- Shanghai Nuclear Engineering Research & Design Institute CO., LTD., Shanghai, China
Nuclear energy plays an important role in the green low-carbon energy. The lead-based reactor is one of the most popular small modular reactor types. The pre-conceptual design of a 100 MW(e) small modular lead-bismuth eutectic cooled reactor was developed by Shanghai Nuclear Engineering Research & Design Institute CO., LTD. to meet the market demand of advanced small-scale nuclear plant. Representative accidents were analyzed for the 100 MW(e) small modular lead-bismuth reactor using ATHLET/MOD 3. The result shows that under the design basis conditions, the performance of the reactor can meet the safety criteria with the protection system working normally. The coolant solidification in the primary circuit under these transients should be prevented. Under the design extension condition, the highest cladding temperature is much lower than the melting point. In the unprotected reactivity insertion condition, there may be a short time of local melting of the fuel, which also meet the acceptance criteria. The safety analysis demonstrated the passive safety of the design with the negative temperature coefficient, strong natural circulation capability, and the passive residual heat removal system.
Introduction
Nuclear energy is an important source of low-carbon energy (World Nuclear Association, 2022; Yue et al., 2022). China announced the strategic vision of “Emission Peak, Carbon Neutrality” and proposed to develop nuclear power actively, safely and orderly (Xing et al., 2022). Small Modular Reactors (SMRs) provide advanced safety features, simplicity of design, the economics and quality afforded by factory production, and flexibility. SMRs are becoming more and more popular nowadays.
The small lead-based reactor (lead or lead-bismuth eutectic) is one of the most popular reactor types of the SMR (Subki, 2020). It has lots of advantages including high safety, high energy grade, is conducive to sustainable development, and has practical experience, high technical maturity. Many small lead-based reactors have been proposed all over the world, such as the 10 MW(e) lead-cooled reactor SEALER proposed by LeadCold in Sweden, the 20 MW(e) movable lead-cooled reactor SSTAR proposed by the Argonne laboratory in the United States, the 100 MW(e) lead-bismuth eutectic (LBE) cooled reactor SVBR-100 and the 300 MW(e) lead-cooled reactor BREST-OD-300 proposed by the Russia‘s State Atomic Energy Company (Alemberti et al., 2014). Among them, the BREST-OD-300 has completed the concrete pouring of the foundation slab in November 2021. Also, many studies have been carried out in China in the past 10 years. In July 2021, the Chinese Academy of Sciences started to build the China Initiative Accelerator Driven System (CiADS), in which the subcritical LBE reactor was adopted.
Since Lead and LBE are important working fluids for the next generation of nuclear power plants, some safety analysis codes have been extended to make these two fluids available, such as RELAP5-3D, ATHLET, and SIMMER-III. The thermophysical properties in these codes are being optimized continuously with the help of new experimental data. Moreover, many studies on safety analysis have been carried out using these optimized system codes, such as the analysis of the European lead-cooled demonstration reactor ALFRED (Advanced Lead Fast Reactor European Demonstrator) using RELAP5-3D (Balestra et al., 2016; Ciurluini et al., 2020), the analysis of the European lead-cooled fast reactor ELFR (The European Lead Fast Reactor) using SIMMER-III (Bubelis et al., 2015), the analysis of the lead-cooled reactor M2LFR-1000 (Shen et al., 2019) and the natural circulation lead-cooled reactor SNCLFR-100 (Guo et al., 2021) using ATHLET.
The pre-conceptual design of a 100 MW(e) small modular LBE reactor was developed by Shanghai Nuclear Engineering Research & Design Institute CO., LTD. (SNERDI), to meet the market demand of advanced small-scale nuclear plant. Lots of work has been done about the safety analysis for the SNERDI LBE reactor, including the selection of initiating events, the evaluation of individual event sequences, the study of the control system and the safety analysis. In this paper, the representative design basis accident (DBA) and the design extension condition (DEC) were analyzed using ATHLET/MOD 3. The purpose is to predict the transient response and safety characteristics of the reactor under accident conditions, and thus support the design optimization of the SNERDI LBE reactor.
Calculation method
Design philosophy and safety features
The SNERDI LBE reactor is a small modular multi-purpose reactor with a nominal power of 100 MW(e) from 280 MW(t). The inherent passive safety of the reactor has been enhanced so that it can be deployed near the population center. The compact plant layout is adopted. Main modules can be fabricated in the factory and transported to the site for installation. The construction schedule can be improved and costs can be reduced. The modular design makes it flexible for the reactor to be deployed for different applications. The offshore electricity generation with zero carbon footprint is the primary target application. The long-term goal is to replace land-based coal plants in symbiosis with renewable energy sources.
Figure 1 shows the main thermal hydraulic circuits of the reactor, including the primary circuit (LBE), the secondary circuit (Water), the passive residual heat removal (PRHR) system and the reactor pool. The entire primary circuit is contained within the reactor vessel. The secondary system includes Steam Generation (SG) modules, separators, pumps, feedwater and steam pipelines. The residual heat can be transferred to the final heat sink with the help of natural circulation in the primary circuit upon loss of flow accident. In the case of loss of feedwater accidents, the isolation valve in the steam pipe will be closed. The valve in the PRHR system will be opened passively by pressure. Thus, the residual heat will be transferred into the water tank of the PRHR system through the natural circulation of the secondary circuit. In the case of beyond design basis accidents in which the PRHR system is failure, the water pool outside the protecting vessel will work as the final heat sink to remove the residual heat to achieve inherent safety.
ATHLET/MOD 3
ATHLET is an advanced best-estimate code developed by GRS. It was initially developed for the simulation of design basis and beyond design basis accidents in light water reactors. ATHLET/MOD 3 includes the thermophysical properties of Lead and LBE fluids.
LBE thermophysical properties in ATHLET/MOD 3 mainly refer to the OECD LBE manual (Fazio et al., 2007). Table 1 shows the main thermophysical properties of LBE fluid.
LBE fluid in ATHLET/MOD 3 uses the following heat transfer relationship:
(1) Heat transfer relationship of circular tube (Cheng and Tak, 2006).
(2) Heat transfer relationship of rod bundle (Mikityuk, 2009).
where P is the fuel rod pitch, the unit is m, and D is the fuel rod diameter.
Scope of application:
Calculation model
Neutronic parameters such as the reactor power distribution and the reactivity feedback coefficient were calculated by the Monte-Carlo code. Figure 2 shows the calculation model of the SNERDI LBE reactor in ATHLET/MOD 3, including the primary circuit system, the secondary circuit system, the PRHR system and the reactor vessel cooling system. The model of the primary circuit system includes the reactor core, pumps, SGs, the cold and hot pool, etc. The core model consists of the hot channel, the average channel and the bypass channel. The model of the secondary circuit system includes SGs, separators, pumps, feedwater pipes, steam pipes and the isolation valve. The model of the PRHR system mainly includes the condensing water tank, the condensing heat exchanger and the passive valve.
The simplified reactor scram protection signal and the PRHR system trigger signal were considered in the control system model. The scram protection signal considered four signals: Over-temperature of the core outlet, low flowrate of the primary circuit, overpower of the core and start-up of the PRHR system. The delay time of the scram signal was set as 0.5 s, and the rod drop time was set as 3 s. Figure 3 shows the logic flow of the simplified control system model. Table 2 lists the trigger signal setting values.
Safety criteria
Table 3 shows the safety criteria of the SNERDI LBE reactor, mainly referring to the safety design guidelines of lead-cooled fast reactor proposed by GIF-IV forum (Alemberti et al., 2017). Under normal conditions (NO), the cladding temperature limit was 620°C. Under anticipated operational occurences (AOO) and design basis accidents (DBA), the cladding temperature limit was 750°C, considering the international experience, especially the research of BREST-OD-300 (Smirnov et al., 2003). Under design extension conditions (DEC), the cladding temperature limit was 1,500°C, which is the melting point of T91 (Shi, 2017; Shen et al., 2019). The melting point of the fuel is 2,804°C at the beginning of the lifetime, which was estimated as 2,484°C conventionally at the end of the lifetime. The boiling point of LBE fluid is 1,670°C, exceeding the cladding temperature limit. The freezing point of LBE is 123.5°C and the solidification limit was set as 223.5°C.
Results
Full-power steady condition
Table 4 shows the results for the full-power steady-state condition. The primary circuit flowrate is 12,842 kg/s. The temperature difference between the inlet and outlet of the core is 151.7°C, which is in good agreement with the design value. The highest temperature of fuel is 2038.5°C. The highest temperature of cladding was 602.9°C. Besides, the liquid level of the cold pool and the hot pool in the reactor vessel were also calculated.
Protected reactivity insertion transient
The rod ejection accident was selected as the starting event, in the case of which a set of control rods was assumed to be ejected at the beginning of the lifetime, introducing 455 pcm (0.62 $) positive reactivity in 5 s. During the process, the protection system worked normally.
Figure 4 shows the results for the PTOP transient. After the introduction of the positive reactivity, the core power increases rapidly to 322 MW in about 2.5 s which triggers the scram protection signal. Since then, the power gradually decreases to the decay heat level due to the insertion of control rods. The flowrate of the primary circuit almost unchanged during the accident. The core inlet temperature is reduced to about 235°C within half an hour, which is close to the solidification limit. The highest temperature of the fuel and the cladding are 2050°C and 603°C.
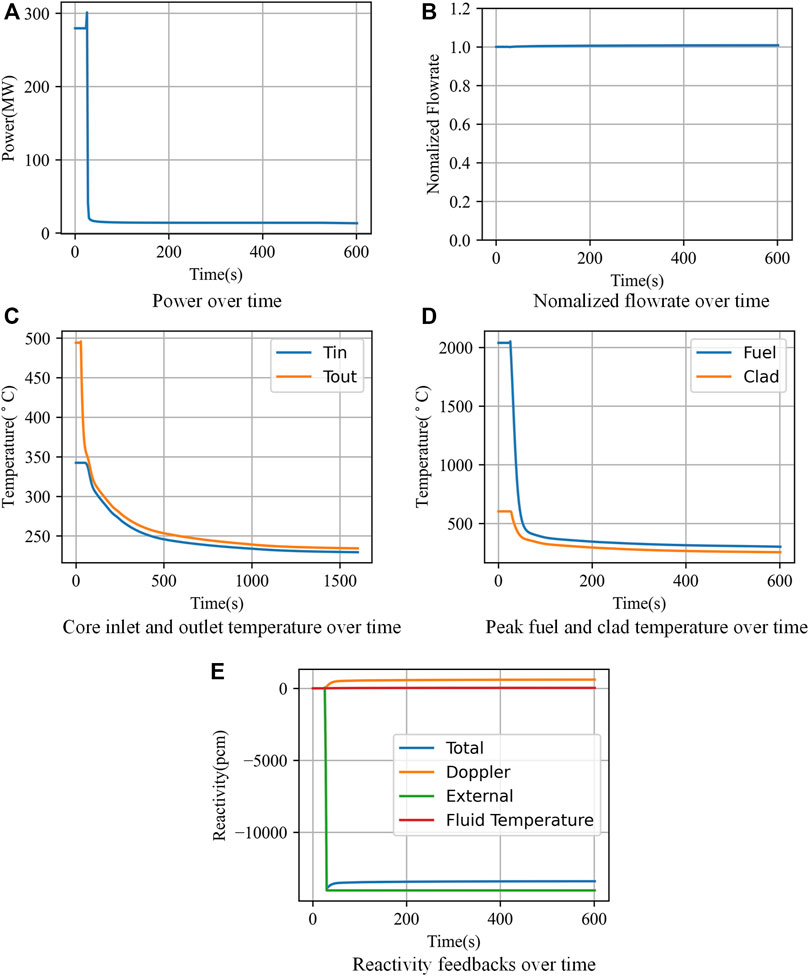
FIGURE 4. Results for the PTOP transient. (A) Power over time. (B) Nomalized flowrate over time. (C) Core inlet and outlet temperature over time. (D) Highest fuel and cladding temperature over time. (E) Reactivity feedbacks over time.
Protected loss of flow transient
The main pump fault was selected as the initial event, and the protection system worked normally during the accident.
Figure 5 shows the results for the PLOF transient. The flowrate of the primary circuit decreases to 90% of the rated value in about 21 s and triggers the scram protection signal, making the power decrease rapidly. After about 400 s, a stable natural circulation is gradually established with a flowrate of ∼6% of the rated value. The core inlet temperature is reduced to about 260°C within half an hour, which is close to the solidification limit. Due to the delay of the protection system, the highest cladding temperature rises rapidly to a peak of 620°C with the decrease of the main loop flowrate. Then, the cladding temperature and fuel temperature gradually decreases with the decrease of the power.
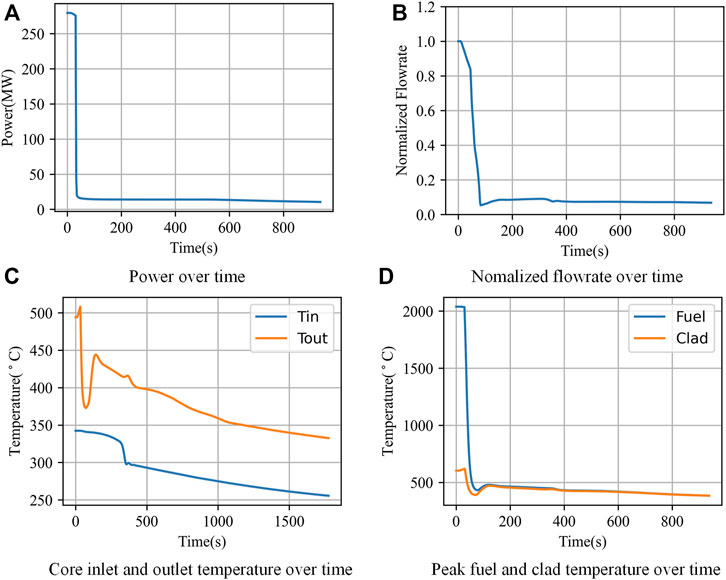
FIGURE 5. Results for the PLOF transient. (A) Power over time. (B) Nomalized flowrate over time. (C) Core inlet and outlet temperature over time. (D) Highest fuel and cladding temperature over time.
Protected loss of heat sink transient
The loss of feedwater was selected as the initial event, and the protection system worked normally during the accident. After the loss of feedwater, the isolation valve in the steam pipeline was closed, and the valve of the PRHR system was opened. Then, the secondary circuit established the natural circulation. The water tank of the PRHR system worked as the final heat sink. In the present calculation model, the delay time between the low flowrate of the feedwater signal and the scram signal were not considered.
Figure 6 shows the results for the PLOHS transient. After the loss of feedwater, the flowrate of the secondary circuit decreases rapidly. And then, the secondary circuit establishes the natural circulation, whose flowrate is stable at 23 kg/s. The flowrate of the primary circuit changes little during the accident. The power reduces rapidly to the decay heat level due to the intervention of the protection system. The core inlet temperature is reduced to about 265°C in about 1 h. The highest temperature of the fuel and the cladding decreases rapidly with the decrease of the core power.
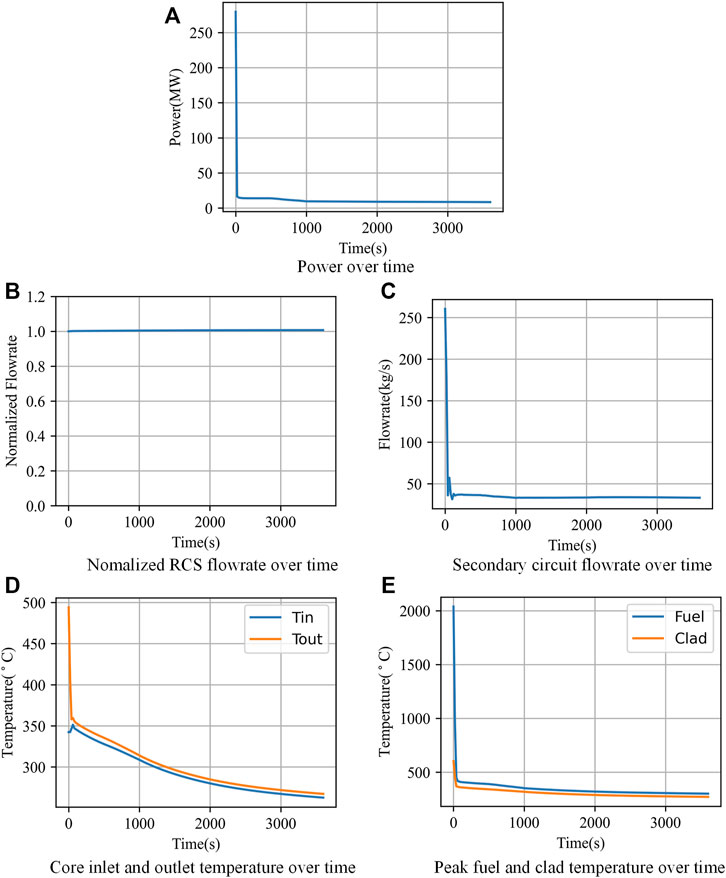
FIGURE 6. Results for the PLOHS transient. (A) Power over time. (B) Nomalized RCS flowrate over time. (C) Secondary loop flowrate over time. (D) Core inlet and outlet temperature over time. (E) Highest fuel and cladding temperature over time.
Unprotected reactivity insertion transient
At the beginning of the lifetime, a set of adjustment rods were ejected and the protection system failed.
Figure 7 shows the results for the UTOP transient. Due to the introduction of 455 pcm positive reactivity, the core power rises rapidly to 660 MW, which is 2.35 times of the rated power. Then, the reactor returns to the critical state by its own negative feedback in about 500 s. The highest temperature of the fuel rises rapidly to the peak of 2,790°C and then decreases to 2,330°C with the decrease of the power. The highest temperature of the cladding rises slowly and is stable at 1,060°C.
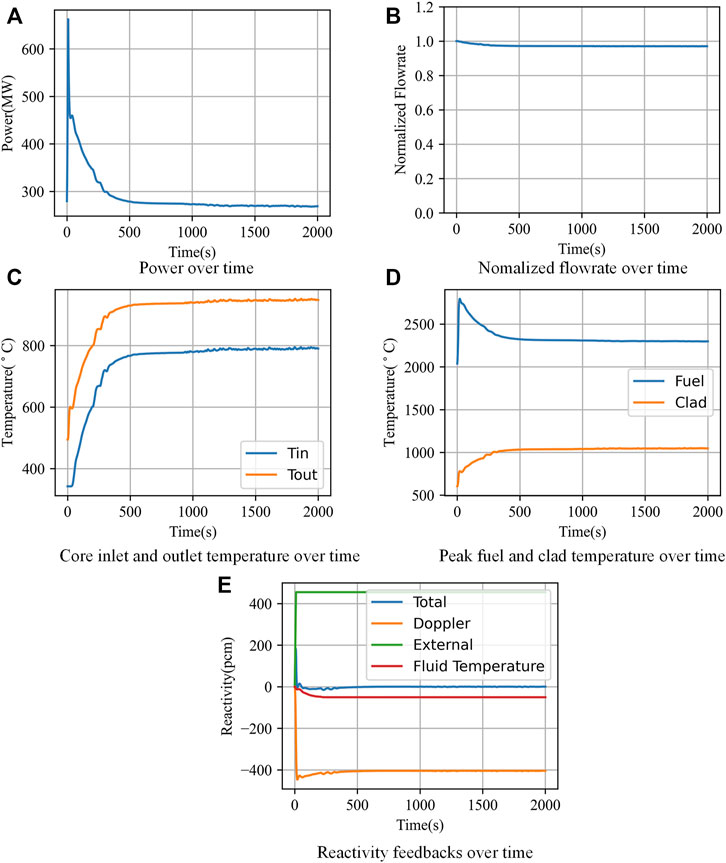
FIGURE 7. Results for the UTOP transient. (A) Power over time. (B) Nomalized flowrate over time. (C) Core inlet and outlet temperature over time. (D) Highest fuel and cladding temperature over time. (E) Reactivity feedbacks over time.
Unprotected loss of flow transient
The fault of the pump in the primary circuit was selected as the initial event. During the accident, the protection system failed and SGs worked normally.
Figure 8 shows the results for the ULOF transient. At the beginning of the accident, the flowrate of the primary circuit decreases rapidly and then the natural circulation is gradually established. After 100 s, the flowrate is stable at 2,327.1 kg/s, which is 18.1% of the rated flowrate. Due to the negative reactivity feedback caused by the increase of the fuel temperature and coolant temperature, the power tends to 180 MW. The highest temperature of the fuel shows a trend from decline to rise, maintaining at 1780°C after 600 s. The highest temperature of the cladding increases rapidly at first, reaching 1,080°C in about 100 s, and then decreases slowly to 1,000°C in 300 s. At the first 80 s, the liquid level in the hot pool reduced, while the liquid level in the cold pool rose. The liquid level difference between the hot pool and the cold pool decreased as the pressure drop of the primary circuit decreased under the ULOF transient.
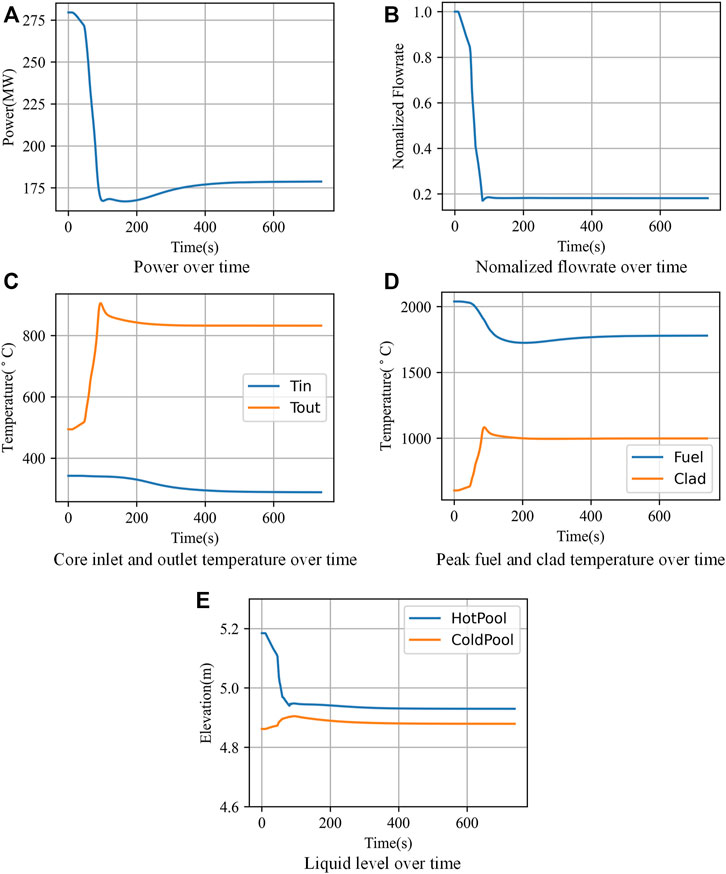
FIGURE 8. Results for the ULOF transient. (A) Power over time. (B) Nomalized flowrate over time. (C) Core inlet and outlet temperature over time. (D) Highest fuel and cladding temperature over time. (E) Liquid level over time.
Unprotected loss of heat sink transient
The loss of feedwater was selected as the initial event. Besides, the safety system was started during the accident, and the protection system failed.
Figure 9 shows the results for the ULOHS transient. During the accident, the flowrate of the primary circuit reduces slightly due to the change of fluid temperature. Due to the negative feedback caused by the increasing temperature, the power decreases significantly and then tends to the decay heat level of 26.64 MW. Because of the significant mismatch between the core power and the thermal removal capability of SGs, the overall temperature in the reactor gradually increases, and the peak cladding temperature reaches ∼930°C at 750 s, challenging the integrity of the reactor in the long term. The highest fuel temperature decreases to 1,050°C with the decrease of power, gradually tending to the cladding temperature.
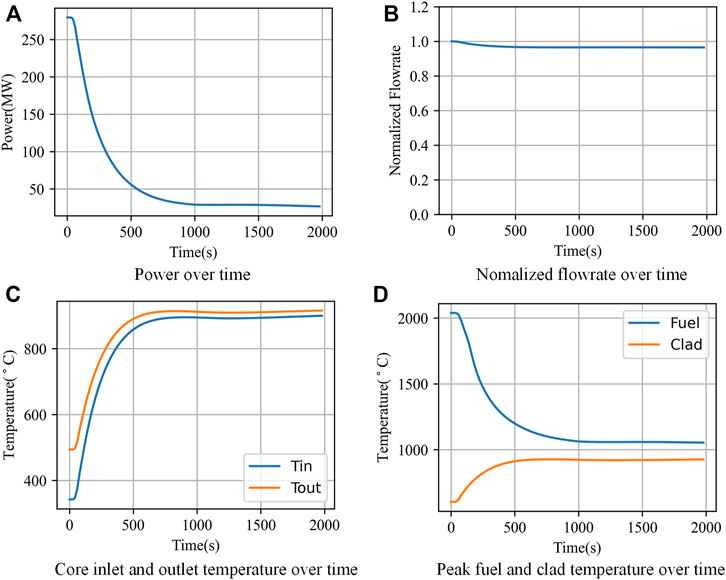
FIGURE 9. Results for the ULOHS transient. (A) Power over time. (B) Nomalized flowrate over time. (C) Core inlet and outlet temperature over time. (D) Highest fuel and cladding temperature over time.
Discussions
Table 5 shows the results of the safety analyses, which can be summarized as follows:
Design basis conditions (PTOP, PLOF, and PLOHS)
Owing to the negative reactivity feedback, the strong natural circulation capacity and the PRHR system, the highest temperature of the fuel and the cladding can meet the safety criteria (fuel 2,484°C, cladding 1,500°C) in all these transients. Half an hour after scram, the coolant temperature is reduced to below 300°C, tending to the solidification limit (223.5°C) and the solidification point (123.5°C). The coolant temperature decreases rapidly mainly because the control system for the temperature and flowrate in the secondary circuit has not been considered in the present calculation model.
Design extension conditions (UTOP, ULOF, and ULOHS)
For the UTOP condition, the highest temperature of the fuel will firstly reach 2,790°C in a short time, which is lower than the melting point of the fuel at the beginning of the lifetime (2,804°C) and higher than the melting point at the end of the lifetime (∼2,484°C), may resulting in a short time of local melting. And then, the fuel temperature will decrease and stabilize at 2,330°C after 500 s, lower than the melting point. The highest temperature of the cladding reaches 1,060°C, meeting the safety criteria. In the current design, the highest fuel temperature under the full-power steady condition reaches 2039°C, which should be optimized in the future. Owing to the strong natural circulation capacity and the PRHR system, the highest fuel temperature changes little in the ULOF and ULOHS conditions, and the highest cladding temperature is much lower than the acceptance criteria.
Conclusion
To meet the market demand of the advanced small reactor, a 100 MW(e) LBE reactor was proposed by SNERDI. The most representative design basis conditions and design extension conditions were analyzed using ATHLET. The results show that the reactor can meet the safety criteria under these representative accident transients. The safety analysis performed for the SNERDI LBE reactor design demonstrated the passive safety of the design with the negative temperature coefficient, strong natural circulation capability and the PRHR system. In the future, more analysis of representative accidents like the sub-assembly blockage transient and the steam generator tube rupture transient will be performed. Also, the design should be optimized to reduce the highest fuel temperature under the full-power steady condition and to prevent LBE fluid in the reactor vessel from solidification under protected transients.
Data availability statement
The raw data supporting the conclusion of this article will be made available by the authors, without undue reservation.
Author contributions
KC, safety analysis of representative transients using ATHLET, writing the paper CT, leading the safety analysis of the SNERDI LBE reactor YQ, selection of initiating events, the evaluation of individual event sequences, optimizing the calculation modle in ATHLET JZ, neutronic calculation YL, studying the control system.
Funding
This work is financially supported by the “Research on Key Technologies of Wide Oxygen Controlled Lead-cooled Fast Reactor” of State Administration of Science, Technology and Industry for National Defence, PRC.
Conflict of interest
Authors KC, CT, YQ, QL, JZ and YL were employed by Shanghai Nuclear Engineering Research & Design Institute CO., LTD.
Publisher’s note
All claims expressed in this article are solely those of the authors and do not necessarily represent those of their affiliated organizations, or those of the publisher, the editors and the reviewers. Any product that may be evaluated in this article, or claim that may be made by its manufacturer, is not guaranteed or endorsed by the publisher.
References
Alemberti, A., Smirnov, V., Smith, C. F., and Takahashi, M. (2014). Overview of lead-cooled fast reactor activities. Prog. Nucl. Energy 77, 300–307. doi:10.1016/j.pnucene.2013.11.011
Alemberti, A., Tuček, K., Takahashi, M., Obara, T., Moiseev, A., Tocheny, L., et al. (2017). “Development of safety design criteria for the lead-cooled fast reactor,” in International Conference on Fast Reactors and Related Fuel Cycles (FR17) (Yekaterinburg Russian Federation), 261–2910.
Balestra, P., Giannetti, F., Caruso, G., and Alfonsi, A. (2016). New RELAP5-3D lead and LBE thermophysical properties implementation for safety analysis of gen IV reactors. Sci. Technol. Nucl. Installations 2016, 1–15. doi:10.1155/2016/1687946
Bubelis, E., Schikorr, M., Frogheri, M., Mansani, L., Bandini, G., Burgazzi, L., et al. (2015). “LFR safety approach and main ELFR safety analysis results,” in International Conference on Fast Reactors and Related Fuel Cycles: Safe Technologies and Sustainable Scenarios (FR13), Paris, France, March, 2013. Available at: https://www.researchgate.net/publication/264422701_LFR_safety_approach_and_main_ELFR_safety_analysis_results (Accessed March 24, 2022).
Cheng, X., and Tak, N. (2006). Investigation on turbulent heat transfer to lead–bismuth eutectic flows in circular tubes for nuclear applications. Nucl. Eng. Des. 236 (4), 385–393. doi:10.1016/j.nucengdes.2005.09.006
Ciurluini, C., Narcisi, V., Giannetti, F., Cretara, L., and Caruso, G. (2020). Preliminary neutron kinetic–thermal hydraulic coupled analysis of the ALFRED reactor using PHISICS/RELAP5-3D. J. Phys. Conf. Ser. 1599 (1), 012023. doi:10.1088/1742-6596/1599/1/012023
Fazio, C., Sobolev, V. P., Aerts, A., Gavrilov, S., Lambrinou, K., Schuurmans, P., et al. (2007). Handbook on lead-bismuth eutectic alloy and lead properties, materials compatibility, thermal-hydraulics and technologies-2007 edition. Paris: Organization for Economic Co-Operation and Development.
Guo, C., Zhao, P., Deng, J., and Yu, H. (2021). Safety analysis of small modular natural circulation lead-cooled fast reactor SNCLFR-100 under unprotected transient. Front. Energy Res. 255. doi:10.3389/fenrg.2021.678939
Mikityuk, K. (2009). Heat transfer to liquid metal: Review of data and correlations for tube bundles. Nucl. Eng. Des. 239 (4), 680–687. doi:10.1016/j.nucengdes.2008.12.014
Shen, C., Zhang, X., Wang, C., Cao, L., and Chen, H. (2019). Transient safety analysis of M2LFR-1000 reactor using ATHLET. Nucl. Eng. Technol. 51 (1), 116–124. doi:10.1016/j.net.2018.08.011
Shi, K. (2017). Preliminary Research on initial events and transient safety characteristic of lead-cooled fast reactor. [master's thesis]. China, Hefei: University of Science and Technology of China.
Smirnov, V. P., Filin, A. I., Sila-Novitsky, A. G., Leonov, V. N., Zhukov, A. V., Efanov, A. D., et al. (2003). “Thermohydraulic research for the core of the BREST-OD-300 reactor,” in ICONE-11. International conference on nuclear engineering, Tokyo (Japan), 20-23, April 2003.
Subki, H. (2020). “Advances in small modular reactor technology developments,” in IAEA-NPTD Webinar on Advances in Small Modular Reactor (SMR) Design and Technology Developments (13p), Vienna (Austria), 24 Sep 2020.
World Nuclear Association (2022). Rising A world nuclear performance Report 2022. Available at: https://www.world-nuclear.org/getmedia/9dafaf70-20c2-4c3f-ab80-f5024883d9da/World-Nuclear-Performance-Report-2022.pdf.aspx.
Xing, J., Gao, L., Huo, X. D., Wu, Y. X., Dong, Y. M., and Luo, Y. B. (2022). Analysis of nuclear energy utilization under the background of “carbon peaking and carbon neutrality”. Nucl. Sci. Eng. 42 (01), 10–17.
Keywords: low-carbon, SMR, LBE, safety analysis, liquid level
Citation: Chen K, Tang C, Qian Y, Lin Q, Zhao J and Lei Y (2023) Safety analysis of representative accidental transients for a 100 MWe small modular LBE-cooled reactor. Front. Energy Res. 10:1088706. doi: 10.3389/fenrg.2022.1088706
Received: 03 November 2022; Accepted: 23 November 2022;
Published: 11 January 2023.
Edited by:
Tianji PENG, Institute of Modern Physics, CAS, ChinaReviewed by:
Pengcheng Zhao, University of South China, ChinaQinglong Wen, Chongqing University, China
Copyright © 2023 Chen, Tang, Qian, Lin, Zhao and Lei. This is an open-access article distributed under the terms of the Creative Commons Attribution License (CC BY). The use, distribution or reproduction in other forums is permitted, provided the original author(s) and the copyright owner(s) are credited and that the original publication in this journal is cited, in accordance with accepted academic practice. No use, distribution or reproduction is permitted which does not comply with these terms.
*Correspondence: Chuntao Tang, dGFuZ2NodW50YW9Ac25lcmRpLmNvbS5jbg==