Sustainable Energy Transition for Renewable and Low Carbon Grid Electricity Generation and Supply
- Industrial Engineering Department, Durban University of Technology, Durban, South Africa
The greatest sustainability challenge facing humanity today is the greenhouse gas emissions and the global climate change with fossil fuels led by coal, natural gas and oil contributing 61.3% of global electricity generation in the year 2020. The cumulative effect of the Stockholm, Rio, and Johannesburg conferences identified sustainable energy development (SED) as a very important factor in the sustainable global development. This study reviews energy transition strategies and proposes a roadmap for sustainable energy transition for sustainable electricity generation and supply in line with commitments of the Paris Agreement aimed at reducing greenhouse gas emissions and limiting the rise in global average temperature to 1.5°C above the preindustrial level. The sustainable transition strategies typically consist of three major technological changes namely, energy savings on the demand side, generation efficiency at production level and fossil fuel substitution by various renewable energy sources and low carbon nuclear. For the transition remain technically and economically feasible and beneficial, policy initiatives are necessary to steer the global electricity transition towards a sustainable energy and electricity system. Large-scale renewable energy adoption should include measures to improve efficiency of existing nonrenewable sources which still have an important cost reduction and stabilization role. A resilient grid with advanced energy storage for storage and absorption of variable renewables should also be part of the transition strategies. From this study, it was noted that whereas sustainable development has social, economic, and environmental pillars, energy sustainability is best analysed by five-dimensional approach consisting of environmental, economic, social, technical, and institutional/political sustainability to determine resource sustainability. The energy transition requires new technology for maximum use of the abundant but intermittent renewable sources a sustainable mix with limited nonrenewable sources optimized to minimize cost and environmental impact but maintained quality, stability, and flexibility of an electricity supply system. Technologies needed for the transition are those that use conventional mitigation, negative emissions technologies which capture and sequester carbon emissions and finally technologies which alter the global atmospheric radiative energy budget to stabilize and reduce global average temperature. A sustainable electricity system needs facilitating technology, policy, strategies and infrastructure like smart grids, and models with an appropriate mix of both renewable and low carbon energy sources.
Highlights
• The Paris Agreement of 2015 set targets to be realized to limit greenhouse gas emissions and related global warming with the objective of reducing greenhouse gas emissions and global average temperature rise.
• The cumulative effect of the Stockholm, Rio, and Johannesburg conferences made sustainable energy development (SED) a very important factor in the sustainable development.
• The energy transition seeks to transform the world order with respect to development and environment and particularly the use of energy in its many forms with priority to electricity.
• Sustainable energy transition should incorporate the three dimensions of sustainable development of social, environment and economic in addition to technical and political/institutional dimension.
• A sustainable global electricity transition will entail increased use of renewable energy sources particularly wind and solar, nuclear energy as a low carbon energy source, electrification of transport and thermal processes in industry, bioenergy, and waste to energy conversion, shift from coal and petroleum to natural gas, hydrogen as a fuel with low carbon footprint, increased energy efficiency.
• This transition can only take place successfully through collaboration between players locally and international with effective facilitating policy framework, facilitating infrastructure and technology development and adaptation, use of smart grids and various modelling and optimization facilities in decision support.
1 Introduction
Decarbonization of the global energy systems is one of the greatest and most important challenges facing man in the 21st Century. The energy sector is vital in tacking the climate change since it accounts for about two thirds of global carbon dioxide (Quitzow, 2021). Energy in form of electricity and primary energy resources drive the prosperity of the world economy (Quitzow, 2021). From the 1970s, the global gross domestic product (GDP) has grown by about 4.5 times, while the consumption of primary energy has grown from 155.22 EJ in 1965 to 556.63 EJ in the year 2020. The total proved energy reserves of the dominant fuels, i.e., oil, natural gas, and coal at the end of 2020 could last just 53.5 years for oil, 48.8 years for natural gas, and 139 years for coal (BP, 2021). These fossil fuels account for 85% of the total primary energy consumption globally. The current era is faced with the challenge of global warming as the most prominent environmental issue thus reduction of carbon emissions is at the center of global environmental policy. It is therefore important to understand the relationship between economic development and energy consumption, and effectively improve energy efficiency for a better relationship and sustainable development (Barasa Kabeyi and Olanrewaju, 2022; Jin et al., 2022). Electricity plays a very important role in modern economies as it provides a rising share of energy generation and consumption in all countries (Solarin et al., 2021). Electricity demand is poised to increase further due to increasing household incomes, and electrification of transport and thermal energy applications as well as continues growth for digital connected devices and air conditioning (International Energy Agency, 2019). Energy is a critical requirement for sustainable development and therefore optimum selection of low carbon and green energy sources remains a key objective for all nations (Bhowmik et al., 2020). Electric power plays an important role in human life because all vital activities and operations today need electricity directly or indirectly (Beaudin and Zareipour, 2015; Bayram and Ustun, 2017).
Electricity as a form of energy is extremely important for socioeconomic development. Global electricity generation stood at 4,114 GW in 2005 and increased to 5,699.3 GW in 2014 and it continues to grow annually. Fossil fuel-based electricity accounted for over 60% of this generation in 2014 and 42% of CO2. There is need to develop evaluation index system and models for sustainable electricity generation to sustainably cope with this ever growing demand (Li et al., 2016). According to the emissions gap report, the total greenhouse gas emissions in 2018 was about 55.3 GtCO2e of which 37.5 GtCO2 were on account of fossil fuels combustion in various operations and activities including electricity generation [United Nations Environmental Program(UNEP), 2019].
Energy sustainability or energy for sustainable development is a challenge for many countries developed and developing countries. There is need for a transition roadmap to renewable energy sources that may be unique to each country based on local resources and prevailing circumstances (Iddrisu and Bhattacharyya, 2015). Energy transition is a reality for all nations because of the targets set in the Paris agreement. The global community is developing decarbonization plans aimed at reducing greenhouse gas emission in a sustainable manner (Kabeyi and Olanrewaju, 2020b). The process is unique to different countries because the transition is affected by local social and economic conditions. The complexity and comprehensiveness of the energy transition is influenced by the diversity of actors involved in their interests which are often in conflict with one another (Krzywda et al., 2021). Electricity is a very important form of end-use energy, and it is a leading factor for economic growth and development. However, electricity generation is a leading source of greenhouse gas emissions which cause global warming and climate change which threatens sustainable development. This is because most of the global electricity is generated from fossil fuel sources of energy. Electricity accounts for a significant share of the three components that make up total energy production and consumption are electricity, transport, and heating (Ritchie and Roser, 2021). The main challenges facing the electricity sector are the ever growing electricity demand, growing need to reduce greenhouse gas emissions and the need realize zero-net carbon emissions in power generation in line with the Paris Agreement which seeks to limit the increase in average global temperature to 1.5°C (Colangelo et al., 2021). This calls for an energy transition from the fossil fuel dominated electricity mix to one dominated by renewable sources of energy and low carbon nuclear as well as clean fuel and conversion technologies (Kabeyi and Olanrewaju, 2020b; Kabeyi and Olanrewaju, 2021a).
The world has so far witnessed three typical energy transitions. The first transition involved replacement of wood with coal as the main energy source. In the second transition, oil replaced coal as the dominant energy resource. In the third transition, there is global commitment to replace fossil fuels with renewable energy. As in 2018, 80% of the global energy was derived from fossil fuel energy resources with 36% being petroleum, 13.2% for coal, and 31% was from natural gas (Lu et al., 2020). Energy transition refers changes undertaken in fundamental processes in charge of evolution of human societies that drive and are driven by technical, economic, and social changes (Smil, 2010). It is a new path for economic development and innovation that does not compromise the environmental integrity and sustainability motivated by challenges caused by greenhouse gas emissions, climate change and natural resource depletion (Mostafa, 2014). Energy transition consists of processes of structural changes to the subsystems of society which lead to greater sustainability in the society (Barasa Kabeyi, 2019a). Therefore energy transitions call for changes in existing policies, technology as well as supply and demand patterns for electricity and other energy resources (Mostafa, 2014). The world is said to be undergoing a fourth energy transition today having witnessed three energy transitions in the past. The main objective of this fourth transition is to fight the global climate change through decarbonization of the energy supply and consumption patterns (Mitrova and Melnikov, 2019). Therefore a sustainable energy transition system must be driven by the climate change agenda, technology developments and innovation, increased energy efficiency, competitive economies, enhanced energy security, development of affordable energy solutions and measures and modernization of the energy sector from traditional energy systems (Smil, 2010; Mitrova and Melnikov, 2019). The International Renewable Energy Agency (IRA) defines energy transition as the pathway in the transformation of the global energy sector from fossil-dominated mix to zero-carbon by the second half of the 21st century (Inglesi-Lotz, 2021).
The selection criteria for development sustainable energy transition should consider the environmental, technical, social, institutional, and economic dimensions of sustainability. While choosing or selecting energy sources for electricity generation, the choice of conversion technology and cost involved play a crucial role in modern economies and societies (Bhowmik et al., 2020; Kabeyi and Olanrewaju, 2020b). With continuous increase in global population and socio-economic activities leading to increased urbanization, and industrialization around the world, the demand for natural energy resources and more so renewable energy is gradually increasing (Ebrahimi and Rahmani, 2019). It is notable that the world’s population has grown by 2.5 times since 1950, while energy demand over the same period has grown by 7 times (Şengül et al., 2015). These increasing energy demand is predominantly met by fossil fuel combustion and nuclear power plants (Tunc et al., 2012). With ever increasing energy demand, the related challenges are depletion of fossil fuel reserves, their price volatility, and global climate change which have attracted much attention to renewable energy sources and other low carbon and cheap sources of energy for power generation. As a result, many countries have adopted policies, strategic and operational measures to support the growth of renewable energy sources and other sustainable energy measures in the energy transition (Ebrahimi and Rahmani, 2019).
Sustainable energy transitions require formulation of effective policies that promote the biomass resources, increased use of renewable and low carbon sources and penalize as well as discourage the use of fossil fuels and unsustainable natural resource use. Directing agricultural resources toward food production (Andress et al., 2011). Renewable energy like solar, wind power, or hydropower can be used as viable options for generating electricity. Solar power plants, for example, could be constructed in countries with vast expanses of desert land. With developing countries like China having huge coal reserves and high electricity demand, coal fired power plants will continue to dominate electricity generation in these countries and options like clean coal technologies and carbon capture and sequestration are critical options. Production of hydrogen from coal is another strategy. For countries with high electricity demand, nuclear power generation is an option for reducing GHG emissions although with a danger of proliferation for politically unstable governments with weapons agenda. Large scale penetration of renewable energy requires development of advanced batteries, high efficiency conversion technologies, and stable and resilient grids to absorb variable renewable energy sources. Electrification of transport with most electricity coming from low carbon and green sources is another strategy for the sustainable energy transition (Andress et al., 2011).
There are various strategies, measures and technologies that can be used to improve sustainability. They include energy efficiency, increasing the contribution of renewable energy in electricity generation, use of Carbon Capture and Storage (CCS) in fossil and biomass power plants, use of low carbon nuclear power, use of hydrogen in the transportation sector and reductions in the demand for energy and electrification as well as use of biofuels in transport services. The main challenges facing various options and technologies include lack of acceptance and behavioral changes as well as cost limitations and availability of cheap fossil fuels (Hildingsson and Johansson, 2016).
1.1 Problem Statement
Most of the electricity generated globally is comes from fossil fuel-based power plants. These energy resources are generally expensive, scarce, exhaustible, polluting, and insecure since not all nations are endowed with the primary resources hence a source of energy insecurity, while the combustion of fossil fuels produces greenhouse gases like carbon dioxide (CO2), Sulphur dioxide (SO2), Nitrous oxides (NOx), which are the main causes of the global warming that is threatening the very existence of humanity and mother nature. This concern is the main motivation behind sustainable energy transition by increased use of renewable and low carbon clean energy sources especially solar, wind, biomass, hydro and nuclear. These renewable and low carbon sources improve and widen power supply, enhance long term access and utility in energy production, decrease dependence on fossil fuel, and reduce greenhouse gas emissions (Rathor and Saxena, 2020a; Nguyen et al., 2020).
Natural increases in CO2 concentrations have historically been warming the Earth during ice age cycles for millions of years. These warm episodes are said to have started with slight increase in solar radiations reaching the Earth due to a slight wobble in Earth’s axis and path of rotation around the Sun that caused some notable warming. This phenomenon caused the warming of oceans leading to an increase in carbon dioxide (CO2) emissions from the oceans. However, CO2 concentration never exceeded 300 ppm during these periods that took place about a million years ago. Before the industrial revolution that started in of mid-1700s, the global average amount of carbon dioxide was about 280 ppm (Lindsey, 2021). Figure 1 shows the historical growth of CO2 emissions and concentration between 1750 and 2020.
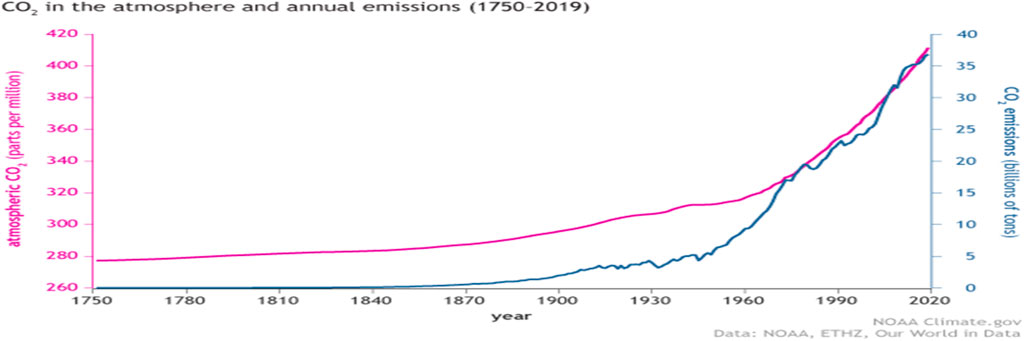
FIGURE 1. Concentration of carbon dioxide emissions between the year 1750 and 2020 (Lidsey, 2020).
Figure 1 shows that the global CO2 emissions remained constant between 1750 and about 1840 where they started to increase rapidly. The atmospheric concentration increased slightly between 1750 and 1960 before the rate increased between 1960 and the year 2020 mainly due to higher level of industrial activities and increasing use of fossil fuels. Therefore, it is the entry of fossil fuels in the energy mix that triggered rapid increase in CO2 emissions as the level of industrialization developed.
Fossil fuels continue to dominate the current energy systems and therefore significantly contribute to the global carbon dioxide (CO2) and other greenhouse gases emissions to the atmosphere. To realize the global climate targets and avoid destructive climate change, there is need for a global transition in electricity generation, transmission, and distribution and as well as its consumption. Humanity must strike a balance between developmental needs and the environmental conservation and protection. The main challenges facing renewable energy sources is resource availability, resource access, resource location, security of supply, sustainability, and affordability (Samaras et al., 2019). The growing demand for electricity has led to increased demand and consumption of fossil fuels and growing level of economic activities have contributed to growth of the greenhouse gas emissions and consequently, global warming (Wang, 2019). The transition challenges are further compounded by the fact that efforts to promote more sustainable, more resilient, and equitable energy disrupts economic, political, and institutional relationships. As a result, issues of power and politics are now central themes in sustainability transition in energy sectors (Lenhart and Fox, 2021).
This study examined sustainability in energy and particularly electricity generation systems and the challenges and opportunities of sustainability. The overall objective was to lay a framework for a sustainable transition to a green and low carbon electricity grid system as a contribution to the global effort to fight the climate change and greenhouse gas emissions. Various pathways to sustainable electricity generation are examined and proposals made on a feasible roadmap to a sustainable energy transition, particularly grid electricity generation, transmission, distribution, and consumption. The transition acknowledges the significance of nonrenewable sources and their main challenges of intermittence and variability as the global community seeks to transition to green energy sources. Using a critical discourse analysis, the study attempts to develop a roadmap that can be adopted by nations based on their local conditions to sustainably transition their electricity production and supply. Of particular concern is how the available energy sources can be used to realize the Paris targets without compromising the socio-economic and environmental set up and hence achieve sustainable energy transition.
1.2 Rationale of the Study
The Paris Agreement of the 21st UNFCCC Conference of Parties (COP21) of 2015 seeks to limit average global temperature increase below 2°C above pre-industrial levels look for measures to limit the average temperature rise to 1.5°C the pre-industrial temperature. This calls for drastic measures to reduce anthropogenic emissions and removals by sinks of greenhouse gases by second half of 21st century (Lawrence et al., 2018). Studies have shown that the climate is changing mostly because of the anthropogenic activities. The report by Intergovernmental Panel on Climate Change (IPCC) for 2021 indicates that several climate changes are already irreversible but adds that we still have hope for the future if action is taken. To mitigate further changes (Inglesi-Lotz, 2021). The climate is very important to man and other living organisms on the planet, yet there is overwhelming evidence that the world is facing changing climatic conditions due to the greenhouse effect as demonstrated by the increase in average global temperature, high incidents of climate related issues like drought, storms and desertification (Wallington et al., 2004). The global anthropogenic activities have led to about 1°C rise in average global temperature above prehistoric level and is further projected to reach 1.5°C between the year 2030 and 2052 if current greenhouse gas emission rates are maintained (Fawzy et al., 2020).
Electricity generation accounts for about 26% of total greenhouse gas emissions making it an important target for emissions control in the war against climate change (Kabeyi and Oludolapo, 2020a). The Intergovernmental Panel on Climate Change (IPCC) sought to stabilize the atmospheric air carbon concentration with a target to limit concentration to 350 ppm for CO2 while maintaining temperature rise of 2°C above the preindustrial level, a target that was ratified by many nations globally. This calls for limitation in the consumption of fossil fuels particularly in electricity generation and transport industries through substitution with renewable energy sources and electrification of transport among other measures. This calls for massive expansion in power generation capacity using renewable energy and low carbon energy sources (Burger et al., 2012). The future of humanity as defined by the sustainable development goals in the face of climate change has made sustainability the concern for all major systems including energy or electricity generation, supply and consumption systems (Vine, 2019). There is need for a shift from current dependence on fossil fuels for power generation, transport, and thermal applications (Burger et al., 2012).
The global greenhouse gas emissions can be presented based on economic activities that lead to their production and emission. Greenhouse gases are mainly released by electricity and heat generation in the energy and related sectors, manufacturing activities, industrial operations, transportation, agriculture and forestry as well as the building industry (Marcus, 1992). The sources of greenhouse gases can be classified into five economic sectors. These sectors are energy, industry, transport, buildings and AFOLU, i.e., agriculture, forestry, and other land uses (Lamb et al., 2021). In Figure 4 below, the greenhouse gas emissions by sector are presented for the years 1990–2018 for the entire world and across 10 ten global regions, namely Asia pacific, Africa, East Asia, Eurasia, Europe, Latin America, Middle east, North America, South Asia, and Southeast Asia. Figure 2 below shows the contribution of greenhouse gases by five economic sectors.
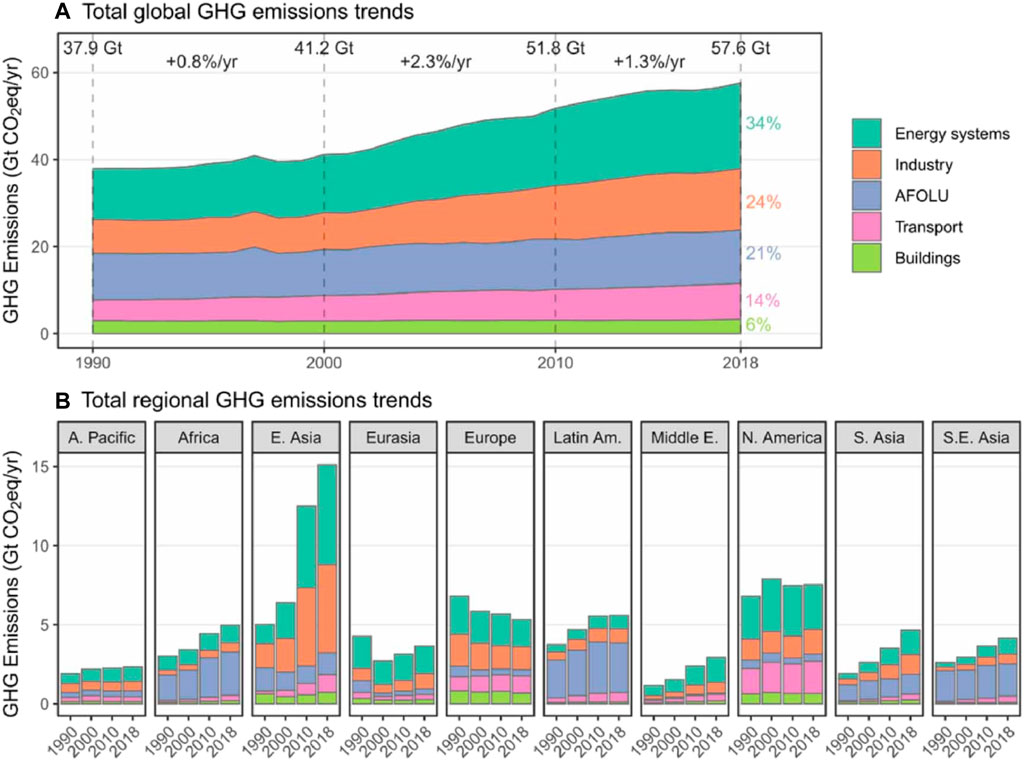
FIGURE 2. Greenhouse gas contribution by economic sector (Boden et al., 2017; Lamb et al., 2021).
From Figure 2, it is noted that the energy sector inn form of electricity and heat production is the largest contributor of green house gases with about 34%, industry at 24% followed by agriculture, forestry and other land activities accounting for 21%, transportation with 14%, while buildings contributed about 6% while the building sector is least with 6% in 2018 (Lamb et al., 2021). Figure 2 further demonstrates that for the African region, most emissions.
The greenhouse gas emissions (GHG) for 2018 were about 11% (5.8 GtCO2eq) higher than GHG emission levels in 2010 (51.8 GtCO2eq). The energy sector accounted for close to 1/3rd of the increase in GHG emissions between 2010 and 2018 of about 1.9 GtCO2eq, followed by industrial sector with 1.8 GtCO2eq which was about 30% of the increase, then the transport accounted for 1.2 GtCO2eq or 20% of the increase. Emissions that came from AFOLU increased by about 0.72 GtCO2eq equivalent to 12% increase while the buildings sector recorded the lowest increase in emissions with about 0.22 GtCO2eq, or 4% (Lamb et al., 2021).
A sustainable energy transition should address the energy sources, energy conversion, transmission, and consumption especially the leading sectors in energy consumptions like heat and power production, transport related activities including fuel use and conversion. These measures include a shift from fossil fuel sources to renewable and low carbon sources, efficient conversion technologies, electrification of transport with most electricity coming from renewable resources, energy conservation measures and elimination of unnecessary energy demand and consumption.
Energy is far much more than just the technical infrastructure. It is through the energy transition that we realize emergence of innovative business models and organization that drives the establishment of new practices and procedures, and new ways of life, reassign responsibilities, reorganize governance, and redistributes power structure. It is for these reasons that the sustainable energy transition calls for consideration of the social, technical, economic, political, and institutional dimensions of sustainability in addressing challenges of the energy sector in order to sustainably shift to a low-carbon economy and electricity systems which is the main focus of this research (Quitzow, 2021).
2 Methodology and Novelity of the Study
In this study, low-carbon energy transitions options and strategies are considered governed and proposed in line with broader sustainability goals and requirements as specified by the dimensions of sustainability. The study sought to identify conflicts and synergies between low-carbon strategies and the attainment of both short term and longer-term environmental, economic, technical, social, and institutional objectives. The research framework is organized across the five dimensions of sustainable energy and specifically electricity development which are environmental, economic dimensions, social aspects, technical dimensions, and institutional, political dimensions. The study adopted secondary method of data collection and analysis from recent primary and secondary data found in original research findings and reports from credible peer reviewed sources. This would facilitate the voice of the common people, professional, experts, and authorities as well as governments for a cleaner global future. For this research, the term primary data refers to the data originated and carried out and presented as peer reviewed academic and professional papers and reports through personal interviews with the expert team or analysis based on primary data and presented as original reports or peer reviewed journal article. Therefore, this study is a review of the energy sustainable transitions globally. Published literature in the form of technical reports, peer reviewed journals and conference papers were reviewed by the authors. The study is a survey using credible literature from peer reviewed journals papers and energy data from various authorities globally.
Most studies on sustainable energy transition are narrow in scope as they tend to concentrate on the environmental dimension of sustainability. Researchers that look at sustainability more wholistically also tend to concentrate on the three pillars of sustainability, namely economic, environmental, and social dimensions of sustainability (Kabeyi, 2019a; Barasa Kabeyi, 2019b; Krzywda et al., 2021). Past reviews also concentrate on energy sustainability in general. However, in this study, the focus is electricity as a secondary form of energy derived from primary sources of energy like wind, solar, hydro, nuclear, coal, and gas through indirect conversion through a generator or direct conversion as in fuel cells and solar photovoltaics.
In most studies undertaken on energy transitions, the focus has been on energy technologies and sources with main objective being minimizing emissions. Others have gone further to address variability and intermittence and to rank sources in order of potential within the framework of the three pillars of sustainability. This study is unique as it looks at technical and institutional dimensions in addition to the economic, social, and environmental and hence the role of energy storage and electrification of transport which induces additional variability to demand side. The study also brings into focus the role of the smart grid in managing the dynamic nature of electricity demand and supply in decentralized generation and with the intermittence and variability of wind and solar. The study therefore recognizes that social foundations and human behaviors have a significant role to play in the future sustainability of the energy sector (Inglesi-Lotz, 2021). Therefore, the study pays attention and extensively analyses to energy and electricity models and modelling tools that wholistically considers sustainability in the energy sector and electricity systems.
3 Greenhouse Gas Emissions and Their Environmental Impacts
Greenhouse effect refers to a natural process that has taken place over millions of years but was first discovered by Jean Baptiste-Joseph de Fourier in 1827 (Wallington et al., 2004; Wang, 2019). Greenhouse gas effect is the long-term increase in the temperature of the planet Earth as a result of accumulation of greenhouse gases in the atmosphere (İpek Tunç et al., 2007). The discovery of greenhouse effect was demonstrated experimentally by John Tyndall in 1861 and quantified in 1896 by Svante Arrhenius. In the work of Svante Arrhenius, the author observed that the release of large amounts of CO2 emissions from the combustion of fossil and doubling of atmospheric CO2 concentration warmed the Earth by 5–6°C, as compared to current climate models which predict a 1.5–4.5°C rise by doubling the concentration of CO2 (Kabeyi and Oludolapo, 2020a; Kabeyi and Oludolapo, 2020b). Greenhouse gas effect results from the interaction between solar energy and greenhouse gases in the atmosphere. The work of Roger Revelle and Hans Suess in 1957, remarked that the build-up of carbon dioxide in the atmosphere constituted a large-scale geophysical experiment whose consequences were unknown and so should be monitored and controlled. As a result, the year 1958 was designated as the International Geophysical Year. This marked the beginning of the ongoing program of continuous measurements of atmospheric CO2 levels at Mauna Loa, Hawaii, in the United States by Charles Keeling. This measurement demonstrated that the levels of carbon dioxide were rising steadily from 315 ppm in 1958 to 370 ppm in 2001 (Wallington et al., 2004).
The global climate change is one of the leading challenges facing humanity today (del RíoJaneiro, 2016). This is because the climate has been changing over time with manifestations like the global increase in average temperature, rising sea level, heat waves, several incidents of flooding, both stronger and frequent ocean waves, and drought in many parts of the world (Intergovernmental Panel on Climate Change(IPCC), 2007; Butt et al., 2012). The United Nations Framework Convention on climate change, attributes these directly or indirectly to human activities that change the atmospheric composition leading variation in the natural climate as observed over a long period of time. There has always been evidence that the global climate has been changing since the beginning of creation, but the current rate is alarming with several consequences already being witnessed today.
Greenhouse gases like carbon dioxide (CO2) can absorb and radiate thermal energy. It is this greenhouse effect that warms the Earth and keeps its average annual temperature above the freezing point which implies that normal global warming maintains life and so is necessary (Lindsey, 2021). Although carbon dioxide (CO2) is less potent than methane and nitrous oxide, it is more abundant and stays longer in the atmosphere thereby making it more significant. It is for this reason that the increase in atmospheric carbon dioxide is responsible for about two-thirds of the total energy imbalance and temperature rise. Additionally, carbon dioxide has a negative impact to the sea water because when dissolved in ocean water, CO2 reacts with water molecules to produce carbonic acid which lowers the PH of the ocean water. It is for this reason that the PH of the ocean’s water has reduced from 8.21 to 8.10 since the beginning of the industrial revolution. This is quite significant because a drop by 0.1 in PH causes about 30% increase in acidity of the ocean. The biological effect of ocean acidification is interference with marine life’s ability to extract calcium from the water to build their shells and skeletons (Lindsey, 2021).
The global atmospheric of carbon dioxide (CO2) has been increasing particularly on the account of fossil fuel combustion in powerplants, transport and several industrial processes. This is not sustainable because the fossil fuels took many millions of years to form, only to be returned to the atmosphere in just few years, hence their lack of renewability [United Nations Economic and Social Commission (ESCAP), 2016]. It is estimated that the last time the atmospheric CO₂ amounts were relatively higher was about 3 million years ago, when temperature was 2–3°C (3.6–5.4°F) above the pre-industrial era, and the sea level was 15–25 m (50–80 feet) higher than it is today (Lindsey, 2021). Industrialization is the main cause for the steady rise in the concentration of carbon dioxide between 1970 and 2020 because of the use of fossil fuels in transportation, industry, and power generation. This trend is demonstrated in Figure 3.
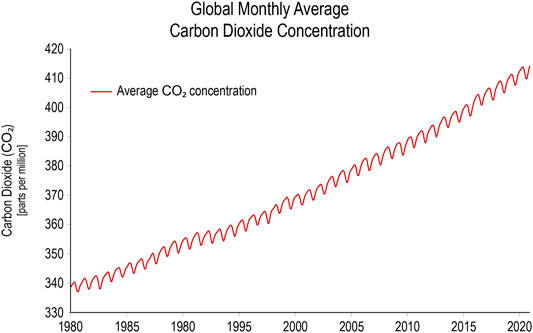
FIGURE 3. Changes in carbon dioxide concentration between 1970 and 2020 (Lindsey, 2021).
From Figure 3, it is noted that the concentration of atmospheric carbon dioxide has increased steadily from about 328 ppm in 1970 to 412.5 ppm in 2020 up from 409.8 ppm in 2019 having increased steadily from the value of 328 in 1970. The annual rate of increase in CO2 in the atmosphere is about 100 times faster than previous natural increases over the past 60 years, e.g., last ice age 11,000–17,000 years ago. Additionally, the ocean has absorbed enough carbon dioxide to lower its pH by 0.1 units causing a 30% increase in ocean acidity (Lindsey, 2021). Although the concentration dropped in 2020, global energy-related CO2 emissions stood 31.5 Gt, which contributed to CO2 that made atmospheric concentration reach about 50% higher than it was at the beginning of the industrial revolution (International Energy Agency, 2021a).
3.1 Greenhouse Gases in Power Generation
Electricity and heat generation is a leading source of CO2 emissions and they generated 13 billion tons of CO2 emissions accounting for 41% of all CO2 emissions coming from fuel combustion globally in 2017. This was due to the use of fossil fuels to generate about 16,947 TWhrs of electricity representing 63% of the total electricity generation (Solarin et al., 2021) At the global scale, the key greenhouse gases emitted by human activities are carbon dioxide, methane, nitrous oxide, and F gases. Energy supply is the largest contributor to global greenhouse gas emissions. About 35% of total anthropogenic GHG emissions in 2010 originated in the energy sector. The global annual growth in greenhouse gas emissions from energy supply sector increased from 1.7% per year in 1990–2000 to 3.1% in 2000–2010 mainly due to faster economic growth which increase demand for heat and electricity consumption. Most of these heat and power demand is met by fossil fuel sources of energy (Bruckner et al., 2014a).
3.1.1 Carbon Dioxide (CO2)
The Natural increases in CO2 concentrations have been warming the Earth over time with increase in temperature during ice age cycles over millions of years. These warm episodes or interglacial started with a small increase in sunlight when the Earth had a tiny wobble in its axis of rotation around the Sun. This event led to some warming of the Earth’s surface and oceans causing increased release of carbon dioxide. The extra CO2 in the atmosphere only magnified the initial natural warming. Among the anthropogenic greenhouse gases, CO2 is responsible for about 60% of the greenhouse gas effect (İpek Tunç et al., 2007). With the industrial revolution, manmade sources have become significant sources of atmospheric carbon dioxide through activities like fossil fuel combustion for power generation, transportation, industrial as well as domestic activities (Lindsey, 2021). Other leading sources are industrial chemical reactions and operations like cement production. Carbon dioxide is mainly removed from the atmosphere or sequestration through photosynthesis [United States Environmental Protection Agency (EPA), 2017]. When carbon dioxide is heated, it absorbs and radiates heat in the form of thermal infrared energy. This radiation has the positive side because without it, the annual average temperature would be just close to 60°F. However, with the rapid increase in greenhouse gas emission, additional heat has been trapped leading to raising planet Earth’s average temperature above the pre-industrial level.
3.1.2 Methane (CH4)
Methane is generated from various natural and artificial processes like anerobic digestion and during the production and transport of fossil fuels like coal, natural gas, and oil [United States Environmental Protection Agency (EPA), 2017]. Therefore, methane is added to the atmosphere through natural and anthropogenic sources, with 30% of the methane flux originating from natural sources while about 70% is contributed by anthropogenic sources (Wallington et al., 2004).
3.1.3 Nitrous Oxide (N2O)
Food production particularly the use of fertilizers is the primary source of N2O emissions. As well as fossil fuel combustion in power generation, industry, and transportation as a product of combustion and wastewater treatment process (Viet et al., 2020). Nitrous oxide (N2O) is the third most abundant well mixed greenhouse gas after CO2 and CH4 with a life span of about 130 years. The natural sources of N2O come from soils and the oceans. Anthropogenic emissions originate from biomass combustion, fossil fuel combustion, and industrial production of adipic and nitric acids, and nitrogen fertilizer use in agriculture (Wallington et al., 2004).
3.1.4 F Gases
The F gases result from industrial processes, refrigeration systems, and the use of some consumer products associated with emissions of F-gases. These gases include hydro fluorocarbons (HFCs), perfluorocarbons (PFCs), and sulfur hexafluoride (SF6). Hydro fluorocarbons, perfluorocarbons, sulfur hexafluoride, and nitrogen trifluoride are synthetic, and come from industrial processes. These Fluorinated gases are occasionally used as substitutes for ozone-depleting substances, e.g., chlorofluorocarbons, hydro chlorofluorocarbons, and halons. These gases have high global warming potential gases even though they are released in small quantities [United States Environmental Protection Agency (EPA), 2017].
Figure 4 below shows the global composition of the greenhouse gases in the atmosphere (Lamb et al., 2021).
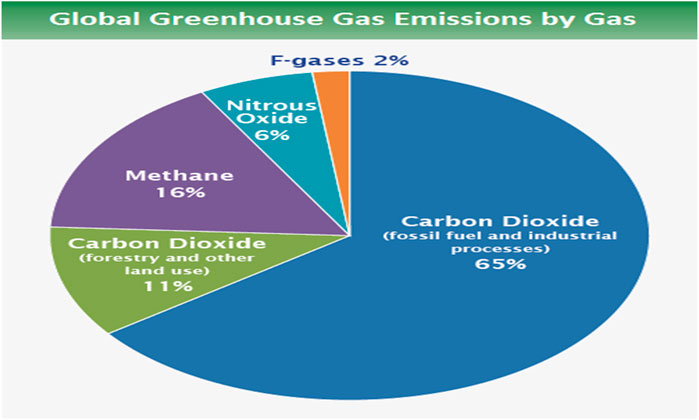
FIGURE 4. Global greenhouse gas emissions by gas (Boden et al., 2017).
From Figure 4, it is noted that carbon dioxide remains the dominant greenhouse gas with most of it coming from fossil fuel combustion in industry, heat, and power generation which accounts for 65% of global emissions. Other sources of carbon dioxide are agriculture and forestry related activities which contribute 11% of the greenhouse gas emission in form of CO2. Methane is the second largest greenhouse gas accounting for 16% of greenhouse gas emissions, followed by nitrous oxide and F-gases respectively with 6 and 2% contribution respectively.
The global average CO2 concentration in 2018 was about 407.4 ppm with uncertainty of + and −0.1 ppm. Today, carbon dioxide levels are the highest over the past 800,000 years (Lindsey, 2021). Over 400 billion metric tons of carbon dioxide has been released to the atmosphere from fossil fuels combustion and cement production since the year 1751, of which about 200 billion metric tons originate from fossil fuel combustion since the late 1980s.
In 2014, the global carbon emissions to the atmosphere were about 36 billion tons, which is approximately 1.6 times the 1990s rates, an increase which has been associated with average global temperature rises (Gamil et al., 2020). Close to 9,855 million metric tons of fossil-fuel based carbon dioxide emissions were emitted between 2013 and 2014, representing an increase of 0.8%. Liquid and solid fuels accounted for 75.1% of the global carbon emissions from fossil-fuel burning and cement production in 2014. Gaseous fuel accounted for 18.5% of the emissions which is about 1,823 million metric tons of carbon emissions from fossil fuels in 2014. In 2014, emissions from cement production were 568 million metric tons of carbon indicating, which shows a more than double increase in the last decade to represent 5.8% of global CO2 emissions from fossil-fuel burning and cement production. Emissions from gas flaring, which accounted for about 2% of global emissions during the 1970s, reduced to less than 1% of global carbon emissions from fossil-fuel (Boden et al., 2017). These statistics show that fossil fuel combustion which mainly comes from power generation and cement production in the manufacturing industry are leading polluters of the atmosphere in terms of carbon emissions.
3.2 Global Warming/Greenhouse Effect
Global warming is the effect of the imbalance between the heat received by the Earth and, the heat reradiated to the space. Terrestrial longwave radiative flux is emitted by the Earth’s surface beyond the 3–100 µm wavelength range while the surface incoming radiation is shortwave solar radiation also called global irradiance or solar surface irradiance. This is the radiation flux density reaching a horizontal unit of Earth surface in the 0.2–3 µm wavelength range (Ming et al., 2014). Global warming refers to the process which leads to the average rise in the Earth’s temperature and that of the atmospheric layers close to Earth because of human activities. Climate change on the other hand is the phenomenon where other climatic factors change due to global warming. Any slight increase in the ocean temperature causes hydrological events which effectively change the physical and chemical characteristics of the water. Aquatic life is affected by change in water as it affects their life cycle, physiology, and behaviors (Ninawe et al., 2018).
Global warming potential (GWP) is a measure of how much a given mass of a chemical substance adds to global warming over a specified time. It refers to the ratio of the warming caused by a substance to the warming caused by a similar mass of carbon dioxide. These therefore allocates 1 as the global warming potential of carbon dioxide. Water has the lowest global warming potential of 0, while Chlorofluorocarbon-12 has a high global warming potential of 8,500. Chlorofluorocarbon-11 has GWP of 5,000. Several hydrochlorofluorocarbons and hydrofluorocarbons have GWP potentials varying between 93 and 12,100 based on a 100-years period (Demirel and Demirel, 2014). The three-time scales used to compute the GWP are 20, 100 or 500 years respectively. The main greenhouse gases from fossil fuel combustion are nitrous oxide (N2O), methane (CH4) and carbon dioxide (CO2). The value of the three main greenhouse gases varies in GWP for the time scales used in evaluation, i.e., 20, 100, and 500 years. The GWP of CO2 is used as a reference in the computation of the relative GWP of other gases (MacLeod et al., 2011; Demirel and Demirel, 2014). Table 1 below shows the relative global warming potential (GWP) for water, carbon dioxide, methane and nitrous oxide based on the 20-, 100- and 500-years’ time scale.

TABLE 1. Global warming potential for various substances/gases (MacLeod et al., 2011; Demirel and Demirel, 2014).
From Table 1, it is noted that the global warming potential of the major greenhouse gases various in value over the three timescales. It shows that N2O has the highest individual and average GWP followed by methane and carbon dioxide while water has no global warming potential.
Carbon dioxide accounts for about 60% of the anthropogenic greenhouse gases which are the cause the greenhouse gas effect with use of fossil fuels especially in power being cited as the major cause (İpek Tunç et al., 2007; Tunc et al., 2012). The global increase in carbon emissions was about 1.4 ppm before 1995 but thereafter increased to 2.0 ppm (Owusu and Asumadu-Sarkodie, 2016). Carbon dioxide concentration has grown from 277 ppm in 1750 to 397 ppm in 2014 representing about 43% and peaked at 400 ppm between March and December 2015 (Berga, 2016). According to Moriarty and Honnery (2019) and Lindsey (2021), the concentration of CO2 in the atmosphere passed 407 ppm in 2018 with energy sector emerging as the largest emitter of the greenhouse gases (Intergovernmental Panel o, 2007; Butt et al., 2012; Berga, 2016; Owusu and Asumadu-Sarkodie, 2016). In 2010, the energy supply sector contributed 35% of all the anthropogenic greenhouse gas emissions realized. The emissions increased at average rate of 1.7% between 1990 and 2000 which increased to average of 3.1% between 2000 and 2010 on account of rapid economic growth experienced due to increased use of coal in the energy mix (Sagan, 2011). Fossil fuel sources like coal, heavy fuel oil and natural gas used in power generation are significant sources of greenhouse gases (Butt et al., 2012), yet fossil fuels inform of gas and coal account for over 60% of global electricity generation (International Energy Agency, 2018).
With the increase in energy demand and continuous use of fossil fuels in power generation, a 62% increase in CO2 emissions is expected between 2011 and 2050 with two thirds of these emissions coming from China and India (Butt et al., 2012). It is for this reason that the Intergovernmental panel on climate change (IPCC) recommended that greenhouse gas emissions should be reduced by 50–80% by the year 2050 to avoid serious consequences of global warming, (Butt et al., 2012). Among the potential consequences are more frequent extreme weather events like heat waves, storms, flooding and droughts, stress due to higher temperatures for plants and humans, rising sea level, and altering occurrence of pathogenic organisms (Streimikienea et al., 2012).
3.3 Other Impacts of Greenhouse Gas Emissions
Besides global warming, some gaseous emissions have other significant negative impacts to the global and local environment. They include contamination of air by pollutants like Sulphur oxides, nitrogen oxides, particulate matter, and volatile organic compounds, which are released into the atmosphere by the combustion of fossil fuels in power plants, vehicles exhausts, industry, and building and equipment. Whereas some pollutants harm human health directly, others lead to atmospheric chemical reactions that yield harmful conditions like depletion of ozone layer besides global warming. This pollution can be controlled or limited through fuel substitution and conversion technologies that are less polluting (United States Department, 2015). For example, power plants should be equipped with pollution monitoring and control systems like desulphurization systems, carbon capture systems and fuel substitution technologies.
The rise in sea level and its acidification is another threat to the global environment and mankind. GHG emissions in the atmosphere lead to changes include sea-level rise, and an increase in the frequency and intensity of certain extreme weather events like drought and storms. Carbon dioxide is also by the oceans, leading to ocean acidification. The solution is increased use of nonpolluting energy sources, carbon capture and energy efficiency measures (Nag, 2008; United States Department, 2015; Kabeyi and Olanrewaju, 2020b).
4 Global Electricity Generation
The ever-growing electricity demand is the main reason for growth in global CO2 emissions from electricity which have now reached a record high. The commercial availability of low emissions generation technologies and energy sources has placed electricity at the center of the global effort to combat climate change and pollution. Decarbonization of electricity has a significant potential to provide a platform for CO2 emissions reduction by means of increased use of electricity-based fuels such as hydrogen and biofuels. Renewable energy sources and efficiency in resource use and generation has a special role to play in increasing access to electricity globally (International Energy Agency, 2019).
The shift and growth in electricity demand adopts two distinct global routes or paths. For most developed countries, demand growth is linked to increasing digitalization and electrification which is largely offset by energy efficiency measures and improvements in process energy efficiency. For the developing countries like China and India, the reasons for growing electricity demand are the growing incomes and better quality of life, industrialization, and growing services sector. It is also worth noting that the developed countries account for about 90% of global electricity demand growth, and the trend may remain so until the year 2040 (International Energy Agency, 2019).
Today, Industry and building sectors are the main users of electricity accounting for over 90% of global electricity demand. Moving forward, the main drivers of electricity demand growth are motors in industry which may account for over 30% of the total growth to 2040. It is projected that industrial and domestic space cooling will account for 17% while large electrical appliances are projected to account for 10% growth while electric vehicles are projected to account for 10% growth in electricity demand. Further growth in electricity demand of about 2% is projected to come from provision of electricity access to 530 million first time users of electricity. The Sustainable Development Scenario, projects that electric vehicles will become a leading source of electricity demand moving to the future towards the year 2040 (International Energy Agency, 2019).
The global energy demand and supply has been growing with supply increase of about 60% between 1990 and 2016 when supply hit 568 EJ. The international bunkers was 16.3 EJ in 2016 accounting for 3% of global total energy supply and was marked by a double growth since 1990, an indication of growing activity and hence energy consumption internationally [United Nations(UN), 2019]. The global electricity generation more than doubled between 1990 and 2016, to reach about 25,000 TWh. Between 1990 and 2016, the largest absolute growth in terms of energy sources came from coal with about 5,300 TWh representing +116% growth. Natural gas supply reached 3,500 TWh representing a growth of +213%.
Renewable sources of energy represented by mainly solar, wind grew by +2,224% or 1,370 TWh over the same period. This was the fastest growth recorded for renewable sources of energy. However, over 75% of electricity in 2016 came from non-renewable sources, mainly from thermal energy accounting for 65% or 16,186 TWh and nuclear 10% or 2,608 TWh. On the positive note, between 2000 and 2016, 50% of new electricity generating capacity came from renewable energy sources [United Nations(UN), 2019; Wanga et al., 2020]. Figure 5 below shows the changes in total energy supply between 1971 and 2019.
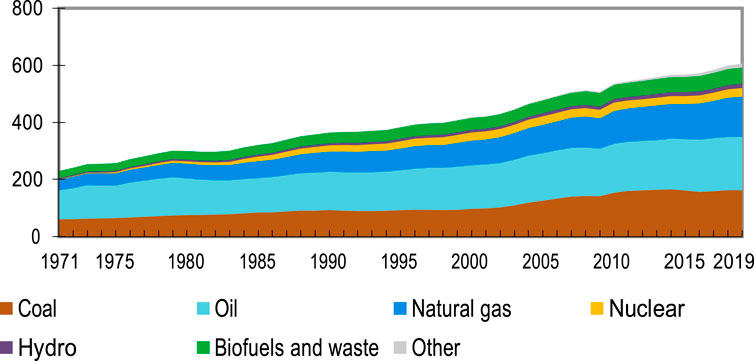
FIGURE 5. World total energy supply between 1971 and 2019 (United Nations, 2019a; International Energy Agency, 2021b).
From Figure 5 above, it is noted that between 1971 and 2019, the proportionate composition of primary energy mix has been changing. The total primary energy consumption increased from 254 EJ in 1973 to 606 EJ in 2019. Biofuels and wastes reduced from 10.5% in 1973 to 9.3% in 2019. Coal consumption increased from 24.7% of total primary energy consumption to 26.8% in 2019. Oil reduced from 46.2% in 1973 to 30.9% in 2019. Natural gas increased from 16.2% of consumption in 1973 to 23.1%. Nuclear increased from 0.9 to 5% while hydro increased from 1.8 to 2.5% of total primary energy consumption in 2019 (International Energy Agency, 2021b).
Fossil fuels generated 61% of global in the year 2020 while combined nuclear, wind and solar accounted for 35% of global electricity generation in the year. Solar energy also surpassed oil in global electricity generation 2020 where solar accounted for 3.2% compared to oil that contributed 2.8% of global electricity generation for the year 2020 (World Energy Data, 2021). Figure 6 below shows global electricity generation from different sources for the year 2020.
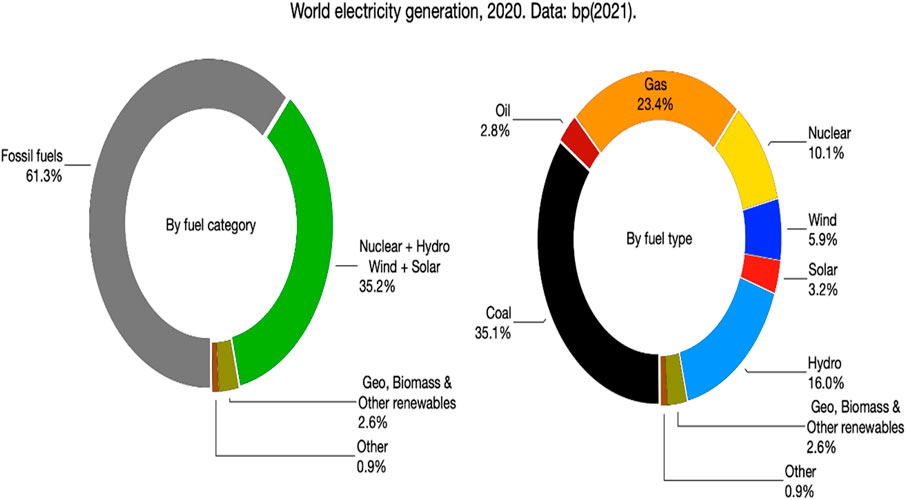
FIGURE 6. World electricity generation by source for 2020 (BP, 2021).
From Figure 6, it is noted that for the year 2020, fossil fuels contributed 61.3% of global electricity production, 35.2% was on account of combination of nuclear, hydro, and solar, other renewable s accounted for 2.6% while other sources accounted for 0.9%. Coal contributed 35.1% of global electricity while gas accounted for 23.4% of global electricity.
5 Sustainable Energy Development
The road to a sustainable energy future has twin challenges of energy access and mitigation of global warming by control of greenhouse gas emissions (Kaygusuz, 2012). Energy is at the center of several Sustainable Development Goals. They include expansion of electricity access, improving clean cooking fuels, curbing pollution, and reducing wasteful energy subsidies. Goal number 7 also referred to as SDG 7–aims to ensure access to reliable, affordable and modern energy for all by the end of the next decade (Birol, 2021). The global adoption of energy specific sustainable development goals was an important milestone towards a more sustainable and equitable society. Although energy must be at the heart of efforts to lead the world to a more sustainable pathway, the current and planned policies fall well short of realizing the critical energy-related sustainable development targets. On the positive note, there is tremendous progress in delivering universal electricity access (SDG 7.1.1) for Asia and parts of sub-Saharan Africa (Birol, 2021). As in the year 2012, about 1.4 billion people globally had no access to electricity of which 85% are based in rural areas. It is projected that by the year 20130, about 2.8 billion people globally will be relying on traditional forms of energy mainly biomass, which is an increase from 2.7 billion people in the year 2012 (Kaygusuz, 2012), where the number of people without access to electricity declined to 1.1 billion in 2016, from about 1.7 billion in the year 2000. However, it is projected that more than 670 million people will still have no access to electricity in 2030. Therefore a lot more remains to be done in terms of electricity access (Birol, 2021).
Lack of access to affordable electricity and reliance on the inefficient and unsustainable traditional energy like fuelwood, charcoal, agricultural waste, and animal dung) are a clear manifestation as well as an indicator of poverty. Modern energy sources and electricity plays an important role in socio-economic development (Tracey and Anne, 2008; Kaygusuz, 2012). Reliable electricity and light lengthen the day activities hence provide extra hours for economic activities. Positive contribution of electricity includes saving women and children from exposure to poisonous smoke and long hours of looking for firewood. Hospitals can better sterilise instruments and store medicines in refrigerators. Electricity improves manufacturing and service enterprises by extending the quality and range of their products hence creating more jobs and higher wage (Kaygusuz, 2012).
5.1 Sustainable Development
There is need to eliminate energy poverty to achieve the Millennium Development Goals but in a way that takes the world away from dependence on the fossil fuels to avoid global warming by moving rapidly towards a green economy (Vezzoli Vezzoliet al., 2018). The three interlinked objectives that must be achieved by the year 2030 to realise sustainable energy for all are ensuring universal access to modern energy services, double the share of renewable energy in the global energy mix and double the rate of energy efficiency improvement (Kaygusuz, 2012; Vezzoli Vezzoliet al., 2018).
Philosophers, economists and scientists introduced the closely related concepts of sustainable development and sustainability in the 18th, 19th, and early 20th centuries (Seghezzo, 2009). Sustainable development can further be defined socio-economic growth that delivers the traditional positive progress and targets in an ecologically acceptable manner and with due regard of the future generations’ welfare and rights to the same (Kabeyi and Olanrewaju, 2020b; Kabeyi and Oludolapo, 2021a). Sustainable is defined as sustained growth, or sustained change or can also be defined simply as development that is successful (Lélé, 1991). Sustainability is necessary in energy and other resources exploitation so as man exploits resources to meet his ever-growing energy demand, he does not compromise the ability of future generations to meet their own energy needs and a stable environment (Broman and Robèrt, 2017; Kabeyi and Olanrewaju, 2020b). Because of these requirement and expectations, society must strike a balance between economic growth and the social wellbeing of the society as a whole, now and in future to realize sustainability, which is a technical, political and economic challenge (Dyllick and Hockerts, 2002). Therefore, the concepts of sustainable development and sustainability has the objective of achieving economic advancement and progress while at the same time conserve the value and integrity of the environment. This calls for a tradeoff between environmental sustainability goals and economic development objectives and targets (Emas, 2015).
The publication of Carson’s book called “Silent Spring” in 1962 was used as the starting point of the global concern over proper use of natural resources. This can be demonstrated by what emerged 10 years later in 1972 as the “Club of Rome” that styled itself as an independent analysts and think tank who later published a book called “The Limits to Growth” (Jacobs et al., 1987; Intergovernmental Panel o, 2007; Akella et al., 2009). In this book, the authors observed that if the global economy and population grew unchecked, the planet Earth’s natural resources would approach depletion at a point in future. These narrative led to the formation of the UN “World Commission on Development and Environment”, also called the Brundtland Commission, named after its chair, Gro Harlem Brundtland, who was a former Norwegian Prime Minister (Seghezzo, 2009; University of Alberta, 2021). The “Brundtland Commission” released its final report that was entitled, “Our Common Future” 4 years later that defined sustainable development. The report defined sustainable development as a positive change that meets the needs of the present generation without compromising the ability of future generations to meet their own needs in future (World Commission on Envir, 1987; University of Alberta, 2021). Therefore the concept of sustainable development is more concerned with whether what is acceptable today and is acceptable or not acceptable to the next generation (Jonathan, 2001). Today, the strategies for sustainable development aim at promoting harmony and wellbeing among human beings and between humanity and Mother nature. Obviously, energy especially in the form of electricity has a central role in any effort to achieve sustainable development. From the use of wastes by industry to generate power and stabilize the grid, and conversion of polluting and eye soring slaughterhouse waste to clean electricity and create jobs keeping humanity clean and healthy fee of diseases, poverty, and physical harm (Kabeyi and Olanrewaju, 2021b; Kabeyi and Olanrewaju, 2021c; Kabeyi and Olanrewaju, 2021d; Kabeyi and Olanrewaju, 2021e).
Sustainable development and the concept of sustainability calls for integration of economic benefits, social considerations and progress with environmental protection and considerations for maximum positive outcome (Mohamad and Anuge, 2021). The United Nations General Assembly in 2015 adopted the 2030 Agenda for sustainable development which as a framework of 17 sustainable development goals (SDGs). This agenda calls for sustainable development which recognizes need to reduce poverty and guarantee equity and integrity of the entire global human community. These 2030 agenda calls for member countries to protect the planet Earth from further degradation by taking sustainability measures which include sustainable resource production and consumption, and sustainable management and conservation of the Earth’s natural resources and prevent climate change (United States Department, 2015; Kabeyi and Oludolapo, 2020a).
There is inherent interdependence between environmental stability and the economy which then lays a strong foundation for sustainable development (Emas, 2015). There is however need for public policy that promotes investment in economic and industrial activities that seek to protect the natural environment, promote human, and social capital, and prevent the damage caused by pollution, social clashes, resource waste, and greenhouse gas emissions which are both indicators and effects of unsustainable practices (United States Department, 2015). Fortunately, policies that seek to protect the environment and mother nature also promote innovation and profitability by organizations, and this should encourage enforcement, either voluntarily or by legislations. Promotion of innovation and strict environmental regulations can enhance competitiveness and hence economic performance and progress. The link between the environmental integrity and development provides a strong rationale for environmental protection (Liu, 2014; Kabeyi and Olanrewaju, 2020b).
The use of polluter pay principle in environmental protection requires authorities to impose penalties upon those who pollute the environment and hence make them bear the cost of their impact instead of leaving it with the environment or others. There is need to integrate economic, environmental, and social objects across sectors, territories, and even generations if sustainable development can be achieved. This implies that energy policy should be an integral part of the entire national and international agenda and should be therefore be integrated in other policies touching on the economy, society and the environment (Emas, 2015). Sustainable energy development should also be taken as a continuous process integrating all aspects of national, local, and international development agendas (Mohamad and Anuge, 2021). Therefore, sustainable energy development can only be realized through integration of energy objectives, development goals and environmental protection to avoid conflict by creating a critical synergy.
5.2 Relationship Between Sustainable Development and Energy
Energy is currently recognized as one of the most important factors that influence the rate of progress as well as sustainable development of all nations (Kolagar et al., 2020). To meet the ever-growing energy demand especially electricity, increase access to electricity for the billions of people with no access to electricity and high-quality low carbon fuels, as well as to reduce greenhouse gas emissions requires a radical shift from the fossil-fuel focused energy systems. There is need for a new energy paradigm to encourage the transformation of the predominantly fossil fuel-based energy systems. Sustainability is an important paradigm in the global energy transition where all dimensions of sustainability are addressed any policy formulation and implementation, planning, operation, and dispatch of the energy resources in both generation and consumption (Davidsdottir and Sayigh, 2012). For a longtime, energy did not seriously factor in sustainable development. However, sustainable development and sustainability issues now play a central role in energy and electricity by anchoring the evolution of the sustainable development paradigm (Iddrisu and Bhattacharyya, 2015; Kabeyi and Olanrewaju, 2021f).
Specific energy projects influence the economic, social, and environmental dimensions of the sustainability country or region. The triangular approach to the three dimensions of sustainable development consisting of economic, social, and environmental is used to assess the sustainability of a specific energy project (Kolagar et al., 2020). Figure 7 below illustrates the triangular approach in energy project assessment.
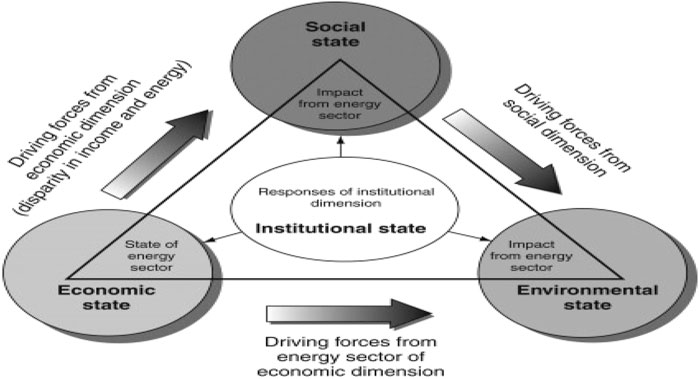
FIGURE 7. The dimensions of sustainability and their interrelationships (Kolagar et al., 2020).
From Figure 7, it is noted that the three dimensions of sustainability are held together by the institutional state. The economic state is the main driver of social and environmental states with the institutional state playing the coordination role.
It is at the Stockholm conference, energy was identified as a source of environmental stress, thus linking energy to the environmental dimension of sustainable development. The United Nations Conference on Environment and Development (UNCED) which was also referred to as the “Earth Summit,” that was held in Rio de Janeiro, Brazil, from 3 to 14 June 1992 led to the Rio Declaration on Environment and Development, that had no specific reference to energy. Energy was however a central them theme in Chapter 9 in Agenda 21 on the protection of the atmosphere in which energy was identified as a major source of atmospheric pollution (Quitzow, 2021). Agenda 21 further illustrated the need to draw a balance between economic growth, energy consumption, and its environmental impacts. Although the Commission for Sustainable Development (CSD) was established by the Rio conference of 1992, it is in 1997 when energy was placed in the agenda of the Commission for Sustainable Development (Gibbes et al., 2020; Kolagar et al., 2020). Based on the progress of the Commission for Sustainable Development (CSD9), the 2002 Johannesburg conference made direct reference to energy as crucial for sustainable development. It is in this conference that energy was addressed within the three dimensions of sustainable development, i.e., economic, social, and environmental. The conference further clearly treated energy as a specific issue of concern rather than a subset of other issues as it was in the Rio Conference. There was strong and specific emphasis on energy use and its social attributes like access to high-quality energy as a basic human right. It is therefore from the Johannesburg conference of 2002 that the social dimension of energy was incorporated in addition to environmental and economic dimensions which had already been incorporated curtesy of the Rio and Stockholm conferences (Gibbes et al., 2020; Kolagar et al., 2020).
Important energy issues that affect sustainability are energy research and development, training and capacity building, and technology development and transfers. Therefore, it is the cumulative effect of the Stockholm, Rio, and Johannesburg conferences that the notion of sustainable energy development (SED) as a very important factor in the sustainable development was mooted by linking energy to the environmental dimension in the Stockholm conference, economy in the Rio conference and society in the Johannesburg conference. Over time, energy consumption and energy development have become a specific issue in the three dimensions of sustainable development.
It is Article 8 from the Johannesburg declaration that we get the most comprehensive definition of sustainable energy development. Therefore, sustainable energy development involves improving access to reliable, affordable, economically viable, socially acceptable, and environmentally sound energy services and resources that consider the national specificities and circumstances. These can be achieved through means like enhanced rural electrification, decentralized electricity generation, greater use of renewable energy, use of clean gaseous and liquid fuels and improved energy use efficiency while recognizing the poor and vulnerable and their right of access to clean energy (Davidsdottir and Sayigh, 2012).
5.3 Characteristics of Sustainable Energy
For energy sources and systems to contribute to sustainable development, they should possess the following characteristics.
• Energy resources and systems are sustainable if they are renewable or perpetual in nature.
• Sustainable energy system should not be wasteful but efficiently produced and used with minimum resource wastage.
• Sustainable energy and energy systems should be economically and financially viable.
• Energy is sustainable if the source is secure and diverse.
• Sustainable energy and energy systems should be equitable or readily accessible, available, and affordable.
• Sustainable energy development should bring positive social impacts.
• Sustainable energy should be associated with minimal environmental impacts (Kolagar et al., 2020).
5.4 Themes/Goals of Sustainable Energy Development
By combining the characteristics or features of the Johannesburg definition with the International Atomic Energy Agency (IAEA) definition, there ae four central goals/themes of sustainable energy development. These are.
5.4.1 Improving Energy Efficiency
This involves improvement in economic and the technical efficiency of energy systems in generation and consumption. With investment in efficient energy systems, costs will reduce as well as output from available energy resources This can be achieved through technology transfer, research, and development and good energy management practices (Kolagar et al., 2020).
5.4.2 Improving Energy Security
Energy security covers the security of both supply and the energy resources infrastructure. Energy security refers to the availability of energy at all times in various forms, in sufficient quantities, and at fair prices that are affordable and predictable. Therefore, for energy to be regarded as secure, it must meet all dimensions of sustainable energy and development. Strategies to improve energy security include decentralizing power generation, wide use of renewable energy resources, investment in redundancy, diversifying energy sources, enhancing supply, more use of local energy resources. Common indicators of energy insecurity include power rationing, frequent blackouts, energy related conflicts and price instability and supply instability (Gibbes et al., 2020; Kolagar et al., 2020).
5.4.3 Reduce Environmental Impact
Sustainable energy development calls for reduction in emission of greenhouse gas emissions, which cause global warming. This can be achieved through reduction in the lifecycle environmental impact of energy systems use and production or generation. Other strategies include waste recycling and treatment and adoption of clean technologies that ensure that disposal of wastes does not exceed the Earth’s assimilative capacity (Kolagar et al., 2020). Decarbonization of the energy supply is a very important function in the transition to low carbon energy grid and economy. Besides technology, the deployment of a powerplant depends on the availability of resources, socioeconomic impact, and smooth integration with the existing electricity system. Energy system planners should consider all these to determine and prioritize energy projects and programs (Colla et al., 2020).
5.4.4 Expand Access, Availability, and Affordability
Sustainable energy should be reliable in supply and access at affordable price or cost and quality. There is need to expand energy resources to ensure supply reliability. Goals one and two correspond to the economic dimension of sustainable development, while the third goal deals with environmental dimension of sustainable development. On the other hand, the fourth goal deals with the social dimension of sustainable development. Progress towards sustainable energy development can be measured by various indicators which are critical in the energy transition to sustainable energy (Davidsdottir and Sayigh, 2012).
6 Sustainable Energy and Electricity Generation
Sustainable energy or electricity refers to the generation and supply of electricity in a way that does not compromise the ability of future generations to meet their own energy or electricity needs (Hollaway and Bai, 2013). It can also be defined as energy sources that do not get depleted in a time frame that is relevant to humanity and hence contribute to the sustainability of all species (Lund and Lund, 2010). Sustainable Energy, just like sustainable development requires significant changes in the way things are done and the exact things that we do with effects on the industrial, production, social infrastructure, and value systems. The development of clean energy would unlock many challenges to sustainable development (Kabeyi and Oludolapo, 2020c; Kabeyi and Olanrewaju, 2021a; Kabeyi and Olanrewaju, 2022). Sustainability is a major concern today as a direct result of the serious concerns over the climate change, of which electricity generation is an important contributor (Vine, 2019). Electricity is a critical product needed to support life, welfare, and global sustainable development (Berga, 2016). Currently, humanity is faced with a significant challenge to realize new sustainable development Goals (SDGs) by the year 2030 (Berga, 2016; Kabeyi and Oludolapo, 2020b). Sustainable development and its correlation with energy became a significant global concern and issue in the 2002 Johannesburg world summit on sustainable development (CS-UNIDO, 2008). Determination of the most appropriate energy systems in an electricity mix is considered as a strategic approach in realization of sustainable development (Ebrahimi and Rahmani, 2019; Kabeyi and Olanrewaju, 2020b). Electricity generation systems can be assessed by a five-dimensional approach consisting of environmental, economic, social, technical, and institutional sustainability as a strong measure of energy sustainability (Ebrahimi and Rahmani, 2019). Therefore, sustainability in energy development seeks to achieve technical sustainability, political or institutional sustainability, social sustainability, environmental sustainability, and economic sustainability which is greatly realized by the development and use of renewable energy resources (Kabeyi, 2020a). Figure 8 illustrates the five main dimensions of energy sustainability.
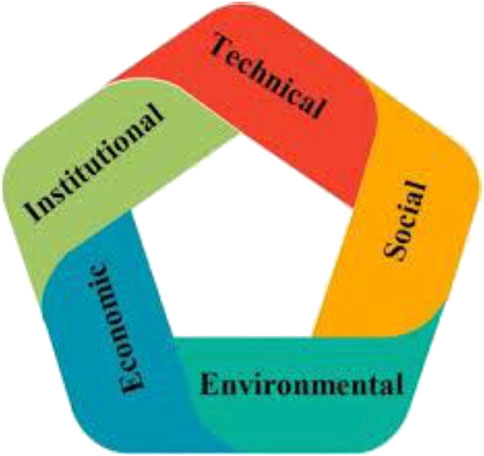
FIGURE 8. Dimensions of energy sustainability (Kabeyi, 2020a).
Figure 8 above summarizes the main dimensions of energy sustainability particularly electricity. The main dimensions of sustainability in energy development and consumption are environmental, social, political, economic, and technical sustainability.
6.1 Technical Sustainability
Technical sustainability of electricity generation refers to the ability to meet the current and future demand in a safe and efficient manner with the use of clean sources of energy and technology (Kabeyi, 2020a). Unsustainable production and consumption of energy resources is the main cause of environmental damage in many organizations and countries globally (Liu, 2014). To realise sustainable development calls for changes in industrial processes and systems, in terms of the type and quantity of energy and other resources for waste management, emission control as well as product and service range or type (Krajnc and Glavic, 2003). Through the development and adoption of appropriate technology, humanity can make development of energy sustainable. Engineering and technology are closely linked to sustainability, but the engineering input so sustainability must be in partnership with other interests by application of constraints to the three pillars of sustainability which are social, environmental, and economic pillars. All engineering systems, products, operations, services, and infrastructure have designed and actual life after which they should be subjected to sustainability analysis by application of three measures in order of priority. These measures are reuse, recycle, and disposal (The Royal Academy of Engineering, 2005).
For evaluation of energy or electricity sources, a number technical and operational indicators have to be analyzed (Dobranskyte-Niskota et al., 2009). The typical technical evaluation criteria for energy systems and sources include efficiency, exergy efficiency, primary energy ration, system reliability, maturity, and safety (Şengül et al., 2015). Other important powerplant performance indicators in power plant generation are specific fuel consumption, specific emissions, power plant availability, load factor, among others.
The transformative and disruptive potential of rapid technological changes, and the danger of using primitive technologies should be avoided for a sustainable transition. Even with advanced science, technology and innovation policies, and technologies, it is unlikely to deliver progress regarding global development unless the environment nurtures learning and innovation for effective management of innovation systems. Both national and international policies should promote international technology assessment and foresight and cooperation including collaborations and technology transfer. This cross border partnerships and cooperation will facilitate rapid sustainable energy development (United Nations, 2019b).
6.2 Environmental Sustainability
Environmental sustainability is concerned with managing the negative impact of energy production and use to the society and to magnify or extend the good ones. The environment should never be allowed to absorb more than it can contain, naturally or artificially (Iddrisu and Bhattacharyya, 2015). Environmental sustainability is concerned with the integrity of natural environment and its ability to remain resilient and productive in support of humanity (Kolagar et al., 2020). Environmental sustainability is further concerned with the integrity and carrying capacity of the natural environment to sustain humanity as a waste sink and source of raw materials (Mensah, 2019). Thus, the environment or ecological dimension of sustainability is concerned with preservation of the environment and habitats, especially against the impacts of waste disposal, excessive consumption of Earth’s resources, and greenhouse gas emissions. The gases that lead global warming include, carbon dioxide, methane, and nitrous oxides. Carbon emissions in the atmosphere have increased from about 280 ppm by volume during pre-industrial times to over 400 ppm today representing more than 40% increase. In the United States, fossil fuel consumption results in average annual emission of about 5.3 billion metric tons of CO2 into the atmosphere (Nag, 2008). The origin of these carbon emissions is from energy consumption, non-energy use and industrial processes like iron and cement production.
The typical environmental evaluation criteria of energy systems include emission levels for SO2, NOx, CO2, particulate emissions, non-methane volatile organic compounds, land use and requirements (Şengül et al., 2015). The main environmental dimension indicators for energy technology assessment include: GHG emissions, environmental external costs, radionuclides external costs, severe accidents perceived in future and fatal accidents from the experience. Additional environmental indicators are land use and solid waste. Life cycle emissions of GHG emissions in kg (CO2-eq.)/kWh are selected to assess electricity generation technologies according policies like the EU environmental policy on climate change mitigation (Streimikienea et al., 2012). GHC emissions in kg CO2eq./kWh were selected instead of external costs of GHG emissions because of the large uncertainties related to evaluation of external costs of GHG emissions. Climate change is the dominating environmental concern of the international environ-mental political discussion of today. Global warming is not only an issue for the environment, but rather for human society, since rising global temperatures might have serious consequences on the economy and social life. The indicator reflects the potential negative impacts of the global climate change caused by emissions of greenhouse gases to produce 1 kWh of electricity. This indicator was used in almost all studies on energy technologies assessment survived (Streimikienea et al., 2012).
Therefore, an environmentally sustainable energy systems should maintain a stable resource base, avoiding energy resource over-exploitation, and avoid depleting non-renewable resources through development of adequate substitutes. These approaches include biodiversity maintenance, atmospheric stability, as well other ecosystem functions that are not necessarily economic resources (Jonathan, 2001).
6.2.1 Atmospheric Temperature Changes
For the world to have a stable atmosphere, it is recommended to maintain temperature increase of 1.5–2°C above preindustrial level which then translates to 400–450 ppm of CO2 equivalence (Dyllick and Hockerts, 2002). The most widely accepted climate change scenarios and projections predict annual temperature increase of 1–3.5°C in coming decades based on existing scenarios (Butt et al., 2012), hence the need for more global commitment. The Intergovernmental panel on climate change (IPCC) predicted that greenhouse gas emissions (GHG) will lead to global temperature increase of between 1.1 and 6.4°C by the end of the 21st Century with cities like London, Los Angeles and Phoenix having already experienced about 1°C average temperature rise within a decade. The United Kingdom (UK) is expected to experience temperature rise of about 3.5°C by 2050 which will also be accompanied by increased winter precipitation of up to 20% as well as increased incidences of storms (Hulme et al., 2002; Intergovernmental Panel o, 2007). This predicted temperature rise is higher than the target set by the Paris Conference of 2015 (COP21) of 2°C above pre-industrial levels (Berga, 2016; Lu, 2017). These statistics paint a picture of a world that is already missing its targets that are necessary to save mother nature and humanity.
6.2.2 Ecological Sustainability
Ecologically sustainability requires organizations to use only natural resources that are consumed at rates that are below their natural reproduction or replenishment rates or at a rate less than the development rate of substitute products or resources. They should also ensure that emissions which have potential of accumulation should not be allowed to accumulate at rates that are more than the capacity of the natural removal or absorption from the ecosystem system (Kabeyi, 2019a; Barasa Kabeyi, 2019b; Kabeyi, 2020b). Sustainable organizations should never engage in actions or activities that degrade any part of their eco-system (Dyllick and Hockerts, 2002). Natural resources should be consumed by mankind at rates that allow them to replenish themselves (University of Alberta, 2021). Therefore, environmentally sustainable systems should maintain a stable resource base free from over exploitation of energy resources. Ecological sustainability also advocates for maintenance of biodiversity, stability of the atmosphere, and other economic and non-economic ecosystem functions and resources (Jonathan, 2001), while non-renewable resource substitution by renewable sources is a solution for the depleting ad polluting resources like fossil fuels namely petroleum, coal, and gas.
Environmental management and protection are a significant indicator of peoples’ culture, values, and ethical principles. Ethics involve making decisions based on acceptable values. This calls for social movements that constitute useful sources of cultural values and environmental movement to influence the environment is managed (Kabeyi, 2018a; Kabeyi, 2018b). Therefore, sustainable environmental management is a result of multiple actors and factors. Environmental management for sustainability is concerned with control of interaction with the environment to protect and enhance human health and welfare together with environmental quality. Energy production, conversion, delivery, and end use can have serious environmental consequences to air, land, and water quality which are important for preservation of human life. These environmental issues related to energy are discussed below (Kabeyi and Olanrewaju, 2020b).
6.2.3 Water Pollution
Energy-related processes impact on water through discharge of water polluting solid and liquid wastes, thermal pollution from waste process heat, and excessive consumption of freshwater, and negative impact on aquatic life. Chemical contaminants like acids from mining operations, and discharge of coal ash to water bodies, radioactive wastes from nuclear power plants as well as oil spills from diesel power plants are common scenes from power plants (Raja et al., 2006; Rajput, 2010). Although considered as gaseous and hence air contaminant, Carbon dioxide (CO2) causes acidification of oceans with serious negative impacts on aquatic life. Process improvements and technology can help mitigate this pollution of water from energy related activities (Nag, 2008; United States Department, 2015). Buried nuclear wastes can cause contamination of underground water source. Energy-related atmospheric emissions of conventional pollutants such as particulates, sulfur, and nitrogen compounds have been reduced through improved combustion strategies and exhaust scrubbing while transition to cleaner energy sources is also proving to be effective (Raja et al., 2006; Nag, 2008; Rajput, 2010; Kabeyi, 2020a). Used brine can also contaminate surface water in geothermal powerplants in powerplants with no reinjection (Kabeyi, 2019b; Kabeyi, 2020c). Some technologies may not be water polluting but the process through which the plant and equipment are producing may be highly polluting.
6.2.4 Land Contamination
Energy and power generation related activities contaminate or pollute land in various ways. Land pollution takes many forms like deposition of atmospheric pollutants with precipitation, direct discharge, and accumulation of pollutants like coal ash from coal power plants. Oil spills from diesel power plants, soil extraction or excavation for fuel mining and production or associated with energy plant and infrastructure siting and development. Although regarded as clean power sources, wind, and solar power plants occupy huge tracts of land which may no longer be used for other economic activities like farming and human settlements. Cases of induced seismicity have been experienced in geothermal power development and generation which affects land use (Barasa Kabeyi, 2019a; Kabeyi, 2020c; Kabeyi et al., 2020). Radioactive wastes from nuclear power plants may also be buried underground rendering the area useless for other economic activities (Rajput, 2010). While liquid and gaseous emissions and effluents still must be handled by nuclear power plants. Buried nuclear wastes can cause contamination of underground water source. Energy-related atmospheric emissions of conventional pollutants such as particulates, sulfur, and nitrogen compounds have been reduced through improved combustion strategies and exhaust scrubbing while transition to cleaner energy sources is also proving to be effective (Raja et al., 2006; Nag, 2008; Rajput, 2010; Kabeyi, 2020a). Lack of reinjection in geothermal power plants can lead to land contamination by the used geothermal fluid (Kabeyi, 2020c; Kabeyi and Olanrewaju, 2021a).
6.3 Economic Sustainability
Economic sustainability in energy and electricity production and use refers to the ability to meet demand in a cost-effective manner. It also a measure of access to requisite financing for energy resource development. The cost-effective operation will ensure that the energy system is s economically viable and feasible and hence makes the investment attractive to investors and financiers (Kabeyi, 2020a). All economies are made up of markets where transactions are made. The main activity in an economy is production of goods and services, distribution and consumption (Mensah, 2019). Therefore, economic dimension of energy sustainability is concerned with the viability of individuals and organizations, products, and services in production and consumption of energy or electricity, distribution, and interactions.
Economic sustainability seeks to maintain the operational stability in terms of liquidity and cash flow and ensure fair or reasonable income and benefits to investors and other stakeholders in energy systems (Dyllick and Hockerts, 2002). Electricity is the most multipurpose energy carrier globally, and therefore it is highly linked to human and economic development (Bazmi and Zahedi, 2011). For economic sustainability to thrive, organizational policies, and operations should not retard economic progress, development, or affluence of society (Hasna, 2007). It is through economic sustainability that humanity can maintain independence and have unlimited access to the required energy resources. Economic sustainability is realized from energy systems if they remain intact while activities and processes are equitably accessible to all to secure their livelihoods in a fair manner (University of Alberta, 2021). Therefore, energy or electricity system must continuously produce goods and services to manage debts, pay bills, pay workers ensure sectorial balance with stable agricultural and industrial production (United States Department, 2015). Energy systems and organizations should remain profitable and useful from one generation to another generation (Kabeyi, 2018b). Therefore, this implies that energy systems are economically sustainable if they are operated profitably by investors or organizations.
Energy costs are embedded in every commodity and service in an economy and therefore the economy requires better and efficient energy technologies to reduce energy costs leading to affordable electricity. This will enhance the level of economic activities through better supply reliability, reduced import bill, and a bigger market for energy goods and services. This leads to higher gross domestic product and balance of payments at a macroeconomic level (United States Department, 2015). The various elements of economic dimension of energy sustainability of include corporate sustainability, energy costs, supply disruption loses, energy import bills, energy technology, and service market.
Renewable energy projects make use of local labor from rural areas, businesses, local material and business, local investors, and other services. Therefore revenue from renewable energy electricity revenue is invested back to local communities in form of taxes, payments for materials and labor as well as profits to investors which leave more economic benefits than imported fossil fuels or imported grid power (Kumar and Okedu, 2019). Different renewable energy sources have different socioeconomic value. For example, biofuel projects create more jobs as compared to jobs created by solar and wind powerplants which gives the different projects a unique rank in socioeconomic evaluation of energy options. The cost and price of generated electricity is another important economic aspect of power generation projects and has a bearing on electricity sustainability (Akella et al., 2009).
In economic evaluation of energy systems, typical evaluation criteria include total cost of investment, operation, and maintenance cost, fuel cost of generation, electricity cost, net present value (NPV), service value and equivalent cost of the energy system (Şengül et al., 2015). The Economic dimension in sustainability assessment of energy technologies and projects is significant since energy or electricity supply cost influences technology adoption and penetration. Indicators that address economic dimension of energy sustainability assessment in electricity and heat generation and supply include the private or investors costs involved, the fuel price increase sensitivity, energy or plant average availability factor, costs involved in grid connection, energy or plant peak load response, and energy security of supply. Very important economic investment indicators are private costs, availability factor and costs of electricity grid connection (Streimikienea et al., 2012). Goods and services should be produced in a continuous manner in an economically sustainable system to be realized. Should produce goods and services continuously, to maintain sustainable levels of debt, and (Jonathan, 2001).
6.3.1 Energy Costs
Several factors influence the cost of energy and electricity and hence the price paid by consumers. These factors include the type of primary energy commodity used, availability of supplies or resources, primary sources price or cost, the capital costs of the power plant and operating costs incurred to convert or process the supplies into energy services like electricity, and prevailing energy demand. The variation in energy cost for various sources of energy leads to market competition among energy resources and services, with alternatives sources of energy. Unfortunately, the costs associated with energy security and environmental factors are often not fully included in the market price of energy sources. Reduced energy costs generally contribute to improved performance in many sectors of the economy, hence the need for low-cost energy and electricity supply. The reduction in cost of solar and wind power generation can significantly affect the competition with other, more traditional generation options like fossil fuels (United States Department, 2015).
Energy systems respond to changes in input price and technology at different rates to in the energy sector and markets. The price of energy responds to the supply and prevailing demand which is dynamic. The factors influencing price include inventory level, level of economic activities, political factors, environmental factors, and market speculation which can drive market price volatility. These instability and volatility in energy price makes planning complicated and difficult, and hence negatively impact the entire economy. It is desirable to have a diversified portfolio comprising of many different sources of energy supply and enabling technologies to provide feasible options that can allow one to hedge the risk of being dependent on a single energy supply (United States Department, 2015).
6.3.2 Energy Related Disruption and Losses
Energy disruptions can occur on the supply side, consumer side or transmission and distribution infrastructure any time, whether planned or not. Any electric power outage causes substantial economic costs and losses to the businesses and activities most of which depend on electricity. As an example, study by Lawrence Berkeley National Laboratory in 2006 on the cost of power outages estimated that disruptions to the U.S. electric power system cost between $22 and $135 billion per year with common causes identified as weather-related events like falling trees, and equipment failures like transformer failures. In another study, it was found outage-related costs ranging from $20 to $50 billion per year for weather-related outages alone. These losses are worse off if damages due to extreme weather events like Hurricanes are considered. The solution to this significant loss is improvements to the transmission and distribution systems (United States Department, 2015). Sustainable engage should not only be affordable but reliable in supply, generation, and transmission.
6.3.3 Energy Import Liabilities
Energy business has significant impact on the balance of payment positions for importing and exporting countries for example the US spent approximately $190 billion in 2014 on petroleum imports. Oil importing countries must content with energy insecurity and fluctuating price and supply of petroleum. Electricity can also be imported and exported between countries leading and sharing of resources with resource rich countries especially with desirable renewable and low carbon sources like hydro and nuclear. Reducing dependence on energy imports reduces the impact of supply disruptions while promoting local investment in sustainable energy options (United States Department, 2015).
6.3.4 Energy Technology Markets
Electricity generation and distribution is a big business in all countries while production and export of energy resources like fossil fuel sustains many countries’ economies with some almost entirely dependent on oil and gas exports. Other commercial primary resources include coal while some countries have relied on charcoal business to generate significant revenue. Export of energy production equipment like generators, turbines, boilers, and other plant equipment represents a substantial market opportunity for many countries like the United States and often generate high-value jobs. The International Energy Agency (IEA) predicts that clean energy will supply between $7 trillion and $10 trillion investment in electricity generation of which $6 trillion will be renewable sources and $1 trillion in low carbon nuclear power generation over between 2015 and 2025. It is observed further about two-thirds of this investment will be done in emerging economies. Additionally, energy efficiency investments will account for a further $8 trillion of investment (United States Department, 2015).
6.4 Social Sustainability
Social sustainability is concerned with the rights of the community as measured by the level of social acceptance and access to the energy resource and systems by the people (Iddrisu and Bhattacharyya, 2015). Social sustainability is the ability to preserve desirable social values, institutions, traditions, and social characteristics of the society before and after a project or an intervention. It is also concerned with social justice and therefore addresses aspects like labour practices, variance in production standards, and promotion of equity among all people (Kabeyi, 2018c; Kabeyi, 2019a; Kabeyi, 2019c). Social sustainability can be achieved by the selection and development of technology and powerplants that provide adequate power and employment to local communities and that don’t interrupt or interfere with their established way of living and value system (Liu, 2014; Kabeyi, 2020a; Kolagar et al., 2020). Therefore, social sustainability implies that people are important because development is basically about the people themselves. Principles that should be applied in energy development to realize social sustainability include accountability, empowerment, participation, cultural identity, and institutional stability (Mensah, 2019).
At institutional level, sustainability is grounded in environmental initiatives which were sometimes referred to as green corporate initiatives. This is the ability of an organization to endure, take care of the needy in society and ensure institutional responsibility for greater good of the human society (Samaras et al., 2019), by taking care of the world’s most vulnerable people in society. Any effort to achieve financial gains for the few while ignoring the needs of the majority is no longer acceptable, reasonable, productive, or justifiable (Kabeyi, 2020b). Therefore, socially sustainable organizations attempt and succeed in adding value to the communities within which they operate or do business. This is achieved by increasing the human capital base of individual partners and social capital of the community. Organizations should manage social capital in a way that stakeholders do understand the objectives and motivations for general agreement and cooperation (Dyllick and Hockerts, 2002). Therefore key requirements for social sustainability of energy transformation is openness and democracy in the process (Miller et al., 2013). Therefore, engaging in community social responsibility (CSR) activities is a strategy for increasing social sustainability of energy activities.
Social sustainability assumes two types of sustainability, i.e., social capital and human capital. Social capital is concerned with quality of public services like good education, water, infrastructure or a supporting culture and value system. Human capital is primarily concerned human skills, level of motivation, and loyalty of employees and business partners in their own capacity (Dyllick and Hockerts, 2002). Therefore, human capital is an intrinsic phenomenon of an individual or individuals while social capital is concerned with communal or common physical projects, infrastructure or facilities meant to improve the quality of human beings in each social system. This implies that sustainable energy systems should have a positive impact on both human and social capital of the society.
Individual organizations have a critical role to play to ensure social sustainability of energy systems during the energy transition. Social sustainability requires organizations to act in a manner that creates welfare to society and all its people and should be ready to take responsibility for their actions (Mohamed et al., 2020). A socially sustainable organization should internalize the social costs, develop the capital stock, exceed the social carrying capacity, enhance self-renewal of natural systems, nature openness, accountability, and democracy, enhance human choices and practice fair distribution of available but scarce energy and other resources among all stakeholders. It is necessary that social sustainability should preserve human rights and human dignity and guarantee equitable access to necessities which leads to a healthy and secure society. Communities that are healthy have fair leadership which ensures that personnel, labor, and cultural rights of all are respected to the later (University of Alberta, 2021). Therefore for energy resources and electricity systems to be socially sustainable, they should be characterized by equity, reliable supply of social services, address gender equity, facilitate political stability, address accountability issues, and nature participation in their governance systems (Jonathan, 2001). Social sustainability requirements call for energy practitioners, organizations, and countries, local and international organizations to go beyond just energy solutions and ensure holistic approach in as far as energy transition is concerned. Energy transition should address human physical and health, system and human safety, human rights, and dignity for sustainable energy development to be achieved.
Energy policies necessary to realize sustainability should be guided by a mixture of robust, objective, empirical, and theoretical principles that consider the impact not just to the current generation, but equally on future generations. Energy resources and electricity systems should consider socio-technical impacts on man and machines for them to be socially acceptable. Adoption of new electricity or energy technologies should bear in mind that systems are operated by man and should therefore be acceptable and comfortable by design and adequate capacity building should be done for man to be comfortable and therefore embrace new ways and systems. The impact of the technology change or acquisition on current and future financial systems, school system, labour market composition, organizational culture and political aspirations of the people should be considered in the energy transition if it has to be sustainable and avoid failure or negative perception by the people (Miller et al., 2013). This implies that man is a very important aspect of the energy transition and should be at the center of the transition and be involved using various participatory methodologies, otherwise the transition may never succeed.
Any social evaluation of energy systems should consider social acceptability, expected job creation, and other social benefits of the energy systems (Şengül et al., 2015). Significant social indicators for energy and electricity technologies are related job opportunities, health effects, food safety and security risk and work-related accidents and fatalities. Technology specific job opportunities in person-year/kWh indicator are based on the average amount of labor used to produce a unit of electricity (Berga, 2016; Lu, 2017). The quality of electricity or energy related work is addressed by Work Quality indicator is based on knowledge and training of average worker in each technology chain, using an ordinal scale indicator (Streimikienea et al., 2012). A system is said to be socially sustainable if it guarantees distributional equity, provides social services like education, health, guarantees gender equity as well as political accountability and adequate public participation of stakeholders (Jonathan, 2001).
Therefore, social sustainability of the energy transition is concerned with the value to community, democratic participation, direct benefits through addition of human and social capital, job creation and other community benefits through activities like corporate social responsibility and respect for local customs, traditions, and beliefs.
6.5 Political and Institutional Sustainability
The development of new energy technologies, new business models, and new policy priorities and frameworks need new market participation and control models and rules and regulations which require new governance and new institutional design (De, 2021; Lenhart and Fox, 2021). Transition historical studies show that whereas technological innovations and market actors are the main drivers of change, extensive studies claims that it is governance systems that influence the distribution of the benefits of new technologies to the society which is an important requirement of sustainability (Kuzemko et al., 2016; Kabeyi, 2020a). The road to the global low-carbon transformation should deal with the climate crisis is within reach, but this requires political actions from world leaders. There is need for action along multiple approaches and models globally that can be scaled up and adapted to suit specific national prevailing circumstances. Cost-effective a low-carbon technologies are available in many fields with several under research but the rate of adoption is still a serious concern because governments need to put in place the right policies, regulations and a facilitating legal framework for faster and successful adaption (Watson, 2014; Krzywda et al., 2021). Political or institutional dimension of energy sustainability is concerned with governance of sustainable energy transformation at all levels. This is achieved by setting and implementing policies and regulations with different political institutions influencing governance choices (Kuzemko et al., 2016). This implies that the political dimension of energy sustainability is concerned with the strategic planning and definition of the energy system and related systems and processes. Therefore, political sustainability concerns address the future structure and indicates some issue on political stability and foreign policies of the energy system (Kabeyi, 2020a). For development to be sustainable, there is need for adequate management of the tradeoff between social equity, protection and integrity of the environment, real economic development and progress and preservation and use of natural resources for equitable use by all (Robyns et al., 2012). This can only be achieved effectively when we have the political will from all if not many nations and groups that have power to influence energy policy (Kabeyi, 2019a).
The institutional dimension of sustainability defines the role of local participation in the control and management of energy resources and energy systems (Kabeyi, 2019a; Kabeyi, 2019c). The institutional dimension embodies elements like local ownership, participation, local contributions, local skill base, local policy and regulation, protection of investors and consumers and sharing of resources and benefits accruing. This dimension is the one that defines the system structure and framework of processes, systems and policy decisions which affect the project or investment (Iddrisu and Bhattacharyya, 2015; Kolagar et al., 2020). There is evidence that highly or adequately institutionalized countries with efficient and effective energy related institutions are more successful in managing the energy transition. This is because the institutions encourage innovation, efficient resource allocation and set desirable policy, legal and financial measures and instruments which are enables of sustainable energy transition (Inglesi-Lotz, 2021).
6.5.1 Politics and Sustainable Energy Transition
Energy activities, products and services constitute big business globally. In 2015, four out of eight top Fortune 500 companies, were energy related companies like Exxon Mobil, Chevron, Phillips 66, and General Electric. Therefore, energy represents a big portion of global economy and therefore it affects jobs, people’s incomes, company performance, profits, and personal or individual economics. Within these dynamics, politics become important especially as an enabler of business through various instruments at their disposal. Internationally, government subsidies have helped the development of new technology for solar and wind power. Many governments subsidize the oil and nuclear power industries which complicates the viability of renewable energy resources and technologies (United States Department, 2015).
Politics play a leading role in the coal industry which continues to survive and thrive in many countries like the United States because of exemptions from federal pollution regulations making its use competitive. The same is true for hydrologic fracturing or fracking, which has survived in the US because of the 2005 Clean Water Act. The US government also subsidizes pipelines and supports military actions in the Middle East as a strategy to ensure a stable and reliable supply of fossil fuels. Energy has also been a leading cause of most political tensions between countries. These tensions or conflicts shape the decisions all countries make about their energy resources which ultimately affects their electricity mix so as to manage costs, security and environmental concerns besides shaping international relationships (Dufour, 2018).
6.5.2 Energy Policies, Regulations, and Governance
Governments should strive to meet the growing energy demand but also meet environmental requirements. To realize these demand and sustainability challenges, Governments should develop regulations and policies that seek to meet the growing energy needs and concerns over emissions and global warming. It is necessary to develop sustainable energy policies to provide relevant and suitable policy recommendations for end-users (Lu et al., 2020). Policymakers should develop sustainable solutions and a conducive environment for a sustainable energy transition. Good governance in the energy sector is an important tool to realise climate change mitigation by investing in sustainable measures and projects. There is need for public intervention by putting in place what is considered as a conducive energy transition’s regulatoryframework. Renewable energy projects and other sustainable energy investments, just like any other investment, require political stability, proper regulatory frameworks, good and effective governance and secure property rights (Inglesi-Lotz, 2021).
Energy policy issues are political in nature, and act as instruments through which governments can influence sustainability in the society. Governments should develop energy policies which can alter consumption habits and patterns, reduce fossil fuel dependency and environmental conservation and protection while stimulating investment and development of clean energy technologies. Interest groups represent interests from energy conservation proponents to nuclear power opponents (Marcus, 1992). Interest groups representing various groups and stakeholders from energy conservation to nuclear power opponents need to be heard during policy formulation on sustainable electricity (Marcus, 1992). Energy policy seeks to establish security of supply, energy affordability, and minimum impact on the environment.
Institutions in energy transition generally refers to the formal and informal rules and their enforcement (Inglesi-Lotz, 2021). The quality of these institutions is measured in terms of ability to create a conducing environment characterised by the following indicators and dimensions.
6.5.2.1 Voice and Accountability
This refers to the extent to which the people in a country can choose and challenge the government of the day which limits executive authority.
6.5.2.2 Peace and Political Stability
The citizens of a country have no incentive to invest in their future in environments of political instability or civil strife. This therefore makes sustainability concerns secondary to the need for immediate survival.
6.5.2.3 Government Effectiveness
Government effectiveness is the quality of public services and the degree of freedom or independence from political pressures and interference. This creates an enabling environment for private sector investment in energy.
6.5.2.4 Regulatory Quality
Regulatory quality is the ability to formulate and implement appropriate policies and regulations that facilitate private sector growth and development. This requires that government lays down fair and uniform rules of economic engagement.
6.5.2.5 Rule of Law
Investment in sustainable energy requires suitable laws governing quality of contract enforcement, private and public property rights, effective police, and courts for arbitration and the enforcement of the rules of society.
6.5.2.6 Control of Corruption
There is need for a strong anticorruption prevention for more the more economic success as a Corruption inhibits investment and increases the cost of doing business and lowers competence and efficiency of performance which in itself and indicator of lack of sustainability (Kabeyi, 2020b).
6.5.2.7 Ease of Doing Business
This is a measure of the multitude of aspects that influence the extent to which the regulatory environment is facilitates business operations. Investment in sustainable renewable energy projectscan delayed or abandoned by too complex and lengthy bureaucratic procedures and corruption (Inglesi-Lotz, 2021).
A sustainable energy transition calls for the design of an appropriate market structure for proper performance of the energy sector in terms of prices, energy efficiency, supply, and technological innovation. Governance mechanisms directly influence the market structures and influence investment decisions. Therefore, bad or improper market structure designs and policies can lead to higher costs of the energy sector unnecessarily which impacts negatively on the welfare of consumers (Inglesi-Lotz, 2021).
6.5.3 Energy Security, Risks, and System Resilience
Energy security generally refers to low probability of damage to acquired values. Energy security is best defined by the four As of energy security which refer to availability, affordability, acceptability, and access to energy resources and systems (Cherp and Jewell, 2014). Energy security is therefore the degree of vulnerability on vital energy resources and systems which is influenced by the degree of exposure to energy related risks, its resilience and links to important energy and social systems. Energy security issues emerged in the early 20th century with respect to the supply of oil to the military more so in the frontline. Academic reflections on energy security emerged in 1960s and became real in 1970s with the oil crisis. It remerged in the 2000s with concerns over rapid demand growth for energy in Asia and gas supply disruptions in Europe and the current pressure over emissions and global warming concerns (Cherp and Jewell, 2014; Austvik, 2016).
Energy systems are entangled with human and national security with reliability concerns shaping public opinion and policy as well as political decisions and agenda with implications on the economy and political systems (Austvik, 2016). It is the desire of everybody and every nation to have uninterrupted supply of vital energy services and hence, energy security is a priority for all nations (Jansen and Seebregts, 2010). The security concerns are robustness, i.e., resource sufficiency, system reliability, stability, and affordability; sovereignty which include protection from internal and external threats; and resilience which is ability to withstand disruptions of energy systems. For many countries, energy insecurity means lack of self-sufficiency and having aging infrastructure, while insecurity issues among developing countries additionally includes lack of adequate capacity, high energy intensity, and high demand growth that is more than ability to supply. For low-income countries, multiple vulnerabilities overlap, making them seriously energy insecure (Jacobs et al., 1987).
Energy security is a very important policy driver with privatization of the electricity sector being used as a tool to secure cheaper energy supplies in some countries in the short term. However, this has led to contrary effects in some places because of stiff competition, resulting in delayed and deferred development of power plant and infrastructure caused by prolonged uncertainties on viability (Bazmi and Zahedi, 2011). Renewable energy sources have the potential to help nations become independent from foreign energy supplies and mitigate risks from conflicts and other disruptions to vital energy resource supplies because most of them do not rely on imports unlike fossil fuels sources (Ölz et al., 2007). A typical example of an energy conflict involved the expansion of the existing pipeline between Germany and Russia through the Baltic Sea. This caused international disputes with the US warning Germany with sanctions (Dettmer, 2019). In this energy project, countries like Poland and Ukraine heavily criticized the pipeline, fearing that Russia would use it for political gain and escalate regional conflicts through arming Eastern European countries (Gurzu, 2019). This is because of the concern that the pipelines can deliver gas to German directly and hence by-pass Ukraine and thus escalate the conflict with Ukraine.
In another case of energy insecurity, the first oil crisis in 1973 brought about a reduction of about 30% in the supply to of oil to Japan leading to Japanese economic downturn and recession in 1974. These reminded policy makers in Japan that energy supply is a serious security issue and should be managed (Cheng, 2009; Mihut and Daniel, 2013). The case was similar in South Korea which was also seriously affected by the first and second oil shocks of 1973 and 1979 (Miller et al., 2013; Azad, 2015). The supply shocks demonstrated that energy supply and national security are seriously intertwined. In 2011, the Tohoku earthquake in Japan brought about massive disruptions to energy supply after Japan was forced to shut down its nuclear power plants after the nuclear accident at Fukushima Daiichi power plant leading to increased use of fossil fuels. This caused significant increase in fossil fuel demand and supply (McCurry, 2015; Energy Information A, 2020). Today, Japan and South Korea have shifted their electricity generation from oil based and to liquefied natural gas and coal which is steal a fossil fuel but with less environmental impact (Korea Energy Economics In, 2017; Ministry of Economy, 2018).
In yet another case of energy insecurity, Taiwan, experienced a massive power outage in the northern half of the island in 201 that lasted 5 h causing an estimated damage of three million US dollars. Although this was partly blamed on human error, and structural challenges within Taiwan Power Corporation, a critical analysis showed that operating electricity reserves had significantly reduced from 6% to just 1% within 1 week to the blackout (Yu, 2017). Therefore, adequate energy planning has an important role to play in energy supply security and that unreliable or insecure energy system can be quite destructive and costly to any economy.
There are several energy related risks to national energy and electricity security, and can be broadly categorized into physical, cyber, economic, and conflict-related risks although with significant overlaps among these categories. Energy technologies must be robust and resistant to these vulnerabilities if they must be sustainable and secure.
6.5.3.1 Physical Energy Risks
Energy security risks are related to the damage and disruption of energy supply, energy storage, and delivery infrastructures. Several energy infrastructure and assets are susceptible to damage and disruptions. Energy infrastructure include the electrical grid infrastructure system, pipelines for oil and gas, and rail transport network and infrastructure, as well as road and marine systems. Examples of damages to the infrastructure include Hurricane Sandy effects and the attacks on substation facilities and power plants as a result of extreme weather with climate change raises these risks (United States Department, 2015).
6.5.3.2 Cyber Security Risks
Cyber security refers to vulnerabilities that compromise the computer-based systems and related operations and functions like data inputs, data analysis, and data processing, the real time operation and coordination of electricity supply systems, energy delivery, and end-use systems control in smart grids. There is need to validate and manage all data inputs, monitor the systems for intrusion, and the need to address other vulnerabilities which come with challenges of maintaining the integrity of these systems. Electricity networks encounter cyber security threats which increase with access to the internet and other computer supported operations as in the case of the smart grid (United States Department, 2015).
6.5.3.3 Economic Energy Risks
Economic energy security risks are related to resource supply and price shocks like the oil crisis of 1973 and 1978. Energy resources that are traded internationally are particularly subject to price and supply fluctuations due to various reasons that include civil war, international war, economic sanctions, and deliberate supply control. The price and supply shocks create uncertainties for energy-dependent businesses, which then invest in energy security measures including research and development of renewable and sustainable energy systems. Supplier related risks include price and supply manipulations. Manufacture of complex energy infrastructure components is often dependent on global supply chains which can be adversely affected by long lead times, long-range shipping logistics, and price volatility (United States Department, 2015).
6.5.3.4 Conflict-Related Energy Insecurity
These are risks that are related to unrest in foreign countries as well as energy fueled domestic or local conflicts. International security risks include those that involve unrest in locations that are critical to global energy supply (Austvik, 2016). They include conflicts in the Middle East which seriously disrupt the supply of petroleum resources. These conflicts also cause deaths, economic meltdown, and environmental disaster like oil spills and destruction of oil resources by enemies in conflicts (United States Department, 2015).
In many countries, conflicts over energy resources are a common denominator. In most of these conflicts, prevailing historical differences and injustices among neighbors has been cited in various areas like religion, tribe, race or even clans and political inclinations. For example, in Syria and Iran, the conflicts appear like they are religious in nature between Sunnis, Shiite Muslims, Kurds, Turkmen, and others while. In Nigeria, it may appear as a conflict is over energy between Muslims, Christians, and other traditional groups, while in the South Sudan, the conflict looks like just differences between the Dinka and Nuer tribes. In Eastern Europe, conflicts in Ukraine, are between Ukrainian loyalists and Russian speakers aligned with Moscow. A deep scrutiny of these conflicts highlighted place energy at the epicenter of the differences, hence the reality is that these conflicts are struggles for control over the principal source of national income which is energy (Marcus, 1992; Dufour, 2018). Therefore, it is only through proper energy resource management that social, political, and economic conflicts can be avoided or resolved. Hence, for energy to be sustainable, governments and groups have a duty to maintain peace and stability and prevent or manage existing and potential energy conflicts within their political environment.
6.5.4 Corporate Sustainability
This is an extension of institutional and political dimension of energy sustainability (Kabeyi and Olanrewaju, 2020b). Organizations and individuals create social impacts that are both positive and negative, through their operational activities. Societies rely on organizations for individual and common good and benefits like employment and social infrastructure, while organizations or corporations need societies to provide workforce and other critical inputs like raw materials to sustain their operations. While we have the interdependence between organizations and society, it is only a healthy and positive society that will create good workers sought organizations (Tharp, 2012). Individuals within the community or society play a significant role in developing sustainable situations and circumstances but organizations can influence this by acting sustainably in their operations and relationships with society (University of Alberta, 2021). Since there is mutual dependence between institution or organizations and society, there is need to have the principle of shared value while making choices and decisions. Sustainability demands for responsibility and facilitates human creativity to develop innovative ways that will further protect the shared environment, respect for all, and empower stakeholders. For organizations, sustainability is important because it creates value and provides them with competitive advantage and leaves a greater positive value to society and stakeholders as well (Nawaz and Koç, 2019).
Energy sustainability programs and activities should facilitate the bridging of the gap between laws and the requirements of good business practice, which include prevention of exploitation, transparence, and accountability to all stakeholders in business or a given system under consideration. This calls for proactive risk identification, assessment, mitigation, and management. This makes sustainability a necessary value and an integral business goal and objective of all organizations including energy companies. Long term success of business operations should incorporate social, environmental, and supply issues in all their undertakings with suppliers, customers, and all other stakeholders. This in return adds value to the respective organizations in terms of corporate reputation, brand visibility, value and equity, better risk management, easier access to capital, talent attraction and retention and higher profitability and return on investment (Kanter, 2021).
Corporate sustainability involves the integration of the economic, ecological, and social aspects in business practice and operations (Dyllick and Hockerts, 2002). The consumption of goods and services is pivotal to enhanced organization’s operational efficiency because sustainable use of goods and services leads to reduced generation of waste during productive operations. The general objective of sustainable corporate development is to realize economic, social, and low carbon sustainability by companies. By embracing sustainable innovation practices, organizations can reduce adverse social and economic impacts of their operations which leads to better corporate performance (Kabeyi and Oludolapo, 2020a; Mohamed et al., 2020).
Environmental sustainability of an organization is an important element of corporate sustainability since it is associated with social consequences of business activities and the environment (Darkwah et al., 2018; Mohamed et al., 2020). An organization that is environmentally proactive should accommodate their stakeholder concerns which leads to better corporate performance and profitability. Environmentally friendly practices are positively related to corporate sustainable performance because of low carbon innovation attitude (Rosen, 2009; Darkwah et al., 2018; Mohamed et al., 2020). Therefore, socially responsible corporate behavior often affects environmental sustainability.
Corporate sustainability strategies incorporate sustainable development principles into business activities and mediation of the relationship between environmental, social sustainability and technology in organizational operations. Nurturing creativity helps to increase environmental, social, and economic efficiency and effectiveness by organizations and in the process, it facilitates advancement of environmentally friendly measures. Therefore, creativity impacts green innovation within organizations and so should be encouraged (Musango et al., 2011; Moriarty and Honnery, 2019; Mohamed et al., 2020).
Various elements have an impact on corporate sustainability which also affects energy sustainability. Energy activities constitute a very important social enterprise with 9 out of 12 most capitalized companies globally engaging in energy business (Miller et al., 2013). Organizations that are economically sustainable have guarantee that they always have sufficient cashflow to ensure liquidity while at the same time they produce above average returns to the investment. Organizations that are ecologically sustainable consume natural resources at a pace lower than the resource reproduction or substitution and they do not pollute the environment with emissions that accumulate beyond the capacity of natural systems to absorb and assimilate them (Kabeyi, 2018b; Barasa Kabeyi, 2019b; Kabeyi and Oludolapo, 2020b; Kabeyi et al., 2020). They also do not engage in ecosystem degrading services. Socially sustainable companies usually add value to the communities where they operate through increment of the human capital of partners. They also improve the societal capital of surrounding communities and are transparent in their activities and operations. (Dyllick and Hockerts, 2002). Therefore, corporate sustainability is a critical part of the wider energy and global sustainable transition.
Therefore, institutional dimension is a very important dimension in determining and influencing investment, competitiveness, prices, investment, and performance of energy sector. The political function sets energy sector institutions, policies and regulations that govern the energy sector and therefore directly influence choices and performance of the energy transition measures and investment.
7 Strategies for Sustainable Energy and Electricity Systems
The sustainable transition strategies typically consist of three major technological changes namely, energy savings on the demand side, generation efficiency at production level and fossil fuel substitution by various renewable energy sources and low carbon nuclear (Lund, 2007; Kabeyi and Oludolapo, 2021b; Kabeyi and Olanrewaju, 2022). For the transition remain technically and economically feasible and beneficial, policy initiatives are necessary to steer the global electricity transition towards a sustainable energy and electricity system. (Bruckner et al., 2014b; IRENA, 2018). Whereas renewable sources energy holds the key for sustainable energy transition, large-scale renewable energy adoption should include measures to improve efficiency of existing nonrenewable sources which still have an important cost reduction and stabilization role (Lund, 2007). A resilient grid with advanced energy storage for storage and absorption of variable renewables should also be part of the transition strategies (Kabeyi and Olanrewaju, 2020b).
7.1 Policy Measures and Initiatives for the Energy Transition
A successful energy transition requires a stable political and economic framework, and support systems, financial measures, technical and as well as well as administrative policy measures to overcome barriers existing as a result of a distorted energy market (Fouquet, 2013). At the center of any sustainable energy strategies is the objective of improving the production and use of energy resources so that they contribute to sustainable development. These requires policies that seek to widen access to reliable and affordable modern energy supplies and while mitigating the negative health and environmental impacts associated with the energy processes and systems. To increase energy supplies comes with economic burdens and so the policies should also foster real socio-economic development to sustain any expansion and access. Measures be taken to make markets work more effectively, and develop new markets while modernizing and expanding old once for efficiency and sustainability in general (Jefferson, 2000).
The focus areas where policy and decision makers should act are as follows.
7.1.1 Develop a Strong Synergy Between Energy Efficiency and Renewable Energy
Policy makers policy design policies that combine the effect of bulk of energy-related decarbonization needs by 2050 in a cost-effective manner through efficient energy production and use (Bruckner et al., 2014b; IRENA, 2018).
7.1.2 Develop an Electric System in Which Renewables Provide a High Share of the Energy
There is need to transform the global energy system through fundamental shift in the way electric power systems are conceived and deployed. This calls for long-term system planning and a shift to policymaking that is more holistic and coordinated across all sectors and nations. There should be timely deployment of infrastructure and the redesign of rules and regulations to achieve cost-effective large-scale integration of solar and wind generation. With their massive potential and renewability with negligible emissions and environmental impact, wind and solar should be made the backbone of electric power systems by the year 2050 to meet the targets set in the Paris agreement (Bruckner et al., 2014b; IRENA, 2018).
7.1.3 Accelerate Electrification in Transport, Building, and Industry
There is need for deep and cost-effective decarbonization of transport and heat sectors by electrification with the bulk of power coming from renewable electricity. Where it is not possible to electrify transport, industry and buildings then other renewable solutions will have to be adopted (Fouquet, 2013). Alternative sources of energy for direct use include modern bioenergy, solar thermal, and geothermal heat applications. The realization of this shift needs the deployment of an enabling policy framework and development of supporting technology and other related initiatives in urban planning, building, transport and industrial sectors (Bruckner et al., 2014b; IRENA, 2018).
7.1.4 Foster System-Wide Innovation
There is need to have continued technological innovation to achieve a successful global energy transition, just the same way new technologies have played a leading role in renewable energy development and deployment. Innovation effort must cover a technology’s full life cycle, that includes demonstration, technology deployment, commercialization, and final disposal. It is worth noting that innovation is broader than technology research and development (R&D) as it includes new approaches to existing energy systems and markets and development of new technical and business models. There is need for coordinated effort by regional, national governments, national and international actors, and the private sector to deliver the needed innovations to facilitate energy (Bruckner et al., 2014b; IRENA, 2018).
7.1.5 Align Socio-Economic Structures and Investment With the Transition
There is need for a globally integrated and holistic approach by alignment of socio-economic systems with the requirements of the energy transition. Implementation of the energy transition requires investments, in addition to those already incurred with respect to the adaptation to climate change. Faster realization of the energy transition would lower the climate change adaptation costs and in addition to reduced socio-economic disruption. This calls for alignment of the financial systems with broader sustainability and energy transition demands that today’s investment decisions made define the energy system of many years ahead. For smooth investment in energy transitions, there is need to allow urgent flows of capital investment to low-carbon solutions, avoid locking economies into a carbon-intensive energy systems and to minimize incidents of stranded assets (Mullen and Dong, 2021).
A smooth and successful energy transition calls for establishment of regulatory and policy frameworks that give all relevant stakeholders a clear, firm, and long-term guarantee of energy systems transformations to meet the emissions and climate goals set. This will create economic incentives that are aligned to the environmental and social costs of fossil fuels and remove barriers to accelerated deployment of low carbon energy systems and solutions (Mullen and Dong, 2021). Energy transition would require increased participation of both private and public institutional investors as well as community-based finance who should be facilitated and motivated with relevant incentives. The requirements and specifications of distributed investment needs including energy efficiency and distributed generation need to be addressed within the socioeconomic structures and investment transformation (Bruckner et al., 2014b; IRENA, 2018).
7.1.6 Ensure That Transition Costs and Benefits are Distributed Fairly
Policies put in place should ensure that the whole society should be involved in a collaborative manner and process to achieve the desired transition. Effective participation is only achievable if the energy transformation costs and benefits are shared and the transition itself is implemented in a fair and just manner. A key requirement and component of a fair and just transition is the universal energy access where all benefit. There is need for the transition scenarios and planning to incorporate access and convergence factors in the transition because there are huge disparities presently in availability of energy services, hence the need for energy services to cover all regions (IRENA, 2018).
A successful energy transition will require the promotion and facilitation of a social accounting framework which enables and visualizes the transition contributions and obligations from the stakeholders in the transition. There is need to make advances in the definition and implementation of a fair context to share costs of transition while at the same time promoting and facilitate structures which enable fair distribution of the benefits of the transition. There is need to explicitly address a just transition considerations from the onset, at both macro, and micro levels, which will enable the creation of structures needed to provide alternatives that allow parties who have been trapped into the fossil fuel dynamics to participate effectively from the transition benefits.
The economic, social, political and technological realities and developments continuously influence the energy mix hence the need to have a rationale in energy system decision making in energy planning and generation deployment (Streimikienea et al., 2012). Renewable energy is considered as a solution for mitigating climate change and environmental pollution; however, an important problem of the application of renewable energy systems (RESs) is that the evaluation of the sustainability of these systems is extremely complicated.
7.1.7 Specific Policy and Legal Measures
There is need to have appropriate policy frameworks, attractive prices for investors and consumers, and a facilitating regulatory framework to realize a sustainable energy transition. Whereas strategies to encourage sustainable energy systems are straightforward, there is need for wider acknowledgement of the challenges and stronger commitment to specific enabling policies (Wanga et al., 2020). It is also necessary to ensure that electricity utilities have adequate generation capacity and that they are financially healthy for them to contribute to sustainable energy and power (Karekezi and Kimani, 2002). Governments should play a proactive role in the transformation, but they cannot single handedly create desired change and the right speed without the involvement and participation of non-state actors to nature the transformation (Pegels, 2010).
With electricity generation being an important contributor to global greenhouse gas emissions, a viable option in the transition is to decarbonize the grid electricity energy sources by use of low carbon and renewable sources (Jefferson, 2000; Colla et al., 2020). Several measures should be put in place to ensure that energy systems promote sustainable socioeconomic development. The main challenges to overcome are expansion of access to affordable, reliable, and adequate energy supplies while addressing environmental impacts at all levels (Jefferson, 2000). With the right policies, prices, and regulations in place, energy markets can achieve many of these objectives. But where markets do not operate or where they fail to protect important public benefits, targeted government policies, programs, and regulations are justified to achieve policy goals. Although strategies to encourage sustainable energy systems are straightforward, there is need for a wider acknowledgement of the challenges and a stronger commitment to specific policies aimed at enhancing sustainability (Wang, 2019; Wanga et al., 2020).
7.2 Electricity/Energy Planning and Resource Allocation for the Energy Transition
It should be noted that a competitive and sustainable energy market is the most efficient allocator of energy resources and provides high levels of consumer service and satisfaction as expected. Thus, a key requirement for any sustainable energy strategy should be to maintain competitive market conditions. However, the market alone cannot meet the needs and expectations of the most vulnerable groups, protect, or preserve the natural environment, and ensure energy security in the face of a complex political environment. In general, governments and societies should put in place proper frameworks to enable competitive pricing and effective regulation of energy markets so as to achieve many of the objectives of sustainable energy (Jefferson, 2000). Therefore, it is critical to have a working policy and supportive political environment.
The selection and deployment of energy and power systems is a multicriterial approach that considers all the dimensions of sustainable energy systems and sources. Figure 9 below illustrates the stages in multicriterial sustainable energy decision analysis.
Figure 9 above shows the recommended steps in the planning for sustainable energy and electricity solutions and options using the multicriteria approach. The process starts with the selection of sustainability indicators for use in selection and planning. Based on the appropriate indicators, appropriate technology is selected from the desirable specifications that were specified. In addition to the social, environmental, and economic indicators which define sustainable development, further considerations are needed to capture the technical and institutional/political dimensions which will guide in the deployment of the best or most appropriate energy and electric power systems.
The multicriteria analysis and decision making often requires the application of energy and electricity models for more effective decision making. The same will also become critical in real time electricity generation and supply for efficient deployment of powerplants and electricity supply as well as consumption by all category of consumers (Bruckner et al., 2014b; IRENA, 2018).
7.3 Renewable Energy in the Energy Transition
Renewable energy sources come from naturally occurring sources which replenish themselves through natural forces. As a source of clean energy that is inexhaustible, renewable energy sources have a significant role to play in the energy transition (del RíoJaneiro, 2016). Although renewable in nature, their consumption should allow for natural replenishment for them to be renewable and competitive (Owusu and Asumadu-Sarkodie, 2016). Strategies to improve access and consumption of renewable energy sources include improvement in conversion efficiency, use of energy storage technologies to deal with fluctuating nature and policies that discourage more consumption of fossil fuels (Sasmaz et al., 2020). Renewable energy sources have been identified as the main solution for mitigation of greenhouse gas emissions and climate change, and environmental pollution (Liu, 2014). Renewable energy sources (RES) can greatly contribute to economic, social, and environmental energy sustainability. The renewable energy sources can be used to improve energy access for most of the population because they are often locally available, reduce greenhouse gas emissions and may create local socioeconomic development opportunities through job creation and improved local economy. (Jaramillo-Nieves and Del Río, 2010). Before the industrial revolution, solar energy was the most readily available form of energy for direct solar application like drying. This changed with the industrial revolution as fossil fuels became dominant source of energy. Fossil fuels constitute the main class of energy sources that cause severe environmental pollution and are thus the main target of substitution with renewable and low carbon sources. The challenge of this substitution is that it may impact negatively on human development (Sasmaz et al., 2020). Sustainable social and economic development goals cannot be achieved without access to clean, reliable, and affordable energy resources and supplies (Kabeyi and Oludolapo, 2020b; Kabeyi and Olanrewaju, 2020b; Sasmaz et al., 2020).
As the world’s population and economy keeps growing, so is the energy demand, a scenario that automatically increase the demand and consumption of conventional sources of energy, particularly fossil fuels (United States Department, 2015; Owusu and Asumadu-Sarkodie, 2016). Renewable energy resources can be used as substitutes for fossil fuels. These sources are characteristically ideal to achieve sustainability in energy use as one of the basic requirements of sustainable development (Wanga et al., 2020). Therefore, replacement of fossil fuels with renewable energy sources in electricity generation is an important measure to reduce carbon emissions (Mohamad and Anuge, 2021).
There are increasing opportunities for companies to increase the use of renewable energy through development in data-driven technology which can help to better understand on real-time basis, internal energy consumption and demand, which may provide information required to control cost. Such information can assist investors in negotiating energy supply contracts that are more appropriate for their unique consumption and demand patterns (Scheneider Electric Company, 2019). Energy planners face challenges in energy planning because of challenges like unequal distribution of natural resource, limited financial resources and other considerations. Factors considered in energy evaluation include economic, institutional, technological development, energy security, environmental protection, and prevailing state of the energy market (Wanga et al., 2020). For energy systems to be sustainable, the consumption should bear in mind the limits of resource supply and the environmental and social impact (Jefferson, 2000). Maximum advantage should be taken of immense resource supply for renewables like geothermal, solar, hydro and wind (Jonathan, 2001).
Although renewable energy sources have significant advantages, their consumption is also associated with challenges like low conversion efficiency, unsteady supply, low conversion efficiency, and general variability and unpredictability in supply. These weaknesses can be addressed by technological advances and application of computer hardware and software which can enhance optimization and hence create stability and reliability in renewable energy supply and use (Bishoge et al., 2019). Since energy production and consumption accounts for over two thirds of the total greenhouse gas emissions and over 80% of Carbon dioxide emissions, countries that seek to meet the long-term climate objectives of the Paris Agreement must develop measures and strategies to mitigate emissions from power plants and other energy related activities. Countries should individually and collectively tackle their challenges through right and effective energy policy measures (Dufour, 2018). Countries may adopt different transition routes due to relative differences in endowment and competitiveness of renewable and nonrenewable energy resources.
Strategies and measures that can be adopted by countries to exploit their renewable energy resources include on site power generation which involves generating power at the location where it is used like photovoltaic panels installed on buildings, farm-based biogas plants, use of geothermal heat pumps located next to the building, and combined heat and power or cogeneration as well as energy saving and efficiency measures. Another strategy to use renewable and low carbon energy is the use of interconnectors whose role is to physically link different grids or countries for more interconnection needed to ensure the countries can import electricity from low carbon producers like France mainly from nuclear and Sweden and Ethiopia with huge hydro potential as a strategy for de-carbonization of their grid electricity (Dutton, 2019). The purchase of green power through Renewable Energy Certificates (RECS) also known as green tags, green energy certificates, also called tradable renewable certificates is another important strategy in promoting electricity generation from renewable sources (Wanga et al., 2020).
7.3.1 Renewable and Non-Renewable Energy Options in the Transition
Through sustainable energy, the dependence on fossil fuel sources is reduced while increasing the use of renewable sources of energy thus reducing greenhouse gases. Renewable energy technologies may be divided into three generations. The first generation commenced in the nineteenth century and relied on hydropower biomass and geothermal energy. The second generation started in the 1980s and consisted of consists of tidal, wind power, wave power, and solar energy. The third stage or generation is still under development today and is based on gasification, bio-refinery, and ocean thermal power (Hollaway and Bai, 2013). As the global fossil fuel reserves and nuclear diminish, the world has an urgent need to increase the use of renewable energy resources and diversify other available resources and efficiency options. Currently renewable energy power generation has focused on solar photovoltaic (PV), hydro, and wind energy resources with limited use of geothermal and biomass. This is despite the abundance of these energy resources, underlined, for instance, by the importance of sugar-mill power generation (CS-UNIDO, 2008).
There is a global energy transition back to renewable energy, after a century of fossil fuel dominance. Solar energy, wind, bioenergy and geothermal among others will play a leading role in the current transition (Moriarty and Honnery, 2019). The transition will however require creativity and enhanced innovation in form of technology and institutional reforms (Pegels et al., 2018). There are various options for future use of renewable energy.
1) Electricity from Intermittent wind, solar, and wave energy.
2) Dispatchable electricity from hydropower, i.e., major, mini, micro, and others based on resource availability.
3) Energy in form of thermal dispatchable power from solar, geothermal, and biomass.
4) Direct use of thermal energy from bioenergy and low-temperature geothermal energy for heating and cooling applications.
5) Biochemical conversion like biomass to biogas and fermentation to produce gaseous and liquid forms energy (Moriarty and Honnery, 2019).
7.3.1.1 Hydropower
Hydroelectric plants convert energy in moving water to electricity. Conventional hydropower plants have a reservoir developed behind a dam to supply water to the hydraulic turbine for generation of a highly flexible, dispatchable electricity supply. Hydropower can be combined with wind, solar and other sources to supply reliable steady and affordable grid electricity. Hydropower can also be exploited from, run-of-the-river resources which have less environmental impact but with overreliance on steady supply of rain water whose supply is unsteady and unpredictable. Apart from power generation, reservoirs can control floods, supply water, and power from stored water even during drought (Wikipedia, 2021). Hydroelectric electric power plants are useful for grid electricity sustainability particularly during peak hours where plants that generate flexible and cheaper electricity are on high demand (Kolagar et al., 2020).
In 2017, whereas fossil fuels supplied 16,947 TWh or 63% of the total global electricity generation, 4,222 TWh or 16% came from hydropower (BP, 2021) while in 2020, hydropower contributed 16% of global electricity generation as fossil fuels supplied 61.3% of global electricity (International Energy Agency, 2019; BP, 2021). Hydropower is environmental-friendly and releases much less greenhouse gases (GHG) compared to fossil fuel sources like oil, natural gas, coal, and diesel. Hydropower also provides energy security as it decreases reliance on fossil fuels, besides other benefits of developing dams like irrigation, supply of water for industrial and domestic use, flood control and employment opportunities (Solarin et al., 2021).
Hydropower has very low emission which vary with the size of the reservoir. Decomposing organic matter release methane and carbon dioxide while deforestation affects the local hydrology and promotes desertification besides displacing many people from their settlements (Wikipedia, 2021).
7.3.1.2 Solar Energy
Solar energy is cheap because the cost of solar energy is usually negligible, beyond the initial cost outlay. The operational costs of solar are also significantly lower than the conventional power plants. Solar is an important source of energy security since it is locally available. Energy security which is guaranteed by solar energy makes a country less susceptible to external interruptions or events which may influence supply or cost. Socially and economically, solar power generation creates employment opportunities, for example in the year 2018, the solar photovoltaic industry supported more than over 3.6 million jobs globally (Solarin et al., 2021). The main challenge facing solar energy is variability and intermittence in supply and relatively low electricity conversion efficiency.
7.3.1.3 Geothermal Energy
Geothermal energy is produced by drilling deep into the Earth’s crust for harnessing to generate electricity or thermal energy. Feasible geothermal resources are available where the thermal gradient is above 30°C/km, permeable rock structure, natural or artificial water replenishment, and an impervious cap rock. Geothermal contributes less than 1% of global electricity generation even though we have significant potential such that it can meet the entire energy needs of humanity at current rates of consumption (Barasa Kabeyi, 2019a; Kabeyi, 2020c).
As a renewable energy resources, geothermal energy is constantly replenished from neighboring hotter regions and the radioactive decay of naturally occurring isotopes deep in the Earth’s crust. The greenhouse gas emissions from geothermal-based electricity are less than 5% of total emissions from coal-based electricity generation (Kabeyi and Olanrewaju, 2022). The risks associated with geothermal energy exploitation include the risk of inducing earthquakes, water and soil pollution from brine, and releases toxic emissions like hydrogen sulphide and greenhouse gas emissions like carbon dioxide (Kabeyi and Olanrewaju, 2021f). The main challenge facing geothermal electricity generation is long project development period, high upfront risks and huge project costs for conventional technologies which also have low electricity conversion efficiency. The adoption of wellhead generators as a project development option can reduce the period and risks involved in development of geothermal powerplants (Kabeyi and Oludolapo, 2020a; Kabeyi et al., 2020; Kabeyi and Olanrewaju, 2021a).
7.3.1.4 Wind Energy and Power
Wind has been used by man for a very long period to drive windmills, pumps, sailing ships and mechanical energy for industrial processes. Wind turbine generators are used to generate electric power and provided about 6% of global electricity in 2019 (Enerdata, 2021). Wind generated electricity is competitive with nuclear and natural gas and is cheaper than electricity from coal Other than installing onshore, wind turbogenerators can be installed offshore where wind is stronger but will cost more in construction and maintenance (Dreyer, 2021).
Wind generators have environmental impact in form of visual impact on the landscape. collisions between turbine blades with birds and bats is common while noise and flickering lights can cause annoyance and constrain human settlement near the installations (Wang and Wang, 2015). Advantages of wind power is low construction energy and the plants have low water requirements but need more land and the turbine blade materials are not fully recyclable (Huang et al., 2017).
7.3.1.5 Bioenergy
Bioenergy is energy that comes from biomass which is organic material that comes from animals and plants. Biomass produce heat and electricity on combustion and can also be converted into biofuels like biodiesel, ethanol, methanol, etc. for use in combustion engines (Ayompe et al., 2021). Biomass or bioenergy resources include solid and liquid waste, industrial and domestic wastewater, forest resource waste, agricultural waste, and livestock waste (Kabeyi, 2020a). All countries around the word have bioenergy in one form or another. This makes biomass an important energy or electricity source that guarantees energy security with limited environmental harm (Kolagar et al., 2020).
The feedstocks used to include how they are grown, harvested, and processed determines the climate impact of biomass sources of energy. As an example, burning wood fuel produces carbon dioxide which can be offset by photosynthesis in fast growing energy trees and well-managed forest cover since trees absorb carbon dioxide as they grow. The negative impact of bioenergy crops is that they displace natural ecosystems, cause soil degradation, and also they consume water resources and synthetic fertilizers which have some carbon value. About a 1/3 of wood used globally is unsustainably harvested and consumed. Additionally the harvesting and processing of bioenergy feedstocks requires energy for harvest, drying, and transportation which adds to its carbon footprint as greenhouse gases are emitted, although in significantly less quantities than fossil fuels (Correa, 2019).
7.3.1.6 Hydrogen Energy
Hydrogen produces electricity with zero emissions at the point of usage. However, the overall lifecycle emissions of hydrogen are determined by the production process used in its production. Currently, hydrogen is mainly produced from fossil fuel sources (Chant, 2021). The main method of hydrogen production is by steam methane reforming where hydrogen is made by chemical reaction between steam and methane. About 6.6–6.9 tons of CO2 are emitted by of this process to produce one ton of hydrogen (Bonheure et al., 2021). Carbon capture process can then be used to remove a large percentage of the CO2 produced making the process cleaner. Although the overall carbon footprint of hydrogen as a fuel is yet to be fully established, it remains a cleaner fuel than natural gas, biogas or methane (Griffiths et al., 2021).
In another method of hydrogen production, electrolysis by use of electricity can be used to split water molecules to hydrogen fuel. However, the process is more expensive compared to methane reforming and sustainability requires that the electricity is from green sources which for now is still a challenge in many parts of the world. Hydrogen fuel can be produced during surplus of intermittent renewable electricity and stored for use during peak and when the variable renewable disappears (Palys and Daoutidis, 2020). Hydrogen can also be processed into synthetic fuels sources of energy like ammonia and methanol (Blank and Molly, 2020).
Research and development are encouraged to develop hydrogen electrolyzers for use in large-scale production of hydrogen for power generation competitively. Hydrogen produces intense heat suitable for industrial production of steel, cement, glass, and chemicals. Therefore, hydrogen can act as a clean fuel in the steal steelmaking, can act as a clean energy carrier and simultaneously as low-carbon catalyst in place of coke (Blank and Molly, 2020). The main limitation of hydrogen as an energy carrier is high storage and distribution costs since it is explosive and occupies a large volume. The gas also embrittles pipes hence it needs special handling facilities which have to be developed (Griffiths et al., 2021).
7.3.1.7 Natural Gas
It is important to note that several cities globally have reached or are reaching epidemically poor-quality atmospheric air quality requirements and urgently need to reduce pollution from engines emissions. There is need for an immediate remedy to pollution caused by urban diesel vehicles. Natural gas as a fuel is also cheaper than refined petroleum products. In most markets around the world [Group of Experts on Pollution & Energy (GRPE), 2001].
Besides direct combustion, natural gas has more hydrogen atoms making it an excellent raw material or feedstock for hydrogen production by using high-temperature steam also called, steam methane reforming, and by partial oxidation. Steam reforming and partial oxidation both produce “synthesis gas,” that produces more hydrogen when reacted with water. These processes make natural gas a pathway to the hydrogen future. This is mainly because several aspects of hydrogen and natural gas distribution and storage, fueling, station siting, and training of technicians and drivers are similar. Hence knowledge from handling of natural gas will make the transition to a hydrogen fuel smoother. Hydrogen and natural gas can also be blended with hydrogen to make transportation fuel. These can take the form of 20% by volume hydrogen also called Hythane or 30% by volume hydrogen called HCNG (Werpy et al., 2010).
7.3.1.8 Marine Energy
This energy resource has one of the smallest contributions to the global energy market. Marine energy consists of tidal power, which is a maturing technology and wave power, which is steal under early stages of research and development as well as ocean thermal energy. A typical example of these resources is the Two Tidal Barrage Systems in France and in South Korea which account for 90% of world marine energy production. The environmental impact of small and single marine energy devices is little except for larger devices are less well known (Wikipedia, 2021). Tidal power is a form of green energy resource, as it emits near zero greenhouse gases and occupies less space per unit power. The largest tidal powerplant project globally is the Sihwa Lake Tidal Power Station in South Korea, which has installed capacity of 254 MW established in 2011, as a development to a 12.5 km-long seawall that was built in the year 1994 to for flood control and support of farming (Husseini, 2021). Tidal power has the benefit of predictability as the gravitational forces of celestial bodies won’t be going anywhere soon. The equipment is also about four times longer lasting than wind equipment and the plant has high power density with limited surface area requirements (Wikipedia, 2021; Husseini, 2021).
The Ocean Thermal Energy Conversion (OTEC) is a marine technology that utilizes the solar energy absorbed by sea water to generate electricity. It takes advantage of the thermal difference between cooler deep waters and warmer shallow or surface ocean waters to operate a heat engine to generate work. The main challenge is that the temperature difference is small which poses a challenge to the technical and economic sustainability (World Energy Council, 2013). This calls for more research and development for ocean thermal energy.
7.3.1.9 Nuclear Power
Nuclear power plants have been in operation since 1950s as sources of low carbon base load electricity. Nuclear power plants operate in over 30 countries generating over 10% of global electric power (Rhodes, 2021; World Nuclear Association, 2021). As of the year 2019, over 25% of the low carbon electricity was generated from nuclear making it the 2nd largest source after hydropower. The main environmental benefit of nuclear power is that the lifecycle greenhouse gas emissions including that of mining and processing of uranium are close to emissions from renewable energy sources (Bruckner and Fulton, 2014). Additionally, its land requirement per unit power output is or less than that of the major renewables, and it doesn’t pollute the local environment. Although uranium ore is a non-renewable resource, its available quantities can provide power for hundreds to thousands of years to come. Therefore, increased use of nuclear power will reduce emissions and related environmental impact (Bruckner and Fulton, 2014; Dunai and De Clercq, 2019).
The main sustainability challenges of nuclear power generation which should be addressed are challenges of nuclear waste handling and disposal, weapon proliferation, and catastrophic accidents (Gill et al., 2014). There is need to manage radioactive nuclear waste over long time scales before final disposal (Gill et al., 2014), while low energy fissile material created is a feasible raw material for low energy nuclear applications including military use in weapon development (Gill et al., 2014). Statistically, nuclear energy has caused fewer accidents and pollution related deaths than fossil fuel (Ritchie, 2021). The challenge to the investment and hence development of nuclear power is politically motivated and is mainly over fear for weapons proliferation (Gill et al., 2014).
The main challenges facing nuclear power development is long developing period, and high cost of capacity and powerplant development, hence the need to reduce cost and delivery time (Timmer, 2021). Technology options include Fast breeder reactors which are capable of recycling nuclear waste hence reduce disposal challenges by reducing waste but they are yet to be commercially deployed (Joint Research Centre, 2021). In terms of energy security, countries with no Uranium can resort to the use of thorium rather than uranium (Gill et al., 2014). Another sustainable option is use of Small modular reactors which are smaller, cheaper and faster to deploy. While their modularization allows flexibility in capacity development for countries with low electricity demand. Modular units also generate less waste and have less risks of explosion (Bruckner and Fulton, 2014; Gill et al., 2014).
7.3.1.10 Coal and Petroleum Resources
Coal is a leading source of energy for grid electricity while countries like Poland rely on coal for significant energy applications and source of revenue to the economy. Poland for example is the largest producer of hard coal and the second largest producer of lignite in the European union followed by Germany. Therefore, coal is an important economic product for several countries, besides being a secure energy resource. Due to abundant supply of coal as a secure energy source, coal accounted for 76.8% of its electricity in Poland. For Germany, which is another leading producer of coal, it accounted for 35.6% of electricity generation, followed by the United Kingdom which produced 5.1% of its electricity from coal. The entire European union produced 18.9% of its electricity from coal. Poland produced 68.3 million tons of hard coal in 2019, which was an 85 reduction over the 2018 production. Of all coal produced, 60.1% was consumed by the energy sector, 24.6 was used by the industry and construction while households used 15.2%. The coal industry has a positive social value worth noting. For example, in 2019, coal mining employed 94% of people in coal mining or 78,500 people in Upper Silesian Basin, of Poland while the remaining 6% worked at the Bogdanka mine in the Lublin province of Poland with monthly salaries in 2020 being twice the average salary in Poland (Krzywda et al., 2021).
Although coal is a fossil fuel with huge environmental impact, it will continue to play an important role in power generation with application of clean coal technologies. Gradual substitution is recommended as coal rich economies continue transition to renewable and low carbon energy resources and diverse their economies to substitute declining coal revenue.
7.3.1.11 Shift to Natural Gas From Coal and Petroleum Fuels
The switching from coal and diesel to natural gas in power generation has significant benefits in terms of sustainability. Natural gas generates about half the emissions of coal when used in power generation and about two-thirds the emissions of coal when applied in heat production. Additionally, natural gas produces less air pollution than coal, but the challenge is to limit gas leakages since methane is highly potent as a greenhouse gas (Information Adminitstr, 2021).
The shift from coal to natural gas reduces emissions as a short term measure but does not provide a long term path to the desirable net-zero emissions. Therefore these transition has the danger of causing carbon lock-in and stranded assets which must be written off or they are allowed to continue operating against the emission targets (Gürsan and de Gooyert, 2021; Plumer, 2021).
7.3.2 Benefits and Challenges of Renewable Energy
There is a relationship between total greenhouse gas emissions and consumption of renewable energy resources. For example between 1990 and 2012, greenhouse gas emissions (GHG) in European Environmental Agency (EEA) with 33 member countries reduced by 14% while GHG emissions per capita declined by 22% over the same period (European Environment Agen, 2016) due to increased use of renewable energy, a scenario that was also witnessed in the United States between 2006 and 2014 (Owusu and Asumadu-Sarkodie, 2016). This brings both environmental and socioeconomic benefits with less environmental impact through substituting polluting fossils with renewable and low carbon energy sources and creation of jobs and social capital in the society [United States Environmental Protection Agency (EPA), 2017].
Renewable energy sources and technologies are competitive energy options particularly for remote areas but encounter barriers to their diffusion like lack of access to capital for the for low and medium-income population. There is need for financing for renewable energy technologies, such as solar PV, micro-hydro, wind power for water pumping and electric power generation, bio digesters and biogas installation costs and improved woodstoves production and installation (Kabeyi and Oludolapo, 2021b). Other barriers include:
1) Lack of competitiveness since most of these renewable energy power plants have higher investment and energy costs as compared to conventional or nonrenewable options particularly in terms of initial cost of the project.
2) Uncoordinated planning, policy and legal and financial instruments has ensured that renewable energy renewable energy projects need support against nonrenewable sources in form carbon tax, tax incentives and subsidies and regulations support to enhance their diffusion and interconnection to electricity grid and general adoption.
3) There is Lack of information, supportive infrastructure, and maintenance, for example in some cases, there is lack of technologies and infrastructure or capabilities to develop renewable energy projects or markets (Kabeyi and Oludolapo, 2021b).
7.4 Decentralized and Distributed Power Generation
Power transmission and distribution networks where initially conceived and designed to distribute electricity from central power stations to consumers kilometers away. This approach is no longer valid because of the increasing presence of distributed generation systems that are mainly based on variable renewable energy sources and a growing number of variable load users like, plug-in electric cars connected to the grid and lower voltage points (Zarco-Soto et al., 2021). There has been a shift to the use of small and distributed powerplants as the world gradually adopts the use of renewable sources of energy for grid electricity generation which requires a bi-directional flow of power through transformers (Colangelo et al., 2021). The centralized model of power generation and distribution has dominated the electricity sector in many countries while distributed energy resources (DER), are slowly being accommodated and remain dominant in remote and isolated areas. The decentralization of electricity generation gathered momentum when economies of scale stopped being significant factor owing to innovation and technology development. The main motivation was the use of diesel engines and gas turbines and the adoption of smart grids. Traditionally, decentralised systems consisted of dispatchable resources; but we have increasing use of non-dispatchable PV as a recent development. The development of decentralised generation is such that today, the global annual distributed generation capacity additions have surpassed the centralized electricity systems (Mitrova and Melnikov, 2019).
For maximum use of DER technologies to be achieved, there is need to develop a systemic architecture and put in place policy measures in the power sector to balance the interests of new players with the existing centralized model players. However, an optimal combination of centralized generation and DER seems to be the most effective and efficient approach in the energy transition. This implementation requires principles and mechanisms for seamless for the integration of the centralized and decentralized for reliability in operations (Mitrova and Melnikov, 2019). The main system benefit distributed renewable energy sources is that it leads to increase in nodal voltages. The growing use of variable loads on distribution networks like electric cars puts significant pressure on the need for nodal voltage control through a flexible and resilient electricity grid that goes beyond mere decentralization of grid power generation (Zarco-Soto et al., 2021). With the new decentralized generation technologies, economies of scale have been turned upside down with improved viability of small energy systems. Increased use of information technologies has generated new opportunities for energy e infrastructure management in a less hierarchical and flexible manner. This combined with consumer demands for control over their energy systems has created energy communities (ECs) on the agenda hence higher opportunities for transition towards more sustainable energy through improvement in efficiency, less emissions, reduced costs, and hence a sustainable energy future.
7.5 Transition From Traditional to Smart Grids
About 10% of the total grid power is lost to transmission and distribution of which 40% is lost at the distribution side alone in the traditional grid (Rathor and Saxena, 2020b). The solutions to the energy or electricity related pollution and losses are significant reduction in use of fossil fuels, increased use of renewable energy sources like photovoltaics and wind, use of fuel cells and integration of energy and battery storage systems and use of plug-in electric cars (Conejo et al., 2010; Azzouz et al., 2015; Rathor and Saxena, 2020b). The world has witnessed significant advances in technology which includes development of better electricity carriers, decentralization of generation and increasing contribution of variable renewable sources energy to grid electricity and electrification of transport which introduces unpredictable load on the grid. All these developments call for development and use of smart grids. The smart grid uses computer programs and hardware to manage electricity generation and distribution resources and hence can help in optimization of the energy mix of both renewable and non-renewable energy for sustainable power generation and supply through smart grids. Smart grids can facilitate increased absorption of variable renewable sources of energy like wind and solar and thus displace fossil fuels from the grid. They enhance decentralization of generation provide the infrastructure and capacity needed to facilitate increased use of renewable energy help increase participation of all stakeholders in the operation and power delivery between sources and users in a two-way manner. This will greatly contribute to the dream of a sustainable grid electricity system.
The current global trend is to develop digital technologies for the entire economies and hence digitalization of the power systems is part of the global technological transition. Digitalization of electricity sector brings opportunities particularly the increased absorption of variable renewable like wind and solar which makes control very difficult (Schiffer and Trüby, 2018). Lack of system flexibility reduces its resilience and hence capacity to absorb the variable sources of energy hence the need to adopt power system digitalization as a transition strategy (Mitrova and Melnikov, 2019). Digitalization of operation and controls in power-generating and supply assets will increase efficiency, security of power systems as well as resilience more efficient, the electric grid more secure and resilient, thus reducing emissions and the threat of global climate change.
7.6 Research and Development of Sustainable Energy Technologies
It is not practical to achieve significant contribution of the intermittent renewable energy sources like solar, wind and hydro in power production without a combination of flexible dispatch able power, a reliable electricity transmission system, energy storage facilities, the smart grids, and demand side electricity management. To realize maximum renewable energy contribution, it is necessary to develop effective business models and policies, modern innovative energy technologies, system operational flexibility, and efficiency through continues research and development (Gielen et al., 2019). The technology and approaches to enable sustainable electricity include the development of smart grids and replacement of the traditional electricity grid, decentralization of grid electricity generation and use which will lead to better absorption of renewable energy and adequate participation of consumers in demand management, electrification transport, development of energy storage technologies, demand side management strategies through measures like time dependent electricity tariff system and smart solutions like smart meters for consumers within a smart grid. Most of the critical technologies for the energy transition like smart grids and cheaper energy storage technologies are still under research and development and require funding and other forms of research support to mature.
7.6.1 Carbon Capture and Storage
Carbon capture and storage technology is an effective technology to absorb emissions, either by natural processes in bio crops or industrial scale plant processes. This process is known as bioenergy with carbon capture and storage (BECCS) can lead to net CO2 removal from the atmosphere while carbon dioxide and other emissions from powerplants and other process plants can be absorbed and stored or buried. Unfortunately, BECCS may lead to net positive emissions based on how the biomass is produced, harvested, transported, and processed. Biomass material is grown, harvested, and transported (Ayompe et al., 2021).
7.6.2 Energy Storage
Energy storage is an important solution to intermittent renewable energy supply and hence a critical aspect of a sustainable energy system. Various storage methods for use include pumped-storage hydroelectricity (Hunt, 2020), and batteries especially lithium-ion batteries (Blanco and Faaij, 2018). The challenge with batteries is that they have limited storage periods which calls for more research into storage technology for both utility-scale batteries and low energy density batteries makes them impractical for the very large energy storage needed to balance inter-seasonal variations in energy production. Other than pumped hydro storage and power-to-gas like hydrogen needs further research (Koohi-Fayegh and Rosen, 2020).
7.6.3 Fuel Cells in Sustainable Electricity
Fuel cells are electrochemical systems that convert chemical energy of a fuel like hydrogen and oxidizing agent often oxygen to electricity through a pair of redox reactions. Unlike batteries they continuously require supply of fuel and oxygen to sustain the process. In batteries the chemical energy comes from metals and their ions or oxides that are already in the battery except for flow batteries (Saikia et al., 2018). The fuel cell will produce power continuously on condition that there is a steady supply of the fuel and oxygen and are more efficient than combustion systems. The heat generated in the process of power generation can be put into thermal application thus further increasing the efficiency through cogeneration. They are able to reduce building facility energy service cost by 20–40% (Fuel Cells, 2000).
Stationary fuel cells are often used for commercial, industrial, and residential primary and backup electricity generation and can be very useful for power supply in remote locations, like spacecraft, isolated weather stations, large parks, telecommunication communication centers, off grid stations including research stations, remote military applications, and standby power supply sources for power stations (Saikia et al., 2018). The main advantage of fuel cell systems like hydrogen fuel cells is that they are compact, light, and have limited moving parts to attend to hence low maintenance and can realize 99.9999% reliability (Fuel Cells, 2000).
7.6.4 Electrification Transport Industrial Processes and Rural Areas
It is possible to reduce emissions faster in electricity systems than many other systems because as in 2019, about 37% of global electricity generation came from low-carbon sources, i.e., renewables and nuclear energy with the rest coming from coal and other fossil fuel sources (Bruckner et al., 2014b). Phasing out coal fired power plants is among the easiest and fastest ways to controlling greenhouse gas emissions and in its place increase the share of renewable and low carbon electricity generation (Ritchie, 2021). A leading limitation in provision of universal access to electric power is rural electrification where both off-grid and on-grid systems based on renewable energy can power villages who predominantly rely on wood fuel, kerosene and diesel generators for heat lighting and power (Rosen, 2009). Wider access to reliable electricity would lead to less use of kerosene lighting particularly for the developing countries (United Nations Develpment programme, 2016).
Electrification of transport is significant because transport sector accounts for about 14% of global greenhouse gas emissions which can be reduced by use of electric cars, buses, and electric trains that consume green electricity (Bigazzi, 2019). The various climate change scenarios predict extensive electrification and substitution of direct fossil fuel combustion with clean electricity for heating building and for transport (Miller et al., 2013). A deliberate climate policy should see double increase in energy share from electric power by 2015 from the 20% of the year 2020 (Bruckner et al., 2014b; IRENA, 2018; United Nations Develpment programme, 2016).
7.6.5 Energy Efficiency and Conservation
Energy efficiency and conservation have potential to provide a means to achieve global emissions and climate change targets set by the Paris agreement and other national and international protocols. Energy efficiency and conservation measures will lead to reduction in greenhouse gas emissions, reduce fuel consumption, reduce the load and strain on the electricity grid, and reduce cost of both generation and cost of electricity consumed (Clark and Clark, 2019). The main challenge facing adoption of efficiency and conservation measures is lack of appropriate technology and high capital requirements which creates financial management risk and undetermined return-on-investment and hence undetermined payback periods which significantly limit their adoption (Clark and Clark, 2019). Significant amount of energy in many forms including heat, electricity and even primary resource id lost or wasted through transmission, heat loss, and application of inefficient technology. This is a huge cost to consumers who must pay for the lost energy as more energy is consumed to carter for the losses resulting in more pollution for every extra unit consumed due to losses (Department of Energy, 2021). Therefore, putting in place various energy efficiency measures is one of the easiest and cost-effective means of combatting climate change, limit emissions and related pollution, reduce energy costs and improve the competitiveness of businesses (Department of Energy, 2021). In a sustainable energy scenario by the International Energy Agency, energy efficiency is expected to deliver more than 40% of targeted reduction in energy-related greenhouse gas emissions between 2020 and 2040 as a strategy to put the world on track to achieve international emissions and related climate change targets (Clark and Clark, 2019; International Energy Agency, 2021c).
7.6.6 Microgrids
Microgrids are becoming an important solution in the sustainable energy transition by improving reliability and resilience of electric power grids, necessary to manage distributed clean energy resources like wind and solar photovoltaic (PV) as well as generation to reduce emissions as well as supply power to off grid locations (Wilson, 2021). A microgrid can be defined as a group of interconnected loads and distributed energy resources existing in a well-defined electrical boundary that is operated as single controllable unit with respect to the grid. It can connect and disconnect from the main grid to enable it to operate in both grid and off grid mode (Valencia et al., 2021; Wilson, 2021).
A grid basically consists of a power source, consumers, wires to connect them, and a system to control generation and supply. On the other hand, a microgrid is a grid but a smaller version of it. A microgrid can cover one or several buildings and can be used to supply power to critical infrastructure, remote or small communities or business and industrial installation (Valencia et al., 2021). Microgrids enable supply of clean and efficient energy, with more resiliency, and improves the operation and stability of the local electric power systems (Wilson, 2021). Microgrids constitute a very important segment of the energy transition representing a shift from centralized power towards more localized and distributed generation solutions. The main benefit of microgrids is ability to isolate from the central or larger grid hence a feasible and attractive option for cities, rural areas, industrial parks, suburbs, and remote installations. With use of microgrids, it possible to balance generation from variable renewable power sources such as solar, wind, and hydro and conventional sources like gas-fueled combustion turbines, coal, and diesel powerplants (Wilson, 2021).
7.7 Earth Radiation Management and the Solar Radiation Management
The current global warming mitigation efforts and future commitments are inadequate to achieve the Paris Agreement temperature targets. Although the various techniques show the physical potential to contribute to limiting climate change, many are still in the early stages of development. For this reason, the climate geoengineering techniques provide alternative or additional measures to contribute to meeting the Paris Agreement temperature goals (Lawrence et al., 2018). The best way so far to reduce global warming is reduction in the anthropogenic emissions of greenhouse gases. However, the global economy with its ever-growing population cannot do without energy most of which is generated from fossil fuels. Replacing this energy with carbon dioxide-free renewable energies, and energy efficiency is a long term, costly, and difficult venture. By use of geoengineering schemes which use solar radiation management technologies to modify terrestrial albedo or reflect incoming shortwave solar radiation back to space provide an alternative solution to the challenge of global warming (Lenton and Vaughan, 2009). We also have power-generating systems that have potential to transfer heat from the Earth surface to the upper layers of the troposphere and then to the space (Ming et al., 2014). The main objective of Geoengineering is to stabilize global climate, reduce global warming and reduce anthropogenic climate changes by two main strategies namely, shortwave (0.3–3 μm) reflection where sunlight is reflected back and then secondly the use of carbon dioxide removal technologies (Ming et al., 2014).
The solar radiation management geoengineering systems work by the parasol effect, i.e., reducing solar incoming radiation, but the carbon dioxide still traps the reduced heat both day and night over the entire world. The effect of solar radiation management would be only experienced during the day particularly at the equator (Ming et al., 2014).
8 Results and Discussion
A good concept of sustainable development should facilitate social equity, prevent environmental degradation, and maintain a sound economic base. There is need for sustainable preservation of natural capital for sustained economic production and equity in intergeneration equity in resource exploitation. Fulfillment of basic health and participatory democracy is crucial in energy resource planning and exploitation to ensure sustainability. Sustainable transition requires governments to use policy instruments and an effective institutional mechanism to deliver working solutions to a sustainable energy future. The three main dimensions of sustainable development are economic, social, and environmental sustainability. However, sustainability in energy resource use and electricity systems has extra dimensions of technical, and political or institutional sustainability.
Humanity has increased the concentration of carbon dioxide in our atmosphere, amplifying Earth’s natural greenhouse effect. This is still ongoing and hence a continuous threat to the global environment. The global average amount of carbon dioxide hit a new record high in 2020: of 412.5 ppm. The annual rate of global increase in atmospheric CO2 over the last 60 years is about 100 times faster than previous natural increases like those that occurred at the end of the last ice age about 11,000–17,000 years ago. As a result, the ocean has absorbed enough carbon dioxide to lower its PH by 0.1 units, from 8.21 to 8.10 since the beginning of the industrial revolution which represents about 30% increase in acidity of the ocean. This is dangerous to aquatic ecological balance due to the biological effect of ocean acidification which interferes with marine life’s ability to extract calcium from the water to build their shells and skeletons.
The energy sector is the largest contributor of global carbon dioxide emissions and second largest contributor of non-carbon dioxide greenhouse gas emissions globally. With electricity being the leading source of greenhouse gases, which are the cause of global warming, any effort to minimize greenhouse gases should address emissions from power generation. Sustainable grid electricity requires facilitating technologies and infrastructure like smart grids, decentralization of generation. A mixture of options is necessary to lower the unit cost and carbon intensity of energy systems to achieve a truly sustainable energy with low carbon world. Energy related GHG emissions are a result from conversion and delivery sectors like extraction/refining, power generation and direct transport of energy carriers in pipelines, cables, ships, tracks an end use sectors and industries like transport, buildings and construction, manufacturing, agriculture, forestry, households, and waste and hence cannot be blamed entirely on one sector or process alone.
The Intergovernmental panel on climate change (IPCC) predicted that a greenhouse gas emission (GHG) will lead to global temperature increase of between 1.1 and 6.4°C by the end of the 21st Century. The world would experience about 62% increase in CO2 emissions between 2011 and 2050 if energy demand and use of fossil fuels to meet the demand does not change. To maintain ecologically sustainability, organizations should consume natural resources whose consumption rates are lower than the rate of natural replenishment or reproduction. Where substitutes exist, the rate of consumption should be lower than the rate of substitute development. The greenhouse gas emissions should be reduced by between 50 and 80% by the year 2050 if the world must avoid the looming consequences of global warming. The composition of atmospheric carbon dioxide (CO2) has been rising as summarized in Table 2 above.
Table 2 shows that between 1750 and 2020, the atmospheric concentration of carbon dioxide has increased from 277 to 412.5 ppm representing an increase of 48.92%.
For a stable atmosphere, the global average temperature increase should be maintained between 1.5 and 2°C above the preindustrial level which translates to atmospheric carbon dioxide concentration of 400–450 Energy resources are sources of various past and current political and social conflicts and therefore it is through proper energy resource management that many social, political, and economic conflicts can be avoided or resolved globally. Countries can use renewable energy sources like solar, wind and even hydro to enhance their national energy security because these resources do not need international trade to secure them and hence cushions countries against energy instigated insecurity.
Today, industry and building sectors are the main users of electricity accounting for over 90% of global electricity demand. Moving forward, the main drivers of electricity demand growth are motors in industry which may account for over 30% of the total growth to 2040, industrial and domestic space cooling will account for 17% while large electrical appliances are projected to account for 10% growth and electric vehicles are projected to account for 10% growth in electricity demand. Further growth in electricity demand of about 2% is projected to come from provision of electricity access to 530 million first time users of electricity. The sustainable development scenario, projects that electric vehicles will become a leading source of electricity demand moving to the future towards the year 2040. Therefore, a sustainable electricity transition should prepare for wider use of variable renewables, low carbon nuclear power, electrification of transportation and industrial processes, better and efficient conversion, and efficient energy use technologies and electrification of thermal application of energy.
Table 3, it is noted that fossil fuels in the form of coal, natural gas and oil contributed 61.3% of the global electricity generation in the year 2020. Low carbon nuclear and renewable energy sources which should be the basis of the sustainable electricity transition accounted for about 38% of global electricity with undefined sources accounting for about 0.7% of the global electricity generation in the year 2020.
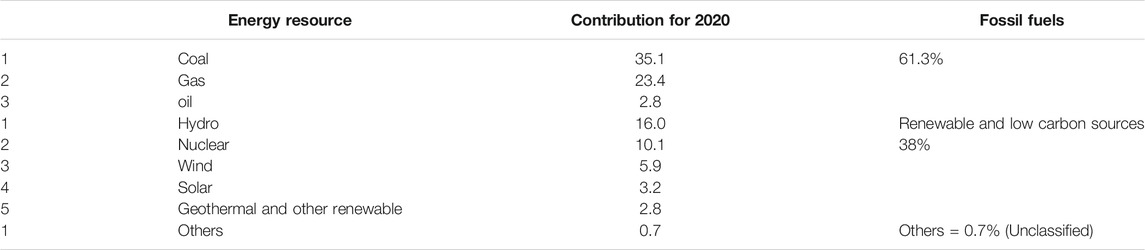
TABLE 3. The global electricity generation can be summarized in Table 3 below.
A sustainable electricity transition calls for eventual transition of the 61.3% of the global electricity production to low carbon and renewable energy sources. In the short and to some extend middle term, natural gas can substitute oil and coal although with the risk of delaying the zero emissions transition and creating transition related carbon lock-in and stranded assets by developing natural gas infrastructure. Since countries with huge coal and oil reserves may find it unsustainable to make immediate transition, increase in the share of natural gas, and investment in efficient technologies like cogeneration and clean coal technology can reduce the carbon footprint.
Sustainable energy transition should address the five major dimensions. They are technical, social, economic, environmental, and institutional dimensions. These dimensions of energy sustainability are summarized in Table 4 below.
From Table 4, it is noted that there are five dimensions of energy sustainability namely environmental, economic, social, technical, and institutional/political sustainability which can be used to design and analyses energy sustainability measures.
Various energy resources have been identified as potential solution to the global transition. They are discussed in summary form in Table 5 below.
From Table 5, it is noted that both renewable and nonrenewable sources of energy have a role to play in the energy transition. The nonrenewable sources like coal and oil are abundant in several countries and therefore are readily available and offer energy security. The steady release of energy by fossil fuel sources is important for reliability and stability and hence quality which are sustainable energy requirements. However, their high carbon footprint, finite supply, price, and supply interruptions and resource related conflicts make fossil fuels unreliable and unsustainable source of energy and hence the need for gradual substitution with renewable and low carbon sources of energy. The use of highly efficient conversion technology and clean coal as well as carbon capture and sequestration can greatly reduce the carbon footprint of fossil fuel sources. For natural gas, controlling leakages along the entire supply chain is paramount due to the high global warming potential of methane which is the main constituent of natural gas.
Solar and wind offer the greatest potential but suffer from unpredictable and unreliable supply challenge hence the need for advanced energy storage facilities. For grid electricity, the unreliability and unpredictable supply nature of wind and solar is a danger to energy security and the grid stability for the traditional grid. However, the use of smart grids with ability to absorb small scale producers and variable supply will greatly increase the absorption of wind and solar energy as well as small hydro sources and decentralized generation.
Various strategies or methods that can be adopted to reduce carbon emissions and hence realize the global energy/electricity transition are summarized in Table 6 below.
From Table 6 above it is noted that various technological strategies can be adopted separately or in combination to reduce emissions and hence achieve a sustainable energy transition. They include electrification of transport which requires efficient and cost-effective energy storage systems and a resilient electricity grid to handle multiple variable consumer and supplier load. This creates the need to transition from the traditional grid to a more resilient smart grid. Electrification of the rural populations that are not electrified also provide an opportunity for increased use of renewable sources of electricity especially through decentralized generation systems. The use of decentralized generation will widen feasible grid connected generation which mostly comes from renewable energy sources. Energy efficiency by consumers will reduce demand and wastage hence avoid emissions while efficiency in generations leads to less fuel consumption and hence emissions and reduced environmental impact.
Although fossil fuels are major contributors of greenhouse gas emissions leading to global warming, most of them are price competitive, have steady release of energy hence the power plants operate at high load and capacity factors with high reliability of electricity supply, and thus provide grid stability. Therefore, their consumption now and soon is key to a stable and reliable electricity grid which is a key requirement of energy sustainability. The consumption of fossil fuels should be reduced but they will continue to supplement the intermittent and unpredictable but abundant wind and solar energy which have the key to the future sustainable energy supply in a highly optimized electricity generation and supply system where technology will play a key role in planning and decision support. The carbon footprint of fossil fuels used in power generation should minimized by adoption of efficient conversion technologies like cogeneration and trigeneration to minimize which reduce fuel consumption and maximize generation from limited energy resources. Other technologies include dual fuel diesel power, use f biofuel substitutes fuel blending with biofuels and use of combined cycle powerplants. Therefore, a sustainable transition for now should involve increase in energy efficiency to reduce the total demand and wastage of fossil fuels in an optimized system in which the grid absorbs all variable renewables. The smart grid and advanced storage technologies will play a significant role in the sustainable electricity transition.
From this study, the technical options to the energy transition can be grouped into three categories. They are substitution technologies, carbon capture and sequestration and climate geoengineering techniques.
9 Conclusion
Sustainable development cannot be achieved without sustainable energy which facilitates sustainable electricity generation. Whereas sustainable development can be analyzed within three dimensions representing the three pillar of sustainable development namely economic, social and environmental dimensions, sustainable energy is best analyzed with five dimensions namely social, economic, environmental, institutional or political, and technical, The sustainable transition strategies typically consist of three major technological changes namely, energy savings on the demand side, generation efficiency at production level and fossil fuel substitution by various renewable energy sources and low carbon nuclear. For the transition to remain technically and economically feasible and beneficial, policy initiatives are necessary to steer the global electricity transition towards a sustainable energy and electricity system. Whereas renewable sources energy holds the key for sustainable energy transition, large-scale renewable energy adoption should include measures to improve efficiency of existing nonrenewable sources which still have an important cost reduction and stabilization role. A resilient grid with advanced energy storage for storage and absorption of variable renewables should also be part of the transition strategies. The world has so far witnessed three typical energy transitions. The first transition involved replacement of wood with coal as the main energy source. In the second transition, oil replaced coal as the dominant energy resource. In the third transition, there is global commitment to replace fossil fuels with renewable energy. Through the cumulative effect of the Stockholm, Rio, and Johannesburg conferences, sustainable energy development (SED) was identified as a key requirement for sustainable development and so energy was linked energy to the environmental dimension in the Stockholm conference, economy in the Rio conference and society in the Johannesburg conference. Sustainable development is expected to bring economic and progress in an environmentally benign manner free from wastage, pollution, destructive emissions, and social strive in a facilitating political environment. Sustainable development has got three main dimensions of economic, social, and environmental aspects while sustainable energy has got two additional dimensions of technical and institutional or political environment.
The greatest sustainability challenge facing humanity today is the greenhouse gas emissions and the global climate change with fossil fuels led by coal, natural gas, and oil contributing 61.3% of global electricity generation in the year 2020. Through sustainable energy, the dependence on fossil fuel sources is reduced while increasing the use of renewable sources of energy thus reducing greenhouse gases. Renewable energy technologies may be divided into three generations. The first generation commenced in the nineteenth century and relied on hydropower biomass and geothermal energy. The second generation started in the 1980s and consisted of consists of tidal, wind power, wave power, and solar energy. The third stage or generation is still under development today and is based on gasification, bio-refinery, and ocean thermal power. Electricity is the most dominant form of energy deriving its supply from both renewable and nonrenewable sources. The optimum operation of the grid electricity system is influenced by many dynamic variables which must be determined and controlled, managed, or accommodated to deliver reliable, affordable, and clean electricity. Sustainable grid electricity transformation needs competitive and cost-effective financing mechanisms to accelerate the transition, needs reliable energy supplies, and application of effective business and operation modelling tools that can deliver sustainable electricity which also needs new technology and data capability to analyze, and optimize results on real-time basis and in medium- and long-term planning.
Technology has very important role to play in the transition to a low carbon electricity grid and economy. Technology options to facilitate the energy transition will include rapid digitalization of the energy sector which will enhance its flexibility and resilience to absorb variable renewable sources of energy particularly wind and solar. Important technology include transition from the traditional grid based on centralized generation to smart grids which support decentralized generation and ability to absorb the fluctuating renewable energy sources like solar and wind and fluctuating demand like electric cars while guaranteeing a stable and reliable electricity supply. Decentralization and enhanced use of variable renewables will further be enhanced by use of microgrid technology. With use of microgrids, it possible to balance generation from variable renewable power sources such as solar, wind, and hydro and conventional sources like gas-fueled combustion turbines, coal, and diesel powerplants. Since greenhouse gas emissions come from sectors like extraction/refining, power generation and direct transport, agriculture, industry, and homes, electrification of all industries and homes with power coming from renewable sources of energy will greatly succeed in cutting down global emissions. The broad strategies adopted for sustainable transition include liberalisation and restructuring of electricity and other energy markets which is made attractive by ever growing energy demand globally. Key polices adopted should aim at making electricity markets work better. More research and development into efficient, environmentally friendly, and competitive technology is needed to facilitate innovation and diffusion of sustainable energy technologies. To successful implement these policies calls for reduction unit cost of electricity and affordable cost of appropriate energy carriers and services, plus regulations to increase efficiency and reduce energy related environmental for greater public benefits.
The implementation of effective sustainable energy technologies to minimize carbon emissions will require the use of renewable and low carbon sources of energy and adoption of three main strategies namely conventional mitigation, negative emissions technologies which capture and sequester carbon emissions and finally technologies which alter the global atmospheric radiative energy budget to stabilize and reduce global average temperature. Besides low emissions, a sustainable electricity grid system should be stable and supply reliable, affordable, and socially acceptable electricity.
Although there is no consensus on quantitative factors and their magnitude there is agreement on the need for supportive policies, regulations, programmes, and international commitments. Other measures are the development and expansion of financial sector, and improvement on the performance and quality of energy sector institutions. This study concludes that both renewable and non-renewable sources of energy have a leading role to play in the short and long-term energy transition. They include energy sources like solar, wind, hydro, hydrogen, bioresources, marine energy, nuclear, natural gas substitute of other fossil fuels and application of clean and efficient technologies for existing fossil fuel and non-renewable systems. Important strategies include electrification of thermal applications and household, and technologies like smart grids and energy storage for variable renewables and carbon capture and sequestration, cogeneration, and energy efficiency measures to limit consumption and wastage of energy resources. Waste to energy and particularly in form of electricity will minimize solid waste load and reduce environmental pollution like water and soil contamination. With the grid connecting different energy sources and infrastructure, decision support systems and optimization models will play a key role in realizing cost effective and environmentally friendly and reliable electricity generation and supply. Technology measures to control global warming can be classified into three broad categories of carbon capture and sequestration, emission mitigation strategies and technologies that alter the radiative properties of incoming and outgoing solar radiations.
10 Recommendations for Future Research
This study lays the foundation for further research into technical and non-technical measures to ensure a sustainable transition to a low carbon electricity grid from different sources, both renewable and nonrenewable and will form a firm foundation for effective policy formulation and implementation necessary to drive the energy transition globally. Further research into practical details of the technologies and measures is recommended to guide the actual implementation of the transition measures and strategies like smart grids, decentralized generation, energy storage, decentralized generation, and computerization and optimization of electricity generation, transmission, and distribution resources to facilitate a sustainable energy future. The study further recommends identification and development of transition specific energy and electricity models to aid in planning and execution of sustainable energy and electricity production, supply and consumption by end users, utilities, and prosumers.
Author Contributions
MK developed the draft manuscript with OO reviewing and making further input including editorial.
Conflict of Interest
The authors declare that the research was conducted in the absence of any commercial or financial relationships that could be construed as a potential conflict of interest.
Publisher’s Note
All claims expressed in this article are solely those of the authors and do not necessarily represent those of their affiliated organizations, or those of the publisher, the editors and the reviewers. Any product that may be evaluated in this article, or claim that may be made by its manufacturer, is not guaranteed or endorsed by the publisher.
Acknowledgments
The authors sincerely acknowledge the contribution of all individuals, reviewers, and editors for their contribution towards the production of this manuscript.
Abbreviations
CHP, Combined heat and power; CO2-eq, Carbon dioxide equivalent; CSR, Corporate social responsibility; EJ, Exajoules; GHG, Greenhouse gases; KenGen, Kenya Electricity Generating Company PLC; KWS, Kenya Wildlife Services; PPM, Parts per million; NEMA, National Environment Management Authority.
References
Akella, A. K., Saini, R., and Sharma, M. (2009). Social, Economical and Environmental Impacts of Renewable Energy Systems. Renew. Energ. 34 (2), 390–396. doi:10.1016/j.renene.2008.05.002
Andress, D., Nguyen, T. D., and Das, S. (2011). Reducing GHG Emissions in the United States' Transportation Sector. Energ. Sustainable Development 15, 117–136. doi:10.1016/j.esd.2011.03.002
Austvik, O. G. (2016). The Energy Union and Security-Of-Gas Supply. Energy Policy 96, 372–382. doi:10.1016/j.enpol.2016.06.013
Ayompe, L. M., Schaafsma, M., and Egoh, B. N. (2021). Towards Sustainable palm Oil Production: The Positive and Negative Impacts on Ecosystem Services and Human Wellbeing. J. Clean. Prod. 278, 123914. doi:10.1016/j.jclepro.2020.123914
Azad, S. (2015). Koreans in the Persian Gulf: Policies and International Relations. New York: USA Routledge.
Azzouz, M. A., Shaaban, M. F., and El‐Saadany, E. F. (2015). Real‐time Optimal Voltage Regulation for Distribution Networks Incorporating High Penetration of PEVs. IEEE Trans. Power Syst. 30 (6), 3234–3245. doi:10.1109/tpwrs.2014.2385834
Barasa Kabeyi, M. J., and Olanrewaju, O. A. (2022). Geothermal Wellhead Technology Power Plants in Grid Electricity Generation: A Review. Energ. Strategy Rev. 39, 100735. doi:10.1016/j.esr.2021.100735
Barasa Kabeyi, M. J. (2019). Geothermal Electricity Generation, Challenges, Opportunities and Recommendations. Ijasre 5 (8), 53–95. doi:10.31695/IJASRE.2019.33408
Barasa Kabeyi, M. J. (2019). Project and Program Evaluation Process, Consultancy and Terms of Reference with Challenges, Opportunities and Recommendations. Ijsrp 9 (12), p9622–194. doi:10.29322/IJSRP.9.12.2019.p9622
Bayram, I. S., and Ustun, T. S. (2017). A Survey on behind the Meter Energy Management Systems in Smart Grid. Renew. Sustainable Energ. Rev. 72, 1208–1232. doi:10.1016/j.rser.2016.10.034
Bazmi, A. A., and Zahedi, G. (2011). Sustainable Energy Systems: Role of Optimization Modeling Techniques in Power Generation and Supply—A Review. Renew. Sustainable Energ. Rev. 15, 3480–3500. doi:10.1016/j.rser.2011.05.003
Beaudin, M., and Zareipour, H. (2015). Home Energy Management Systems: A Review of Modelling and Complexity. Renew. Sustainable Energ. Rev. 45, 318–335. doi:10.1016/j.rser.2015.01.046
Berga, L. (2016). The Role of Hydropower in Climate Change Mitigation and Adaptation: A Review. Engineering 2, 313–318. doi:10.1016/J.ENG.2016.03.004
Bhowmik, C., Bhowmik, S., and Ray, A. (2020). Optimal green Energy Source Selection: An eclectic Decision. Energ. Environ. 31 (5), 842–859. doi:10.1177/0958305X19882392
Bigazzi, A. (2019). Comparison of Marginal and Average Emission Factors for Passenger Transportation Modes. Appl. Energ. 242, 1460–1466. doi:10.1016/j.apenergy.2019.03.172
Birol, F. (2021). Energy Is at the Heart of the Sustainable Development Agenda to 2030. Paris, France: International Energy Agency. Available at: https://www.iea.org/commentaries/energy-is-at-the-heart-of-the-sustainable-development-agenda-to-2030 (accessed, 2021).
Bishoge, O. K., Zhang, L., and Mushi, W. G. (2019). The Potential Renewable Energy for Sustainable Development in Tanzania: A Review. Clean. Tech. 1 (1), 70–88. doi:10.3390/cleantechnol1010006
Blanco, H., and Faaij, A. (2018). A Review at the Role of Storage in Energy Systems with a Focus on Power to Gas and Long-Term Storage. Renew. Sustainable Energ. Rev. 81, 1049–1086. doi:10.1016/j.rser.2017.07.062
Blank, T. K., and Molly, P. (2020). “Hydrogen’s Decarbonization Impact for Industry,” in Near-term Challenges and Long-Term Potential (New York, NY, January: Rocky Mountain Institute). [Online]. Available: https://rmi.org/wp-content/uploads/2020/01/hydrogen_insight_brief.pdf.
Boden, T. A., Marland, G., and Andres, R. J. (2017). Global, Regional, and National Fossil-Fuel CO2 Emissions. Oak Ridge, Tenn., U.S.A.: U.S. Department of Energy. [Online]. Available: https://cdiac.ess-dive.lbl.gov/trends/emis/overview_2014.html.
Bonheure, M., Vandewalle, L. A., Marin, G. B., and Geem, K. M. V. (2021). Dream or Reality? Electrification of the Chemical Process Industries. Chem. Eng. Prog. 117 (7), 37–42. [Online]. Available: https://www.aiche.org/resources/publications/cep/2021/march/dream-or-reality-electrification-chemical-process-industries.
Bp, (2021). Statistical Review of World Energy. [Online]. Available: https://www.bp.com/en/global/corporate/energy-economics/statistical-review-of-world-energy.html.
Broman, G. I., and Robèrt, K.-H. (2017). A Framework for Strategic Sustainable Development. J. Clean. Prod. 140, 17–31. doi:10.1016/j.jclepro.2015.10.121
Bruckner, T., Fulton, L., Hertwich, E., McKinnon, A., Perczyk, D., Roy, J., et al. (2014). Annex III: Technology-specific Cost and Performance Parameters. [Online]. Available: https://www.ipcc.ch/site/assets/uploads/2018/02/ipcc_wg3_ar5_annex-iii.pdf.
Bruckner, T. (2014). “Energy Systems,” in Climate Change 2014: Mitigation of Climate Change. Contribution of Working Group III to the Fifth Assessment Report of the Intergovernmental Panel on Climate Change. Editors K. Parikh, and J. Skea (Cambridge, United Kingdom and New York, NY, USA: Cambridge University Press), 77. ch. 7.
Bruckner, T., et al. (2014). “Energy Systems,” in Climate Change 2014: Mitigation of Climate Change. Contribution of Working Group III to the Fifth Assessment Report of the Intergovernmental Panel on Climate Change. Editors K. Parikh, and J. Skea (United Kingdom and New York, NY, USA: Cambridge University Press). ch. 7.
Burger, A., Lünenbürger, B., and Osiek, D. (2012). “Sustainable Electricity for the Future,” in Costs and Benefits of Transformation to 100% Renewable Energy (Dessau-Roßlau, Germany: German Federal Environment Agency). [Online]. Available: https://www.umweltbundesamt.de/sites/default/files/medien/378/publikationen/sustainable_electricity_for_the_future_-neu.pdf.
Butt, T. E., Giddings, R. D., and Jones, K. G. (2012). Environmental Sustainability and Climate Change Mitigation-CCS Technology, Better Having it Than Not Having it at All!. Environ. Prog. Sustainable Energ. 31 (4), 642–649. doi:10.1002/ep.10590
Chant, News. (2021). Hydrogen Is One Answer to Climate Change. Getting it Is the Hard Part. Chant: News Chant. Available at: https://us.newschant.com/business/hydrogen-is-one-answer-to-climate-change-getting-it-is-the-hard-part/ (accessed, 2021).
Cheng, J. Y. S. (2009). The 1979 Oil Shock and the “Flying Geese Model” in East AsiaThe 1979 “Oil Shock:” Legacy, Lessons, and Lasting Reverberations. Washington, DC: The Middle East Institute. [Online]. Available: https://www.mei.edu/sites/default/files/publications/2009.09.The%201979%20Oil%20Shock%20-%20Legacy%20Lessons%20and%20Lasting%20Reverberations.pdf.
Cherp, A., and Jewell, J. (2014). The Concept of Energy Security: Beyond the Four as. Energy Policy 75, 415–421. doi:10.1016/j.enpol.2014.09.005
Clark, W. W. (2019). “Chapter 11 - Conclusion: The Global Green Paradigm Shift,” in Climate Preservation in Urban Communities Case Studies. Editor W. W. Clark. Ed. (Butterworth-Heinemann), 439–451. doi:10.1016/b978-0-12-815920-0.00011-3
Colangelo, G., Spirto, G., Milanese, M., and de Risi, A. (2021). Progresses in Analytical Design of Distribution Grids and Energy Storage. Energies 14 (14), 4270. doi:10.3390/en14144270
Colla, M., Ioannou, A., and Falcone, G. (2020). Critical Review of Competitiveness Indicators for Energy Projects. Renew. Sustainable Energ. Rev. 125, 109794. doi:10.1016/j.rser.2020.109794
Conejo, A. J., Morales, J. M., and Baringo, L. (2010). Real-time Demand Response Model. IEEE Trans. Smart Grid 1 (3), 236–242. doi:10.1109/tsg.2010.2078843
Correa, D. F. (2019). Towards the Implementation of Sustainable Biofuel Production Systems. Renew. Sustainable Energ. Rev. 107, 250–263. doi:10.1016/j.rser.2019.03.005
Cs-Unido, (2008). “Renewable Energy Technologies: Wind, mini-hydro,thermal, Photovoltaic Biomass and Waste,” in Survey of Appropriate Technologies and Perspectives for Latin America and the Caribbean (Trieste, Italy: International Centre for Science and High Technology). [Online]. Available: https://www.academia.edu/5958430/Renewable_Energy_Technologies_wind_mini_hydro.
Darkwah, W. K., Odum, B., Addae, M., and Koomson, D. (2018). Greenhouse Gas Effect: Greenhouse Gases and Their Impact on Global Warming. J. Scientific Res. Rep. 17 (6), 1–9. doi:10.9734/JSRR/2017/39630
Davidsdottir, B. (2012). “7.10 - Sustainable Energy Development: The Role of Geothermal Power,” in Comprehensive Renewable Energy. Editor A. Sayigh (Oxford: Elsevier), 273–297. doi:10.1016/b978-0-08-087872-0.00715-0
De, A. (2021). Institutional Structures for Making Electricity Sustainable. India, 31–38. [Online]. Available: https://www.brookings.edu/wp-content/uploads/2015/01/renewable-energy_ch4.pdf.
del Río, P., and Janeiro, L. (2016). Overcapacity as a Barrier to Renewable Energy Deployment: The Spanish Case. J. Energ. 2016, 1–10. doi:10.1155/2016/8510527
Demirel, Y. (2014). “Thermoeconomics,” in Nonequilibrium Thermodynamics. Editor Y. Demirel. Third Edition (Amsterdam: Elsevier), 265–302. doi:10.1016/b978-0-444-59557-7.00005-9
UD Department of Energy (2021). Energy Efficiency. Washington, DC: United States Department of Energy. Available at: https://www.energy.gov/eere/energy-efficiency (accessed, 2021).
Dobranskyte-Niskota, A., Perujo, A., Jesinghaus, J., and Jensen, P. (2009). Indicators to Assess Sustainability ofTransport Activities. Ispra (VA) -ITALY: European Commission, Institute for Environment and Sustainability, 88. [Online]. Available: https://publications.jrc.ec.europa.eu/repository/handle/JRC54971.
Dreyer, J. (2021). The Benefits and Drawbacks of Offshore Wind Farms. Available at: http://large.stanford.edu/courses/2017/ph240/dreyer2/ (accessed, 2021).
Dufour, F. (2018). The Costs and Implications of Our Demand for Energy: A Comparative and Comprehensive Analysis of the Available Energy Resources. [Online]. Available: https://www.academia.edu/36768579/The_Costs_and_Implications_of_Our_Demand_for_Energy_A_Comparative_and_Comprehensive_Analysis_of_the_Available_Energy_Resources?auto=download&email_work_card=view-paper.
Dunai, M., and De Clercq, G. (2019). Nuclear Energy Too Slow, Too Expensive to Save Climate: Report. Reuters. Available at: https://www.reuters.com/article/us-energy-nuclearpower-idUSKBN1W909J.
Dutton, J. (2019). JSTOR. [Online]. Available: https://www.jstor.org/tc/accept?origin=%2Fstable%2Fpdf%2Fresrep21758.pdf&is_image=False.UK-EU Electricity Interconnection: THE UK'S Low Carbon Future and Regional Cooperation after Brext
Dyllick, T., and Hockerts, K. (2002). Beyond the Business Case for Corporate Sustainability. Business Strategy Environ. 11 (2), 130–141. doi:10.1002/bse.323
Ebrahimi, M., and Rahmani, D. (2019). A Five-Dimensional Approach to Sustainability for Prioritizing Energy Production Systems Using a Revised GRA Method: A Case Study. Renew. Energ. 135, 345–354. doi:10.1016/j.renene.2018.12.008
Emas, J. (2015). The Concept of Sustainable Development: Definition and Defining Principles. Florida: Florida International University. [Online]. Available: https://sustainabledevelopment.un.org/content/documents/5839GSDR%202015_SD_concept_definiton_rev.pdf.
Enerdata (2021). Share of Wind and Solar in Electricity Production. Enerdata. Available at: https://yearbook.enerdata.net/renewables/wind-solar-share-electricity-production.html (accessed, 2021).
U.S. Energy Information Administration (2020). Country Analysis Executive Summary: Japan. United States Energy Information Administartion. [Online]. Available: https://www.eia.gov/beta/international/analysis_includes/countries_long/Japan/japan.pdf.
European Environment Agency (2016). Energy and Environment in the European Union. Copenhagen, Denmark. [Online]. Available: No 8/2006.
Fawzy, S., Osman, A. I., Doran, J., and Rooney, D. W. (2020). Strategies for Mitigation of Climate Change: a Review. Environ. Chem. Lett. 18 (6), 2069–2094. doi:10.1007/s10311-020-01059-w
Fouquet, D. (2013). Policy Instruments for Renewable Energy – from a European Perspective. Renew. Energ. 49, 15–18. doi:10.1016/j.renene.2012.01.075
Fuel Cells (2000). Fuel Oil Basics. Rochester, New York: Fuel Cells. Available at: https://web.archive.org/web/20070928225430/http://www.fuelcells.org/basics/benefits.html (accessed, 2021).
Gamil, M. M., Sugimura, M., Nakadomari, A., Senjyu, T., Howlader, H. O. R., Takahashi, H., et al. (2020). Optimal Sizing of a Real Remote Japanese Microgrid with Sea Water Electrolysis Plant under Time-Based Demand Response Programs. Energies 13 (14), 3666. doi:10.3390/en13143666
Gibbes, C., Hopkins, A. L., Díaz, A. I., and Jimenez-Osornio, J. (2020). Defining and Measuring Sustainability: a Systematic Review of Studies in Rural Latin America and the Caribbean. Environ. Development Sustainability 22 (1), 447–468. doi:10.1007/s10668-018-0209-9
Gielen, D., Boshell, F., Saygin, D., Bazilian, M. D., Wagner, N., and Gorini, R. (2019). The Role of Renewable Energy in the Global Energy Transformation. Energ. Strategy Rev. 24, 38–50. doi:10.1016/j.esr.2019.01.006
Gill, M., Livens, F., and Peakman, A. (2014). “Chapter 9 - Nuclear Fission,” in Future Energy. Editor T. M. Letcher. Second Edition (Boston: Elsevier), 181–198. doi:10.1016/b978-0-08-099424-6.00009-0
Griffiths, S., Sovacool, B. K., Kim, J., Bazilian, M., and Uratani, J. M. (2021). Industrial Decarbonization via Hydrogen: A Critical and Systematic Review of Developments, Socio-Technical Systems and Policy Options. Energ. Res. Soc. Sci. 80, 102208. doi:10.1016/j.erss.2021.102208
Group of Experts on Pollution & Energy (Grpe), (2001). Dual Fuel (Natural Gas/diesel) Engines: Operation, Applications & Contribution. Geneva, Swizerland: The European Natural Gas Vehicle Association. Available at: https://unece.org/DAM/trans/doc/2001/wp29grpe/TRANS-WP29-GRPE-42-inf18.pdf (accessed.
Gürsan, C., and de Gooyert, V. (2021). The Systemic Impact of a Transition Fuel: Does Natural Gas Help or Hinder the Energy Transition? Renew. Sustainable Energ. Rev. 138, 110552. doi:10.1016/j.rser.2020.110552
Hasna, A. M. (2007). “Dimensions of Sustainability,” in presented at the 3rd International SIIV Conference, Bari, Italy, September 22-24.1.
Hildingsson, R., and Johansson, B. (2016). Governing Low-Carbon Energy Transitions in Sustainable Ways: Potential Synergies and Conflicts between Climate and Environmental Policy Objectives. Energy Policy 88, 245–252. doi:10.1016/j.enpol.2015.10.029
Hollaway, L. C. (2013). “19 - Sustainable Energy Production: Key Material Requirements,” in Advanced Fibre-Reinforced Polymer (FRP) Composites for Structural Applications. Editor J. Bai (Sawston, United Kingdom: Woodhead Publishing), 705–736. doi:10.1533/9780857098641.4.705
Huang, Y.-F., Gan, X.-J., and Chiueh, P.-T. (2017). Life Cycle Assessment and Net Energy Analysis of Offshore Wind Power Systems. Renew. Energ. 102, 98–106. doi:10.1016/j.renene.2016.10.050
Hulme, M., Jenkins, G. J., Lu, X., Turnpenny, J. R., Mitchell, T. D., Jones, R. G., et al. (2002). “Climate Change Scenarios for the United Kingdom,” in The UKCIP 2002 Scientific Report (Norwich, UK: Tyndall Centre for Climate Change Research). [Online]. Available: https://www.academia.edu/2986789/Climate_Change_Scenarios_for_the_United_Kingdom_The_UKCIP02_Scientific_Report.
Hunt, J. D. (2020). Global Resource Potential of Seasonal Pumped Hydropower Storage for Energy and Water Storage. Nat. Commun. 11 (1), 947. doi:10.1038/s41467-020-14555-y
Husseini, T. (2021). Riding the Renewable Wave: Tidal Energy Advantages and Disadvantages. New York, USA: Power Tech.. Available at: https://www.power-technology.com/features/tidal-energy-advantages-and-disadvantages/ (Accessed, 2021).
Iddrisu, I., and Bhattacharyya, S. C. (2015). Sustainable Energy Development Index: A Multi-Dimensional Indicator for Measuring Sustainable Energy Development. Renew. Sustainable Energ. Rev. 50, 513–530. doi:10.1016/j.rser.2015.05.032
UD Information Adminitstration (2021). Natural Gas and the Environment. Available at: https://www.eia.gov/energyexplained/natural-gas/natural-gas-and-the-environment.php (accessed, 2021).
Inglesi-Lotz, R. (2021). Energy Transitions: The Role of Institutions and Market Structures. Available at: https://theconversation.com/energy-transitions-the-role-of-institutions-and-market-structures-168156 (accessed, 2021).
Intergovernmental Panel on Climate Change(IPCC) (2007). “Climate Change 2007:The Physical Science Basis,” in Contribution of Working Group I to the Fourth Assessment Report of the Intergovernmental Panel on Climate Change " Intergovernmental Panel on Climate Change 2007 (Cambridge, United Kingdom and New York, NY: Cambridge University). [Online]. Available: https://www.ipcc.ch/site/assets/uploads/2020/02/ar4-wg1-sum_vol_en.pdf.
International Energy Agency (2018). CO2 Emissions from Fuel Combustion 2018. Soregraph, France: International Energy Agency.
International Energy Agency (2019). World Energy Outlook 2019. Paris, France: International Energy Agency. [Online]. Available: https://www.iea.org/reports/world-energy-outlook-2019/electricity.
International Energy Agency (2021a). Global Energy Review 2021. Paris, France: International Energy Agency. Available at: https://www.iea.org/reports/global-energy-review-2021/co2-emissions (accessed, 2021).
International Energy Agency (2021b). Key World Energy Statistics 2021. Paris: International Energy Agency. [Online]. Available: https://www.iea.org/reports/key-world-energy-statistics-2021.
International Energy Agency (2021c). Energy Efficiency the First Fuel of a Sustainable Global Energy System. Paris, France: International Energy Agency. Available at: https://www.iea.org/topics/energy-efficiency (accessed, 2021).
İpek Tunç, G., Türüt-Aşık, S., and Akbostancı, E. (2007). CO2 Emissions vs. CO2 Responsibility: An Input-Output Approach for the Turkish Economy. Energy Policy 35, 855–868. doi:10.1016/j.enpol.2006.02.012
IRENA (2018). “A Roadmap to 2050,” in Global Energy Transformation (Abu Dhabi: International Renewable Energy Agency). [Online]. Available: https://www.irena.org/-/media/Files/IRENA/Agency/Publication/2018/Apr/IRENA_Report_GET_2018.pdf.
Jacobs, P., Gardner, J., and Munro, D. (1987). “Sustainable and Equitable Development: An Emerging Paradigm,” in Conservation with Equitable Development: Strategies for Sustainable Development. Editors P. Jacobs, and D. A. Munro (Cambridge: International Union for onservation of Nature and Natural Resource).
Jansen, J. C., and Seebregts, A. J. (2010). Long-term Energy Services Security: What Is it and How Can it Be Measured and Valued? Energy Policy 38 (4), 1654–1664. doi:10.1016/j.enpol.2009.02.047
Jaramillo-Nieves, L., and Del Río, P. (2010). Contribution of Renewable Energy Sources to the Sustainable Development of Islands: An Overview of the Literature and a Research Agenda. Sustainability 2 (3), 783–811. doi:10.3390/su2030783
Jefferson, M. (2000). “Energy Policies for Sustainable Development,” in World Energy Assessment: Energy and the Challenge of Sustainability (London, UK: United Nations Development Program), 416–452. ch. 12.
Jin, Z., Wang, J., Yang, M., and Tang, Z. (2022). The Effects of Participation in Global Value Chains on Energy Intensity: Evidence from International Industry-Level Decomposition. Energ. Strategy Rev. 39, 100780. doi:10.1016/j.esr.2021.100780
Joint Research Centre (JRC) (2021). Technical Assessment of Nuclear Energy with Respect to the ‘do No Significant Harm’ Criteria of Regulation (EU) 2020/852 (‘Taxonomy Regulation’). Netherlands: European Atomic Energy Community. [Online]. Available: https://ec.europa.eu/info/sites/default/files/business_economy_euro/banking_and_finance/documents/210329-jrc-report-nuclear-energy-assessment_en.pdf.
Jonathan, M. H. (2001). Basic Principles of Sustainable Development " Global Developement and Environment Institute. Medford, MA, USA: Tufts University. [Online]. Available: https://notendur.hi.is/bdavids/UAU101/Readings/Harris_2000_Sustainable_development.pdf.
Kabeyi, M. J. B., and Olanrewaju, O. A. (2021a). Central versus Wellhead Power Plants in Geothermal Grid Electricity Generation. Energ Sustain. Soc. 11 (1), 7. doi:10.1186/s13705-021-00283-8
Kabeyi, M. J. B., and Olanrewaju, O. A. (2021b). “Development of a Cereal Grain Drying System Using Internal Combustion Engine Waste Heat,” in presented at the 11th Annual International Conference on Industrial Engineering and Operations Management, Singapore, March 7-11, 2021. [Online]. Available: http://www.ieomsociety.org/singapore2021/papers/188.pdf.
Kabeyi, M. J. B., and Olanrewaju, O. A. (2021c). “Fuel from Plastic Wastes for Sustainable Energy Transition,” in presented at the 11th Annual International Conference on Industrial Engineering and Operations Management, Singapore, March 7-11, 2021. [Online]. Available: http://www.ieomsociety.org/singapore2021/papers/199.pdf.
Kabeyi, M. J. B., and Olanrewaju, O. A. (2021d). “Dual Cycle Cogeneration Plant for an Operating Diesel Powerplant,” in presented at the 11th Annual International Conference on Industrial Engineering and Operations Management, Singapore, March 7-11, 2021. [Online]. Available: http://www.ieomsociety.org/singapore2021/papers/200.pdf.
Kabeyi, M. J. B., and Olanrewaju, O. A. (2021e). “Performance Analysis of a Sugarcane Bagasse Cogeneration Power Plant in Grid Electricity Generation,” in presented at the 11th Annual International Conference on Industrial Engineering and Operations Management, Singapore, March 7-11, 2021. [Online]. Available: http://www.ieomsociety.org/singapore2021/papers/201.pdf.
Kabeyi, M. J. B., and Olanrewaju, A. O. (2021f). Geothermal Wellhead Technology Power Plants in Grid Electricity Generation: a Review. Energ. Strategy Rev. 27, 1. doi:10.1016/j.esr.2021.100735,
Kabeyi, M. j. B., and Olanrewaju, O. A. (2022). Geothermal Wellhead Technology Power Plants in Grid Electricity Generation: A Review. Energ. Strategy Rev. 39, 100735. doi:10.1016/j.esr.2021.100735
Kabeyi, M. J. B., and Oludolapo, A. O. (2020c). “Viability of Wellhead Power Plants as Substitutes of Permanent Power Plants,” in presented at the 2nd African International Conference on Industrial Engineering and Operations Management, December 7-10 (Harare, Zimbabwe, 77. [Online]. Available: http://www.ieomsociety.org/harare2020/papers/77.pdf.
Kabeyi, M. J. B., and Oludolapo, A. O. (2020d). “Performance Analysis of an Open Cycle Gas Turbine Power Plant in Grid Electricity Generation,” in presented at the 2020 IEEE International Conference on Industrial Engineering and Engineering Management (IEEM), Singapore, 2020. [Online]. Available: https://ieeexplore.ieee.org/stamp/stamp.jsp?tp=&arnumber=9309840.
Kabeyi, M. J. B., and Oludolapo, A. O. (2020e). “Managing Sustainability in Electricity Generation,” in presented at the 2020 IEEE International Conference on Industrial Engineering and Engineering Management, Singapore, 14-17 December 2020. doi:10.1109/ieem45057.2020.9309994
Kabeyi, M. J. B., and Oludolapo, A. O. (2021g). “Preliminary Design of a Bagasse Based Firm Power Plant for a Sugar Factory,” in presented at the South African Universities Power Engineering Conference (SAUPEC), Nortn West University, South Africa, 27-28 January 2021, 104. [Online]. Available: https://ieeexplore.ieee.org/abstract/document/9377242.
Kabeyi, M. J. B., and Oludolapo, A. O. (2021h). Central versus Wellhead Power Plants in Geothermal Grid Electricity Generation. Energ. Sustainability Soc. 1, 1. doi:10.1186/s13705-021-00283-8
Kabeyi, M. J. B., and Oludolapo, A. O. (2020a). “Characteristics and Applications of Geothermal Wellhead Powerplants in Electricity Generation,” in SAIIE31 Proceedings, South Africa. Editor H. Teresa (South Africa: South African Institution of Industrial Engineers), 31222–31235. [Online]. Available: https://www.dropbox.com/s/o0sj1l08v8n9sgh/SAIIE31%20Conference%20Proceedings.pdf?dl=.
Kabeyi, M. J. B. (2018). Ethical and Unethical Leadership Issues, Cases, and Dilemmas with Case Studies. Int. J. Appl. Res. 4 (7), 373–379. doi:10.22271/allresearch.2018.v4.i7f.5153
Kabeyi, M. J. B. (2018). Transformational vs Transactional Leadership with Examples. Int. J. Business Management 6 (5), 191–193. [Online]. Available: http://www.internationaljournalcorner.com/index.php/theijbm/article/view/129786/90079.
Kabeyi, M. J. B. (2018). Michael porter’s Five Competitive Forces and Generic Strategies, Market Segmentation Strategy and Case Study of Competition in Global Smartphone Manufacturing Industry. Int. J. Appl. Res. 10 (4), 39–45. doi:10.22271/allresearch.2018.v4.i10a.5275
Kabeyi, M. J. B. (2019). Evolution of Project Management, Monitoring and Evaluation, with Historical Events and Projects that Have Shaped the Development of Project Management as a Profession. Int. J. Sci. Res. (Ijsr) 8 (12), 1. doi:10.21275/ART20202078
Kabeyi, M. J. B. (2019). Geothermal Electricity Generation, Challenges, Opportunities and Recommendations. Int. J. Adv. Scientific Res. Eng. (Ijasre) 5 (8), 53–95. doi:10.31695/IJASRE.2019.33408
Kabeyi, M. J. B. (2019). Organizational Strategic Planning, Implementation and Evaluation with Analysis of Challenges and Benefits for Profit and Nonprofit Organizations. Int. J. Appl. Res. 5 (6), 27–32. doi:10.22271/allresearch.2019.v5.i6a.5870
Kabeyi, M. J. B. (2020). Investigating the Challenges of Bagasse Cogeneration in the Kenyan Sugar Industry. Int. J. Eng. Sci. Res. Technology 9 (5), 7–64. doi:10.5281/zenodo.3828855
Kabeyi, M. J. B. (2020). Corporate Governance in Manufacturing and Management with Analysis of Governance Failures at Enron and Volkswagen Corporations. Am. J. Operations Management Inf. Syst. 4 (4), 109–123. doi:10.11648/j.ajomis.20190404.11
Kabeyi, M. J. B. (2020). Feasibility of Wellhead Technology Power Plants for Electricity Generation. Int. J. Computer Eng. Res. Trends 7 (2), 1–16. doi:10.22362/ijcert/2020/v7/i02/v7i0201
Kabeyi, M. J.B., and Olanrewaju, O. A. (2020b). Managing Sustainability in Electricity Generation " Presented at the 2020. IEEE Int. Conf. Ind. Eng. Eng. Management (Ieem) Singapore 14. doi:10.1109/IEEM45057.2020.9309994
Kanter, R. M. (2021). How to Do Well and Do Good. MIT Sloan Management Review. [Online]. Available: http://sloanreview.mit.edu/the-magazine/2010-fall/52118/how-to-do-well.
Karekezi, S., and Kimani, J. (2002). Status of Power Sector Reform in Africa: Impact on the Poor. Energy Policy 30, 923–945. doi:10.1016/S0301-4215(02)00048-4
Kaygusuz, K. (2012). Energy for Sustainable Development: A Case of Developing Countries. Renew. Sustainable Energ. Rev. 16 (2), 1116–1126. doi:10.1016/j.rser.2011.11.013
Kolagar, M., Hosseini, S. M. H., Felegari, R., and Fattahi, P. (2020). Policy-making for Renewable Energy Sources in Search of Sustainable Development: a Hybrid DEA-FBWM Approach. Environ. Syst. Decisions 40 (4), 485–509. doi:10.1007/s10669-019-09747-x
Koohi-Fayegh, S., and Rosen, M. A. (2020). A Review of Energy Storage Types, Applications and Recent Developments. J. Energ. Storage 27, 101047. doi:10.1016/j.est.2019.101047
Korea Energy Economics Institute (KEEI) (2017). Energy Info Korea " Joo-Heon Park, South Korea. [Online]. Available: http://www.keei.re.kr/keei/download/EnergyInfo2017.pdf.
Krajnc, D., and Glavic, P. (2003). Indicators of Sustainable Production. Clean. Tech. Environ. Pol. 5, 279–288. doi:10.1007/s10098-003-0221-z
Krzywda, J., Krzywda, D., and Androniceanu, A. (2021). Managing the Energy Transition through Discourse. The Case of Poland. Energies 14 (20), 6471. doi:10.3390/en14206471
Kumar, M. (2019). “Social, Economic, and Environmental Impacts of Renewable Energy Resources,” in Wind, Solar, Renewable Energy Hybrid Systems. Editor K. E. Okedu, A. Tahour, and A. G. Aissaou (London, UK: IntertechOpen).
Kuzemko, C., Lockwood, M., Mitchell, C., and Hoggett, R. (2016). Governing for Sustainable Energy System Change: Politics, Contexts and Contingency. Energ. Res. Soc. Sci. 12, 96–105. doi:10.1016/j.erss.2015.12.022
Lamb, W. F., Wiedmann, T., Pongratz, J., Andrew, R., Crippa, M., Olivier, J. G. J., et al. (2021). A Review of Trends and Drivers of Greenhouse Gas Emissions by Sector from 1990 to 2018. Environ. Res. Lett. 16 (7), 073005. doi:10.1088/1748-9326/abee4e
Lawrence, M. G., Schäfer, S., Muri, H., Scott, V., Oschlies, A., Vaughan, N. E., et al. (2018). Evaluating Climate Geoengineering Proposals in the Context of the Paris Agreement Temperature Goals. Nat. Commun. 9 (1), 3734. doi:10.1038/s41467-018-05938-3
Lélé, S. M. (1991). Sustainable Development: A Critical Review. World Development 19 (6), 607–621. doi:10.1016/0305-750X(91)90197-P
Lenhart, S., and Fox, D. (2021). Structural Power in Sustainability Transitions: Case Studies of Energy Storage Integration into Regional Transmission Organization Decision Processes (In English), Frontiers in Climate. Front. Clim. 3 (145), 1. doi:10.3389/fclim.2021.749021
Lenton, T. M., and Vaughan, N. E. (2009). The Radiative Forcing Potential of Different Climate Geoengineering Options. Atmos. Chem. Phys. 9 (15), 5539–5561. doi:10.5194/acp-9-5539-2009
Li, J., Geng, X., and Li, J. (2016). A Comparison of Electricity Generation System Sustainability Among G20 Countries. Sustainability 8 (12), 1276. doi:10.3390/su8121276
Lidsey, R. (2020). “Climate Change: Atmospheric Carbon Dioxide,” in Understanding Climate. Washington, DC: US Government. [Online]. Available: https://www.climate.gov/news-features/understanding-climate/climate-change-atmospheric-carbon-dioxide.
Lindsey, R. (2021). “Climate Change: Atmospheric Carbon Dioxide,” in Understanding Climate (Washington, DCM: US Government). [Online]. Available: https://www.climate.gov/sites/default/files/BAMS_SOTC_2019_co2_paleo_1000px.jpg.
Liu, G. (2014). Development of a General Sustainability Indicator for Renewable Energy Systems: A Review. Renew. Sustainable Energ. Rev. 31, 611–621. doi:10.1016/j.rser.2013.12.038
Lu, Y., Khan, Z. A., Alvarez-Alvarado, M. S., Zhang, Y., Huang, Z., and Imran, M. (2020). A Critical Review of Sustainable Energy Policies for the Promotion of Renewable Energy Sources. Sustainability 12 (12), 5078. doi:10.3390/su12125078
Lu, W.-C. (2017). Electricity Consumption and Economic Growth: Evidence from 17 Taiwanese Industries. Sustainability 9 (1), 50. . doi:10.3390/su9010050
Lund, H. (2010). “Chapter 1 - Introduction,” in Renewable Energy Systems. Editor H. Lund (Boston: Academic Press), 1–12. doi:10.1016/b978-0-12-375028-0.00001-7
Lund, H. (2007). Renewable Energy Strategies for Sustainable Development. Energy 32 (6), 912–919. doi:10.1016/j.energy.2006.10.017
MacLeod, M. G. (2011). “19 - Environmental Sustainability of Egg Production and Processing,” in Improving the Safety and Quality of Eggs and Egg Products. Editors Y. Nys, M. Bain, and F. Van Immerseel (United Kingdom: Woodhead Publishing), 445–462. doi:10.1533/9780857093912.4.445
Marcus, A. A. (1992). Energy Policy. Phoenix, AZ: Sage Press. [Online]. Available: https://www.encyclopedia.com/environment/encyclopedias-almanacs-transcripts-and-maps/energy-policy.
McCurry, J. (2015). Can Japan’s Climate Policy Get Back on Track after Fukushima? London, UK: The Guardian. Available at: https://www.theguardian.com/environment/2015/apr/17/can-japans-climate-policy-get-back-on-track-after-fukushima.
Mensah, J. (2019). Sustainable Development: Meaning, History, Principles, Pillars, and Implications for Human Action: Literature Review. Cogent Soc. Sci. 1 (5), 1–21. doi:10.1080/23311886.2019.1653531
Mihut, M. I., and Daniel, D. L. (2013). First Oil Shock Impact on the Japanese Economy. Proced. Econ. Finance 3 (12), 1042–1048. doi:10.1016/S2212-5671(12)00271-7
Miller, C. A., Iles, A., and Jones, C. F. (2013). The Social Dimensions of Energy Transitions. Sci. as Cult. 22 (2), 135–148. doi:10.1080/09505431.2013.786989
Ming, T., de_Richter, R., Liu, W., and Caillol, S. (2014). Fighting Global Warming by Climate Engineering: Is the Earth Radiation Management and the Solar Radiation Management Any Option for Fighting Climate Change? Renew. Sustainable Energ. Rev. 31, 792–834. doi:10.1016/j.rser.2013.12.032
Ministry of Economy, T. A. I. M. (2018). Japan’s Energy 2017," Ministry of Economy. Japan: Trade and Industry. [Online]. Available: http://www.enecho.meti.go.jp/en/category/brochures/pdf/japan_energy_2017.pdf.
Mitrova, T., and Melnikov, Y. (2019). Energy Transition in Russia. Energy Transit 3, 73–80. doi:10.1007/s41825-019-00016-8
Mohamad, M. R. A., and Anuge, J. (2021). The challenge of Future Sustainable Development in Power Sector. [Online]. Available: https://usir.salford.ac.uk/id/eprint/37674/7/Mostafa%20Mohamad.pdf.
Mohamed, R. K. M. H., Raman, G., Mani, R., and Subramaniam, V. (2020). Green HRM Practices towards Sustainable Performance in Facility Management Industry. Int. J. Management (Ijm) 11 (9), 137–147. doi:10.34218/IJM.11.9.2020.015
Moriarty, H., and Honnery, D. (2019). Energy Accounting for a Renewable Energy Future, (in English). Energies 12 (4280), 1–16. doi:10.3390/en12224280
Mostafa, M. (2014). Challenges to Energy Transition in Egypt: A Study of Wind and Solar Sectors. Germany: Masters, Public Management University of Potsdam.
Mullen, J. D., and Dong, L. (2021). Effects of State and Federal Policy on Renewable Electricity Generation Capacity in the United States. Energ. Econ. 105, 105764. doi:10.1016/j.eneco.2021.105764
Musango, J. K., Amigun, B., and Brent, A. C. (2011). Sustainable Electricity Generation Technologies in South Africa: Initiatives, Challenges and Policy Implications. Energ. Environ. Res. 1 (1), 124–138. doi:10.5539/eer.v1n1p124
Nawaz, W., and Koç, M. (2019). Exploring Organizational Sustainability: Themes, Functional Areas, and Best Practices. Sustainability 11 (16), 4307. doi:10.3390/su11164307
Nguyen, T.-H., Nguyen, L. V., Jung, J. J., Agbehadji, I. E., Frimpong, S. O., and Millham, R. C. (2020). Bio-Inspired Approaches for Smart Energy Management: State of the Art and Challenges. Sustainability 12 (20), 8495. doi:10.3390/su12208495
Ninawe, A. S., Indulkar, S. T., and Amin, A. (2018). “Impact of Climate Change on Fisheries,” in Biotechnology for Sustainable Agriculture. Editors R. L. Singh, and S. Mondal (United Kingdom: Woodhead Publishing), 257–280. doi:10.1016/b978-0-12-812160-3.00009-x
Ölz, S., Sims, R., and Kirchner, N. (2007). Contribution of Renewables to Energy Security. Paris, France: INTERNATIONAL ENERGY AGENCY. [Online]. Available: https://www.iea.org/reports/contribution-of-renewables-to-energy-security.
Owusu, A., and Asumadu-Sarkodie, S. (2016). A Review of Renewable Energy Sources, Sustainability Issues and Climate Change Mitigation. Cogent Eng. 3 (1167990), 1–14. doi:10.1080/23311916.2016.1167990
Palys, M. J., and Daoutidis, P. (2020). Using Hydrogen and Ammonia for Renewable Energy Storage: A Geographically Comprehensive Techno-Economic Study. Comput. Chem. Eng. 136, 106785. doi:10.1016/j.compchemeng.2020.106785
Pegels, A. V.-A., Lutkenhorst, G., and W Altenburg, T. (2018). Politics of Green Energy Policy. J. Environ. Development 27 (1), 26–45. doi:10.1177/1070496517747660
Pegels, A. (2010). Renewable Energy in South Africa: Potentials, Barriers and Options for Support. Energy Policy 38 (9), 4945–4954. doi:10.1016/j.enpol.2010.03.077
Plumer, B. (2021). As Coal Fades in the U.S., Natural Gas Becomes the Climate Battleground. Manhattan, New York City: The New York Times Company. Available at: https://www.nytimes.com/2019/06/26/climate/natural-gas-renewables-fight.html (Accessed, 2021).
Quitzow, R. (2021). Energy Transitions and Societal Change. Berliner Strasse, Germany: Institute of Advanced Sustainability Studies. Available: https://www.iass-potsdam.de/en/research-area/energy-systems-and-societal-change (Accessed, 2021).
Raja, A. K., Srivastava, A., and Dwivedi, M. (2006). Power Plant Engineering. New Delhi, India: New Age International Publishers Ltd.
Rathor, S. K., and Saxena, D. (2020). Energy Management System for Smart Grid: An Overview and Key Issues. Int. J. Energ. Res 44, 4067–4109. doi:10.1002/er.4883
Rathor, S. K., and Saxena, D. (2020). Energy Management System for Smart Grid: An Overview and Key Issues. Int. J. Energ. Res. 44, 4067–4109. doi:10.1002/er.4883
Rhodes, R. (2021). Why Nuclear Power Must Be Part of the Energy Solution. New Haven, USA: Yale school of the Environment. Available at: https://e360.yale.edu/features/why-nuclear-power-must-be-part-of-the-energy-solution-environmentalists-climate (accessed, 2021).
Ritchie, H., and Roser, M. (2021). Electricity Mix. Oxford, UK: Our World of data. Available at: https://ourworldindata.org/electricity-mix#citation (accessed, 2021).
Ritchie, H. (2021). What Are the Safest and Cleanest Sources of Energy? Oxford, UK: Our World in Data. Available at: https://ourworldindata.org/safest-sources-of-energy. (accessed, 2021).
Robyns, B., Davigny, A., Francois, B., Henneton, A., and Sprooten, J. (2012). Electricity Production from Renewable Energies. New Jersey, USA: John Wiley & Sons.
Rosen, M. A. (2009). Energy Sustainability: A Pragmatic Approach and Illustrations. Sustainability 1 (1), 55–80. doi:10.3390/su1010055
Sagan, S. D. (2011). The Causes of Nuclear Weapons Proliferation. Annu. Rev. Polit. Sci. 14, 225–244. [Online]. Available: http://www.scopus.com/inward/record.url?eid=2-s2.0-79955949040&partnerID=40&md5=a53c9fe0cb5f3e6b2a071b3afd868b06. doi:10.1146/annurev-polisci-052209-131042
Saikia, K., Kakati, B. K., Boro, B., and Verma, A. (2018). “Current Advances and Applications of Fuel Cell Technologies,” in Recent Advancements in Biofuels and Bioenergy Utilization. Editors P. K. Sarangi, S. Nanda, and P. Mohanty (Singapore: Springer), 303–337. doi:10.1007/978-981-13-1307-3_13
Samaras, C., Nuttall, W. J., and Bazilian, M. (2019). Energy and the Military: Convergence of Security, Economic, and Environmental Decision-Making. Energ. Strategy Rev. 26, 100409. doi:10.1016/j.esr.2019.100409
Sasmaz, M. U., Sakar, E., Yayla, Y. E., and Akkucuk, U. (2020). The Relationship between Renewable Energy and Human Development in OECD Countries: A Panel Data Analysis. Sustainability 12 (18), 7450. doi:10.3390/su12187450
Scheneider Electric Company (2019). Schneider Sustainability Report 2017 – 2018. Nanterre, France: Schneider Electric SE, France. [Online]. Available: https://www.se.com/ww/en/assets/564/document/46127/schneider-sustainability-report-2017-2018.pdf.
Schiffer, H., and Trüby, J. (2018). A Review of the German Energy Transition: Taking Stock, Looking Ahead, and Drawing Conclusions for the Middle East and North Africa. Energy Transitions 2, 1. doi:10.1007/s41825-018-0010-2
Seghezzo, L. (2009). The Five Dimensions of Sustainability. Environ. Polit. 18 (4). doi:10.1080/09644010903063669
Şengül, Ü., Eren, M., Eslamian Shiraz, S., Gezder, V., and Şengül, A. B. (2015). Fuzzy TOPSIS Method for Ranking Renewable Energy Supply Systems in Turkey. Renew. Energ. 75, 617–625. doi:10.1016/j.renene.2014.10.045
Smil, V. (2010). Energy Transitions: History, Requirements, Prospects. Santa Barbara, CA, USA: Praeger.
Solarin, S. A., Bello, M. O., and Bekun, F. V. (2021). Sustainable Electricity Generation: the Possibility of Substituting Fossil Fuels for Hydropower and Solar Energy in Italy. Int. J. Sustainable Development World Ecol. 28 (5), 429–439. doi:10.1080/13504509.2020.1860152
Streimikienea, D., BalezentisbIrena, T., Krisciukaitienėb, K., and Balezentisa, A. (2012). Prioritizing Sustainable Electricity Production Technologies: MCDM Approach. Renew. Sustainable Energ. Rev. 16 (5), 3302–3311. doi:10.1016/j.rser.2012.02.067
Tharp, J. (2012). “Project Management and Global Sustainability,” in Global Congress 2012—EMEA, Marsailles, France. Newtown Square (PA: Project Management Institute).
The Royal Academy of Engineering, (2005). in The Royal Academy of Engineering, Engineering for Sustainable Development: Guiding Principles. Editors R. Dodds, and R. Venables (London, UK: The Royal Academy of Engineering), 52. [Online]. Available: https://www.raeng.org.uk/publications/reports/engineering-for-sustainable-development (Accessed on September, 2005).
Timmer, J. (2021). Why Are Nuclear Plants So Expensive? Safety’s Only Part of the story. California, USA: Technica. Available at: https://arstechnica.com/science/2020/11/why-are-nuclear-plants-so-expensive-safetys-only-part-of-the-story (accessed, 2021).
Tracey, S., and Anne, B. (2008). OECD Insights Sustainable Development Linking Economy, Society, Environment: Linking Economy, Society, Environment. Paris, France: OECD Publishing.
Tunc, M., Sisbot, S., and Camdali, U. (2012). Exergy Analysis of Electricity Generation for the Geothermal Resources Using Organic Rankine Cycle: Kızıldere-Denizli Case. Environ. Prog. Sustainable Energ. 32 (3), 830–836. doi:10.1002/ep.11662
United Nations Develpment programme, (2016). Delivering Sustainable Energy in a Changing Climate. [Online]. Available at: http://www.un-energy.org/wp-content/uploads/2017/01/UNDP-Energy-Strategy-2017-2021.pdf.
United Nations Economic and Social Commission (Escap), (2016). Regional Trends Report on Energy for Sustainable Development in Asia and the Pacific 2016. Bangkok, Thailand: United Nations Economic Commision fo Asia and the Pacific. [Online]. Available: https://www.unescap.org/sites/default/files/publications/publication_RTRWEB%20%285%29v2.pdf.
United Nations Environmental Program(Unep), (2019). Emissions Gap Report 2019. Nairobi, Kenya: United Nations Environmental Program. [Online]. Available: https://wedocs.unep.org/bitstream/handle/20.500.11822/30797/EGR2019.pdf?sequence=1&isAllowed=y (Accessed June 16, 2021).
United Nations(Un), (2019). Energy Statistics Pocketbook in Statistics Papers " Department of Economic and Social Affairs (New York, USA. [Online]. Available: https://unstats.un.org/unsd/energy/pocket/2019/2019pb-web.pdf.
United Nations (2019). Energy Statistics Pocketbook. New York: Department of Economic and Social Aairs. [Online]. Available:at: https://unstats.un.org/unsd/energy/pocket/2019/2019pb-web.pdf.
United Nations (2019). The Impact of Rapid Technological Change on Sustainable Development. Geneva, Switzerland: United Nations Commission on Science and Technology for Development, 17. [Online]. Available: https://www.un.org/pga/74/event/the-impact-of-rapid-technological-change-on-the-sustainable-development-goals-and-targets/.
United States Department of Energy (2015). “An Assessment of Energy Technologies and Research Opportunities,” in Quadrennial Technology Review. [Online]. Available: https://www.energy.gov/sites/prod/files/2017/03/f34/qtr-2015-chapter1.pdf.
United States Environmental Protection Agency (Epa), (2017). Energy Resources for State and Local Governments. January: United States Environmental Protection Agency " United States Environmental Protection Agency. [Online]. Available: https://www.epa.gov/statelocalenergy/local-renewable-energy-benefits-and-resources.
University of Alberta (2021). What Is Sustainability? Edmonton, AB, Canada: Office of Sustainability. Available at: https://www.mcgill.ca/sustainability/files/sustainability/what-is-sustainability.pdf (accessed, 2021).
Valencia, F., Billi, M., and Urquiza, A. (2021). Overcoming Energy Poverty through Micro-grids: An Integrated Framework for Resilient, Participatory Sociotechnical Transitions. Energ. Res. Soc. Sci. 75, 102030. doi:10.1016/j.erss.2021.102030
Vezzoli, C., Ceschin, F., Osanjo, L., M’Rithaa, M. K., Moalosi, R., Nakazibwe, V., et al. (2018). “Energy and Sustainable Development,” in Designing Sustainable Energy for All: Sustainable Product-Service System Design Applied to Distributed Renewable Energy. Editor C. Vezzoli, F. Ceschin, L. Osanjo, M. K. M’Rithaa, R. Moalosi, V. Nakazibweet al. (Cham: Springer International Publishing), 3–22.
Viet, D. T., Phuong, V. V., Duong, M. Q., and Tran, Q. T. (2020). Models for Short-Term Wind Power Forecasting Based on Improved Artificial Neural Network Using Particle Swarm Optimization and Genetic Algorithms. Energies 13 (11), 2873. doi:10.3390/en13112873
Vine, E. (2019). Building a Sustainable Organizational Energy Evaluation System in the Asia Pacific. Glob. Energ. Interconnection 2 (5), 378–385. doi:10.1016/j.gloei.2019.11.012
Wallington, T. J., Srinivasan, J., Nielsen, O. J., and Highwood, E. J. (2004). “Greenhouse Gases and Global Warming,” in Environmental and Ecological Chemistry in Encyclopedia of Life Support Systems (EOLSS), Developed under the Auspices of the UNESCO. Editor A. Sabljic (Oxford, UK: Eolss Publishers).
Wang, S., and Wang, S. (2015). Impacts of Wind Energy on Environment: A Review. Renew. Sustainable Energ. Rev. 49, 437–443. doi:10.1016/j.rser.2015.04.137
Wang, H. K. H. (2019). Climate Change and Clean Energy Management Challenges and Growth Strategies. 1st ed.. London: Routledge, 192. [Online]. Available: https://www.taylorfrancis.com/books/mono/10.4324/9781351050715/climate-change-clean-energy-management-henry-wang.
Wanga, Y., Zhanga, D., Ji, Q., and Shi, X. (2020). Regional Renewable Energy Development in China: A Multidimensional Assessment. Renew. Sustainable Energ. Rev. 124, 1–12. doi:10.1016/j.rser.2020.109797
Watson, R. (2014). Tackling the challenge of Climate Change. Alliance of Small Island States AOSIS. [Online]. Available: https://www.aosis.org/wp-content/uploads/2014/09/Tackling-Climate-Change-K.pdf.
Werpy, M. R., Santini, D., Burnham, A., and Mintz, M. (2010). Natural Gas Vehicles: Status, Barriers, and Opportunities. Argonne, Illinois: Energy Systems Division, U.S. Department of Energy.
Wilson, M. (2021). Providing Reliable, Resilient, and Sustainable Energy Solutions. Edmonton, Canada: Stantec. Available at: https://www.stantec.com/en/ideas/content/blog/2021/microgrids-a-critical-key-to-the-energy-transition (accessed, 2021).
World Commission on Environment and Development (WCED) (1987). Our Common Future. New York, USA: Oxford University Press.
World Energy Council (2013). Marine Energy. [Online]. Available: https://www.worldenergy.org/assets/images/imported/2013/10/WER_2013_11_Marine_Energy.pdf.
World Energy Data (2021). World Electricity Generation. Newcastle, Australia: World Energy Data. Available at: https://www.worldenergydata.org/world-electricity-generation/, (accessed, 2021).
World Nuclear Association (2021). Nuclear Power in the World Today. World Nuclear Association. June 2021. Archivedfrom the Original on 16 July 2021. London, United Kingdom: World Nuclear ssociation. Retrieved 19 July 2021. Available at: https://world-nuclear.org/information-library/current-and-future-generation/nuclear-power-in-the-world-today.aspx (accessed, 2021).
Yu, J. M. (2017). Taiwan Power Outage Affected 151 Companies, Caused $3 Million in Damages. Available at: https://www.reuters.com/article/us-taiwan-power-Outages/taiwan-power-outage-affected-151-companies-caused-3-million-in-damages-dUSKCN1AX0S3.
Keywords: renewable energy, sustainable electricity, energy and electricity sustainability, energy transition, energy security, energy transition strategies, global climate change, greenhouse gas emissions
Citation: Kabeyi MJB and Olanrewaju OA (2022) Sustainable Energy Transition for Renewable and Low Carbon Grid Electricity Generation and Supply. Front. Energy Res. 9:743114. doi: 10.3389/fenrg.2021.743114
Received: 17 July 2021; Accepted: 28 December 2021;
Published: 24 March 2022.
Edited by:
Aldo Bischi, Skolkovo Institute of Science and Technology, RussiaCopyright © 2022 Kabeyi and Olanrewaju. This is an open-access article distributed under the terms of the Creative Commons Attribution License (CC BY). The use, distribution or reproduction in other forums is permitted, provided the original author(s) and the copyright owner(s) are credited and that the original publication in this journal is cited, in accordance with accepted academic practice. No use, distribution or reproduction is permitted which does not comply with these terms.
*Correspondence: Moses Jeremiah Barasa Kabeyi, mkabeyi@uonbi.ac.ke, moseskabeyi@yahoo.com