A Review on Combustion Characteristics of Ammonia as a Carbon-Free Fuel
- 1Guangzhou Institute of Energy Conversion, Chinese Academy of Sciences, Guangzhou, China
- 2Guangdong Key Laboratory of New and Renewable Energy Research and Development, Guangzhou, China
- 3Southern Marine Science and Engineering Guangdong Laboratory (Guangzhou), Guangzhou, China
- 4School of Energy Science and Engineering, University of Science and Technology of China, Guangzhou, China
- 5Department of Chemical Systems Engineering, Nagoya University, Nagoya, Japan
A comprehensive review of combustion characteristics of ammonia (NH3) as a carbon free fuel is presented. NH3 is an attractive alternative fuel candidate to reduce the consumption of fossil fuel and the emission of CO2, soot, and hydrocarbon pollutants, due to its comparable combustion properties, productivities from renewable sources, and storage and transportation by current commercial infrastructure. However, the combustion properties of NH3 are quite different from conventional hydrocarbon fuels, which highlight the specific difficulties during the application of NH3. Therefore, this paper presents comparative experimental and numerical studies of the application of NH3 as a fuel during combustion process, including the combustion properties of laminar burning velocity, flame structures, pollutant emissions for the application of NH3 as a carbon free fuel. This paper presents the burning velocity and pollutant emissions of NH3 alone and mixtures with other fuels to improve the combustion properties. The aim of this paper is to review and describe the suitability of NH3 as a fuel, including the combustion and emission characteristics of NH3 during its combustion process.
Introduction
With the rapid economic development and population explosion of recent decades, the global primary energy consumption has increased dramatically. Coal, petroleum, and natural gas remain the major sources of energy across the world. Pollutants such as CO2, CO, SO2, NOx, volatile organic compounds, particulate matter et al., are released by the combustion of petroleum, coal, and natural gas fuels (IEA, 2021). CO2 is the major contributor to global warming. In the recent years, the development of new technologies aiming to reduce problems related to energy consumption, for application in both developed and developing countries, has become urgent, because of the increasing strictness of governmental regulation of energy and CO2 emissions. Such developments lead to the search for carbon-free fuels, and the increasing importance of renewable energy sources (Astbury, 2008).
Combustion process during various industry processes contributed huge amount of CO2 emissions. In order to obtain the target of zero-carbon emissions, it is necessary to reduce CO2 emissions with the application of carbon-free fuel in combustion system. NH3 is an attractive hydrogen carrier with a high hydrogen density of 17.8% per unit weight, which can be considered as a carbon-free fuel with the advantage of 1) CO2, SOx, and soot emission free; 2) production from various sources, such as fossil fuels, renewable sources, and biomass; 3) transport and storage with existing facility, such as fuel tanks, ships, trucks, and pipelines, which makes NH3 as a favorable alternative fuel candidate (Wang et al., 2007; Zamfirescu and Dincer, 2008; Zamfirescu and Dincer, 2009; Andersson and Lundgren, 2014; Habgood et al., 2015; Afif et al., 2016; Chai et al., 2021). As shown in Figure 1, NH3 is synthesized from energy sources such as wind power, nuclear energy, and fossil fuels with CO2 capture and storage (CCS), etc., which means that NH3 is fully CO2 free recyclable as a promising carbon-free fuel.
The potential of NH3 as a carbon-free fuel owing to its suitable storage and transportation properties has been demonstrated, and applications for its combustion in vehicular engines and turbines are apparent. However, major challenges for NH3 combustion are to provide its alternative fuels capabilities with outstanding performance, durability and reliability, optimize combustion properties, and lower pollutant emissions, which needs a strict determination of combustion properties during the application of NH3 as a fuel. The lack of understanding of the NH3 combustion characteristics, methods of combustion enhancement, and optimization of NOx formation in combustion have placed limits on the utilization of NH3 as a fuel. It is necessary to have a comprehensive understanding of the combustion and emission characteristics of pure NH3 and mixture with other fuels, which are expected to supply power for transport vehicles, electricity for power generation, and heating for industry with suitable combustion properties in future energy system.
Properties of NH3
As a carbon-free chemical compound, NH3 is a colorless gas with a characteristic pungent smell. The chemical and physical properties of NH3 are listed in Table 1. In 1909, German chemists Fritz Haber and Carl Bosch developed the NH3 production process, now known as the Haber-Bosch process, which catalytically converts N2 (from air) and H2 (from industrial processes) to NH3 using a metal catalyst under high-pressure (150–250 atm) and high-temperature (400–600°C) conditions. The production of NH3 has dramatically increased in recent years. In 2020, the global NH3 production was around 144 million metric ton (shown in Figure 2). The highest individual producers are China is (48.0 million ton: 33.3%), India (12.0 million ton: 8.3%), Russia (10.3 million ton: 7.2%), and the United States (9.2 million ton: 6.4%) (USGS, 2021). The majority of NH3 is used in industrial and agricultural applications such as fertilizer, plastics, nitric acid, and explosives production, and refrigeration. NH3 is a poisonous gas, and its safety hazard is a significant obstacle in its use. According to the US Department of Health and Human Services, the permissible exposure limit (PEL) for NH3 is 35 ppm (27 mg m−3) as a short-term (15 min) exposure limit (CDC, 1994).
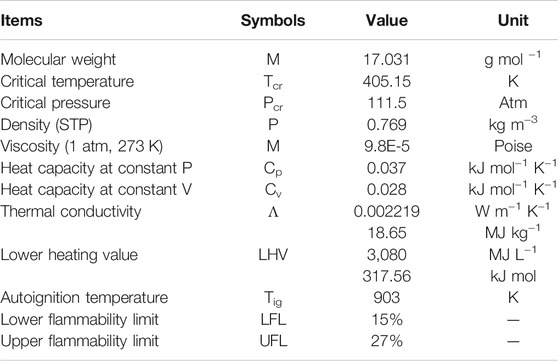
TABLE 1. Chemical and physical properties of gaseous NH3 (NCBI, 2021).
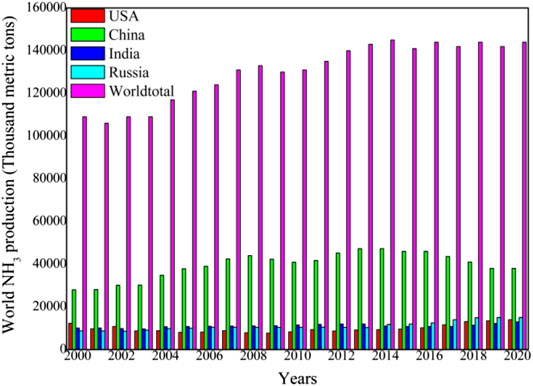
FIGURE 2. World NH3 production (Data from USGS, 2021).
Some characteristics of ammonia are compared with conventional liquefied fuels such as diesel, gasoline, and natural gas, which are listed in Table 2 (Dimitriou and Javaid, 2020). The liquefied ammonia can be easily stored at 298 K and 10.2 atm. But the stored pressure of compressed liquid natural gas is 245.0 atm at 298 K, which is much higher than that of ammonia. Although the fuel density and energy density of ammonia are lower than those of gasoline and diesel, the fuel density of ammonia is three times as many as that of natural gas and the energy density is also much higher than that of natural gas. The octane rating of ammonia is 110 RON, which is higher than those of natural gas and gasoline. It indicates that the engine fueled by ammonia can be operated at higher pressure. What’s more, ammonia exhibits much higher absolute minimum ignition energy than gasoline, which can be improved through some methods like co-firing with hydrogen. Ammonia has potential to be used as a fuel and the performance can be comparable to that of conventional fuel. For example, when directly using ammonia in an internal combustion engine, the driving range can reach 592 km, which is comparable to that of LPG and slightly lower than that of gasoline (Zamfirescu and Dincer, 2008).

TABLE 2. Some characteristics of NH3 versus natural gas, gasoline, and diesel (Dimitriou and Javaid, 2020).
NH3 has been widely used in the industry. It has been used in the manufacture of fertilizers, in organic and inorganic synthesis of chemical compounds as HNO, urea, plastics fibers and so on, in mining and metallurgy, in petroleum refining as neutralizing agent, and also NH3 can be used as commercial refrigerant in food processing, as a substitute for calcium in bisulfite pulping of wood, and to purify municipal and industrial water supplies. Effective methods such as process enclosure, local exhaust ventilation, general dilution ventilation, and personal protective equipment, should be followed in controlling worker exposures or lead of this substance when used it as a fuel.
The use of NH3 as a fuel by adding it to methane (Williams et al., 1991; Bell et al., 2002; Sullivan et al., 2002; Henshaw et al., 2005; Barbas et al., 2015), diesel (Reiter and Kong, 2011; Gill et al., 2012), DME (Gross and Kong, 2013), and H2 (Skreiberg et al., 2004; Mendiara and Glarborg, 2009; Mørch et al., 2011; Joo and Kwon, 2012; Um et al., 2013; Choi et al., 2015) has been investigated. NH3 has often been used as an agent for decreasing NOx emissions in industrial treatment processes through selective catalytic reactions (Xu et al., 2002; Grossale et al., 2009; Yun and Kim, 2013). For CH4 combustion, both the CO and NO emissions decreased with increasing excess oxygen coefficient. A higher CO emission and lower NO emission would result from a decreased oxygen concentration in the oxidizer under the O2/CO2 combustion atmosphere. Conversely, a higher NOx emission and a significantly lower CO emission are formed in oxy-fuel firing compared with those formed with air firing. CO and NOx formation and decomposition would be completely different when NH3 is added to carbon-based fuels. In the absence of NH3 seeding combustion, the most important NO formation process for CH4 combustion is prompt-NO formation, followed by the NNH, thermal-NO, and N2O formation processes. When the combustion mixture is seeded with NH3 at concentrations of several to several thousand ppm, fuel-NO formation becomes the dominant process; with higher NH3 concentrations, a greater portion is converted to N2 rather than to NO (Williams et al., 1991; Barbas et al., 2015). In the ignition engine, the addition of NH3 leads to a longer ignition delay and limits the engine load because of the high autoignition temperature and low combustion rate (or low burning velocity). The combustion pressure and temperature would also be reduced with the addition of NH3, leading to higher CO and HC emissions. NOx formation would be enhanced because of NOx formation from fuel-nitrogen when NH3 is added. However, soot formation would be extremely low (Gross and Kong, 2013). Furthermore, the substitution of NH3 could be favored, not only because of the replacement of the carbon-based fuel, but also because it is an efficient method for reducing overall CO2 emissions (Gill et al., 2012; Gross and Kong, 2013).
Laminar Burning Velocity of NH3
Laminar burning velocity is a significant important parameter during fuel combustion. The laminar burning velocity is the speed at which unburned gases move through the combustion wave in the direction normal to the wave surface, which can be calculated by the Metghalchi and Kech power-law relation as follows (Metghalchi and Keck, 1982):
where
Many researches about laminar burning velocity of ammonia/air flames have been reported, most of which were studied under ambient condition (Zakaznov et al., 1978; Ronney, 1988; Pfahl et al., 2000; Takizawa et al., 2008; Hayakawa et al., 2015a; Han et al., 2019, Mei et al., 2019). Zakaznov et al. measured the burning velocity by cylindrical-tube method at room temperature and atmospheric pressure (Zakaznov et al., 1978). Ronney et al. measured the flame speed by a constant-volume cylindrical combustion vessel at microgravity condition (Ronney, 1988). Pfahl et al. measured the behavior of ammonia/air flames by using the schlieren system in a constant volume combustion vessel (Pfahl et al., 2000). In order to minimize the uncertainty caused by the buoyancy effect, they also measured the horizontal component of the flame motion. Takizawa et al. measured the laminar burning velocity using the spherical-vessel method in a constant volume combustion vessel (Takizawa et al., 2008). Hayakawa et al. measured the flame speed using high speed schlieren photography in a constant volume combustion chamber (Hayakawa et al., 2015a). Recently, Han et al. measured the flame propagation of ammonia/air by using heat flux method (Han et al., 2019). And the laminar burning velocities of ammonia/air mixtures was also investigated using a high-pressure constant-volume cylindrical combustion vessel by Mei et al. (2019). Figure 3 shows the relationship between laminar burning velocities of NH3/air flames and equivalence ratio at 298 K and 1 atm. The range of the flame velocity is roughly from 1.4 to 8.23 cm/s at φ = 0.7–1.3. It can be observed that the results measured by different groups reach their peaks around φ = 1.1, while the maximum values vary from 6.3 to 8.2 cm/s. The relative differences are little at lean conditions, however, a discrepancy more than 2 cm/s appears under fuel-rich condition between the experimental results. The burning velocity of NH3/air flame is relatively low when compared with that of CH4/air flame whose maximum value is about 35 cm/s (Law, 2006).
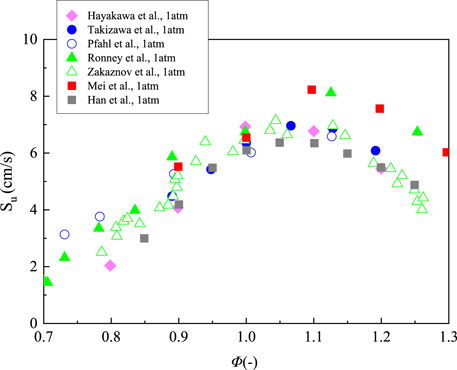
FIGURE 3. Laminar burning velocity of NH3 flame with a function of equivalence ratio at 298 K and 1 atm, replotted from (Han et al., 2019; Kobayashi et al., 2019; Mei et al., 2019).
Ronney et al. measured the flame speed at p = 0.066, 0.132, 0.329, 1, and 1.974 atm. They found that the change of pressure had a modest influence on the flame velocity (Ronney, 1988). Duynslaegher et al. investigated the ammonia/air flame speed by using Konnov mechanism at T = 300K, P = 1–40 atm and φ = 1.0 (Duynslaegher et al., 2010). Results show an increase of pressure had a negative effect on the flame propagation, especially at the low pressure. With the pressure increased from 1 to 10 atm, the flame speed rapidly decreased from 12.67 cm/s to 8.15 cm/s. But with the pressure increased from 30 to 40 atm, the burning velocity decreased smoothly from 6.13 to 5.65 cm/s. Hayakawa et al. studied the laminar burning velocity of ammonia/air flames at elevated pressures by five detailed mechanism, which was validated by the measured experimental results (shown in Figure 4, Hayakawa et al., 2015a). Figure 4 shows the laminar burning velocities under different initial pressure Pi and equivalence ratio φ, plotted along with simulation results by using Tian, Miller, Konnov, GRI 3.0, and Lindsted mechanism (Lindstedt et al., 1994; Miller et al., 1983; Frenklach et al., 2000; Konnov, 2009; Tian et al., 2009). The flame velocity decreases with the increase of initial pressure under the equivalence ratios of 0.9, 1.0, and 1.2. The tendency of the experimental and simulation results is the same as those of hydrocarbon. But it is different from the results of Ronney et al., which are indicated that pressure had a little influence on the flame speed (Ronney, 1988). The initial pressure has a larger effect on flame propagation speed at φ = 1. For example, as pressure increased from 1 to 5 atm, the laminar burning velocity of measured stoichiometric NH3/air flame deceased from 6.9 to 4 cm/s (shown in Figure 4B), while the differences is about 1 cm/s at φ = 0.9 and 1.2 (shown in Figures 4A,C). As shown in Figure 4, most mechanism significantly over-predict the measured burning velocity. It is notable that the simulation results by Konnov mechanism is about two times larger than the measured values while the prediction results by GRI 3.0 mechanism closely agree with the experimental data. However, GRI 3.0 mechanism lacks some ammonia oxidation reactions which are very important for the numerical simulation of NO concentration in ammonia/air flame. Therefore, further improvements of those mechanisms are very necessary. Liu et al. experimentally and numerically studied the variations of laminar burning velocity with initial pressure for ammonia/air flame (Liu et al., 2019a). The flame speed has been measured at initial pressure from 0.3 to 1.6 atm, covering equivalence ratios from 0.5 to 1.3 and at 298 K. The measured laminar burning velocities of NH3 flame firstly increased slightly and then decreased with the increasing pressure. The burning velocity of stoichiometric flame reaches the peak value at 0.5 atm. The maximum values exist at 0.7 atm when the equivalence ratio is 0.5 or 1.3. Generally, the laminar burning velocity decreases with an increase in pressure, which is agreeable with the observation obtained by Hayakawa et al. (2015a). The numerical simulation was calculated by using the GRI 3.0 mechanism under initial pressure ranged from 0.3 to 1.6 atm, and equivalence ratio of 1.0 and temperature of 298 K. The difference between the measurements and the calculations is around 12 cm/s.
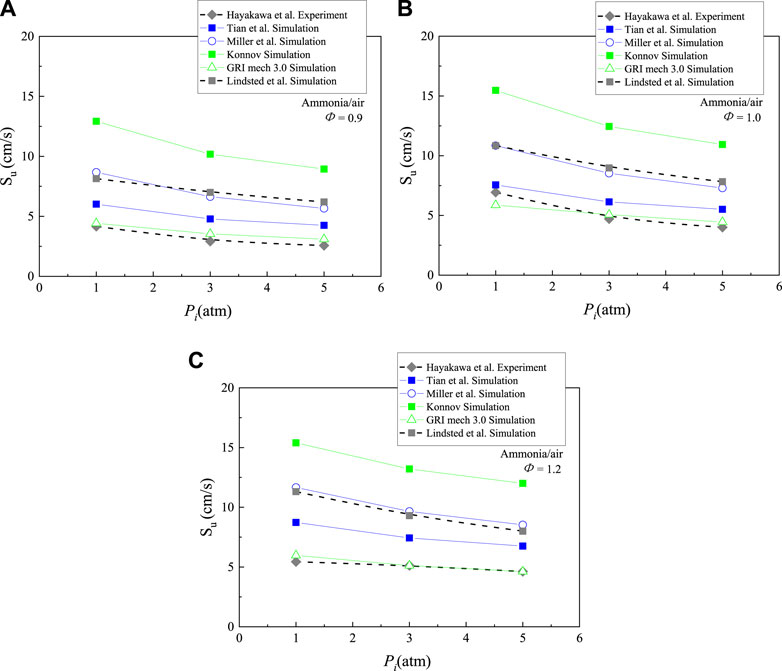
FIGURE 4. Laminar burning velocity of NH3 flame with a function of initial mixture pressure at φ = 0.9, 1 and 1.2, replotted from (Hayakawa et al., 2015a).
The influence of temperature is rarely investigated. Cohen measured the laminar burning velocity of ammonia/air flame at temperature ranged from 323 to 423 K by using a flat flame burner (Cohen, 1955). Recently, Lhuillier et al. measured the flame speed at 1 atm and temperature from 298 to 473 K by the spherical flame method (Lhuillier et al., 2020a). Han et al. measured the laminar burning velocity of NH3 flame using the heat flux method at temperature from 298 to 448 K and 1 atm (shown in Figure 5, Han et al., 2020). Li et al. numerically investigated the effect of preheating temperature on the combustion and NO emission characteristics of ammonia flame (Li et al., 2016a; Li et al., 2016b). In all temperature ranges, the laminar burning velocities increase firstly before the equivalence ratio reaches 1.1, and then decrease when the equivalence ratio is large than 1.1. The maximum value of flame speed at different temperatures ranges from 6.98 to 14.87 cm/s. With the temperature increasing, the flame speed exponentially increases. The results measured by Han et al. at 423 K were compared with those from Cohen and Lhuillier (Cohen, 1955; Han et al., 2020; Lhuillier et al., 2020b). It was found that the experimental values obtained by Han et al. are lower than those from Cohen and Lhuillier. The difference between the measurements is around 2 cm/s, which is larger at fuel-lean conditions. The simulation results by using five mechanisms were also performed. Comparison of numerical and experimental data showed that the simulations results by using Okafor mechanism (Okafor et al., 2018) and Otomo mechanism (Otomo et al., 2018) performed best.
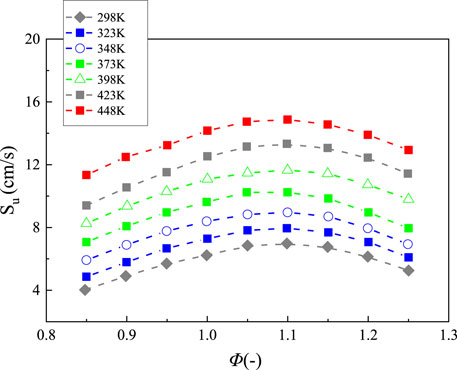
FIGURE 5. Laminar burning velocity of NH3 flame with a function of equivalence ratio at various temperature, replotted from (Han et al., 2020).
Oxygen enriched combustion can be considered as a promising approach to increase burning velocity. The experimental results measured by Takeishi are shown in Figure 6 (Takeishi et al., 2015). It can be observed that the velocities can be close to those of CH4/air flames when the O2 concentration up to 0.35 and the maximum velocity improves to 36.1 cm/s, which is around 5 times the value measured under the air condition. Li et al. (2015) numerically investigated the effect of the oxygen content towards the burning velocity of NH3/air flame by using the Millar mechanism (Millar and Bowman, 1989) and the Reductive Konnov mechanisms (Duynslaegher et al., 2009; Duynslaegher et al., 2012). The maximum laminar burning velocity increases from 27.5 to 33.9 cm/s when the O2 content increases from 0.27 to 0.30, and the values of laminar burning velocity at the O2 content of 0.30 are always 6–7 cm/s higher than those at the O2 content of 0.27. It is mainly due to an increase of the reaction rates of OH, H, O, and NH2 radicals under a higher O2 concentration. It is found that the laminar burning velocities calculated by Li et al. are over-predicted when compared with the results of Takeishi’s experiment at the O2 content of 0.30, especially at fuel rich conditions. For example, the difference between experimental and numerical results reaches 7.9 cm/s at the equivalence ratio of 1.1, while the discrepancy is around 1.5 cm/s at the equivalence ratio of 0.8. Recently, it was found that the burning velocities of ammonia are proper for the practical applications when the range of O2 volume concentration in O2/N2 mixture is roughly from 0.35 to 0.40 (Kim et al., 2021). Mei et al. measured the ammonia/air flame speed under the O2 content ranged from 0.21 to 0.45 at 298 K, the pressure ranged from 1 to 5 atm and the equivalence ratios from 0.7 to 1.5 by using more complicated constant pressure spherical flame method (Mei et al., 2019). The flame speed increases with an increase of the O2 concentration, but decrease with an increase of initial pressure. The experimental results showed that the flame propagation speeds are lower than those of CH4/air flames at the O2 concentration of 0.35. It is different from the results of Takeishi et al., which indicated that the laminar burning velocities at the O2 concentration of 0.35 are almost the same as those of CH4/air flames (Takeishi et al., 2015). In addition, they constructed a model of ammonia combustion with 38 species and 265 reactions, which can predict the results with a favorable satisfaction. Wang et al. measured the oxy-ammonia flame speed in a constant volume vessel at the temperatures ranged from 303 to 393 K, the O2 content ranged from 0.6 to 1.0 and the equivalence ratios ranged from 0.6 to 1.4 (Wang et al., 2020). Results show that the peak value of laminar burning velocity can reach 125.1 cm/s at the O2 content of 1.0. The equivalence ratio corresponding maximum value is around 0.9. Besides, the flame velocity increases with the increasing temperature, which is more sensitive to the change of temperature under lower O2 content.
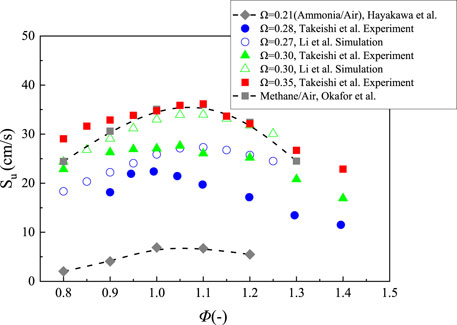
FIGURE 6. Laminar burning velocity of NH3 flame with a function of equivalence ratio at various oxygen enrichment level, replotted from (Hayakawa et al., 2015a, Takeishi et al., 2015; Li et al., 2015; Okafor et al., 2018; Kobayashi et al., 2019).
Due to low burning velocity, ammonia usually is burned with the addition possessing higher reactivity such as hydrogen. Ichikawa et al. measured the ammonia/hydrogen/air flame speed at pressure of 1, 3, and 5 atm by using a high-pressure constant volume chamber (shown in Figure 7, Ichikawa et al., 2015). The experimental results show an exponential variation of laminar burning velocity under different volumetric hydrogen fraction and the ammonia/hydrogen/air flame speed decreased with an increase in pressure under the same hydrogen concentration. It is obvious that the laminar burning velocity largely decreased when the pressure increased from 1 to 3 atm, while the values of burning velocities at 3 atm were almost the same as those of flame speeds at 5 atm. This means that the influence of initial pressure conspicuously appeared under low pressure, but that effects barely occurred under high pressure. Lee et al., Kumar et al., and Li et al. also investigated the flame propagation speed of ammonia/hydrogen/air mixture at 1 atm (Lee et al., 2010; Kumar and Meyer, 2013; Li et al., 2014; Li et al., 2017; Li et al., 2019a). The differences among experimental results were small and the tendencies were consistent with that measured by Ichikawa et al. (2015). It also can be observed that the value of flame speed was around 35.1 cm/s at the hydrogen concentration of 0.45 which was close to that of CH4/air mixture, indicating that the addition of hydrogen can effectively improve ammonia combustion. Han et al. measured the laminar burning velocities of NH3/H2/air by using the heat flux method (covering φ = 0.7–1.6,
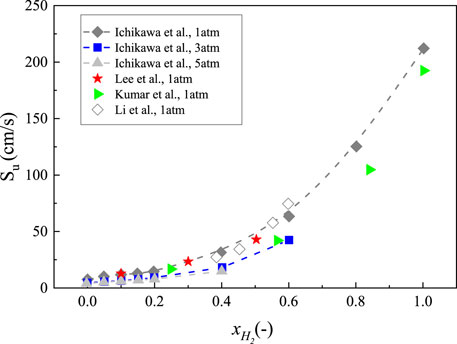
FIGURE 7. Laminar burning velocity of NH3 flame with a function of volumetric hydrogen fraction at 298 K, replotted from (Lee et al., 2010; Kumar and Meyer, 2013; Li et al., 2014; Ichikawa et al., 2015).
Different from adding hydrogen, hydrogen production can also be from ammonia decomposition, since ammonia has high hydrogen density (Valera-Medina et al., 2018; Kobayashi et al., 2019). It was found that ammonia started to crack when the temperature reached 473.15 K and the conversion efficiency can reach 98–99% when the temperature up to 698.15 K (Klerke et al., 2008; Lan et al., 2012). What’s more, in contrast to co-firing hydrogen, using partially cracked ammonia is more economical and easier to implement (Yang et al., 2019). However, the deep insight into the combustion characteristics of NH3/H2/N2 mixtures are limited (Mei et al., 2021; Wiseman et al., 2021). Very recently, Mei et al. experimentally investigated the laminar burning velocities of partially cracked NH3/air mixtures in a high-pressure constant volume cylindrical combustion vessel at the initial pressure of 1–10 atm, the equivalence ratios from 0.7 to 1.4 and the initial temperature of 298 K (Mei et al., 2021). Results showed that the laminar flame speed of NH3/H2/N2/air mixtures increased with an increase of the cracking ratio due to the increase of H2 concentration. It was found that the flame speed could reach 38.1 cm/s at 1 atm and the cracking ratio of 40%, which is close to that of methane/air mixtures. They also found that the increase of N2 concentration and the initial pressure had a negative effect on the flame speed. Then though the numerical simulation, they recognized that H + O2 (+M) = HO2 (+M) plays an important in flame propagation.
Blending ammonia with methane is also an effective method to improve the combustion characteristics of NH3/air flames propagation. Konnov et al. experimentally investigated the laminar burning velocity of CH4/O2/N2 flames doped with 0.5% NH3 by using a heat flux method. Results show that ammonia had no significant influence on the flame speed (Konnov et al., 2006). Okafor et al. and Henshaw et al. measured the flame speeds of NH3/CH4/air flames as a function of the equivalence ratio at 298 K and 1 atm (Henshaw et al., 2005; Okafor et al., 2018). Zhou et al. experimentally and numerically investigated the laminar flames of NH3/syngas/air and NH3/bio-syngas/air at elevated temperature (Zhou et al., 2021). Wang et al. experimentally and numerically investigated the laminar burning velocities of NH3 mixing with CH3OH and C2H5OH in premixed flames (Wang et al., 2021a). Figure 8 shows that the flame speeds decreased with the heat fraction of ammonia ENH3 increased and the flame speed were close to those of methane/air mixture when ENH3 reached 0.016. Obviously, the burning velocity decreased more sharply when ENH3 ranged from 0 to 0.1. The difference between the flame speed at ENH3 = 0.1 and ENH3 = 0.3 is about 8.8 cm/s, while the discrepancy is around 9.8 cm/s when the ENH3 increases from 0 to 0.1. Xiao et al. investigated the laminar burning velocity of NH3/CH4 mixtures with the ammonia fraction ranged from 0 to 100% (Xiao et al., 2017b). They also found that ammonia had a negative impact on flame propagation. Shu et al. studied the fundamental combustion characteristics of NH3/CH4/air mixtures by using expending spherical flames (Shu et al., 2021). They found that the laminar burning velocity varied almost linearly with the methane volume fraction and the H/OH radicals were very important for the propagation of flames under fuel lean conditions. Furthermore, the laminar burning velocity had a strong linear relationship with the maximum mole fraction of (H + OH) radicals under lean conditions when the pressure ranged from 1 to 15 atm and the methane volume fraction ranged from 0.30 to 0.70. The laminar burning velocity of ammonia/methane mixture under variable O2 and CO2 mole fractions of 0.35–0.40 and 0.45 to 0.65, respectively, was experimentally investigated by Liu et al. (2019b). They found that the maximum flame speed decreased from 35.6 to 25.6 cm/s when the CO2 mole fraction increased from 0.45 to 0.65, while the peak speed increased from 25.6 to 36.6 cm/s when the O2 concentration increased from 0.35 to 0.40. Through the simulation results by using HUST Mechanism, it can be observed that the burning velocity has linear correlations between CO2 and O2 concentration.
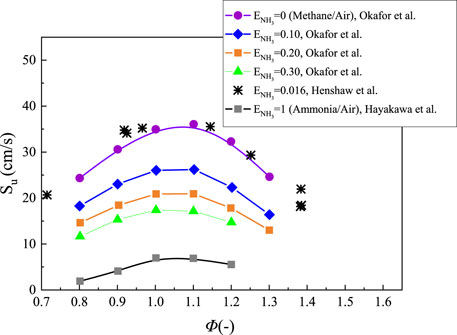
FIGURE 8. Laminar burning velocity of NH3 flame with a function of equivalence ratio at various ammonia addition in Methane/ammonia/air flames, replotted from (Okafor et al., 2018; Henshaw et al., 2005; Hayakawa et al., 2015a).
NOx Emissions
When ammonia is completely combusted, it only produces nitrogen and water without involving the production of NOx. However, NOx emission is relatively high in practical combustion, which is a main challenge of ammonia combustion. In order to reduce the production of NOx, some detailed mechanism of ammonia combustion is necessary. NOx is mainly composed of thermal NOx and fuel NOx. Thermal NO is usually produced by the oxidation of N2 at temperature up to 1800 K. The extended Zeldovich mechanism is widely used to describe the formation of thermal NO. The reactions of thermal NO have three important pathways: N2 + O=NO + N, N + O2 = NO + O, and N + OH = NO + H. The first reaction limits the reaction rate and usually take place when the temperature above 1,800 K. Therefore, controlling temperature is an effective way to reduce thermal NO production.
Fuel NOx emission is widely studied by many researchers. Miller et al. studied the reaction path of NH3 oxidation and proposed a kinetics including 22 species and 98 elementary reactions (shown as Figure 9, Millar and Bowman, 1989). Firstly, ammonia is converted to NH2 by the reaction with OH, which is the primary path under both fuel rich and lean conditions. It is also consumed by O to produce NH2 under fuel lean condition and is consumed by H under rich condition. The reason of this phenomenon is that rich burning flame needs more oxygen or oxygen atoms, which results in the proportion of H in O/H radicals is high when compared to fuel lean conditions (Chai et al., 2021). It is obvious that the reactions involving NHi (i = 0, 1, 2) have very significant impact on the formation and reduction of NO. And HNO intermediate make great contribution to NO formation via reacting with OH, NH2, and M. Lindstedt et al. also found that fuel NO is mainly produced by the reactions involving HNO (Lindstedt et al., 1994). For ammonia flames, HNO + M = NO + H + M reaction plays a key role and HNO + OH = NO + H2O reaction becomes important when the equivalence ratio less than 1. What’s more, for the conversion of NO, the reaction between NO and NH2 is the major path.
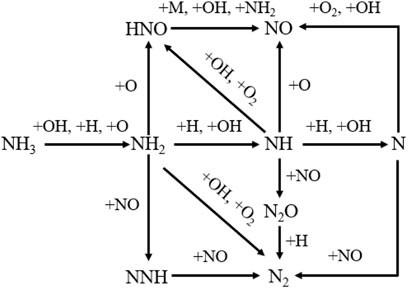
FIGURE 9. Diagram of ammonia oxidation pathway, replotted from (Millar and Bowman, 1989).
Dean et al. found that NNH dissociation plays an important role in the formation of N2 under fuel rich conditions, which mainly produced by N2H2 (Dean et al., 1984). The NHi radicals can be converted to N2 without including NO, which has a potential to reduce the formation of NO. They developed the NNH mechanism (Dean and Bozzelli, 2000). The reaction between NNH intermediate and O atom is the main path to generate NO, and the related reaction are shown as follow: NNH + O=NH + NO, NNH + O=N2O + H, and NNH + O=N2 + OH. Klippenstein et al. calculated the reaction rate constant of NNH by using an improved model based on the Miller mechanism (Miller and Glarborg, 1999; Klippenstein et al., 2011). They found that the rate constant of NNH + O=N2 + OH is over-predicted by Dean et al. and the NNH + O reaction is very fast.
Some factors like equivalence ratio and pressure are also very important for NOx emissions. The main component of NOx is NO. It was found that with an increase of the equivalence ratio, the mole fraction of NO in NH3/air flame firstly increased before the equivalence ratio reaches 0.9 and then rapidly decreased when the equivalence ratio is large than 0.9 (Kobayashi et al., 2019). The production of NO is extremely low after the equivalence ratio up to 1.3. Therefore, it seems that burning under fuel rich condition is an effective way to reduce NOx emission. Hayakawa et al. experimentally and numerically studied the characteristics of NOx formation in ammonia/air flames at different pressures and equivalence ratios (covering p = 1.0–3.0 atm and φ = 0.7–1.1) (Hayakawa et al., 2015b). The experiments were performed in a nozzle-type burner and the numerical simulation used the Tian mechanism (Tian et al., 2009). Results show that the NO concentration decreased with an increasing equivalence ratio at pressure of 1 atm, which is mainly due to surplus production of NHi (i = 0, 1, 2) under fuel rich conditions. Besides, with the increasing pressure, the concentration of NO decreased while the reaction rate of OH + H + M = H2O + M increased. Therefore, they proposed that H and OH radicals mentioned above limited the formation of NO.
In order to use pure ammonia in practical applicants, some researches were performed to provide a deep insight into the characteristic of NOx emission. Lee et al. proposed a combustion strategy in order to use pure ammonia in the internal combustion engine (Lee and Song, 2018). In this strategy, they injected the lean ammonia/air mixture during the intake process which was auto-ignited in the next step. This method can raise the temperature and pressure of the cylinder and it has a positive effect on ammonia spray combustion. Then they studied the mechanism of NO formation and found that NO emission in this engine largely depend on the main SOI variation. For instance, the maximum value of NO formation up to 8,500 ppm, while the reduction of NO emission can reach 5,460 ppm by changing the SOI timing. Kurata studied the emission characteristics of non-premixed NH3/air in gas turbine power generations (Kurata et al., 2017). It was found that the production of NOx and slip NH3 were strongly influenced by the combustor inlet temperature. The existence of NOx and slip NH3 at the mid-range of combustor inlet temperature implied that there is a possibility of NO reduction through selective non-catalytic reduction. They also developed a low-NOx NH3-air non-premixed combustor for actual gas-turbine operations and found that the NO production of this gas turbine power generation could be lowered to 337 ppm when O2 content reached 16% (Kurata et al., 2019). Okafor et al. designed a two-stage combustor for a micro gas turbine and found that NOx emission was around 42 ppm at initial pressure of 3 atm and initial temperature of 298K when the equivalence ratio of the primary stage reached 1.1 and the equivalence ratio of the secondary stage was 0.4 (Okafor et al., 2019). Somarathne et al. numerically investigated the NOx and NH3 emission of turbulent premixed ammonia/air flames in a gas turbine like combustor by using the large eddy simulation at elevated pressure up to 5 atm and various equivalence ratios (Somarathne et al., 2017). They found that NO production decreased when the initial pressure increased and the minimum value of NO and unburnt NH3 emission could reach 200 ppm at initial pressure of 5 atm and the equivalence ratio of 1.2. What’s more, the NO emission of ammonia/air flames with the secondary air injection could be lowered to 100 ppm at O2 content of 16%. Rocha et al. numerically studied the NOx emission characteristics of ammonia/air mixtures under the typical conditions of commercial gas turbines by using three modern stationary gas turbine concepts (Rocha et al., 2020). They found that rich-burn, quick-quench and lean-burn (RQL) concept and moderate or intense low oxygen dilution (MILD) concept are able to reach low NOx emission while the NOx emission by using lean-burn dry-low emissions (DLE) concept is high.
There are also some researches about the NOx emission of NH3/H2/air flames. Li et al. experimentally measured the NOx concentration of NH3/H2/air flames covering the NH3 concentration from 0.440 to 0.544 at the equivalence ratios from 1.00 to 1.25 (Li et al., 2014). They found that NOx emission decreased when the equivalence ratio increased from 1.00 to 1.25 under different NH3 contents. It can be observed that a more significant change occurs when the equivalence ratio increased from 1.00 to 1.10, while the change is small when the equivalence ratio increased from 1.10 to 1.25. As the NH3 concentration increase the production of NOx decrease, which due to the low flame temperature. The peak value of NOx content is 1,450 ppm at NH3 content of 0.500 and stoichiometric condition, while the maximum content is around 1,660 ppm when NH3 content of NH3/H2 mixture reaches 0.416 under stoichiometric condition. They also concluded that fuel NOx plays a significant role in NOx emission, whereas thermal NOx is negligible. Nozari et al. also studied the variation of the NOx emission with the equivalence ratio at elevated pressure of 17 atm and temperature of 673 K (Nozari, 2015). They found that the NOx concentration increased first and then decreased when the equivalence ratio ranged from 0.5 to 1.2 in all hydrogen content ranges. The maximum mole fraction of NOx occurred under fuel lean conditions, which is similar to that of ammonia/air flames. Wang et al. also found that the NO concentration in exhaust gas was non-monotonically changed with equivalence ratios and hydrogen mole ratio, because the competition between the effects by the reduction of N-atom and the enrichment of H/O radicals when hydrogen is added to ammonia flame (Wang et al., 2021b). Lee et al. also found that both NOx and N2O emissions are low in fuel rich condition when compared to those under lean conditions (Lee et al., 2010).
The influences of pressure and hydrogen content on NOx emissions are also significant. Xiao et al. numerically investigated the influence of pressure in NH3/H2 flames by using an improved mechanism based on the work performed by Mathieu et al. (Mathieu and Petersen, 2015; Xiao et al., 2017a). Results show that the concentration of NOx significantly decreased with an increasing pressure. NOx emission can be less than 5 ppm when the pressure reaches 10 atm and less than 1 ppm when the pressure up to 20 atm. Rocha et al. numerically investigated the variation of NOx emission with hydrogen addition ratio ranged from 0 to 1 by ten mechanisms for ammonia/hydrogen/air flames at 1 atm and 298 K (Rocha et al., 2019). Results show that with an increase of the H2 content, the NOx emission increased before the mole fraction of H2 reached 0.80 and then rapidly decreased when the mole fraction of H2 large than 0.80. They presumed that the presence of OH/O radicals at high temperature resulted in the increasing content of NOx before the H2 concentration reached 0.80, while the decreasing concentration of ammonia leads to the decrease of NOx when the mole fraction of H2 large than 0.80.
Lhuillier et al. experimentally investigated the NOx emission of NH3/H2/air mixtures in spark ignition engine at the intake temperature of 323 K and pressure of 1.2 atm (Lhuillier et al., 2020a). It was found that NOx production firstly increased and then decreased with an increased of the equivalence ratio. The peak values of NOx formation occurred at the equivalence ratio of 0.8–0.9 and the minimal NOx emission was found under rich conditions. What’s more, as the H2 concentration of NH3/H2/air mixtures increased, the emission increased. Very recently, Franco et al. also designed a laboratory combustor which had good performance in NOx emission (Franco et al., 2021). Valera-Medina et al. numerically and experimentally investigated the NOx emission of premixed 50%NH3/50%H2 mixtures under fuel lean condition in a swirl combustor and found that the pollution emission was high when compared with that under fuel rich condition (Valera-Medina et al., 2017). Then they studied characteristics of 70%NH3/30%H2 mixtures under gas turbine conditions through a numerical GT cycle model and found that NOx emission could as low as 50 ppm because the hot unburned ammonia was able to react with NOx (Valera-Medina et al., 2019). Hussein et al. found that injecting some NH3/H2 blend downstream the primary zone could significantly reduce the NOx emission which was a promising method to minimize the pollution emission (Hussein et al., 2019).
Recently, many researches have studied the NOx emission of CH4/NH3 flames. Among them, Tian mechanism is widely used to predict the NOx emission of CH4/NH3 mixtures, which has a satisfactory prediction of the experimental results (Tian et al., 2009). Tian et al. investigated the intermediates and products for ammonia/methane/air/Ar flames at 0.04 atm and φ = 1 by using tunable synchrotron vacuum ultraviolet photoionization and molecular-beam mass spectrometry (Tian et al., 2009). Results show that the formation of NO2 decreased while those of NO and N2 increased with the mole ratios of NH3/CH4 increasing from 0 to 1. They also developed a detailed mechanism including 84 species and 703 elementary reactions, which can predict the major combustion species and intermediates well (shown as Figure 10). The process of CO formation is described below. Firstly, methane is converted to CH3 by the reaction with OH and H. Then CH3 mainly reacts with O addition to generate CH2O and reacts with OH to form singlet CH2 which can transform to triplet CH2. CH2 is converted to CH by reacting with H and then CH transforms to HCO by reacting with O2. Besides, HCO also can be produced by the reaction of CH2O with OH. Finally, HCO is converted to CO and H2 by reacting with OH, O2, and H. However, NO is produced by the reaction of NH with O and the reaction of HNO with H, OH, and O. It seems that the addition of CH4 has no significant influence on the major formation and reduction of NO. Though the numerical simulation, it also can be found that four reactions including H + O2 = O + OH, NH2 + O = HNO + H, NH2 + NO = N2 + H2O, and NH + NO = N2O + H are important for the conversion of NO and N2.
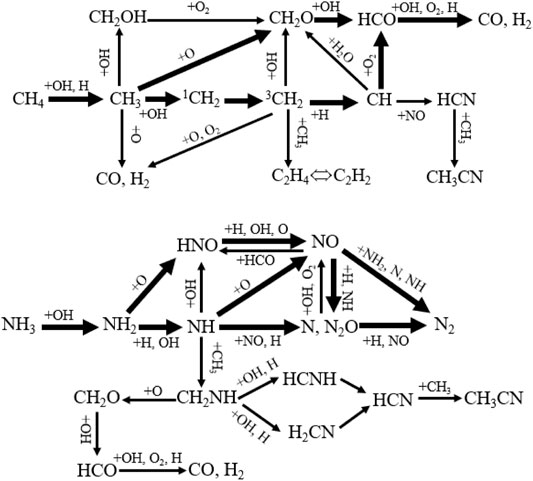
FIGURE 10. Diagram of ammonia/methane mixture oxidation pathway, replotted from (Tian et al., 2009).
Ramos et al. measured the NOx emissions from CH4/NH3 flames at NH3 content ranged from 0 to 0.7 and the equivalence ratio of 0.8, 0.9, and 1 by using a laboratory scale laminar flame burner (Filipe Ramos et al., 2019). They found that the production of NOx firstly increased and then slightly decreased with an increasing NH3 content and the maximum NOx emission occurred when the concentration of NH3 reached 0.50. Xiao et al. numerically studied the emission characteristics of CH4/NH3 mixtures at the ammonia ratio from 0 to 1 by using an improved model based on the Konnov mechanism and found the same tendency (Konnov, 2009; Xiao et al., 2017b). It was found that the addition of ammonia had a significant positive effect on NOx emission under high methane proportion mixtures while it had a negative effect under high ammonia concentration. The probable reason of this phenomenon is that H + NO = HNO inhibits the formation of NO at high ammonia concentration (Rocha et al., 2021). The equivalence ratio and initial pressure also has a strong impact on NOx emission. Ramos et al. found that the peak values of the NOx concentration are around 4,300, 3,600, and 2,800 ppm at the equivalence ratio of 1, 0.9, and 0.8, respectively, (Filipe Ramos et al., 2019). It is obvious that NOx emissions decrease with a decrease of the equivalence ratio. Xiao et al. also studied the influence of the equivalence ratio and found that the maximum NOx emission is presented at the equivalence ratio of 0.9 when the ammonia mole fraction ranged from 0.20 to 0.80 (Xiao et al., 2017b). Then they numerically studied the reaction involving NO by using Tian mechanism (Xiao et al., 2020). It was found that NH2+O=HNO + H reaction is the most promoting reaction at φ = 0.8, while H + O2 = O + OH is the most promoting reaction at φ = 1, 1.2. The most inhibiting reaction is NH2+NO = N2+H2O, NH + NO = N2O + H, NH2+NO = NNH + OH at φ = 0.8, 1 and 1.2, respectively. For NOx emission, the initial pressure plays an important role. The NO mole fraction decreased with the increasing pressure and the NO production was more sensitive to the change of the pressure than CO (Xiao et al., 2017b). What’s more, it was found that the formation of NO is mainly through the NH + OH = HNO + H reaction at high pressure (Valera-Medina et al., 2017). Zhang et al. investigated the emission characteristics of ammonia/air combustion in a model combustor with the addition of methane and hydrogen. They found NO and OH radicals showed a positive correlation, and the temperature had a secondary role on promoting NOx formation comparing with CH4/air flame (Zhang et al., 2021a). The thermal performance and NOx emission on a premixed methane/ammonia/flame at a micro-planar combustor were also investigated. Results showed that ammonia addition reduced CO2 emission, but increased NO emission increased. While N2O emission increased first and then decreased with increasing ammonia ratio. (Cai et al., 2020; Cai et al., 2021; Han et al., 2021; Sun et al., 2021). An et al. found that the OH and NO were closely correlated in premixed CH4/NH3/air flames as they were both strongly related to flame temperature. While N2O had a correlation with NH and HNO components (An et al., 2021), which is similar with the results by the same combustion group. (Zhang et al., 2020; Zhang et al., 2021b; Wei et al., 2021).
Recently, CH4/NH3 blends are also considered as a substitute fuel in practical applications. Liquefied natural gas is an alternative fuel and has been widely used in marine engines (Schinas and Butler, 2016). What’s more, it also can improve the poor combustion characteristics of pure ammonia. Therefore, Oh et al. investigated the pollution emission of ammonia mixed with methane and found that NOx emissions of CH4/NH3 blends in spark ignited engine rapidly increased before the split ratio of NH3 reached 40% and then slightly decreased when the split ratio large than 40% (Oh et al., 2021). Besides, the maximum NOx emission of this study was lower than 40 g/kWh. Xiao et al. compared the simulation results under gas turbine conditions by using Tian, Konnov, Mendiara, GRI 3.0 and ÅA mechanism and found that Tian mechanism performed best in NOx prediction (Xiao et al., 2017c, Tian et al., 2009, Mendiara and Glarborg, 2009, GRI, 2000, Åbo Akademi University, 2005). What’s more, they found that the oxidation of N and HNO played the most important role in the production of NO under gas turbine conditions. Li et al. also studied the NOx emission of CH4/NH3 mixtures under gas turbine conditions and recognized that NOx formation increased when the NH3 concentration increased, which is mainly due to the enhanced HNO pathway (Li et al., 2019b). Furthermore, they found that the availability of oxygen has significant influence on the production of NOx through HNO pathway and proposed a combustion system in which NOx production can as low as 30 ppm even when the NH3 concentration up to 40%. Okafor et al. used rich-lean combustion to control NOx emission and proposed the optimum equivalence ratio of the primary zone ranged from 1.30 to 1.35 (Okafor et al., 2020). This method can significantly reduce the formation of NOx, for instance, NOx emission can be lowered to 49ppm. Okafor et al. investigated the characteristics of liquid NH3 spray co-fired with CH4 and found that the minimum NOx emission occurred when the equivalence ratio reached 1.06 (Okafor et al., 2021a). They also studied the NOx emission characteristics of this kind of fuel in two-stage micro gas turbine combustors and recognized that the increased inlet temperature and decreased wall heat loss led to the reduction of NO and N2O (Okafor et al., 2021b).
Conclusions and Perspectives
This paper presents the burning velocity and pollutant emissions of NH3 flames, NH3/H2 flames and CH4/NH3 flames. At 1 atm and 298 K, the laminar burning velocities increase before the equivalence ratio reaches 1.1, and then decrease when the equivalence ratio is large than 1.1. The maximum value of laminar burning velocity is less than 10 cm/s, which is relatively low when compared with that of CH4/air flame. Under different pressure conditions, it can be found that the flame velocity decreases with an increase of the initial pressure. The influence of temperature is also studied. With the initial temperature increasing, the flame speed exponentially increases. In order to increase the laminar burning velocity of NH3/air flames, oxygen enriched combustion is proposed as a promising approach. The flame speed of NH3/air flames under oxygen enrichment conditions increases with an increase of the O2 concentration, but decrease with an increase of initial pressure under oxygen enrichment conditions. And the flame velocity increases with the increasing temperature, which is more sensitive to the change of temperature under lower O2 content. Besides, ammonia is usually burned with the additions of hydrogen and methane to improve the combustion characteristics. The addition of H2 or CH4 can significantly increase the flame speed.
NOx is composed of the thermal NOx and the fuel NOx, which is the major pollution of ammonia combustion. Thermal NOx is usually produced by the oxidation of N2 when the temperature reaches 1,800 K. The generation of the thermal NO is satisfactorily described by the extended Zeldovich mechanism. It can be reduced by controlling temperature. Fuel NOx is mainly generated by the oxidation of NH3. HNO intermediate plays an important role in NO formation by reacting with OH and NH2. It was found that burning under rich condition and high pressure can effectively reduce NOx emission. The effects of equivalence ratio and pressure in NH3/H2 flames are similar to those of NH3/air flames. The NOx production firstly increased with an increase of the H2 content before the H2 concentration reached 0.8, and then rapidly decreased after the mole fraction of H2 large than 0.8. The reaction pathways in the combustion of NH3/CH4 fuels are described by using Tian mechanism. It seems that the addition of CH4 has no significant influence on the major formation and reduction of NO. The NOx production is high under high ammonia content, high temperature and stoichiometric condition. What’s more, the concentration of CO2 also influences the formation of NOx. The high CO2 content has a positive impact on NOx production under fuel rich condition, while has a negative effect under stoichiometric and lean conditions.
Although the fundamental characteristics of ammonia-based flames has been widely studied, the practical application of this kind of fuel is still unsatisfying. Therefore, further studies are needed to overcome the problems, including low burning velocities and high NOx emission. For example, one of the main challenges in the investigation of ammonia-based fuel is that the numerical simulations by using mechanisms usually overpredict or underpredict when compared with those are obtained during the actual combustion processing. In order to fully understand the combustion characteristics of ammonia-based flames, developing an accurate mechanism is necessary. What’s more, for emission characteristics of CH4/NH3 mixtures, many researches focused on reducing NOx emission, and few studies on the carbon capture can be found. Therefore, it still need further studied.
Author Contributions
Conceptualization, JL, HH, and NK; literature review and resources, JL, SL, DC, RW, and LD; writing, JL and SL; review and editing, HH and NK; funding acquisition, JL and HH. All authors have read and agree to the published version of the article.
Funding
This work was supported by Leading Key Projects of Chinese Academy of Sciences (No.QYZDY-SSW-JSC038), Key Special Project for Introduced Talents Team of Southern Marine Science and Engineering Guangdong Laboratory (Guangzhou) (GML2019ZD0108), and Science and Technology Planning Project of Guangdong Province, China (No. 2017A050501046).
Conflict of Interest
The authors declare that the research was conducted in the absence of any commercial or financial relationships that could be construed as a potential conflict of interest.
Publisher’s Note
All claims expressed in this article are solely those of the authors and do not necessarily represent those of their affiliated organizations, or those of the publisher, the editors and the reviewers. Any product that may be evaluated in this article, or claim that may be made by its manufacturer, is not guaranteed or endorsed by the publisher.
Reference
Åbo Akademi University (2005). Åbo Akademi (ÅA) Scheme. Available at: http://www.abo.fi/fakultet/ookforskkinetschem (Accessed Oct 5, 2020).
Afif, A., Radenahmad, N., Cheok, Q., Shams, S., Kim, J. H., and Azad, A. K. (2016). Ammonia-fed Fuel Cells: a Comprehensive Review. Renew. Sustain. Energ. Rev. 60, 822–835. doi:10.1016/j.rser.2016.01.120
An, Z., Zhang, M., Zhang, W., Mao, R., Wei, X., Wang, J., et al. (2021). Emission Prediction and Analysis on CH4/NH3/air Swirl Flames with LES-FGM Method. Fuel 304, 121370. doi:10.1016/j.fuel.2021.121370
Andersson, J., and Lundgren, J. (2014). Techno-economic Analysis of Ammonia Production via Integrated Biomass Gasification. Appl. Energ. 130, 484–490. doi:10.1016/j.apenergy.2014.02.029
Astbury, G. R. (2008). A Review of the Properties and Hazards of Some Alternative Fuels. Process Saf. Environ. Prot. 86, 397–414. doi:10.1016/j.psep.2008.05.001
Barbas, M., Costa, M., Vranckx, S., and Fernandes, R. X. (2015). Experimental and Chemical Kinetic Study of CO and NO Formation in Oxy-Methane Premixed Laminar Flames Doped with NH3. Combust. Flame 162, 1294–1303. doi:10.1016/j.combustflame.2014.10.020
Bell, J. B., Day, M. S., Grcar, J. F., Bessler, W. G., Schulz, C., Glarborg, P., et al. (2002). Detailed Modeling and Laser-Induced Fluorescence Imaging of Nitric Oxide in a NH3-seeded Non-premixed Methane/air Flame. Proc. Combust. Inst. 29, 2195–2202. doi:10.1016/s1540-7489(02)80267-x
Cai, T., Zhao, D., Wang, B., Li, J., and Guan, Y. (2020). NO Emission and thermal Performances Studies on Premixed Ammonia-Oxygen Combustion in a CO2-free Micro-planar Combustor. Fuel 280, 118554. doi:10.1016/j.fuel.2020.118554
Cai, T., Zhao, D., Li, X., Shi, B., and Li, J. (2021). Mitigating NO Emissions from an Ammonia-Fueled Micro-power System with a Perforated Plate Implemented. J. Hazard. Mater. 401, 123848. doi:10.1016/j.jhazmat.2020.123848
CDC (1994). Immediately Dangerous to Life or Health Concentrations. Centers for Disease Control and Prevention. Available at: https://www.cdc.gov/niosh/idlh/7664417.html (Accessed Dec 24, 2020).
Chai, W. S., Bao, Y., Jin, P., Tang, G., and Zhou, L. (2021). A Review on Ammonia, Ammonia-Hydrogen and Ammonia-Methane Fuels. Renew. Sustain. Energ. Rev. 147, 111254. doi:10.1016/j.rser.2021.111254
Choi, S., Lee, S., and Kwon, O. C. (2015). Extinction Limits and Structure of Counterflow Nonpremixed Hydrogen-Doped Ammonia/air Flames at Elevated Temperatures. Energy 85, 503–510. doi:10.1016/j.energy.2015.03.061
Cohen, L. (1955). Burning Velocities of Ammonia in Air and in Oxygen. Fuel 34, S123–S127. doi:10.1002/j.2164-4918.1955.tb01284.x
da Rocha, R. C., Costa, M., and Bai, X.-S. (2019). Chemical Kinetic Modelling of Ammonia/hydrogen/air Ignition, Premixed Flame Propagation and NO Emission. Fuel 246, 24–33. doi:10.1016/j.fuel.2019.02.102
Dean, A. M., and Bozzelli, J. W. (2000). “Combustion Chemistry of Nitrogen,” in Gas-Phase Combustion Chemistry (New York: Springer), 125–341. doi:10.1007/978-1-4612-1310-9_2
Dean, A. M., Chou, M.-S., and Stern, D. (1984). Kinetics of Rich Ammonia Flames. Int. J. Chem. Kinet. 16, 633–653. doi:10.1002/kin.550160603
Dimitriou, P., and Javaid, R. (2020). A Review of Ammonia as a Compression Ignition Engine Fuel. Int. J. Hydrogen Energ. 45, 7098–7118. doi:10.1016/j.ijhydene.2019.12.209
Duynslaegher, C., Jeanmart, H., and Vandooren, J. (2009). Flame Structure Studies of Premixed Ammonia/hydrogen/oxygen/argon Flames: Experimental and Numerical Investigation. Proc. Combust. Inst. 32, 1277–1284. doi:10.1016/j.proci.2008.06.036
Duynslaegher, C., Jeanmart, H., and Vandooren, J. (2010). Ammonia Combustion at Elevated Pressure and Temperature Conditions. Fuel 89, 3540–3545. doi:10.1016/j.fuel.2010.06.008
Duynslaegher, C., Contino, F., Vandooren, J., and Jeanmart, H. (2012). Modeling of Ammonia Combustion at Low Pressure. Combust. Flame 159, 2799–2805. doi:10.1016/j.combustflame.2012.06.003
Filipe Ramos, C., Rocha, R. C., Oliveira, P. M. R., Costa, M., and Bai, X.-S. (2019). Experimental and Kinetic Modelling Investigation on NO, CO and NH3 Emissions from NH3/CH4/air Premixed Flames. Fuel 254, 115693. doi:10.1016/j.fuel.2019.115693
Franco, M. C., Rocha, R. C., Costa, M., and Yehia, M. (2021). Characteristics of NH3/H2/air Flames in a Combustor Fired by a Swirl and bluff-body Stabilized Burner. Proc. Combust. Inst. 38, 5129–5138. doi:10.1016/j.proci.2020.06.141
Frenklach, M., Bowman, T., and Smith, G. (2000). GRI-Mech 3.0. Available at: http://www.me.berkeley.edu/gri-mech/index.html (Accessed Jan 7, 2021).
Gill, S. S., Chatha, G. S., Tsolakis, A., Golunski, S. E., and York, A. P. E. (2012). Assessing the Effects of Partially Decarbonising a Diesel Engine by Co-fuelling with Dissociated Ammonia. Int. J. Hydrogen Energ. 37, 6074–6083. doi:10.1016/j.ijhydene.2011.12.137
Gross, C. W., and Kong, S.-C. (2013). Performance Characteristics of a Compression-Ignition Engine Using Direct-Injection Ammonia-DME Mixtures. Fuel 103, 1069–1079. doi:10.1016/j.fuel.2012.08.026
Grossale, A., Nova, I., and Tronconi, E. (2009). Ammonia Blocking of the "Fast SCR" Reactivity over a Commercial Fe-Zeolite Catalyst for Diesel Exhaust Aftertreatment. J. Catal. 265, 141–147. doi:10.1016/j.jcat.2009.04.014
Habgood, D. C. C., Hoadley, A. F. A., and Zhang, L. (2015). Techno-economic Analysis of Gasification Routes for Ammonia Production from Victorian Brown Coal. Chem. Eng. Res. Des. 102, 57–68. doi:10.1016/j.cherd.2015.06.008
Han, X., Wang, Z., Costa, M., Sun, Z., He, Y., and Cen, K. (2019). Experimental and Kinetic Modeling Study of Laminar Burning Velocities of NH3/air, NH3/H2/air, NH3/CO/air and NH3/CH4/air Premixed Flames. Combust. Flame 206, 214–226. doi:10.1016/j.combustflame.2019.05.003
Han, X., Wang, Z., He, Y., Liu, Y., Zhu, Y., and Konnov, A. A. (2020). The Temperature Dependence of the Laminar Burning Velocity and Superadiabatic Flame Temperature Phenomenon for NH3/air Flames. Combust. Flame 217, 314–320. doi:10.1016/j.combustflame.2020.04.013
Han, L., Li, J., Zhao, D., Xi, Y., Gu, X., and Wang, N. (2021). Effect Analysis on Energy Conversion Enhancement and NOx Emission Reduction of Ammonia/hydrogen Fuelled Wavy Micro-combustor for Micro-thermophotovoltaic Application. Fuel 289, 119755. doi:10.1016/j.fuel.2020.119755
Hayakawa, A., Goto, T., Mimoto, R., Arakawa, Y., Kudo, T., and Kobayashi, H. (2015a). Laminar Burning Velocity and Markstein Length of Ammonia/air Premixed Flames at Various Pressures. Fuel 159, 98–106. doi:10.1016/j.fuel.2015.06.070
Hayakawa, A., Goto, T., Mimoto, R., Kudo, T., and Kobayashi, H. (2015b). NO Formation/reduction Mechanisms of Ammonia/air Premixed Flames at Various Equivalence Ratios and Pressures. Mech. Eng. J. 2, 14–00402. doi:10.1299/mej.14-00402
Henshaw, P. F., D'Andrea, T., Mann, K. R. C., and Ting, D. S.-K. (2005). Premixed Ammonia-Methane-Air Combustion. Combust. Sci. Techn. 177, 2151–2170. doi:10.1080/00102200500240695
Hussein, N. A., Valera-Medina, A., and Alsaegh, A. S. (2019). Ammonia- Hydrogen Combustion in a Swirl Burner with Reduction of NOx Emissions. Energ. Proced. 158, 2305–2310. doi:10.1016/j.egypro.2019.01.265
Ichikawa, A., Hayakawa, A., Kitagawa, Y., Kunkuma Amila Somarathne, K. D., Kudo, T., and Kobayashi, H. (2015). Laminar Burning Velocity and Markstein Length of Ammonia/hydrogen/air Premixed Flames at Elevated Pressures. Int. J. Hydrogen Energ. 40, 9570–9578. doi:10.1016/j.ijhydene.2015.04.024
IEA (2021). Global Energy Review 2021. Paris: International Energy Agency. Available at: https://www.iea.org/reports/global-energy-review-2021.
Joo, J. M., Lee, S., and Kwon, O. C. (2012). Effects of Ammonia Substitution on Combustion Stability Limits and NOx Emissions of Premixed Hydrogen-Air Flames. Int. J. Hydrogen Energ. 37, 6933–6941. doi:10.1016/j.ijhydene.2012.01.059
Kim, H. K., Ku, J. W., Ahn, Y. J., Kim, Y. H., and Kwon, O. C. (2021). Effects of O2 Enrichment on NH3/air Flame Propagation and Emissions. Int. J. Hydrogen Energ. 46 (46), 23916–23926. doi:10.1016/j.ijhydene.2021.04.154
Klerke, A., Christensen, C. H., Nørskov, J. K., and Vegge, T. (2008). Ammonia for Hydrogen Storage: Challenges and Opportunities. J. Mater. Chem. 18, 2304–2310. doi:10.1039/b720020j
Klippenstein, S. J., Harding, L. B., Glarborg, P., and Miller, J. A. (2011). The Role of NNH in NO Formation and Control. Combust. Flame 158, 774–789. doi:10.1016/j.combustflame.2010.12.013
Kobayashi, H., Hayakawa, A., Somarathne, K. D. K. A., and Okafor, E. C. (2019). Science and Technology of Ammonia Combustion. Proc. Combust. Inst. 37, 109–133. doi:10.1016/j.proci.2018.09.029
Konnov, A. A., Dyakov, I. V., and De Ruyck, J. (2006). Probe Sampling Measurements of No in Ch4+O2+N2 Flames Doped with NH3. Combust. Sci. Techn. 178 (6), 1143–1164. doi:10.1080/00102200500296788
Konnov, A. A. (2009). Implementation of the NCN Pathway of Prompt-NO Formation in the Detailed Reaction Mechanism. Combust. Flame 156, 2093–2105. doi:10.1016/j.combustflame.2009.03.016
Kumar, P., and Meyer, T. R. (2013). Experimental and Modeling Study of Chemical-Kinetics Mechanisms for H2-NH3-air Mixtures in Laminar Premixed Jet Flames. Fuel 108, 166–176. doi:10.1016/j.fuel.2012.06.103
Kurata, O., Iki, N., Matsunuma, T., Inoue, T., Tsujimura, T., Furutani, H., et al. (2017). Performances and Emission Characteristics of NH3-air and NH3CH4-air Combustion Gas-Turbine Power Generations. Proc. Combust. Inst. 36, 3351–3359. doi:10.1016/j.proci.2016.07.088
Kurata, O., Iki, N., Inoue, T., Matsunuma, T., Tsujimura, T., Furutani, H., et al. (2019). Development of a Wide Range-Operable, Rich-Lean Low-NOx Combustor for NH3 Fuel Gas-Turbine Power Generation. Proc. Combust. Inst. 37, 4587–4595. doi:10.1016/j.proci.2018.09.012
Kwon, O. C., and Faeth, G. M. (2001). Flame/stretch Interactions of Premixed Hydrogen-Fueled Flames: Measurements and Predictions. Combust. Flame 124, 590–610. doi:10.1016/s0010-2180(00)00229-7
Lan, R., Irvine, J. T. S., and Tao, S. (2012). Ammonia and Related Chemicals as Potential Indirect Hydrogen Storage Materials. Int. J. Hydrogen Energ. 37, 1482–1494. doi:10.1016/j.ijhydene.2011.10.004
Lee, D., and Song, H. H. (2018). Development of Combustion Strategy for the Internal Combustion Engine Fueled by Ammonia and its Operating Characteristics. J. Mech. Sci. Technol. 32 (4), 1905–1925. doi:10.1007/s12206-018-0347-x
Lee, J. H., Kim, J. H., Park, J. H., and Kwon, O. C. (2010). Studies on Properties of Laminar Premixed Hydrogen-Added Ammonia/air Flames for Hydrogen Production. Int. J. Hydrogen Energ. 35 (3), 1054–1064. doi:10.1016/j.ijhydene.2009.11.071
Lhuillier, C., Brequigny, P., Contino, F., and Mounaïm-Rousselle, C. (2020a). Experimental Study on Ammonia/hydrogen/air Combustion in Spark Ignition Engine Conditions. Fuel 269, 117448. doi:10.1016/j.fuel.2020.117448
Lhuillier, C., Brequigny, P., Lamoureux, N., Contino, F., and Mounaïm-Rousselle, C. (2020b). Experimental Investigation on Laminar Burning Velocities of Ammonia/hydrogen/air Mixtures at Elevated Temperatures. Fuel 263, 116653. doi:10.1016/j.fuel.2019.116653
Li, J., Huang, H., Kobayashi, N., He, Z., and Nagai, Y. (2014). Study on Using Hydrogen and Ammonia as Fuels: Combustion Characteristics and NOx formation. Int. J. Energ. Res. 38, 1214–1223. doi:10.1002/er.3141
Li, J., Huang, H., Kobayashi, N., He, Z., Osaka, Y., and Zeng, T. (2015). Numerical Study on Effect of Oxygen Content in Combustion Air on Ammonia Combustion. Energy 93, 2053–2068. doi:10.1016/j.energy.2015.10.060
Li, J., Huang, H., Yuan, H., Zeng, T., Yagami, M., and Kobayashi, N. (2016a). Modelling of Ammonia Combustion Characteristics at Preheating Combustion: NO Formation Analysis. IJGW 10, 230–241. doi:10.1504/ijgw.2016.077915
Li, J., Huang, H., Kobayashi, N., He, Z., Osaka, Y., and Zeng, T. (2016b). Research on Combustion and Emission Characteristics of Ammonia under Preheating Conditions. J. Chem. Eng. Jpn. 49, 641–648. doi:10.1252/jcej.15we075
Li, J., Huang, H., Kobayashi, N., Wang, C., and Yuan, H. (2017). Numerical Study on Laminar Burning Velocity and Ignition Delay Time of Ammonia Flame with Hydrogen Addition. Energy 126, 796–809. doi:10.1016/j.energy.2017.03.085
Li, J., Huang, H., Deng, L., He, Z., Osaka, Y., and Kobayashi, N. (2019a). Effect of Hydrogen Addition on Combustion and Heat Release Characteristics of Ammonia Flame. Energy 175, 604–617. doi:10.1016/j.energy.2019.03.075
Li, S., Zhang, S., Zhou, H., and Ren, Z. (2019b). Analysis of Air-Staged Combustion of NH3/CH4 Mixture with Low NOx Emission at Gas Turbine Conditions in Model Combustors. Fuel 237, 50–59. doi:10.1016/j.fuel.2018.09.131
Lindstedt, R. P., Lockwood, F. C., and Selim, M. A. (1994). Detailed Kinetic Modelling of Chemistry and Temperature Effects on Ammonia Oxidation. Combust. Sci. Techn. 99, 253–276. doi:10.1080/00102209408935436
Liu, Q., Chen, X., Huang, J., Shen, Y., Zhang, Y., and Liu, Z. (2019a). The Characteristics of Flame Propagation in Ammonia/oxygen Mixtures. J. Hazard. Mater. 363, 187–196. doi:10.1016/j.jhazmat.2018.09.073
Liu, S., Zou, C., Song, Y., Cheng, S., and Lin, Q. (2019b). Experimental and Numerical Study of Laminar Flame Speeds of CH4/NH3 Mixtures under Oxy-Fuel Combustion. Energy 175, 250–258. doi:10.1016/j.energy.2019.03.040
Mathieu, O., and Petersen, E. L. (2015). Experimental and Modeling Study on the High-Temperature Oxidation of Ammonia and Related NOx Chemistry. Combust. Flame 162 (3), 554–570. doi:10.1016/j.combustflame.2014.08.022
Mei, B., Zhang, X., Ma, S., Cui, M., Guo, H., Cao, Z., et al. (2019). Experimental and Kinetic Modeling Investigation on the Laminar Flame Propagation of Ammonia under Oxygen Enrichment and Elevated Pressure Conditions. Combust. Flame 210, 236–246. doi:10.1016/j.combustflame.2019.08.033
Mei, B., Zhang, J., Shi, X., Xi, Z., and Li, Y. (2021). Enhancement of Ammonia Combustion with Partial Fuel Cracking Strategy: Laminar Flame Propagation and Kinetic Modeling Investigation of NH3/H2/N2/air Mixtures up to 10 Atm. Combust. Flame 231, 111472. doi:10.1016/j.combustflame.2021.111472
Mendiara, T., and Glarborg, P. (2009). Ammonia Chemistry in Oxy-Fuel Combustion of Methane. Combust. Flame 156, 1937–1949. doi:10.1016/j.combustflame.2009.07.006
Metghalchi, M., and Keck, J. C. (1982). Burning Velocities of Mixtures of Air with Methanol, Isooctane, and Indolene at High Pressure and Temperature. Combust. Flame 48, 191–210. doi:10.1016/0010-2180(82)90127-4
Miller, J. A., and Bowman, C. T. (1989). Mechanism and Modeling of Nitrogen Chemistry in Combustion. Prog. Energ. Combust. Sci. 15, 287–338. doi:10.1016/0360-1285(89)90017-8
Miller, J. A., and Glarborg, P. (1999). Modeling the thermal De-NOx Process: Closing in on a Final Solution. Int. J. Chem. Kinet. 31, 757–765. doi:10.1002/(sici)1097-4601(1999)31:11<757:aid-jck1>3.0.co;2-v
Miller, J. A., Smooke, M. D., Green, R. M., and Kee, R. J. (1983). Kinetic Modeling of the Oxidation of Ammonia in Flames. Combust. Sci. Techn. 34, 149–176. doi:10.1080/00102208308923691
Mørch, C. S., Bjerre, A., Gøttrup, M. P., Sorenson, S. C., and Schramm, J. (2011). Ammonia/hydrogen Mixtures in an SI-Engine: Engine Performance and Analysis of a Proposed Fuel System. Fuel 90, 854–864.
NCBI (2021). PubChem-Ammonia. National Center for Biotechnology Information. Available at: https://pubchem.ncbi.nlm.nih.gov/compound/222 (Accessed May 15, 2021).
Nozari, H., and Karabeyoğlu, A. (2015). Numerical Study of Combustion Characteristics of Ammonia as a Renewable Fuel and Establishment of Reduced Reaction Mechanisms. Fuel 159, 223–233. doi:10.1016/j.fuel.2015.06.075
Oh, S., Park, C., KimKim, S. Y., Kim, Y., Choi, Y., and Kim, C. (2021). Natural Gas-Ammonia Dual-Fuel Combustion in Spark-Ignited Engine with Various Air-Fuel Ratios and Split Ratios of Ammonia under Part Load Condition. Fuel 290, 120095. doi:10.1016/j.fuel.2020.120095
Okafor, E. C., Naito, Y., Colson, S., Ichikawa, A., Kudo, T., Hayakawa, A., et al. (2018). Experimental and Numerical Study of the Laminar Burning Velocity of CH4-NH3-air Premixed Flames. Combust. Flame 187, 185–198. doi:10.1016/j.combustflame.2017.09.002
Okafor, E. C., Somarathne, K. D. K. A., Hayakawa, A., Kudo, T., Kurata, O., Iki, N., et al. (2019). Towards the Development of an Efficient Low-NOx Ammonia Combustor for a Micro Gas Turbine. Proc. Combust. Inst. 37, 4597–4606. doi:10.1016/j.proci.2018.07.083
Okafor, E. C., Somarathne, K. D. K. A., Ratthanan, R., Hayakawa, A., Kudo, T., Kurata, O., et al. (2020). Control of NOx and Other Emissions in Micro Gas Turbine Combustors Fuelled with Mixtures of Methane and Ammonia. Combust. Flame 211, 406–416. doi:10.1016/j.combustflame.2019.10.012
Okafor, E. C., Kurata, O., Yamashita, H., Inoue, T., Tsujimura, T., Iki, N., et al. (2021a). Liquid Ammonia spray Combustion in Two-Stage Micro Gas Turbine Combustors at 0.25 MPa; Relevance of Combustion Enhancement to Flame Stability and NOx Control. Appl. Energ. Combust. Sci. 7, 100038. doi:10.1016/j.jaecs.2021.100038
Okafor, E. C., Yamashita, H., Hayakawa, A., Somarathne, K. D. K. A., Kudo, T., Tsujimura, T., et al. (2021b). Flame Stability and Emissions Characteristics of Liquid Ammonia spray Co-fired with Methane in a Single Stage Swirl Combustor. Fuel 287, 119433. doi:10.1016/j.fuel.2020.119433
Otomo, J., Koshi, M., Mitsumori, T., Iwasaki, H., and Yamada, K. (2018). Chemical Kinetic Modeling of Ammonia Oxidation with Improved Reaction Mechanism for Ammonia/air and Ammonia/hydrogen/air Combustion. Int. J. Hydrogen Energ. 43, 3004–3014. doi:10.1016/j.ijhydene.2017.12.066
Pfahl, U. J., Ross, M. C., Shepherd, J. E., Pasamehmetoglu, K. O., and Unal, C. (2000). Flammability Limits, Ignition Energy, and Flame Speeds in H2-CH4-NH3-N2O-O2-N2 mixtures. Combust. Flame 123, 140–158.
Reiter, A. J., and Kong, S.-C. (2011). Combustion and Emissions Characteristics of Compression-Ignition Engine Using Dual Ammonia-Diesel Fuel. Fuel 90, 87–97. doi:10.1016/j.fuel.2010.07.055
Rocha, R. C., Costa, M., and Bai, X. S. (2020). Combustion and Emission Characteristics of Ammonia under Conditions Relevant to Modern Gas Turbines. Combust. Sci. Technol., 1–20. doi:10.1080/00102202.2020.1748018
Rocha, R. C., Zhong, S., Xu, L., Bai, X.-S., Costa, M., Cai, X., et al. (2021). Structure and Laminar Flame Speed of an Ammonia/methane/air Premixed Flame under Varying Pressure and Equivalence Ratio. Energy Fuels 35 (9), 7179–7192. doi:10.1021/acs.energyfuels.0c03520
Ronney, P. D. (1988). Effect of Chemistry and Transport Properties on Near-Limit Flames at Microgravity. Combust. Sci. Techn. 59, 123–141. doi:10.1080/00102208808947092
Schinas, O., and Butler, M. (2016). Feasibility and Commercial Considerations of LNG-Fueled Ships. Ocean Eng. 122, 84–96. doi:10.1016/j.oceaneng.2016.04.031
Shrestha, K. P., Lhuillier, C., Barbosa, A. A., Brequigny, P., Contino, F., Mounaïm-Rousselle, C., et al. (2021). An Experimental and Modeling Study of Ammonia with Enriched Oxygen Content and Ammonia/hydrogen Laminar Flame Speed at Elevated Pressure and Temperature. Proc. Combust. Inst. 38, 2163–2174. doi:10.1016/j.proci.2020.06.197
Shu, T., Xue, Y., Zhou, Z., and Ren, Z. (2021). An Experimental Study of Laminar Ammonia/methane/air Premixed Flames Using Expanding Spherical Flames. Fuel 290, 120003. doi:10.1016/j.fuel.2020.120003
Skreiberg, Ø., Kilpinen, P., and Glarborg, P. (2004). Ammonia Chemistry below 1400 K under Fuel-Rich Conditions in a Flow Reactor. Combust. Flame 136, 501–518. doi:10.1016/j.combustflame.2003.12.008
Somarathne, K. D. K. A., Hatakeyama, S., Hayakawa, A., and Kobayashi, H. (2017). Numerical Study of a Low Emission Gas Turbine like Combustor for Turbulent Ammonia/air Premixed Swirl Flames with a Secondary Air Injection at High Pressure. Int. J. Hydrogen Energ. 42, 27388–27399. doi:10.1016/j.ijhydene.2017.09.089
Sullivan, N., Jensen, A., Glarborg, P., Day, M. S., Grcar, J. F., Bell, J. B., et al. (2002). Ammonia Conversion and NOx Formation in Laminar Coflowing Nonpremixed Methane-Air Flames. Combust. Flame 131, 285–298. doi:10.1016/s0010-2180(02)00413-3
Sun, Y., Cai, T., and Zhao, D. (2021). Thermal Performance and NOx Emission Characteristics Studies on a Premixed Methane-Ammonia-Fueled Micro-planar Combustor. Fuel 291, 120190. doi:10.1016/j.fuel.2021.120190
Takeishi, H., Hayashi, J., Kono, S., Arita, W., Iino, K., and Akamatsu, F. (2015). Characteristics of ammonia/N2/O2 Laminar Flame in Oxygen-Enriched Air Condition. Trans. JSME 81, 14–00423. doi:10.1299/transjsme.14-00423
Takizawa, K., Takahashi, A., Tokuhashi, K., Kondo, S., and Sekiya, A. (2008). Burning Velocity Measurements of Nitrogen-Containing Compounds. J. Hazard. Mater. 155, 144–152. doi:10.1016/j.jhazmat.2007.11.089
Tian, Z., Li, Y., Zhang, L., Glarborg, P., and Qi, F. (2009). An Experimental and Kinetic Modeling Study of Premixed NH3/CH4/O2/Ar Flames at Low Pressure. Combust. Flame 156, 1413–1426. doi:10.1016/j.combustflame.2009.03.005
Um, D. H., Joo, J. M., Lee, S., and Kwon, O. C. (2013). Combustion Stability Limits and NOx Emissions of Nonpremixed Ammonia-Substituted Hydrogen-Air Flames. Int. J. Hydrogen Energ. 38, 14854–14865. doi:10.1016/j.ijhydene.2013.08.140
USGS (2021). Nitrogen Statistics and Information. U. S. Geological Survey. Available at: https://www.usgs.gov/centers/nmic/nitrogen-statistics-and-information (Accessed May 20, 2021).
Valera-Medina, A., Pugh, D. G., Marsh, P., Bulat, G., and Bowen, P. (2017). Preliminary Study on Lean Premixed Combustion of Ammonia-Hydrogen for Swirling Gas Turbine Combustors. Int. J. Hydrogen Energ. 42, 24495–24503. doi:10.1016/j.ijhydene.2017.08.028
Valera-Medina, A., Xiao, H., Owen-Jones, M., David, W. I. F., and Bowen, P. J. (2018). Ammonia for Power. Prog. Energ. Combust. Sci. 69, 63–102. doi:10.1016/j.pecs.2018.07.001
Valera-Medina, A., Gutesa, M., Xiao, H., Pugh, D., Giles, A., Goktepe, B., et al. (2019). Premixed Ammonia/hydrogen Swirl Combustion under Rich Fuel Conditions for Gas Turbines Operation. Int. J. Hydrogen Energ. 44, 8615–8626. doi:10.1016/j.ijhydene.2019.02.041
Wang, F., Zhou, Z., Dai, Z., Gong, X., Yu, G., Liu, H., et al. (2007). Development and Demonstration Plant Operation of an Opposed Multi-Burner Coal-Water Slurry Gasification Technology. Front. Energ. Power Eng. China 1, 251–258. doi:10.1007/s11708-007-0035-5
Wang, D., Ji, C., Wang, Z., Wang, S., Zhang, T., and Yang, J. (2020). Measurement of Oxy-Ammonia Laminar Burning Velocity at normal and Elevated Temperatures. Fuel 279, 118425. doi:10.1016/j.fuel.2020.118425
Wang, D., Ji, C., Wang, S., Yang, J., and Wang, Z. (2021a). Numerical Study of the Premixed Ammonia-Hydrogen Combustion under Engine-Relevant Conditions. Int. J. Hydrogen Energ. 46, 2667–2683. doi:10.1016/j.ijhydene.2020.10.045
Wang, Z., Han, X., He, Y., Zhu, R., Zhu, Y., Zhou, Z., et al. (2021b). Experimental and Kinetic Study on the Laminar Burning Velocities of NH3 Mixing with CH3OH and C2H5OH in Premixed Flames. Combust. Flame 229, 111392. doi:10.1016/j.combustflame.2021.02.038
Wei, X., Zhang, M., An, Z., Wang, J., Huang, Z., and Tan, H. (2021). Large Eddy Simulation on Flame Topologies and the Blow-Off Characteristics of Ammonia/air Flame in a Model Gas Turbine Combustor. Fuel 298, 120846. doi:10.1016/j.fuel.2021.120846
Williams, W. R., Zhao, J., and Schmidt, L. D. (1991). Ignition and Extinction of Surface and Homogeneous Oxidation of NH3 and CH4. AIChE J. 37, 641–649. doi:10.1002/aic.690370502
Wiseman, S., Rieth, M., Gruber, A., Dawson, J. R., and Chen, J. H. (2021). A Comparison of the Blow-Out Behavior of Turbulent Premixed Ammonia/hydrogen/nitrogen-Air and Methane-Air Flames. Proc. Combust. Inst. 38, 2869–2876. doi:10.1016/j.proci.2020.07.011
Xiao, H., Valera-Medina, A., and Bowen, P. J. (2017a). Modeling Combustion of Ammonia/Hydrogen Fuel Blends under Gas Turbine Conditions. Energy Fuels 31 (8), 8631–8642. doi:10.1021/acs.energyfuels.7b00709
Xiao, H., Valera-Medina, A., and Bowen, P. J. (2017b). Study on Premixed Combustion Characteristics of Co-firing Ammonia/methane Fuels. Energy 140, 125–135. doi:10.1016/j.energy.2017.08.077
Xiao, H., Valera-Medina, A., Marsh, R., and Bowen, P. J. (2017c). Numerical Study Assessing Various Ammonia/methane Reaction Models for Use under Gas Turbine Conditions. Fuel 196, 344–351. doi:10.1016/j.fuel.2017.01.095
Xiao, H., Lai, S., Valera-Medina, A., Li, J., Liu, J., and Fu, H. (2020). Study on Counterflow Premixed Flames Using High Concentration Ammonia Mixed with Methane. Fuel 275, 117902. doi:10.1016/j.fuel.2020.117902
Xu, L., McCabe, R. W., and Hammerle, R. H. (2002). NOx Self-Inhibition in Selective Catalytic Reduction with Urea (Ammonia) over a Cu-Zeolite Catalyst in Diesel Exhaust. Appl. Catal. B: Environ. 39, 51–63. doi:10.1016/s0926-3373(02)00074-7
Yang, G., Guo, H., Kang, Z., Zhao, L., Feng, S., Jiao, F., et al. (2019). Green Hydrogen Separation from Nitrogen by Mixed‐Matrix Membranes Consisting of Nanosized Sodalite Crystals. ChemSusChem 12, 4529–4537. doi:10.1002/cssc.201802577
Yun, B. K., and Kim, M. Y. (2013). Modeling the Selective Catalytic Reduction of NOx by Ammonia over a Vanadia-Based Catalyst from Heavy Duty Diesel Exhaust Gases. Appl. Therm. Eng. 50, 152–158. doi:10.1016/j.applthermaleng.2012.05.039
Zakaznov, V. F., Kursheva, L. A., and Fedina, Z. I. (1978). Determination of Normal Flame Velocity and Critical Diameter of Flame Extinction in Ammonia-Air Mixture. Combust. Explos. 14, 710–713.
Zamfirescu, C., and Dincer, I. (2008). Using Ammonia as a Sustainable Fuel. J. Power Sourc. 185, 459–465. doi:10.1016/j.jpowsour.2008.02.097
Zamfirescu, C., and Dincer, I. (2009). Ammonia as a green Fuel and Hydrogen Source for Vehicular Applications. Fuel Process. Techn. 90, 729–737. doi:10.1016/j.fuproc.2009.02.004
Zhang, M., Wei, X., Wang, J., Huang, Z., and Tan, H. (2020). The Blow-Off and Transient Characteristics of Co-firing Ammonia/methane Fuels in a Swirl Combustor. Proc. Combust. Inst. 38, 5181–5190. doi:10.1016/j.proci.2020.08.056
Zhang, M., An, Z., Wang, L., Wei, X., Jianayihan, B., Wang, J., et al. (2021a). The Regulation Effect of Methane and Hydrogen on the Emission Characteristics of Ammonia/air Combustion in a Model Combustor. Int. J. Hydrogen Energ. 46, 21013–21025. doi:10.1016/j.ijhydene.2021.03.210
Zhang, M., An, Z., Wei, X., Wang, J., Huang, Z., and Tan, H. (2021b). Emission Analysis of the CH4/NH3/air Co-firing Fuels in a Model Combustor. Fuel 291, 120135. doi:10.1016/j.fuel.2021.120135
Keywords: ammonia, combustion characteristics, laminar burning velocity, pollutant emissions, carbon-free fuel
Citation: Li J, Lai S, Chen D, Wu R, Kobayashi N, Deng L and Huang H (2021) A Review on Combustion Characteristics of Ammonia as a Carbon-Free Fuel. Front. Energy Res. 9:760356. doi: 10.3389/fenrg.2021.760356
Received: 18 August 2021; Accepted: 20 September 2021;
Published: 06 October 2021.
Edited by:
Xuezhong He, Guangdong Technion-Israel Institute of Technology (GTIIT), ChinaCopyright © 2021 Li, Lai, Chen, Wu, Kobayashi, Deng and Huang. This is an open-access article distributed under the terms of the Creative Commons Attribution License (CC BY). The use, distribution or reproduction in other forums is permitted, provided the original author(s) and the copyright owner(s) are credited and that the original publication in this journal is cited, in accordance with accepted academic practice. No use, distribution or reproduction is permitted which does not comply with these terms.
*Correspondence: Hongyu Huang, huaghy@ms.giec.ac.cn