Assessment of the calendar aging of lithium-ion batteries for a long-term—Space missions
- 1Department of Electrical Engineering, SBA School of Science and Engineering, Lahore University of Management Sciences, Lahore, Pakistan
- 2Center for Advanced Life Cycle Engineering (CALCE), University of Maryland, College Park, MD, United States
- 3Department Industrial Systems Engineering and Design Universitat Jaume I of Castelló, Castelló de la Plana, Spain
- 4National Aeronautics and Space Administration (NASA) Goddard Space Flight Center (GSFC) Reliability, Maintainability, and Availability, Greenbelt, MD, United States
Energy availability is a critical challenge for space missions, especially for those missions designed to last many decades. Space satellites have depended on various combinations of radioisotope thermoelectric generators (RGTs), solar arrays, and batteries for power. For deep space missions lasting as long as 50 + years, batteries will also be needed for applications when there is no sunlight and RTGs cannot support peak power demand due to their insufficient specific power. This paper addresses the potential use of lithium-ion batteries for long-term space missions. Using data collected from the literature and internal experiments, a calendar aging model is developed to assess the capacity fade as a function of temperature, state-of-charge and time. The results for various LIB chemistries are used to identify the best candidate chemistries and determine the conditions, with a focus on low temperatures, that can best enable deep space missions.
1 Introduction
The National Aeronautics and Space Administration (NASA), China National Space Administration (CNSA), and European Space Agency (ESA) have started to plan deep space missions that may continue for 50 years or more (Forbes, 2021; Skyatnightmagazine, 2022; Spacenews, 2022; NSSC, 2030). To carry out such missions, various combinations of radioisotope thermoelectric generators (RTGs) and batteries will be needed for power (Bushnell et al., 2021; Baraskar et al., 2022), since solar arrays will provide negligible value in deep space. Although the power systems inherent in these designs have varied and the design strategies have evolved over the years, batteries will likely be needed for a long-term deep space mission (Hepp et al., 2022; Jones et al., 2022).
NASA, the ESA, the CNSA, and the Russian Federal Space Agency (Roscosmos) rely on RTGs for deep space missions when long-term power is needed and there is no access to sunlight (Bairstow et al., 2018; ESA, 2022; Spacenews, 2022; World-nuclear, 2022). In addition, the Indian Space Research Organization (ISRO) planned to build a 100 W RTG for its upcoming space mission (nuclear-energy, 2022). In collaboration with the U.S. Department of Energy (DoE), NASA developed the first flown RTG and launched it into earth’s orbit in 1961 (NASA, 2022a). RTGs were then used on the deep space exploration probes of Voyager 1 and 2 (NASA, 2022a; NASA, 2022b), showing that it was possible to produce continuous electrical energy from decaying radioactive material for long missions (NASA, 2022b). This success was followed by continued use of RTGs on New Horizons (2006) and Cassini missions (2008) (NASA, 2022c; NASA, 2022d).
RTG systems have been found to be reliable, uninterruptable energy sources; however, they have low specific power (∼2.4 W/kg to 8.1 W/kg) and many safety concerns (Hendricks et al., 2019; Durka et al., 2022). RTGs generally utilize Plutonium-238 oxide as a fuel with a half-life of 88 years to generate the residual heat and produce electrical power (Gusev et al., 2011; Urban-Klaehn et al., 2021). RTGs have historically experienced no known failure issues since they have no moving parts that can fail or wear out, and they perform well until the radioactive energy source decays (Ehrenfried, 2022; Energy, 2022). However, Plutonium-238 is very expensive ($4 million/pound (Frobes, 2015)), and in the event of a launch-related accident, loading the space probe with a large amount of radioactive material poses environmental concerns of radioactive fallout (Shmelev et al., 2020; Zillmer et al., 2022).
The specific power of RTGs is being improved through the development of enhanced multi-mission radioisotope thermoelectric generators (MMRTGs) and Stirling radioisotope generators (Houtmann, 2020). These new-generation RTGs have better efficiency than the 4%–6% conversion efficiency of conventional RTG systems (Matthes et al., 2018; Houtmann, 2020). The MMRTG utilized in the 2020 Mars rover mission weighs 45 kg, includes 4.8 kg of fuel, and is designed to provide 110 W of electrical power to equipment (NASA, 2020; Energy, 2022). The MMRTG is guaranteed to provide 110 W of output power for a minimum of 14 years, after which the output power may decrease to 100 W (Energy, 2022). Table 1 details the RTGs used for several space missions.
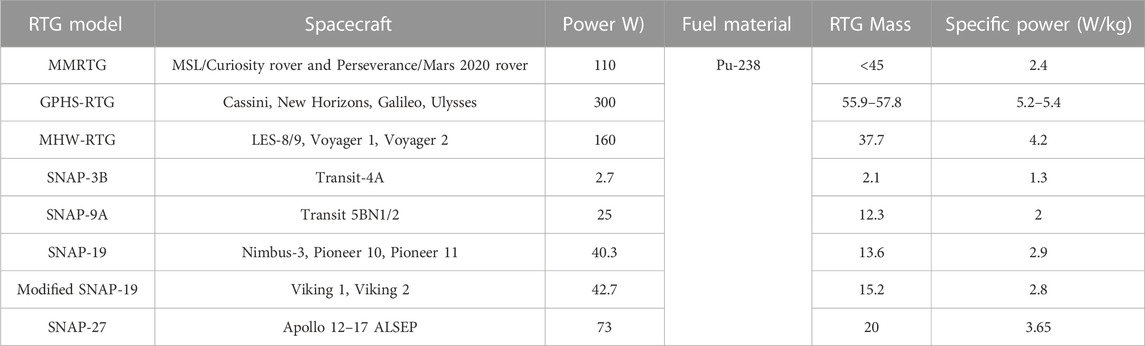
TABLE 1. RTGs used in spacecraft (Bennett, 2006; Bennett, 2018; Woerner, 2018; Clarke et al., 2022).
To operate all the electric components of a spacecraft, including the computers, radio transmission, motors, and data storage devices, 300 W–2500 W of electrical power are required (NASA, 2022e). Due to weight constraints in spacecraft, researchers build RTGs that supply up to 300 W of power, as indicated in Table 1. Hence, batteries are required to support the peak power requirements of space missions. Furthermore, if the batteries are rechargeable, excess energy from RTGs can be stored in the batteries during off-peak power time and used subsequently during peak power duration (Designnews, 2022; NASA, 2022f). For instance, two lithium-ion batteries (LIBs) were incorporated into the Mars rover mission in 2020 to meet peak power requirements when demand temporarily exceeded the electrical power generated by RTGs (NASA, 2020).
From 1983 to 2014, nickel-hydrogen (Ni-H2) batteries were primarily used on missions due to their energy density and capacity. From 1993 to 2015, nickel-cadmium (NiCd) batteries were used, and from 2003 to the present, LIBs have been used in space missions (NASA, 2016; NASA, 2022f). Ni-H2 batteries have been used in AQUA (Brewer, 2000), CloudSat (Witkowski et al., 2018), and TIMED (Yee et al., 1999). Similarly, DSCOVR (Dudac, 2022), INTEGRAL (Spaceref, 2022), and DSCS-3s (Thierfelder, 1982) relied on NiCd batteries. Ni-H2 has replaced NiCd because of its longer projected lifespan and fewer discharge voltage issues (Smithrick and O'Donnell, 1996; Deroy et al., 2019). The discharge voltage problem pertains to the decrease in the discharge curve voltage with operational life (NASA, 1997). In addition, some space missions have employed Ag/Zn and LiSO2 rechargeable batteries (Surampudi et al., 2006; Bugga and Brandon, 2020).
Recent trends suggest that LIBs are preferred in space missions because of their lower operating temperature tolerance, two to three times greater specific energy (265 Wh/kg), and higher energy density (670 Wh/L) compared to other types of rechargeable batteries (NASA, 2010; Institute, 2022). In addition, the specific power density of LIBs is around 1000 W/kg, allowing them to meet the peak power requirements of a space mission when the demand exceeds the RTG’s steady electrical power levels (Zheng et al., 2017).
LIBs have been used in the 2020 Mars rover, where the battery’s operational temperature was controlled to range within −40°C–40 °C (NASA, 2010; NASA, 2016). LIBs have also been used in the OSIRIS-Rex (Bierhaus et al., 2018), the Parker Solar Probe (Kinnison et al., 2020), and THEMIS satellites (Harvey et al., 2008), as well as the Eutelsat W3A satellite (S. Agency, 2022).
In spite of the advances in battery technology, malfunctions in spacecraft owing to battery failure have occurred. On 2 November 2006, the Mars Global Surveyor lost contact with earth (NASA, 1742) when the spacecraft’s batteries were exposed to direct sunlight, which caused overheating and ultimately depleted the batteries (NASA, 1742).
Another battery (Ni-H2) anomaly occurred on the CloudSat spacecraft. Late in 2009, CloudSat’s battery began exhibiting early indications of aging. Despite being deteriorated, the battery’s capacity was adequate to maintain the satellite’s power requirements. However, in April 2011, the first of a series of under-voltage faults occurred, resulting in the activation of an emergency mode that disabled scientific operations and posed a collision threat to nearby orbiters in the A-Train constellation (Nayak, 20122012; Gravseth and Pieper, 2013).
Based on the space missions that used rechargeable batteries, the observed reliability to date is around 14 years for Ni-H2 (bitstream, 2014), 20 years for Ni-Cd (NASA, 1998; Saftbatteries, 2023), and 10 years for Li-ion (NASA, 2023), although LIBs implemented in the latest missions, are still under operation, and are expected to have much greater lifetimes (Halpert et al., 1999; Zaghib et al., 2011).
A combination of RTGs and batteries will be required for 50 years space missions, where the RTG powers the spacecraft to its destination and batteries conduct the high-power-demanding tasks (300W–2500 W (NASA, 2022e)) when the spacecraft arrives at its destination. RTGs can then be used to recharge the batteries. This requires that the batteries be able to spend the majority of their lifespan in storage mode while maintaining enough capacity to carry out the mission upon arrival to its destination. This paper develops a calendar aging (loss of capacity in storage) model based on existing experimental data from the literature and experiments that we conducted. The model is used to determine storage conditions that could be implemented in deep space missions, Section 2 of this paper overviews the storage-related aging mechanisms that occur in various LIBs; Section 3 presents calendar aging data including experimental data from the authors. In Section 4, a calendar aging modeling is developed using the experimental data. The results for various LIB chemistries are used to identify the best candidate chemistries and determine the temperature, state of charge (SOC) and other conditions that can lessen capacity fade when subjected to long term storage. Section 5 presents the conclusions and the environmental management conditions for long-term space missions.
2 Li-ion batteries and calendar aging
LIBs are electrochemical systems comprised of two intercalation electrodes with their corresponding current collectors, an electrolyte, and a separator used to prevent an internal short between the anode and cathode (Winter et al., 2018; Shodiev et al., 2021). Commercial LIB chemistries integrate graphite or Li2TiO3 (LTO) as anode material and transition metal oxides such as LiCoO2 (LCO), LiNiMnCoO2 (NCM), LiNiCoAlO2 (NCA), LiMn2O4 (LMO), or LiFePO4 (LFP) as cathodes (Chayambuka et al., 2020; Ali et al., 2021). The successful combinations of components currently available in the marketplace correspond to those that grant high energy density (80–260 Wh/kg), and electrochemical stability within the operation range without posing safety risks (Diaz et al., 2020; Ali et al., 2022). Since cost is not a major issue for satellite applications analyzed in this paper, the main parameters to be considered when comparing the available LIB technologies pertain to energy density (a higher specific energy leads to a lighter battery pack for a given energy capacity) and the lifespan. When coupled with graphite anodes, NCA cells currently present the highest specific energy (200–260 Wh/kg), followed by NMC (150–250 Wh/kg), LCO (120–210 Wh/kg), LMO (100–130 Wh/kg) and LFP (80–150 Wh/kg) (Ogumi, 2020; Walvekar et al., 2022). On the other hand, LFP cells can deliver more than 4,000 charge-discharge cycles compared to NMC (2000 cycles) or NCA cells (1,500 cycles) (Preger et al., 2020; Tran et al., 2021).
Calendar aging occurs when a battery is not operating (e.g. no current passes through it) (Redondo-Iglesias et al., 2018; El Ghossein et al., 2021). Due to the type of use envisioned for LIBs in space missions (most of their time in storage mode and only operated when high-power equipment ranging from 300 W to 2500 W is in use (NASA, 2022e)), this study focuses on the calendar aging of these batteries, and not degradation due to charge-discharge cycles.
Figure 1 illustrates that temperature, SOC and time are key factors related to calendar aging in LIBs via three main mechanisms: loss of lithium inventory (Mao et al., 2017), loss of active material in the electrodes (Lu et al., 2017), and a rise in cell internal impedance (Birkl et al., 2017; Li et al., 2019). The primary cause of loss of lithium inventory is the consumption of Li-ions by side reactions, including the creation of solid electrolyte interface (SEI) on the surface of graphite negative electrodes, the electrolyte breakdown processes and binder decomposition (Barré et al., 2013; Kabir and Demirocak, 2017). Such side reactions permanently devour Li-ions, rendering them inaccessible for further charge/discharge (Raj et al., 2020). Loss of active material is often caused by electrolyte decomposition, and electrode particle cracking during storage (Stiaszny et al., 2014; Kabir and Demirocak, 2017). Internal impedance in LIB increases owing to SEI growth, electrolyte degradation, and binder decomposition during storage (Kabir and Demirocak, 2017; Liu et al., 2022).
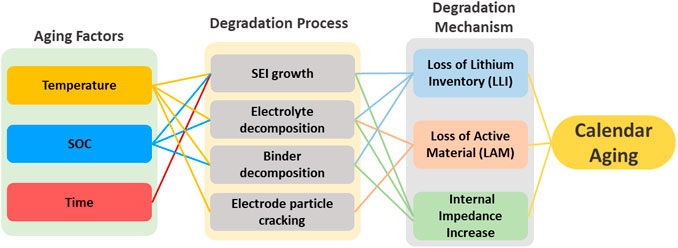
FIGURE 1. Overview of the factors accelerating the overall calendar aging rate (Birkl et al., 2017; Kabir and Demirocak, 2017; Li et al., 2019; Li et al., 2021).
Temperature and SOC are the two key factors that accelerate the processes that induce calendar aging in LIBs (Birkl et al., 2017; Li et al., 2019). In particular, SEI growth increases with as SOC, temperature and storage duration increases (Edge et al., 2021). Continuous expansion of the SEI layer leads to loss of lithium inventory and increase in internal impedance (Keil et al., 2016; Li et al., 2019). Furthermore, as the SEI layer thickens, some active material such as electrolyte become less electrochemically active, eventually resulting in negative electrode active material loss, which accelerates calendar aging (Keil et al., 2016; Li et al., 2019). In addition, SOC (above 90%) and high temperature (above 45°C) may accelerate binder degradation, which ultimately leads to calendar aging, as illustrated in Figure 1 (Kabir and Demirocak, 2017).
For some chemistries, storage temperatures of −27 °C and lower, degrade the performance of the LIBs (Dubarry and Devie, 2018; Li et al., 2021) and cause compressive strain and the deformation of the lattice on an atomic scale, leading to electrode particle cracking (Li et al., 2021). On the other hand, many LIB datasheets suggest that storage even at temperatures as low as −30 °C are acceptable (Samsung, 1865a; Samsung, 1865b).
Most researchers have concluded that the aging process slows down at low SOCs (Hahn et al., 2018; Naumann et al., 2018). However, discharging the battery below 0% SOC can cause the terminal voltage to decrease, resulting in performance and safety issues (Chen et al., 2021; Zhang et al., 2022). For instance, Yao et al. (Yao et al., 2019) and Guo et al. (Guo et al., 2016) indicate that dropping the terminal voltage of the battery well below the 0% SOC defined operation range (to values such as 0.2 V) may result in loss of electric contact, transition metal dissolution, and current collector corrosion, all of which lead to calendar aging.
Other factors, including mechanical stressors (externally imposed stack pressure), gamma radiation, and rapid gravitational fluctuations, have been found to influence calendar aging (NASA, 2009; Li et al., 2019; Logan et al., 2022). For instance, when stress exceeds a specific threshold, the electrode fails due to cracking or fracture (Li et al., 2019). Consequently, cell performance and capacity degrade significantly. Gamma radiation is another factor that may reduce the life of LIBs (NASA, 2022g). This kind of radiation can have an impact on the electrodes, electrolytes and separator, which will eventually lead to a decline in cell capacity (NASA, 2022g). Qiu et al. (Qiu et al., 2015) reported that irradiation caused a 36%–45% increase in particle size of the cathode material as well as crystal structure disordering. All of these factors contributed to an 8.4% loss in battery capacity after receiving the radiation dose of 2.744 Mrad. Additional effects, such as abrupt changes in gravity, are also observed, particularly in low earth orbit. It may also lead to mass and volume changes, which may have an impact on the lifespan of LIBs (Bagnardi et al., 2014). However, additional investigation is required to determine the actual contribution of gravity on the calendar ageing of LIBs.
To reduce the impact of stress factors, the battery storage in the space mission is carefully maintained in a regulated environment. For instance, to maintain environmental conditions, some battery packs are enclosed in an Inconel (oxidation-corrosion-resistant materials) pressure vessel (NASA, 2014; NASA, 2022g) or placed in an aluminum structure (Zaragoza-Asensio et al., 2021). These battery packs are further placed in an insulated bulkhead alongside power electronics and communication devices in a controlled environment (Zaragoza-Asensio et al., 2021; NASA, 2022h). In deep space missions, these factors are absent or their influence on calendar aging is minimal compared to the impacts of temperature and SOC (NASA, 2009). For example, NASA Johnson Space Center released a document (Calendar Life Aging of Two Models of 18650 Lithium Ion Cells (NASA, 1865)) in 2018 that only identifies temperature and SOC as the driving factors of lithium-ion calendar aging (deployed in human space flight applications).
To assess calendar aging, the experimental studies available in the literature usually focus on the LIB chemistry. Non-etheless, there are design decisions and components that may significantly influence the LIBs’ storage life (Geisbauer et al., 2020). For instance, the selection of an appropriate electrolyte as a crucial component to limit the capacity degradation during storage (Klein et al., 2022). Electrolytes are created by dissolving lithium salts in solvents and operate as ionic charge carriers (Xu and Angell, 2002). As one of the fundamental factors that determine the performance of LIBs, it is desirable for electrolytes to present a large potential window, great thermal and chemical stability, high ionic conductivity, a high Li + transference number, and a low barrier to desolvation of solvated Li+ (Wang and Zhong, 2015; Fan et al., 2021). Moreover, the thickness of the SEI layer varies depending on the kind of electrolyte. Kim et al. (Kim et al., 2011) evaluated the SEI growth in ethylene carbonate (EC), dimethyl carbonate (DMC), and combinations of these electrolytes. Results indicated that the growth of the SEI layer was reduced by 25% in the DMC electrolyte and by 13% in the DMC + EC combination as compared to the EC electrolyte. In addition, lithium plating is also significantly affected by the electrolyte composition (Lin et al., 2021). Nevertheless, the characteristics of the electrolytes change with time as well as with temperature and SOC. These phenomena must be considered in LIB chemistry research during calendar aging evaluations.
The electrolyte/electrode interphase layer is another component that might influence on calendar aging (Uitz et al., 2017). To ensure the improved electrochemical performance of the electrodes, it is essential to have an electrolyte/electrode interphase layer that is thin and stable with high ionic conductivity due to a side chemical reaction between the electrolyte and the electrode (Uitz et al., 2017; Pang et al., 2021). Appropriate electrolyte/electrode interphase is achieved by tuning electrolyte components, electrode material surface structures, and binders (Guo and Han, 2022). Additional additives, such as lithium difluoro borate (LiDFOB) (Xu et al., 2011), lithium difluorophosphate (LiPO2F2) (Yang et al., 2017) and flouro-o-phenylenedimaleimaide (F-MI) (Hamidah et al., 2019) are also added to LIB to improve its thermal stability and electrolyte/electrode interphase layer.
The active materials, conductive agent, and current collector in electrodes are linked together by binders (Kim et al., 2014). Although just a little amount of binder is used in the electrodes, it is critical to preserve electrode integrity and, hence, improve the electrochemical performance of LIBs. The electrochemical performance of LIBs may be enhanced by using binders with sufficient flexibility, electrolyte adsorption, and ionic conductivity (Mong et al., 2021). Polyvinylidene fluoride (PVDF) has been employed extensively as a binder for cathode/anode electrodes (Wang et al., 2019). Other binders like styrene-butadiene rubber/carboxymethylcellulose (SBR/CMC) are gaining popularity because of their improved cycle performance compared to PVDF (Wang et al., 2017; Jung et al., 2022). In addition, the features of each binder also alter with time as well as in response to temperature and SOC. Consequently, while designing LIBs for long-term storage, the selection of binder must also be considered. In addition to these factors, doping, surface coating, particle size selection, and the design of electrode material structures should be considered in calendar aging evaluations (Tang et al., 2015; Wang et al., 2015).
3 Empirical measurement of calendar aging
Table 2 presents a summary of works with experimental calendar aging data registered for the different chemistries and storage conditions that are found in the literature. Together with our own experiments and results, these are detailed in the following.
Geisbauer et al. (Geisbauer et al., 2021) conducted a calendar aging design of experiments of six LIB chemistries (NCA, NMC, LFP, LCO, LMO, and LTO-LCO) subject to three SOC settings (2%, 38%, and 100%) and three ambient temperature conditions (18.5°C, 50°C, and 60°C). The experimental measurements of the LIB chemistries were conducted from 120 days to 150 days Figure 2 depicts the capacity degradation over 120 days of the six chemistries under different storage conditions. The results demonstrated that various LIB chemistries degrade differently owing to calendar aging. Extreme circumstances, such as 100% SOC and higher temperatures (such as 60°C), cause increased capacity degradation and a rise in internal resistance in the majority of instances. Overall, the effects of calendar aging are more severe at 60°C storage than at 50°C storage.
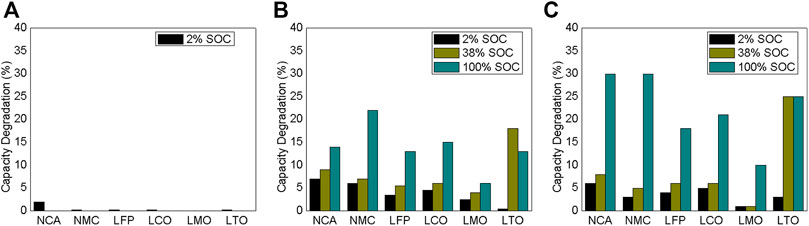
FIGURE 2. Capacity degradation of six chemistries over 120 days at storage temperature (A) 18.5°C, (B) 50°C, and (C) 60°C.
Keil et al. (Keil et al., 2016) conducted calendar aging experiments of NCA, NMC, and LFP at 25°C, 45°C, and 50°C with SOC values of 0%–100% (with an increment step of roughly 10%) over 300 days. After 9–10 months of storage, the LIBs’ capacity was measured at room temperature. It was observed that calendar aging did not increase linearly with SOC for any of the chemistry types Instead, there were stable areas where the capacity fade was almost constant, covering SOC ranges of more than 20%–30% of the cell capacity. Furthermore, it was also noted that the NMC cells had accelerated capacity fading at 100% SOC. For NCA cells, a storage SOC exceeding 90% produced a modest acceleration in battery aging. Overall, it was indicated that in order to improve battery life, LIB should not be stored at high SOC levels.
Eddahech et al. (Eddahech et al., 2015) conducted periodic calendar aging of four LIB technologies (NMC, LMO, NCA, and LFP) from 75 to 920 days at 45°C and 60 C with SOC values of 30%, 65%, and 100%. After extracting each cell for testing from the storage chamber, it was allowed to rest for 6 hours at room temperature to guarantee thermal stability prior to capacity testing. Then, two complete charge and discharge cycles at 1C were carried out to assess the cell’s capacity. The authors mainly concluded that the capacity fade is larger for LIBs containing manganese (such as LMO and NMC) than for NCA and LFP types under high-temperature (60 °C) environments. The reason is the manganese dissolution due to electrolyte reactions that occurred in LMO and NMC.
Sui et al. (Sui et al., 2021) conducted calendar again tests of LFP cells subject to five combinations of temperature and SOC over a period ranging from 189 to 301 days. The capacity of the cells was tested periodically at room temperature under a 1C constant current procedure during charging/discharging. It was observed that, during long-term storage, the battery deterioration followed a fairly regular trend and that the rate of capacity fading and rise in internal resistance got reduced as the aging progressed. Low storage temperatures (25 C) helped to minimize the rate of change in capacity and resistance. As storage temperatures rose from 25 C to 55°C, capacity and resistance increased exponentially. However, the SOC level had a piecewise influence on capacity fading. Before capacity fade reached 20%, which may be considered the cutoff point of LIB lifespan, a higher SOC (90%) was associated with relatively higher capacity fade. After the cutoff point, the impact of mid-SOC (50%) increased, resulting in a substantial loss of capacity.
Liu et al. (Liu et al., 2020) conducted calendar aging of NCA under nine different combinations of SOC levels (20%, 50% and 90%) and ambient temperature conditions (10°C, 25°C and 45°C) for 435 days. Periodic inspections were undertaken to determine the cell capacity throughout the experiment. For each test, all thermal chambers were warmed up to 25°C. LIBs were charged using a constant current-constant voltage profile, with 0.3 C in the constant current stage until the terminal voltage reached 4.2 V, followed by a constant voltage stage until the charging current dropped below 0.1 C. After 3 hours of rest, each cell was discharged with a continuous current profile of 0.3 C to the lower cutoff voltage of 2.5 V, from which the corresponding capacity value was determined. They showed that calendar aging accelerated with increasing SOC and temperature. The rate of capacity fading slowed down as the aging process continued. In addition, performance comparisons of several modelling strategies such as electrochemical models, semi-empirical models, and data-driven models were presented (Liu et al., 2020).
Our team at the University of Maryland’s Center for Advanced Life Cycle Engineering (CALCE) conducted a 6-month calendar aging assessment of 144 LCO batteries at four temperature settings (−40°C, −5°C, 25°C, and 50 C) and three SOC values (0%, 50%, and 100%) (Center for advanced life cycle engineering, 2022). The capacity of the batteries was determined using constant current constant voltage (CCCV) charging at a rate of 0.5 C (Center for advanced life cycle engineering, 2022). After 6-month, the results revealed that the capacity fade was almost negligible for all SOC situations when the temperature was below zero (−5°C and −40°C). On the contrary, substantial capacity deterioration was detected at 25°C and 50°C, increasing it with higher SOCs.
Hahn et al. (Hahn et al., 2018) determined the calendar aging of NMC, and Naumann et al. (Naumann et al., 2018) determined the calendar aging of LFP at various temperature and SOC combinations. Both showed that calendar aging increased with SOC and temperature.
In general, reducing the SOC and temperature during storage reduces calendar aging. However, for the case of the SOC influence, Dubarry et al. (Dubarry and Devie, 2018) demonstrated that reducing SOC accelerates the calendar aging of LTO (NMC + LCO). In addition, they demonstrated that as the temperature drops below zero (−27°C), there is an increase in capacity degradation. Li et al. (Li et al., 2021) suggest that compressive strain and atomic-scale lattice deformation leading to electrode particle cracking might be the root cause of the capacity degradation at subzero temperatures.
Uddin et al. (Uddin et al., 2017) found that capacity degradation for NCA is 2%–3% lower at 25°C than at 10°C. But Liu et al. (Liu et al., 2020) found the opposite; that capacity deterioration for NCA is greater at 25°C than at 10°C. Liu et al. (Liu et al., 2020) is more in line with Geisbauer et al. (Geisbauer et al., 2021). Keil et al. (Keil et al., 2016) conducted experiments at 25°C, 40°C, and 50°C, while Eddahech et al. (Eddahech et al., 2015) conducted at 45°C and 60°C. Because there is no calendar aging data at 10°C in these investigations, it is not possible to compare the capacity fading trend between 25°C and 10°C.
There are also some rare instances in which capacity initially rises during storage (Naumann et al., 2018; Geisbauer et al., 2021). In commercial LIBs, lithium atoms are retained during storage in the anode overhang area, which is the portion of the anode that extends beyond the cathode electrode. By performing multiple full cycles, the lithium atoms stored in the anode overhang areas may be retrieved (Naumann et al., 2018; Geisbauer et al., 2021). Naumann et al. (Naumann et al., 2018) suggested initial capacity increases due to the influence of this overhang effect, but capacity fading ultimately takes over resulting in an overall capacity loss over time.
The cited researchers have reported varying levels of deterioration under identical experimental conditions. For instance, Liu et al. (Liu et al., 2020) reported a residual capacity of 94% after 435 days at 10°C ambient temperature and 20% SOC for NCA. Under the same experimental conditions, Uddin et al. (Uddin et al., 2017) reported 85% remaining capacity over a shorter time of 385 days. Eddahech et al. (Eddahech et al., 2015) demonstrated a remaining capacity of 95% after 800 days at an ambient temperature of 60°C and SOC of 100%, while Geisbauer et al. (Geisbauer et al., 2021) indicated only 70% after 120 days for NCA. The variations are likely attributable to differences in the tested cells’ materials, including the electrolytes.
In order to facilitate the comparison of the multiple results in the literature, the published data for each type of chemistry has been normalized to percentage of degradation per year and represented in Figure 3. This figure shows this normalized degradation capacity for the different tested storage conditions.
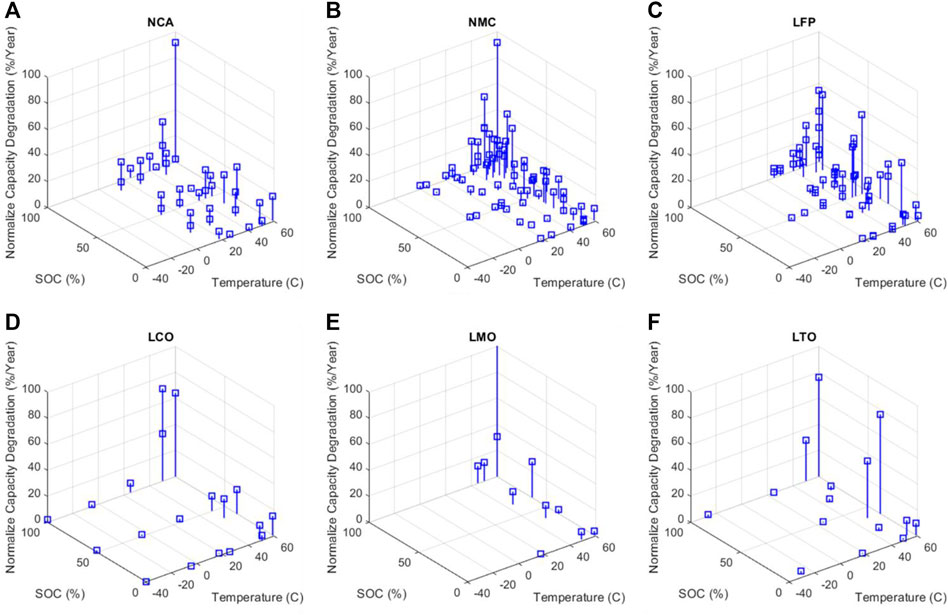
FIGURE 3. Empirical measurements of calendar aging reported in the literature.(A), (B), (C), (D), (E), (F).
Similar results are presented in Figure 4 which labels the degree of normalized degradation within certain storage ranges registered in average for each LIB chemistry.
As shown in Figure 4, the degradation of NCA is more than 3% per year at 20°C and 10% SOC, based on the results published by the majority of researchers (Liu et al., 2020; Geisbauer et al., 2021). Increases in temperature and SOC further enhance the normalized degradation. For instance, at 45°C or 70% SOC, the normalized degradation rate will grow around 10% per year.
NMC exhibits less than 1% deterioration at 20°C and 10% SOC. At 20°C and SOC between 0% and 100%, NMC chemistry exhibits superior performance over NCA with a degradation rate lower than 3% per year. By raising the temperature, the rate of degradation increases, although the impact is relatively less severe as compared to NCA chemistry.
For LFP, degradation is less than 1% per year at 20°C and 10% SOC. Even for temperatures ranging from 20°C to 45°C, LFP show less than 10% capacity degradation per year, which is relatively low as compared to NCA.
In the case of LCO, evaluation of its calendar aging has received relatively little attention in recent literature, as appreciated in Figure 3. According to the most recent data available, LCO shows less than 1% degradation at 20°C, but the capacity decreases by more than 10% on an annual basis when temperatures are higher than 45°C.
Like for the LCO chemistry, investigation into the calendar aging of LMO chemistry is limited. However, calendar aging data at temperatures higher than 20°C are available, and they show degradation of more than 10% each year.
In the case of LTO technology, research on the calendar aging of LTO-LCO and LTO-(NMC + LCO) shows that degradation is about 1%–3% per year at 20°C and 10% SOC. As the temperature drops from 20 °C to −27°C, the degradation rate increases (Dubarry and Devie, 2018).
In summary, it can be concluded from the published results that for temperatures in the range from 0°C to 60°C, regardless of the SOC, a unit increase in SOC implies an approximately doubled increase in the rate of degradation than that registered with a unit increase in temperature. Also, according to most researchers, decreasing SOC and temperature reduces calendar aging (Keil et al., 2016; Redondo-Iglesias et al., 2017; Naumann et al., 2018).
Finally, note that although just a few works have characterized the calendar aging of LIB at temperatures below 0°C, they can be stored at temperature as low as −20°C in the majority of cases (Samsung, 1865c; Samsung, 2170) and even −30°C in certain instances (Samsung, 1865a), based on the datasheet provided by manufacturers for different types of LIBs. It is therefore likely that the optimal temperature for calendar aging will depend on the LIB chemistry.
To evaluate the calendar aging evolution of all the LIB chemistries when subject to subzero temperatures in space, the following storage conditions are chosen: 0% SOC and 0°C, 0% SOC and −10°C, 0% SOC and −20°C, and 0% SOC and −30 °C.
4 Capacity degradation modeling
To estimate capacity loss during storage, different approaches such as electrochemical models (Ashwin et al., 2018; Liu et al., 2020), semi-empirical models (Sarasketa-Zabala et al., 2014; Redondo-Iglesias et al., 2017), and data-driven models (Lucu et al., 2018; Li et al., 2019) are used in the literature. In this work, a semi-empirical mathematical model is utilized to estimate the capacity deterioration of LIB chemistries. This is defined as:
where a1, a2, b1, b2, and c1 are the fitting parameters, SOC is state of charge ranges 0 to 1, T is temperature in Kelvin and t is time in days. The fitting parameters for each chemistry are obtained using MATLAB’s curve fitting tool and are based on the data sets shown in Table 2. Table 3 displays the values of the fitting parameters for each chemistry obtained during the analysis.
Figure 5 represents the capacity degradation evolution with time obtained for each type of LIB using Eq. 1 and the parameters in Table 3 at four different temperatures. Even with identical storage conditions, different references report varying trends and deterioration values for the same chemistry. This divergence is shown in Figure 5 and is represented by the deviation range (also referred to as the confidence interval).
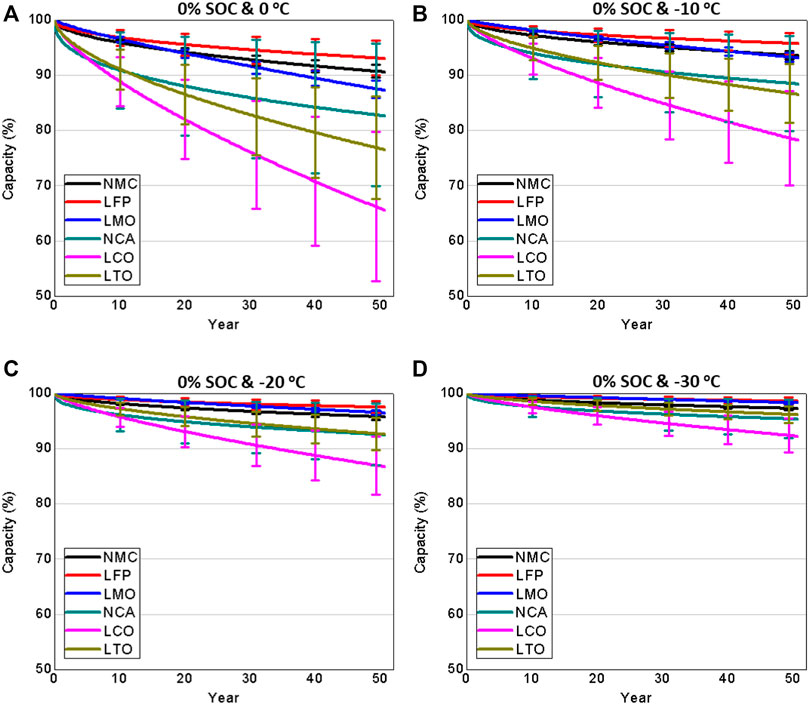
FIGURE 5. Capacity degradation of various LIB chemistries at various storage conditions (A) 0% SOC and 0 C (B) 0% SOC and –10 °C (C) 0% SOC and –20 C and (D) 0% SOC and –30 C.
For 0% SOC and 0 C storage condition, the results demonstrate that LFP presents a 7% capacity degradation, whereas NMC has a 9% after 50 years, as shown in Figure 5 a). In addition, the confidence interval is less than 3%, indicating that the majority of researchers working with LFP and NMC present a similar pattern and capacity degradation. After 50 years, LMO capacity degrades by 13% with confidence interval of less than 2%. In addition, NCA has a capacity degradation of around 18%, with confidence interval of 16%, indicating that different research centers report significantly different degradation rates for this chemistry. LTO capacity degrade by 24% and LCO by 35%, with confidence interval of around 10% for both chemistries.
When the storage temperature is reduced to −10°C, all chemistries exhibit a lower capacity degradation. For instance, both NMC and LFP exhibit a capacity decline of just 6% over a period of 50 years, as shown in Figure 5B. In addition, LTO capacity degrades by around 14%, whereas LCO capacity degrades by approximately 22%.
As noted, some manufacturers have low temperature storage limits as low as −20 C or −30 C (Samsung, 1865a; Samsung, 1865c; Samsung, 2170). By lowering the storage temperature of LIBs to −20 C or −30°C, capacity loss after 50 years can be reduced to less than 8% for nearly all chemistries, as shown in Figures 5C,D.
5 Conclusion
Current deep-space mission energy sources are based on RTGs, which have low specific power and cannot be employed alone to meet spacecraft peak power requirements. Rechargeable batteries can provide energy for additional power requirements but there have been concerns about their long-term degradation in storage conditions. LIBs are good candidates for deep space missions because of their technical benefits (specific energy, specific power, and cycle life) over Ni-H2 and Ni-Cd batteries. This study investigated the capacity degradation of LIBs during storage as a function of time, temperature and SOC conditions.
Significant effort has been devoted in the literature to determine the calendar aging of different battery chemistries under varying experimental conditions. Published results demonstrate that NMC and LFP degrade by less than 1% per year at low temperatures (less than 20°C) and low SOC (less than 10%). In comparison to NMC and LFP batteries, all other chemistries exhibit greater calendar aging.
Results from the semi-empirical model developed to fit the calendar aging published experimental datasets show that, depending on the specific chemistry, LIBs degrade between 7% and 35% at 0°C and 0% SOC during a 50-year period. The capacity degradation is 7% for LFP and 9% for NMC at 0% SOC and 0°C with a confidence interval of around 3%, indicating similar patterns among published results when subjected to identical temperature and SOC conditions. Results at −10°C and 0% SOC show that both LFP and NMC capacity degrade by about 6%. In addition, if LIBs are stored at −20°C or −30°C and 0% SOC, the capacity loss could be less than 8% for nearly all the chemistries.
For the chemistries (LTO and LCO) which have the highest capacity degradation when subject to calendar aging, at 0% SOC and 0°C, the capacity fade of 24% for LTO and 35% for LCO, with confidence intervals of 10% in the predicted value, could be acceptable for a deep space mission. For the LFP and NMC chemistries, there is the potential to maintain over 90% capacity for a 50-year deep space mission.
Data availability statement
The original contributions presented in the study are included in the article/supplementary material, further inquiries can be directed to the corresponding author.
Author contributions
MP drafted the paper, and with HB and Miss NL conceptualized the work and defined the approach. HA conducted the technical assessment and contributed to the drafts.
Acknowledgments
The authors thank the more than 150 companies and organizations that support research activities at the Center for Advanced Life Cycle Engineering (CALCE) at the University of Maryland, the Centre for Advanced Innovations in Reliability and Safety (CAIRS) in conjunction with Polytechnic University of Hong Kong and Ravin Singh for his insights.
Conflict of interest
The authors declare that the research was conducted in the absence of any commercial or financial relationships that could be construed as a potential conflict of interest.
Publisher’s note
All claims expressed in this article are solely those of the authors and do not necessarily represent those of their affiliated organizations, or those of the publisher, the editors and the reviewers. Any product that may be evaluated in this article, or claim that may be made by its manufacturer, is not guaranteed or endorsed by the publisher.
References
Ali, H., Khan, H. A., and Pecht, M. G. (2021). Circular economy of Li batteries: Technologies and trends. J. Energy Storage 40, 102690. doi:10.1016/j.est.2021.102690
Ali, H., Khan, H. A., and Pecht, M. (2022). Preprocessing of spent lithium-ion batteries for recycling: Need, methods, and trends. Renew. Sustain. Energy Rev. 168, 112809. doi:10.1016/j.rser.2022.112809
Ashwin, T., Barai, A., Uddin, K., Somerville, L., McGordon, A., and Marco, J. (2018). Prediction of battery storage ageing and solid electrolyte interphase property estimation using an electrochemical model. J. Power Sources 385, 141–147. doi:10.1016/j.jpowsour.2018.03.010
Bagnardi, M., Poland, M. P., Carbone, D., Baker, S., Battaglia, M., and Amelung, F. (2014). Gravity changes and deformation at Kīlauea Volcano, Hawaii, associated with summit eruptive activity, 2009–2012. J. Geophys. Res. Solid Earth 119 (9), 7288–7305. doi:10.1002/2014JB011506
Bairstow, B., Lee, Y. H., and Oxnevad, K. (2018). “Mission analysis for next-generation RTG study,” in Proceeedings of the IEEE Aerospace Conference, Big Sky, MT, USA, 03-10 March 2018, 1–19. doi:10.1109/AERO.2018.8396411
Baraskar, A., Yoshimura, Y., Nagasaki, S., and Hanada, T. (2022). Space solar power satellite for the Moon and Mars mission. J. Space Saf. Eng. 9 (1), 96–105. doi:10.1016/j.jsse.2021.10.008
Barré, A., Deguilhem, B., Grolleau, S., Gérard, M., Suard, F., and Riu, D. (2013). A review on lithium-ion battery ageing mechanisms and estimations for automotive applications. J. Power Sources 241, 680–689. doi:10.1016/j.jpowsour.2013.05.040
Center for advanced life cycle engineering (CALCE) (2022). Storage Data and Test Description. Available at: https://calce.umd.edu/battery-data (accessed Sep 8, 2022).
Beltran, H., Ayuso, P., Vicente, N., Beltrán-Pitarch, B., García-Canadas, J., and Pérez, E. (2022). Equivalent circuit definition and calendar aging analysis of commercial Li (NixMnyCoz) O2/graphite pouch cells. J. Energy Storage 52, 104747. doi:10.1016/j.est.2022.104747
Bennett, G. L. (2018). “Space applications,” in CRC handbook of thermoelectrics (Boca Raton, FL: CRC Press), 515–538.
Bennett, G. (2006). “Space nuclear power: Opening the final frontier,” in Proceeedings of the4th International Energy Conversion Engineering Conference and Exhibit (IECEC), San Diego, CA, 26-29 June, 2006, 4191.
Bierhaus, E., Clark, B. C., Harris, J. W., Payne, K. S., Dubisher, R. D., Wurts, D. W, et al. (2018). The OSIRIS-REx spacecraft and the touch-and-go sample acquisition mechanism (TAGSAM). Space Sci. Rev. 214 (7), 1–46. doi:10.1007/s11214-018-0521-6
Birkl, C. R., Roberts, M. R., McTurk, E., Bruce, P. G., and Howey, D. A. (2017). Degradation diagnostics for lithium ion cells. J. Power Sources 341, 373–386. doi:10.1016/j.jpowsour.2016.12.011
bitstream (2014). Batteries and fuel cells in space. Available at: https://trs.jpl.nasa.gov/bitstream/handle/2014/17938/99-1388.pdf?sequence=1 (accessed Jan 2, 2023).
Brewer, J. C. (2001). The 2000 NASA aerospace battery workshop. NASA/CP-2001-210883. Available at: https://ntrs.nasa.gov/citations/20010083604.
Bugga, R. V., and Brandon, E. J. (2020). Energy storage for the next generation of robotic space exploration. Electrochem. Soc. Interface 29 (1)–59. doi:10.1149/2.F08201IF
Bushnell, D. M., Moses, R. W., and Choi, S. H. (2021). Front. space power energy. Available at: https://en.wikipedia.org/wiki/Space-based_solar_power.
Chayambuka, K., Mulder, G., Danilov, D. L., and Notten, P. H. (2020). From li-on batteries toward Na-ion chemistries: Challenges and opportunities. Adv. Energy Mater. 10 (38), 2001310. doi:10.1002/aenm.202001310
Chen, Y., Li, Y., Liang, Z., He, X., Li, X., Tavajohi, N., et al. (2021). A review of lithium-ion battery safety concerns: The issues, strategies, and testing standards. J. Energy Chem. 59, 83–99. doi:10.1016/j.jechem.2020.10.017
Clarke, E., Gates, A., Birch, J., Davis, S., Horkley, B., Rich, L., et al. (2022). Multi-Mission Thermoelectric Generator Fueling Testing and Integration Operations for Mars 2020. Nucl. Technol. 208, s11–s17. doi:10.1080/00295450.2022.2031497
Deroy, C., Dobley, A., Gitzendanner, R., and Morrison, E. (2019). Next generation materials for lithium-ion space batteries. ECS Meet. Abstr. 57, 2452. doi:10.1149/MA2019-02/57/2452
Designnews (2022). Space batteries go from infinity to even further. Available at: https://www.designnews.com/batteryenergy-storage/space-batteries-go-infinity-even-further (accessed Oct 2, 2022).
Diaz, L. B., Marinescu, M., Barreras, J. V., Patel, Y., Offer, G., et al. (2020). Meta-review of fire safety of lithium-ion batteries: Industry challenges and research contributions. J. Electrochem. Soc. 167 (9), 090559. doi:10.1149/1945-7111/aba8b9
Dubarry, M., and Devie, A. (2018). Battery durability and reliability under electric utility grid operations: Representative usage aging and calendar aging. J. Energy Storage 18, 185–195. doi:10.1016/j.est.2018.04.004
Durka, M. J., Barry, M. M., Woerner, D. F., Wielgosz, S. E., Fleurial, J. P., Drymiotis, F., et al. (2022). “A novel high-performance mission-enabling multi-purpose radioisotope heat source,” in Proceedings of the 2022 IEEE Aerospace Conference (AERO), Big Sky, MT, USA, 05-12 March 2022 (IEEE), 1–10. doi:10.1109/AERO53065.2022.9843667
Eddahech, A., Briat, O., and Vinassa, J.-M. (2015). Performance comparison of four lithium–ion battery technologies under calendar aging. Energy 84, 542–550. doi:10.1016/j.energy.2015.03.019
Edge, J. S., Prosser, R., Kirkaldy, N. D., Patel, A. N., Hales, A., Ghosh, A., Ai, W., et al. (2021). Lithium ion battery degradation: What you need to know. Phys. Chem. Chem. Phys. 23 (14), 8200–8221. doi:10.1039/D1CP00359C
Ehrenfried, M. (2022). “Perseverance’s design,” in Perseverance and the Mars 2020 mission (Berlin, Germany: Springer), 27–74. doi:10.1007/978-3-030-92118-7_3
El Ghossein, N., Sari, A., Venet, P., Genies, S., and Azaïs, P. (2021). Post-Mortem analysis of lithium-ion capacitors after accelerated aging tests. J. Energy Storage 33, 102039. doi:10.1016/j.est.2020.102039
Energy, O. o. N. (2022). What is a radioisotope power system? Available at: https://www.energy.gov/ne/articles/what-radioisotope-power-system (accessed Oct 2, 2022).
Esa, E. S. A. (2022). Spacecraft power for ulysses. Available at: https://www.cosmos.esa.int/web/ulysses/rtg (accessed Oct 2, 2022).
Fan, P., Liu, H., Marosz, V., Samuels, N. T., Suib, S. L., Sun, L., Liao, L., et al. (2021). High performance composite polymer electrolytes for lithium-ion batteries. Adv. Funct. Mater. 31 (23), 2101380. doi:10.1002/adfm.202101380
Forbes (2021). NASA’s new ‘voyager’: 7 things to know about the 50-year ‘interstellar probe’ mission to burst our cosmic bubble. Available at: https://www.forbes.com/sites/jamiecartereurope/2021/04/27/nasas-new-voyager-7-things-to-know-about-the-50-year-interstellar-probe-mission-to-burst-our-cosmic-bubble/?sh=104f9e596279 (accessed Oct 2, 2022).
Frobes (2015). Peak plutonium-238? U.S. Starts making nuclear fuel for deep space missions. Available at: https://www.forbes.com/sites/williampentland/2015/11/08/peak-plutonium-238-u-s-starts-making-nuclear-fuel-for-deep-space-missions/?sh=4b42977b53b4 (accessed Oct 29, 2022).
Geisbauer, C., Wöhrl, K., Koch, D., Wilhelm, G., Schneider, G., and Schweiger, H.-G. (2021). Comparative study on the calendar aging behavior of six different lithium-ion cell chemistries in terms of parameter variation. Energies 14 (11), 3358. doi:10.3390/en14113358
Geisbauer, C., Wöhrl, K., Mittmann, C., and Schweiger, H.-G. (2020). Review of safety aspects of calendar aged lithium ion batteries. J. Electrochem. Soc. 167 (9), 090523. doi:10.1149/1945-7111/ab89bf
Gravseth, I. J., and Pieper, B. (2013). CloudSat’s return to the A-Train. Int. J. Space Sci. Eng. 51 (4), 410–431. doi:10.1504/IJSPACESE.2013.059269
Grolleau, S., Delaille, A., Gualous, H., Gyan, P., Revel, R., Bernard, J., Redondo-Iglesias, E., et al. (2014). Calendar aging of commercial graphite/LiFePO4 cell–Predicting capacity fade under time dependent storage conditions. J. Power Sources 255, 450–458. doi:10.1016/j.jpowsour.2013.11.098
Guo, R., and Han, W. (2022). The effects of electrolytes, electrolyte/electrode interphase and binders on lithium-ion batteries at low temperature. Mater. Today Sustain. 19, 100187. doi:10.1016/j.mtsust.2022.100187
Guo, R., Lu, L., Ouyang, M., and Feng, X. (2016). Mechanism of the entire overdischarge process and overdischarge-induced internal short circuit in lithium-ion batteries. Sci. Rep. 6 (1), 1–9. doi:10.1038/srep30248
Gusev, V., Pustovalov, A., Rybkin, N., Anatychuk, L., Demchuk, B., and Ludchak, I. Y. (2011). Milliwatt-power radioisotope thermoelectric generator (RTG) based on plutonium-238. J. Electron. Mater. 40 (5), 807–811. doi:10.1007/s11664-011-1579-z
Hahn, S. L., Storch, M., Swaminathan, R., Obry, B., Bandlow, J., and Birke, K. P. (2018). Quantitative validation of calendar aging models for lithium-ion batteries. J. Power Sources 400, 402–414. doi:10.1016/j.jpowsour.2018.08.019
Halpert, G., Frank, H., and Surampudi, S. (1999). Batteries and fuel cells in space. Electrochem. Soc. Interface 8 (3), 25. doi:10.1149/2.F06993IF
Hamidah, N. L., Wang, F. M., and Nugroho, G. (2019). The understanding of solid electrolyte interface (SEI) formation and mechanism as the effect of flouro-o-phenylenedimaleimaide (FMI) additive on lithium-ion battery. Surf. Interface Analysis 51 (3), 345–352. doi:10.1002/sia.6586
Harvey, P., Taylor, E., Sterling, R., and Cully, M. (2008). The THEMIS constellation. Space Sci. Rev. 141 (1), 117–152. doi:10.1007/978-0-387-89820-9_6
Hendricks, T., Boca, A., Woerner, D. F., Donitz, B., and Bairstow, B. K. (2019). Solar Power System and Radioisotope Thermoelectric Generation Technologies at Jupiter-Saturn-Uranus Environments: New Insights and Paradigms. Pasadena, CA: Jet Propulsion Laboratory, National Aeronautics and Space.
Hepp, A. F., Kumta, P. N., Velikokhatnyi, O. I., and Datta, M. K. (2022). Batteries for aeronautics and space exploration: Recent developments and future prospects. Lithium-Sulfur Batter. 2022, 531–595. doi:10.1016/B978-0-12-819676-2.00011-6
Houtmann, J. (2020). Enhancing NASA's multi-mission radioisotope thermoelectric generator using highly efficient thermoelectric materials. Maneto Undergrad. Res. J. 3 (1). doi:10.15367/m:turj.v3i1.318
Institute, C. E. (2022). What is a lithium-ion battery and how does it work? Available at: https://www.cei.washington.edu/education/science-of-solar/battery-technology/ (accessed Oct 3, 2022).
Jones, J.-P., Smart, M. C., Krause, F. C., West, W. C., and Brandon, E. J. (2022). Batteries for robotic spacecraft. Joule 6 (5), 923–928. doi:10.1016/j.joule.2022.04.004
Jung, H. Y., Lee, J. S., Han, H. T., Jung, J., Eom, K., and Lee, J. T. (2022). Lignin-based materials for sustainable rechargeable batteries. Polymers 14 (4), 673. doi:10.3390/polym14040673
Kabir, M., and Demirocak, D. E. (2017). Degradation mechanisms in Li-ion batteries: A state of the art review. Int. J. Energy Res. 41 (14), 1963–1986. doi:10.1002/er.3762
Keil, P., Schuster, S. F., Wilhelm, J., Travi, J., Hauser, A., Karl, R. C., Jossen, A., et al. (2016). Calendar aging of lithium-ion batteries. J. Electrochem. Soc. 163 (9), A1872. doi:10.1149/2.0411609jes
Kim, J.-M., Park, H.-S., Park, J.-H., Kim, T.-H., Song, H.-K., and Lee, S.-Y. (2014). Conducting polymer-skinned electroactive materials of lithium-ion batteries: Ready for monocomponent electrodes without additional binders and conductive agents. ACS Appl. Mater. Interfaces 6 (15), 12789–12797. doi:10.1021/am502736m
Kim, S.-P., Van Duin, A. C., and Shenoy, V. B. (2011). Effect of electrolytes on the structure and evolution of the solid electrolyte interphase (SEI) in Li-ion batteries: A molecular dynamics study. J. Power Sources 196 (20), 8590–8597. doi:10.1016/j.jpowsour.2011.05.061
Kinnison, J., Vaughan, R., Hill, P., Raouafi, N., Guo, Y., and Pinkine, N. (2020). “Parker solar probe: A mission to touch the sun,” in Proceedings of the 2020 IEEE Aerospace Conference (IEEE), Big Sky, MT, USA, 07-14 March 2020, 1–14. doi:10.1109/AERO47225.2020.9172703
Klein, S., Haneke, L., Harte, P., Stolz, L., Wickeren, S., Borzutzki, K., Nowak, S., et al. (2022). Suppressing electrode crosstalk and prolonging cycle life in high voltage Li ion batteries: Pivotal role of fluorophosphates in electrolytes. ChemElectroChem 9 (13), e202200469. doi:10.1002/celc.202200469
Li, J., Li, S., Zhang, Y., Yang, Y., Russi, S., Qian, G., Mu, L., Lee, S. J., et al. (2021). Multiphase, multiscale chemomechanics at extreme low temperatures: Battery electrodes for operation in a wide temperature range. Adv. Energy Mater. 11 (37), 2102122. doi:10.1002/aenm.202102122
Li, Y., Liu, K., Foley, A. M., Zulke, A., Berecibar, M., Nanini-Maury, E., Van Mierlo, J., et al. (2019). Data-driven health estimation and lifetime prediction of lithium-ion batteries: A review. Renew. Sustain. energy Rev. 113, 109254. doi:10.1016/j.rser.2019.109254
Lin, X., Khosravinia, K., Hu, X., Li, J., and Lu, W. (2021). Lithium plating mechanism, detection, and mitigation in lithium-ion batteries. Prog. Energy Combust. Sci. 87, 100953. doi:10.1016/j.pecs.2021.100953
Liu, J., Yue, M., Wang, S., Zhao, Y., and Zhang, J. (2022). A review of performance attenuation and mitigation strategies of lithium-ion batteries. Adv. Funct. Mater. 32 (8), 2107769. doi:10.1002/adfm.202107769
Liu, K., Ashwin, T., Hu, X., Lucu, M., and Widanage, W. D. (2020). An evaluation study of different modelling techniques for calendar ageing prediction of lithium-ion batteries. Renew. Sustain. Energy Rev. 131, 110017. doi:10.1016/j.rser.2020.110017
Logan, E., Eldesoky, A., Liu, Y., Lei, M., Yang, X., Hebecker, H., Luscombe, A., Johnson, M. B., et al. (2022). The effect of LiFePO4 particle size and surface area on the performance of LiFePO4/graphite cells. J. Electrochem. Soc. 169 (5), 050524. doi:10.1149/1945-7111/ac6aed
Lu, T., Luo, Y., Zhang, Y., Luo, W., Yan, L., and Xie, J. (2017). Degradation analysis of commercial lithium-ion battery in long-term storage. J. Electrochem. Soc. 164 (4), A775. doi:10.1149/2.1321704jes
Lucu, M., Martinez-Laserna, E., Gandiaga, I., and Camblong, H. (2018). A critical review on self-adaptive Li-ion battery ageing models. J. Power Sources 401, 85–101. doi:10.1016/j.jpowsour.2018.08.064
Mao, Z., Farkhondeh, M., Pritzker, M., Fowler, M., and Chen, Z. (2017). Calendar aging and gas generation in commercial graphite/NMC-LMO lithium-ion pouch cell. J. Electrochem. Soc. 164 (14), A3469. doi:10.1149/2.0241714jes
Matadi, B. P., Genies, S., Delaille, A., Waldmann, T., Kasper, M., Wohlfahrt-Mehrens, M., et al. (2017). Effects of biphenyl polymerization on lithium deposition in commercial graphite/NMC lithium-ion pouch-cells during calendar aging at high temperature. J. Electrochem. Soc. 164 (6), A1089. doi:10.1149/2.0631706jes
Matthes, C. S., Woerner, D. F., Hendricks, T. J., Fleurial, J. P., Oxnevad, K. I., Barklay, C. D., et al. (2018). “Next-generation radioisotope thermoelectric generator study,” in Proceeedings of the 2018 IEEE Aerospace Conference (IEEE), Big Sky, MT, USA, 03-10 March 2018, 1–9. doi:10.1109/AERO.2018.8396738
Mong, A. L., Shi, Q. X., Jeon, H., Ye, Y. S., Xie, X. L., and Kim, D. (2021). Tough and flexible, super ion conductive electrolyte membranes for lithium based secondary battery applications. Adv. Funct. Mater. 31 (12), 2008586. doi:10.1002/adfm.202008586
Nasa (2016). Batteries at nasa - today and beyond. Available at: https://ntrs.nasa.gov/citations/20160004091 (accessed Oct 2, 2022).
Nasa (2022a). Battery applications for NASA's missions - a historical perspective. Available at: https://www.arpa-e.energy.gov/sites/default/files/documents/files/Miller_RANGE_Kickoff_2014.pdf (accessed Dec 12, 2022).
Nasa (2022b). Calendar life aging of two models of 18650 lithium ion cells. Available at: https://ntrs.nasa.gov/citations/20180003308 (accessed Dec 12, 2022).
Nasa (2022c). Cassini RTG. Available at: https://rps.nasa.gov/resources/158/cassini-rtg/ (accessed Sep 12, 2022).
Nasa (2023a). Commercial nickel cadmium batteries for space use: A proven alternative for leo satellite power storage. Available at: https://adsabs.harvard.edu/full/1998ESASP.416.715C (accessed Jan 2, 2023).
Nasa (2022d). Electrical power supply and distribution subsystems. Available at: https://solarsystem.nasa.gov/basics/chapter11-3/ (accessed Nov 2, 2022).
Nasa (2022e). Energy storage technologies for future space science missions. Available at: https://solarsystem.nasa.gov/resources/295/energy-storage-technologies-for-future-space-science-missions/ (accessed Dec 12, 2022).
Nasa (2022f). ExoMars rover battery modeling and life tests. Available at: https://www.nasa.gov/sites/default/files/atoms/files/2-exomars_rover_battery_tests_gdudley.pdf (accessed Dec 24, 2022).
Nasa (2023b). Guidelines on lithium-ion battery use in space applications. Available at: https://ntrs.nasa.gov/citations/20090023862 (accessed Jan 1, 2023).
Nasa (2022g). Mars global surveyor (MGS) spacecraft loss of contact. Available at: https://www.nasa.gov/sites/default/files/174244main_mgs_white_paper_20070413.pdf (accessed Nov 2, 2022).
Nasa, M. (2022h). Electrical power. Available at: https://mars.nasa.gov/mars2020/spacecraft/rover/electrical-power/ (accessed Oct 2, 2022).
Nasa (2022i). New Horizons mission powered by space radioisotope power systems. Available at: https://www.energy.gov/ne/articles/new-horizons-mission-powered-space-radioisotope-power-systems (accessed Sep 12, 2022).
Nasa (2022j). NiH2 battery reconditioning for LEO applications. Available at: https://ntrs.nasa.gov/citations/19970013728 (accessed Oct 29, 2022).
Nasa (2022k). Power generation and energy storage. Available at: https://www.nasa.gov/sites/default/files/atoms/files/edwards_060822.pdf (accessed Oct 2, 2022).
Nasa (2022L). Radioisotope thermoelectric generators (RTGs). Available at: https://solarsystem.nasa.gov/missions/cassini/radioisotope-thermoelectric-generator/ (accessed Sep 12, 2022).
Nasa (2022m). Rover temperature controls. Available at: https://mars.nasa.gov/mer/mission/rover/temperature/#:∼:text=Like%20the%20human%20body%2C%20the,Fahrenheit%20to%20104%C2%B0%20Fahrenheit (accessed Oct 2, 2022).
Nasa (2023c). Spacewalkers complete multi-year effort to upgrade space station batteries. Available at: https://www.nasa.gov/feature/spacewalkers-complete-multi-year-effort-to-upgrade-space-station-batteries (accessed Jan 2, 2023).
Nasa (2022n). Voyager 1 and 2. Available at: https://rps.nasa.gov/missions/12/voyager-1-2/ (accessed Sep 12, 2022).
Naumann, M., Schimpe, M., Keil, P., Hesse, H. C., and Jossen, A. (2018). Analysis and modeling of calendar aging of a commercial LiFePO4/graphite cell. J. Energy Storage 17, 153–169. doi:10.1016/j.est.2018.01.019
Nssc, N. S. S. C. (2030). China’s deep-space exploration to. Available at: http://english.nssc.cas.cn/ns/NU/201410/W020141016603613379886.pdf (accessed Oct 29, 2022).
nuclear-energy (2022). ISRO plans for nuclear energy use in space. Available at: https://www.orfonline.org/expert-speak/isro-plans-for-nuclear-energy-use-in-space/ (accessed Oct 29, 2022).
Ogumi, Z. (2020). “Batteries: present and future energy storage challenges,” in Present LIB chemistries (Hoboken, NY, USA: John Wiley and Sons), 149.
Pang, Y., Pan, J., Yang, J., Zheng, S., and Wang, C. (2021). Electrolyte/electrode interfaces in all-solid-state lithium batteries: A review. Electrochem. Energy Rev. 4 (2), 169–193. doi:10.1007/s41918-020-00092-1
Preger, Y., Barkholtz, H., Fresquez, A., Campbell, D., Juba, B., Romàn-Kustas, J., et al. (2020). Degradation of commercial lithium-ion cells as a function of chemistry and cycling conditions. J. Electrochem. Soc. 167 (12), 120532. doi:10.1149/1945-7111/abae37
Qiu, J., Barkholtz, H. M., Fresquez, A., Campbell, D. L., Juba, B. W., et al. (2015). Effects of neutron and gamma radiation on lithium-ion batteries. Nucl. Instrum. Methods Phys. Res. Sect. B Beam Interact. Mater. Atoms 345, 27–32. doi:10.1016/j.nimb.2014.12.058
Raj, T., Wang, A. A., Monroe, C. W., and Howey, D. A. (2020). Investigation of path dependent degradation in lithium-ion batteries. Batter. Supercaps 3 (12), 1377–1385. doi:10.1002/batt.202000160
Redondo-Iglesias, E., Venet, P., and Pelissier, S. (2018). Calendar and cycling ageing combination of batteries in electric vehicles. Microelectron. Reliab. 88, 1212–1215. doi:10.1016/j.microrel.2018.06.113
Redondo-Iglesias, E., Venet, P., and Pelissier, S. (2017). Eyring acceleration model for predicting calendar ageing of lithium-ion batteries. J. Energy Storage 13, 176–183. doi:10.1016/j.est.2017.06.009
Saftbatteries (2023). How Saft batteries made space travel possible. Available at: https://www.saftbatteries.com/media-resources/our-stories/how-saft-batteries-made-space-travel-possible (accessed Jan 2, 2023).
S. Agency, T. E. (2022). Nobel-winning lithium-ion batteries powering space. Available at: https://www.esa.int/Enabling_Support/Space_Engineering_Technology/Nobel-winning_lithium-ion_batteries_powering_space (accessed Oct 2, 2022).
Samsung (2022a). INR21700-50E. Available at: https://cdn.shopify.com/s/files/1/0481/9678/0183/files/samsung_50e-2_1d3ffd2c-0e25-4f97-9f01-15cc1539f57f.pdf?v=1663389721 (accessed Dec 24, 2022).
Samsung (2022b). INR18650-20S. Available at: https://www.imrbatteries.com/content/samsung_20S.pdf (accessed Dec 24, 2022).
Samsung (2023c). INR18650-30Q. Available at: https://www.18650batterystore.com/products/samsung-30q (accessed Jan 2, 2023).
Samsung (2022c). INR18650-32E. Available at: https://cdn.shopify.com/s/files/1/0481/9678/0183/files/Samsung_32E_Specification_Sheet.pdf?v=1614810892 (accessed Dec 24, 2022).
Sarasketa-Zabala, E., Gandiaga, I., Rodriguez-Martinez, L., and Villarreal, I. (2014). Calendar ageing analysis of a LiFePO4/graphite cell with dynamic model validations: Towards realistic lifetime predictions. J. Power Sources 272, 45–57. doi:10.1016/j.jpowsour.2014.08.051
Schmalstieg, J., Käbitz, S., Ecker, M., and Sauer, D. U. (2014). A holistic aging model for Li (NiMnCo) O2 based 18650 lithium-ion batteries. J. Power Sources 257, 325–334. doi:10.1016/j.jpowsour.2014.02.012
Schmitt, J., Maheshwari, A., Heck, M., Lux, S., and Vetter, M. (2017). Impedance change and capacity fade of lithium nickel manganese cobalt oxide-based batteries during calendar aging. J. Power Sources 353, 183–194. doi:10.1016/j.jpowsour.2017.03.090
Shmelev, A., Geraskin, N., Kulikov, G., Kulikov, E., Apse, V., and Glebov, V. (2020). The problem of large-scale production of plutonium-238 for autonomous energy sourcesJournal of Physics: Conference Series. IOP Publ. 1689 (1), 012030. doi:10.1088/1742-6596/1689/1/012030
Shodiev, A., Primo, E., Arcelus, O., Chouchane, M., Osenberg, M., Hilger, A., et al. (2021). Insight on electrolyte infiltration of lithium ion battery electrodes by means of a new three-dimensional-resolved lattice Boltzmann model. Energy Storage Mater. 38, 80–92.
Skyatnightmagazine (2022). The biggest European Space Agency missions of the coming decade. Available at: https://www.skyatnightmagazine.com/space-missions/european-space-agency-future-missions/ (accessed Oct 29, 2022).
Smithrick, J. J., and O'Donnell, P. M. (1996). Nickel-hydrogen batteries-An overview. J. Propuls. power 12 (5), 873–878. doi:10.2514/3.24116
Spacenews (2022). China to launch a pair of spacecraft towards the edge of the solar system. Available at: https://spacenews.com/china-to-launch-a-pair-of-spacecraft-towards-the-edge-of-the-solar-system/ (accessed Oct 2, 2022).
Spaceref (2022). Integral, ESA’s scientific observatory built by alenia spazio, in safe orbit after perfect launch. Available at: https://spaceref.com/press-release/integral-esas-scientific-observatory-built-by-alenia-spazio-in-safe-orbit-after-perfect-launch/ (accessed Oct 2, 2022).
Stiaszny, B., Ziegler, J. C., Krauß, E. E., Zhang, M., Schmidt, J. P., and Ivers-Tiffée, E. (2014). Electrochemical characterization and post-mortem analysis of aged LiMn2O4–NMC/graphite lithium ion batteries part II: Calendar aging. J. Power Sources 258, 61–75. doi:10.1016/j.jpowsour.2014.02.019
Sui, X., Świerczyński, M., Teodorescu, R., and Stroe, D.-I. (2021). The degradation behavior of LiFePO4/C batteries during long-term calendar aging. Energies 14 (6), 1732. doi:10.3390/en14061732
Surampudi, S., Bugga, R., Smart, M. C., Narayanan, S., Frank, H. A., and Halpert, G. (2006). Overview of energy storage technologies for space applications. Pasadena, CA: Jet Propulsion Laboratory, National Aeronautics and Space.
Tang, Y., Zhang, Y., Li, W., Ma, B., and Chen, X. (2015). Rational material design for ultrafast rechargeable lithium-ion batteries. Chem. Soc. Rev. 44 (17), 5926–5940. doi:10.1039/C4CS00442F
Thierfelder, H. (1982). “DSCS 3 Life Test Progress Report,” in The 1981 GSFC Battery Workshop, Greenbelt, MD, November 17-19, 1981, 293–304.
Tran, M. K., DaCosta, A., Mevawalla, A., Panchal, S., and Fowler, M. (2021). Comparative study of equivalent circuit models performance in four common lithium-ion batteries: LFP, NMC, LMO, NCA. Batteries 7 (3), 51. doi:10.3390/batteries7030051
Uddin, K., Jackson, T., Widanage, W. D., Chouchelamane, G., Jennings, P. A., and Marco, J. (2017). On the possibility of extending the lifetime of lithium-ion batteries through optimal V2G facilitated by an integrated vehicle and smart-grid system. Energy 133, 710–722. doi:10.1016/j.energy.2017.04.116
Uitz, M., Sternad, M., Breuer, S., Taubert, C., Traussnig, T., Hennige, V., Hanzu, I., Wilkening, M., et al. (2017). Aging of tesla's 18650 lithium-ion cells: Correlating solid-electrolyte-interphase evolution with fading in capacity and power. J. Electrochem. Soc. 164 (14), A3503. doi:10.1149/2.0171714jes
Urban-Klaehn, J., Miller, D., Gross, B. J., Tyler, C. R., and Dwight, C. C. (2021). Initial phase of Pu-238 production in Idaho national laboratory. Appl. Radiat. Isotopes 169, 109517. doi:10.1016/j.apradiso.2020.109517
Walvekar, H., Beltran, H., Sripad, S., and Pecht, M. (2022). Implications of the electric vehicle manufacturers’ decision to mass adopt lithium-iron phosphate batteries. IEEE Access 10, 63834–63843. doi:10.1109/ACCESS.2022.3182726
Wang, K. X., Li, X. H., and Chen, J. S. (2015). Surface and interface engineering of electrode materials for lithium-ion batteries. Adv. Mater. 27 (3), 527–545. doi:10.1002/adma.201402962
Wang, M., Hu, J., Wang, Y., and Cheng, Y.-T. (2019). The influence of polyvinylidene fluoride (PVDF) binder properties on LiNi0. 33Co0. 33Mn0. 33O2 (NMC) electrodes made by a dry-powder-coating process. J. Electrochem. Soc. 166 (10), A2151. doi:10.1149/2.1171910jes
Wang, R., Feng, L., Yang, W., Zhang, Y., Zhang, Y., Bai, W., Liu, B., et al. (2017). Effect of different binders on the electrochemical performance of metal oxide anode for lithium-ion batteries. Nanoscale Res. Lett. 12 (1), 1–11. doi:10.1186/s11671-017-2348-6
Wang, Y., and Zhong, W. H. (2015). Development of electrolytes towards achieving safe and high performance energy storage devices: A review. ChemElectroChem 2 (1), 22–36. doi:10.1002/celc.201402277
Winter, M., Barnett, B., and Xu, K. (2018). Before Li ion batteries. Chem. Rev. 118 (23), 11433–11456. doi:10.1021/acs.chemrev.8b00422
Witkowski, M. M., Vane, D., and Livermore, T. (2018). “CloudSat-life in daylight only operations (DO-Op),” in Proceedings of the 2018 SpaceOps Conference, France, Europe, October 2018, 2562.
Woerner, D. (2018). Rtgs: The Enduring and the future. Pasadena, CA: Jet Propulsion Laboratory, National Aeronautics and Space.
World-nuclear (2022). Nuclear reactors and radioisotopes for space. Available at: https://world-nuclear.org/information-library/non-power-nuclear-applications/transport/nuclear-reactors-for-space.aspx (accessed Oct 29, 2022).
Xu, K., and Angell, C. A. (2002). Sulfone-based electrolytes for lithium-ion batteries. J. Electrochem. Soc. 149 (7), A920. doi:10.1016/j.elecom.2009.03.020
Xu, M., Zhou, L., Hao, L., Xing, L., Li, W., and Lucht, B. L. (2011). Investigation and application of lithium difluoro (oxalate) borate (LiDFOB) as additive to improve the thermal stability of electrolyte for lithium-ion batteries. J. Power Sources 196 (16), 6794–6801. doi:10.1016/j.jpowsour.2010.10.050
Yang, G., Shi, J., Shen, C., Wang, S., Xia, L., Hu, H., Luo, H., et al. (2017). Improving the cyclability performance of lithium-ion batteries by introducing lithium difluorophosphate (LiPO 2 F 2) additive. RSC Adv. 7 (42), 26052–26059. doi:10.1039/c7ra03926c
Yao, K. P., Okasinski, J. S., Kalaga, K., Almer, J. D., and Abraham, D. P. (2019). Operando quantification of (de) lithiation behavior of silicon–graphite blended electrodes for lithium-ion batteries. Adv. Energy Mater. 9 (8), 1803380. doi:10.1002/aenm.201803380
Yee, J. H., Cameron, G. E., and Kusnierkiewicz, D. Y. (1999). Overview of timed. Opt. Spectrosc. Tech. Instrum. Atmos. space Res. III 3756, 244–254.
Zaghib, K., Dontigny, M., Guerfi, A., Charest, P., Rodrigues, I., et al. (2011). Safe and fast-charging Li-ion battery with long shelf life for power applications. J. power sources 196 (8), 3949–3954. doi:10.1016/j.jpowsour.2010.11.093
Zaragoza-Asensio, J. A., Pindado, S., and Pérez-Álvarez, J. (2021). Li-ion battery for space missions based on COTS cells: Mechanical analysis and design. Egypt. J. Remote Sens. Space Sci. 24 (2), 311–317. doi:10.13009/EUCASS2019-1004
Zhang, G., Wei, X., Chen, S., Zhu, J., Han, G., and Dai, H. (2022). Unlocking the thermal safety evolution of lithium-ion batteries under shallow over-discharge. J. Power Sources 521, 230990. doi:10.1016/j.jpowsour.2022.230990
Zheng, J.-S., Zhang, L., Shellikeri, A., Cao, W., Wu, Q., and Zheng, J. P. (2017). A hybrid electrochemical device based on a synergetic inner combination of Li ion battery and Li ion capacitor for energy storage. Sci. Rep. 7 (1), 1–8. doi:10.1038/srep41910
Keywords: calendar aging, lithium-ion batteries (LIB), NASA, space mission, storage
Citation: Ali H, Beltran H, Lindsey NJ and Pecht M (2023) Assessment of the calendar aging of lithium-ion batteries for a long-term—Space missions. Front. Energy Res. 11:1108269. doi: 10.3389/fenrg.2023.1108269
Received: 25 November 2022; Accepted: 18 January 2023;
Published: 07 February 2023.
Edited by:
Yang Zhao, Western University, CanadaReviewed by:
Jian Liu, University of British Columbia-Okanagan Campus, CanadaJian Qin, Xi’an University of Technology, China
Copyright © 2023 Ali, Beltran, Lindsey and Pecht. This is an open-access article distributed under the terms of the Creative Commons Attribution License (CC BY). The use, distribution or reproduction in other forums is permitted, provided the original author(s) and the copyright owner(s) are credited and that the original publication in this journal is cited, in accordance with accepted academic practice. No use, distribution or reproduction is permitted which does not comply with these terms.
*Correspondence: Hayder Ali, Hayder.ali@lums.edu.pk