette, J. L., and de Rezende, S. T. (2013). Chrysoporthe Cubensis: a New Source of Cellulases and Hemicellulases to Application in Biomass Saccharification Processes. Bioresour. Tech. 130, 296–305. doi:10.1016/j.biortech.2012.11.140
Fasanella, C. C., Montes, C. R., Rossi, M. L., Aguiar, M. M., Ferreira, L. F. R., Pupo, M. M. S., et al. (2018). Microscopic Analysis of Sugarcane Bagasse Following Chemical and Fungal Treatment. Cellulose Chem. Technol. 52, 59–64. Available at: https://www.cellulosechemtechnol.ro/pdf/CCT1-2(2018)/p.59-64.pdf.
Gouveia, E. R., Nascimento, R. T. d., Souto-Maior, A. M., and Rocha, G. J. d. M. (2009). Validação de metodologia para a caracterização química de bagaço de cana-de-açúcar. Quím. Nova 32, 1500–1503. doi:10.1590/s0100-40422009000600026
Guilherme, A. A., Dantas, P. V. F., Santos, E. S., Fernandes, F. A. N., and Macedo, G. R. (2015). Evaluation of Composition, Characterization and Enzymatic Hydrolysis of Pretreated Sugar Cane Bagasse. Braz. J. Chem. Eng. 32 (1), 23–33. doi:10.1590/0104-6632.20150321s00003146
Guo, G.-L., Hsu, D.-C., Chen, W.-H., Chen, W.-H., and Hwang, W.-S. (2009). Characterization of Enzymatic Saccharification for Acid-Pretreated Lignocellulosic Materials with Different Lignin Composition. Enzyme Microb. Tech. 45, 80–87. doi:10.1016/j.enzmictec.2009.05.012
Gupta, R., Khasa, Y. P., and Kuhad, R. C. (2011). Evaluation of Pretreatment Methods in Improving the Enzymatic Saccharification of Cellulosic Materials. Carbohydr. Polym. 84, 1103–1109. doi:10.1016/j.carbpol.2010.12.074
Han, L., Feng, J., Zhang, S., Ma, Z., Wang, Y., and Zhang, X. (2012). Alkali Pretreated of Wheat Straw and its Enzymatic Hydrolysis. Braz. J. Microbiol. 43, 53–61. doi:10.1590/s1517-83822012000100006
Harrison, M. D., Zhang, Z., Shand, K., O’Hara, I. M., Doherty, W. O. S., and Dale, J. L. (2013). Effect of Pretreatment on Saccharification of Sugarcane Bagasse by Complex and Simple Enzyme Mixtures. Bioresour. Tech. 148, 105–113. doi:10.1016/j.biortech.2013.08.099
Hofsetz, K., and Silva, M. A. (2012). Brazilian Sugarcane Bagasse: Energy and Non-energy Consumption. Biomass and Bioenergy 46, 564–573. doi:10.1016/j.biombioe.2012.06.038
Karthika, K., Arun, A. B., Melo, J. S., Mittal, K. C., Kumar, M., and Rekha, P. D. (2013). Hydrolysis of Acid and Alkali Presoaked Lignocellulosic Biomass Exposed to Electron Beam Irradiation. Bioresour. Tech. 129, 646–649. doi:10.1016/j.biortech.2012.12.048
Ko, J. K., Um, Y., Woo, H. M., Kim, K. H., and Lee, S.-M. (2016). Ethanol Production from Lignocellulosic Hydrolysates Using Engineered Saccharomyces cerevisiae Harboring Xylose Isomerase-Based Pathway. Bioresour. Tech. 209, 290–296. doi:10.1016/j.biortech.2016.02.124
Kumar, R., Mago, G., Balan, V., and Wyman, C. E. (2009). Physical and Chemical Characterizations of Corn stover and poplar Solids Resulting from Leading Pretreatment Technologies. Bioresour. Tech. 100, 3948–3962. doi:10.1016/j.biortech.2009.01.075
Labbe, N., Rials, T. G., Kelley, S. S., Cheng, Z. M., Kim, J. Y., and Li, Y. (2005). FT-IR Imaging and Pyrolysis-Molecular Beam Mass Spectrometry: New Tools to Investigate wood Tissues. Wood Sci. Technol. 39, 61–77.
Ladeira-Ázar, R. I. S., Morgan, T., Maitan-Alfenas, G. P., and Guimarães, V. M. (2018). Inhibitors Compounds on Sugarcane Bagasse Saccharification: Effects of Pretreatment Methods and Alternatives to Decrease Inhibition. Appl. Biochem. Biotechnol. 188, 29–42. doi:10.1007/s12010-018-2900-6
Lai, L. W., and Idris, A. (2016). Comparison of Steam-Alkali-Chemical and Microwave-Alkali Pretreatment for Enhancing the Enzymatic Saccharification of Oil palm Trunk. Renew. Energ. 99, 738–746. doi:10.1016/j.renene.2016.07.059
Lamounier, K. F. R., Rodrigues, P. O., Pasquini, D., and Baffi, M. A. (2018). Saccharification of Sugarcane Bagasse Using an Enzymatic Extract Produced by Aspergillus fumigatus. J. Renew. Mat. 6 (2), 169–175. doi:10.7569/jrm.2017.634151
Lv, X., Lin, J., Luo, L., Zhang, D., Lei, S., Xiao, W., et al. (2018). Enhanced Enzymatic Saccharification of Sugarcane Bagasse Pretreated by Sodium Methoxide with Glycerol. Bioresour. Tech. 249, 226–233. doi:10.1016/j.biortech.2017.09.137
Mood, S. H., Golfeshan, A. H., Tabatabaei, M., Abbasalizadeh, S., and Ardjmand, M. (2013). Comparison of Different Ionic Liquids Pretreatment for Barley Straw Enzymatic Saccharification. 3 Biotech. 3, 399–406. doi:10.1007/s13205-013-0157-x
Moretti, M. M. d. S., Bocchini-Martins, D. A., Nunes, C. d. C. C., Villena, M. A., Perrone, O. M., Silva, R. d., et al. (2014). Pretreatment of Sugarcane Bagasse with Microwaves Irradiation and its Effects on the Structure and on Enzymatic Hydrolysis. Appl. Energ. 122, 189–195. doi:10.1016/j.apenergy.2014.02.020
Mosier, N., Wyman, C., Dale, B., Elander, R., Lee, Y. Y., Holtzapple, M., et al. (2005). Features of Promising Technologies for Pretreatment of Lignocellulosic Biomass. Bioresour. Tech. 96, 673–686. doi:10.1016/j.biortech.2004.06.025
Nakagame, S., Chandra, R. P., Kadla, J. F., and Saddler, J. N. (2011). The Isolation, Characterization and Effect of Lignin Isolated from Steam Pretreated Douglas-fir on the Enzymatic Hydrolysis of Cellulose. Bioresour. Tech. 102, 4507–4517. doi:10.1016/j.biortech.2010.12.082
Nelson, M. L., and O'Connor, R. T. (1964). Relation of Certain Infrared Bands to Cellulose Crystallinity and crystal Lattice Type. Part II. A New Infrared Ratio for Estimation of Crystallinity in Celluloses I and II. J. Appl. Polym. Sci. 8, 1325–1341. doi:10.1002/app.1964.070080323
Nelson, N. (1944). A Photometric Adaptation of the Somogyi Method for the Determination of Glucose. J. Biol. Chem. 153, 375–380. doi:10.1016/s0021-9258(18)71980-7
Nogales, E. G., and Webber, M. (2017). Territorial Tools for Agro-Industry Development A Source- Book Territorial Tools for Agro-Industry Development. Rome: Food And Agriculture Orga- nization Of The United Nations.
Pandey, K. K., and Pitman, A. J. (2003). FTIR Studies of the Changes in wood Chemistry Following Decay by Brown-Rot and white-rot Fungi. Int. Biodeterioration Biodegradation 52, 151–160. doi:10.1016/s0964-8305(03)00052-0
Ponce, J., Andrade, J. G. d. S., dos Santos, L. N., Bulla, M. K., Barros, B. C. B., Favaro, S. L., et al. (2021). Alkali Pretreated Sugarcane Bagasse, rice Husk and Corn Husk Wastes as Lignocellulosic Biosorbents for Dyes. Carbohydr. Polym. Tech. Appl. 2, 100061. doi:10.1016/j.carpta.2021.100061
Qing, Q., Hu, R., He, Y., Zhang, Y., and Wang, L. (2014). Investigation of a Novel Acid-Catalyzed Ionic Liquid Pretreatment Method to Improve Biomass Enzymatic Hydrolysis Conversion. Appl. Microbiol. Biotechnol. 98, 5275–5286. doi:10.1007/s00253-014-5664-0
Quintero, J. A., Montoya, M. I., Sánchez, O. J., Giraldo, O. H., and Cardona, C. A. (2008). Fuel Ethanol Production from Sugarcane and Corn: Comparative Analysis for a Colombian Case. Energy 33, 385–399. doi:10.1016/j.energy.2007.10.001
Remli, N. A. M., Shah, U. K. M., Mohamad, R., and Abd Aziz, S. (2014). Effects of Chemical and thermal Pretreatments on the Enzymatic Saccharification of rice Straw for Sugars Production. BioResour 9, 510–522. doi:10.15376/biores.9.1.510-522
Richardson, S., and Gorton, L. (2003). Characterisation of the Substituent Distribution in Starch and Cellulose Derivatives. Analytica Chim. Acta 497, 27–65. doi:10.1016/j.aca.2003.08.005
Romero, C. M., Arce, G. L. A. F., and Avila, I. (2020). Optimization of Slow Pyrolysis Process Parameters Using a Fixed Bed Reactor for Biochar Yield from rice Husk. Biomass and Bioenergy 132, 105412. doi:10.1016/j.biombioe.2019.105412
Santos, V. T. O., Esteves, P. J., Milagres, A. M. F., and Carvalho, W. (2011). Characterization of Commercial Cellulases and Their Use in the Saccharification of a Sugarcane Bagasse Sample Pretreated with Dilute Sulfuric Acid. J. Ind. Microbiol. Biotechnol. 38, 1089–1098. doi:10.1007/s10295-010-0888-1
Segal, L., Creely, J. J., Martin, A. E., and Conrad, C. M. (1959). An Empirical Method for Estimating the Degree of Crystallinity of Native Cellulose Using the X-ray Diffractometer. Textile Res. J. 29, 786–794. doi:10.1177/004051755902901003
Shi, T., Lin, J., Li, J., Zhang, Y., Jiang, C., Lv, X., et al. (2019). Pre-treatment of Sugarcane Bagasse with Aqueous Ammonia-Glycerol Mixtures to Enhance Enzymatic Saccharification and Recovery of Ammonia. Bioresour. Technol. 289, 121628. doi:10.1016/j.biortech.2019.121628
Shuler, M. L., and Kargi, F. (1992). Bioprocess Engineering: Basic Concepts. 2nd ed. New Jersey: Prentice-Hall.
Sluiter, A., Ruiz, R., Scarlata, C., Sluiter, L., and Templeton, D. (2008). Determination of Extractives in Biomass. Laboratory Analytical Procedure (LAP). NREL, Technical Report.
Somogyi, M. (1952). Notes on Sugar Determination. J. Biol. Chem. 195, 19–23. doi:10.1016/s0021-9258(19)50870-5
Sun, F. F., Zhao, X., Hong, J., Tang, Y., Wang, L., Sun, H., et al. (2016). Industrially Relevant Hydrolyzability and Fermentability of Sugarcane Bagasse Improved Effectively by Glycerol Organosolv Pretreatment. Biotechnol. Biofuels. 9, 59. doi:10.1186/s13068-016-0472-7
Ummalyma, S. B., Sahoo, D., Pudiyamadam, A., Adarsh, V. P., Sukumaran, R. K., Bhaskar, T., et al. (2021). Sono-Assisted Alkali and Dilute Acid Pretreatment of Phragmites Karka (Tall Reed Grass) to Enhance Enzymatic Digestibility for Bioethanol Conversion. Front. Energ. Res. 8, 594452. doi:10.3389/fenrg.2020.594452
Wiercigroch, E., Szafraniec, E., Czamara, K., Pacia, M. Z., Majzner, K., Kochan, K., et al. (2017). Raman and Infrared Spectroscopy of Carbohydrates: a Review. Spectrochimica Acta A: Mol. Biomol. Spectrosc. 185, 317–335. doi:10.1016/j.saa.2017.05.045
Yoon, L. W., Ngoh, G. C., May Chua, A. S., and Hashim, M. A. (2011). Comparison of Ionic Liquid, Acid and Alkali Pretreatments for Sugarcane Bagasse Enzymatic Saccharification. J. Chem. Technol. Biotechnol. 86, 1342–1348. doi:10.1002/jctb.2651
Zhang, H., Huang, S., Wei, W., Zhang, J., and Xie, J. (2019). Investigation of Alkaline Hydrogen Peroxide Pretreatment and Tween 80 to Enhance Enzymatic Hydrolysis of Sugarcane Bagasse. Biotechnol. Biofuels. 12, 107. doi:10.1186/s13068-019-1454-3
Zhao, X., Zhang, L., and Liu, D. (2008). Comparative Study on Chemical Pretreatment Methods for Improving Enzymatic Digestibility of Crofton weed Stem. Bioresour. Tech. 99, 3729–3736. doi:10.1016/j.biortech.2007.07.016
Keywords: FTIR spectroscopy, scanning electron microscopy, X-ray diffraction, acid and alkali pretreatment, sugarcane baggase
Citation: Tiwari S, Yadav J, Gaur R, Singh R, Verma T, Yadav JS, Pandey PK and Rath SK (2022) Multistep Structural and Chemical Evaluation of Sugarcane Baggase, Pretreated With Alkali for Enhancing the Enzymatic Saccharification by Cellulase and Xylanase of the Pseudomonas sp. CVB-10 (MK443365) and Bacillus paramycoides T4 (MN370035) Mix-Culture System. Front. Energy Res. 9:726010. doi: 10.3389/fenrg.2021.726010
Received: 16 June 2021; Accepted: 30 November 2021;
Published: 11 January 2022.
Edited by:
Kalpit V. Shah, RMIT University, AustraliaReviewed by:
Li Qun Jiang, Guangzhou Institute of Energy Conversion (CAS), ChinaRicha Arora, Punjab Agricultural University, India
Copyright © 2022 Tiwari, Yadav, Gaur, Singh, Verma, Yadav, Pandey and Rath. This is an open-access article distributed under the terms of the Creative Commons Attribution License (CC BY). The use, distribution or reproduction in other forums is permitted, provided the original author(s) and the copyright owner(s) are credited and that the original publication in this journal is cited, in accordance with accepted academic practice. No use, distribution or reproduction is permitted which does not comply with these terms.
*Correspondence: Soni Tiwari, c3QxOTc5NUBnbWFpbC5jb20=