- 1Japan Oil, Gas and Metals National Corporation (JOGMEC), Tokyo, Japan
- 2Japan Methane Hydrate Operating Co., Ltd. (JMH), Tokyo, Japan
Even in the carbon-neutral age, natural gas will be valuable as environment-friendly fuel that can fulfill the gap between the energy demand and supply from the renewable energies. Marine gas hydrates are a potential natural gas source, but gas production from deposits requires additional heat input owing to the endothermic nature of their dissociation. The amount of fuel needed to produce a unit of energy is important to evaluate energy from economic and environmental perspectives. Using the depressurization method, the value of the energy return on investment or invested (EROI) can be increased to more than 100 for the dissociation process and to approximately 10 or more for the project life cycle that is comparable to liquefied natural gas (LNG) import. Gas transportation through an offshore pipeline from the offshore production facility can give higher EROI than floating LNG; however, the latter has an advantage of market accessibility. If the energy conversion from methane to hydrogen or ammonia at the offshore facility and carbon capture and storage (CCS) can be done at the production site, problems of carbon dioxide emission and market accessibility can be solved, and energy consumption for energy conversion and CCS should be counted to estimate the value of the hydrate resources.
Introduction
Unlike conventional oil and gas that can be produced by natural flow, combustible hydrocarbon gas extraction from naturally occurring gas hydrates, ice-like solid in the earth crust and stable under high pressure and low temperature, requires some sort of production technology that can consume energy. To bring fuel gas from gas hydrates in geological formation, the solid form hydrocarbon should be dissociated into fluid phase (gas and water) that can move in porous geological formation and in the tubing, but gas hydrate dissociation is an endothermic phenomenon, and thus, the process needs continuous thermal energy input (Makogon, 1997; Sloan, 1998). Even using depressurization method that does not require input of thermal energy, work to bring water from deep underground to surface is required, and energy to operate a pump is required. Those points are different from conventional fluid hydrocarbons (natural gas or oil) that are pressurized and move up to surface as natural flow. If other methods such as thermal stimulation are used, the energy return on investment (EROI) would be much lower than conventional oil and gas. The methane (CH4) produced from the gas hydrate is relatively low carbon emission fuel than other fossil energies; however, it is a fossil energy that emits carbon dioxide (CO2) on combustion. Therefore, the EROI value of gas hydrate production is important from the economic and environmental viewpoints.
Naturally occurring gas hydrate is found in several forms, such as pore-filling hydrates in sandy sediments, bulky hydrate nodules on the seafloor or near the surface, and fault- and fracture-filling hydrates in clayey sediments. Among these, the hydrate deposits in sandy formation in either onshore permafrost or offshore deepwater environments could be the closest target for commercial gas production because currently available technologies for the conventional petroleum productions can be applied (Boswell and Collett, 2006). Moreover, its continuous and concentrated occurrences can give large production volumes from single production and transport facilities such as an offshore platform, well clusters, and a gas pipeline to the shore. Therefore, offshore gas hydrates in deepwater sandy sediments are the highest priority target for resource development from quantity and accessibility viewpoints.
The in-place volume of naturally occurring gas hydrates has been estimated worldwide or regionally. The early estimation counted 1015–1018 m3 in the methane gas volume under the normal condition (Kvenvolden, 1988), but recent estimations are in the range of 1014–1017 m3 (Boswell and Collett, 2011; Johnson, 2011). In the case of the eastern Nankai Trough area in the Pacific coast of Japan, 1.1 × 1012 m3 of methane gas was delineated by seismic surveys with borehole data support (the mean value of a stochastic analysis), and half of the methane was highly concentrated in turbidite sand sediments (Fujii et al., 2008). In the case of Outer Continental Shelf of Gulf of Mexico, among 607 × 1012 m3 of estimated methane volume (mean estimate) in the gas hydrate form, 190 × 1012 m3 was evaluated to reside in the sandstone reservoir (Frye, 2008). In the Alaska North Slope, the methane gas volume within gas hydrate reservoirs was estimated as 2.42 × 1012 m3. The gas hydrate deposits in the South China Sea has unique features in which gas hydrates exist in the pore spaces of finer silty sediments and the estimated gas volume is 64.6 × 1012 m3 (Zhu et al., 2021). Recoverable reserves from the in place gas volume would be limited by technical, economic and environmental reasons. EROI is one of the dominant factors to estimate the value of gas hydrates as a fuel resource. The value of EROI depends on the production technique and systems to realize the technique.
Even in the carbon-neutral age, natural gas will be valuable as an environment-friendly fuel that can fulfill the gap between the energy demand and supply from the renewable energies. In this article, how much EROI can be expected for each type of production method and system, and its ecological value in the future carbon neutral society are discussed.
Heat Demand and Output by Gas Production Methods
Production Techniques and Theoretical and Laboratory Evaluation
To produce methane from the marine gas hydrate in sandy sediments, in situ dissociation via depressurization or thermal stimulation can be the most realistic method, because the existing techniques and equipment used in deepwater petroleum production can be applied, and environmental footprints at the seafloor are limited within the vicinity of the drilled borehole. However, due to the endothermic nature of gas hydrate dissociation, difference of two methods can be regarded as the difference of heat source; the thermal stimulation needs an artificial heat source, but the depressurization uses sensible heat of sediments. Figures 1, 2 illustrate the basic concept of two methods with heat and mass fluxes. We try to calculate the energy consumption necessary for each method.
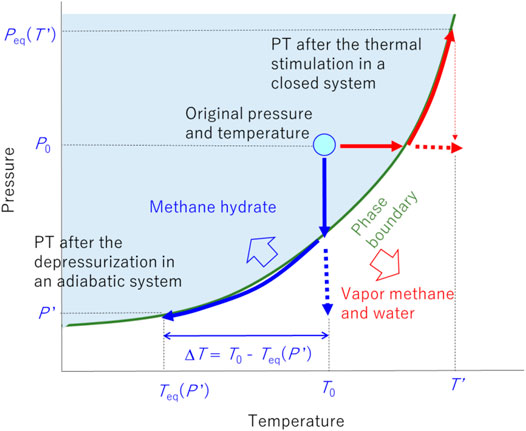
FIGURE 1. Phase equilibrium line and PT changes during the application of the “depressurization method (blue arrows)” and the “thermal stimulation method (red arrows).”
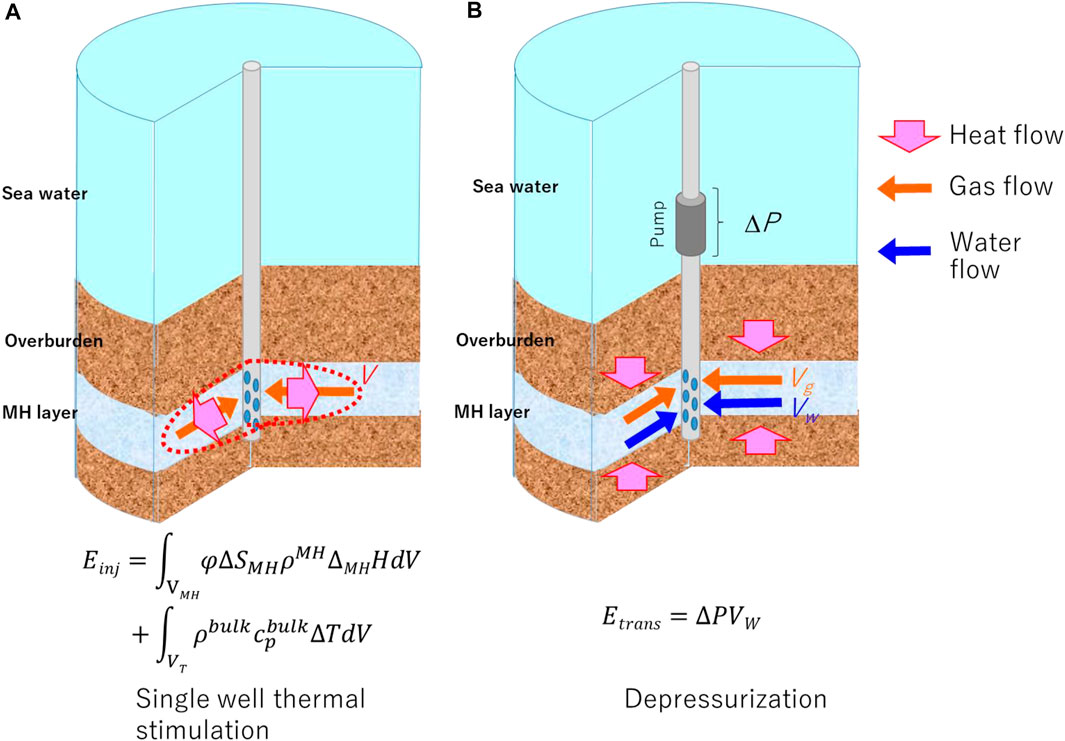
FIGURE 2. Thermal energy injection for the “thermal stimulation method” (Einj) and work to displace water to realize the “depressurization method” (Etrans). In the case of the “depressurization method,” the necessary thermal energy is supplied from the explicit heat of the initial and phase equilibrium temperature, as shown in Figure 1.
The 1-kg structure I methane hydrate [nCH4⋅5.75H2O or CH4⋅m5.75H2O, where n is a cage occupancy and has a value around 0.95, and m is a hydrate number and its measured value from the natural sample is 6.1 (Kida et al., 2015)] contains 8.47-mol CH4, and the latent heat of gas hydrate dissociation ΔMHH is approximately 437 kJ (Anderson, 2004); depending on cage occupancy conditions, gas hydrates were formed. This value is the minimum thermal energy demand for dissociating the unit mass of the gas hydrate. When the produced gas is combusted, the enthalpy of the combustion ΔcH° is 6,800 kJ; therefore, the maximum ratio of producible energy to the heat demand ΔcH°/ΔGHH is around 15.6.
If the thermal stimulation method is applied, the necessary thermal input is not only the dissociation heat but also the heat to increase the formation temperature that contains sand particles and pore fluids as well as gas hydrates. The heated region can extend beyond the targeted gas hydrate-bearing sediment, and the heat can escape to the surrounding formations by conduction and advection, so more heat input should be necessary. The necessary energy input Einj can be written as follows:
where φ is the porosity, VMH is the dissociated hydrate, ΔSMH is the change in the gas hydrate saturation (volume fraction of the gas hydrate in pore space), ρMH and ρbulk are densities of the gas hydrate and bulk sediment (sand, pore fluid, and gas hydrate),
The volume of the influenced domain VT highly depends on the process of thermal stimulation, and to maximize EROI of thermal stimulation, VT should be minimized by reducing the escaping heat from the gas hydrate dissociation domain VMH. There can be three different schemes of thermal stimulation, namely, 1) single borehole conduction type, 2) single borehole huff and puff type (a method of cyclic hot fluid injection and gas production), and 3) multi-well hot water flooding type (Figure 3). The simplest single borehole conduction type stimulation has a problem that the direction of heat flow is opposite to the fluid flow direction so the heat transport far away from the borehole is difficult. Other two methods can be regarded as a combination of the depressurization and thermal stimulation techniques; they can be used for sorts of enhanced recovery measures and depressurization is the primary recovery measure with natural thermal energy. It is important to apply an effective heat source and to reduce loss in the heat generation process. Electrical heating (Callarotti, 2011; Minagawa et al., 2018), an application of heat pump to collect sea water temperature (Mori et al., 2012), geothermal energy (Japan Drilling Company, 2009), and backfilling of chemical heat sources (Xu et al., 2021) have been proposed. Among those techniques, Callarotti (2011) estimated EROI of the thermal stimulation with electric heating using analytical solutions and obtained as in the range of 4–5 without considering the efficiency of power generation. For the hot water injection, the estimation of EROI by Callarotti (2012) began at a value of 28 as the water starts flowing and reduced to a value of 2.8 after fifty years of production. Alternatively, if the reservoir pore pressure can be dropped by displacing water from the reservoir to the surface through a borehole, the phase equilibrium temperature of the gas hydrate and vapor methane is dropped, and explicit heat between the original formation temperature and phase equilibrium temperature (ΔT = T0−Teq(P′), where Teq(P′) is the phase equilibrium temperature after depressurization from the original pressure P0 to P′) can be used for the gas production. The necessary artificial energy input to realize the depressurization method Etrans is the work needed to displace water from the reservoir to the surface to establish the depressurized situation and can be written as follows:
where Vw is the volume of the produced water, ρW is the density of water, Δz is the head of the displaced water that is equivalent to the degree of depressurization ΔP at the bottom of the well, and g is the gravitational acceleration. This equation is based on the idea that all of the water should be transported by a pump, but natural gas lift effect (buoyancy of the produced gas bubbles hold up liquid) can reduce the value of Etrans can be reduced. Such phenomena were observed in some field cases, as stated later.
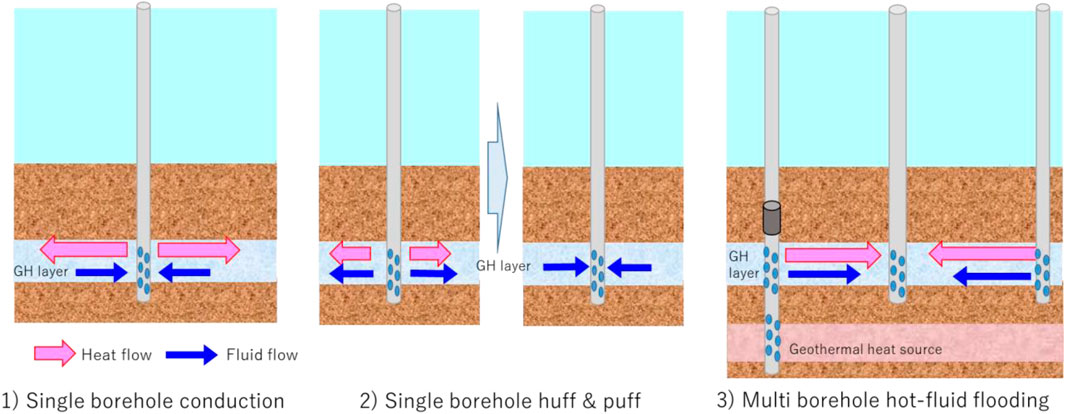
FIGURE 3. Possible thermal stimulation options with heat and fluid flows involved. To minimize the heat that is not involved into the gas hydrate dissociation is the key to maximize EROI of the depressurization method.
From 1-kg gas hydrate (methane hydrate), 0.95 × 10–3 m3 of water is produced, and if the degree of the drawdown is 10 MPa, the energy necessary to transport the water from the reservoir to the surface is Etrans = 9.31 kJ, and EROI of the depressurization method, which is written as ΔcH°/Etran, is approximately 730. In the real situation, water from the gas hydrate dissociation and associated original pore fluid should be produced, so EROI should be less than the theoretical value of 730. This means that EROI of the depressurization method is governed by the gas to water volume ratio (GWR), and GWR should be less than the theoretical value (approximately 200) due to the water from the original pore fluid. Therefore, how to reduce the excess water production will be the key technology, but the situation of water production highly depends on the geological conditions such as the existence of low gas hydrate saturation streaks, and fluid conduits such as faults and fractures. The current Japanese gas hydrate resource research and development plan gives the study on the excess water management high priority (Yamamoto et al., 2021a). Some physical barriers to stop the migration of unnecessary water are possible ideas, and biotechnical barriers using in-situ microble (Hata et al., 2020) and waterglass (sodium silicate, Ito et al., 2014) may reduce the permeability in the broader domain far away from the borehole because the treatment media do not contain solid particles and then penetrate the formation easily. As a different idea, Gao et al. (2021) proposed to manage the water production by optimizing the depressurization process using data from laboratory experiments. Guo et al. (2020) proposed that the stepwise control of the depressurization process can decrease the water production, with enhancement of gas production by thermal stimulation. For the actual field development, both soft (operational) and hard (physical) measures to control water production should be quite important for maintaining high EROI. Moreover, geophysical techniques to identify the water-bearing zone is necessary. The combination of the seismic and geophysical log was useful to identify the water-producing streaks during the offshore production test (Yamamoto et al., 2019), and this knowledge should be useful for the design or production process and application of countermeasures.
In the case of the depressurization method, methane productivity from gas hydrate deposits solely depends on its natural condition and is hardly controllable. Kurihara et al. (2004) conducted a parametric study on gas productivity using a numerical simulator and revealed that the formation temperature and intrinsic and initial effective permeability (permeability of the sediment without gas hydrate and permeability before hydrate dissociation, respectively) with initial formation temperature are the dominant factors that control the effectiveness of the depressurization method.
In both thermal stimulation and depressurization cases, the real energy efficiencies in the reservoir are dominated by the heat transport in the formations and highly dependent on the employed technologies and reservoir characters, and detailed numerical modeling works are required to obtain the values. Kurihara et al. (2008) and Nagao (2015) provided a comparison of EROIs and recovery rates among three production techniques using numerical simulation applied to model three Nankai Trough gas hydrate reservoirs and found that the thermal situation that could give relatively high recovery rate could realize EROI less than 1 so the energy deficit should happen (Figure 4). On the contrary, the depressurization technique could bring positive energy from the output to the input, and a combined technique of depressurization and thermal stimulation (huff and puff technology, or recurrent cycles of hot fluid injection and pressure drawdown) was at the intermediate position. In the calculation, some energy losses of each production technique were assumed. Other main factors that governed the efficiency and recovery rates were reservoir temperature and formation permeabilities.
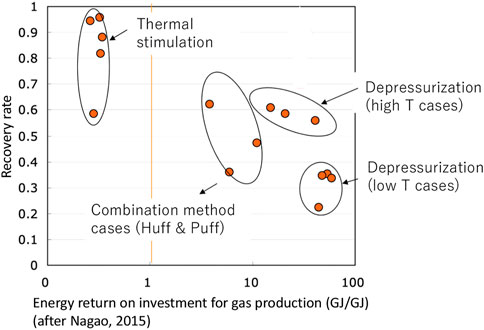
FIGURE 4. Estimated recovery rate and energy return on investment of each of the production technologies (depressurization, combination method, and thermal stimulation) at three Nankai Trough gas hydrate reservoirs with different temperatures and pressures evaluated by numerical simulations (Kurihara et al., 2008; Nagao, 2015). The recovery rate by the depressurization method was governed by the formation temperature along with permeability of the formations.
Because the calculation of the energy input necessary for the production is complicated coupled phenomena of mass and heat flow happening in heterogeneous geological formation, the reliability of the result depends on the accuracy of the numerical method, kinetic and reservoir characteristics models applied. Intensive model comparison efforts have been conducted as international efforts (Wilder et al., 2008; White et al., 2020) and by Yin et al. (2016). Song et al. (2016) made laboratory observation of each process using magnetic resonance imaging (MRI), and the combined method of both depressurization and thermal stimulation establishes both recovery rate and productivity.
Field Records
The world’s first intended gas production from gas hydrate deposits was made in the Mallik site, Mackenzie Delta, Northwest territories, Canada, in 2002, and 468 m3 of gas was produced during the five-day thermal stimulation operation with hot fluid circulation (Dallimore and Collett, 2005; Hancock et al., 2005), and slightly positive energy return was observed.
Two onshore gas production projects by depressurization were conducted in the arctic area of North America. One was done in the same site of 2002 project in Canada in 2007–2008 (Dallimore et al., 2012; Ashford et al., 2012). The other project done in Alaska North Slope in 2012 (Iġnik Sikumi) consists CH4–CO2 exchange trial and depressurization (Boswell et al., 2017). The twice offshore gas production tests were conducted in the eastern Nankai Trough of Japan (the first in 2013 and the second in 2017) and the produced gas and water volumes were recorded with a degree of depressurization. The values of EROI of each depressurization operations were calculated applying production histories (gas and water production rates and pressure drawdown records) into Eq. 2 and combustion heat of the produced methane, and it was found that the range of EROIs was between 250 and 600 in offshore test cases and 600 to 1,300 in the onshore test cases (Figure 5). However, any loss because of pump efficiency, hydraulic friction in the flow line, and enhancement of liquid transport by the gas lift effect was not counted in the calculation. On the other hand, energy input for water transport Etrans of Mallik 2008 and Nankai Trough AT1-P and AT1-P2 cases could be less than the value in Eq. 2 because of unintended gas lift effects that were observed as gas flow from water production line of water production from the gas flow line.
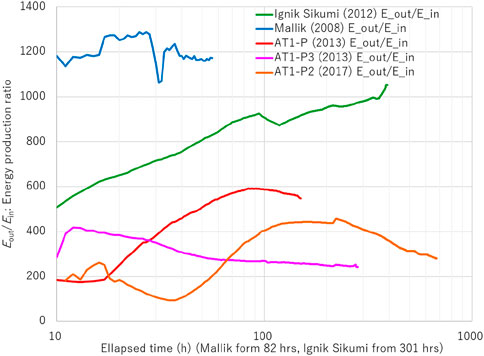
FIGURE 5. Energy return (combustion heat from the produced gas, Eout) on investment as a work of the pump to displace water (Ein) of the offshore production test wells (AT1-P, P2, and P3) in the eastern Nankai Trough (after Yamamoto et al., 2019).
The main reason for the difference between the onshore and offshore cases is different GWRs. In the offshore test cases, GWRs were less than 100, but onshore test data gave from 100 to 200 of GWR so that works needed to bring water to surface were relatively small. The fact shows that the energy efficiency of gas production from the gas hydrate reservoir relies on how water production volume is limited.
Life Cycle EROI
Gas Production System Scenarios
Energy consumption for the gas production stated in the former section (HEAT DEMAND AND OUTPUT BY GAS PRODUCTION METHODS) did not consider any indirect energy demand for exploration, construction of facilities, indirect operational demands such as utility for the production facilities, transport of gas from the production site to shore, and treatment and disposal of produced water, plug and abandonment of boreholes, and decommissioning of the facilities that are required in the entire life cycle of the commercial gas production.
A Japanese research team of MH21 tried counting all those demands to evaluate the life cycle of EROI of the gas production system (Research Consortium for Methane Hydrate Resources in Japan, 2019) and to evaluate the economic conditions that allow the commercial production of gas from gas hydrates. For the evaluation, the considered gas production system contains boreholes; subsea facilities such as electric submersible pumps, subsea well heads, manifold, topside facilities of the offshore production platform (semi-submersible is assumed), and devices on it for liquefaction; and gas pipelines and compressors. The considered system terminal is at the shore where gas is handed to the consumers, and thus, the energy demand and loss during the energy conversion (power generation, etc.) and delivery to final consumers were not counted.
In the study, three offshore gas production and transport systems, namely, 1) offshore platform, 2) floating LNG (FLNG), and 3) long tie-back from the subsea production system to the shore (LTB), were modeled (Figure 6). Three scale reservoir models (A (small, 0.83 trillion cubic feet (tcf) of gas in place), B (large, 2.73 tcf), and C (medium, 1.02 tcf)) represent some existing gas hydrate-concentrated areas around Japan. The MH21-developed numerical simulator (MH21 Hydres; Masuda et al., 2005 and Kurihara et al., 2009) could give the gas and water production rates from single borehole in each reservoir considering site-specific reservoir data such as resource volumes, temperature, and permeability. For users, the gas supply should be at a stable rate for a sufficiently long term. To realize this situation under the condition of gas hydrate wells with relatively short lifetime and small drainage area, well clusters should be drilled repeatedly to make the plateau of the gas production rate. This situation is illustrated in Figure 7 (Research Consortium for Methane Hydrate Resources in Japan, 2019). Figure 7A illustrates an example of calculated gas and water rates from single borehole using the numerical simulator MH21 Hydres and one of the assumed reservoir models mentioned above. The produced gas was combined well to well and time to time into total gas supply to match with the gas demand as shown in Figure 7B.
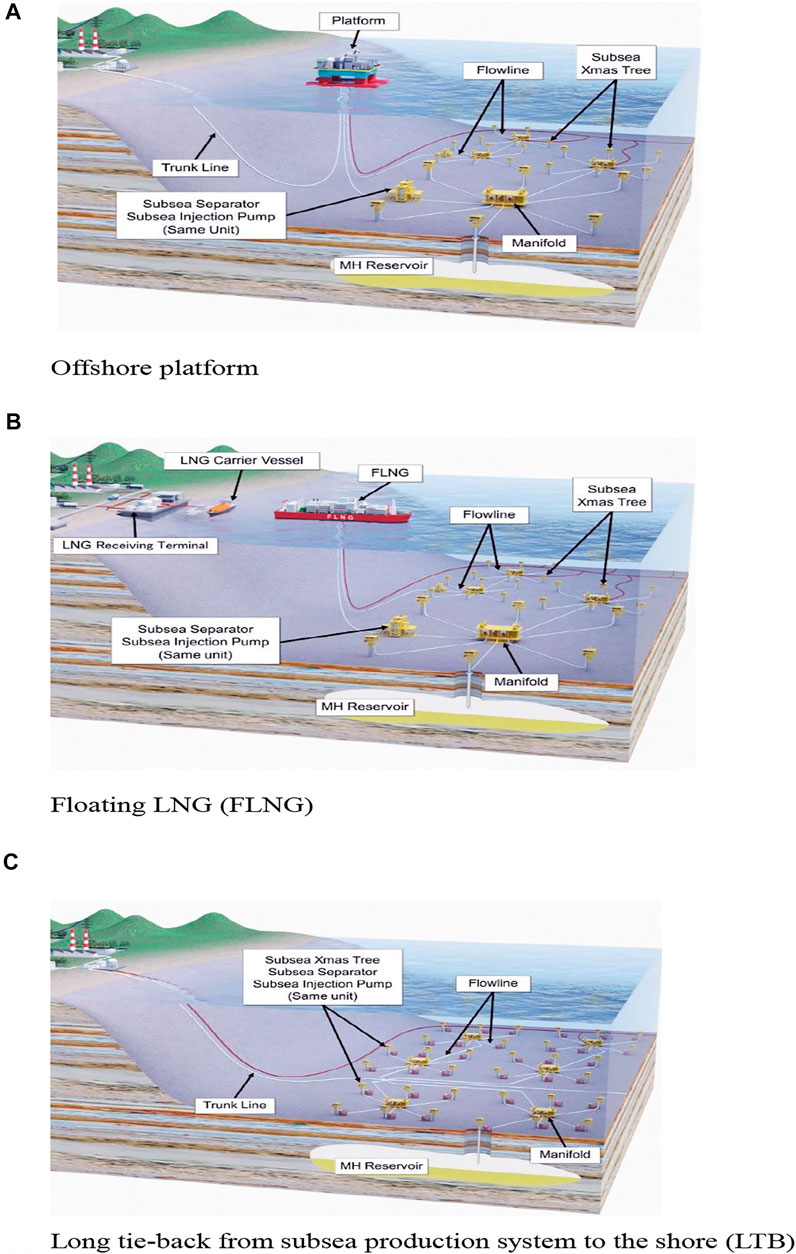
FIGURE 6. Three concepts of offshore gas production and the transport system. (A) Offshore platform; (B) floating LNG (FLNG); (C) long tie-back from the subsea production system to the shore (LTB)
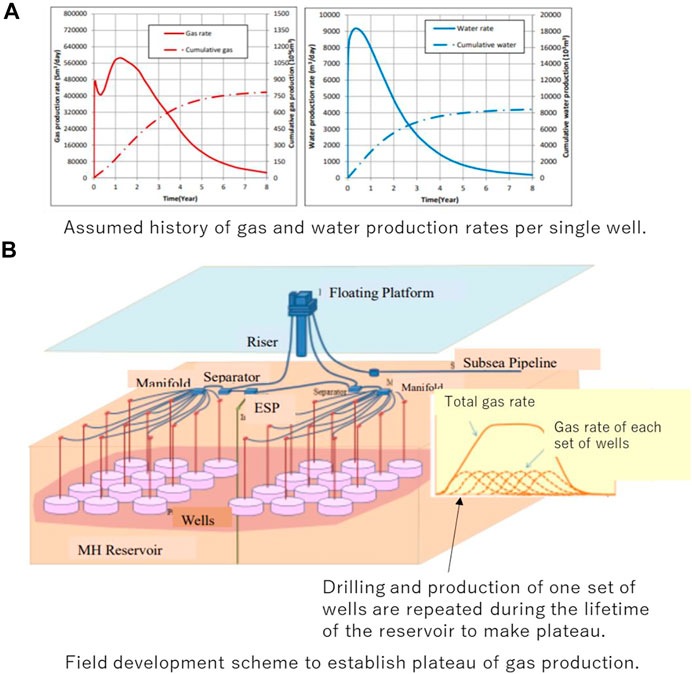
FIGURE 7. (A) Example of gas and water production behavior calculated by the numerical simulator MH21-Hydres and an assumed reservoir model, and (B) schematic of the well drilling plan to make the plateau of the total production rate. (Modified from Research Consortium for Methane Hydrate Resources in Japan, 2019).
Calculation of the Energy Return on Investment
For the above situation, EROI for each scenario was calculated and was compared with other energy sources. The gas and energy produced by combustion and the EROI numerator were given from the numerical simulator. The energy consumption of the life cycle is the sum of the total energy demand that is divided into the capital energy demand (exploration, manufacturing, and construction of whole facilities such as an offshore platform, subsea devices, pipeline, transportation of the devices from factory to the site, drilling of wells, plugging and abandonment of the wells, and decommissioning of the facilities) and operational demand (which includes electricity to operate pumps and any facilities such as the platform itself and liquefaction facility, and gas compressor to transport gas to shore). Consumed fuels, energy to create materials, and other operational and capital energy demands are counted to produce a unit energy output.
Figure 8 shows the structure of energy demand for energy production from various fuels. According to the figure, major portions of the energy demand of the gas hydrate case are for production operations (depressurization) and transportation (compression of gas). To calculate the energy demand, the systems for conventional resources were used for reference, but optimization for the gas hydrate should be necessary, and the current estimations have room for improvement.
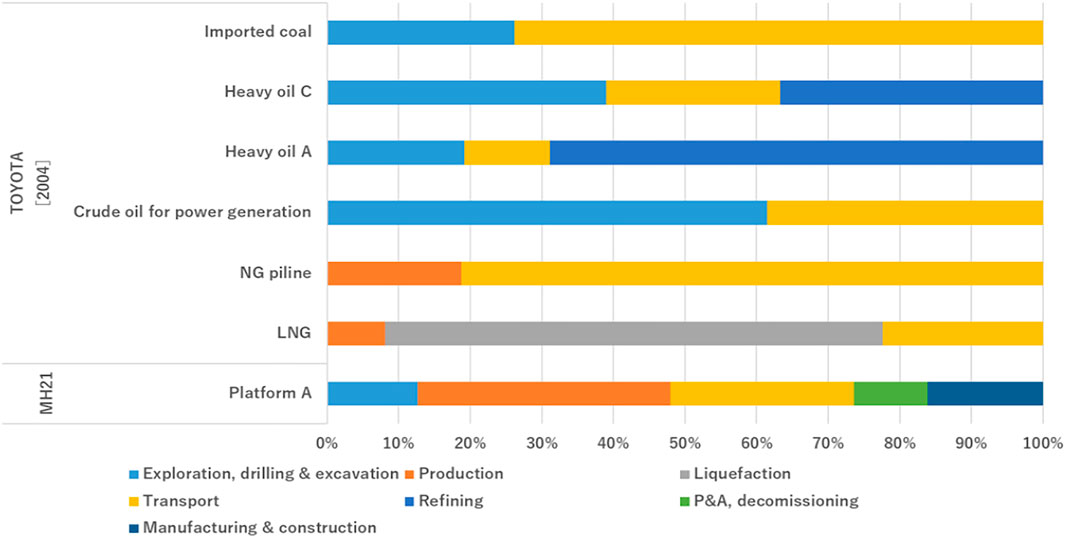
FIGURE 8. Energy input structure to produce each energy (coal, heavy oil, crude oil, natural gas (NG), LNG imported to Japan, and gas hydrate with offshore platform option). (Toyota Motor Corporation and Mizuho Information and Research Institute, Inc., 2004; Research Consortium for Methane Hydrate Resources in Japan, 2019).
Total energy outputs of gas hydrate cases are calculated from the produced gas volume estimated by numerical simulation. Figure 9 shows the comparison of EROI among energy sources. The data of other energies were obtained from the published data. The figure shows that EROI of the gas hydrate can be more than one and in the range 5–17. In the case of pipeline transport (floating platform or LTB scenarios), the values of more than 10 were expected; these values were higher than those of imported LNG and comparable with heavy oil. The main reason that EROI of gas from gas hydrates can be higher than LNG is that the resource existing at the site near the Japanese coast did not require energy demand for transportation and liquefaction. In the case of FLNG, this advantage in the value of EROI was lost. Gas production from gas hydrates needs more energy than from conventional natural gas. Transporting these gases requires energy for compression because pressure of the gas produced by the depressurization method was low and gas compression was necessary. Interestingly, the reservoir size does not have the grave effect on EROI. The total carbon intensity and portion of the production process and combustion of the product are shown in Figure 10.
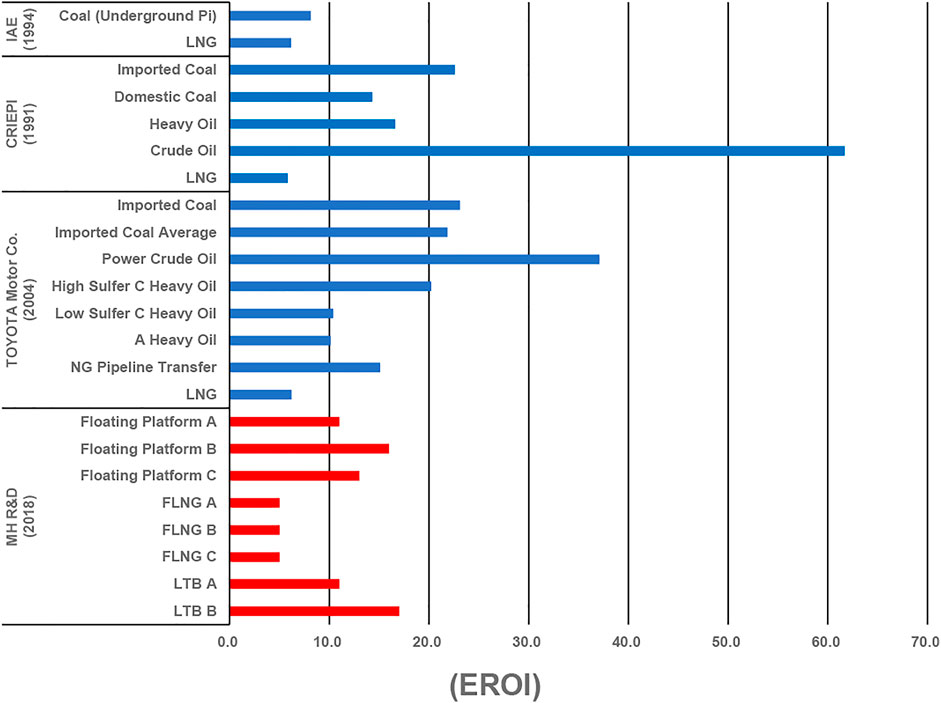
FIGURE 9. Energy return on investment of each energy source consumed in Japan. Toyota Motor Corporation and Mizuho Information and Research Institute, Inc., 2004, Central Research Institute of Electric Power Industry (1991), The Institute of Applied Energy (1994).
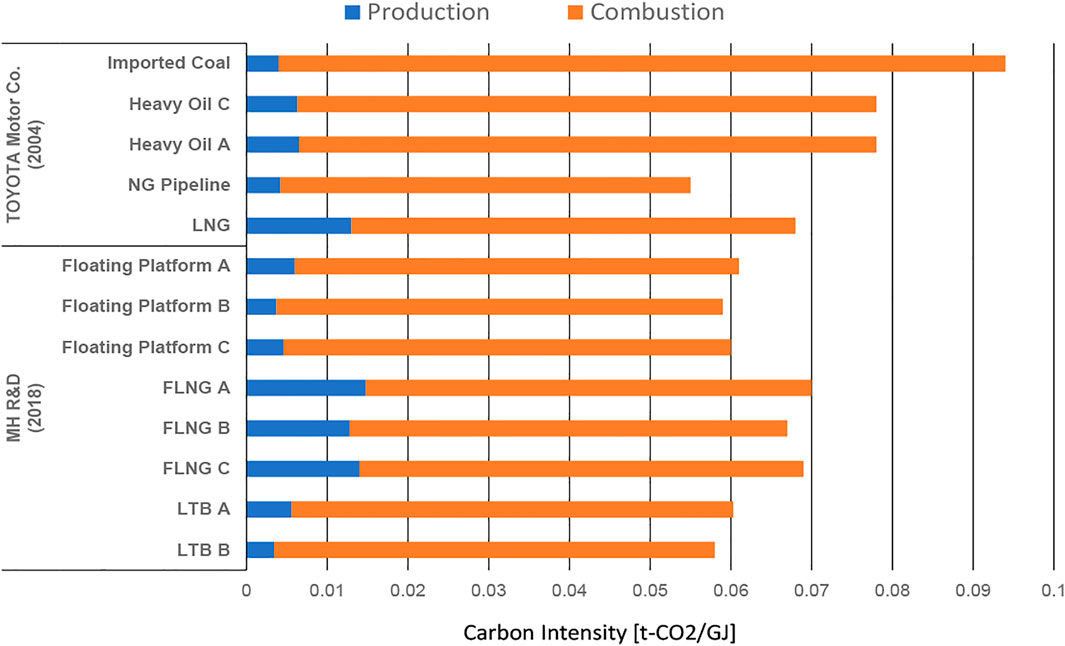
FIGURE 10. Carbon intensity of each energy—emission from the production process and combustion of products.
Discussions and Conclusions
Gas production from gas hydrate-bearing underground sediments is an energy-consuming process owing to its endothermic nature, resulting in low EROI. The theoretical value of the EROI of the production process is more than 100, and the actual values measured during gas production tests in the eastern Nankai Trough proved the value. However, along with the energy necessary for gas production, other processes in the reservoir life cycle, such as construction of offshore production systems, processing on the surface and gas transportation to the shore, and produced water disposal, require additional energy input. If the gas production rate predicted by numerical models can be realized in the real reservoir, a higher EROI than the imported liquid natural gas can be expected under certain reservoir conditions. Among offshore production systems, long tiebacks to shore or offshore platforms are a better choice than floating LNG; however, floating LNG has an advantage of market accessibility, so it is an option in the early stage of gas production.
Those values mean that the net CO2 emission from the gas hydrate production can be comparable or even lower than the conventional oil and gas. To bring the CO2 emission lower and close to zero, a combination of gas production with CO2 capture and storage should be considered. Energy conversion from methane to the “blue” hydrogen or ammonia with CO2 capture at offshore facilities and CO2 storage in deepwater gas hydrate reservoirs or aquifers may extend the chance to reduce the net CO2 emissions and contribute to the carbon-neutral society. Moreover, if the gas is transformed into liquid fuel, the problem of market accessibility can be solved; however, such additional process for energy conversion can reduce the value of EROI. As another idea, conversion of methane into electricity on the platform (gas-to-wire, or GTW) is possible, too (Yamamoto et al., 2021b). Fortunately, a CO2 storage site can be planned around gas hydrate deposits because gas hydrate reservoirs have been developed in geological conditions where sandy sediments could be developed and the area is favorable for CO2 storage, so the combination of gas production from hydrate and deepwater CO2 storage can be a reasonable option (Figure 11). The remaining problems are the cost and technical difficulty in constructing and operating such a processing facility on a floating offshore platform.
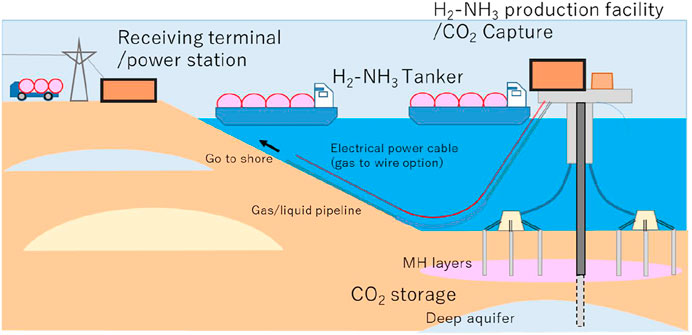
FIGURE 11. Schematic of the integration of offshore gas hydrate production, energy conversion, and CCS (modified from Yamamoto et al., 2021b). There can be options to transport converted fuels from offshore to land by pipelines or as electricity (gas-to-wire, GTW).
Another idea of CO2 utilization is CH4–CO2 exchange for gas production, which is an idea since CO2 is more favorable to form gas hydrate than CH4 in a certain pressure and temperature range (Ohgaki et al., 1996). This idea was applied and verified in the early stage of the Iġnik Sikumi field experiment in 2012 (Boswell et al., 2017) but is not viable in high pressure and condition in which CO2 is in liquid form. However, the idea is still valid for a possible mitigation measure of gas hydrate leakage from the seafloor and enhanced recovery measure for cooled reservoir after the depressurization. Chen et al. (2015) proposed a combination of methane gas production using CO2 and gas hydrate technology applications for separation, storage, and transportation as “Hydrate Chain Energy System.”
Methane has another face as a strong greenhouse gas, which has 28 times larger global warming potential than CO2 (IPCC Fifth Assessment Report, 2014). Using the depressurization method, methane cannot be discharged into the sea floor or atmosphere, except in accidental situations. If any trouble or damage of subsea and subsurface production facilities such as the well structure, subsea pump, and manifold and flow line lead to the flow-in of cold sea water into the well that affects the pressure and temperature of the borehole, gas hydrate dissociation will be terminated immediately. However, any injection activities for well stimulation or enhanced recovery may create a gas leakage pathway. Furthermore, gas leakage is possible because of the damaged surface facility and flow line through accidents or natural disasters. Therefore, activities and facilities should be carefully designed to minimize the chance of any unintended greenhouse gas release.
All the values of EROI presented here and field development scenarios are based on the gas production behavior predicted by numerical simulators. Until 2021, there are a few attempts to produce gas from marine gas hydrate deposits in Japanese (Yamamoto et al., 2017; Yamamoto et al., 2019) and Chinese (Li et al., 2018; Ye et al., 2020) waters, and long-term behavior of the gas production, specifically stability of them, is not verified in real fields, and gravest uncertainty lies there. Currently, Japanese phase four research program of gas hydrate is focusing on the validation of the long-term gas production concept by depressurization and evaluation of its performance and modeling technologies through an on-shore long-term (one year) production test in Alaska (Yamamoto et al., 2021a).
Author Contributions
KY had worked for the organization of the study, computation of EROI from physical process, analysis of the field test data, and design of combination system of gas production and CCS. SN evaluated and designed the offshore production system and evaluated the economics and EROI of them from the industrial viewpoints.
Conflict of Interest
SN was employed by the company Japan Methane Hydrate Operating Co., Ltd. (JMH).
The other author declares that the research was conducted in the absence of any commercial or financial relationships that could be construed as a potential conflict of interest.
Publisher’s Note
All claims expressed in this article are solely those of the authors and do not necessarily represent those of their affiliated organizations, or those of the publisher, the editors and the reviewers. Any product that may be evaluated in this article, or claim that may be made by its manufacturer, is not guaranteed or endorsed by the publisher.
Acknowledgments
This paper is a part of the MH21-S research program funded by the Ministry of Economy, Trade and Industry. The authors acknowledge H. Kameda of Tokyo Gas and Mizuho Information and Research Institute Inc. (currently, Mizuho Research and Technologies, Ltd.) for their contribution to this work.
References
Anderson, G. K. (2004). Enthalpy of Dissociation and Hydration Number of Methane Hydrate from the Clapeyron Equation. The J. Chem. Thermodynamics 36, 1119–1127. doi:10.1016/j.jct.2004.07.005
Ashford, D. I., Mizuta, T., Dallimore, S., and Yamamoto, K. (2012). Overview of Engineering and Operations Activities Conducted as Part of the JOGMEC/NRCan/Aurora Mallik 2007-2008 Gas Hydrate Production Research Well Program, Part B: 2008 Field Program. Geol. Surv. Can. Bull. 601, 53–65. doi:10.4095/292082
Boswell, R., and Collett, T. S. (2011). Current Perspectives on Gas Hydrate Resources. Energy Environ. Sci. 4, 1206–1215. doi:10.1039/c0ee00203h
Boswell, R., and Collett, T. (2006). The Gas Hydrate Resource Pyramid, Fire in the Ice. Natl. Energ. Technol. Lab. 6 (3), 5–7. https://netl.doe.gov/sites/default/files/publication/HMNewsFall06.pdf (Accessesed October 5, 2021).
Boswell, R., Schoderbek, D., Collett, T. S., Ohtsuki, S., White, M., and Anderson, B. J. (2017). The Iġnik Sikumi Field Experiment, Alaska North Slope: Design, Operations, and Implications for CO2-CH4 Exchange in Gas Hydrate Reservoirs. Energy Fuels 31 (1), 140–153. doi:10.1021/acs.energyfuels.6b01909
Callarotti, R. C. (2011). Energy Return on Energy Invested (EROI) for the Electrical Heating of Methane Hydrate Reservoirs. Sustainability 3 (11), 2105–2114. doi:10.3390/su3112105
Callarotti, R. C. (2012). EROI for the Production of Methane from Hydrates by Hot Water Injection. WIT Tran. Eng. Sci. 75 (10), 221–230. Available at: https://www.witpress.com/Secure/elibrary/papers/HT12/HT12019FU1.pdf (cited on 08 30, 2021). doi:10.2495/ht120191
Central Research Institute of Electric Power Industry (CRIEPI) (1991). Energy Analysis of Power Plants. Research Report
Dallimore, S. R., and Collett, T. S. (2005). “S. Summary and Implications of the Mallik 2002 Gas Hydrate Production Research Well Program,” in Scientific Results from the Mallik 2002 Gas Hydrate Production Well Program. Editors S. R. Dallimore, and T. S. Collett (Mackenzie Delta, Northwest Territories, Canada: Geological Survey of Canada Bulletin), 585, 1–36. doi:10.4095/221040
Dallimore, S. R., WrightYamamoto, J. F. K., and Bellefleur, G. (2012). “Proof of Concept for Gas Hydrate Production Using the Depressurization Technique, as Established by the G. JOGMEC/NRCan/Aurora Mallik 2007-2008 Gas Hydrate Production Research Well Program,” in Scientific Results from the JOGMEC/NRCan/Aurora Mallik 2007-2008 Gas Hydrate Production Research Well Program. Editors S. R. Dallimore, K. Yamamoto, J. F. Wright, and G. Bellefleur (Mackenzie Delta, Northwest Territories, Canada: Geological Survey of Canada Bulletin), 601, 1–15.
Frye, M. (2008). Preliminary Evaluation of In-Place Gas Hydrate Resources: Gulf of Mexico Outer Continental Shelf, OCS Report MMS 2008-004, Minerals Management Service. Resource Evaluation Division. Available at: https://www.boem.gov/sites/default/files/documents//MMS2008-004.pdf (cited on 09 30, 2021).
Fujii, T., Saeki, T., Kobayashi, T., Inamori, T., Hayashi, M., Takano, O., Takayama, O., Kawasaki, T., Nagakubo, S., Nakamizu, M., and Yokoi, K. (2008). “Resource Assessment of Methane Hydrate in the Eastern Nankai Trough Japan,” OTC19310 in 2008 Offshore Technology Conference, Houston, TX, May 2008.
Gao, Q., Yin, Z., Zhao, J., Yang, D., and Linga, P. (2021). Tuning the Fluid Production Behaviour of Hydrate-Bearing Sediments by Multi-Stage Depressurization. Chem. Eng. J. 406, 127174. doi:10.1016/j.cej.2020.127174
Guo, X., Xu, L., Wang, B., Sun, L., Liu, Y., Wei, R., et al. (2020). Optimized Gas and Water Production from Water-Saturated Hydrate-Bearing Sediment through Step-wise Depressurization Combined with thermal Stimulation. Appl. Energ. 276, 115438. doi:10.1016/j.apenergy.2020.115438/
Hancock, S. H., Collett, T. S., Dallimore, S. R., Satoh, T., Inoue, T., Huenges, E., et al. (2005). “Overview of Thermal-Stimulation Production-Test Results for the JAPEX/JNOC/GSC et al. Mallik 5L-38 Gas Hydrate Production Research Well,” in Scientific Results from the Mallik 2002 Gas Hydrate Production Well Program. Editors S. R. Dallimore, and T. S. Collett (Mackenzie Delta, Northwest Territories, Canada: Geological Survey of Canada Bulletin), 585. doi:10.4095/221040
Hata, T., Saracho, A. C., Haigh, S. K., Yoneda, J., and Yamamoto, K. (2020). Microbial-Induced Carbonate Precipitation Applicability with the Methane Hydrate-Bearing Layer Microbe. J. Nat. Gas Sci. Eng. 81, 103490. doi:10.1016/j.jngse.2020.103490
Ito, T., Xu, T., Tanaka, H., Taniuchi, Y., and Okamoto, A. (2014). Possibility to Remedy CO2 Leakage from Geological Reservoir Using CO2 Reactive Grout. Int. J. Greenhouse Gas Control. 20, 310–323. doi:10.1016/j.ijggc.2013.11.014
Japan Drilling Company (2009). Japan Patent 2009-30378. Available at: https://astamuse.com/ja/published/JP/No/2009030378 (Accessed October 5, 2021).
Johnson, A. H. (2011). “Global Resource Potential of Gas Hydrate – A New Calculation,” in Fire in the Ice, Natl. Energ. Technol. Lab. (DOE-NETL), 11(2), 1–4. Available at: https://lt/files/publication/MHNews-2011-12.pdf (Accessed October 5, 2021).
Kida, M., Jin, Y., Watanabe, M., KonnoYoneda, Y. J., Yoneda, J., Egawa, K., et al. (2015). Chemical and Crystallographic Characterizations of Natural Gas Hydrates Recovered from a Production Test Site in the Eastern Nankai Trough. Mar. Pet. Geology. 66 (2), 396–403. doi:10.1016/j.marpetgeo.2015.02.019
Kurihara, M., Ouchi, H., Masuda, Y., Narita, H., and Okada, Y. (2004). “Assessment of Gas Productivity of Natural Methane Hydrates Using MH21 Reservoir Simulator,” in Proceedings of the AAPG Hedberg Conference, Vancouver, B.C., Canada, September 12-16, 2004.
Kurihara, M., Sato, A., Ouchi, H., Narita, H., Masuda, Y., Saeki, T., and Fujii, T. (2008). “Prediction of Gas Productivity from Eastern Nankai Trough Methane Hydrate Reservoirs,” in OTC 19382, 2008 Offshore Technology Conference, Houston, TX, 5-8 May, 2008. doi:10.4043/19382-MS
Kurihara, M., Sato, A., Ouchi, H., Narita, H., Masuda, Y., Saeki, T., et al. (2009). Prediction of Gas Productivity from Eastern Nankai Trough Methane-Hydrate Reservoirs. SPE Reservoir Eval. Eng. 12, 477–499. SPE125481. doi:10.2118/125481-PA
Kvenvolden, K. A. (1988). Methane Hydrates and Global Climate. Glob. Biogeochem. Cycles 2 (3), 221–229. doi:10.1029/GB002i003p00221
Li, J.-F., Ye, J.-L., Ye, J.-L., Qin, X.-W., Qiu, H.-J., Wu, N.-Y., et al. (2018). The First Offshore Natural Gas Hydrate Production Test in South China Sea. China Geology. 1, 5–16. doi:10.31035/cg2018003
Masuda, Y., Konno, Y., Kurihara, M., Ouchi, H., Kamata, Y., Ebinuma, T., and Narita, H. (2005). “Validation Study of Numerical Simulator Predicting Gas Production Performance from Sediments Containing Methane Hydrate,” in Proc. 5th Int. Conf. Gas Hydrate, Trondheim, Norway, 13-16 June, 2005, 1076–1085. (ref. 3056).
Minagawa, H., Ito, T., Kimura, S., Kaneko, H., Noda, S., and Tenma, N. (2018). Depressurization and Electrical Heating of Methane Hydrate Sediment for Gas Production: Laboratory-Scale Experiments. J. Nat. Gas Sci. Eng. 50, 147–156. doi:10.1016/j.jngse.2017.10.024
Mori, Y. H., Ochiai, J.-i., and Ohmura, R. (2012). Using Submarine Heat Pumps for Efficient Gas Production from Seabed Hydrate Reservoirs. Energies 5 (3), 599–604. doi:10.3390/en5030599
Nagao, J. (2015). Development of Methane Hydrate Enhanced Production Methods. Methane Forum 2015. (in Japanese) Available at: https://www.mh21japan.gr.jp/mh21wp/wp-content/uploads/mh21form2015_doc02.pdf (Accessed October 5, 2021).
Ohgaki, K., Takano, K., Sangawa, H., Matsubara, T., and Nakano, S. (1996). Methane Exploitation by Carbon Dioxide from Gas Hydrates. Phase Equilibria for CO2-CH4 Mixed Hydrate System. J. Chem. Eng. Jpn. 29 (3), 478–483. doi:10.1252/jcej.29.478
Research Consortium for Methane Hydrate Resources in Japan (MH21) (2019). Section IV, Study of MH Production System and Evaluation of Economics and EROI, Comprehensive Report of Phase 2&3 Research Results. Available at: https://www.mh21japan.gr.jp/mh21wp/wp-content/uploads/phase2_3_6_1e.pdf (Accessed October 5, 2021).
Siažik, J., Malcho, M., and Lenhard, R. (2017). Proposal of Experimental Device for the Continuous Accumulation of Primary Energy in Natural Gas Hydrates. EPJ Web Conf. 143, 02106. doi:10.1051/epjconf/201714302106
Song, Y., Zhang, L., Lv, Q., Yang, M., Ling, Z., and Zhao, J. (2016). Assessment of Gas Production from Natural Gas Hydrate Using Depressurization, thermal Stimulation and Combined Methods. RSC Adv. 6 (6), 47357–47367. doi:10.1039/C6RA05526E
The Institute of Applied Energy (IAE) (1994). Study of the Methods for the Comprehensive Fossil Fuel Cycle Analysis Viewed from Global Environment. Tokyo, Japan: New Energy and Industrial Technology Development Organization.
Toyota Motor Corporation and Mizuho Information and Research Institute, Inc. (2004). Well to Wheel Evaluation of the Transportation Fuels. Available at: (in Japanese) https://www.mizuho-ir.co.jp/solution/improvement/csr/lca/pdf/jisseki02_wtwghg2004.pdf (Accessed October 5, 2021).
White, M. D., Kneafsey, T. J., Seol, Y., Waite, W. F., Uchida, S., Lin, J. S., et al. (2020). An International Code Comparison Study on Coupled thermal, Hydrologic and Geomechanical Processes of Natural Gas Hydrate-Bearing Sediments. Mar. Pet. Geology. 120, 104566. doi:10.1016/j.marpetgeo.2020.104566
Wilder, J. W., Moridis, G. J., Wilson, S. J., Kurihara, M., White, M., Masuda, Y., Anderson, B. J., Collet, T., Hunder, R. B., Narita, H., Pooladi-Darvish, M., Rose, K., and Boswell, R. (2008). “An International Effort to Compare Gas Hydrate Reservoir Simulator,” in Proc. 6th Internatinal Conference on Gas Hydrates, Vancouver, BC, Canada, Jul. 6-10, 2008.
Xu, T., Zhang, Z., Li, S., Li, X., and Lu, C. (2021). Numerical Evaluation of Gas Hydrate Production Performance of the Depressurization and Backfilling with an In Situ Supplemental Heat Method. ACS Omega 6 (18), 12274–12286. doi:10.1021/acsomega.1c01143
Yamamoto, K., Kanno, T., Wang, X.-X., Tamaki, M., Fujii, T., Chee, S.-S., et al. (2017). Thermal Responses of a Gas Hydrate-Bearing Sediment to a Depressurization Operation. RSC Adv. 7, 5554–5577. doi:10.1039/C6RA26487E
Yamamoto, K., Wang, X.-X., Tamaki, M., and Suzuki, K. (2019). The Second Offshore Production of Methane Hydrate in the Nankai Trough and Gas Production Behavior from a Heterogeneous Methane Hydrate Reservoir. RSC Adv. 9, 25987–26013. doi:10.1039/C9RA00755E
Yamamoto, K., Tenma, N., and Abe, M. (2021a). Japan’s Phase Four Methane Hydrate Research Program, Fire in the Ice, Methane Hydrate News. Natl. Energ. Technol. Lab. 21 (1), 1–5. Available at: https://netl.doe.gov/sites/default/files/publication/MHNews_Spring2021_0.pdf (Accessed October 5, 2021).
Yamamoto, K., Abe, M., and Tenma, N. (2021b). “Issues to Realize Carbon Neutral in Developing Methane Hydrate Resource Development in Japanese Water,” in 40th Annual meeting, Japan Society of Energy and Resources, August 2-3, 2021, Osaka, Japan. (in Japanese with English abstract).
Ye, J.-L., Qin, X.-W., Xie, W.-W., Lu, H.-L., Ma, B.-J., Qiu, H.-J., et al. (2020). The Second Natural Gas Hydrate Production Test in the South China Sea. China Geology. 3, 197–209. doi:10.31035/cg2020043
Yin, Z., Chong, Z. R., Tan, H. K., and Linga, P. (2016). Review of Gas Hydrate Dissociation Kinetic Models for Energy Recovery. J. Nat. Gas Sci. Eng. 35 (B), 1362–1387. doi:10.1016/j.jngse.2016.04.050
Keywords: gas hydrates, gas production, depressurization, deep water, energy return on investment, carbon capture and storage
Citation: Yamamoto K and Nagakubo S (2021) Review of Energy Efficiency of the Gas Production Technologies From Gas Hydrate-Bearing Sediments. Front. Energy Res. 9:741715. doi: 10.3389/fenrg.2021.741715
Received: 15 July 2021; Accepted: 06 September 2021;
Published: 12 October 2021.
Edited by:
Jing Cai, Technical University of Denmark, DenmarkReviewed by:
Zhenyuan Yin, Tsinghua University, ChinaBo Li, Chongqing University, China
Jiafei Zhao, Dalian University of Technology, China
Copyright © 2021 Yamamoto and Nagakubo. This is an open-access article distributed under the terms of the Creative Commons Attribution License (CC BY). The use, distribution or reproduction in other forums is permitted, provided the original author(s) and the copyright owner(s) are credited and that the original publication in this journal is cited, in accordance with accepted academic practice. No use, distribution or reproduction is permitted which does not comply with these terms.
*Correspondence: Koji Yamamoto, eWFtYW1vdG8ta29qaUBqb2dtZWMuZ28uanA=