- 1Joint Institute for Strategic Energy Analysis, National Renewable Energy Laboratory, Golden Colorado, CO, United States
- 2U S Department of Energy, Washington, DC, United States
- 3Renewable Energy Consulting Services, Inc, Palo Alto, CA, United States
Clean energy research and development (R and D) leading to commercial technologies is vital to economic development, technology competitiveness, and reduced environmental impact. Over the past 30 years, such efforts have advanced technology performance and reduced cost by leveraging network effects and economies of scale. After demonstrating promise in applied R and D, successful clean energy and energy efficiency technologies are incorporated into an initial product sold by the private sector. Despite its importance, processes by which first commercialization occurs are difficult to generalize while capturing specific insights from practitioners in markets and technologies. This paper presents a policy-focused qualitative assessment of the first commercialization of four diverse energy technologies: thin film photovoltaics, wind turbine blades, dual-stage refrigeration evaporators, and fuel cells for material handling equipment. Each technology presents distinct value propositions, markets, and regulatory drivers. The case studies indicate three common characteristics of successful first commercialization for new energy technologies: 1) good fit between the technology, R&D infrastructure, and public-private partnership models; 2) high degree of alignment of government regulations and R&D priorities with market forces; and 3) compatibility between time scales required for R&D, product development, and opportunities. These findings may inform energy investment decision-making, maximize benefits from R&D, and advance the transition to a low-emission future.
Introduction
Innovations in energy technologies are needed to mitigate the worst effects of climate change, improve resilience (DOE, 2020), and confer other benefits (Fuss et al., 2014; Hao, 2022). In energy, similar to all business sectors, market forces drive innovation (Perez, 2002; Holmqvist, 2004; Markman et al., 2009), with governments mitigating risk for initial investments and addressing problems that markets cannot address themselves (Janeway, 2012). Private sector commercializing of innovations, i.e., achieving financial benefits by selling useful and new developments, often depends on success in niche segments before expanding (Porter, 2002). This pattern is particularly true for technologies that improve sustainability, for which robust sociotechnical models and research exists (Geels, 2010; Smith et al., 2010; Jørgensen, 2012; Geels, 2018; Geels, 2019; Geddes and Schmidt, 2020). However, there are limits to these general theories, and specific, practical case studies are important complements to assess such transitions (Kanger, 2021).
The specific barriers to commercializing new renewable power, sustainable transportation, and energy efficiency technologies present unique challenges. Such technologies often compete with mature incumbents (Bonvillian and Weiss, 2015), including hydrocarbon, nuclear, and earlier-generation clean technologies (Sivaram, 2017) in fragmented, regulated markets (Energy Gov, 2020a). Moreover, clean and efficient energy technologies are at varying stages of development Wind and solar are fully mature and commercialized (Balachandra et al., 2010) while carbon capture and utilization (Sanchez and Kammen, 2016) is neither. Investment needs for technologies at different stages and shortfalls described as “valley(s) of death” are well described (Clyde et al., 1996; Brown et al., 2007); yet, relative to many externally funded businesses, clean energy companies have considerable time (Balachandra et al., 2010) and capital requirements, which limit their growth rates and/or profit margins (Powell et al., 2015) and make for poor fits with most venture capital (Gaddy et al., 2017).
To lower barriers to clean and efficient energy technology development and commercialization, governments have had roles in energy innovation as sponsors, partners, regulators, customers, or some combination (Fuchs, 2010; Bonvillian, 2018; Kattel and Mazzucato, 2018). Governments have directly influenced technology commercialization (Zahra and Nielsen, 2002) via policy, including regulations, tariffs, taxes, rebates (Bronzini and Piselli, 2016); legal fines and court rulings; research funding (Azoulay et al., 2018; Goldstein et al., 2020); and by being a critical first customer for a new technology. Studies spanning many countries have explored the impact of government on technology commercialization extensively (de Almeida, 1998; Foxon et al., 2005; Yeh, 2007; Mazzucato, 2013; Tse and Oluwatola, 2015; Lewis et al., 2017) including comparative studies of impact (Popp, 2016; Goldstein et al., 2020; Popp et al., 2020). The public sector has also stimulated commercialization indirectly by supporting an “innovation ecosystem,” or R&D infrastructure that promotes cooperation and open shared resources between public (Anadon et al., 2016) and private (Oh et al., 2016; Pinto, 2020) organizations. In some situations, researchers have argued the impact of government policy on technology development has been equal to or greater than prices and market forces (Wiser, 2000; Jacobsson and Lauber, 2006).
The rate of technology development and diffusion also depends on business factors, including the stage of commercialization for investment (Nevens et al., 1990; Murphy et al., 2003), corporate culture (Nevens et al., 1990; Treacy and Wiersema, 2007), and management focus (Buckley-Golder et al., 1984; Christensen, 2015). For clean and efficient energy technologies, increased attention to environmental and social impacts has helped attract capital, though such impacts have been insufficient to entirely realign investor priorities (Balachandra et al., 2010) or customers’ tolerance for cost or technology risk (Gompers and Lerner, 2001; Brown et al., 2007; Verbruggen et al., 2010; Gross et al., 2018). In practice, adoption of new technology occurs only if it presents value that is unavailable elsewhere (von Hippel, 1988).
Considering the intense and diverse risks entailed by any business operation (Hall and Woodward, 2010) and especially new ventures (Linton and Walsh, 2003; Popp et al., 2020), the barrier to technology diffusion decreases once a successful product exists. This fact highlights the importance of the initial private sector commercialization of clean and efficient energy technologies, and the paths these technologies take to their respective first markets may therefore contain insights for clean tech commercialization.
The purpose of this policy and practice review paper is to evaluate the conditions and identify generalizable approaches for successful first commercialization of clean energy technologies, a rarely studied phase of research and demonstration and a technology sector of considerably less focus in the literature compared to consumer products. The paper seeks to inform research investment by government program managers and industry decision-makers for technology commercialization in order to advance the transition to a low-emission future. To that end, this work presents four case studies detailing public-private partnerships that resulted in clean energy and energy efficient technology commercialization. While case study papers typically focus on a single technology or technology type, this paper uses diverse case studies to identify key details of the technologies’ transition from lab to first market with emphasis on the enabling factors of the innovation and the market landscape that led to initial success.
The paper does not cover later developments of these technologies toward full market acceptance, nor does it address current early or pre-commercial technologies; instead, the case studies focus on the critical period between advanced research demonstrations and first commercial market success. Common features relevant to broader decision-making in R&D and commercialization processes were identified across the case studies, drawing on primary sources and interviews with government program managers and industry partners that were involved in the adoption of these technologies. The conclusions present specific approaches for key stakeholders involved in energy technology commercialization—government research program managers, technology developers, and business decision makers—to further energy technology development and commercialization initiatives.
Methodology
The data and arguments put forth in this analysis came from primary sources based on a case study approach. These sources include one-on-one and panel interviews from subject matter experts who hold or have held critical leadership roles and contributed to the development of their respective technologies, and four workshops (one on each technology) conducted with government research sponsors. Over 50 experts contributed input over 6 months in mid-2020, including key individuals from the companies involved, their research partners from national laboratories, and the government program managers for each technology (see list of names in the Acknowledgements). The government subject matter experts included the U.S.-based program managers responsible for establishing targets and overseeing technology research programs in these specific areas. Industry partners and research collaborators interviewed were involved in the technology development and the relevant public-private partnerships. All participants were from the United States with one exception from Europe.
The case studies selected originated from the U.S. Department of Energy (DOE) Office of Energy Efficiency and Renewable Energy’s (EERE) portfolio of investments. The following criteria were used to select the cases for this paper:
Diversity of technology type within the broad category of clean energy and energy efficiency. Selected technologies covered renewable energy generation, energy efficiency, and transportation-related equipment that are implemented by power developers and manufacturers with the end users being power companies, industrial operators, and general consumers. This diversity enabled exploration of various drivers of first commercialization of dissimilar technologies and applications.
Diversity of commercialization approaches and strategies. Selected technologies were commercialized by both start-ups and established companies as completely new technologies to the market, major changes to an existing technology, and efficiency enhancements largely invisible to the consumer. This diversity enabled exploration of approaches to first commercialization by different types of organizations based on a variety of policy and market drivers.
Fully commercialized technology. Technologies that had achieved market success enabled identification of the pathways and elements around first commercialization, rather than selecting technologies that were still in development and had not completed their early commercial stage. This was a relatively small subset of technologies with a few caveats, as elaborated below.
Data for these case studies came from internal program metrics, contracts with industry, patent portfolios, published research papers, and government documents that recorded industry interviews and collaborations (and the terms and conditions that are associated with these interactions). Targeted interviews with questionnaires prepared for each technology were conducted with program managers, industry management, and researchers who were participants in the technology development at the time. Generalized energy technology development approaches were derived from these primary data collection sources through careful evaluation by case study participants and the authors. Specific source materials and interviews are referenced within each of the case studies presented in section 3.
Note that there are several areas of constraint within this study. First, the technologies selected for the case studies all reached some level of commercial success, although limited in some cases, since the focus is on first successful commercialization. As with any program directed toward high-risk innovation, many clean energy and energy efficiency technologies supported by government programs never move beyond the lab or demonstration stage or are partially commercialized before failure to fully reach the market (Mufson, 2011; Kao, 2013). This paper focuses on success factors, whereas limits to success represent an area for future evaluation. Second, there is no counterfactual data on the development of these specific technologies without the involvement of DOE. So, the findings may be relevant to specific circumstances for U.S. government R&D programs and not universal strategies as every technology’s path to commercialization differs. Third, the study’s data and information were largely historically collected, not on-going real-time data collection during the development of a portfolio of technologies. This also represents a future area of study for new research and commercialization investments that is briefly discussed in the Conclusions.
Research and commercialization case studies
This section presents summaries and key findings pertinent to the development of four technologies—thin film photovoltaic solar panels, highly efficient wind turbine blades, dual-stage refrigeration evaporators, and fuel cells for material handling equipment—with generalized findings in section 4.
Thin film solar photovoltaics
Thin film cadmium telluride (CdTe) photovoltaic (PV) modules became a commercial product after nearly 30 years of R&D and collaboration among national labs, universities, and private companies (Cheese et al., 2016). This case study focuses on the commercialization success of the company First Solar, which benefited from U.S. DOE solar research, directly received DOE funding in research partnerships in the 1980s–2000s, and subsequently led cost reductions for PV module commercialization (Hegedus and Luque, 2005; Scheer and Schock, 2011; Cheese et al., 2016). This case study argues that addressing regulatory needs within this thin film PV technology’s first major market and establishing a proven product at a price and time for a market that was ready for it, led to its early success.
DOE collaboration generated an innovation ecosystem of thin film PV research that made key advances in CdTe PV technology by funding universities and industrial partnerships from the late 1980s to early 2000s, primarily through programs at the DOE’s National Renewable Energy Laboratory (NREL). An early notable advance during this period was the demonstration of 15.8% cell efficiency (a record at the time) (Britt and Ferekides, 1993) using a cadmium chloride (CdCl2) heat treating process (US Patent 4873198). First Solar co-developed a high-rate vapor transport deposition manufacturing technique (US 5945163) to produce CdTe-based panels at a larger scale—an alternative to the slower, costlier close space sublimation manufacturing process. With suitable device efficiency and scalable manufacturing procedures in place, R&D focus shifted to testing and validation of product reliability. First Solar used testing standards, product quality certifications, and outdoor testing facilities funded by DOE and led by Arizona State University and NREL to prove by 2003 that its modules were ready to enter the solar market. Figure 1 shows a 0.6-kW CdTe test array at NREL’s outdoor testing facility, as well as the structure of a CdTe solar cell.
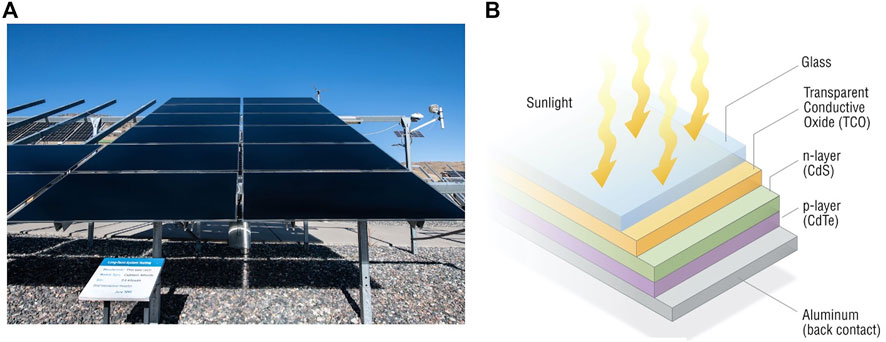
FIGURE 1. A 0.6 kW First Solar CdTe PV test array installed June 1995 at NREL’s Outdoor Test Facility (A) and CdTe PV cell structure (B).
First Solar entered Germany’s major solar market in the 2000s. To do this, First Solar’s modules needed to meet energy performance and regulatory requirements, which included electronic waste regulation and restrictions on the use of certain toxic substances like cadmium (Directive 2002/96/EC, 2003). In 2004, the European Union (EU) Commission evaluated these policies through a workshop on life-cycle analysis and recycling and disseminated DOE-funded research on CdTe from the DOE’s PV Environmental Health and Safety Assessment and Assistance Center at Brookhaven National Laboratory. This effort helped resolve concerns about emissions and recyclability of CdTe PV modules with independent, peer-reviewed studies. Later in 2004, First Solar secured its first contract for its compound thin semiconductor modules in the German PV market—a commercial turning point for CdTe pV. In 2005, First Solar announced a module takeback and recycling program to respond to evolving EU policy directives. These efforts helped communicate First Solar’s responsiveness to regulatory issues, and they addressed public perception of risk sufficiently to access key markets.
CdTe modules are less efficient than silicon-based panels, but owing to their reduced manufacturing costs, they led the lowest price per watt from the mid-2000s until the mid-2010s (Figure 2) (First Solar Inc, 2020; Fraunhofer Institute of Solar Energy Systems, 2020; Mints, 2020). Significant demand for photovoltaics in the European market during this time coincided with insufficient residual wafers from integrated circuit silicon, as well as a temporary shortage in polysilicon, which were used to make silicon solar photovoltaics (Photon Energy Group, 2020).
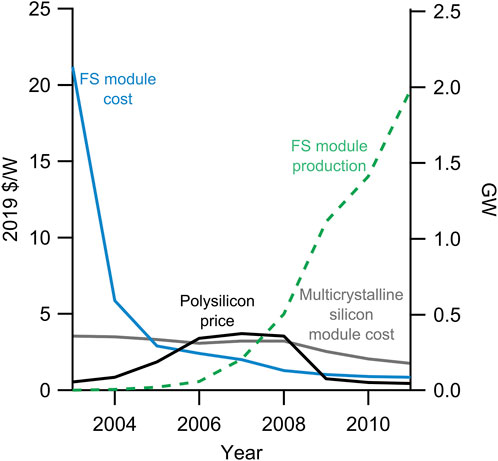
FIGURE 2. First Solar’s production, capacity, and manufacturing cost enabled a decrease in module prices to levels lower than silicon module costs during an increase in polysilicon spot prices (which signaled underlying polysilicon market trends), producing a serendipitous window for CdTe module market entry. Cost per watt adjusted to be real values for 2019. Data sources: (Bernreuter Research, 2020; First Solar Inc, 2020; Photon Energy Group, 2020).
Key Findings: The thin film solar PV case study identified the successful use of three key commercialization strategies: development of technology with many commercially relevant inputs through public/private partnerships, alignment of set technology cost goals and product development that achieved them, and timing compatibility of technology readiness and market opportunity. In this case, government funding over decades enabled foundational materials research and consistent testing standards, that could be taken up by industry as the technology neared commercial readiness. Chance also favored a prepared company with the right product at the right time: CdTe photovoltaics of proven reliability were a lower-cost replacement in a clean energy market with an open window of opportunity, allowing the early commercialization success of this solar technology.
Wind blade improvements
Between 1995 and 2008, a funded ecosystem of universities, national labs, and private companies pursued advances in wind turbine blade design agnostic to a specific approach or design solution. This initiative was conceived and managed by Sandia National Laboratories, in partnership with NREL, and culminated in innovations that substantially increased adoption of wind energy and decreased the levelized cost of electricity (LCOE) for wind (Larwood et al., 2014). From 2009 through 2018, wind energy prices, as indicated by executed power purchase agreements, decreased by over 60% (R. Wiser et al., 2021), holding steady from 2018 to 2021. Although several factors contributed, wind subject matter experts identify improved blade designs as one of the largest innovation factors contributing to wind energy technology cost reductions during this period. This case considers blade design advances and the enabling R&D environment that ultimately led to LCOE improvements, and thus to early market success.
Historically, blade lengths have increased over time to capture more energy. With traditional blade designs, the corresponding increase in the blade mass incurred costs not justified by the associated increase in energy capture. The longer, heavier blades resulted in higher loads and increased cost throughout the turbine system. The exploration in the early 2000s of blade design advances for wind turbine system optimization led to the development of turbine blades with flat backs and bend-twist coupling geometries. These two separate innovations, developed in parallel, allowed for significantly longer blades and thus more energy delivered by each turbine without compromising reliability.
The bend-twist innovation is an inherent structural design for blades to twist as they experienced a wind gust, thus passively reducing the pitch of the blade and lowering the load (Figure 3). This technology was much simpler in concept and operation than contemporary suggestions to change blade pitch with active aerodynamic control devices requiring multiple actuators and moving parts. The flat-back design creates a structurally enhanced portion of the blade closest to the connection to the hub by flattening the trailing edge while the outboard portion remains shaped like a traditional airfoil. Flat-back blades balance ease of manufacturing, aerodynamic performance, and structural strength while reducing weight and enabling a longer, more reliable blade (Miller et al., 2018).
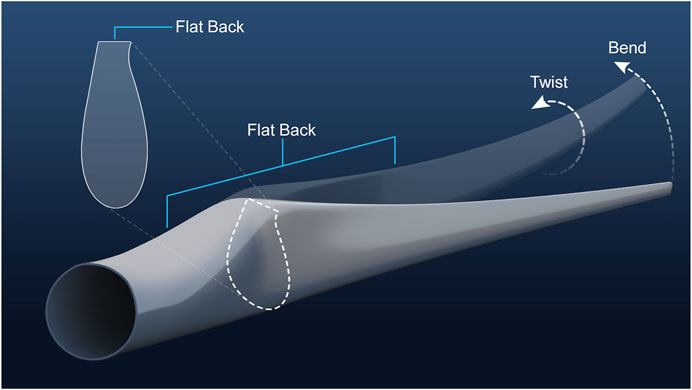
FIGURE 3. Digital rendering of a modern bend-twist flat backed wind turbine blade. Cross sectional view of the flat back is seen in the upper left corner.
The combination of bend-twist and flat-back design enabled longer blades with less mass (Paquette and Veers, 2009). Figure 4 illustrates industry trends in rotor mass and diameter before and after the bend-twist and flat-back innovations (Thewindpower, 2020).
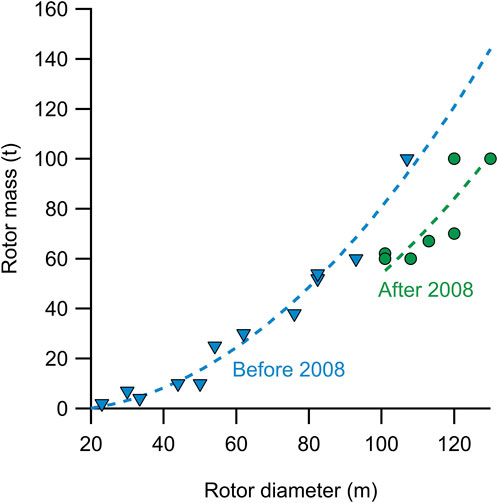
FIGURE 4. Rotor mass in tons vs rotor diameter in meters for Siemens wind turbine products with trend lines fit before (blue triangles) and after (green circles) the adoption of bend-twist coupling and flatback airfoils (2008). Significant reduction in scaling trends enables larger rotors and lower levelized cost of energy. Data source: (Thewindpower, 2020).
The genesis of these design concepts was in the aerospace industry, and academic papers and presentations in open fora documented their innovative extension to wind turbine blades. As the flat-back and bend-twist designs proved out in the R&D ecosystem, private companies adapted the innovations to their own proprietary blades and analysis tools. There were no patents protecting the fundamental applications to wind blades. There were, however, demanding engineering and manufacturing requirements, especially for bend-twist blades, which deterred smaller and less engineering-focused firms. The twin considerations of intellectual property and engineering complexities have shifted focus in the wind turbine industry away from patents toward trade secrets and the development of proprietary internal computational design and analysis tools. Ultimately, industry responsiveness to flat-back and bend-twist coupled blade designs led to innovations that were commercially successful in first markets and, combined with related supporting design tools, drove diversity in blades across the industry, serving as differentiators across companies.
Key Findings: Public-private partnerships that connect universities and private companies with national lab research infrastructure, along with a selectively open approach to intellectual property, spurred the development of advanced wind blade designs. In this case, government played a convening role for innovation in a nascent industry and funded shared research user facilities. In turn, the wind turbine industry successfully commercialized the resulting advanced engineering designs that overcome the tradeoff between rotor diameter and mass inherent to incumbent technologies. The resulting decrease in LCOE, which wind technologists estimate to be nearly 33%, enabled significant wind power expansion post-2008 and led to a worldwide market over $100 billion per year (Global Wind Energy Council, 2019). Given that most major commercial turbines now use elements of flat-back and/or bend twist innovation in their turbine designs, these innovations had a substantial impact on wind deployment and the global economy.
Efficiency in refrigerators
Refrigerators and freezers account for ∼7% of the total electricity usage in U.S. homes, or 105 billion kilowatt-hours and 74 million metric tons of CO2 annually (EIA, 2020; Energy Star Portfolio Manager, 2020). Historically, the bulk of this electricity demand has driven vapor compression to achieve cooling with a single compressor, evaporator, and condenser. This design unavoidably mixes air between the fresh food and freezer compartments, causing fresh food to lose moisture that forms frost on the evaporator coil. Inadequate or over-cooling degrades food preservation quality and is difficult to prevent with a single evaporator, which cannot simultaneously accommodate the different cooling requirements for the two separate compartments. Two evaporators with a post-condenser valve system allows each evaporator and heat exchanger to receive the correct amount of flow for the cooling load, while increasing energy efficiency (see Figure 5). However, dual-evaporator systems driven by two compressors (i.e., two separate vapor compression systems) require extra components, driving up production costs.
Higher costs may be unacceptable to manufacturers, who already have low margins from most refrigerator sales. Consumers are especially price sensitive when purchasing a refrigerator and may be unwilling to pay a premium for increased energy efficiency, opting instead to pay more for such design features as extra compartments or embedded touchscreens. These market forces incentivize manufacturers to invest enough into efficiency R&D to meet minimum efficiency standards, but little more. In this way, efficiency innovations may be driven more by minimum standards requirements than direct consumer demand. Figure 6 shows the annual energy use over time for units sold in a given year and highlights the step-like nature corresponding with new minimum efficiency standards.
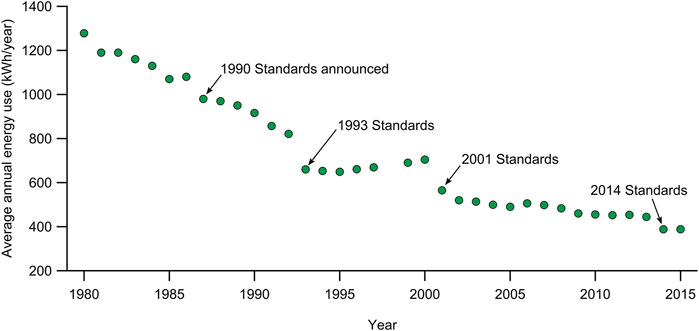
FIGURE 6. Average annual electricity use of new refrigerator-freezers and freezers. Data sources: (Rosenfeld, 1999; Energy Conservation Standards for Residential Refrigerators, Refrigerator-Freezers, and Freezers, 2010; AHAM, 2018).
Whirlpool Corporation and DOE began work on a single-compressor, dual-evaporator system in 2014 as part of a DOE initiative to increase appliance efficiency. Funded by the American Recovery and Reinvestment Act (ARRA), Oak Ridge National Lab (ORNL) provided R&D resources and staffing in collaboration with Whirlpool. A cooperative R&D agreement allowed Whirlpool access to ORNL modeling tools and advanced experimental facilities to assist in the design, validation, and prototyping of this new technology while retaining ownership of the intellectual property. The team was able to demonstrate an advanced refrigerator design with more than 50% energy reduction per unit volume (as compared to the 2001 federal minimum efficiency standard), with a cost increase of less than $100. The innovation led to a family of 14 patents for Whirlpool and enabled the company to meet new minimum efficiency standards (Energy Gov, 2020b).
Key Findings: The refrigerator efficiency case study typifies a successful commercialization pathway driven by alignment between regulatory constraints and R&D priorities. In this case, the government collaborated with an established company through cooperative research agreements utilizing government research models and facilities. The progress in refrigerator efficiency mandated by standards and achieved by Whirlpool’s dual-evaporator technology spurred other companies to develop similar systems to meet the minimum efficiency requirements, until more R&D could be done on other components such as compressors and insulation materials. As those components achieved cost-competitiveness with the dual-evaporator system, a diversity of solutions to comply with standards emerged. Dual evaporator systems are still present on modern day refrigerators, primarily on higher-end units where cost is already at a premium level; lower-end models are simply equipped with higher efficiency and less complex components with advanced adaptive compressors emerging as a technology for highly efficient temperature control. The dual-stage evaporator thus served to satisfy the needs of the first-market conditions set by regulatory policy, and in turn compelled additional R&D of components that met similar needs with reduced complexity and cost.
Fuel cells for material handling equipment
Fuel cells can provide electricity via redox chemistry for stationary, transportation, and portable power applications. DOE has invested in hydrogen fuel cell research since the early 1990s, when successful fuel cell applications (such as in spacecraft and satellites) were too costly for commercial products. Today, large-scale follow-on investment occurs worldwide (Hydrogen Society of Australia, 2020). This case study focuses on fuel cells deployed in forklift and other material handling equipment (MHE) and consider the unique compatibility of this niche market for the technology.
The “captive” nature of MHE fleets made them a practical fit as a first-commercialization target for fuel cells in transportation applications. Integrators forgo the need for a large network of hydrogen refueling stations across the country, opting instead for one location within a warehouse facility. Historically, gasoline-, propane-, or diesel-fueled engines powered MHE for outdoor operations while lead acid batteries powered indoor applications where emissions must be controlled. Lead acid battery-powered MHE exhibit performance issues at low charge, requires long charging and cool down times that can disrupt warehouse throughput, and have limitations in cold environments like refrigerated warehouses. Fuel-cell-powered MHE resolves these issues, as fuel cells do not emit harmful air pollutants or carbon dioxide at the point of operation, and they work in cold environments without degradation of performance (Figure 7). These attributes can lead to reduction of labor costs associated with changing and recharging batteries by as much as 80% while also eliminating the need for battery rooms, shrinking the infrastructure footprint by 75% (Ramsden, 2013).
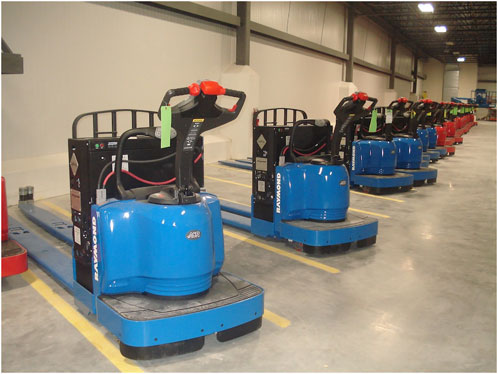
FIGURE 7. Fuel-cell-powered forklifts in a Sysco warehouse in Houston, where they operated in part in a refrigerated environment.
As potential entrants to this niche market in the late 2000s, fuel cell MHEs made a strong case for displacing lead acid battery-powered MHEs. Other emerging power technologies such as lithium-ion batteries were not yet price competitive. At the time, hybrid electric vehicle lithium-ion batteries needed a 3–5x cost decrease to achieve wide commercialization (FY, 2009; Annual Progress Report for Energy Storage R&D, 2009). In 2009, funding from ARRA enabled a large-scale fuel cell MHE demonstration. Through competitive awards with industry, DOE deployed hundreds of hydrogen fuel-cell-powered lift trucks along with supporting systems (fueling infrastructure, data collection and analysis, and operator training). The U.S. Department of Defense also deployed 100 fuel-cell-powered lift trucks at three centers and an army base. A detailed analysis conducted by NREL documented the techno-economics of fuel cell MHE, summarizing the conditions where the technology was cost competitive (Ramsden, 2013).
Throughout the 2010s, guidance and education originating from ARRA deployment and follow-on work led to the integration of 40,000 MH E units within the industry (John, 2021). At the same time, technology competitors surged and the cost of lithium-ion batteries decreased beyond projections (89% since 2010) (BloombergNEF, 2021). Additionally, these batteries’ recharge speed increased, and they gained acceptance in a variety of markets. Comparisons continued to show fuel cells’ utility for refueling in high throughput applications compared to similar fast charging batteries such as lithium-ion (Cano et al., 2018). In the last year, some MHE manufacturers that had announced production manufacturing of fuel cell forklifts have pivoted to advertising forklifts that work with lithium-ion battery technology for similar use cases. The opening of the MHE market to new innovations created by fuel cell forklifts helped spawn further electrification of MHEs and interest from industry in converting to cleaner technologies (Nuvera, 2021).
Key Findings: The fuel cell MHE case study demonstrates all three approaches for commercialization success, including collaboration between private industry and publicly funded research testing opportunities (e.g., ARRA DoD demonstration), R&D advancing technology performance that could meet market requirements, and technology that had performance advantages in time for addressable opportunities. In this case, the government support for research continued through full-scale demonstration funding and direct procurement of early commercial technologies for private and government facilities. Ultimately, MHEs powered by fuel cell technology achieved an overlap of technology readiness and market opportunity, demonstrating energy density, fast refueling, and fuel storage capacities that exceeded performances by competitive contemporary alternative technologies.
Generalized commercialization approaches for energy decisionmakers
Although the four case studies have distinct technologies and stakeholders, they also have common approaches that influenced the technologies’ commercial successes, described below, and summarized in Figure 8. These approaches are relevant for all stakeholders interested in future innovation related to energy. In particular, success depended on a combination of three approaches, and while these have been mentioned in the literature, their application to energy technology R&D has not been made explicit until now. Also noted are key distinctions between market contexts for the four technologies.
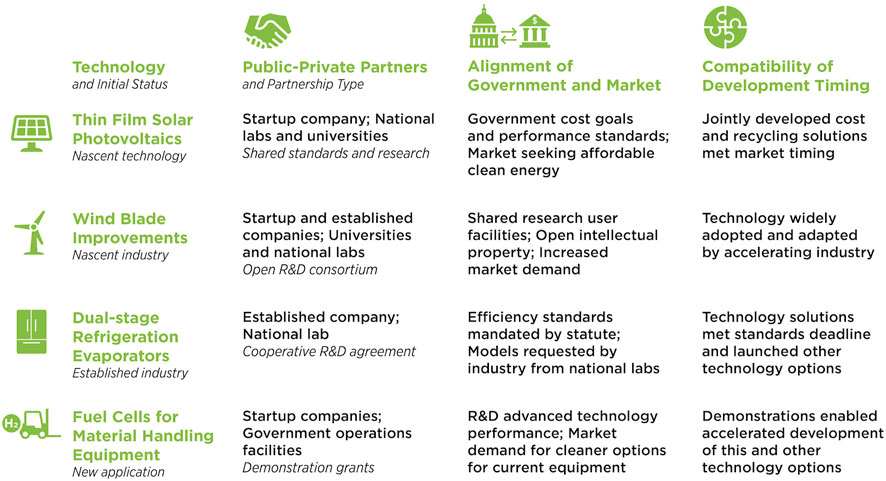
FIGURE 8. Graphical summary of common approaches and key distinctions across four case studies of first commercialization.
First, technology development and commercialization depend on technology fit with research infrastructure and public-private partnership models. Each case study featured collaboration between the private sector and a research institution with critical shared infrastructure supported by an innovation ecosystem. Private sector technology developers leveraged government funding in research capabilities, including testing facilities, standards, and independent analysts. Large energy test facilities are beyond the resources of many companies, especially those in a nascent industry (e.g., PV and wind in the late 1990s). Likewise, field validation and consensus standards often emerge from efforts no company can pursue on its own. In these cases, government often has an important role as a steward of shared resources—physical and informational—that are required for progress. Our findings indicate that at this stage, open access to intellectual property may benefit a nascent industry most broadly. For pre-commercial energy technologies, the long time horizons and competitive challenges mean that it is often necessary to identify and develop shared-use facilities and standards to meet needs through many unexpected technology and market developments. Because public agencies can take risks that private entities cannot, the government is often the first investor in any innovative area; these investments both de-risk and leverage private capital aimed at commercialization. Industry leaders who successfully engage government often begin collaborating on pre-commercial technology, followed by independent innovation that differentiates their companies’ products. Such strategies require significant knowledge of government programs, flexibility in contracting, and strong relationships among individual researchers. For example, with wind blade improvements, technologists collaborated across multiple institutions and companies within an open innovation ecosystem to share or discard ideas, stimulating rapid iteration to overcome technical hurdles. In other cases, individual companies collaborated more independently with government resources, as with the development of dual-evaporator systems for refrigerators.
The second area of commonality among the case studies was a high degree of alignment between government regulations, R&D priorities, and market forces. With extensive stakeholder input, government leaders and program managers publish strategic objectives (e.g., increase energy efficiency) and technical targets aimed at specific priorities to incent innovation. Given higher tolerance for technology risk in government than the private sector, program managers can follow a correspondingly longer path for commercialization. These paths may have a commercialization endpoint identified (e.g., PV panel cost per watt target) or related technology performance targets (e.g., seeking materials with fuel cell properties before reduction to practice), and often require revision in response to technological and external developments. In areas where there is a national objective (e.g., efficiency of refrigerators) but insufficient consumer demand to drive change, government programs may support meeting regulations and standards and enabling industry innovation through access to research and testing capabilities. Each case study technology catered to a specific target market that was in turn responsive to policy, regulation, economics, environment, and manufacturer needs. Fit between product and market is a well-established success factor, and clean energy and energy efficient technologies are no exception. However, unlike most consumer products where the market fit is to consumer demand, clean and efficient energy technologies must also meet specific economic and regulatory requirements, often while contributing toward government or societal objectives noted above.
Finally, each case study found compatibility between time scales required for R&D, product development, and addressable opportunities, including a degree of serendipity. Building on years of fundamental research, funding for later-stage technologies focused on demonstration and commercialization based on market requirements for success. Early development decisions addressed constraints such as environmental health, manufacturability, customer price sensitivity, and demand for drop-in solutions. The case studies profiled various timing compatibilities based on the stage of technology acceptance and market readiness. Highly efficient turbine blades and thin film solar PV advanced fundamentally new technologies, timed with increasing demand for low-emission energy sources—a high-risk market approach rewarded with rapidly increasing sales of renewable energy technologies. While less conspicuous, fuel cells and dual evaporators had performance advantages versus incumbents (e.g., reduced fueling time for MHE and increased efficiency across multiple cooling loads for refrigeration). These advantages led to inclusion in established products that have been viable first markets, and, as with any technology, further growth depends on overcoming increasing competition.
The diverse cases revealed a key distinction between new power generation technologies versus those that create incremental efficiency or energy source changes. Electricity suppliers have widely adopted the core energy generation technologies (thin film photovoltaic cells and efficient wind turbine blades), and these technologies continue to find success in the market. The dual-stage refrigeration evaporators and fuel cells for MHEs achieved lower market penetration as individual technologies. Instead, their development instigated an opening of the market to a multitude of options for cleaner or more efficient energy within their target technology. There are multiple explanations for this difference. First, there are national incentives and sub-national mandates for adoption of renewable power generation that do not exist for other technologies. Moreover, first commercialization of end-use technologies often introduces consumer-focused features (such as better food preservation or reduced equipment downtime) with efficiency improvements receiving lower priority.
Conclusions and policy implications
Commercialization pathways of energy technologies are as diverse as research fields and markets themselves. Each case involved an appropriate set of research policy tools for the stage of the technology development and the partners. Thin-film solar developed through decades of research funding and was enabled through standardized testing protocols. Wind blades improved through a government-convened innovation network and shared research facilities. Advances in refrigeration efficiency emerged from collaboration between government researchers and a motivated established company. Fuel cell equipment launched through direct procurement support after years of government funded technology research. While the specific approaches varied, these diverse case studies did allow generalizable conclusions for both the private and government sector.
Successful private sector decision makers have a deep knowledge of the technology as well as the market and relevant policies, and their strategies account for all these arenas. Leaders at successful companies take advantage of available research infrastructure, including opportunities for cost share and access to shared knowledge or other assets. The timing of such opportunities lends an element of serendipity to commercialization that favors technologies and organizations that are well-prepared, for example through familiarity with resources and priorities of research agencies, government regulators, and other stakeholders.
Government research program managers and policy makers have an array of policy tools to support first commercialization of technologies, although they should be applied differently based on the technology and opportunity. Research funding, shared-use facilities, technology targets, open innovation, and deployment incentives can create the success factors for new energy generating technologies. Regulations, standards, testing, and demonstrations enable advancement in efficiency and powering existing technologies. Every case relied on mission-driven, personalized engagement between government and other stakeholders—in industry, academia, standards-development organizations, and others—that informed ambitious but realistic strategic targets and forged partnerships around them. Together, these elements were essential to creating the first commercialization at the right time. They also establish a self-reinforcing cycle, where successful projects lead both to technology and market impact, and also encourage further engagement between stakeholders and government. R&D agencies have encouraged such cycles for solid-state lighting (National Academies of Sciences, 2017; National Research Council (2013), geothermal energy (Burr, 2000), and in other cases.
This policy and practice review paper and similar business case studies highlight the need for a new approach to understanding success factors for commercialization. Commercialization and related industrial policy case studies are largely historical, retrospectively collecting data and conducting interviews to extract findings. A future approach for government and industry research program managers would be a proactive longitudinal study that would start with defining a set of measurable inputs and success metrics to be applied during research on a diverse portfolio of energy technologies. These data would be collected periodically and interviews with researchers and various stakeholders would be recorded in real-time, indexed, and archived. Over a decade or more of the technologies’ development through either failure, stagnation, or first commercialization, a set of analyzable information would become available to quantitatively model and statistically assess for common definable conditions for success. Ideally, the information might also be used to identify the preparedness needed to take advantage of serendipity to make the leap from research to successful energy product.
The case studies presented here demonstrate that productive interactions between innovative businesses and government have led repeatedly to successful first commercialization of clean energy and energy efficiency technologies. Together, they reveal generalized approaches to new research and interaction with industries comprising the clean energy economy. Future longitudinal and structured cross-cutting studies of energy technology research programs could further enable successful investment and commercialization of advanced energy technologies.
Author contributions
All authors contributed to the initial writing. Additionally, JE, WM, MM, BM, and BW contributed to the conceptualization, methodology, and revisions. BW also supported with funding.
Funding
This work was authored in part by the Joint Institute for Strategic Energy Analysis (JISEA) and the National Renewable Energy Laboratory, operated by Alliance for Sustainable Energy, LLC, for the U.S. Department of Energy (DOE) under Contract No. DE-AC36-08GO28308. Funding provided by U.S. DOE Office of Energy Efficiency and Renewable Energy Building Technologies Office. The views expressed herein do not necessarily represent the views of the DOE, the U.S. Government, or sponsors.
Acknowledgments
Special thanks for photos and graphic design to Dennis Schroeder (Figure 1A), Alfred Hicks (Figure 1B), Besiki Kazaishvili (Figure 3), Jennifer Kurtz (Figure 7), and Nicole Leon (Figure 8). The authors would also like to thank the many experts we interviewed, who reviewed drafts, or otherwise contributed to this paper, including: Andenet Alemu, Jeff Alexander, Sam Baldwin, Garrett Barter, Erin Beaumont, Markus Beck, Jen Bristol, Steve Capanna, Tom Catania, Al Compaan, Fernando Corral, Tim Cortes, Andrea Crooms, Peter Devlin, David Feldman, David Eaglesham, Chris Ferekides, Steve Freilich, Christina Freyman, Vasilis Fthenakis, Nancy Garland, Sarah Garman, Jennifer Garson, Charlie Gay, Markus Gloeckler, Alberto Gomes, Marcos Gonzales-Harsha, Bill Hadley, Michael Heben, Mark Johnson, Stephanie Johnson, Becca Jones-Albertus, Richard King, Jennifer Kurtz, Jeff Logan, Sumanth Lokanath, Robert Margolis, Wyatt Metzger, Anne Miller, Steve Ringel, Doug Rose, Karma Sawyer, Jared S. Silvia, Jim Sites, Henrik Stiesdal, Martha Symko-Davies, Govindasamy Tamizhmani, Lenny Tinker, Christopher Tully, Paul Veers, Alan Ward, Johanna Wolfson, Jetta Wong, Leah Zibulsky and Ken Zweibel. We also thank our journal reviewers for their insightful comments.
Conflict of interest
Author ED is employed by Renewable Energy Consulting Services, Inc.
The remaining authors declare that the research was conducted in the absence of any commercial or financial relationships that could be construed as a potential conflict of interest.
Publisher’s note
All claims expressed in this article are solely those of the authors and do not necessarily represent those of their affiliated organizations, or those of the publisher, the editors and the reviewers. Any product that may be evaluated in this article, or claim that may be made by its manufacturer, is not guaranteed or endorsed by the publisher.
References
AHAM. (2018). Trends in energy effeciency 2018 association of home appliance manufacturers. Available at: https://aham.org
Almeida, E. L. F. (1998). Energy efficiency and the limits of market forces: The example of the electric motor market in France. Energy Policy 26 (8), 643–653. doi:10.1016/S0301-4215(98)00023-8
Anadon, L. D., Chan, G., Bin-Nun, A. Y., and Narayanamurti, V. (2016). The pressing energy innovation challenge of the US National Laboratories. Nat. Energy 1 (10), 16117. doi:10.1038/nenergy.2016.117
Azoulay, P., Fuchs, E., Goldstein, A. P., and Kearney, M. (2019). Funding breakthrough research: Promises and challenges of the "ARPA model". Innovation Policy Econ. 19, 69–96. doi:10.1086/699933
Balachandra, P., Kristle Nathan, H. S., and Reddy, B. S. (2010). Commercialization of sustainable energy technologies. Renew. Energy 35 (8), 1842–1851. doi:10.1016/j.renene.2009.12.020
Bernreuter Research (2020). Polysilicon price: Chart, forecast, history | bernreuter research. Available at: https://www.bernreuter.com/polysilicon/price-trend/#1981-2004-regular-pork-cycle-of-the-contract-price.
BloombergNEF (2021). Battery pack prices fall as market ramps up with market average at $156/kWh in 2019 BloombergNEF. Available at: https://about.bnef.com/blog/battery-pack-prices-fall-to-an-average-of-132-kwh-but-rising-commodity-prices-start-to-bite/.
Bonvillian, W. B. (2018). DARPA and its ARPA-E and iarpa clones: A unique innovation organization model | industrial and corporate change. Oxford, United Kingdom: Oxford Academic.
Bonvillian, W. B., and Weiss, C. (2015). Technological innovation in legacy sectors. Oxford, United Kingdom: Oxford University Press.
Britt, J., and Ferekides, C. (1993). Thin-film CdS/CdTe solar cell with 15.8% efficiency. Appl. Phys. Lett. 62 (22), 2851–2852. doi:10.1063/1.109629
Bronzini, R., and Piselli, P. (2016). The impact of R&D subsidies on firm innovation. Res. Policy 45 (2), 442–457. doi:10.1016/j.respol.2015.10.008
Brown, J., Hendry, C., and Harborne, P. (2007). Developing radical technology for sustainable energy markets. Int. Small Bus. J. 25 (6), 603–629. doi:10.1177/0266242607082524
Buckley-Golder, D. H., Derwent, R. G., Langley, K. F., Walker, J. F., and Ward, A. V. (1984). Contribution of renewable energy technologies to future energy requirements. Statistician 33 (1), 111–132. doi:10.2307/2987719
Burr, R. (2000). OUT success stories: Diamond-cutter drill bits. Available at: https://www.osti.gov/servlets/purl/14528.
Cano, Z. P., Banham, D., Ye, S., Hintennach, A., Lu, J., Fowler, M., et al. (2018). Batteries and fuel cells for emerging electric vehicle markets. Nat. Energy 3 (4), 279–289. doi:10.1038/s41560-018-0108-1
Cheese, E., Mapes, M. K., Turo, K. M., and Jones-Albertus, R. E. (2016). “U.S. department of energy photovoltaics research evaluation and assessment,” in 2016 IEEE 43rd Photovoltaic Specialists Conference (PVSC), 1 June 2016 (Berlin, Germany: IEEE). doi:10.1109/PVSC.2016.7750314
Christensen, C. M. (2015). The innovator’s dilemma: When new technologies cause great firms to fail. Boston, Massachusetts, United States: Harvard Business Review Press.
Clyde, F., Claire, S., Leann, M., Richard, R., and Andee, R. (1996). Surviving the “valley of death”: A comparative analysis. Berlin, Germany: Springer.
Devlin, P., and Moreland, G. (2018). Industry deployed fuel cell powered lift trucksWashington, DC: U.S. Department of Energy. Available at: https://www.hydrogen.energy.gov/pdfs/18002_industry_deployed_fc_powered_lift_trucks.pdf.
DOE (2020). Staff Report to the secretary on electricity markets and reliability | department of energy. Available at: https://www.energy.gov/staff-report-secretary-electricity-markets-and-reliability.
EC (2003). Directive 2002/96/EC of the European Parliament and of the Council of 27 January 2003 on waste electrical and electronic equipment (WEEE)-Joint declaration of the European Parliament. Available at: http://data.europa.eu/eli/dir/2002/96/oj/eng.
EIA (2020). Annual energy outlook 2020. Available at: https://www.eia.gov/outlooks/aeo/pdf/AEO2020%20Full%20Report.pdf.
Energy Conservation Standards for Residential Refrigerators, refrigerator-Freezers, and Freezers (2010). Energy efficiency and renewable energy Office. Available at: https://beta.regulations.gov/document/EERE-2008-BT-STD-0012-0060.energy.gov.
Energy Gov (2020a). Implementation, certification and enforcement. Available at: https://www.energy.gov/eere/buildings/implementation-certification-and-enforcement.energy.gov.
Energy Star Portfolio Manager (2020). Source energy technical reference. Available at: https://portfoliomanager.energystar.gov/pdf/reference/Source%20Energy.pdf.
Energy.Gov (2020b). Quadrennial energy review: Second installment. Available at: https://www.energy.gov/policy/initiatives/quadrennial-energy-review-qer/quadrennial-energy-review-second-installment.
First Solar Inc (2020). Financials-SEC filings. Available at: https://investor.firstsolar.com/financials/sec-filings/default.aspx.
Foxon, T. J., Gross, R., Chase, A., Howes, J., Arnall, A., and Anderson, D. (2005). UK innovation systems for new and renewable energy technologies: Drivers, barriers and systems failures. Energy Policy 33 (16), 2123–2137. doi:10.1016/j.enpol.2004.04.011
Fraunhofer Institute of Solar Energy Systems (2020). Photovoltaics Report. Freiberg, Germany: Fraunhofer ISE.
Fuchs, E. R. H. (2010). Rethinking the role of the state in technology development: DARPA and the case for embedded network governance. Res. Policy 39 (9), 1133–1147. doi:10.1016/j.respol.2010.07.003
Fuss, S., Canadell, J. G., Peters, G. P., Tavoni, M., Andrew, R. M., Ciais, P., et al. (2014). Betting on negative emissions. Nat. Clim. Change 4 (10), 850–853. doi:10.1038/nclimate2392
FY (2009). Annual progress Report for energy storage R&D. Washington, DC: U.S. Department of Energy, 380. Available at: https://www.energy.gov/sites/default/files/2014/03/f8/2009_energy_storage.pdf.
Gaddy, B. E., Sivaram, V., Jones, T. B., and Wayman, L. (2017). Venture Capital and Cleantech: The wrong model for energy innovation. Energy Policy 102, 385–395. doi:10.1016/j.enpol.2016.12.035
Geddes, A., and Schmidt, T. (2020). Integrating finance into the multi-level perspective: Technology niche-finance regime interactions and financial policy interventions. Res. Policy 49 (6), 103985. doi:10.1016/j.respol.2020.103985
Geels, F. (2018). Disruption and low-carbon system transformation: Progress and new challenges in socio-technical transitions research and the Multi-Level Perspective. Energy Res. Soc. Sci. 37, 224–231. doi:10.1016/j.erss.2017.10.010
Geels, F. (2010). Ontologies, socio-technical transitions (to sustainability), and the multi-level perspective. Res. Policy 39 (4), 495–510. doi:10.1016/j.respol.2010.01.022
Geels, F. (2019). Socio-technical transitions to sustainability: A review of criticisms and elaborations of the multi-level perspective. Curr. Opin. Environ. Sustain. 39, 187–201. doi:10.1016/j.cosust.2019.06.009
Global Wind Energy Council (2019). Annual wind Report 2019. Available at: https://gwec.net/wp-content/uploads/2020/08/Annual-Wind-Report_2019_digital_final_2r.pdf.
Goldstein, A., Doblinger, C., Baker, E., and Anadón, L. D. (2020). Startups supported by ARPA-E were more innovative than others but an investment gap may remain. Nat. Energy 5, 741–742. doi:10.1038/s41560-020-00691-8
Gompers, P., and Lerner, J. (2001). The venture capital revolution—American economic association. Available at: https://www.aeaweb.org/articles?id=10.1257/jep.15.2.145.
Gross, R., Hanna, R., Gambhir, A., Heptonstall, P., and Speirs, J. (2018). How long does innovation and commercialisation in the energy sectors take? Historical case studies of the timescale from invention to widespread commercialisation in energy supply and end use technology. Energy Policy 123, 682–699. doi:10.1016/j.enpol.2018.08.061
Hall, R., and Woodward, S. (2010). The burden of the nondiversifiable risk of entrepreneurship—American economic association. Available at: https://www.aeaweb.org/articles?id=10.1257/aer.100.3.1163.
Hao, Y. (2022). Effect of economic indicators, renewable energy consumption and human development on climate change: An empirical analysis based on panel data of selected countries. Front. Energy Res. 10. doi:10.3389/fenrg.2022.841497
Hegedus, S. S., and Luque, A. (2005). “Status, trends, challenges and the bright future of solar electricity from photovoltaics,” in Handbook of photovoltaic science and engineering (Hoboken, New Jersey, United States: John Wiley & Sons), 1–43. doi:10.1002/0470014008.ch1
Holmqvist, M. (2004). Experiential learning processes of exploitation and exploration within and between organizations: An empirical study of product development. Organ. Sci. 15 (1), 70–81. doi:10.1287/orsc.1030.0056
Hydrogen Society of Australia (2020). The hydrogen highway Australia’s opportunity for global leadership in clean heavy transport. Perth: Hydrogen Society of Australia. Available at: https://treasury.gov.au/sites/default/files/2019-03/360985-InfraNomics-supporting-document.pdf.
Jacobsson, S., and Lauber, V. (2006). The politics and policy of energy system transformation-explaining the German diffusion of renewable energy technology. Energy Policy 34 (3), 256–276. doi:10.1016/j.enpol.2004.08.029
Janeway, W. H. (2012). Doing capitalism in the innovation economy: Markets, speculation and the state. Cambridge, United Kingdom: Cambridge University Press.
John, J. (2021). Plug power raises $1B for US green hydrogen infrastructure build-out. Available at: https://greentechmedia.com/articles/read/plug-power-raises-1b-for-u.s-green-hydrogen-infrastructure-buildout.
Jørgensen, U. (2012). Mapping and navigating transitions-The multi-level perspective compared with arenas of development. Res. Policy 41 (6), 996–1010. doi:10.1016/j.respol.2012.03.001
Kanger, R. (2021). Rethinking the Multi-level Perspective for energy transitions: From regime life-cycle to explanatory typology of transition pathways. Energy Res. Soc. Sci. 71, 101829. doi:10.1016/j.erss.2020.101829
Kao, H. (2013). Beyond solyndra: Examining the department of Energy’s loan guarantee program. Williamsburg, Virginia: William & Mary Environmental Law and Policy Review, 86. Available at: https://scholarship.law.wm.edu/wmelpr/vol37/iss2/4.
Kattel, R., and Mazzucato, M. (2018). Mission-oriented innovation policy and dynamic capabilities in the public sector. Industrial Corp. Change 27 (5), 787–801. doi:10.1093/icc/dty032
Larwood, S., van Dam, C. P., and Schow, D. (2014). Design studies of swept wind turbine blades. Renew. Energy 71, 563–571. doi:10.1016/j.renene.2014.05.050
Lewis, A., Oliver, S., Lymburner, L., Evans, B., Wyborn, L., Mueller, N., et al. (2017). The Australian geoscience data cube—foundations and lessons learned. Remote Sens. Environ. 202, 276–292. doi:10.1016/j.rse.2017.03.015
Linton, J., and Walsh, S. (2003). From bench to business Nature Materials. Available at: https://www.nature.com/articles/nmat882.
Markman, G. D., Gianiodis, P. T., and Phan, P. H. (2009). Supply-side innovation and technology commercialization. J. Manag. Stud. 46 (4), 625–649. doi:10.1111/j.1467-6486.2009.00835.x
Mazzucato, M. (2013). The entrepreneurial state: Debunking public vs. Private sector myths. Cambridge: Anthem Press.
Miller, M., Slew, K. L., and Matida, E. (2018). The development of a flatback wind turbine airfoil family. Wind Energy 21 (12), 1372–1382. doi:10.1002/we.2260
Mufson, S. (2011). Before Solyndra, a long history of failed government energy projects. Available at: https://www.washingtonpost.com/opinions/before-solyndra-a-long-history-of-failed-government-energy-projects/2011/10/25/gIQA1xG0CN_story.html.
Murphy, L. M., Laboratory, N. R. E., Edwards, P. L., and Llc, A. G. (2003). Bridging the valley of death: Transitioning from public to private sector financing. Golden, Colorado: NREL. Available at: https://www.nrel.gov/docs/gen/fy03/34036.pdf.
National Academies of Sciences (2017). Assessment of solid-state lighting, phase two. Washington, DC: The National Academies Press. doi:10.17226/24619Engineering, and medicine
National Research Council (2013). Assessment of advanced solid-state lighting. Washington, DC: The National Academies Press. doi:10.17226/18279
Nevens, T., Sumne, G., and Uttal, B. (1990). Commercializing technology: What the best companies do. Available at: https://hbr.org/1990/05/commercializing-technology-what-the-best-companies-do.
Nuvera (2021). Making ports greener. Available at: https://www.nuvera.com/ports.
Oh, D.-S., Phillips, F., Park, S., and Lee, E. (2016). Innovation ecosystems: A critical examination. Technovation 54, 1–6. doi:10.1016/j.technovation.2016.02.004
Paquette, J., and Veers, P. (2009). Increased rotor size through passive load control and weight reduction concepts. Brussels, Belgium: The European Wind Energy Association.
Perez, C. (2002). Technological revolutions and financial capital: The dynamics of bubbles and golden ages. Cheltenham, United Kingdom: Edward Elgar.
Photon Energy Group (2020). Reports investor relations Photon energy Group. Available at: http://www.photonenergy.com/reports.
Pinto, M. (2020). Open science for private interests? How the logic of open science contributes to the commercialization of research. Front. Res. Metrics Anal. 5. 588331. doi:10.3389/frma.2020.588331
Popp, D. (2016). Economic analysis of scientific publications and implications for energy research and development. Nat. Energy 1 (4), 1–8. doi:10.1038/nenergy.2016.20
Popp, D., Pless, J., Haščič, I., and Johnstone, N. (2020). Innovation and entrepreneurship in the energy sector. Cambridge, Massachusetts, United States: National Bureau of Economic Research. doi:10.3386/w27145
Porter, M. E. (2002). How competitive forces shape strategy. Milton Park, Abingdon-on-Thames, Oxfordshire United Kingdom: Taylor & Francis.
Powell, D., Fu, R., Horowitz, K., Basore, P., Woodhouse, M., and Buonassisi, T. (2015). The capital intensity of photovoltaics manufacturing: Barrier to scale and opportunity for innovation. Energy & Environ. Sci. 8 (12), 3395–3408. doi:10.1039/C5EE01509J
Ramsden, T. (2013). An evaluation of the total cost of ownership of fuel cell-powered material handling equipment. United States: Renewable Energy.
Rosenfeld, A. (1999). The art of eenrgy efficiency: Protecting the enviornment with better technology. Annu. Rev. Energy Environ. 24, 33–82. doi:10.1146/annurev.energy.24.1.33
Sanchez, D., and Kammen, D. (2016). A commercialization strategy for carbon-negative energy | Nature Energy. Available at: https://www.nature.com/articles/nenergy20152.
Scheer, R., and Schock, H.-W. (2011). Chalcogenide photovoltaics: Physics, technologies, and thin film devices. Available at: https://www.wiley.com/en-us/Chalcogenide+Photovoltaics%3A+Physics%2C+Technologies%2C+and+Thin+Film+Devices-p-9783527314591.
Sivaram, V. (2017). Unlocking clean energy issues in science and technology. Available at: https://issues.org/unlocking-clean-energy/.
Smith, A., Voß, J., and Grin, J. (2010). Innovation studies and sustainability transitions: The allure of the multi-level perspective and its challenges. Res. Policy 39 (4), 435–448. doi:10.1016/j.respol.2010.01.023
Thewindpower (2020). Wind energy database. Available at: https://www.thewindpower.net/index.php.
Treacy, M., and Wiersema, F. (2007). The discipline of market leaders: Choose your customers, narrow your focus, dominate your market. New York, United States: Basic Books.
Tse, L., and Oluwatola, O. (2015). Eval. Renew. Energy Technol. Transf. Dev. Ctries. Enabling Factors Barriers 6 (1), 10.
Verbruggen, A., Fischedick, M., Moomaw, W., Weir, T., Nadaï, A., Nilsson, L. J., et al. (2010). Renewable energy costs, potentials, barriers: Conceptual issues. Energy Policy 38 (2), 850–861. doi:10.1016/j.enpol.2009.10.036
von Hippel, E. (1988). “Predicting the source of innovation: Lead users,” in The sources of innovation (Oxford, United Kingdom: Oxford University Press), 112–117.
Wiser, R., Bolinger, M., Hoen, B., Millstein, D., Rand, J., Barbose, G., et al. (2021). Land-based wind market Report. 2021 Edition. DOE/GO-102021-5611. 47.
Wiser, R. H. (2000). The role of public policy in emerging green power markets: An analysis of marketer preferences. Renew. Sustain. Energy Rev. 4 (2), 177–212. doi:10.1016/S1364-0321(99)00015-5
Yeh, S. (2007). An empirical analysis on the adoption of alternative fuel vehicles: The case of natural gas vehicles. Energy Policy 35 (11), 5865–5875. doi:10.1016/j.enpol.2007.06.012
Zahra, S., and Nielsen, A. (2002). Sources of capabilities, integration and technology commercialization—zahra—2002—strategic management journal—wiley online library. Available at: https://onlinelibrary.wiley.com/.
Keywords: renewable energy, energy policy, technology commercialization, photovoltaics, wind turbines, refrigeration, fuel cells
Citation: Engel-Cox JA, Merrill WG, Mapes MK, McKenney BC, Bouza AM, DeMeo E, Hubbard M, Miller EL, Tusing R and Walker BJ (2022) Clean energy technology pathways from research to commercialization: Policy and practice case studies. Front. Energy Res. 10:1011990. doi: 10.3389/fenrg.2022.1011990
Received: 04 August 2022; Accepted: 25 October 2022;
Published: 09 November 2022.
Edited by:
Sgouris Sgouridis, Dubai Electricity and Water Authority, United Arab EmiratesReviewed by:
Ali Sohani, University of Rome Tor Vergata, ItalyAngel Barragan Cervera, University of Jaume I, Spain
Copyright © 2022 Engel-Cox, Merrill, Mapes, McKenney, Bouza, DeMeo, Hubbard, Miller, Tusing and Walker. This is an open-access article distributed under the terms of the Creative Commons Attribution License (CC BY). The use, distribution or reproduction in other forums is permitted, provided the original author(s) and the copyright owner(s) are credited and that the original publication in this journal is cited, in accordance with accepted academic practice. No use, distribution or reproduction is permitted which does not comply with these terms.
*Correspondence: Jill A. Engel-Cox, amlsbC5lbmdlbGNveEBucmVsLmdvdg==