- 1College of Chemistry and Pharmaceutical Engineering, Huanghuai University, Zhumadian, China
- 2College of Petroleum Engineering, Xi’an Shiyou University, Xi’an, China
As an environment-friendly natural gas hydrate exploitation method, CO2 replacement method can not only achieve the purpose of mining natural gas hydrate, but also store the current greenhouse gas CO2 in the form of hydrate on the seabed, and maintain the stratum stability of hydrate deposit area. In order to improve the rate and efficiency of CH4-CO2 replacement reaction, researchers proposed to use CO2 contained gas mixture instead of pure CO2 to replace CH4 in natural gas hydrate. Based our previous work about CH4 hydrate recovery with 40% CO2 + 60% H2, in this study, the effect of gas concentration in gas phase on final CH4 recovery are investigated by implying different time interval of gas exchange operation. Experimental results show that The CH4 recovery efficiency is 10.41 when the gas exchange is continues through the whole replacement process, and CH4 recovery efficiency changes to 12.25, 32.24 and 28.86 when gas exchange operation is carried out every 12, 24, 36 h. Indicating that replaced CH4 needs to be discharged in time to avoid CH4 molecules being replaced to form hydrates again, and it is necessary to accurately control the time interval of gas exchange operation to avoid insufficient contact time between CO2 and H2 molecules and CH4 hydrate, which affects the final replacement efficiency. In addition, the mechanism of CO2 gas mixture containing small gas molecule such as H2, N2 are studied. The results indicate that when CO2 containing small molecules such as H2 and N2 displace CH4 hydrate, the existence of small molecules (H2, N2) can give rise to decompose the hydrate lattice and release CH4 gas. If the gas molecules (CO2, N2, H2, CH4) in the gas phase have enough driving force to enter the hydrate lattice and remain stability, CH4 hydrate will not decompose completely; If not, CH4 hydrate will be completely decomposed.
Introduction
Natural gas hydrate (NGH), which is widely distributed in continental margin and permafrost, is naturally formed when excess gas and water molecules exist in high and low temperature zones (Sloan and Koh, 2007; Chong et al., 2016). The estimated worldwide NGH reserves are about 105–108 trillion cubic feet, twice the total reserves of natural gas, coal and oil resources (Kvenvolden et al., 1993; Boswell and Collett, 2011; Chong et al., 2016). The traditional scheme of recovering CH4 gas from reservoirs is to use the driving potential based on temperature, pressure and chemical potential difference to change the equilibrium condition of NGH reservoir and decompose NGH (Li et al., 2016), including thermal stimulation (Wang et al., 2017; Li et al., 2008; Fitzgerald and Castaldi, 2013), depressurization (Yang et al., 2012; Zhao et al., 2013) and chemical inhibitor injection (Yuan et al., 2011; Javanmardi et al., 2013). Besides these methods, CH4 recovery with CO2 injection into the NHG reserves was firstly proposed by Ohgaki et al. (1996), and it has become a promising way to exploit CH4 from NGH reserves while sequestrating CO2 at the same time (Koh et al., 2012; Lee et al., 2013; Bo et al., 2014; Cha et al., 2015; Zhang et al., 2017). In this method, the heat required for the decomposition of CH4 hydrate (54.49 kJ mol−1) is provided by the heat released during the formation of CO2 hydrate (-57.98 kJ mol−1) (Lee et al., 2003; Ersland, 2007; Falenty et al., 2016; Mu and Solms, 2017). Subsequently, the researchers proposed the exploitation of CH4 hydrate with CO2 containing mixture (Koh et al., 2012; Sun et al., 2019; Tupsakhare and Castaldi, 2019; Wang et al., 2017) and the combined use of the above methods (Li et al., 2011; Kou et al., 2019; Kou et al., 2020; Wan et al., 2020) But there are still many problems, such as low efficiency due to large energy loss to surrounding stratum for thermal stimulation, obstacles of front propagation resulted from hydrate regeneration for depressurization, environmental issues and low productivity for inhibitor injection, and inability of monitoring CO2 utilization for CO2-CH4 replacement. In addition, the influence of instability of NGH reserves has not been well understood, which may lead to sea sediment instability and more serious environmental problems because methane is an about 20 times more efficient greenhouse gas than CO2 (Dlugokencky et al., 2003).
The feasibility of replacing methane hydrate with CO2 has been proven previously (Ohgaki et al., 1996; Nakano et al., 1999), Uchida et al. (2010) investigated the CO2-CH4 replacement process with Raman spectroscopy, and found that methane can occupy large and small cages of sI hydrate, and CO2 often occupies large cages in the process of hydrate reformation. Ota et al. (2005a); Ota et al. (2005b) found that, CH4 hydrate was decomposed during the replacement process, and the decomposition rate of large cage in CH4 hydrate was faster than that of small cage. Lim et al. (2017) investigated the cage occupancy of CH4/N2/CO2 with different gas concentration and found that N2 and CO2 preferentially occupied small cages and large cages respectively. Sun et al. (2017) demonstrated that CO2 molecules in gas mixture control the entrance into hydrate cages. Wang et al. (2017) and Sun et al. (2018) studied the CH4 recovery by CO2/H2 gas mixture, and demonstrated that addition of H2 can improve the CH4 recovery.
During the replacement of CH4 in hydrate with CO2 or CO2 containing gas mixture, the concentration of each gas component in the gas phase changes as the replacement reaction proceeds. These concentration variations, especially for the CH4, affect the driving force of the gas molecule participating in the replacement and final replacement efficiency. Replaced CH4 molecules can form CH4 hydrate again or form CH4-CO2 mixed hydrate together with CO2 molecules, as a result, the new hydrate formed on the surface of the original CH4 hydrate becomes an obstacle to the further contact between CO2 or gas mixture containing CO2 with CH4 hydrate, this will eventually affect the replacement efficiency. Therefore, based on the experimental results of replacement of CH4 hydrate with 40% CO2 + 60% H2 mixture at 275.15 K, 4.5 and 6.0 MPa in our previous work (Ding et al., 2017; Ding et al., 2020), the effects of gas exchange every 12, 24, 36 h and continuous gas exchange (i.e., time interval of gas exchange) on the final replacement efficiency were studied.
Besides, the replacement mechanism of CH4 replacement from CH4 hydrate with CO2 + H2 gas mixture is also proposed: when H2 molecules contact with CH4 hydrate, the lattice of hydrate is disturbed and decomposed, and CH4 molecules escape out. If CO2 has enough driving force to replace CH4 and simultaneously occupy the hydrate cages, the hydrate lattice becomes stable again; If the driving force of CO2 molecule is not enough to occupy the hydrate cages, the lattice will be unstable and decompose to produce water and gas molecules. But when methane hydrate replaced by other gas mixtures containing small gas molecules, is the displacement mechanism the same as that of CO2 + H2 gas mixture? Therefore, 40% CO2 + 60% N2 and 20% CO2 + 80% N2 are used to study the replacement mechanism of CH4 hydrate with CO2 containing gas mixture.
Experimental Device and Method
Experimental Apparatus and Materials
The experimental device is composed of gas supply system, reactor for hydrate formation and decomposition, cooling water circulation system and detection system, as shown in Figure 1. The pure CH4 gas and 40% CO2 + 60% H2, 40% CO2 + 60% N2, 20% CO2 + 80% N2 mixtures used in experiments are supplied by Foshan Huate Gas Co., Ltd. The deionized water used in experiments is supplied by Nanjing ultrapure water technology Co, Ltd. The Raman spectrometer (LabRam, Jobin Yvon) uses 50 times long focusing lens, a 600 grooves/mm monochromator and a multi-channel air-cooled electrically coupled device (CCD) detector. It can release 532 nm wavelength laser Ar ion laser source as the laser emission source. The single crystal silicon standard sample with Raman band at 520.7 cm−1 is used to calibrate the Raman spectrometer.
The gas samples collected during the experiment were analyzed on Agilent 7890A, which is equipped with FID and TCD detector. The test method for gas samples is: the detector is heated from 298.15 to 523.15 K at a constant speed, the flow rate of combustion gas H2 is 30 ml min−1, the flow rate of combustion gas air is 400 ml min−1, and the flow rate of carrier gas helium is 250 ml min−1.
Experimental Steps
In order to compare the effects of different gas mixture on CH4 recovery efficiency, all experiments were carried out at 275.15 K and 6.0 MPa. The volume of reactor is 100 ml, and the amount of water used to form hydrate is 60 ml. The CH4 hydrate is generated by bubbling at the bottom of the reactor under magnetic stirring, and the gas mixture is injected through the bottom of the reactor. About 120 h later, the water in reactor has completely transformed to hydrate which is confirmed by Raman spectroscopy where there is no characteristic peak of water, as the same as the method used before (Ding et al., 2017; Ding et al., 2020). What should be noted is that the experimental data is the average value of two groups of experiments, because each experiment was carried out in two parallel reactors.
Experiment 1, 2 and 3 were conducted using 40% CO2 + 60% H2 to replace CH4 hydrate. In Experiment 1, the mixture of 40% CO2 + 60% H2 was injected after the complete transformation of H2O to hydrate which was confirmed by Raman spectroscopy, and the top vent valve of the reactor was opened at the same time to exhaust slowly (0.45 ml min−1 of exhaust speed) to shift the CH4 in gas phase to 40% CO2 + 60% H2. The top vent valve and the bottom inlet valve were kept open during the whole replacement process. What should be noted is that the pressure in reactor was remained at 6.0 MPa.
In Experiment 2, when the concentration of CH4 in the gas phase was lower than 2% during gas exchange operation, the top vent valve of the reactor was shut off, and the time marked as the beginning of the replacement reaction. After the replacement reaction proceeded 12 h, one gas sample was collected, and then the gas exchange operation was carried out. Till that CH4 concentration was lower than 2% again, another gas sample was collected as beginning of next 12 h of replacement reaction, and the top vent valve was shut off. Afterwards, the gas exchange operation was carried out every 12 h (the inlet and vent valves were opened simultaneously to inject 40% CO2 + 60% H2 gas mixture) to renew the gas in the gas phase till CH4 concentration was lower than 2% again. The gas samples were collected at the beginning and end of the exchange process and detected by gas chromatography to determine the amount of CH4 that were replaced out from hydrate phase within 12 h. Notedly, the inlet valve at the bottom of reactor was open during the whole replacement reaction. The only one difference between Experiment 3 and Experiment 2 is that the gas exchange operation was carried out every 36 h.
In order to study the reaction mechanism of CH4 hydrate replacement by CO2 mixture containing small molecules, the replacement of CH4 hydrate by CO2/N2 mixture with different concentrations was studied at different pressure. CH4 hydrate was replaced by 40% CO2 + 60% N2 mixture at 275.15 K and 6.0 MPa in Experiment 4, 20% CO2 + 80% N2 mixture at 275.15 K and 6.0 MPa in Experiment 5, and 20% CO2 + 80% N2 mixture at 275.15 K and 8.0 MPa in Experiment 6. The comparison of experimental conditions of three experiments is also listed in Table 1. Gas samples were collected every 24 h during the replacement reaction, and the concentration changes of each gas component in the gas phase in the reactor were determined by gas chromatography. After the replacement reaction, the reactor was treated with liquid nitrogen, and then the hydrate was decomposed at room temperature. The decomposed gas was collected and each component concentration in the hydrate phase was determined by gas chromatography.

TABLE 1. The conditions of three different gas exchange intervals in Experiment 1, 2, 3, 4, 5 and 6.
Results and Discussion
In our previous work (Ding et al., 2017; Ding et al., 2020), it has been repeatedly proved that the pure CH4 hydrate formed at 275.15 K and 4.5–6.0 MPa is structure I hydrate, and the proportion of CH4 molecules in large and small cages is about 3:1, so the structure of pure CH4 hydrate formed at 275.15 K and 6.0 MPa and the proportion of CH4 molecules in large and small cages are not determined in this study.
Effect of Ventilation Interval on Displacement Efficiency
In Experiment 1, the gas was continuously discharged at the rate of 0.45 ml min−1 during the whole replacement reaction (the inlet valve of 40% CO2 + 60% H2 was also open and the pressure in reactor was maintained at 6.0 MPa). After the replacement reaction, the gas phase was discharged quickly and the reactor was treated with liquid nitrogen. Immediately, the hydrate phase in reactor was decomposed at room temperature, and the content of each gas component originating from hydrate decomposition was determined by gas chromatography. In Experiment 1, the composition of the final hydrate decomposition gas is 89.59% CH4 and 10.41% CO2, that is, the recovery rate of CH4 is 10.41%.
In Experiment 2, gas samples were collected at the beginning and end of the gas exchange operation, and the gas content in each gas sample were compared to have a deeper understanding of the replacement process. The increment of CH4 and the decrement of CO2 in the gas samples collected during the experiment are listed in Table 2, and is plotted in Figure 2.
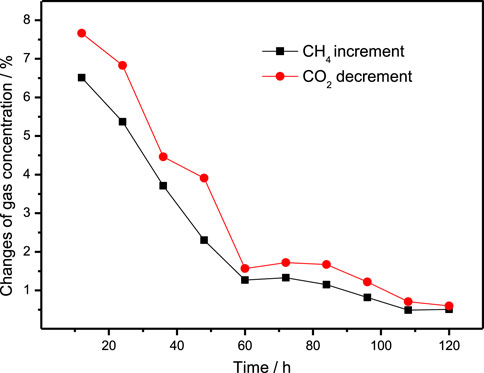
FIGURE 2. The curve of CH4 increment and CO2 decrement in gas samples collected every 12 h in Experiment 2.
It can be seen that the increment of CH4 and the decrement of CO2 in the gas samples collected every 12 h are gradually decreasing, and the decrement of CO2 every 12 h is slightly higher than the increment of CH4, indicating that more CO2 is consumed due to the decomposition of hydrate in the replacement process. This result is consistent with the experimental results of CH4 hydrate replacement with 40% CO2 + 60% H2 mixture, which are present in our previous work (Ding et al., 2017; Ding et al., 2020). The composition of final hydrate decomposition gas is 87.75 CH4 and 12.25% CO2, that is, the CH4 recovery rate is 12.25%.
In Experiment 3, gas samples were collected at the beginning and end of the gas exchange operation every 36 h, the increment of CH4 and the decrement of CO2 in the gas samples collected during the experiment are listed in Table 3, and is plotted in Figure 3. It can be seen from the figure that the increment of CH4 and the decrement of CO2 decrease almost linearly, and the decrement of CO2 is also slightly higher than the increment of CH4 which is agreement with Experiment 2. The composition of the final hydrate decomposition gas is 72.64% CH4 and 28.36% CO2, that is, the CH4 recovery rate is 28.36%.
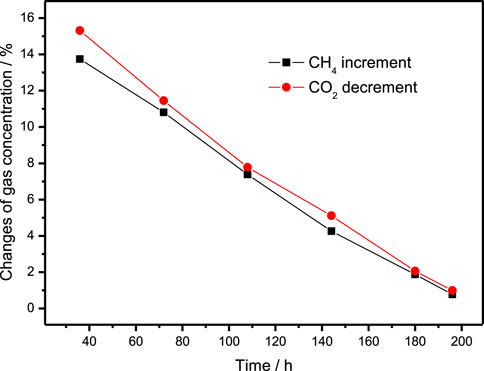
FIGURE 3. The curve of CH4 increment and CO2 decrement in gas samples collected every 36 h in Experiment 3.
The results of Experiment 1, 2 and 3 and experimental results in our previous work (Ding et al., 2020) signed as EP1, are compared in Table 4. It can be seen that different time intervals of gas exchange operation eventually led to different CH4 recovery efficiency in replacement reaction. The lowest CH4 recovery efficiency (10.41%) is obtained with continuous gas exchange meaning that the condition of continuous gas exchange, i.e., the mixture of 40% CO2 + 60% H2 passes through the hydrate area at a relatively faster speed, result in a shorter contact time between CO2 or H2 molecules and CH4 hydrate. Thus, the replacement reaction was cannot effectively carried out, resulting in the final lower CH4 recovery efficiency. The CH4 recovery efficiency increased (12.25%) with the time interval changed to 12 h, and was significantly improved (32.24%) as the gas exchange interval increase to 24 h. However, when time interval increased to 36 h, the recovery efficiency decreased slightly (28.86%). The reason may be that as the time interval increases, CH4 gas that replaced from hydrate phase during the replacement reaction reformed CH4 hydrate again or formed CH4-CO2 mixed hydrate together with CO2.
With the above experimental results, it can be proposed that in the real process of using gas replacement method to exploit NGH, the replaced CH4 needs to be discharged from the sediment in time to avoid the replaced CH4 gas forming CH4 hydrate again or forming CH4-CO2 mixed hydrate together with CO2 and so affecting the exploitation efficiency. At the same time, it is necessary to control the frequency of gas extraction from hydrate sediment, so as to avoid the incomplete contact between CO2 molecules or other small gas molecules and CH4 hydrate, which makes the lower CH4 recovery efficiency.
The Replacement Mechanism of CH4 Hydrate by CO2 Mixture Containing N2, H2
In Experiments 4, 5 and 6, CH4 hydrate was replaced by 40% CO2 + 60% N2 at 6.0 MPa, and by 20% CO2 + 80% N2 at 6.0 and 8.0 MPa. Combined with the experimental results of replacing CH4 hydrate with 40% CO2 + 60% H2, the replacement mechanism of CH4 hydrate by CO2 mixture containing H2 or N2 was discussed.
In Experiment 4, after the water in the reactor was completely converted into hydrate, the gas mixture of 40% CO2 + 60% N2 was injected into the reactor until the CH4 concentration in the gas phase was less than 2%, the replacement reaction began. The gas exchange operation was carried out every 24 h. Table 5 shows the CH4 and CO2 content changes in the gas samples collected during the replacement process of Experiment 4, and these data are plotted in Figure 4. It can be seen that more CH4 is replaced in the first 48 h of the replacement process (the increments of CH4 concentration in the gas sample every 24 h were 8 and 4% respectively), while less CH4 is replaced out in the subsequent replacement process. This result is quite different from that of CH4 hydrate replacement with CO2/H2 mixture (more CH4 is replaced in the first 5 days). Similar to that of CH4 hydrate replacement with CO2/H2 mixture, the reduction of CO2 in gas samples is slightly higher than the increment of CH4 every 24 h. It is suggested that the decomposition of CH4 hydrate may also occur in the process of CH4 hydrate replacement with 40% CO2 + 60% N2, resulting in the decrement of CO2 being higher than the increment of CH4, as observed in CH4 hydrate replacement with 40% CO2 + 60% H2.
Finally, the concentration of each gas component in the hydrate decomposition gas was detected by gas chromatography, and showed as 26.31% CO2, 2.54% N2 and 71.15% CH4. In other words, at 275.15 K and 6.0 MPa, the CH4 recovery efficiency is 28.85% by using 40% CO2 + 60% N2, which is lower than that using 40% CO2 + 60% H2 at the same temperature and pressure (32.24%). However, in the experiment of replacing CH4 hydrate with 40% CO2 + 60% H2, there are only CO2 and CH4 in the final hydrate dissociation gas, and H2 does not exist in the hydrate phase; In contrast, there is 2.5% N2 in the final hydrate phase in the experiment of replacing CH4 hydrate with 40% CO2 + 60% N2, indicating that N2 molecules entered the hydrate lattice and occupied the hydrate cages.
The obtained low CH4 recovery efficiency may be resulted from that the partial pressure of CO2 reaches 2.4 MPa (above the pressure of CO2 hydrate formation at 275.15 K) during the replacement process, which bring about CO2 hydrate formed quickly on the surface of CH4 hydrate and hindered the further contact between the injected gas mixture and CH4 hydrate, resulting in the low final replacement efficiency. So, in the following Experiment 5, CH4 hydrate was replaced by 20% CO2 + 80% N2 at 275.15 K and 6.0 MPa, and the partial pressure of CO2 was only 1.2 MPa (below the pressure of CO2 hydrate formation at 275.15 K).
After the water in the reactor is completely converted into hydrate, 20% CO2 + 80% N2 is injected and the CH4 gas in the gas phase area of the reactor is discharged at the same time. The photos of the reactor taken during the experiment are shown in Figure 5. Figure a is the picture of the reactor before injecting 20% CO2 + 80% N2 mixture, and figure b, c, d, e and f are the picture of the reactor at 4, 6, 8, 10 and 12 h after injecting gas respectively. It can be seen that most of the hydrate decomposes within 12 h, and the hydrate completely decomposes within 24 h. It shows that the mixture of 20% CO2 + 80% N2 cannot react with the decomposed water to form stable hydrate at this temperature and pressure (275.15 K, 6.0 MPa).
On the basis of Experiment 5, the reaction of CH4 hydrate with 20% CO2 + 80% N2 mixture at 275.15 K and 8.0 MPa, where the partial pressure of CO2 increased to 1.6 MPa, was carried out in Experiment 6. The experimental results are the same as those of Experiment 5. CH4 hydrate is decomposed in 24 h and no new hydrate is formed in the reactor.
In addition to the above experiments, the interaction between CH4 hydrate and pure N2 was also carried out. The pictures taken during the experiment are shown in Figure 6, the pictures a, b, c, d and e in the figure are the pictures of the reactor taken before the start of reaction and after 4, 8, 12 and 20 h of reaction respectively. It can be seen that CH4 hydrate gradually decomposes after the replacement process begins, and almost decomposes within 24 h. It shows that the contact of N2 with CH4 hydrate will lead to the destruction of hydrate lattice and release CH4 gas.
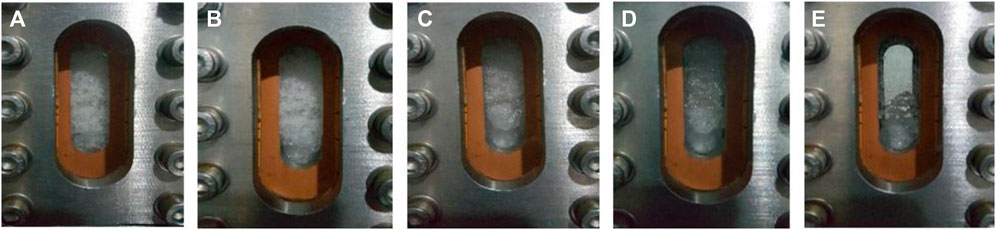
FIGURE 6. The pictures during replacement process with pure N2 (A∼0 h, B∼4 h, C∼8 h, D∼12 h, E∼20 h).
Through the above experiments, it can be seen that CH4 hydrate will be completely decomposed when the partial pressure of CO2 in the mixture is too small to form hydrate; When the mixture can form hydrate stably, CH4 hydrate will not decompose completely.
Conclusion
According to Experiments 1, 2, 3 and previous work, in the process of replacing CH4 hydrate with CO2/H2 mixture, the replaced CH4 needs to be discharged in time to avoid the replacement of CH4 molecules to form hydrate again. At the same time, the time interval of CH4 gas exchange process needs to be controlled accurately to avoid that the contact time of CO2, N2, H2 molecules with CH4 hydrate is not enough which affect the final replacement efficiency.
Probably, when CO2 mixture containing small molecules such as H2 and N2 replace CH4 hydrate, small molecules such as H2 and N2 attack the hydrate lattice, which can give rise to decompose the hydrate lattice and release CH4 gas. If the gas molecules (CO2, N2, H2, CH4) in the gas phase have enough driving force to enter the hydrate lattice and remain stability, CH4 hydrate will not decompose completely; If CO2 in the mixture does not have enough driving force to form hydrate or mixed hydrate, CH4 hydrate will be completely decomposed. Nevertheless, the further research is needed to elaborate the mechanism more thoroughly.
Data Availability Statement
The raw data supporting the conclusions of this article will be made available by the authors, without undue reservation.
Author Contributions
Y-LD did the initial experiment and wrote the first draft. H-QW assisted in the experiment and revised the paper. TL proofread the figures and tables.
Funding
We are grateful for the support of Key Projects of Universities in Henan Province (21B530004), Key Research and Development and Promotion Projects in Henan Province (21 212102311152) and Cultivation Project of National Scientific Research Projects in Huanghuai University (XKPY-202105).
Conflict of Interest
The authors declare that the research was conducted in the absence of any commercial or financial relationships that could be construed as a potential conflict of interest.
Publisher’s Note
All claims expressed in this article are solely those of the authors and do not necessarily represent those of their affiliated organizations, or those of the publisher, the editors and the reviewers. Any product that may be evaluated in this article, or claim that may be made by its manufacturer, is not guaranteed or endorsed by the publisher.
References
Boswell, R., and Collett, T. S. (2011). Current Perspectives on Gas Hydrate Resources. Energy Environ. Sci. 4 (4), 1206–1215. doi:10.1039/c0ee00203h
Cha, M., Shin, K., Lee, H., Moudrakovski, I. L., Ripmeester, J. A., and Seo, Y. (2015). Kinetics of Methane Hydrate Replacement with Carbon Dioxide and Nitrogen Gas Mixture Using In Situ NMR Spectroscopy. Environ. Sci. Technol. 49 (3), 1964–1971. doi:10.1021/es504888n
Chong, Z. R., Yang, S. H. B., Babu, P., Linga, P., and Li, X.-S. (2016). Review of Natural Gas Hydrates as an Energy Resource: Prospects and Challenges. Appl. Energ. 162, 1633–1652. doi:10.1016/j.apenergy.2014.12.061
Ding, Y.-L., Wang, H.-Q., Xu, C.-G., and Li, X.-S. (2020). The Effect of CO2 Partial Pressure on CH4 Recovery in CH4-CO2 Swap with Simulated IGCC Syngas. Energies 13 (5), 1017. doi:10.3390/en13051017
Ding, Y.-L., Xu, C.-G., Yu, Y.-S., and Li, X.-S. (2017). Methane Recovery from Natural Gas Hydrate with Simulated IGCC Syngas. Energy 120, 192–198. doi:10.1016/j.energy.2016.12.129
Dlugokencky, E. J., Houweling, S., Bruhwiler, L., Masarie, K. A., and Tans, P. P. (2003). Atmospheric Methane Levels off: Temporary Pause or a New Steady-State? Geophys. Res. Lett. 30 (19), ASC 5-1–ASC 5-4. doi:10.1029/2003GL018126
Ersland, B. (2007). Storage of CO2 in Natural Gas Hydrate Reservoirs and the Effect of Hydrate as an Extra Sealing in Cold Aquifers. Int. J. Greenh. Gas Contr. 1 (2), 236–246. doi:10.1016/S1750-5836(06)00002-8
Falenty, A., Qin, J., Salamatin, A. N., Yang, L., and Kuhs, W. F. (2016). Fluid Composition and Kinetics of the In Situ Replacement in CH4-CO2 Hydrate System. J. Phys. Chem. C 120 (48), 27159–27172. doi:10.1021/acs.jpcc.6b09460
Fitzgerald, G. C., and Castaldi, M. J. (2013). Thermal Stimulation Based Methane Production from Hydrate Bearing Quartz Sediment. Ind. Eng. Chem. Res. 52 (19), 6571–6581. doi:10.1021/ie400025f
Javanmardi, J., Babaee, S., Eslamimanesh, A., and Mohammadi, A. H. (2012). Experimental Measurements and Predictions of Gas Hydrate Dissociation Conditions in the Presence of Methanol and Ethane-1,2-Diol Aqueous Solutions. J. Chem. Eng. Data 57 (5), 1474–1479. doi:10.1021/je2013846
Koh, D.-Y., Kang, H., Kim, D.-O., Park, J., Cha, M., and Lee, H. (2012). Recovery of Methane from Gas Hydrates Intercalated within Natural Sediments Using CO2 and a CO2/N2 Gas Mixture. ChemSusChem 5 (8), 1443–1448. doi:10.1002/cssc.201100644
Kou, X., Li, X.-S., Wang, Y., Zhang, Y., and Chen, Z.-Y. (2020). Distribution and Reformation Characteristics of Gas Hydrate during Hydrate Dissociation by thermal Stimulation and Depressurization Methods. Appl. Energ. 277, 115575. doi:10.1016/j.apenergy.2020.115575
Kou, X., Wang, Y., Li, X.-S., Zhang, Y., and Chen, Z.-Y. (2019). Influence of Heat Conduction and Heat Convection on Hydrate Dissociation by Depressurization in a Pilot-Scale Hydrate Simulator. Appl. Energ. 251, 113405. doi:10.1016/j.apenergy.2019.113405
Kvenvolden, K. A., Ginsburg, G. D., and Soloviev, V. A. (1993). Worldwide Distribution of Subaquatic Gas Hydrates. Geo-Mar. Lett. 13 (1), 32–40. doi:10.1007/BF01204390
Lee, B. R., Koh, C. A., and Sum, A. K. (2014). Quantitative Measurement and Mechanisms for CH4 Production from Hydrates with the Injection of Liquid CO2. Phys. Chem. Chem. Phys. 16 (28), 14922–14927. doi:10.1039/c4cp01780c
Lee, H., Seo, Y., Seo, Y.-T., Moudrakovski, I. L., and Ripmeester, J. A. (2003). Recovering Methane from Solid Methane Hydrate with Carbon Dioxide. Angew. Chem. Int. Ed. 42 (41), 5048–5051. doi:10.1002/anie.200351489
Lee, S., Lee, Y., Lee, J., Lee, H., and Seo, Y. (2013). Experimental Verification of Methane-Carbon Dioxide Replacement in Natural Gas Hydrates Using a Differential Scanning Calorimeter. Environ. Sci. Technol. 47 (22), 13184–13190. doi:10.1021/es403542z
Li, G., Li, X.-S., Wang, Y., and Zhang, Y. (2011). Production Behavior of Methane Hydrate in Porous media Using Huff and Puff Method in a Novel Three-Dimensional Simulator. Energy 36 (5), 3170–3178. doi:10.1016/j.energy.2011.03.006
Li, X.-S., Wan, L.-H., Li, G., Li, Q.-P., Chen, Z.-Y., and Yan, K.-F. (2008). Experimental Investigation into the Production Behavior of Methane Hydrate in Porous Sediment with Hot Brine Stimulation. Ind. Eng. Chem. Res. 47 (23), 9696–9702. doi:10.1021/ie8009582
Li, X.-S., Xu, C.-G., Zhang, Y., Ruan, X.-K., Li, G., and Wang, Y. (2016). Investigation into Gas Production from Natural Gas Hydrate: a Review. Appl. Energ. 172, 286–322. doi:10.1016/j.apenergy.2016.03.101
Lim, D., Ro, H., Seo, Y., Seo, Y.-j., Lee, J. Y., Kim, S.-J., et al. (2017). Thermodynamic Stability and Guest Distribution of CH4/N2/CO2 Mixed Hydrates for Methane Hydrate Production Using N2/CO2 Injection. J. Chem. Thermodyn. 106, 16–21. doi:10.1016/j.jct.2016.11.012
Mu, L., and von Solms, N. (2017). Methane Production and Carbon Capture by Hydrate Swapping. Energy Fuels 31 (4), 3338–3347. doi:10.1021/acs.energyfuels.6b01638
Nakano, S., Moritoki, M., and Ohgaki, K. (1999). High-pressure Phase Equilibrium and Raman Microprobe Spectroscopic Studies on the Methane Hydrate System. J. Chem. Eng. Data 44 (2), 254–257. doi:10.1021/je980152y
Ohgaki, K., Takano, K., Sangawa, H., Matsubara, T., and Nakano, S. (1996). Methane Exploitation by Carbon Dioxide from Gas Hydrates. Phase Equilibria for CO2-CH4 Mixed Hydrate System. J. Chem. Eng. Jpn. 29 (3), 478–483. doi:10.1252/jcej.29.478
Ota, M., Abe, Y., Watanabe, M., Smith, R. L., and Inomata, H. (2005a). Methane Recovery from Methane Hydrate Using Pressurized CO2. Fluid Phase Equilibria 228-229, 553–559. doi:10.1016/j.fluid.2004.10.002
Ota, M., Morohashi, K., Abe, Y., Watanabe, M., Smith, Jr., R. L., and Inomata, H. (2005b). Replacement of CH4 in the Hydrate by Use of Liquid CO2. Energ. Convers. Manage. 46 (11-12), 1680–1691. doi:10.1016/j.enconman.2004.10.002
Sloan, E. D., and Koh, C. (2007). Clathrate Hydrates of Natural Gases. 3rd edition. Boca Raton, FL, USA: CRC Press. ISBN: 9780429129148.
Sun, Y.-F., Wang, Y.-F., Zhong, J.-R., Li, W.-Z., Li, R., Cao, B.-J., et al. (2019). Gas Hydrate Exploitation Using CO2/H2 Mixture Gas by Semi-continuous Injection-Production Mode. Appl. Energ. 240, 215–225. doi:10.1016/j.apenergy.2019.01.209
Sun, Y.-F., Zhong, J.-R., Li, R., ZhuCao, T. X. Y., Cao, X.-Y., Chen, G.-J., et al. (2018). Natural Gas Hydrate Exploitation by CO2/H2 Continuous Injection-Production Mode. Appl. Energ. 226, 10–21. doi:10.1016/j.apenergy.2018.05.098
Sun, Y.-H., Li, S.-L., Zhang, G.-B., GuoZhu, W. Y. H., and Zhu, Y.-H. (2017). Hydrate Phase Equilibrium of CH4+N2+CO2 Gas Mixtures and Cage Occupancy Behaviors. Ind. Eng. Chem. Res. 56 (28), 8133–8142. doi:10.1021/acs.iecr.7b01093
Tupsakhare, S. S., and Castaldi, M. J. (2019). Efficiency Enhancements in Methane Recovery from Natural Gas Hydrates Using Injection of CO2/N2 Gas Mixture Simulating In-Situ Combustion. Appl. Energ. 236, 825–836. doi:10.1016/j.apenergy.2018.12.023
Uchida, T., Ikeda, I. Y., Takeya, S., Kamata, Y., Ohmura, R., Nagao, J., et al. (2005). Kinetics and Stability of CH4-CO2 Mixed Gas Hydrates during Formation and Long-Term Storage. Chemphyschem 6 (4), 646–654. doi:10.1002/cphc.200400364
Wan, Q.-C., Si, H., Li, B., Yin, Z.-Y., Gao, Q., Liu, S., et al. (2020). Energy Recovery Enhancement from Gas Hydrate Based on the Optimization of thermal Stimulation Modes and Depressurization. Appl. Energ. 278, 115612. doi:10.1016/j.apenergy.2020.115612
Wang, X.-H., Sun, Y.-F., Wang, Y.-F., Li, N., Sun, C.-Y., Chen, G.-J., et al. (2017a). Gas Production from Hydrates by CH4-CO2/H2 Replacement. Appl. Energ. 188, 305–314. doi:10.1016/j.apenergy.2016.12.021
Wang, Y., Feng, J.-C., Li, X.-S., and Zhang, Y. (2017b). Experimental Investigation of Optimization of Well Spacing for Gas Recovery from Methane Hydrate Reservoir in sandy Sediment by Heat Stimulation. Appl. Energ. 207, 562–572. doi:10.1016/j.apenergy.2017.06.068
Yang, X., Sun, C.-Y., Su, K.-H., Yuan, Q., Li, Q.-P., and Chen, G.-J. (2012). A Three-Dimensional Study on the Formation and Dissociation of Methane Hydrate in Porous Sediment by Depressurization. Energ. Convers. Manage. 56, 1–7. doi:10.1016/j.enconman.2011.11.006
Yuan, Q., Sun, C.-Y., Yang, X., Ma, P.-C., Ma, Z.-W., Li, Q.-P., et al. (2011). Gas Production from Methane-Hydrate-Bearing Sands by Ethylene Glycol Injection Using a Three-Dimensional Reactor. Energy Fuels 25, 3108–3115. doi:10.1021/ef200510e
Zhang, X., Li, Y., Yao, Z., Li, J., Wu, Q., and Wang, Y. (2017). Experimental Study on the Effect of Pressure on the Replacement Process of CO2-CH4 Hydrate below the Freezing Point. Energy Fuels 32 (1), 646–650. doi:10.1021/acs.energyfuels.7b02655
Keywords: CH4 hydrate, CO2 replacement, gas exchange interval, mechanism, small molecules
Citation: Ding Y-L, Wang H-Q and Lv T (2021) Effect of Gas Exchange Interval on CH4 Recovery Efficiency and Study of Mechanism of CH4 Hydrate Replacement by CO2 Mixture. Front. Energy Res. 9:760235. doi: 10.3389/fenrg.2021.760235
Received: 17 August 2021; Accepted: 22 September 2021;
Published: 11 October 2021.
Edited by:
Chungang Xu, Guangzhou Institute of Energy Conversion (CAS), ChinaReviewed by:
Xuebing Zhou, Guangzhou Institute of Energy Conversion (CAS), ChinaBai Jing, Zhengzhou University, China
Dongyu Wu, Central South University, China
Copyright © 2021 Ding, Wang and Lv. This is an open-access article distributed under the terms of the Creative Commons Attribution License (CC BY). The use, distribution or reproduction in other forums is permitted, provided the original author(s) and the copyright owner(s) are credited and that the original publication in this journal is cited, in accordance with accepted academic practice. No use, distribution or reproduction is permitted which does not comply with these terms.
*Correspondence: Ya-Long Ding, ZGluZ3lhbG9uZ0BodWFuZ2h1YWkuZWR1LmNu