Food Waste Valorisation for Biogas-Based Bioenergy Production in Circular Bioeconomy: Opportunities, Challenges, and Future Developments
- 1Bioenergy Lab and BDTC, School of Biotechnology, KIIT Deemed-to-be University, Bhubaneswar, India
- 2School of Biotechnology, KIIT Deemed-to-be University, Bhubaneswar, India
The fossil fuel-based linear economy has inherent intricacies such as environmental pollution and the continued need for energy sourcing. Consequently, there has been a shift to a more sustainable circular bio-economy, in which biomass waste is valorised for energy generation while reducing the bulk waste materials and greenhouse gas emissions. In modern bioeconomy, biogas is a primary energy production vehicle. Bio-based economy-enabled technologies result in heat and electricity generation, considerable substitution of fossil fuels for transport, and also the manufacture of additional value-added products and byproducts of economic benefits. Wastes from industrial operations, agriculture, and other anthropogenic activities such as food waste (FW) can be biodigested and transformed into valuable energy sources, nutrient-rich manure, and speciality chemicals. However, for instance, although closed anaerobic membrane bioreactors can totally avoid a microbial runoff, membrane fouling frequently affects the hydraulic performance. Recent developments in anaerobic digestion (AD) of FW have diversified into pretreatment, organic loading, additive supplementation, parametric optimisation, and digestate recirculation to enhance the utility potential of biomass for energy and environment. These numerous anaerobic and microbial interventions support biomass valorisation and related processes, resulting in more efficient biomethanation. Valorisation of FW through biogas-based energy production could serve as an essential cog in the wheel of a circular bioeconomy.
1 Introduction
Considering the farm-to-fork supply line, around one-third to one-half of the total food produced is damaged before consumption, adding up to the total food waste (FW) generated (Xu et al., 2018). A major portion of FW is the food material (including their drinkable forms), once safe for human consumption but no more suitable for the same and thus not sold by food businesses. FW includes any food not intended to be consumed by humans, although it could be suitable as animal feed or for industrial use. FW often consists of both edible and non-edible fractions that are hard to differentiate from each other. As FW is inevitable, food firms are mandated to manage this for hygiene and environmental reasons.
Owing to the rapid rise in the global economy and consumerism, the estimated amount of food lost and wasted has risen considerably in recent years. Annually, around one-third (33.3%) or an average of 1.6 gigatonnes of food meant for human consumption is wasted globally (Slorach et al., 2019). Wastage of food is, in fact, a waste of precious resources such as land, water, and fertiliser that could contribute to environmental damage in the long run (Slorach et al., 2019). The annual global per capita FW generated across the world stands at 160–295 kg (Baroutian et al., 2018). Owing to serious microbial and environmental implications, FW management is a dicey issue that the human race is dealing with (Mishra et al., 2022). The Sustainable Development Goal of the UN to “ensure sustainable consumption and production patterns” specifically aims at FW reduction in particular and reducing food losses throughout the food supply chain in general (Gracia-Gracia et al., 2017). An increased number of socio-political, legislative, and awareness campaign initiatives need to be taken up to achieve it. Reducing the present FW level calls for its better management; certain foods contain inedible components that inevitably end up as waste (Awasthi et al., 2022).
As FW has negative environmental consequences, managing and treating it has become a global priority. The carbon legacy from earlier life cycles of FW is a key environmental implication. Despite recent progress in managing FW, particularly by the affluent nations, the best solution to address the issue is yet to surface. Thus, FW management is a critical research approach that has gained momentum in recent years, with numerous worthwhile research studies to find sustainable solutions to manage FW (Mishra et al., 2022). However, instead of a wholesome approach to the issue, the majority tend to address a lopsided aspect of sustainability, addressing either the environmental, economic, or social consequences. The annual biomass production is increasing 8 times the total energy demand globally. Hence, the economic utilisation of biomass contributes to society in an environment-friendly manner and could attract more research around the world (Duan et al., 2021).
Landfilling has many negative effects on the environment, of which groundwater contamination from the landfill leachate, GHG emissions, and malodour are well-known. Although the traditional environmentally harmful and health-wise risky landfilling practice is the most prevalent, there are various alternate, technically sound, and better options to deal with FW. These include incineration, composting, and anaerobic digestion (AD). AD results in the production of methane, volatile fatty acids, and nitrogen-rich digestate (Duan et al., 2020; Wainaina et al., 2020). The VFAs from feedstock could potentially change the AD in a biorefinery system to produce several chemicals (Wainaina et al., 2019; Liu et al., 2021). VFA production in membrane-based semi-continuous AD has been successful, although feedstock such as citrus fruits could be toxic (Awasthi et al., 2022). Clercq et al. (2017) reported the release of a significant quantity of greenhouse gases (GHG) such as methane (CH4) and carbon dioxide (CO2) when FW is disposed of through landfill, methane being 25-fold potentially stronger GHG than CO2. Food loss and waste account for 6.7% of the total anthropogenic GHG emissions worldwide (Slorach et al., 2019). The biogenic phases of phosphatic and nitrogenous fertilisers for crops have strong environmental consequences (Pramanik et al., 2019). To get a sense of the scale of the GHG problem, consider that 12% of global CH4 emission is caused due to the decomposition of organic waste in landfills (Lytras et al., 2021).
Burning of FW is an energetically inefficient process due to its high moisture content (usually greater than 80%) and also pollutes the air. Such approaches, on the other hand, fail to notice the potential of profitable resource recovery from FW; it has the potential as an important resource option. The chemical complexity of FW makes it a source of several useful molecules such as chemicals, materials, and fuels (Michalopoulos et al., 2020).
2 Why Food Waste–Based Circular Bioeconomy?
Once disposed of, FW degrades rapidly, being nutritionally rich. Also, often, it is the cooked and ready-to-consume version. Upon degradation, issues such as malodour and GHG emission arise. Thus, its reuse as a substrate for industrial production of value-added products faces numerous challenges. The primary role of a circular bioeconomy is to use waste as a substrate for production, as explained diagrammatically in Figure 1.
2.1 Chemical Characteristics of Food Waste
FW is characterised by complex organic components. It contains proteins, lipids, carbohydrate polymers (starch, cellulose, hemicelluloses, and lignin), and organic acids, the proximate fluctuating between regions, seasons, cooking processes, and consumption patterns (Xu et al., 2018). Proteins, degradable carbohydrates, and lipids range between 2.3 and 28.4%, 5.7 –53%, and 1.3–30.3%, respectively (Table 1). Carbohydrates and proteins hydrolyse faster (Xu et al., 2018). FW rich in lipids could release more CH4 than the ones rich in carbohydrates and protein (Li et al., 2017). High lipid content, on the other hand, could lead to system failure due to the synthesis of volatilisable fatty acids (VFA). Table 1 details the varying total (10.7–41%) and volatile (10–34.4%) solids of various FW forms confirming 60–90% moisture contents in fruit and vegetable waste, kitchen waste, and FW. The C/N ratio fell in the range of 12.7–28.84, while the pH ranges between 4.1 and 6.5 (mildly acidic). FW produces methane at 346–551.4 ml/g VS, higher than conventional cattle manure (which stands at 233 ml/g VS) (Pramanik et al., 2019).
2.2 Sources and Classification of Food Waste
This classification considers all categories to link various FW types and the treatment. The most obvious classification is based on cereals, fruits, meat, fish, drinks, and so on. This is useful to calculate the quantity of food lost based on mass, energy content, and economic cost. Elimelech et al. (2018) classified FW based on nutrient content (for example, carbohydrate-rich, protein-rich, and fat-rich), recommended storage temperature of the food sourced from (for example, ambient, chilled, or frozen), or elemental contents (for example, C, H, N, O, and S). They are also classified as avoidable (the edible portion of food), unavoidable (inedible portions, viz., bones, skins, and offal), and preventable (a portion of food that some may consume and some may not, such as bread crusts and potato skin). FW is further classified at the household level as cooked/uncooked and unpackaged/packaged. A set of indicators exist to classify FW, presenting valuable information for their effective management (Garcia-Garcia et al., 2017). Classification based on several indicators is as follows:
2.2.1 Edibility
Anything edible could be ingested at any stage during its life cycle. Otherwise, it is inedible, such as the skin of fruits and vegetables, animal bones, and various stalks. When a portion of food is technically edible but is not an entity of the human food market (for example, leftover grain from a brewery), it is inedible, as it cannot be distributed for human consumption. The off-the-shelf food may contain inedible components (for example, banana skin).
2.2.2 State
Food is classified as unprocessed/processed, ready-to-cook, and ready-to-eat without losing the food attributes in the process for human consumption. A food or its product as a whole, comprising both edible and inedible components, is treated or classified as inedible, although the product may be edible from a biological standpoint. This is grossly due to the ethical concern of being unfit for human consumption; however, it could be safe to feed animals.
2.2.3 Origin
Food items are sourced from various plants or animals. Food of animal origin may particularly need separate tagging due to the selective eating habits/preferences across regions, nations, races or ethnicity, and religious beliefs. A product combining the components from both plant and animal origins is categorised based on its primary constituent.
If a product is of animal origin, it must be classified as meat (including fish), animal product (egg), or other broadly non-edible portion of the animal bodies (for example, offal or the slaughterhouse byproduct). In plant-based and mixed products, it must be ascertained if that includes either any content of animal origin or was in contact with animal-sourced content.
2.2.4 Complexity
This indicator is only applied for food products of plant origin. The product is considered single if it contains only one major component; else, it is a mixed type.
2.2.5 Packaging Material
Packaging material is a necessary evil and critical in ensuring the shelf-life and wholesomeness of the food for a finite duration. There are two specific concerns regarding the packaging material intended for food products. On the one hand, it is the food compatibility (food-grade) owing to food safety, and on the other, its degradability owing to environmental safety. Biodegradability refers to the ability of microbes to digest the material. Paper, plant-based products, bioplastics, and wood are commonly used in eco-friendly biodegradable packaging. Plastics, glass, and metal are common non-biodegradable packaging materials.
2.2.6 Supply Chain Stage
Wastes are generated in the food service across the various stages of the supply chain. “Catering trash” includes household kitchen garbage to the wastes from food services (such as restaurants, residential or day boarding schools, and hospitals). FW is also generated elsewhere at the various levels of the supply chain (viz., cropping, manufacturing/processing, distribution, and retailing).
3 Technologies and Strategies for Improved Anaerobic Digestion Performance
AD involves a series of microbial bioprocesses steps wherein the biodegradable material is broken down anaerobically. The entire process completes in four stages, hydrolysis, acidogenesis, acetogenesis, and methanogenesis (Figure 2). Microbial enzymes such as cellulase, amylase, protease, and lipase play vital roles in the degradation of complex carbohydrates, proteins, and lipids into simpler substrates such as sugars and amino acids during the hydrolysis stage (Mishra et al., 2019). In acidogenesis, the acid-producing bacteria expedite the fermentation and synthesise various organic acids. There is a risk of very low pH conditions at this stage inside the digester once the accumulating organic acids level exceeds the carrying capacity. Acetogenesis involves the conversion of all of the formed organic acids into acetic acid, which is a methane precursor. During methanogenesis, the final stage, methanogens become optimally active to facilitate biomethanation. Methanogens degrade the molecules of low molecular weight anaerobically and produce CH4 using H2, CO2, and acetic acid. It is to note that methanogenesis biologically occurs through two routes, the acetogenic route (primarily anaerobic process) and the hydrogenic route (fully anaerobic process). Mostly, the ambient temperature and pressure, solid content, loading rate, and the pH of the substrate affect the AD inside the digester. Any precaution that could be advocated is, preventing the activity of methanotrophs, if any, inside the digester as they could actively consume the generated methane and reduce the overall methane yield. As it produces biogas as an alternate energy source, AD has emerged as a popular approach to treat FW since concerns about energy security have grown in recent times (Mishra et al., 2019). Furthermore, while reducing the release of GHG, AD reduces the usage of fossil fuels, including the generation of electricity for human energy needs. AD is practically a zero-waste approach that produces the least pollution as compared to other approaches such as incineration, gasification, and pyrolysis (Chew et al., 2021). The digestate produced as a solid residue could supplement as quality manure that improves soil quality (Mohanty et al., 2021). AD entails relatively less land and consumes less net energy compared to other conventional FW disposal and treatment practices, making it a more feasible proposition. However, substrate pretreatment becomes a predisposing factor increasingly to ensure efficient performance before subjecting any organic waste to AD.
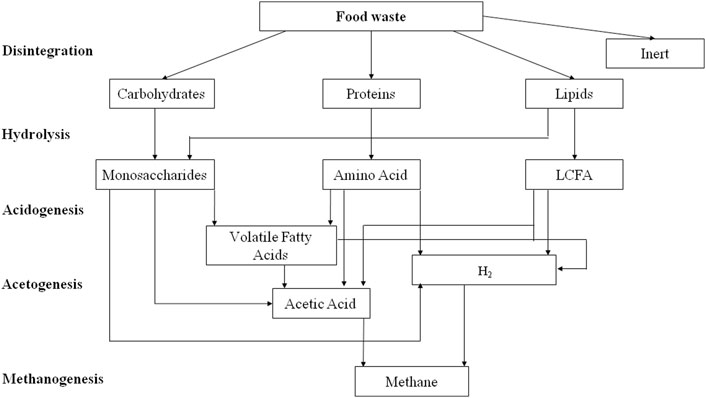
FIGURE 2. Step-wise detailed bioprocess mechanism in anaerobic digestion vis-à-vis the biological products generated.
3.1 Pretreatment
The objective behind pretreating organic wastes such as FW (or its co-digestion) for AD is to enhance the substrate solubility and facilitate expeditious biodegradation while increasing CH4 transformation (Mirmohamadsadeghi et al., 2019). It boosts substrate depolymerisation during hydrolysis leading to better substrate availability to anaerobes during methanogenesis, resulting in a qualitatively and quantitatively higher biogas output. Substrate pretreatment weakens the strong bonds, such as the lignin backbone in the cell wall, thereby ensuring rapid hydrolysis, which is accomplished by the primarily extracellular enzymes (Mirmohamadsadeghi et al., 2019). Pretreatment also shortens the substrate retention time. Various FW pretreatments could be categorised under physical, chemical, and biological strategies. As FW is readily biodegradable, caution is advised while pretreating it (Mirmohamadsadeghi et al., 2019) to prevent system breakdown due to, for instance, high acidification owing to the accumulating VFA.
3.1.1 Physical Pretreatment
Physical pretreatment disintegrates solid particles resulting in increased available surface area for enzymatic activities (Ren et al., 2018). It improves hydrolysis through enhanced substrate-microbe interaction. Particle size reduction to 2.5–8 mm could increase biomethanation (Chew et al., 2021). Ultrasonication pretreatment generated a considerable quantity of hydromechanical shear force through high-intensity waves and broke up the sludge (Rasapoor et al., 2019; Chew et al., 2021). Biogasifiation improved by 59% when the organic loading rate (OLR) was 500 g Vs/m3 and by 80% when the OLR was 1500 g Vs/m3 in the pretreated FW compared to its untreated counterpart. Biogas production is maximised at 20 kHz and 80 min of sonification (Li X et al., 2018). Liquid shearing is another method of mechanical pretreatment wherein high pressure is used, resulting in cell lysis. Milling is yet another approach. Substrates could be dissolved through thermal pretreatment that dissolves the cell wall and membranes (Chew et al., 2021). Thermal pretreatment between 90 and 120°C increased biogas output by 30% and volatile solid (V) degradability by 36% (Li and Jin, 2015). A thermal treatment below or above this temperature range decreased the AD performance. Thermal pretreatment of FW at 80°C increased the methane output by 52% (Pramanik et al., 2019). Undesirable microbes in FW could be removed through thermal pretreatment. The EU law (EC1772/2002) recommends pasteurising FW at 70°C for at least an hour before or after the AD. An additional benefit of this pretreatment is that it boosts the digester loading rate. Very high temperatures could cause VS degradation and deplete the substrate availability for AD. Low-temperature thermal pretreatment could be recommended for enzyme-combined hydrolysis (Chew et al., 2021).
3.1.2 Chemical Pretreatment
In this process, the cell walls and membranes were disintegrated using strong acids and alkalis. Lime treatment boosted biogas yield by 172% compared to the untreated (Chew et al., 2021), attributable to increased surface area and enzymatic activity. Ammonium hydroxide, aqueous ammonia, sodium hydroxide, calcium hydroxide, and potassium hydroxide are some typical alkalis used in pretreating FW to improve biogas yield (Pramanik et al., 2019). The existence of cations in certain reagents, such as calcium, sodium, potassium, and magnesium, is unavoidable and could have inhibitory effects, particularly on certain methanogens (Karthikeyan et al., 2018). Alkaline pretreatment performed at ambient temperature is the most preferred (Kim et al., 2016). Low pH possibly triggered the synthesis of reaction inhibiting phenolic chemicals. HCl pretreatment reduced biogasification by 66% (Chew et al., 2021). Acid pretreatment is not cost-effective as that alkaline pretreatment, albeit it takes less time to degrade the substrate (Chew et al., 2021).
3.1.3 Biological Pretreatment
Biological pretreatment is divided into two categories: aerobic and anaerobic. Microaeration or composting under aerobic conditions expectedly enhanced the hydrolytic enzymatic activity. Biogas yield increased by 23% when FW was pretreated through microaeration. Excess aeration, on the other hand, demonstrably reduced biogasification resulting in low anaerobic fermentation. Separating the first and second stages of AD from the subsequent stages can boost bioprocessing. Chew et al. (2021) demonstrated a 21% improved methane yield in pretreated FW in a two-stage digester. However, a disadvantage of biological pretreatment necessitates a longer retention time and a slower process compared to other approaches (Karthikeyan et al., 2018). Although commercial enzymes are costly, they are being employed in the hydrolysis of starch in FW (Karthikeyan et al., 2018). The single commercial enzyme may be less efficient than multiple enzymes in pretreatment. The use of crude combined enzymes was more energy-efficient compared to the pure commercial enzymes (Karthikeyan et al., 2018). Various FW forms generate enzymes such as cellulases, proteases, lipases, amylases, and pectinases, primarily through SSF, that could boost the enzymatic hydrolysis of FW economically.
4 Biorefinery and Bioeconomy
Increasing energy demand has forced mankind to transit from a fossil-based linear economy to a sustainable circular economy. The onset of such transition is well observed with the national-level policies being translated into civic and social life. The acceptance of higher (up to 20% and above) ethanol blending in vehicular fossil fuel is one such case instance. Biofuel technology is carbon-neutral and follows well the concept of carbon sequestration. Thus, such a guiding bioeconomy is circular and follows a global sustainability model. Biogenic waste is being viewed as a promising feedstock in the circular bioeconomy (Mohan et al., 2018). Its cascading effect shall raise waste transformation to the recovery of economically useful products through integrated acidogenesis, fermentation, methanogenesis, solventogenesis, photosynthesis, oleogenesis, and bioelectrogenesis. Biofuels (such as H2, CH4, biohythane, and biodiesel), chemicals (such as sugars, carboxylic acids, bioethanol, and biobutanol), bioelectricity, biocomposites, biopolymers, biofertilisers, animal feed, and other products could be generated from FW through the biorefinery approach (Esteban and Ladero, 2018). Table 2 presents a range of bioproducts that could be envisaged from an FW biorefinery. A scheme of FW biorefinery is presented in Figure 3. Through this, the dependency on the fossil-based refinery could be replaced to a substantial extent. In fact, FW-based biorefinery could create a sustainable green trail with minimal environmental impact. Also, every AD phase produces key bioproducts: hydrolysis produces sugars and 5-hydroxymethylfurfural (HMF), whereas acidogenesis produces volatile fatty acid (VFAs) and H2, acetogenesis produces acetic acid and H2, and methanogenesis produces CH4.
4.1 Sugars
A major consideration while accounting for product recovery options is the saccharification of FW. During the course of the hydrolytic pretreatment of FW, sugars such as glucose, fructose, galactose, and ribose are predominantly recovered that have predominant use in industry. The pretreatment approach adopted determines the quantity of sugar generated in the hydrolysate. For carbohydrate-rich FW, dilute acid pretreatment seems to be promising. To minimise the production of sugar degrading compounds (such as hydroxymethyl furfurals), low hydrochloric and sulphuric acid pretreatment is typically used either alone or in combinations.
4.2 Biohydrogen
Biohydrogen (H2) is a futuristic green fuel, and researchers’ interest in its synthesis from organic wastes has gathered momentum all over the world in recent times. H2 can be biologically generated through photofermentation, biophotolysis, and AF or their combinations (Mahidhara et al., 2019). Acetogenic bacteria also produce H2 as a byproduct. Tsegaye et al. (2021) reported the biological synthesis of H2 from a variety of wastes. Process parameters such as the type or origin of inoculum, pretreatment strategy, pH, co-digestion, reactor configuration, and temperature influence H2 production through AD directly (Mohan et al., 2019). Using FW as feedstock, H2 synthesis with the help of biocatalyst has numerous benefits, including the economics, non-sterile operating conditions, operational flexibility, using the carbon-rich substrate, and production of a range of products with broad biochemical functions (Goud et al., 2017). Advances in biological H2 production from a range of biogenic waste, particularly FW, may lead to the establishment of biorefinery pilot plants globally and result in H2 slowly but steadily replacing as the key transport force.
4.3 Volatilisable Fatty Acids
Together with H2, another principal coproduct in AD is the VFA or short-chain carboxylic acids such as acetic acid, propionic acid, butyric acid, isobutyric acid, and valeric acid. Nowadays, FW is extensively utilised as a substrate for CH4 production through the VFA pathway. However, due to the relevance of VFAs in food and beverage, plastics, textile, polymer, pharmaceutical, and other sectors, attention has switched in recent decades to VFA synthesis through AD. The kind of VFA generated is largely determined by the nature of the FW, for example, the breakdown of a specific amino acid or the acidification of long-chain fatty acids (LCFA) creates only acetic acid–based VFA. Anaerobic bacteria could synthesise acetic acid, propionic acid, and butyric acid while acidifying monosaccharides. Thus, the major VFA fraction from FW primarily containing carbohydrates is expected to be the acetate.
4.4 Biomethane
Methane (CH4) is the main product of biogasification. Anaerobic synthesis of CH4 from FW is likely the most promising bioenergy approach. CH4 output from FW is influenced by factors such as pH, temperature, C:N ratio, reactor design, inoculum type, co-digestion, VFA, and organic loading rate. A biomethanation technology with better process performance has evolved from the recent advances in biogasification research studies (Dahiya et al., 2018). As full-scale commercialised technology models developed, quantitative and qualitative CH4 synthesis from FW is tasting entrepreneurial success in India in particular and the world in general.
4.5 Bioethanol
Addressing the food vs. fuel issue, the proximate of FW primarily makes it a good bioethanol production candidate. The option for bioethanol synthesis and the high solids content reduction from FW has been investigated using vacuum recovery technology. As compared to the traditional fermentation (326.55.4 g/kg FW), vacuum recovery fermentation produced more (357.55.4 g/kg FW) ethanol (Jarunglumlert et al., 2021). Biphasic operation linking FW saccharification by Saccharomyces cerevisiae followed by fermentation yielded 0.43 g ethanol/g TS. Ethanol yield was low (0.31 g ethanol/g TS) in saccharification and fermentation combined single-stage approach (Dahiya et al., 2018).
4.6 Biobutanol
Butyl alcohol, commonly termed as biobutanol, is a precursor, common solvent, eluent, and extractant for other chemicals. To synthesise butanol, starch-rich crops, including rice, maize, wheat, energy crops, and algae, are often utilised as substrates, classified as first-, second-, and third-generation biobutanol. A method to produce biobutanol is extracting sugars from the substrate through multiple pretreatments and subjecting the pretreated material to Clostridiaceae (Kumar et al., 2021). The high carbon content in FW facilitates profitable biobutanol synthesis, although research initiative to validate its scope and potential is imminent.
4.7 Biopolymer
Polyhydroxyalkanoates (PHAs) are a class of biopolymers produced as secondary metabolites by a variety of prokaryotes, including bacteria, in response to nutritional scarcity or stress (Raza et al., 2018). Dahiya et al. (2018) produced PHA from biogenic wastes (FW), wasted wash effluent, sugarcane molasses, and bakery waste hydrolysate by employing Halomonas boliviensis. Acidogenic fermentation can be combined with PHA synthesis. They produced 23.7% PHA through multi-stage bioprocessing of FW-based fermented acidogenic effluent. Readily available VFA in fermented acidogenic FW effluent facilitated higher PHA synthesis (39.6%) as against 35.6% by the unfermented (Nielsen et al., 2017). FW-based bioprocess was efficient when acidogenesis and bioplastics manufacturing were combined as compared to the raw FW substrate (Dahiya et al., 2018).
4.8 Biofertiliser
AD-based FW composting could generate biofertiliser/manure. Parameters such as pH, temperature, moisture, aeration, and C:N ratio influence the quality of the formed compost. Composting could be accomplished aerobically (aerobic compost) and anaerobically (anaerobic compost). Biofertiliser manufacturing from FW assists in redirecting the carbon emissions from landfills and controlling GHG emissions while generating nutrient-rich organic fertiliser. Setting up of community-level composting facilities to transform FW into a nutrient-rich biofertiliser could be considered a cost-effective way to reduce ecological consequences and ensure sustainable development. Microbial pretreatment of FW with the lipolytic and thermophilic Brevibacillus borstelensis SH168 enhanced the biofertiliser quality with increased nitrogen (2.01–2.10%) and ash (24.94–29.21%) contents (Tsegaye et al., 2021). Thermal hydrolysis of FW could yield safe liquid organic fertiliser by reducing its bio- and phyto-toxicities (Gao et al., 2021).
5 Environmental Impact
The pressure on effective disposal of municipal solid waste has significantly increased owing primarily to the massive quantity of FW that has added up in recent times. However, such waste biomass is abundantly rich in renewable resources (Ding et al., 2021; Khan et al., 2022) that could be of high human economic benefits. FW valorisation has gained increased research and commercial attention for the dual purpose of waste management and energy self-sufficiency. One approach to assess the environmental impact of any material of concern is the life cycle assessment (LCA). This environmental socioeconomic technique is useful to analyse the complexity of interactions between the environment and a product or activity.
LCA involves gathering a list of appropriate inputs and outputs of the system, evaluating the potentials associated with them, and assessing the results of inventory analysis and their phase-wise impact (De Menna et al., 2018). Such assessment in FW considers the material and energy balance at each stage of its life cycle and also the associated ecological consequences by assessing and combining the inputs and outputs. Upstream steps such as collection, transportation, and treatment processes and downstream steps such as post-treatment product recovery and disposal of the residue are involved (Bartocci et al., 2020). The range of elements for LCA application of FW varies based on the uniqueness of each study.
LCA strategies are broadly of two types, attributional and consequential. The former is designed to provide a stable picture of the average conditions while omitting the market-mediated effects, while the latter determines the impacts related to the alteration introduced to the system. The three major factors out of the numerous ones that determine the environmental consequences of FW are CO2 production (implying the global warming potential) and the water and land usage patterns. The global warming potential (GWP) is most widely used in assessing the overall impact. However, as the outcome of LCA frequently is dependent on rational and often incomparable methodological choices, it leads to confusion among the subject novice (De Menna et al., 2018).
Kim and Kim (2010) documented one such study involving GHG emissions from FW. Von Massow et al. (2019) reported the weekly and the annual global warming potentials (GWP; calculated as kg CO2) of the untreated household FW as 23.3 and 1.2 T, respectively. They also opined that although FW of animal origins such as meat, fish, milk, cheese, and eggs contributed relatively more to CO2 production, fruits and vegetables produced nearly 40% of the total CO2 while comprising 66% of the overall weight of the FW. Judicious valorisation of FW could lead to the production of clean energy such as biogas that could help partially meet the growing energy demand while being ecofriendly. Biogas has multifarious use as a vehicular fuel (BioCNG), for electricity generation, and for heat generation. (Srivastava et al., 2020). The unit to generate electricity and heat from biogas typically uses combined heat and power. The electricity generated could be used at the digester site to maintain the desired reactor temperature while the excess could be transported to various end-users. The system to treat industrial exhaust gas leads to decreased detrimental effects on the environment (Chew et al., 2021).
AD by itself cannot stabilise FW, which may lead to the inefficient biotransformation of the locked nutrients in FW that are discharged as a liquid or solid digestate (Peng et al., 2020). Extensive AD applications will lead to increased generation of digestate that needs proper disposal. This digestate contains residual indigestible material, process intermediates, and dead microbes (Cheng et al., 2021). Because of its high nutrient content, digestate has huge agricultural manure potential (Cheong et al., 2020). Apart from the nutrients, digestate also contains heavy metals such as Cu and Zn and pathogenic microbes, posing a significant threat to the handlers in particular and the environment in general (Logan and Visvanathan, 2019). There is a need for an effective rational way to treat solid digestate. Effectively treating digestate has become a critical determining factor in viable AD engineering for FW management.
Composting, landfill disposal, and incineration are a few other common digestate handling practices. Of these, composting seems to be a technically-sound and sustainable practice to better the agricultural and environmental conditions. It is reportedly extensively practised in North America, Europe, and elsewhere (Grigatti et al., 2020; Herbes et al., 2020). Because of its high moisture content and recalcitrance of components to biodegradation, solid digestate disposal via composting necessitates incorporating supplementary materials or applying drying procedures (Awiszus et al., 2018). This approach, however, is controversial because of its limitations, which include GHG emissions, heavy metals issues, and malodour (Awiszus et al., 2018; Chew et al., 2021). Incineration consumes high calories to handle the organic matter content of digestate. Landfill seems to be cost-effective and simple and ensures the final step of solid digestate disposal.
Of these three available options, mostly landfill disposal and incineration are dependent on the available municipal solid waste (MSW) treatment facilities (Ma and Liu, 2019). Even though these two approaches do not incorporate material recycling and, thus, do not cater to the circular economy and global sustainable development, they remain preferred choices by most Chinese decision-makers owing to the convenience of utilising the available MSW facilities. It is unknown if such disposal options are cost-effective and environmentally sustainable considering the FW treatment cycle. Currently, studies on integrated FW-based biogas systems standing on the principles of the circular economy have gathered popularity (Li et al., 2020; Nordahl et al., 2020; Cong and Thomsen, 2021). Studies have shown that digestate treatment has a significant influence on the overall environmental impact, with various disposal approaches having varied effects. Nordahl et al. (2020) demonstrated that relying solely on landfill techniques to manage solid digestate from dry AD systems might adversely impact the global warming potential of a 100-year (GWP100) footprint reaching 40 kg CO2 per tonne of organic waste. According to Nhubu et al. (2020), digestate composting can improve the potential for eutrophication and acidification. Researchers have looked into the environmental and economic impacts of the numerous technologies in digestate processing (Herbes et al., 2020; Nhubu et al., 2020).
6 Opportunities and Prospects
Global sustainable development based on the circular economy has now taken centre stage on the global agenda. The circular economy is built on the principles of resource efficiency, minimising waste, recycling and valorisation. FW has been an underutilised resource, a crucial circular economy focus area, which can potentially be converted into usable products (Usmani et al., 2021). In this context, an immobilised biocatalytic enzyme system is a novel solution to maximise the value of FW while improving the economics and environment of sustainable food production (Chapman et al., 2018), although considerable impediments to their field-scale applicability remain. This necessitates additional extensive research efforts to address the issue.
While being one large product of FW, municipal solid waste is a heterogeneous collection of various inorganic and organic wastes. The presence of undesirable materials in AD biodigester can lead to operational complications, ultimately leading to reactor breakdown. Waste segregation, therefore, seems to be the plausible first step forward in addressing the issue (Franca and Bassin, 2020). Segregation of FW from municipal solid garbage is uncommon in many countries. Adequate segregation strategies, thus, need to be taken since these will aid in better management of municipal solid waste with value-addition options (Franca and Bassin, 2020).
Pretreatment strategies could improve the performance of AD and expedite the FW degradation rate. Dedicated research to ascertain the effects of the added micronutrients on the AD process is warranted (Ambaye et al., 2021). It involves decreasing the production of recalcitrant compounds during chemical pretreatment, increasing the hydrolysis rate during biological pretreatment, and lowering the energy needed for thermal and ultrasonic pretreatments (Mishra et al., 2018). The majority of pretreatment studies are limited to laboratory scales, and the data generated from these studies may not be applicable for scaled-up operations. This needs serious thought and field-level validation of novel promising strategies.
FW-based biorefinery is presently in its preliminary stages of theoretical research, which must be developed diligently and expeditiously owing to several process execution bottlenecks (Paramanik et al., 2019). These include reducing the expenses on feedstock transportation, minimising the production cost, and maximising reactor performance. FW-based biorefinery may lead the way to efficient enzymatic bioremediation and an array of high-value products (for example, bio-oil, biosurfactant, lactic acid, bioplastics, and wax.), bioenergy (for example, biogas, bioethanol, biodiesel, and syngas), and nanoparticles (Nayak and Bhushan, 2019). The FW-based biorefinery is foreseen to play a significant role in material, chemical, and energy production (Mata et al., 2018). There is an urgent need to improve biogas technology not just for its use as a domestic energy source but also as a vehicular fuel, fuel cell, and injection into the natural gas systems. Accessible technologies that are required to upgrade biogas include water scrubbing, membrane separation, cryogenic, pressure swing adsorption, and ammonia scrubbing (Mishra et al., 2021). These approaches could further be fine-tuned and validated for enhanced system efficiency.
Technologies based on biological and hybrid approaches are currently under development. The economic aspects and technical improvement of biogas scrubbing could be a major focus of research. Adding a cosubstrate to FW during AD is an alternate operation optimising strategy to ease the high capital costs in building the plants to fix process instability (Gao et al., 2021). Research studies have emphasised the process of adding sewage sludge to enhance biogas production in FW codigestion, and the same on optimising and developing anaerobic codigestion needs to be pursued (Karki et al., 2021).
The addition of nanoparticles primarily as micronutrients to AD has been proposed to promote bacterial growth. Chew et al. (2021) showed that the yield of biogas increased by 50% with a significant decrease in the degradation time when micronutrients such as nickel, magnesium, cobalt, and calcium were added. Extensive research studies are recommended to determine the effect of the added micronutrients in AD. Being a renewable energy source, biogas can potentially act as a fossil fuel alternative for the sake of economic and environmental benefits and may be promoted. Studies on anaerobic codigestion to enhance biogas production have recently been reported (Hagos et al., 2017; Perin et al., 2020; Lohani et al., 2021; Singh et al., 2021). When employed as a cosubstrate and only substrate, FW has demonstrated positive outcomes, at least at the laboratory scale. Comparative field-scale research on FW as the only substrate vis-à-vis primary substrate to determine the optimal operating parameters may be followed before full-scale implementation of its anaerobic codigestion.
Therefore, as an underutilised resource, FW could be a crucial circular economy focus point that could potentially be converted into usable products. Keeping this in view, the thrust areas to propel the research and development on FW valorisation in line with the global sustainable goal could be summarised as follows:
1 The heterogeneous collection of various inorganic and organic wastes in municipal solid waste could complicate biodigester operations. Waste segregation may be useful to address it.
2 Codigestion of multiple substrates is an alternate optimisation strategy to economically fix process instability and is another envisaged research intervention.
3 Research works on the pretreatment (physical, chemical, and biological) strategies to ascertain their effects on AD may be undertaken.
4 FW-based biorefinery could offer an array of high-value products (for example, bio-oil, biosurfactant, lactic acid, bioplastics, and wax), bioenergy (for example, biogas, bioethanol, biodiesel, and syngas), and nanoparticles the process with regard to which could be optimised and standardised.
5 Nanoparticles as micronutrients allegedly promote microbial growth, which could be extensively researched.
6 Before field-scale implementation of codigestion, research on FW as a stand-alone substrate vis-à-vis as a primary substrate to optimise the operation may be recommended.
7 Field-level validation of strategies, including the FW-based biorefinery, with a focus on circular bioeconomy, may be developed.
8 Although considerable field-scale application impediments remain, an immobilised biocatalytic enzyme system is a novel solution to maximise FW valorisation.
9 The upgraded application of biogas as a vehicular fuel, fuel cell, and injection into the natural gas system is suggested.
7 Conclusion
AD, which has the potential to meet local energy needs, minimise waste, and enhance energy security and environmental upkeep, seems to be a cost-effective strategy to treat organic wastes. A second or extended life to the materials that would otherwise be considered waste is ensured via the AD process. Biogas, with multiple functional benefits, including being used as a source of heat and power, and as an alternate vehicular fuel, is a plausible green energy option. Analysing and categorising their biodegradability, microbial activities, geographical accessibility, and determining the specific limiting elements during the bioprocess stages are motivating research aspects. Process moderation according to the prevailing local environmental conditions and the available raw material (due to the diverse nature of feedstock composition) is necessary for the optimal operation of field-scale FW-based biogas plants. During AD, the biodigester typically contains a mix of VFAs and other secondary products. Since a single microbe may be incapable of transforming all feedstock into useful VFAs, applying niche-specific suitable microbial consortia for enhanced biomethanation could be a critical intervention. Such intervention may entail operational modifications. The evolving bioengineering practices in advancing the tolerance of the developed microbial consortia for enhanced VFAs production and its expeditious transformation to numerous useful products, such as methane, syngas, hydrogen, and organic acids, could be a useful consideration. Biogas has distinct advantages over other renewable energy alternatives. The technologies for generating and storage of biogas are relatively easy and simple. Its end-user delivery can be done using the existing gas pipelines and can also be utilised in a way similar to natural gas. Sooner or later, biogas is set to replace fossil fuels in transportation, apart from its conventional usage in generating heat and electricity. Proper process control with monitoring systems for critical parameters, on the other hand, would help in-process monitoring and optimisation, thereby enhancing the output. Furthermore, the biogas production system must be geared toward lowering capital and management costs. For the AD approach to attain its full potential, process standardisation by the researchers and its popularisation by the policymakers must be ensured. The “waste to landfill” approach needs to be recoined as “waste to wealth”, and utilising low-carbon gas to meet the energy needs must be encouraged. The governments should enhance support for the popularisation and production of biogas and take into account the significance of biogas technology. With continued concerted efforts, biogas will be a remarkable alternate energy source that would reduce GHG emissions while managing waste and making the nation energy self-sufficient.
Author Contributions
SM and TA designed the skeleton of the manuscript and provided their valuable inputs and suggestions during the course of articulation of the article. PS and PM analysed the secondary data, wrote the draft manuscript, and developed the compiled tables and figures.
Funding
The authors are thankful to the Department of Science and Technology (DST), Government of India, for financial support under the Indo-Norway Binational Project entitled “Greenhouse gas emissions from biogas digestate application to rice production systems” (Project Grant No. Int/Nor/Rcn/Bio/P-03/2018).
Conflict of Interest
The authors declare that the research was conducted in the absence of any commercial or financial relationships that could be construed as a potential conflict of interest.
Publisher’s Note
All claims expressed in this article are solely those of the authors and do not necessarily represent those of their affiliated organizations, or those of the publisher, the editors, and the reviewers. Any product that may be evaluated in this article, or claim that may be made by its manufacturer, is not guaranteed or endorsed by the publisher.
Acknowledgements
The authors are also thankful to BDTC-KIIT and KIIT School of Biotechnology for extending the infrastructural facility and necessary administrative support.
References
Ambaye, T. G., Rene, E. R., Nizami, A.-S., Dupont, C., Vaccari, M., and van Hullebusch, E. D. (2021). Beneficial Role of Biochar Addition on the Anaerobic Digestion of Food Waste: A Systematic and Critical Review of the Operational Parameters and Mechanisms. J. Environ. Manag. 290, 112537. doi:10.1016/j.jenvman.2021.112537
Awasthi, M. K., Lukitawesa, L., Duan, Y., Taherzadeh, M. J., and Zhang, Z. (2022). Bacterial Dynamics during the Anaerobic Digestion of Toxic Citrus Fruit Waste and Semi-continues Volatile Fatty Acids Production in Membrane Bioreactors. Fuel 319, 123812. doi:10.1016/j.fuel.2022.123812
Awiszus, S., Meissner, K., Reyer, S., and Müller, J. (2018). Ammonia and Methane Emissions during Drying of Dewatered Biogas Digestate in a Two-Belt Conveyor Dryer. Bioresour. Technol. 247, 419–425. doi:10.1016/j.biortech.2017.09.099
Baroutian, S., Munir, M. T., Sun, J., Eshtiaghi, N., and Young, B. R. (2018). Rheological Characterisation of Biologically Treated and Non-treated Putrescible Food Waste. Waste Manag. 71, 494–501. doi:10.1016/j.wasman.2017.10.003
Bartocci, P., Zampilli, M., Liberti, F., Pistolesi, V., Massoli, S., Bidini, G., et al. (2020). LCA Analysis of Food Waste Co-digestion. Sci. Total Environ. 709, 136187. doi:10.1016/j.scitotenv.2019.136187
Bolzonella, D., Battista, F., Cavinato, C., Gottardo, M., Micolucci, F., Lyberatos, G., et al. (2018). Recent Developments in Biohythane Production from Household Food Wastes: a Review. Bioresour. Technol. 257, 311–319. doi:10.1016/j.biortech.2018.02.092
Chapman, J., Ismail, A., and Dinu, C. (2018). Industrial Applications of Enzymes: Recent Advances, Techniques, and Outlooks. Catalysts 8 (6), 238. doi:10.3390/catal8060238
Cheong, J. C., Lee, J. T. E., Lim, J. W., Song, S., Tan, J. K. N., Chiam, Z. Y., et al. (2020). Closing the Food Waste Loop: Food Waste Anaerobic Digestate as Fertilizer for the Cultivation of the Leafy Vegetable, Xiao Bai Cai (Brassica Rapa). Sci. Total Environ. 715, 136789. doi:10.1016/j.scitotenv.2020.136789
Chew, K. R., Leong, H. Y., Khoo, K. S., Vo, D.-V. N., Anjum, H., Chang, C.-K., et al. (2021). Effects of Anaerobic Digestion of Food Waste on Biogas Production and Environmental Impacts: a Review. Environ. Chem. Lett. 19 (4), 2921–2939. doi:10.1007/s10311-021-01220-z
Chuyen, H. V., Nguyen, M. H., Roach, P. D., Golding, J. B., and Parks, S. E. (2018). Microwave-assisted Extraction and Ultrasound-Assisted Extraction for Recovering Carotenoids from Gac Peel and Their Effects on Antioxidant Capacity of the Extracts. Food Sci. Nutr. 6 (1), 189–196. doi:10.1002/fsn3.546
Clercq, D., Wen, Z., Gottfried, O., Schmidt, F., and Fei, F. (2017). A Review of Global Strategies Promoting the Conversion of Food Waste to Bioenergy via Anaerobic Digestion. Renew. Sustain. Energy Rev. 79, 204–221. doi:10.1016/j.rser.2017.05.047
Cong, R.-G., and Thomsen, M. (2021). Review of Ecosystem Services in a Bio-Based Circular Economy and Governance Mechanisms. Ecosyst. Serv. 50, 101298. doi:10.1016/j.ecoser.2021.101298
Dahiya, S., Kumar, A. N., Shanthi Sravan, J., Chatterjee, S., Sarkar, O., and Mohan, S. V. (2018). Food Waste Biorefinery: Sustainable Strategy for Circular Bioeconomy. Bioresour. Technol. 248, 2–12. doi:10.1016/j.biortech.2017.07.176
De Menna, F., Dietershagen, J., Loubiere, M., and Vittuari, M. (2018). Life Cycle Costing of Food Waste: A Review of Methodological Approaches. Waste Manag. 73, 1–13. doi:10.1016/j.wasman.2017.12.032
Ding, Y., Zhao, J., Liu, J.-W., Zhou, J., Cheng, L., Zhao, J., et al. (2021). A Review of China's Municipal Solid Waste (MSW) and Comparison with International Regions: Management and Technologies in Treatment and Resource Utilization. J. Clean. Prod. 293, 126144. doi:10.1016/j.jclepro.2021.126144
Duan, Y., Mehariya, S., Kumar, A., Singh, E., Yang, J., Kumar, S., et al. (2021). Apple Orchard Waste Recycling and Valorization of Valuable Product-A Review. Bioengineered 12 (1), 476–495. doi:10.1080/21655979.2021.1872905
Duan, Y., Pandey, A., Zhang, Z., Awasthi, M. K., Bhatia, S. K., and Taherzadeh, M. J. (2020). Organic Solid Waste Biorefinery: Sustainable Strategy for Emerging Circular Bioeconomy in China. Industrial Crops Prod. 153, 112568. doi:10.1016/j.indcrop.2020.112568
Edwiges, T., Frare, L., Mayer, B., Lins, L., Mi Triolo, J., Flotats, X., et al. (2018). Influence of Chemical Composition on Biochemical Methane Potential of Fruit and Vegetable Waste. Waste Manag. 71, 618–625. doi:10.1016/j.wasman.2017.05.030
Elimelech, E., Ayalon, O., and Ert, E. (2018). What Gets Measured Gets Managed: A New Method of Measuring Household Food Waste. Waste Manag. 76, 68–81. doi:10.1016/j.wasman.2018.03.031
Esteban, J., and Ladero, M. (2018). Food Waste as a Source of Value-Added Chemicals and Materials: a Biorefinery Perspective. Int. J. Food Sci. Technol. 53 (5), 1095–1108. doi:10.1111/ijfs.13726
Fazzino, F., Mauriello, F., Paone, E., Sidari, R., and Calabrò, P. S. (2021). Integral Valorization of Orange Peel Waste through Optimized Ensiling: Lactic Acid and Bioethanol Production. Chemosphere 271, 129602. doi:10.1016/j.chemosphere.2021.129602
Franca, L. S., and Bassin, J. P. (2020). The Role of Dry Anaerobic Digestion in the Treatment of the Organic Fraction of Municipal Solid Waste: a Systematic Review. Biomass Bioenergy 143, 105866. doi:10.1016/j.biombioe.2020.105866
Gao, S., Lu, D., Qian, T., and Zhou, Y. (2021). Thermal Hydrolyzed Food Waste Liquor as Liquid Organic Fertilizer. Sci. Total Environ. 775, 145786. doi:10.1016/j.scitotenv.2021.145786
Garcia-Garcia, G., Woolley, E., Rahimifard, S., Colwill, J., White, R., and Needham, L. (2017). A Methodology for Sustainable Management of Food Waste. Waste Biomass Valor 8 (6), 2209–2227. doi:10.1007/s12649-016-9720-0
Garrido, T., Gizdavic-Nikolaidis, M., Leceta, I., Urdanpilleta, M., Guerrero, P., de la Caba, K., et al. (2019). Optimizing the Extraction Process of Natural Antioxidants from Chardonnay Grape Marc Using Microwave-Assisted Extraction. Waste Manag. 88, 110–117. doi:10.1016/j.wasman.2019.03.031
Grigatti, M., Barbanti, L., Hassan, M. U., and Ciavatta, C. (2020). Fertilizing Potential and CO2 Emissions Following the Utilization of Fresh and Composted Food-Waste Anaerobic Digestates. Sci. Total Environ. 698, 134198. doi:10.1016/j.scitotenv.2019.134198
Hagos, K., Zong, J., Li, D., Liu, C., and Lu, X. (2017). Anaerobic Co-digestion Process for Biogas Production: Progress, Challenges and Perspectives. Renew. Sustain. Energy Rev. 76, 1485–1496. doi:10.1016/j.rser.2016.11.184
Helenas Perin, J. K., Biesdorf Borth, P. L., Torrecilhas, A. R., Santana da Cunha, L., Kuroda, E. K., and Fernandes, F. (2020). Optimization of Methane Production Parameters during Anaerobic Co-digestion of Food Waste and Garden Waste. J. Clean. Prod. 272, 123130. doi:10.1016/j.jclepro.2020.123130
Herbes, C., Roth, U., Wulf, S., and Dahlin, J. (2020). Economic Assessment of Different Biogas Digestate Processing Technologies: A Scenario-Based Analysis. J. Clean. Prod. 255, 120282. doi:10.1016/j.jclepro.2020.120282
Jarunglumlert, T., Bampenrat, A., Sukkathanyawat, H., and Prommuak, C. (2021). Enhanced Energy Recovery from Food Waste by Co-production of Bioethanol and Biomethane Process. Fermentation 7 (4), 265. doi:10.3390/fermentation7040265
Jiang, J., Li, L., Cui, M., Zhang, F., Liu, Y., Liu, Y., et al. (2018). Anaerobic Digestion of Kitchen Waste: the Effects of Source, Concentration, and Temperature. Biochem. Eng. J. 135, 91–97. doi:10.1016/j.bej.2018.04.004
Jung, J.-H., Sim, Y.-B., Baik, J.-H., Park, J.-H., and Kim, S.-H. (2021). High-rate Mesophilic Hydrogen Production from Food Waste Using Hybrid Immobilized Microbiome. Bioresour. Technol. 320, 124279. doi:10.1016/j.biortech.2020.124279
Kanniah Goud, R., Arunasri, K., Yeruva, D. K., Krishna, K. V., Dahiya, S., and Mohan, S. V. (2017). Impact of Selectively Enriched Microbial Communities on Long-Term Fermentative Biohydrogen Production. Bioresour. Technol. 242, 253–264. doi:10.1016/j.biortech.2017.03.147
Karki, R., Chuenchart, W., Surendra, K. C., Shrestha, S., Raskin, L., Sung, S., et al. (2021). Anaerobic Co-digestion: Current Status and Perspectives. Bioresour. Technol. 330, 125001. doi:10.1016/j.biortech.2021.125001
Khan, S., Nisar, A., Wu, B., Zhu, Q.-L., Wang, Y.-W., Hu, G.-Q., et al. (2022). Bioenergy Production in Pakistan: Potential, Progress, and Prospect. Sci. Total Environ. 814, 152872. doi:10.1016/j.scitotenv.2021.152872
Kim, J. S., Lee, Y. Y., and Kim, T. H. (2016). A Review on Alkaline Pretreatment Technology for Bioconversion of Lignocellulosic Biomass. Bioresour. Technol. 199, 42–48. doi:10.1016/j.biortech.2015.08.085
Kim, M.-H., and Kim, J.-W. (2010). Comparison through a LCA Evaluation Analysis of Food Waste Disposal Options from the Perspective of Global Warming and Resource Recovery. Sci. Total Environ. 408 (19), 3998–4006. doi:10.1016/j.scitotenv.2010.04.049
Kumar, R., Kim, T. H., Basak, B., Patil, S. M., Kim, H. H., Ahn, Y., et al. (2022). Emerging Approaches in Lignocellulosic Biomass Pretreatment and Anaerobic Bioprocesses for Sustainable Biofuels Production. J. Clean. Prod. 333, 130180. doi:10.1016/j.jclepro.2021.130180
Li, K., Wang, K., Wang, J., Yuan, Q., Shi, C., Wu, J., et al. (2020). Performance Assessment and Metagenomic Analysis of Full-Scale Innovative Two-Stage Anaerobic Digestion Biogas Plant for Food Wastes Treatment. J. Clean. Prod. 264, 121646. doi:10.1016/j.jclepro.2020.121646
Li, X., Guo, S., Peng, Y., He, Y., Wang, S., Li, L., et al. (2018). Anaerobic Digestion Using Ultrasound as Pretreatment Approach: Changes in Waste Activated Sludge, Anaerobic Digestion Performances and Digestive Microbial Populations. Biochem. Eng. J. 139, 139–145. doi:10.1016/j.bej.2017.11.009
Li, Y., Jin, Y., Borrion, A., Li, H., and Li, J. (2017). Effects of Organic Composition on Mesophilic Anaerobic Digestion of Food Waste. Bioresour. Technol. 244, 213–224. doi:10.1016/j.biortech.2017.07.006
Li, Y., and Jin, Y. (2015). Effects of Thermal Pretreatment on Acidification Phase during Two-phase Batch Anaerobic Digestion of Kitchen Waste. Renew. Energy 77, 550–557. doi:10.1016/j.renene.2014.12.056
Li, Y., Jin, Y., Li, H., Borrion, A., Yu, Z., and Li, J. (2018). Kinetic Studies on Organic Degradation and its Impacts on Improving Methane Production during Anaerobic Digestion of Food Waste. Appl. Energy 213, 136–147. doi:10.1016/j.apenergy.2018.01.033
Liu, C., Ren, L., Yan, B., Luo, L., Zhang, J., and Awasthi, M. K. (2021). Electron Transfer and Mechanism of Energy Production Among Syntrophic Bacteria during Acidogenic Fermentation: A Review. Bioresour. Technol. 323, 124637. doi:10.1016/j.biortech.2020.124637
Liu, C., Wang, W., Anwar, N., Ma, Z., Liu, G., and Zhang, R. (2017). Effect of Organic Loading Rate on Anaerobic Digestion of Food Waste under Mesophilic and Thermophilic Conditions. Energy fuels. 31 (3), 2976–2984. doi:10.1021/acs.energyfuels.7b00018
Logan, M., and Visvanathan, C. (2019). Management Strategies for Anaerobic Digestate of Organic Fraction of Municipal Solid Waste: Current Status and Future Prospects. Waste Manag. Res. 37 (1_Suppl. l), 27–39. doi:10.1177/0734242X18816793
Lohani, S. P., Shakya, S., Gurung, P., Dhungana, B., Paudel, D., and Mainali, B. (2021). Anaerobic Co-digestion of Food Waste, Poultry Litter and Sewage Sludge: Seasonal Performance under Ambient Condition and Model Evaluation. Energy Sources, Part A Recovery, Util. Environ. Eff., 1–16. doi:10.1080/15567036.2021.1887976
Lytras, G., Lytras, C., Mathioudakis, D., Papadopoulou, K., and Lyberatos, G. (2021). Food Waste Valorization Based on Anaerobic Digestion. Waste Biomass Valor 12 (4), 1677–1697. doi:10.1007/s12649-020-01108-z
Ma, Y., and Liu, Y. (2019). Turning Food Waste to Energy and Resources towards a Great Environmental and Economic Sustainability: An Innovative Integrated Biological Approach. Biotechnol. Adv. 37 (7), 107414. doi:10.1016/j.biotechadv.2019.06.013
Mahidhara, G., Burrow, H., Sasikala, C., and Ramana, C. V. (2019). Biological Hydrogen Production: Molecular and Electrolytic Perspectives. World J. Microbiol. Biotechnol. 35 (8), 1–13. doi:10.1007/s11274-019-2692-z
Mata, T. M., Martins, A. A., and Caetano, N. S. (2018). Bio-refinery Approach for Spent Coffee Grounds Valorization. Bioresour. Technol. 247, 1077–1084. doi:10.1016/j.biortech.2017.09.106
Michalopoulos, I., Lytras, G. M., Mathioudakis, D., Lytras, C., Goumenos, A., Zacharopoulos, I., et al. (2020). Hydrogen and Methane Production from Food Residue Biomass Product (FORBI). Waste Biomass Valor 11 (5), 1647–1655. doi:10.1007/s12649-018-00550-4
Mirmohamadsadeghi, S., Karimi, K., Tabatabaei, M., and Aghbashlo, M. (2019). Biogas Production from Food Wastes: A Review on Recent Developments and Future Perspectives. Bioresour. Technol. Rep. 7, 100202. doi:10.1016/j.biteb.2019.100202
Mishra, A., Kumar, M., Bolan, N. S., Kapley, A., Kumar, R., and Singh, L. (2021). Multidimensional Approaches of Biogas Production and Up-Gradation: Opportunities and Challenges. Bioresour. Technol. 338, 125514. doi:10.1016/j.biortech.2021.125514
Mishra, S., Adhya, T. K., and Ojha, S. K. (2019). Biogas Technology. New Delhi: New India Publishing Agency, 366.
Mishra, S., Singh, P. K., Dash, S., and Pattnaik, R. (2018). Microbial Pretreatment of Lignocellulosic Biomass for Enhanced Biomethanation and Waste Management. 3 Biotech. 8 (11), 1–12. doi:10.1007/s13205-018-1480-z
Mishra, S., Singh, P. K., Mohanty, P., Adhya, T. K., Sarangi, P. K., Srivastava, R. K., et al. (2022). Green Synthesis of Biomethanol-Managing Food Waste for Carbon Footprint and Bioeconomy. Biomass Conv. bioref. 12, 1889–1909. doi:10.1007/s13399-021-02188-0
Mohan, S. V., Chiranjeevi, P., Dahiya, S., and Kumar., N. A. (2018). Waste Derived Bioeconomy in India: a Perspective. New Biotechnol. 40, 60–69. doi:10.1016/j.nbt.2017.06.006
Mohanty, P., Singh, P. K., Adhya, T. K., Pattnaik, R., and Mishra, S. (2021). A Critical Review on Prospects and Challenges in Production of Biomethanol from Lignocellulose Biomass. Biomass Conv. bioref. 12, 1835–1849. doi:10.1007/s13399-021-01815-0
Nayak, A., and Bhushan, B. (2019). An Overview of the Recent Trends on the Waste Valorization Techniques for Food Wastes. J. Environ. Manag. 233, 352–370. doi:10.1016/j.jenvman.2018.12.041
Nhubu, T., Muzenda, E., Mbohwa, C., and Agbenyeku, E. O. M. (2020). Comparative Assessment of Compositing and Anaerobic Digestion of Municipal Biodegradable Waste in Harare, Zimbabwe. Environ. Prog. Sustain. Energy 39 (4), e13376. doi:10.1002/ep.13376
Nielsen, C., Rahman, A., Rehman, A. U., Walsh, M. K., and Miller, C. D. (2017). Food Waste Conversion to Microbial Polyhydroxyalkanoates. Microb. Biotechnol. 10 (6), 1338–1352. doi:10.1111/1751-7915.12776
Nordahl, S. L., Devkota, J. P., Amirebrahimi, J., Smith, S. J., Breunig, H. M., Preble, C. V., et al. (2020). Life-cycle Greenhouse Gas Emissions and Human Health Trade-Offs of Organic Waste Management Strategies. Environ. Sci. Technol. 54 (15), 9200–9209. doi:10.1021/acs.est.0c00364
Parthiba Karthikeyan, O., Trably, E., Mehariya, S., Bernet, N., Wong, J. W. C., and Carrere, H. (2018). Pretreatment of Food Waste for Methane and Hydrogen Recovery: a Review. Bioresour. Technol. 249, 1025–1039. doi:10.1016/j.biortech.2017.09.105
Pramanik, S. K., Suja, F. B., Zain, S. M., and Pramanik, B. K. (2019). The Anaerobic Digestion Process of Biogas Production from Food Waste: Prospects and Constraints. Bioresour. Technol. Rep. 8, 100310. doi:10.1016/j.biteb.2019.100310
Rasapoor, M., Adl, M., Baroutian, S., Iranshahi, Z., and Pazouki, M. (2019). Energy Performance Evaluation of Ultrasonic Pretreatment of Organic Solid Waste in a Pilot-Scale Digester. Ultrason. Sonochemistry 51, 517–525. doi:10.1016/j.ultsonch.2018.04.021
Raza, Z. A., Abid, S., and Banat, I. M. (2018). Polyhydroxyalkanoates: Characteristics, Production, Recent Developments and Applications. Int. Biodeterior. Biodegrad. 126, 45–56. doi:10.1016/j.ibiod.2017.10.001
Ren, Y., Yu, M., Wu, C., Wang, Q., Gao, M., Huang, Q., et al. (2018). A Comprehensive Review on Food Waste Anaerobic Digestion: Research Updates and Tendencies. Bioresour. Technol. 247, 1069–1076. doi:10.1016/j.biortech.2017.09.109
Sengar, A. S., Rawson, A., Muthiah, M., and Kalakandan, S. K. (2020). Comparison of Different Ultrasound Assisted Extraction Techniques for Pectin from Tomato Processing Waste. Ultrason. Sonochemistry 61, 104812. doi:10.1016/j.ultsonch.2019.104812
Shi, X., Guo, X., Zuo, J., Wang, Y., and Zhang, M. (2018). A Comparative Study of Thermophilic and Mesophilic Anaerobic Co-digestion of Food Waste and Wheat Straw: Process Stability and Microbial Community Structure Shifts. Waste Manag. 75, 261–269. doi:10.1016/j.wasman.2018.02.004
Singh, P. K., Srichandan, H., Ojha, S. K., Pattnaik, R., Verma, S. K., Pal, S., et al. (2021). Evaluation of Biomethane Potential of Codigested Sheep Manure and Kitchen Refuse. Biomass Conv. bioref., 1–11. doi:10.1007/s13399-021-01961-5
Slorach, P. C., Jeswani, H. K., Cuéllar-Franca, R., and Azapagic, A. (2019). Environmental Sustainability of Anaerobic Digestion of Household Food Waste. J. Environ. Manag. 236, 798–814. doi:10.1016/j.jenvman.2019.02.001
Srivastava, R. K., Shetti, N. P., Reddy, K. R., and Aminabhavi, T. M. (2020). Biofuels, Biodiesel and Biohydrogen Production Using Bioprocesses. A Review. Environ. Chem. Lett. 18 (4), 1049–1072. doi:10.1007/s10311-020-00999-7
Tsegaye, B., Jaiswal, S., and Jaiswal, A. K. (2021). Food Waste Biorefinery: Pathway towards Circular Bioeconomy. Foods 10 (6), 1174. doi:10.3390/foods10061174
Usmani, Z., Sharma, M., Awasthi, A. K., Sharma, G. D., Cysneiros, D., Nayak, S. C., et al. (2021). Minimizing Hazardous Impact of Food Waste in a Circular Economy - Advances in Resource Recovery through Green Strategies. J. Hazard. Mater. 416, 126154. doi:10.1016/j.jhazmat.2021.126154
Venkata Mohan, S., Dahiya, S., Amulya, K., Katakojwala, R., and Vanitha, T. K. (2019). Can Circular Bioeconomy Be Fueled by Waste Biorefineries - A Closer Look. Bioresour. Technol. Rep. 7, 100277. doi:10.1016/j.biteb.2019.100277
Von Massow, M., Parizeau, K., Gallant, M., Wickson, M., Haines, J., Ma, D. W. L., et al. (2019). Valuing the Multiple Impacts of Household Food Waste. Front. Nutr. 6, 143. doi:10.3389/fnut.2019.00143
Wainaina, S., Awasthi, M. K., Sarsaiya, S., Chen, H., Singh, E., Kumar, A., et al. (2020). Resource Recovery and Circular Economy from Organic Solid Waste Using Aerobic and Anaerobic Digestion Technologies. Bioresour. Technol. 301, 122778. doi:10.1016/j.biortech.2020.122778
Wainaina, S., LukitawesaKumar Awasthi, M., Kumar Awasthi, M., and Taherzadeh, M. J. (2019). Bioengineering of Anaerobic Digestion for Volatile Fatty Acids, Hydrogen or Methane Production: A Critical Review. Bioengineered 10 (1), 437–458. doi:10.1080/21655979.2019.1673937
Xiao, B., Qin, Y., Wu, J., Chen, H., Yu, P., Liu, J., et al. (2018). Comparison of Single-Stage and Two-Stage Thermophilic Anaerobic Digestion of Food Waste: Performance, Energy Balance and Reaction Process. Energy Convers. Manag. 156, 215–223. doi:10.1016/j.enconman.2017.10.092
Xiao, B., Zhang, W., Yi, H., Qin, Y., Wu, J., Liu, J., et al. (2019). Biogas Production by Two-Stage Thermophilic Anaerobic Co-digestion of Food Waste and Paper Waste: Effect of Paper Waste Ratio. Renew. Energy 132, 1301–1309. doi:10.1016/j.renene.2018.09.030
Xu, F., Li, Y., Ge, X., Yang, L., and Li, Y. (2018). Anaerobic Digestion of Food Waste - Challenges and Opportunities. Bioresour. Technol. 247, 1047–1058. doi:10.1016/j.biortech.2017.09.020
Yahmed, N. B., Dauptain, K., Lajnef, I., Carrere, H., Trably, E., and Smaali, I. (2021). New Sustainable Bioconversion Concept of Date By-Products (Phoenix Dactylifera L.) to Biohydrogen, Biogas and Date-Syrup. Int. J. Hydrogen Energy 46 (1), 297–305. doi:10.1016/j.ijhydene.2020.09.203
Keywords: food waste, anaerobic digestion, biofuel, biogas, circular economy, organic fertiliser
Citation: Singh PK, Mohanty P, Mishra S and Adhya TK (2022) Food Waste Valorisation for Biogas-Based Bioenergy Production in Circular Bioeconomy: Opportunities, Challenges, and Future Developments. Front. Energy Res. 10:903775. doi: 10.3389/fenrg.2022.903775
Received: 24 March 2022; Accepted: 18 May 2022;
Published: 11 August 2022.
Edited by:
Lu Feng, Norwegian Institute of Bioeconomy Research (NIBIO), NorwayReviewed by:
Dillirani Nagarajan, National Cheng Kung University, TaiwanMukesh Kumar Awasthi, Northwest A&F University, China
Copyright © 2022 Singh, Mohanty, Mishra and Adhya. This is an open-access article distributed under the terms of the Creative Commons Attribution License (CC BY). The use, distribution or reproduction in other forums is permitted, provided the original author(s) and the copyright owner(s) are credited and that the original publication in this journal is cited, in accordance with accepted academic practice. No use, distribution or reproduction is permitted which does not comply with these terms.
*Correspondence: Snehasish Mishra, snehasish.mishra@gmail.com; Tapan Kumar Adhya, adhyas@yahoo.com
†These authors have contributed equally to this work and share first authorship