Design of triple cogeneration system for hydrogen fuel cell in greenhouse based on resource analysis
- 1Advanced Vehicle Design and Control LAB, Department of Mechanical Engineering, Inha University, Incheon, Republic of Korea
- 2School of Automation, Chongqing University of Posts and Telecommunications, Chongqing, China
Introduction: Hydrogen energy has a massive advantage in the energy supply mode regarding greenhouse gas emissions. Nevertheless, the energy supply system needs to be redesigned to meet the heating/cooling needs and the goals of using hydrogen energy.
Methods: The comprehensive utilization efficiency of system energy can be improved using resource analysis to solve the problem of waste heat and formulate a technical plan for triple cogeneration of hydrogen greenhouse.
Results: By calculation, the energy efficiency of the coal boiler heating system, the hydrogen energy heating system and the hydrogen greenhouse triple heating system are 65%, 52.93%, and 73.36%, respectively. Compared to the coal boiler heating system and the hydrogen energy heating system, the advantages of the hydrogen greenhouse triple cogeneration system are that the comprehensive utilization efficiency of the system energy has been improved, and no CO2 is generated when it works, which does not cause pressure on environmental protection. At the same time, the exergy efficiency of the hydrogen greenhouse triple cogeneration system is higher. Currently, due to the high price of hydrogen and fuel cells, the economic advantages are not outstanding. Still, coal energy is expected to be replaced in the future with the continuous decline in the cost of hydrogen and fuel cells. According to the current coal price, when the hydrogen price decreases to below $1.3/kg, coal will lose its price advantage in terms of fuel. At the same time, hydrogen has a high energy density, small volume, and low transportation cost. According to IRENA and Hydrogen Council, the cost of producing hydrogen from renewable energy will drop to $1/kg by 2050. The system will significantly reduce the operating costs of greenhouses in the future.
Discussion: As a result, the hydrogen greenhouse triple cogeneration system is suitable for producing greenhouse agriculture and the heating method of fossil fuel combustion that will replace coal in the future.
1 Introduction
In the context of the growing global energy crisis (Berahab, 1967), non-renewable resources, such as oil and coal, are facing depletion (Bekhet and Harun, 2016). Because of this situation, the importance of renewable energy (for example, solar energy, hydrogen, and wind energy) is growing (Petrović-Ranđelović et al., 2020) and the speed of development and utilization of renewable energy needs to be accelerated (Kataria and Khan, 2021). For example, the energy balance needs to be optimized. In addition, the dependence on non-renewable energy needs to be reduced, and the proportion of renewable energy in total energy consumption needs to be increased (Qiu et al., 2021). The agricultural production process also requires considerable energy. Currently, the main form of energy dependence is non-renewable resources, such as coal or oil, and the utilization of renewable energy sources, such as hydrogen and solar power, is lower. In agricultural production, greenhouses are widely used to promote crop growth and increase crop yield, particularly in high-latitude regions. According to the data, even if various energy-saving technologies are adopted, the energy consumed by greenhouse heating still accounts for more than 70% of the total energy consumed by agriculture (Djevic and Dimitrijevic, 2004; Sanford, 2011; Ahamed et al., 2019a). Therefore, research on greenhouse energy technology plays a vital role in optimizing the agricultural energy structure.
Energy sources, such as wind energy and solar energy, are greatly affected by the climate and region and cannot supply power continuously (Lakatos et al., 2011). The indoor environment of the greenhouse cannot be maintained within a specific temperature range to ensure the average growth of crops. For example, in poor weather, such as rain and snow, or at night, solar panels will not generate electricity, but this is when the greenhouse needs more energy supply. If the energy supply continues to rely on non-renewable resources, such as coal or oil, this way of using renewable energy will have little effect on improving the energy structure. To solve the uneven temporal–spatial distribution of renewables, hydrogen can be used as short- and long-term energy storage mediums (Wen and Aziz, 2022). Hydrogen is the ultimate solution to energy problems in the 21st century. Hydrogen energy is clean energy with abundant reserves. On the other hand, hydrogen energy has a high energy density and is convenient for storage and transportation (Jain, 2009).
Therefore, hydrogen energy can be used as an energy supply for greenhouses. The transformation of the energy supply mode and the improvement of the energy utilization rate will have a more significant impact on the energy conservation of greenhouses.
Regarding the research on the utilization of greenhouse energy, the current primary studies focus on greenhouse energy management, energy alternatives, energy-saving technologies, waste heat utilization, and other aspects. Among them, Zhuang P et al. proposed a stochastic multi-timescale energy management scheme of greenhouses with renewable energy sources, but the optimal choice of the time scale for each energy path is a problem (Zhuang et al., 2018); Vourdoubas J operated a floral greenhouse heated with olive stone wood, proving that this solid fuel is an inexpensive energy source that can meet all the heating needs of the greenhouse while reducing CO2 emissions from energy use, but it is not possible to achieve zero carbon dioxide emissions (Vourdoubas, 2015); Ahamed M S et al. summarizes the different energy-saving technologies that can be used to reduce the cost of heating greenhouses but does not fundamentally solve the problems existing in the way energy is supplied (Ahamed et al., 2019b); Denzer A et al. proposed the use of industrial waste heat to provide greenhouse heating, which can reduce the energy consumption of the greenhouse, but it is not suitable for long-distance waste heat utilization (Denzer et al., 2017); The above research on the application of renewable energy in greenhouses considers the energy saving of the greenhouse energy system from one or several aspects. Nevertheless, these studies did not consider the comprehensive utilization of renewable energy in the system. Only from the overall consideration of the energy system can the system energy be optimized; the energy utilization efficiency of the system can be improved. In terms of energy utilization of the system, particularly the operation of the air conditioning system, combined with the energy usage, the triple thermal system can improve the energy efficiency of the system by more than 20% (Jabari et al., 2018; Leonzio, 2018; Gholizadeh et al., 2019; Zhang et al., 2020a). Therefore, in the design process of the greenhouse energy system, a triple cogeneration system can be used to improve the utilization efficiency of the system energy. In addition, the resource analysis method in TRIZ provides an adequate scientific tool (Ilevbare et al., 2013) and the objective should be to use the least amount of resources, cheapest resources, readily available resources, and resources available within the system (Mishra, 2212). Using this tool, energy utilization can be considered for the overall system, and system energy can be utilized to the maximum extent to avoid energy waste. Therefore, the advantage of this study is that it considers resource utilization on the whole from the design stage and uses the waste heat generated by the fuel cell itself to improve the energy utilization efficiency of the system through the triple cogeneration system, avoiding the increase in operating costs and heat loss due to long-distance transportation of industrial waste heat. The aim is to achieve the purpose of optimizing the greenhouse energy system.
2 System description and analysis method
2.1 Greenhouse energy system
The primary function of the greenhouse is to protect crops from overheating or undercooling and to provide a suitable growth environment according to the crop type and growth habits (Zhang et al., 2020b). The realization of this function needs to rely on heating or cooling equipment, which is also why the greenhouse consumes considerable energy. The energy consumed by greenhouse heating accounts for approximately 65%–85% of the total energy consumption. In addition to the labor costs, energy costs account for about 70%–85% of the total cost (Runkle and Both, 2011; Ahamed et al., 2019c). Based on the above situation, the research on the temperature control module helps reduce operating energy consumption and improve energy utilization efficiency.
The energy systems of greenhouses are slightly different, but the energy supply principle is similar. The greenhouse energy systems mainly include energy supply devices, cooling/heating equipment, environmental sensors, cooling/heating devices, and transportation pipelines, as shown in Figure 1. Its working process is as follows. First, the energy supply device provides energy. According to the feedback information from the environmental sensor, the refrigeration equipment will work when the temperature in the greenhouse is higher than the set temperature range, and the cold air will be sent into the greenhouse through the transmission pipeline. Once it reaches the set temperature range, it will stop working; the heating equipment will work in the opposite way.
Energy systems analysis is the study of energy use, energy production, and energy conversion in society (Blok and Nieuwlaar, 2016). By analyzing the energy system, rational allocation can optimize and improve energy utilization efficiency. Because the energy consumed by the temperature control module in the greenhouse accounts for 65%–85% of the total energy consumption, there are many greenhouses, and their functions and structures are slightly different. Still, the standard part is the temperature control module. To simplify the research model, the temperature control module of the greenhouse was taken as the research focus. Figure 2 presents the primary energy utilization forms in the greenhouse energy system. Greenhouse energy system mainly includes energy utilization ways of non-renewable resources and renewable resources. For example, in renewable energy utilization, hydrogen is discharged through the fuel cell to operate the air conditioner and provide cooling or heating for the greenhouse.
Triple cogeneration is an effective way to improve energy efficiency. Triple cogeneration refers to simultaneous electricity production and proper heat and cooling from fuel combustion or solar collectors. Compared to the traditional single mode, the triple cogeneration mode has higher efficiency, lower cost, and can reduce greenhouse gas emissions (Isa et al., 2018). The triple cogeneration system uses low-grade waste heat in the power generation process for heating or cooling, significantly saving system energy (Wang et al., 2011). The triple cogeneration system is relatively mature in applying high-parameter large-capacity units, for example, a novel triple pressure HRSG integrated with MED/SOFC/GT for cogeneration of electricity and freshwater (Vojdani et al., 2021),a thermodynamic cycle integrating a dual and a triple-pressure cogeneration cycle (Shankar and Rivera, 2022), etc. Many scholars are actively exploring and researching small methods that do not require long-distance transportation and have high energy efficiency.
2.2 Resource analysis
There are available resources for any system that does not reach the ideal solution (Liu et al., 2011), and by the ideal solution, it helps us to improve an existing system (Mishra, 2273). These available resources are used to promote the continuous improvement of the idealization of the system. Therefore, in the system design process, the rational use of resources makes the system easier to approach the ideal solution and obtain better benefits. Scientifically analyzing and utilizing system resources means that it is necessary to understand the classification of resources (Mueller, 2005), ways to find resources, and ways of using resources (De Carvalho and Back, 1999).
System resources can be divided into eight categories: nature, space, time, system, material, energy, information, and function. At the same time, the classification of resources can also be simplified into available resources, derived resources, and differential resources. Table 1 lists the concepts and characteristics. In the system design process, the comprehensive utilization method of system resources, as shown in Figure 3, is used to find and utilize resources conveniently. This method is used to obtain available system resources and solve problems in the system. Resources can improve the idealization level of the system and realize the innovation of system design (Liang et al., 2013). The utilization of system resources can be considered from the following perspectives.
Prioritize all resources to the most critical subsystems or functions as much as possible.
Make use of the resources lost or wasted by other processes as much as possible and tap and utilize the hidden and idle resources of the system.
Consider resource utilization from the perspective of system integrity and pay attention to the dynamic characteristics of various parts of the system.
Readily available and inexpensive resources should be used as much as possible to meet the requirements for use.
In the context of green design, more consideration should be given to the utilization of renewable resources while paying attention to the impact of waste on the environment.
3 Design of heating system
3.1 Design of traditional greenhouse energy system
The energy supply of traditional greenhouses uses boilers to burn coal or coal power to drive heating equipment to provide energy. Figure 4 shows the energy system of a boiler burning coal to provide energy as an example.
The energy supply system has the advantages of being simple in structure and low in cost. On the other hand, coal combustion releases sulfur dioxide, carbon monoxide, soot, radioactive dust, nitrogen oxides, and carbon dioxide (Hendryx et al., 2020). These substances harm human health (Burt et al., 2013), produce acid rain, and have a greenhouse effect. Therefore, this energy system should use renewable, clean energy as an energy supply. Renewable energy sources, such as solar, wind, and tidal energy, are greatly affected by climate and region and cannot provide a continuous energy supply to crop growth and crop yield (Kosonen, 2018). The cost of establishing energy storage devices is relatively high. By contrast, hydrogen energy has two significant advantages as an energy source. First, hydrogen energy has high energy density (the calorific value per unit mass is approximately four times that of coal, 3.1 times that of gasoline, and 2.6 times that of natural gas). Second, hydrogen energy can be stored and transported, providing energy stably and efficiently without polluting emissions. Given the above situation, hydrogen energy can be used as an alternative energy source for greenhouses (Wang et al., 2022a).
3.2 Design of hydrogen greenhouse energy system
A redesign is required to meet the design requirements to use hydrogen energy as an energy supply method for the greenhouse energy system. Hydrogen energy cannot directly provide power to the greenhouse, but hydrogen energy can be converted into electricity through hydrogen fuel cells. According to the different electrolytes, fuel cells are divided into proton exchange membrane fuel cells (PEMFC), molten carbonate fuel cells (MCFC), phosphoric acid fuel cells (PAFC), solid oxide fuel cells (SOFC), and alkaline fuel cells (AFC) (Gasik, 2008). PEMFC batteries are used widely because of the low operating temperature, short start-up time, and low oxidant requirements. Therefore, PEMFC is used as the energy supply of a system (Wee, 2007). Using PEMFC as an energy supply requires redesigning the greenhouse energy system to meet the heating and cooling requirements and provide a suitable growth environment for plants in the greenhouse. A design scheme is shown in Figure 5. The system is supplied with hydrogen by a hydrogen storage device, and the oxidation reaction of the PEMFC generates electricity to operate the air-conditioning system. The air-conditioning system produces heating/cooling to adjust the greenhouse’s temperature. According to the feedback signal from the sensor, the start and stop of the fuel cell and the air conditioning system are controlled. The system is supplied with hydrogen by a hydrogen storage device, and the oxidation reaction of the proton membrane exchange fuel cell generates electricity to supply the air-conditioning system to operate. The air conditioning system produces heating/cooling to adjust the greenhouse temperature. According to the feedback signal from the sensor, the start and stop of the fuel cell and the air conditioning system are controlled.
Hydrogen energy is a renewable energy source, and the use of hydrogen energy can effectively alleviate the energy crisis. The hydrogen fuel cell only produces water during the working process, which does not cause pollution to the environment and belongs to clean energy. At the same time, compared with other energy sources, the energy efficiency of internal combustion engines is 20%–37% (Albatayneh et al., 2020), while the energy efficiency of hydrogen fuel cells alone is 50%–60% (Singla et al., 2021), which is a high-efficiency energy source. Therefore, the hydrogen greenhouse energy system meets the high efficiency and cleanliness design requirements.
3.3 Design of triple cogeneration system in hydrogen greenhouse
Resource analysis is an effective means of improving system energy utilization efficiency and it has advantages in sustainable energy utilization (Spreafico, 2021) and sustainable development (Liu et al., 2020). At the same time, it is also possible to analyze the hydrogen greenhouse energy system through resource analysis, understand the resource allocation, and realize the specific functions through the combination of resource forms. Therefore, the resource analysis of the hydrogen greenhouse energy system is carried out, the utilization form of the system resources is comprehensively considered, and the system’s efficiency is maximized under the condition that the design requirements are met.
The hydrogen greenhouse energy system was analyzed according to the resource analysis method; Figure 6 shows the resource utilization form. After analyzing the resource utilization form of the system, a large amount of heat is generated during the discharge process of the hydrogen fuel cell, resulting in a waste of resources, and the need for cooling to avoid excessive temperature. According to the resource utilization method, the system’s resource utilization efficiency can be improved by reusing the waste resources of the system. The purpose of the greenhouse energy system design is to control the temperature of the greenhouse, and waste heat can be used as the heat source for heating the greenhouse to increase the temperature in the greenhouse. At the same time, heat is also generated during cooling, but it cannot be used directly. To use this part of the energy, the adsorption refrigerator method can cause cold air in the greenhouse to cool down. This method can solve the waste heat utilization problem and improve the comprehensive energy utilization efficiency of the system.
The greenhouse energy system was designed according to the strategy of the triple cogeneration system (Asgari et al., 2021). The greenhouse energy system was comprised mainly of a proton membrane exchange fuel cell device, an electric heat pump (EHP), an adsorption refrigerator (AR), and a delivery pipeline, as shown in Figure 7. The working process can be described as hydrogen being stored in a storage tank; the PEMFC discharge process consumes hydrogen, generates electricity and waste heat. The waste heat is taken away by cooling water. When the system needs cooling, the EHP starts working, and the hot wastewater is converted to cold air by the adsorption refrigeration system. The EHP with waste heat water starts working when the system requires heating. It can heat the greenhouse as a heat source for the EHP. The waste heat has been utilized effectively, which is of great help for improving the comprehensive utilization efficiency of system energy.
4 Energy system evaluation and simulation
To verify the feasibility of the hydrogen greenhouse triple cogeneration system, the characteristics of the three systems (traditional greenhouse energy system, hydrogen greenhouse energy system, and hydrogen greenhouse triple cogeneration system) were considered from the four aspects of energy, exergy, environment, and economy (Colakoglu and Durmayaz, 2022).
4.1 Energy analysis
Energy efficiency refers to the proportion of energy used in energy and can also measure the level and economy of system energy utilization technology. The energy efficiency of a system is calculated using the primary energy ratio (PER).
The primary energy efficiency calculation formula of the boiler system (Liu et al., 2017) is
where η boiler is the boiler efficiency and η fuel, trans is the fuel transfer efficiency. The process of using boiler heating, pipeline transmission, and radiator will cause the total energy efficiency of the system to be lower than that of the PER boiler. The primary energy utilization efficiency of bulk coal is 40% (Cheng et al., 2017), and the heating efficiency of coal-fired heating boilers is 60%–70% (Xu et al., 2014; Chao et al., 2017). For the convenience of research, the energy efficiency PER boiler, total of the greenhouse boiler is approximately 65%.
The hydrogen greenhouse energy system comprises the PEMFC and air conditioning subsystem. Therefore, the calculation method for the total efficiency of the hydrogen greenhouse energy system is as follows:
The efficiency of PEMFC alone power generation is 50%–60% (Barbir and Gomez, 1997; Hwang, 2013), taking η PEMFC as 55%. Owing to the use of different technical means, the energy conversion efficiency of each brand of air conditioner is slightly different. An air conditioner system with a higher energy efficiency level was adopted to improve the energy efficiency of the system. The energy conversion efficiency of the inverter air conditioning system was 95.48%–96.5%, and the average efficiency was 96.25% (Wu et al., 2021), taking η air conditioning as 96.25%. According to Eq. 2, the total efficiency of the hydrogen greenhouse energy system was 52.93%. Although the hydrogen greenhouse energy system changed the energy structure, the energy utilization rate is low, and part of the energy is lost in the form of heat.
The working process of the hydrogen greenhouse triple-cogeneration system is that the PEMFC produces electricity and heat through redox reactions. When cooling is required, the electric heat pump system uses electricity to generate cold air to cool the greenhouse. At the same time, the waste hot water generated by the PEMFC is converted to cold air using an adsorption refrigerator. Taking the system cooling work as an example, the method to calculate the total efficiency of the system is as follows:
Part of the heat output by the electric heat pump unit during operation comes from the electric energy consumed and the heat obtained from the low-temperature environment. When the energy lost by the system is less than the energy obtained from the environment, the heat generated by the combustion of the primary fuel consumed by the unit heating (or cooling capacity) is called the introductory energy utilization rate. Therefore, the direct energy utilization efficiency of the heat pump will be greater than 1.
In this situation, Fu Yongzheng et al. (Fu and Liang, 2022) reported that when the COP (coefficient of performance) = 3, the primary energy efficiency of the electric heat pump unit is approximately 100%, and η heat pump is taken as 100%; COP = 4, the primary energy efficiency of the electric heat pump unit is approximately 150%, and the η heat pump is taken as 150%. The waste heat recovery efficiency is 80%–90% (Hydrogen Energy [EB/OL], 2022), and η waste heating is 85%. The energy efficiency of the adsorption refrigerator system is 38%–58% (Saha et al., 2003; Sztekler et al., 2020; Chauhan et al., 2022), and the η adsorption refrigerator is 48%. According to Equation 3, when COP = 3 (low performance) and the η heat pump is taken as 100%, the PER hydrogen triple cogeneration can be calculated to be 73.36%; when COP = 4 (high performance), when the η heat pump is taken as 150%, the calculated PER hydrogen triple cogeneration is 100.86%. Combined with the above analysis, the advantage of the hydrogen greenhouse triple-cogeneration system lies in the high energy utilization rate of the system, which is of great help in changing the energy supply structure and saving energy.
4.2 Exergy analysis
Exergy is the maximum work potential of a system, stream of matter, or a heat interaction as the datum state about the reference environment (Rosen, 2004), which can be used to evaluate energy value independently. Exergy efficiency is an index used to measure the degree of energy conversion and system thermodynamic perfection, and it is one of the commonly used evaluation indexes for exergy analysis. Suppose the exergy balance relationship is to be established. In that case, not only the input and output energy of the system must be considered, but also the exergy loss caused by irreversible factors during the energy conversion process. Taking a steady-state steady-flow control body as an example to illustrate the exergy balance equation, the relationship between its various parts is shown in Figure 8 (Zhou, 2021).
The exergy balance equation is as follows:
Where E
According to Eq. 4, the advantage of exergy analysis is that not only the adequate energy of the input and output of the system must be considered, but also the losses caused by irreversible factors inside the system (such as heat transfer, friction, etc.) The source of inefficiency provides a way and direction for making full use of energy quality. Comparing the advantages of different energy systems, it is more conducive to making decisions based on the calculation results and actual needs.
Exergy efficiency can more directly reflect the degree of energy utilization. Exergy efficiency is the ratio of system revenue exergy to cost exergy. Its expression is as follows:
Where E obtained is exergy of the system used to achieve the purpose of energy utilization (income exergy) and E consumed is exergy paid by the system to achieve the purpose of energy utilization (cost exergy).
In Eq. 5, Eobtained is the exergy (Ws) taken away by the control body’s external output work, E consumed is the exergy (E
Through exergy efficiency, the energy system and the energy usage of each part of the system can be better understood. At the same time, it also provides a reference for optimizing the energy system and improving each part of the system. Traditional greenhouse energy systems use coal boilers for heating, which are hot water boilers. Regarding the research on the exergy efficiency of hot water boilers, Zhang Q, Yi H, Yu Z, et al. (Zhang et al., 2018) analyzed 141 coal-fired boilers, proposed a calculation mode, and obtained an exergy efficiency of 12.88%, so the exergy efficiency of the coal-fired boiler greenhouse heating system η ex boiler is 12.88%.
Regarding the research on the exergy efficiency of PEMFC, Arshad A, Ali H M, Habib A, et al. (Arshad et al., 2019)summarized the fundamental overview of theoretical and practical aspects of thermodynamics analysis for mainly used fuel cells (FCs). On the basis, they performed exergy analysis of PEMFC in terms of pressure and voltage parameters, and the result was 50.4%. Therefore, the exergy efficiency of hydrogen in the hydrogen-fueled greenhouse heating system η ex hydrogen is 50.4%.
Regarding the research on the exergy efficiency of air conditioners, Dincer I and Rosen MA (Dincer and Rosen, 2015) analyzed the overall air conditioning system at an ambient temperature of 25°C–35°C. We obtained an exergy efficiency of 17.4%–13.9%. Therefore, taking the median, the exergy efficiency of the hydrogen-fueled greenhouse heating system η ex air conditioner is 15.65%.
Regarding the research on the exergy efficiency of EHP, Bilgen E, Takahashi H (Bilgen and Takahashi, 2002) conducted an exergy analysis on the EHP system and concluded that the exergy efficiency of the EHP system is 0.25∼0.37. Therefore, taking the median, the exergy efficiency of the electric heat pump system in the hydrogen greenhouse triple cogeneration energy system η ex EHP is 31%.
From the above results, it can be found that the exergy efficiency of PEMFC is about 3.9 times that of coal boilers. The reason is that hydrogen has a more significant advantage in the energy supply, which is conducive to improving the overall energy utilization of the system. The exergy efficiency of electric heat pumps is about twice that of air conditioners, so EHPs have apparent advantages in terms of equipment selection. In terms of exergy efficiency, the hydrogen greenhouse triple cogeneration system is superior to the traditional and hydrogen greenhouse energy systems.
4.3 Environmental analysis
Environmental pollution has become a significant issue affecting development, and environmental factors must be considered when evaluating systems. CO2 emissions are an essential factor in ecological analysis. Coal boilers produce CO2, and the calculation method is as follows:
According to Shengchun Liu et al. (2017), if a coal boiler is used for heating, providing 30 tons of hot water per day will generate approximately 410 kg of CO2. The working process of hydrogen fuel cells produces only water, especially when using solar energy to produce hydrogen and other methods to achieve zero carbon emissions. Therefore, the hydrogen energy system has significant advantages regarding environmental friendliness.
4.4 Economic analysis
In the process of energy system evaluation, the economy is also a significant factor. At the same time, the economy will also play a positive role in promoting the transformation of the energy supply structure. In particular, in the current situation of increasingly serious environmental problems, environmental costs need to be considered, mainly with carbon dioxide emissions as the leading indicator. Many factors affect the economy, such as equipment cost, operation cost, installation and maintenance cost, design cost, and construction cost. Because the same greenhouse has the exact construction cost, to facilitate calculation and comparisons, only the main costs of the energy system, such as equipment cost, fuel cost, and environmental cost, were considered. The following costs were based on Korea now. Therefore, the total cost of the energy system was calculated as follows:
C total—total cost of the system;
C equipment—total cost of equipment;
C fuel—total cost of fuel;
C environment—total cost of the environment.
Among them, environmental costs are the negative impacts of human activities on the environment, including depletion of natural resources, pollution, climate change, and destruction of habitat and biodiversity. The calculation of environmental costs requires many aspects, such as identifying ecological impacts, quantifying impacts, and assigning monetary values. Due to the complexity of ecological systems and the uncertainties involved in predicting future results, calculating environmental costs takes much work. Based on this situation, the calculation of the ecological cost of this study only considers the cost of CO2 emissions, and other factors are not considered for the time being.
According to the weather data, the greenhouse for growing tomatoes requires heating during November, December, January, and February in an area of northern mid-high latitudes. Owing to the difference in outside temperature, the heating time is different every day. For the convenience of calculation, a 1,500-square-meter greenhouse for growing tomatoes requires 30 tons of hot water per day, and the water temperature needs to be heated from 15°C to 55°C. In addition, it needs to be supplied for 90 days a year. This total heat can meet the energy needs of the greenhouse for a year.
The three systems differ significantly in how they work, but the total energy required by the systems is the same. Each system calculates the amount of fuel consumed based on the characteristics of the fuel. First, it should calculate the total energy Q1 required by the system, and the heat calculation method is as follows:
c—specific heat capacity, in which the specific heat capacity of water CH2O is 4200J/(kg°C);
m1—mass of heating liquid;
Δt—liquid temperature difference;
Therefore, according to Eq. 8, a daily calorie requirement is calculated, and Q total day is 1.008ⅹ107kJ. Therefore, it is calculated according to the supply of 90 days and Q total is 9.072ⅹ108kJ.
In addition, the total energy Q2 produced by the system can be calculated from the energy density as follows:
w—energy density;
m2—mass;
η-system efficiency.
According to the energy conservation law, the total energy Q1 generated by the system equals the total energy Q2 required. The energy loss in the transmission process is not considered for the time being. Therefore,
From this,
For the greenhouse energy system of coal boilers, the coal energy density w coal is approximately 23900 kJ/kg, and the PER boiler is 65% (η boiler is 0.65). From this, the total mass of coal consumed is approximately 58.397 tons in 1 year. According to the information on the energy website, the international coal price is approximately $230/t, so C fuel coal = $13431.31.
For the hydrogen greenhouse energy system, the energy density of hydrogen is approximately 120000 kJ/kg, and the PER hydrogen is 52.93% (η hydrogen is 0.5293). From this, the total mass of hydrogen consumed by the hydrogen greenhouse energy system in 1 year is approximately 14.283 tons. The hydrogen price is approximately $5220/t, so C fuel hydrogen = $74557.26.
For the hydrogen greenhouse triple cogeneration system, the energy density of hydrogen is 120000 kJ/kg, and PER hydrogen triple cogeneration is 73.36% (COP = 3) (η hydrogen triple cogeneration is 0.7336). From this, the total mass of hydrogen consumed by the hydrogen greenhouse triple-cogeneration energy supply system in 1 year is approximately 9.772 tons, so the C fuel hydrogen triple-cogeneration is $51009.84. PER hydrogen triple cogeneration is 100.86% (COP = 4) (η hydrogen triple cogeneration is1.0086). The total mass of hydrogen consumed by the hydrogen greenhouse triple-cogeneration energy system in 1 year is approximately 7.496 tons. Therefore, the calculated C fuel hydrogen triple cogeneration = $39126.71.
Countries worldwide are considering how to reduce carbon dioxide emissions and have formulated a series of policies to achieve carbon neutrality. Therefore, carbon dioxide emissions require social costs. The unit damage cost of carbon dioxide emission is $90/t (Aminyavari et al., 2014), and for the coal boiler energy-supply system, which will produce 36.9 tons of carbon dioxide per year, the carbon dioxide emission cost of C environment coal is $3321. The hydrogen greenhouse energy system and the hydrogen greenhouse triple-cogeneration energy system generate only water as waste during operation, so the environmental cost of the two systems is zero.
The main equipment prices and total equipment cost of the coal boiler energy system, the hydrogen greenhouse energy system, and the hydrogen greenhouse triple-cogeneration energy system were obtained through the survey, as shown in Table 2.
According to the above calculation results, the total cost of the energy supply system was obtained, as shown in Table 3.
In addition, depending on the service life and maintenance cost of each piece of equipment, to compare the economics of the three systems more directly, the 1-day operating cost of the greenhouse can be calculated (mainly including equipment purchase costs and maintenance costs, environmental costs and fuel costs. cost.). In the calculation process, the design life of the equipment is used as the time limit, and the 1-day operating cost of the equipment will be calculated.
For the traditional greenhouse energy system, the design service life of the hot water boiler is generally 10 years, and it works 90 days a year, so the 1-day operating cost of the coal-fired boiler greenhouse energy system C day boiler is $200.58/day. The design service life of PEMFC is generally 20,000 h, the design service life of air conditioners is typically 15 years, and the design service life of EHP is usually 20 years, and it works 90 days a year. Therefore, the 1-day operating cost of the hydrogen greenhouse energy system C day fuel hydrogen average is $933.43/day, and the 1-day operating cost of the hydrogen greenhouse triple-cogeneration energy system C day fuel hydrogen triple cogeneration is $640.74/day.
The economic comparison results of the three systems are as follows:
First, in terms of equipment investment, the coal-fired boiler energy system has a minor investment. The reason is that the system has a simple structure and only has a heating and energy supply. It is suitable for use in high-latitude areas and has a limited scope of application. And Luo J, Xue W, et al. studied the economic performance of coal-fired boilers and groundwater-source heat pumps in greenhouses. The calculation results show that coal boilers will be replaced by clean and renewable energy (Luo et al., 2020). The PEMFC powers the hydrogen greenhouse energy system and the hydrogen greenhouse triple cogeneration system. PEMFCs have not been commercialized on a large scale because of their small output. In addition, the research and development costs are high, so it is expensive, accounting for 60%–70% of the total cost. On the other hand, the price of hydrogen fuel cell systems has decreased with technological progress and the expansion of the production scale. The production cost is expected to be 50% of the current level in the next 10 years (Liu et al., 2021). Therefore, the equipment investment in hydrogen greenhouses will be reduced significantly. In addition, the selection of energy-saving equipment, such as air conditioners and heat pumps, is expensive, and the investment-reporting period will be longer. It can realize cooling/heating energy supply and has a more substantial constant temperature control effect on the greenhouse temperature, which can meet the needs of different regions.
Second, coal ($0.23/kg) has a price advantage in terms of fuel consumption, so coal boilers consume the lowest fuel cost for 1 year. The high price of hydrogen fuel ($5.22/kg) is because the cost of hydrogen production, hydrogen storage, and hydrogen transportation are still being actively explored. With the continuous advances of commercialization, hydrogen prices continue to decline. According to the current coal price, without considering the cost of carbon dioxide emissions, when the hydrogen price decreases to below $1.3/kg, coal will lose its price advantage in terms of fuel. At the same time, hydrogen has a high energy density, small volume, and low transportation cost. According to IRENA and Hydrogen Council, the cost of producing hydrogen from renewable energy will drop to $1/kg by 2050 (Clerici and Furfari, 2021; Wang et al., 2022b). Hydrogen energy will replace coal energy, and its advantages will become increasingly evident in the future.
Third, regarding the environmental cost, coal combustion will generate a large amount of carbon dioxide, resulting in a specific environmental cost. The ecological costs will increase as countries worldwide tighten restrictions on carbon dioxide emissions. Because of environmental pollution, coal boilers are gradually being replaced with other new energy heating methods. Hydrogen is an important energy source for energy transition and carbon neutrality (Zou et al., 2022). In this regard, the hydrogen greenhouse energy system and the hydrogen greenhouse triple cogeneration energy system have apparent advantages.
Fourth, compared to the hydrogen greenhouse energy system, the hydrogen greenhouse triple cogeneration system has obvious advantages. In terms of equipment investment, it can be reduced by 20.20%. The main reason is that the waste heat generated by the fuel cell is used, and the PEMFC with lower power can be used, and the price will be reduced. In addition, the PEMFC can reduce fuel consumption by 31.60%. In this way, the overall economic benefit of the system is improved.
Fifth, through the analysis of the 1-day operating costs of the three systems, it can be found that the hydrogen greenhouse energy system and the hydrogen greenhouse triple cogeneration system are 4.7 times and 3.2 times that of the coal boiler greenhouse energy system, respectively. But in terms of the total cost, the hydrogen greenhouse energy system and the hydrogen greenhouse triple cogeneration system are 5.9 times and 4.4 times that of the coal boiler greenhouse energy system, respectively. The difference is that the service life and maintenance costs of different equipment differ. Although some equipment has a significant one-time investment, it has a long service life. Therefore, the operating price can reflect the system’s economy more comprehensively.
From the perspective of the total cost, the use of hydrogen fuel in the greenhouse energy system, even if the hydrogen greenhouse triple cogeneration system is used to improve the energy utilization efficiency, compared with the coal-fired boiler energy supply system, there is a significant investment and a long-term question. On the other hand, transforming the traditional energy structure into a renewable energy structure is necessary. More study is needed to solve the problem of fossil fuel energy depletion. Hydrogen energy is an efficient energy solution that will play a more significant role in the future. The advantages of the hydrogen greenhouse triple cogeneration system will increase gradually in the context of falling prices for hydrogen and hydrogen fuel cells.
4.5 Simulation of the hydrogen greenhouse triple cogeneration system
The advantages of simulation software are more prominent (Martelli et al., 2021). First, the simulation can be repeated continually, and it is relatively easy to change the system structure and coefficients. Second, it is not limited by time, space, and conditions, which is more conducive to developing research work. Finally, simulation can reduce the R&D costs and shorten the R&D cycle. The simulation of the hydrogen greenhouse triple cogeneration system can verify the system’s operability and optimize the system parameters. Figure 9 shows the working strategy of the hydrogen greenhouse triple-cogeneration system.
According to the above method, the simulation of the hydrogen fuel cell and the power supply part was completed. The simulation process is shown in Figure 10. Simulation software was used to simulate the fuel cell working for some time. Figures 11A,B show the simulation results of fuel consumption power and output electric power.
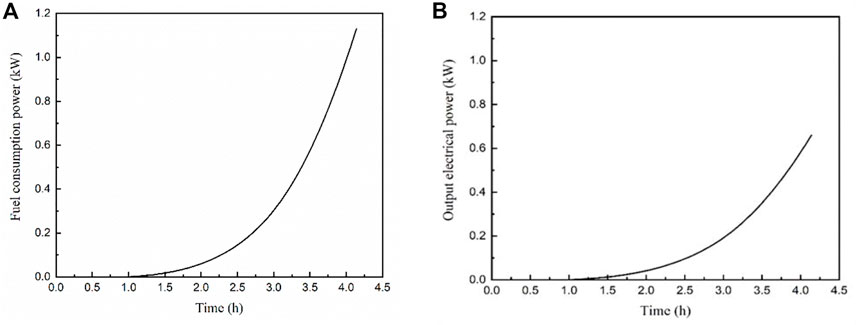
FIGURE 11. Simulation results of a hydrogen fuel cell system with (A) the fuel consumption power change and (B) the output electrical power change.
Because the output result is power, the output result needs to be processed to obtain conveniently the energy conversion efficiency of the system. According to the mathematical method, the output results are integrated. The ratio of the total output electric energy to the total energy consumed by the system was 61.15%, and the energy efficiency of the hydrogen fuel cell system was 61.15%. Its value was higher than 60% (the experiment efficiency of PEMFC alone power generation was 50%–60%). The difference between the simulation results and the actual experimental results was that changes in the external environment, such as changes in temperature, would reduce the working efficiency of the hydrogen fuel cell system. On the other hand, the simulation environment is more idealized, and the work efficiency will be higher.
It shows the simulation process (heating process) of the triple cogeneration system part in Figure 12. Without considering the waste hot water as a heat source, by the simulation software, the EHP was simulated to work for some time; Figure 13A,B show the simulation results of the electric power demand and output heat energy, respectively. According to the simulation results, the ratio of the total heat output of the EHP to the total electricity demand was 98.94% (the experimental energy efficiency of the electric heat pump unit was approximately 100%), and the working efficiency of the EHP was 98.94%. The difference is because the experimental result is the energy conversion of the electric heat pump working alone. In contrast, the simulation result is the energy conversion efficiency of the whole system, and other parts have a specific energy consumption.
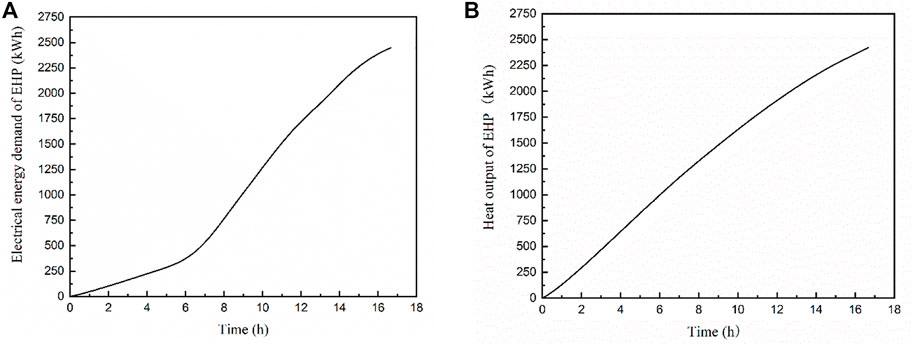
FIGURE 13. Simulation results of a triple cogeneration system (heating process) with (A) the Electrical energy demand of an EHP change and (B) heat output of an EHP change.
The waste hot water generated by the fuel cell is used as the heat source of the EHP. Through simulation software, it simulated the triple cogeneration system (heating process) to work for some time, as shown in Figure 14A,B. According to the simulation results, the ratio of the total heat output of the EHP to the total electricity demand is 144.20%. Therefore, the heating energy efficiency of the hydrogen greenhouse triple-cogeneration system can be obtained as 88.17%. Because the energy efficiency of an electric heat pump is much higher than that of an adsorption refrigerator, the energy efficiency for heating is higher than that for cooling.
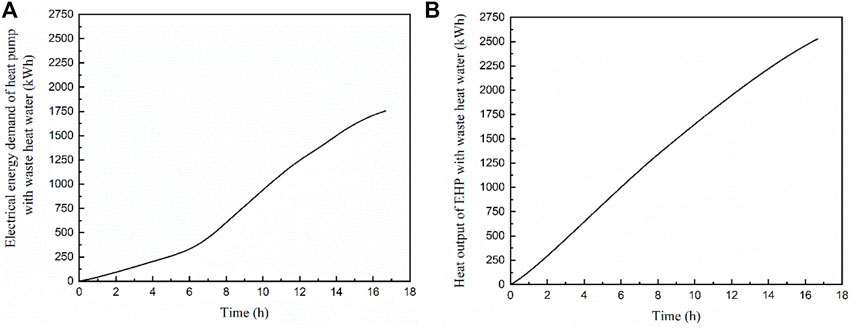
FIGURE 14. Simulation results of a triple cogeneration system (heating process with waste heat water) with (A) electrical energy demand of an EHP change and (B) heat output of an EHP change.
5 Conclusion
In this study, the resource analysis method was used in the design stage to consider the utilization of system resources as a whole. The hydrogen fuel triple cogeneration system uses the waste heat generated by the hydrogen fuel cell itself, which can not only solve the problem of heat dissipation but also improve the energy utilization efficiency of the system and reduce energy consumption. Through analysis and calculation, compared with traditional greenhouse energy systems and hydrogen greenhouse energy systems, the advantages in terms of energy efficiency, exergy efficiency, and environmental costs are.
1) By calculating the system energy efficiency, the hydrogen energy triple-cogeneration system can increase the total system energy efficiency to more than 73% (the traditional greenhouse energy efficiency is 65% and the hydrogen greenhouse energy efficiency is only 52.93%).
2) In terms of exergy efficiency, the efficiency of hydrogen in the hydrogen-fueled greenhouse energy supply system is 50.4%. In comparison, the exergy efficiency of the traditional greenhouse energy system is 12.88%, so the advantages of using hydrogen energy are prominent. In addition, the exergy efficiency of EHP (31%) is about twice that of the air conditioner (15.65%). Therefore, the hydrogen greenhouse triple cogeneration system is superior to the traditional and hydrogen greenhouse energy systems regarding exergy efficiency.
3) Regarding environmental costs, hydrogen energy can achieve zero emissions of pollutants, but the CO2 emission standard for coal energy is $90/t. Therefore, traditional greenhouse energy systems will incur additional emissions costs.
In terms of economy, the traditional greenhouse energy system still has an advantage. The reason is that hydrogen fuel and fuel cells are expensive, and the return-on-investment period of air conditioners and electric heat pumps is higher than that of coal-fired boilers. However, with the decline in the cost of hydrogen energy (estimated $1/kg by 2050) and hydrogen fuel cells, according to calculations, when the price of hydrogen fuel is $1.3/kg, coal will lose its price advantage. Therefore, it will play an essential economic role in the future. The models of the hydrogen fuel cell power supply and electric heat pump power supply system are established using simulation software, and the simulation is completed. The simulation results are roughly the same as the calculation results, and the hydrogen greenhouse triple cogeneration system is feasible, which lays the foundation for the physical experiment. This research progress is an active exploration of transforming the traditional greenhouse energy supply system to a renewable one, which will help alleviate the energy crisis and improve the ecological environment.
Data availability statement
The original contributions presented in the study are included in the article/supplementary material, further inquiries can be directed to the corresponding authors.
Author contributions
JY: Responsible for proposing the research plans and carrying out research work, sorting out research results, and drafting the paper. RL: Responsible for guiding the conduct of research and the revision of the paper. C-HL: Responsible for proposing research propositions, guiding the conduct of research, and revising the paper.
Funding
This research was financially supported by the High-Level Foreign Expert Introduction Program (No. G2022035005L). This study was also carried out with the support of “Cooperative Research Program for Agriculture Science and Technology Development (Project No. PJ016257022022)” Rural Development Administration, Republic of Korea.
Conflict of interest
The authors declare that the research was conducted in the absence of any commercial or financial relationships that could be construed as a potential conflict of interest.
Publisher’s note
All claims expressed in this article are solely those of the authors and do not necessarily represent those of their affiliated organizations, or those of the publisher, the editors and the reviewers. Any product that may be evaluated in this article, or claim that may be made by its manufacturer, is not guaranteed or endorsed by the publisher.
References
Ahamed, M. S., Guo, H., and Tanino, K. (2019). Energy saving techniques for reducing the heating cost of conventional greenhouses. Biosyst. Eng. 178, 9–33. doi:10.1016/j.biosystemseng.2018.10.017
Ahamed, M. S., Guo, H., and Tanino, K. (2019). Energy saving techniques for reducing the heating cost of conventional greenhouses. Biosyst. Eng. 178, 9–33. doi:10.1016/j.biosystemseng.2018.10.017
Ahamed, M. S., Guo, H., Taylor, L., and Tanino, K. (2019). Heating demand and economic feasibility analysis for year-round vegetable production in Canadian Prairies greenhouses. Inf. Process. Agric. 6 (1), 81–90. doi:10.1016/j.inpa.2018.08.005
Albatayneh, A., Assaf, M. N., Alterman, D., and Jaradat, M. (2020). Comparison of the overall energy efficiency for internal combustion engine vehicles and electric vehicles. Rigas Teh. Univ. Zinat. Raksti 24 (1), 669–680. doi:10.2478/rtuect-2020-0041
Aminyavari, M., Najafi, B., Shirazi, A., and Rinaldi, F. (2014). Exergetic, economic and environmental (3E) analyses, and multi-objective optimization of a CO2/NH3 cascade refrigeration system. Appl. Therm. Eng. 65 (1-2), 42–50. doi:10.1016/j.applthermaleng.2013.12.075
Arshad, A., Ali, H. M., Habib, A., Bashir, M. A., Jabbal, M., and Yan, Y. (2019). Energy and exergy analysis of fuel cells: A review. Therm. Sci. Eng. Prog. 9, 308–321. doi:10.1016/j.tsep.2018.12.008
Asgari, A., Yari, M., and Mahmoudi, S. M. S. (2021). Exergy and exergoeconomic analyses and multi-objective optimization of a novel cogeneration system for hydrogen and cooling production. Int. J. Hydrogen Energy 47, 26114–26134. doi:10.1016/j.ijhydene.2021.11.037
Barbir, F., and Gomez, T. (1997). Efficiency and economics of proton exchange membrane (PEM) fuel cells. Int. J. hydrogen energy 22 (10-11), 1027–1037. doi:10.1016/s0360-3199(96)00175-9
Bekhet, H. A., and Harun, N. H. (2016). Role of non-renewable and renewable energy for sustainable electricity generation in Malaysia[J]. Int. J. Environ. Ecol. Eng. 10 (9), 907–916.
Berahab, R. (1967). The energy crisis of 2021 and its implications for africa[J]. Rabat: Policy, 2022.
Bilgen, E., and Takahashi, H. (2002). Exergy analysis and experimental study of heat pump systems. [J]. Exergy, Int. J. 2 (4), 259–265. doi:10.1016/s1164-0235(02)00083-3
Burt, E., Orris, P., and Buchanan, S. (2013). Scientific evidence of health effects from coal use in energy generation[J]. Chicago and Washington: School of Public Health, University of Illinois and Health Care Without Harm.
Chao, L., Ke, L., Yongzhen, W., Zhitong, M., and Yulie, G. (2017). The effect analysis of thermal efficiency and optimal design for boiler system. Energy Procedia 105, 3045–3050. doi:10.1016/j.egypro.2017.03.629
Chauhan, P. R., Kaushik, S. C., and Tyagi, S. K. (2022). Current status and technological advancements in adsorption refrigeration systems: A review. Renew. Sustain. Energy Rev. 154, 111808. doi:10.1016/j.rser.2021.111808
Cheng, M., Zhi, G., Tang, W., Liu, S., Dang, H., Guo, Z., et al. (2017). Air pollutant emission from the underestimated households' coal consumption source in China. Sci. Total Environ. 580, 641–650. doi:10.1016/j.scitotenv.2016.12.143
Clerici, A., and Furfari, S. (2021). Future energy scenarios, impact on hydrogen development and EU green energy strategy[C]//2021 AEIT International Annual Conference (AEIT). IEEE, 1–6.
Colakoglu, M., and Durmayaz, A. (2022). Energy, exergy and economic analyses and multiobjective optimization of a novel solar multi-generation system for production of green hydrogen and other utilities. Int. J. Hydrogen Energy 47, 19446–19462. doi:10.1016/j.ijhydene.2021.12.203
De Carvalho, M. A., and Back, N. (1999). TRIZ methodology and its use in systematic engineering design[J]. Paraná: Congrès Brésilien d'Ingénierie Mécanique.
Denzer, A., Wang, L., and Thomas, Y. (2017). Greenhouse design with waste heat: Principles and practices[M]//AEI 2017, 440–455.
Dincer, I., and Rosen, M. A. (2015). Exergy analysis of heating, refrigerating and air conditioning: Methods and applications[M]. Academic Press.
Djevic, M., and Dimitrijevic, A. (2004). Greenhouse energy consumption and energy efficiency[J]. Balk. Agric. Eng. Rev. 5, 1–9.
Fu, Y., and Liang, M. (2022). Calculation and analysis of primary energy utilization rate of heat pump [J]. HVAC 52 (2), 5.
Gasik, M. (2008). Introduction: Materials challenges in fuel cells[M]//Materials for fuel cells. Sawston: Woodhead Publishing, 1–5.
Gholizadeh, T., Vajdi, M., and Rostamzadeh, H. (2019). A new biogas-fueled bi-evaporator electricity/cooling cogeneration system: Exergoeconomic optimization. Energy Convers. Manag. 196, 1193–1207. doi:10.1016/j.enconman.2019.06.053
Hendryx, M., Zullig, K. J., and Luo, J. (2020). Impacts of coal use on health. Annu. Rev. public health 41, 397–415. doi:10.1146/annurev-publhealth-040119-094104
Hwang, J. J. (2013). Effect of hydrogen delivery schemes on fuel cell efficiency. J. Power Sources 239, 54–63. doi:10.1016/j.jpowsour.2013.03.090
Hydrogen Energy [EB/OL] (2022). Hyundai motor group. Available at: https://tech.hyundaimotorgroup.com/fuel-cell/hydrogen-energy/.
Ilevbare, I. M., Probert, D., and Phaal, R. (2013). A review of TRIZ, and its benefits and challenges in practice. Technovation 33 (2-3), 30–37. doi:10.1016/j.technovation.2012.11.003
Isa, N. M., Tan, C. W., and Yatim, A. H. M. (2018). A comprehensive review of cogeneration system in a microgrid: A perspective from architecture and operating system. Renew. Sustain. Energy Rev. 81, 2236–2263. doi:10.1016/j.rser.2017.06.034
Jabari, F., Mohammadi-ivatloo, B., Bannae-Sharifian, M. B., and Ghaebi, H. (2018). Design and performance investigation of a biogas fueled combined cooling and power generation system. Energy Convers. Manag. 169, 371–382. doi:10.1016/j.enconman.2018.05.026
Jain, I. P. (2009). Hydrogen the fuel for 21st century. Int. J. hydrogen energy 34 (17), 7368–7378. doi:10.1016/j.ijhydene.2009.05.093
Kataria, A., and Khan, T. I. (2021). Necessity of paradigm shift from non-renewable sources to renewable sources for energy demand[M]//Urban growth and environmental issues in India. Singapore: Springer, 337–352.
Kosonen, I. (2018). Intermittency of renewable energy; review of current solutions and their sufficiency[J].
Lakatos, L., Hevessy, G., and Kovács, J. (2011). Advantages and disadvantages of solar energy and wind-power utilization. World Futur. 67 (6), 395–408. doi:10.1080/02604020903021776
Leonzio, G. (2018). An innovative trigeneration system using biogas as renewable energy. Chin. J. Chem. Eng. 26 (5), 1179–1191. doi:10.1016/j.cjche.2017.11.006
Liang, J., Jiang, P., and Jia, L. (2013). Resource analysis and application based on the attribute module of complete system[C]//2013 international conference on mechanical and automation engineering. IEEE, 237–244.
Liu, S., Li, Z., and Dai, B. (2017). Energy, economic and environmental analyses of the CO2 heat pump system compared with boiler heating system in China[J]. Energy Procedia 105, 3895–3902. doi:10.1016/j.egypro.2017.03.803
Liu, X., Cao, H., and Chen, G. (2011). TRIZ theory and application [M]. Beijing: Peking University Press, 69–73.
Liu, Y., Guo, H., and Ouyang, X. (2021). Development status and future prospects of hydrogen fuel cell technology. China Eng. Sci. 23 (4), 162–171. doi:10.15302/j-sscae-2021.04.019
Liu, Z., Feng, J., and Wang, J. (2020). Resource-constrained innovation method for sustainability: Application of morphological analysis and TRIZ inventive principles. Sustainability 12 (3), 917. doi:10.3390/su12030917
Luo, J., Xue, W., and Shao, H. (2020). Thermo-economic comparison of coal-fired boiler-based and groundwater-heat-pump based heating and cooling solution – a case study on a greenhouse in Hubei, China. Energy Build. 223, 110214. doi:10.1016/j.enbuild.2020.110214
Martelli, E., Alobaid, F., and Elsido, C. (2021). Design optimization and dynamic simulation of steam cycle power plants: A review[J]. Front. Energy Res., 319.
Mueller, S. (2005). The TRIZ resource analysis tool for solving management tasks: Previous classifications and their modification. Creativity innovation Manag. 14 (1), 43–58. doi:10.1111/j.1467-8691.2005.00324.x
Petrović-Ranđelović, M., Kocić, N., and Stojanović-Ranđelović, B. (2020). The importance of renewable energy sources for sustainable development. Econ. Sustain. Dev. 4 (2), 15–24. doi:10.5937/esd2002016p
Qiu, S., Lei, T., Wu, J., and Bi, S. (2021). Energy demand and supply planning of China through 2060. Energy 234, 121193. doi:10.1016/j.energy.2021.121193
Saha, B. B., Koyama, S., Lee, J. B., Kuwahara, K., Alam, K., Hamamoto, Y., et al. (2003). Performance evaluation of a low-temperature waste heat driven multi-bed adsorption chiller. Int. J. Multiph. Flow 29 (8), 1249–1263. doi:10.1016/s0301-9322(03)00103-4
Shankar, R., and Rivera, W. (2022). Modeling of a thermodynamic cycle integrating a dual and a triple-pressure cogeneration cycle. Appl. Therm. Eng. 201, 117705. doi:10.1016/j.applthermaleng.2021.117705
Singla, M. K., Nijhawan, P., and Oberoi, A. S. (2021). Hydrogen fuel and fuel cell technology for cleaner future: A review. Environ. Sci. Pollut. Res. 28 (13), 15607–15626. doi:10.1007/s11356-020-12231-8
Spreafico, C. (2021). Quantifying the advantages of TRIZ in sustainability through life cycle assessment. J. Clean. Prod. 303, 126955. doi:10.1016/j.jclepro.2021.126955
Sztekler, K., Kalawa, W., Nowak, W., Mika, L., Gradziel, S., Krzywanski, J., et al. (2020). Experimental study of three-bed adsorption chiller with desalination function. Energies 13 (21), 5827. doi:10.3390/en13215827
Vojdani, M., Fakhari, I., and Ahmadi, P. (2021). A novel triple pressure HRSG integrated with MED/SOFC/GT for cogeneration of electricity and freshwater: Techno-economic-environmental assessment, and multi-objective optimization. Energy Convers. Manag. 233, 113876. doi:10.1016/j.enconman.2021.113876
Vourdoubas, J. (2015). Overview of heating greenhouses with renewable energy sources a case study in Crete-Greece[J]. J. Agric. Environ. Sci. 4 (1), 70–76.
Wang, H., Xu, W., and Zhang, Z. (2022). Development status and suggestions of green hydrogen energy produced by water electrolysis from renewable energy[J/OL]. Chem. Progress, 1-19. doi:10.16085/j.issn.1000-6613.2022-0159
Wang, Y., Huang, Y., Chiremba, E., Roskilly, A. P., Hewitt, N., Ding, Y., et al. (2011). An investigation of a household size trigeneration running with hydrogen. Appl. energy 88 (6), 2176–2182. doi:10.1016/j.apenergy.2011.01.004
Wang, Y., Ramadan, M. M., and Tooteja, P. (2022). Analysis of hydrogen production costs across the United States and over the next 30 years[J]. arXiv preprint arXiv:2206.10689.
Wee, J. H. (2007). Applications of proton exchange membrane fuel cell systems. Renew. Sustain. energy Rev. 11 (8), 1720–1738. doi:10.1016/j.rser.2006.01.005
Wen, D., and Aziz, M. (2022). Flexible operation strategy of an integrated renewable multi-generation system for electricity, hydrogen, ammonia, and heating. Energy Convers. Manag. 253, 115166. doi:10.1016/j.enconman.2021.115166
Wu, W., Wang, J., Lin, B., and Zhao, Z. (2021). Research on energy efficiency evaluation of photovoltaic direct drive air conditioning system [J]. Refrig. Air Cond. 021 (004), 32–37.
Xu, X., You, S., Zheng, X., and Li, H. (2014). A survey of district heating systems in the heating regions of northern China. Energy 77, 909–925. doi:10.1016/j.energy.2014.09.078
Zhang, Q., Yi, H., Yu, Z., Gao, J., Wang, X., Lin, H., et al. (2018). Energy-exergy analysis and energy efficiency improvement of coal-fired industrial boilers based on thermal test data. Appl. Therm. Eng. 144, 614–627. doi:10.1016/j.applthermaleng.2018.08.069
Zhang, S., Guo, Y., Zhao, H., Wang, Y., Chow, D., and Fang, Y. (2020). Methodologies of control strategies for improving energy efficiency in agricultural greenhouses. J. Clean. Prod. 274, 122695. doi:10.1016/j.jclepro.2020.122695
Zhang, S., Guo, Y., Zhao, H., Wang, Y., Chow, D., and Fang, Y. (2020). Methodologies of control strategies for improving energy efficiency in agricultural greenhouses. J. Clean. Prod. 274, 122695. doi:10.1016/j.jclepro.2020.122695
Zhou, J. (2021). Analysis and entropy analysis of gas turbine combined cooling, heating and power system (exergy) based on transient simulation[D]. Qingdao: Qingdao University of Technology.
Zhuang, P., Liang, H., and Pomphrey, M. (2018). Stochastic multi-timescale energy management of greenhouses with renewable energy sources. IEEE Trans. Sustain. Energy 10 (2), 905–917. doi:10.1109/tste.2018.2854662
Keywords: hydrogen energy, triple cogeneration system, resource analysis, energy efficiency, economic analysis
Citation: Yang J, Li R and Lee C-H (2023) Design of triple cogeneration system for hydrogen fuel cell in greenhouse based on resource analysis. Front. Energy Res. 11:1081455. doi: 10.3389/fenrg.2023.1081455
Received: 27 October 2022; Accepted: 11 April 2023;
Published: 26 April 2023.
Edited by:
Michael Carbajales-Dale, Clemson University, United StatesReviewed by:
Muhammad Aziz, The University of Tokyo, JapanBheru Lal Salvi, Maharana Pratap University of Agriculture and Technology, India
Copyright © 2023 Yang, Li and Lee. This is an open-access article distributed under the terms of the Creative Commons Attribution License (CC BY). The use, distribution or reproduction in other forums is permitted, provided the original author(s) and the copyright owner(s) are credited and that the original publication in this journal is cited, in accordance with accepted academic practice. No use, distribution or reproduction is permitted which does not comply with these terms.
*Correspondence: Chul-Hee Lee, chulhee@inha.ac.kr; Rui Li, lirui@cqupt.edu.cn