- 1Merchant Marine College, Shanghai Maritime University, Shanghai, China
- 2Shanghai Marine Diesel Engine Research Institute, Shanghai, China
- 3Shenzhen Huawei Offshore Shipping Transport Co., Ltd, Shenzhen, China
In recent years, the rapid increase of CO2 emission has caused severe environmental issues. The environmental concern has made how to reduce the carbon emissions become a hot topic. Many scholars and research teams believe that the organic amine chemical absorption technology is the most favored and promising carbon capture technology due to its highly CO2 removal effectiveness. However, it is not applied wildly in industrial environment since the desorption process energy consumption is too much, over 4 GJ/t CO2. Many researchers report that catalysts can help to reduce the desorption energy. And it is generally assumed that four key properties of solid acid catalysts determined the performance of solid acid catalysts in the process of CO2 desorption: the total number of acid sites; specific surface area; the ratio of Brønsted acid sites to Lewis acid sites; the amount of Brønsted acid sites. Therefore, this paper reviews the recent research on the effect of different catalysts on the energy consumption of CO2 desorption and the progress of research on improving catalyst performance. Also, it provides views on the possible problems in practical industrial applications.
Introduction
Global warming has become an international hot issue (Montzka et al., 2011; Rhodes, 2016), and CO2 is widely considered as a significant contributor to the global warming due to its greenhouse effect (Kerr, 2006; Barzagli and Mani, 2021; Huang et al., 2021). Human society has emitted huge amount of CO2 since the beginning of the industrial revolution (AR5 Synthesis Report: Climate Change, 2014; Godin et al., 2021) and CO2 concentrations have been increased from an average of 280 ppm in pre-industrial times to 410–419 ppm in 2019–2021 (Benhelal et al., 2021; Rashid et al., 2022). Usually, these anthropogenic CO2 emissions are from industries like petrochemicals, cement, refineries, and power plants (Freund, 2003; Peters et al., 2013; Mercure et al., 2018); since the beginning of the industrial revolution, human society has developed to the point where today fossil fuels meet 85% of the global energy demand nowadays (David and Herzog, 2000; Uyanga and Idem, 2007; Yang et al., 2008; Herzog et al., 2012; Zhang et al., 2014). And the years ahead, what’s more, it is estimated that fossil fuels will remain the primary energy source for decades (Agarwal et al., 2010; Scott et al., 2013; Shah et al., 2023). And the massive consumption of fossil fuels will continue to generate large amounts of CO2 emissions (Chen et al., 2018; Chuenphan et al., 2022). So, it will still accelerate global warming, glacier melting, seawater acidification, sea level rise and other greenhouse effect problems (Doney et al., 2009; Foster and Rohling, 2013; Xiao et al., 2016; Sun et al., 2022). As a result, how to reduce and effectively utilize CO2 has become a hot issue for the world (Rao and Rubin, 2019; Fu et al., 2022). The current capture methods for CO2 capture include pre-combustion capture, oxygen-enriched combustion and post-combustion capture (Schreiber et al., 2009; Zhou et al., 2010; Xia et al., 2020). Due to the low cost of the retrofit work on existing equipment (Wang et al., 2011; Zhao et al., 2013; Wang et al., 2017), post-combustion capture technology is more widely used in industry (Dou et al., 2010; Bhown and Freeman, 2011). The technology captures CO2 from the flue gas of combustion equipment selectively (boilers and gas engines, etc.) by using chemical or physical methods (Li et al., 2016; Fu et al., 2022). It mainly includes adsorption, low-temperature distillation, membrane, biomimetic, physical, and chemical absorption technology (Davy, 2009; McGurk et al., 2017; Yousef et al., 2017; Luis Míguez et al., 2018; Liu et al., 2022). Among these methods, the chemical absorption is applied widely in industries because of its large absorption capacity, fast absorption rate (Shakerian et al., 2015), ability to handle large amounts of gas, and applicability to low CO2 partial pressure gases (Muchan et al., 2022). Amine-based solvent is the solvent commonly used in chemical absorption methods (Rochelle, 2009), for example, monoethanolamine (MEA), butylethanolamine (BEA), methyldiethanolamine (MDEA), butyldiethanolamine (BDEA), 2-amino-2-methyl-1-propanol (AMP) (Nakrak et al., 2023), piperazine (PZ) (Carson et al., 2000; Zhao et al., 2023), listed in Table 1. Among all the amine solution, the monoethanolamine (MEA) is most used because of its relatively low cost, excellent CO2 absorption performance and strong reactivity to CO2 even at very low CO2 partial pressures (Lepaumier et al., 2009; Muchan et al., 2017; Narku-Tetteh et al., 2017). However, this technology still faces three drawbacks that prevent its widespread industrial application, the relatively high energy consumption of CO2 desorption, the degradation of amine solvents and the corrosion of equipment (Goff and Rochelle, 2004; Lepaumier et al., 2011; Liang et al., 2015; Barzagli et al., 2018; Bui et al., 2018). The high desorption energy consumption is considered the most significant drawback (Romeo et al., 2008; Lin and Rochelle, 2016; Ji et al., 2018; Herzog et al., 2022), with the CO2 desorption process accounting for 70%–80% of the total energy consumption of the whole CCS system (Oyenekan and Gradworks, 2007; Haszeldine, 2009; Shi et al., 2018). Therefore, the key to realizing the industrial application of the chemical absorption method to capture CO2 is to reduce the energy consumption of the desorption process of CO2(Singh and Versteeg, 2008).
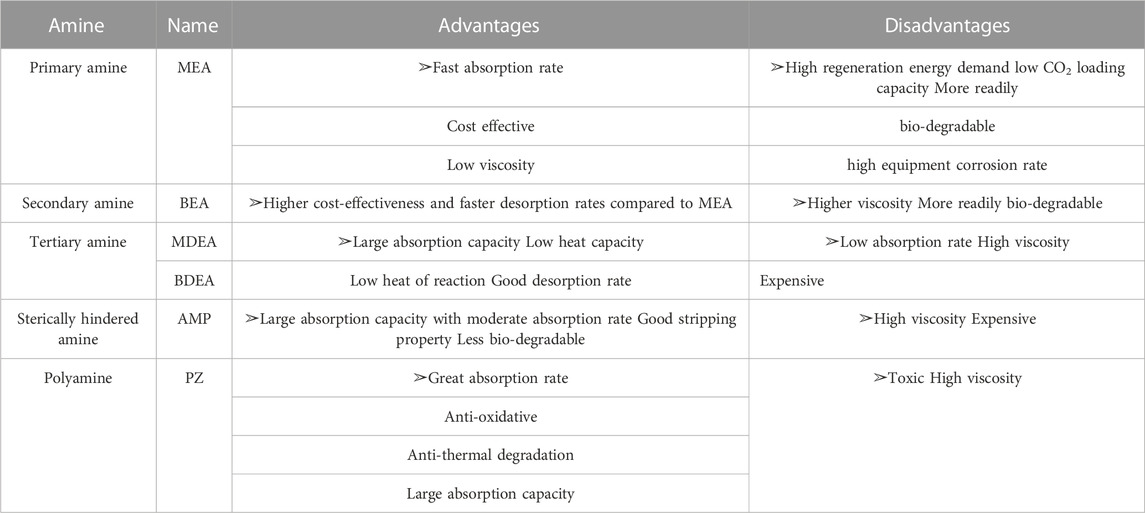
TABLE 1. Advantages and disadvantages of solvents (Li et al., 2022).
The regeneration duty of amine process involves three parts: the desorption heat to break chemical bonds in CO2-absorbed products, the sensible heat to raise the temperature of the CO2-loaded solution and the latent heat to produce the water vapor (Li et al., 2015; Zhang et al., 2018c), and among which sensible heat and latent heat can be reduced by process improvement, according to existing reports (Le Moullec et al., 2014; Wang et al., 2015; Decardi-Nelson et al., 2017). However, the overall energy consumption of the MEA process can be reduced by about 30% by process improvement; the regeneration energy still accounts for more than 50% of the total energy consumption of the carbon capture system (Oyenekan and Rochelle, 2006; Rochelle, 2009). The main reason is that the temperature of the CO2 desorption process is usually high (393K–413 K) (Finotello et al., 2008; Yu et al., 2012), which causes a large proportion of the heat to be used to boil the water in the solution (Sakwattanapong et al., 2005). In other words, the low energy utilization efficiency is attributed to the high temperature required for desorption. Therefore, if the regeneration temperature can be controlled below the boiling point of the water, the energy consumption for CO2 desorption will be significantly reduced. Furthermore, high regeneration temperature can cause equipment corrosion (Li et al., 2016). Therefore, the key to controlling the energy consumption of chemical absorption desorption is to achieve CO2 desorption at lower temperatures.
Research has found that absorptive thermal regeneration can be replaced by integrating the absorption and mineralization of CO2, and this process can achieve CO2 regeneration at 40 °C by usually using MEA solvent as the absorber and fly ash as the regeneration; however, the current development of this process is limited by the reaction rate of the CO2 mineralization process (Ji et al., 2018; Rashid et al., 2020). In addition, adding catalysts can improve CO2 desorption efficiency at low temperatures, reducing the temperature required for solvent regeneration (Idem et al., 2011). It has led to an increasing focus on developing new catalysts by researchers. We conducted a brief review of relevant research in recent years, as shown in Table 2. Introducing catalysts into MEA solutions makes it possible to change the proportion of sensible heat, latent heat of vaporization, and chemical reaction heat in the total heat demand effectively. Additionally, by reducing the activation energy of the reaction and increasing the effective collision frequency, the CO2 desorption rate can be significantly increased. Therefore, MEA solutions containing catalysts exhibit faster desorption rates and lower CO2 desorption energy consumption than blank experiments under the same experimental conditions, such as temperature and pressure. And it is attributed to the presence of Brønsted acidic sites or Lewis acidic sites in the catalyst, which effectively promote the decomposition of carbamates and the deprotonation of protonated amines in MEA solutions (Srisang et al., 2018). And the advantages of catalytic desorption are shown in Figure 1. In this paper, we present the mechanism of the promotion of the regeneration of organic amine solutions by Brønsted acid catalysts (represented by HZSM-5), Lewis acid catalysts (represented by γ-Al2O3) and acid-base bifunctional catalysts and highlight four characteristics that affect the catalytic performance of solid acid catalysts: the total number of acid sites, specific surface area, especially mesoporous surface area (MSA), the number of acid sites, and the number of pores. We also compared the disparities in operating conditions between laboratory settings and engineering applications. We discussed potential challenges in the engineering application of catalysts and presented various viewpoints. Finally, we concisely compared the advantages and disadvantages of different catalysts in terms of cost, stability and environmental impact. As shown in Table 3.
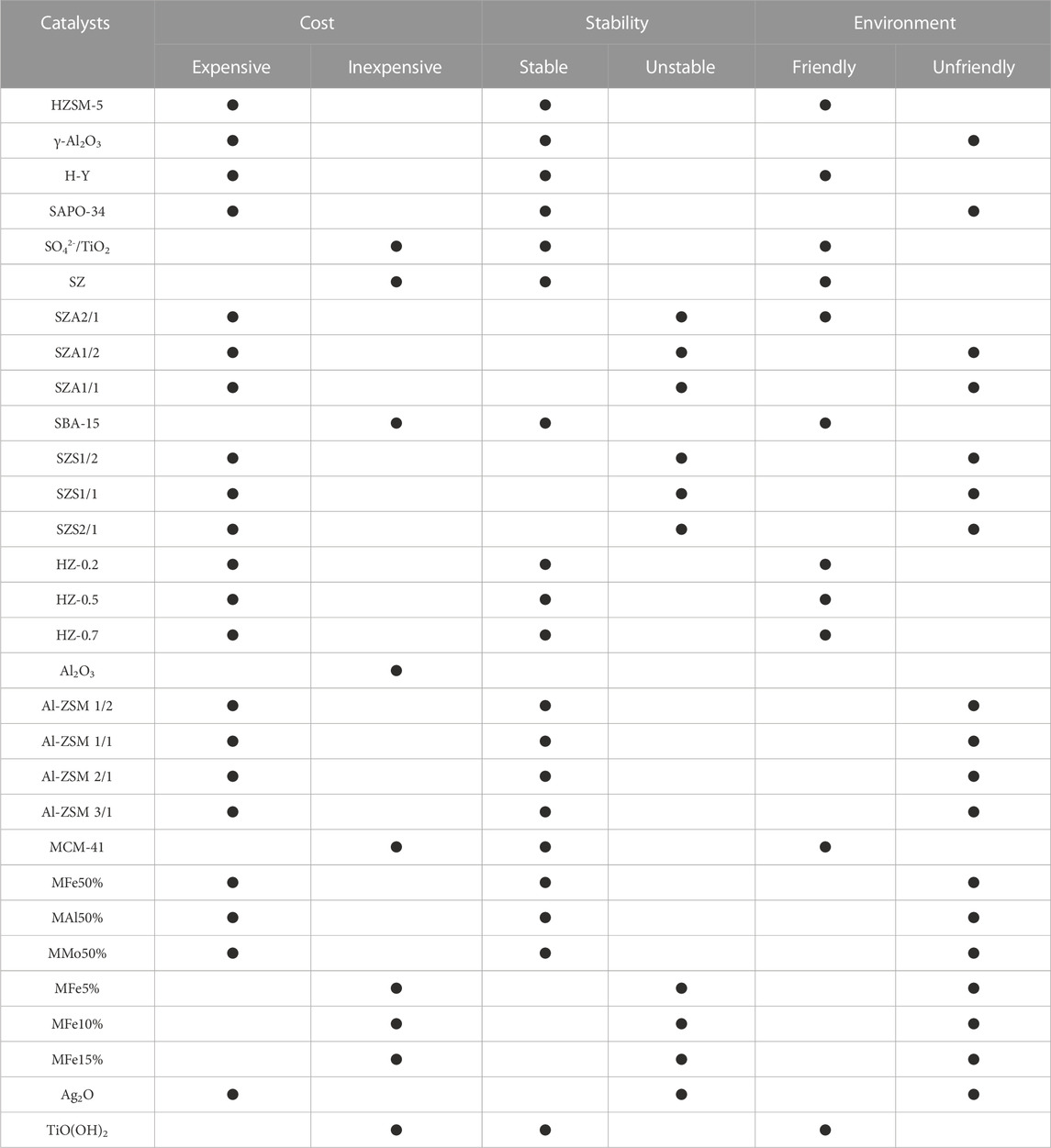
TABLE 3. The advantages and disadvantages of different catalysts in terms of cost, stability and environmental impact.
Solid acid catalyst
The CO2 absorption and desorption process can be described according to the amphiphilic ion mechanism proposed by Caplow et al. (Caplow, 1968). Firstly the absorption process of tertiary amines produces amphoteric ions when reacting with CO2, and subsequently, these amphoteric ions decompose to form carbamates and protonated amines; according to this mechanism, the regeneration process can be carried out in two main steps: the decomposition of carbamates (MEACOO−) and the deprotonation of protonated amines (MEAH+) (Afari et al., 2018; Li et al., 2023).
Zwitterion formation:
Carbamate formation:
Protonated amine formation:
Decomposition of carbamate (MEACOO-):
Deprotonation of protonated amine (MEAH+):
For the whole regeneration process of MEA solution MEACOO-decomposition process needs enough protons (H3O+) as reactants to participate in the reaction; in the absence of a catalyst, deprotonation of protonated amine becomes the primary source of protons in Reaction (4), however, due to the high alkalinity of MEA which makes the deprotonation of protons from MEAH+ has a very high energy potential barrier, it is hard for the reaction equilibrium of Reaction (5) to toward the right side, which means that in this case, the deprotonation of MEAH+ becomes the most crucial rate-limiting step of the whole reaction, in addition to the fact that the MEACOO− decomposition is a strongly heat-absorbing reaction with a high demand for heat load. Feng et al. (Feng et al., 2010; Du et al., 2011) proposed adjusting the amine-rich solution’s pH with weak acids (octanedioic acid, phthalic acid, oxalic acid, etc.) to provide more acid protons and promote the desorption process of CO2. These weak acids should have the characteristic of increasing their solubility in water with increasing temperature, thereby reducing the pH value of the amine solution to promote CO2 desorption. However, as the temperature decreases, the solubility of weak acids also decreases, allowing them to crystallize and precipitate from the amine solution. The results showed that adding weak acids could reduce the energy consumption of rich amine solution regeneration. Still, the residual weak acid dissolved in the lean amine solution reduces the CO2 absorption performance significantly. Inspired by this method, Tontiwachwuthikul et al. proposed using solid acid catalysts to promote CO2 desorption and reduce the energy consumption of solvent regeneration (Shi et al., 2014b; Srisang et al., 2017). Idem et al. (Idem et al., 2011) proposed adding HZSM-5 (mainly a proton donor or Brønsted acid) and γ-Al2O3 (mainly a solid electron acceptor or Lewis acid) as two solid acids in the amine-rich solution to reduce the regeneration load of the solvent. Liang et al. (Liang et al., 2016) further advanced this approach by developing various solid acid catalysts (including TiO2, Al2O3 and HZSM-5). They demonstrated that their addition to the MEA solvent regeneration step could reduce the regeneration heat demand by up to 34%.
Shi et al. (Shi et al., 2014b) conducted experiments to verify that two different catalysts (γ-Al2O3 and HZSM-5) did not exhibit any degradation effect on amine solutions during a continuous one-week test. Liang et al. (Liang et al., 2016) investigated the catalytic regeneration of CO2-rich MEA solutions at 378 K. They found that HZSM-5, γ-Al2O3, and H-Y could effectively improve the desorption rate of CO2 and reduce energy consumption during desorption. By comparing the MSA and the ratio of Brønsted acid sites to Lewis acid sites of the three catalysts, they found that higher MSA and a higher ratio of Brønsted acid sites to Lewis acid sites could enhance the catalytic performance of the catalysts. Furthermore, they explored the performance of solid acid catalysts based on the combined effect of these two factors, using the product of B/L and MSA (B/L*MSA) as a binding value. The results showed the higher the binding value of these two features, the faster the rate of catalytic CO2 desorption, which also indirectly reflected that, compared to the Lewis acid sites on the catalyst surface, the Brønsted acid sites played a more active role. Srisang et al. (Srisang et al., 2017) experimentally investigated the role of solid acid catalysts (including HZSM-5 and γ-Al2O3) in reducing the thermal load of CO2 desorption from MEA solution. They showed that HZSM-5 promoted carbamate decomposition by providing free protons and increased the absorption efficiency by 38% compared to non-catalytic conditions while reducing the thermal load by 42%. Compared to non-catalytic conditions, γ-Al2O3 improved absorption efficiency by 23.6% and reduced heat load by 30%. By comparing the application of HZSM-5, γ-Al2O3, H-Y and SiO2- Al2O3 in the desorption process of CO2, the correlation between the specific surface area of the catalyst, the ratio of Brønsted acid sites to Lewis acid sites, the total acid amount and other characteristics on the cycle capacity, absorption efficiency and thermal load of MEA absorbent was analyzed by regression. The results indicated that the acid strength had the most significant effect on reducing the heat load in the desorption of solid acid catalysts. It is followed by a larger ratio of Brønsted acid sites to Lewis acid sites for the catalytic desorption of CO2. In addition, Liu et al. (Liu et al., 2017) investigated the impact of three different catalysts (HZSM-5, MCM-41, and SO42-/ZrO2) with varying total acid sites, mesoporous surface areas, and Brønsted and Lewis acid sites on thermal loading and CO2 desorption rates using an intermittent reaction device. The results revealed that the catalytic performance of the three catalysts, in terms of thermal loading efficiency and CO2 desorption rate in single and mixed amines, followed the order of HZSM-5 > MCM-41 > SO42-/ZrO2. The NH3-TPD results showed that the acidic strength of the three catalysts followed the order of SO42-/ZrO2 > HZSM-5 > MCM-41. Although HZSM-5 was moderately acidic compared to MCM-41 and SO42-/ZrO2, it exhibited the best catalytic performance among the three catalysts due to its more significant Brønsted acid site to Lewis acid site ratio. Zhang et al. (Zhang et al., 2017) experimented with studying the impact of adding two solid acid catalysts, SAPO-34 and SO42-/TiO2, to MEA solutions with high CO2 content on the energy demand for solvent regeneration. As depicted in Figure 2, Figure 3, Figure 4, the experimental results indicate that the catalyst’s performance correlates strongly with its surface properties, which comprise the total acid amount, mesoporous surface area, Brønsted acid sites to Lewis acid sites ratio, and the interplay between these surface properties.
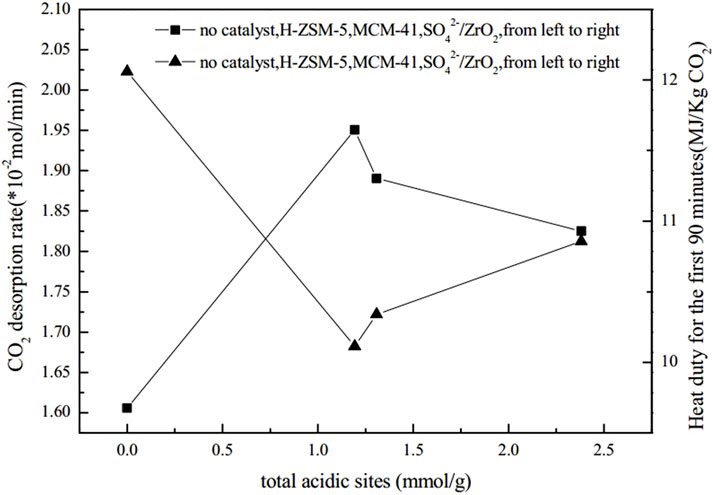
FIGURE 2. Influence of total acid sites on heat duty and CO2 desorption rate (Liu et al., 2017)
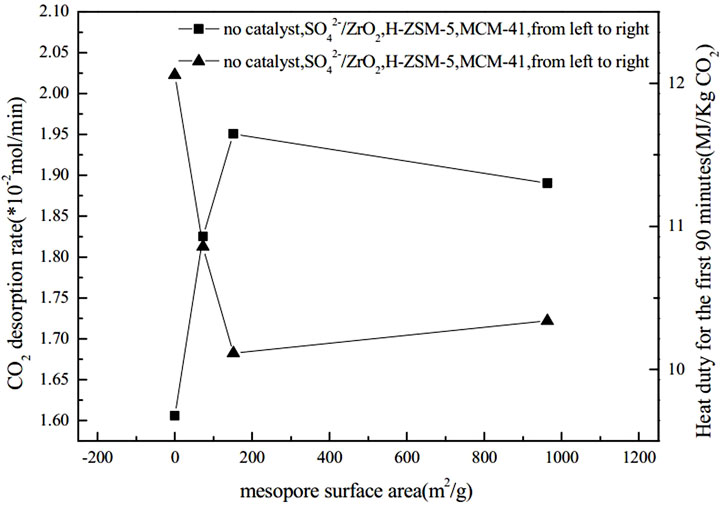
FIGURE 3. Influence of mesopore surface area on heat duty and CO2 desorption rate (Liu et al., 2017)
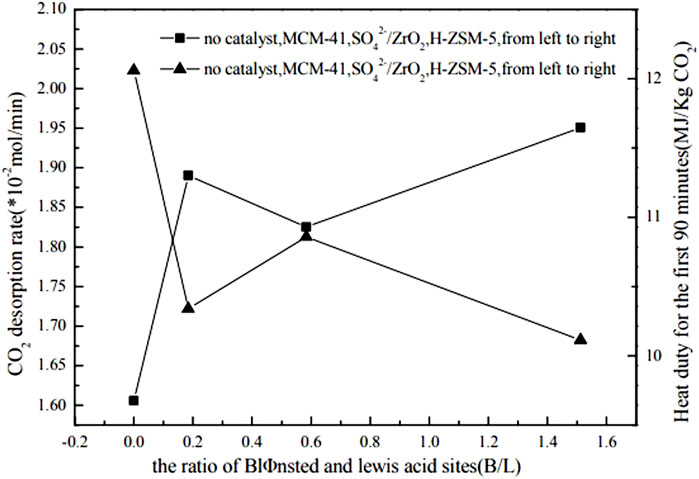
FIGURE 4. Influence of the ratio of Brønsted and Lewis acid sites (B/L) on heat duty and CO2 desorption rate (Liu et al., 2017)
Brønsted acid catalysts
Brønsted acid catalysts possess multiple Brønsted acidic sites, which can provide a significant amount of H+ to participate in the carbamate decomposition reaction during the CO2 desorption process (Shi et al., 2014a). On the other hand, the catalytic active sites that have lost protons can be restored by obtaining acidic protons from MEAH+. A proposed catalytic mechanism of the Brønsted acid catalyst for CO2 desorption is shown in Figure 5 (Idem et al., 2011). Three active sites on the carbamate are involved in the desorption reaction of CO2: a) the nitrogen atom, which is the crucial active center because the decomposition of the carbamate involves the dissociation of the C-N bond; b) the oxygen anion on the carbonyl group, which can serve as a carrier for the acidic proton (H+); c) another site located on the oxygen atom of the carbonyl group, which is a suitable attacking site for the catalyst (Idem et al., 2011; Zhang et al., 2019). With the addition of a solid acid catalyst (in the case of HZSM-5), the system can now provide protons even without the deprotonation step. MEACOO− in CO2-rich MEA solution is converted to MEACOOH after being on the surface of HZSM-5. Then, MEACOOH undergoes chemical adsorption on the catalyst surface through the bonding of O atoms and Al atoms. The H atom attached to the O atom migrates to the adjacent N atom; and this transformation converts MEACOOH into a zwitterion. As a result of the H atom transfer, the N-C bond begins to stretch and weaken, leading to the attachment of N+ to a second Al atom on the catalyst surface, while the N-C bond begins to break. After the bond breakage, the zwitterion becomes MEA and CO2; since the solubility of CO2 is low at high temperatures, CO2 will transfer to the gas phase.
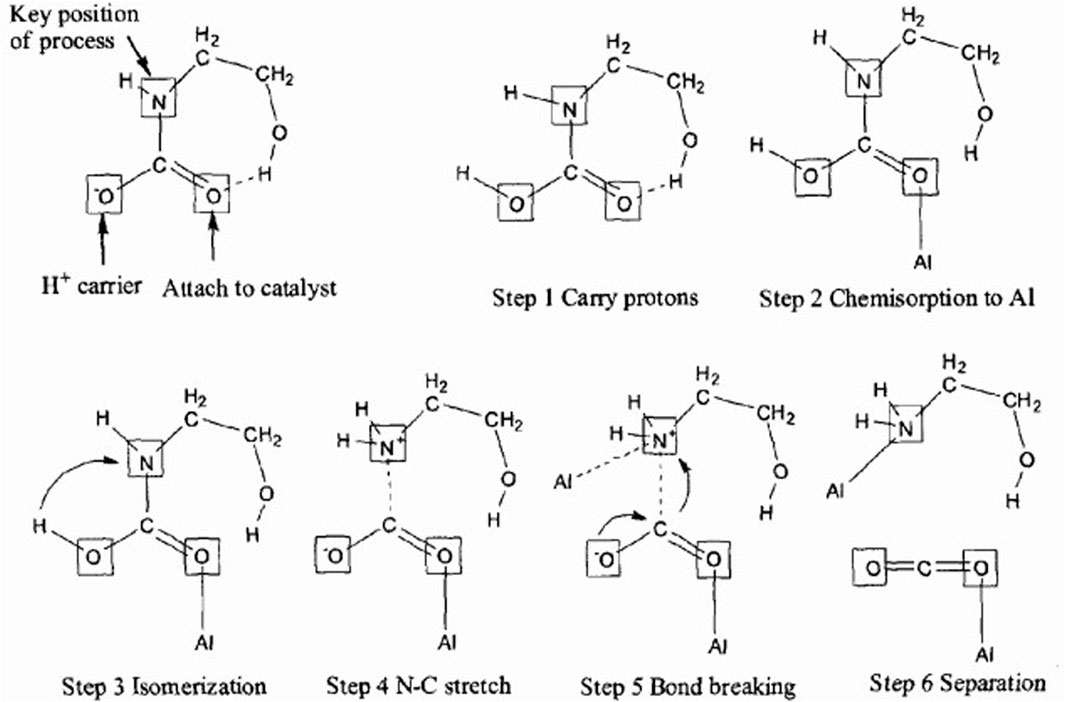
FIGURE 5. A possible catalytic mechanism of Brønsted acid catalyst for CO2 desorption (Idem et al., 2011)
Zeolite is a typical Brønsted acid catalyst, which is an aluminosilicates with a three-dimensional crystalline structure composed primarily of silicon, aluminum, and oxygen atoms (Wei et al., 2015; Javad Kalbasi et al., 2018); commonly used zeolite molecular sieves are square sodium zeolite (A-type molecular sieves), octahedral zeolite (X-type, Y-type molecular sieves), mercerized zeolite (M-type molecular sieves), high-silica zeolite (ZSM-5, ZSM-11, etc.), among many zeolite molecular sieves, HZSM-5 is the most thoroughly researched molecular sieve catalyst optimized for CO2 desorption due to its unique crystalline structure, strong dispersive force and electrostatic force, large specific surface area, uniform microporous pore size distribution, good thermal and hydrothermal stability, and a wide range of adjustable SiO2/A12O3. Wang et al. (Wang et al., 2023) compared the catalytic effects of three different Si/Al ratios of HZSM-5 zeolites (HZSM-5-25, HZSM-5-50, HZSM-5-80) on CO2 desorption through intermittent and continuous CO2 desorption experiments. The results showed that all three Si/Al ratios of HZSM-5 catalysts had specific catalytic effects, and the catalytic effect increased as the Si/Al ratio decreased, i.e., HZSM-5-25 > HZSM-5-50 > HZSM-5-80 > Blank. The reason was the lower the Si/Al ratio of the HZSM-5 catalyst, the stronger the acidity on its particle surface. The total acidities of the three catalysts were 2.34, 1.4, and 0.6 mmol/g, respectively. Therefore, it was believed the more substantial the acidity on the particle surface of HZSM-5, the more pronounced the promotion effect on desorption, which ranged from 9.41% to 15.75%. Bhatti et al. (Bhatti et al., 2020) synthesized a series of mesoporous HZSM-5 catalysts by removing silicon in an alkaline medium and improving the mesoporous and surface acidity of HZSM-5. The results showed that all catalysts improved the CO2 desorption rate of MEA at lower temperatures, and the catalytic performance trend was HZ-0.7 > HZ-0.5 > HZSM-5 > HZ-0.2 > Blank. The surface acidity of the catalysts after silicon removal and recombination showed an overall trend of HZSM-5 < HZ-0.2 < HZ-0.5 < HZ-0.7. HZ-0.5 and HZ-0.7 significantly improved the CO2 desorption rate (up to 350%–580% at 82 °C). At the same time, HZ-0.2 had lower performance due to the limited number of Brønsted acid sites, resulting in a performance gap of about 20% compared to HZSM-5.
Lewis acid catalysts
Lewis acid, also known as an electrophile reagent, γ-Al2O3 is a typical Lewis acid. The Al atoms in γ-Al2O3 have empty 3p orbitals that can accept the lone pair of electrons from N atoms, which is present in the amino ester salt. Therefore, γ-Al2O3 can attack the N atom in the amino ester and weaken the strength of the C-N bond, leading to the bond break and release CO2 (Liang et al., 2016). It provides a significant reduction in the thermal load for solvent regeneration. In addition, as shown in Figure 6, the hydroxyl group in γ-Al2O3 can react with CO2 in low CO2 load solutions to form HCO3−.

FIGURE 6. Catalytic desorption mechanism of γ-Al2O3(Liang et al., 2016)
On the one hand, HCO3− can act as a proton acceptor, accepting protons from MEAH+ to H2CO3, which can be directly thermally decomposed to form H2O and CO2, (
(Idem et al., 2011) performed CO2 desorption experiments by adding γ-Al2O3 to the MEA solution, and they successfully achieved a reduction in the regeneration temperature of the CO2-loaded MEA solution from 393K-413 K to 363K–368K, resulting in a 27% decrease in the energy required for regeneration; (Xu et al., 2020) introduced γ-Al2O3 into the MEA solution and investigated the regeneration of the solution at 70 °C. The results showed that adding a catalyst could reduce the regeneration energy and accelerate the desorption of CO2 at low regeneration temperature conditions. Under this experimental condition, γ-Al2O3 can reduce the relative heat load by 8.1% compared to the blank case. (Akachuku et al., 2019) catalyzed the regeneration of MEA solution by adding a solid acid catalyst γ-Al2O3 to the absorption desorption device. The results showed that γ-Al2O3 could effectively improve the CO2 conversion rate and reduce the energy demand related to CO2 desorption at a temperature below 373 K. The desorption rate of MEA was increased by 55.8% compared with the non-catalytic process when the solution temperature was 365 K.
Metal oxide catalysts
Metal oxides or metal oxide nanoparticles have been widely used as a viable solid acid catalyst on a commercial scale. The catalytic behavior of metal oxides is attributed to their active acidic sites available through surface defects (Alivand et al., 2020), The interaction between metal oxides and water forms Brønsted acid sites by converting the oxide groups on the surface into hydroxyl groups, while Lewis acid sites are generated above the coordinatively unsaturated metal ions (Bhatti et al., 2018). Lewis acid sites and Brønsted acid sites are emerged on the surface of metal oxide, which provide the metal atoms (Lewis acid) and H+ (Brønsted acid) to the N atoms of carbamate to rob it of its lone pair of electrons. In this way, the configuration of N atoms will be changed from sp2 to sp3, and the N–C bond strength would also be weakened by stretching. Consequently, the carbamate would be broken up by using less thermal energy, which will lead to faster CO2 stripping at lower temperatures (Liang et al., 2016). The proposed catalytic mechanism for CO2 desorption of MEA sorbent with metal oxide catalysts is shown in Figure 7. Metal nanoparticles will also benefit from physical enhancement mechanisms in addition to the chemical catalytic mechanisms described above. The physical enhancement mechanism is focused around Brownian motion of nanoparticles in the solution that break down gas bubbles, increase mass transfer surface area, and decrease mass transfer resistance at gas−liquid interfaces. This results in a large increase in the CO2 desorption rate.
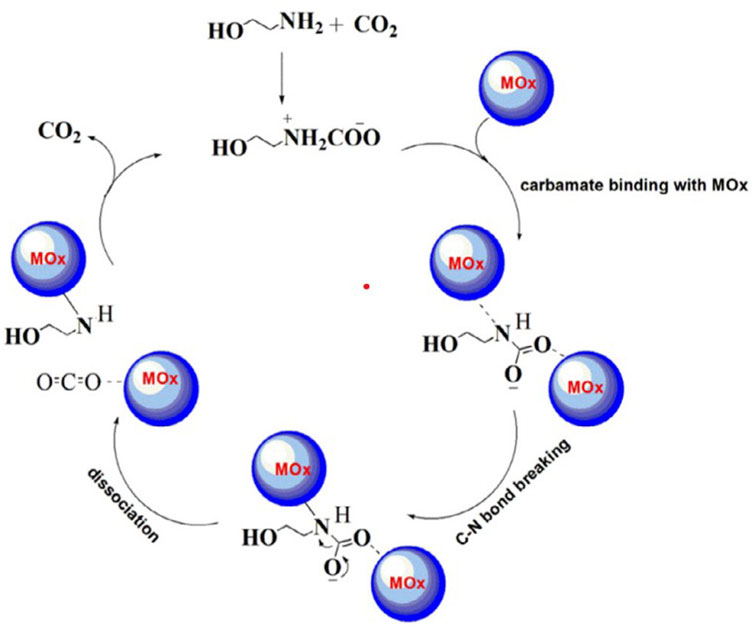
FIGURE 7. Proposed catalytic mechanism for CO2 desorption of MEA sorbent with metal oxide catalysts (Bhatti et al., 2018)
(Bhatti et al., 2017) investigated the catalytic MEA solution regeneration performance of five transition metal nanoparticles oxides, including V2O5, MoO3, WO3, TiO2, and Cr2O3. They researched the impact of these catalysts on amine regeneration within a temperature range of 308K–359 K. The results indicated that all the catalyst species could promote the regeneration of MEA. The trend in amine regeneration performance was found to be MoO3 > V2O5 > Cr2O3 > TiO2 > WO3. Catalysts with both types of acid sites (MoO3 and V2O5) desorbed 94% and 84% more CO2 than the control experiment, respectively. On the other hand, catalysts with only Lewis acid sites (Cr2O3, TiO2, WO3) desorbed nearly 44% more CO2 than the control experiment; And they also compared the regeneration of MEA solution catalyzed by five metal oxide catalysts, Ag2O, Nb2O5, NiO, CuO and MnO2, and the results showed that all five catalysts improved the regeneration of MEA, and with Ag2O having the best regeneration performance (Bhatti et al., 2018); Subsequently, in the Ag2O-catalyzed MEA solution regeneration step, they found that the addition of Ag2CO3 catalyst to the saturated MEA solution at 80 °C effectively increased the CO2 desorption rate by about 1000% (Bhatti et al., 2019). Xing et al. (Xing et al., 2021) compared five different transition metals (Co2+, Mn2+, Fe3+, Cu2+, and Ni2+). The results revealed that adding catalysts at 361 K led to increased CO2 desorption ranging from 5% to 48%, as depicted in Figure 8.
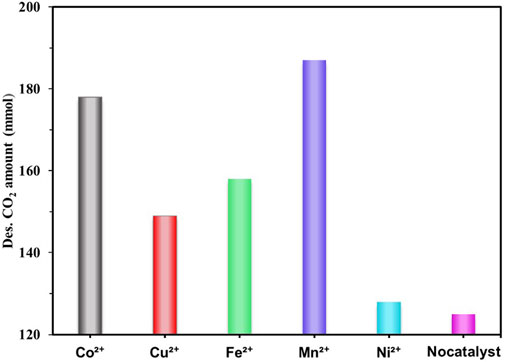
FIGURE 8. Amount of desorbed CO2 at 60 min and 361 K with different metals at the concentration of 0.05 mol/L (Xing et al., 2021)
Acid-base bifurcation catalysts
Among various catalysts, including zeolites, solid superacid, and metal oxide catalysts, HZSM-5 (a Brønsted acid catalyst) and γ-Al2O3 (a Lewis acid catalyst) have demonstrated superior catalytic performance. Some studies have reported that HZSM-5 exhibits better CO2 desorption catalytic performance than γ-Al2O3 in the high CO2 loading region of MEA solution due to its significant Brønsted acid sites, which plays a crucial role in the decomposition of amino formate. However, when the CO2 loading is low, γ-Al2O3 exhibits better catalytic CO2 desorption performance than HZSM-5, which may be attributed to the presence of prominent primary sites. Basic sites have a positive effect on the deprotonation reaction of protonated amine. Brønsted acid sites and basic sites enhance the regeneration process by different mechanisms. Therefore, combining Brønsted acid sites and primary sites to obtain a bifunctional catalyst for the MEA regeneration process in rich CO2 gives an excellent opportunity to improve catalytic performance and further reduce energy requirements (Zhang et al., 2018b). The possible catalytic mechanism is shown in Figure 9.
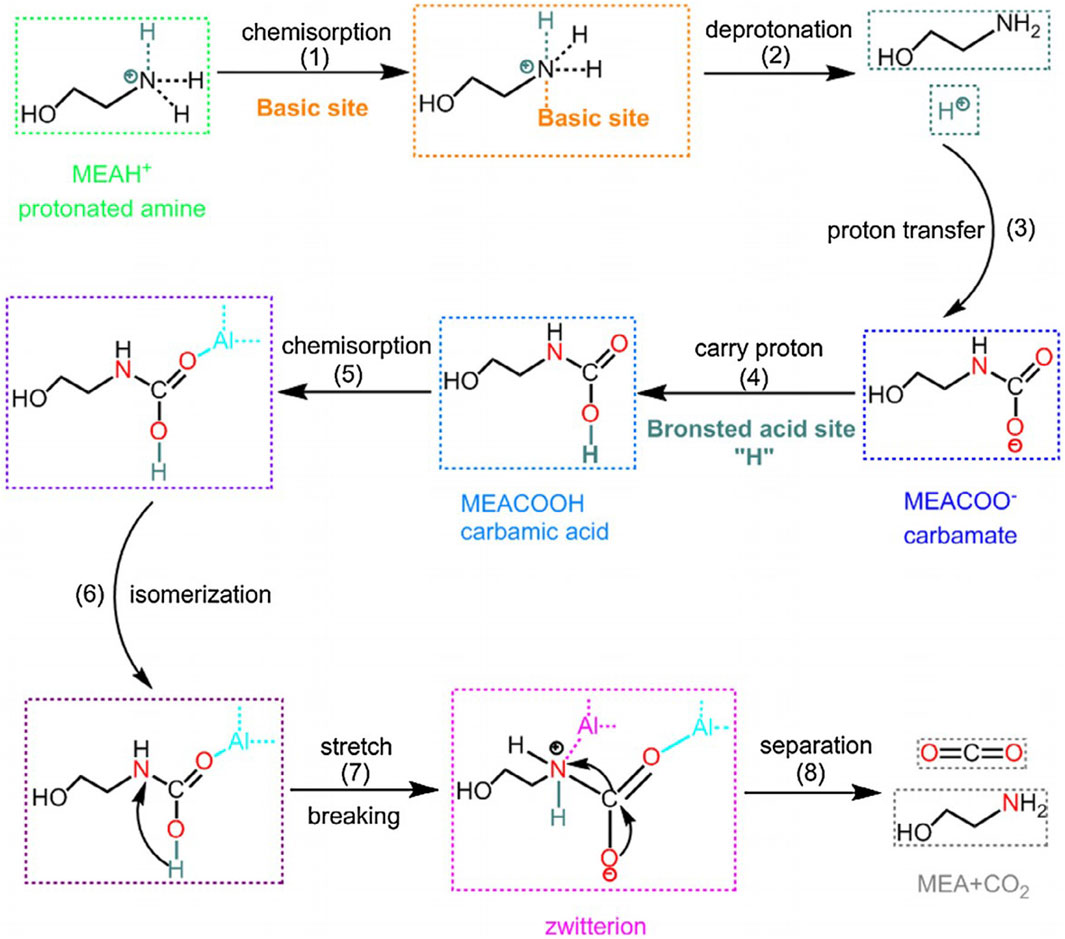
FIGURE 9. A proposed catalytic mechanism for CO2 desorption process with bifunctional catalyst (Zhang et al., 2018b).
(Zhang et al., 2018b) prepared a series of bifunctional Al2O3/HZSM-5 catalysts (Al-ZSM) by a combined precipitation-ultrasound method. Four different weight ratios of Al2O3 and HZSM-5 bifunctional catalysts (including Al-ZSM1/2, Al-ZSM1/1, Al-ZSM2/1, and Al-ZSM3/1) were studied, and they tested the catalytic performance, as shown in Figure 10. The Al-ZSM catalysts exhibited higher catalytic performance than the single Al2O3 and HZSM-5 catalysts during the MEA regeneration. Moreover, it can be seen from Figure 11 that the catalyst exhibited optimal performance when the Al2O3/HZSM-5 weight ratio was 2, and compared to the blank run, the regeneration heat load decreased by 34.2%, and the desorption coefficient increased threefold. The main reason for this improvement is that after introducing metal oxides into the zeolite, all Al-ZSM catalysts have increased Brønsted acidity, mesoporous surface area, and primary sites, which are beneficial for the CO2 desorption process. (Zhang et al., 2020) reported a novel acid-base bifunctional catalyst system MCM-41, a mesoporous molecular sieve with a large surface area and low Brønsted acid sites. By modifying MCM-41 with three different metals (Fe, Al, Mo), the surface Brønsted and Lewis acidity sites and Lewis primary sites of the catalyst can be effectively increased. The results showed that all three catalysts could accelerate the MEA regeneration process, and the MCM-41 catalyst modified with Fe2O3 (MFe) exhibited catalytic performance better. Adding MFe improved the CO2 desorption performance significantly compared to the blank run, reaching 206.3%–337.1%. A possible catalytic mechanism for this catalyst was also analyzed and is shown in Figure 12. (Lai et al., 2018) reported a novel CO2 desorption catalyst TiO(OH)2, which could dramatically increase the CO2 desorption rate of a saturated solution of 5 M MEA by 4500% at a low temperature of 361 K . TiO(OH)2 exhibited superior catalyst performance compared to conventional catalysts such as HZSM-5, notably promoting the CO2 desorption rate of the MEA solution at low temperatures. Additionally, the authors proposed a possible catalytic mechanism for the MEA solution, illustrated in Figure 13. Based on the findings of their study, TiO(OH)2 has promising potential as a catalyst for CO2 desorption in MEA systems.
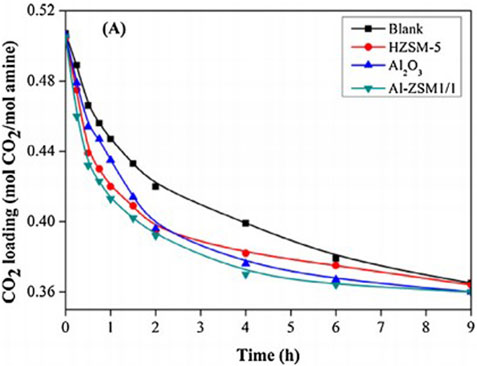
FIGURE 10. Catalytic CO2 desorption performance in MEA solution at 96 °C Catalytic performance of various bifunctional catalysts (Zhang et al., 2018b).
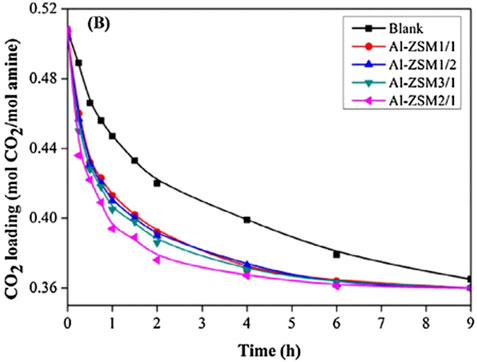
FIGURE 11. Catalytic CO2 desorption performance in MEA solution at 96 °C Comparison of catalytic performance the single catalysts with the worst bifunctional catalyst (Zhang et al., 2018b).
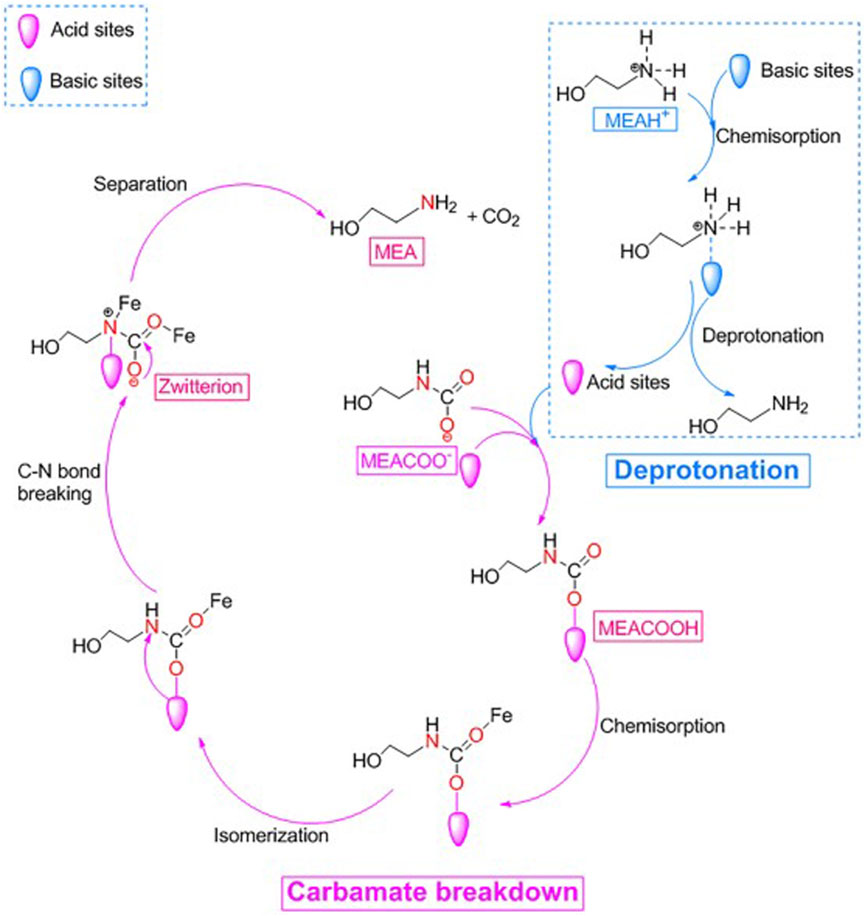
FIGURE 12. A possible catalytic CO2 desorption mechanism in MEA solution over MFe catalyst (Zhang et al., 2020)
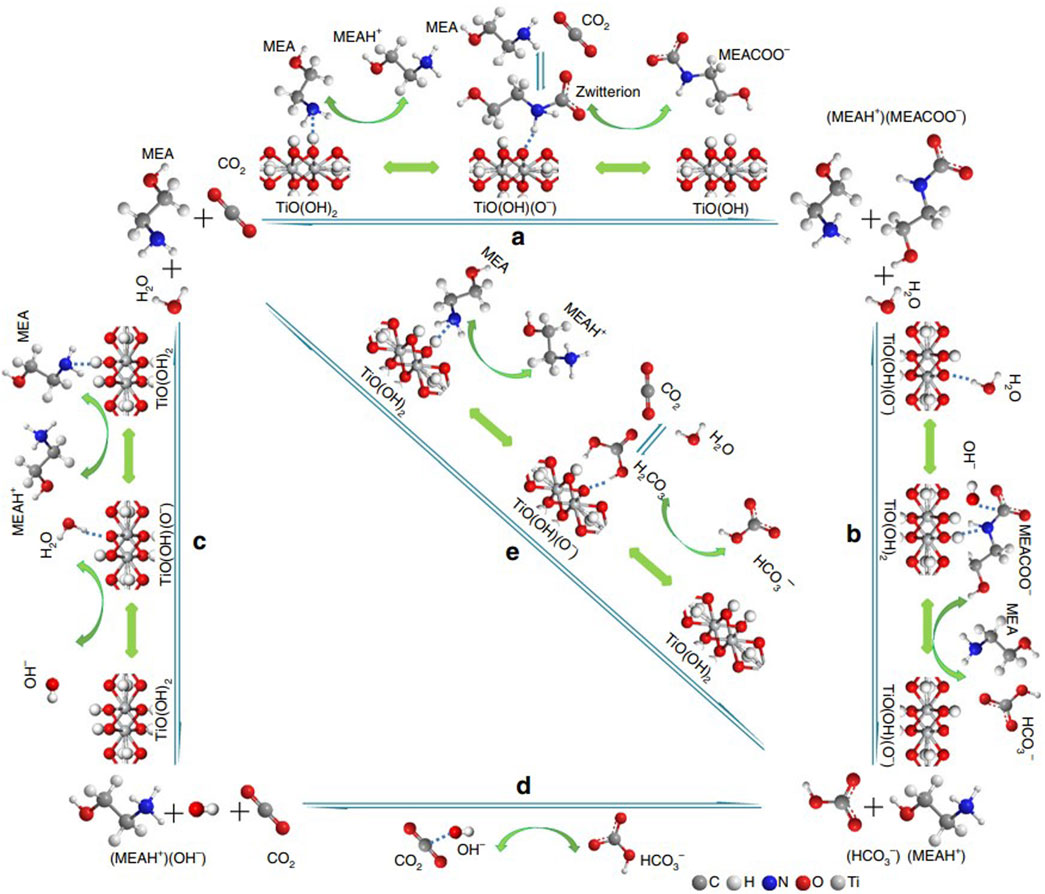
FIGURE 13. A possible catalytic CO2 desorption mechanism in MEA solution over TiO(OH)2 catalyst (Lai et al., 2018)
As mentioned above, the excellent catalytic effect of acid-base bifunctional catalysts is mainly attributed to their large mesopore area and the possession of some acidic and basic active sites at the same time, and the improvement of the mesopore area and the number of acid-base sites is often realized during the preparation of the catalysts. For example, the prepared Al-ZSM bifunctional catalyst realized the conversion of part of the microporous structure into a mesoporous structure while retaining the prominent acidic sites possessed by HZSM-5 and also increased, to a certain extent, the basic sites on the surface of HZSM-5; for the preparation of MFe catalysts, firstly, MCM-41, which is a kind of MFe catalyst with an ordered mesoporous structure and a large specific surface area. However, it is limited by the smaller ratio of Brønsted acid sites to Lewis acid sites and the number of Brønsted acid sites, which leads to the relatively low catalytic CO2 desorption performance of MCM-41, the partial replacement of silicon ions in MCM-41 by Fe3+ and the formation of Fe-O-Si bonds by oxygen ions linking to the framework. Typically, the Fe3+ centers formed upon reaction with water can be considered as Lewis acid sites for accepting electrons, and the resulting hydroxyl groups are regarded as Brønsted acid sites for releasing protons.
Summary and outlook
The chemical absorption method based on MEA solution that is widely used in industrial production for CO2 removal, carbon capture system operation high energy consumption is considered the most crucial reason hindering its development. However, many researchers have made many efforts in process optimization, but the ideal desorption energy consumption is still a certain distance; this is mainly because the MEA solution regeneration process temperature is very high, resulting in large part of the energy being used for the vaporization of water in solution. The Brønsted acid site and Lewis acid site contained in a solid acid catalyst can effectively change the CO2 desorption reaction pathway so that the reaction can proceed at a lower temperature, thereby significantly reducing the energy consumption of amine solution regeneration; this paper mainly introduces the promotion mechanism and promotion effect of several different solid acid catalysts (including Brønsted acid catalyst, Lewis acid catalyst, metal oxide catalyst, and acid-base bifunctional catalyst) in the process of CO2 desorption. It is not difficult to find that the introduction of a solid acid catalyst can control the regeneration temperature of MEA solution below 373K, which can effectively reduce the heat load of 10%–50% in the process of CO2 desorption, and the desorption amount of CO2 can be increased by up to 4500%.
Different techniques were used to characterize the physicochemical properties of the catalyst surface, and the results showed that the catalytic performance of the catalysts is usually influenced by the total number of acidic sites on the surface; the ratio of Brønsted to Lewis acid sites; the amount of Brønsted acid sites and the specific surface area, especially the mesoporous surface area. Therefore, it is important to develop new and efficient solid acid catalysts with a high number of acid sites, a large ratio of Brønsted acid sites to Lewis acid sites, a high amount of Brønsted acid sites, and a large mesoporous surface area or to improve the catalytic performance of existing solid acid catalysts by improving the above physicochemical properties.
At this stage, most studies on catalyst performance are based on intermittent laboratory reactors. Although these experimental studies show that the addition of catalysts effectively reduces the energy consumption of the amine solution regeneration process, the industrial carbon capture process is often a continuous absorption and desorption process. Firstly, the CO2 desorption promotion effect in this continuous process is limited to some extent by the contact time between the solution and the catalyst; secondly, the amount of catalyst and the size of the regeneration equipment need to be considered because of the large amount of solution circulation in the industrial carbon capture process; finally, the decay of the catalytic effect of the catalyst in this continuous use process, i.e., the lifetime of the catalyst, is also a key consideration.
Industrial regeneration towers usually enhance CO2 desorption by solid packing. Some studies have shown that acidic catalytic packing can lower the regeneration temperature and thus reduce the sensible heat and latent heat of vaporization in the regeneration process. However, there are few studies in this area and no clear results on the catalytic effect of acidic catalytic packing in regeneration towers compared to the catalyst immersion reaction process in the laboratory stage.
Finally, we compared the advantages and disadvantages of different catalysts in terms of cost, stability and environmental impact of catalyst desorption of CO2. We found that the preparation process of catalysts with better performance (including catalytic effect and stability) is often more complicated, which leads to the environmental impact caused by the consumption of large quantities of chemicals in the preparation process, as well as higher production costs, so how to ensure the performance of the catalysts on the premise of achieving low-pollution and low-cost preparation of catalysts is also a primary research direction in the future.
Author contributions
ZY: Formal analysis, Funding acquisition. YS: Accessing information, Writing–Original draft and Editing. HaY: Supervision, Resources, Review. HuY: Data curation, Review. HG: Polishing. XZ: Proof.
Funding
The author(s) declare financial support was received for the research, authorship, and/or publication of this article. This paper is funded by the entrusted projects for enterprises and institutions (H20210252).
Conflict of interest
HuY was employed by Shenzhen Huawei Offshore Shipping Transport Co., Ltd.
The remaining authors declare that the research was conducted in the absence of any commercial or financial relationships that could be construed as a potential conflict of interest.
Publisher’s note
All claims expressed in this article are solely those of the authors and do not necessarily represent those of their affiliated organizations, or those of the publisher, the editors and the reviewers. Any product that may be evaluated in this article, or claim that may be made by its manufacturer, is not guaranteed or endorsed by the publisher.
References
Afari, D. B., Coker, J., Narku-Tetteh, J., and Idem, R. (2018). Comparative kinetic studies of solid absorber catalyst (K/MgO) and solid desorber catalyst (HZSM-5)-Aided CO2 absorption and desorption from aqueous solutions of MEA and blended solutions of BEA-AMP and MEA-MDEA. Industrial Eng. Chem. Res. 57 (46), 15824–15839. doi:10.1021/acs.iecr.8b02931
Agarwal, A., Biegler, L. T., and Zitney, S. E. (2010). A superstructure-based optimal synthesis of PSA cycles for post-combustion CO2 capture. Aiche J. 56 (7), 1813–1828. doi:10.1002/aic.12107
Akachuku, A., Osei, P. A., Decardi-Nelson, B., Srisang, W., Pouryousefi, F., Ibrahim, H., et al. (2019). Experimental and kinetic study of the catalytic desorption of CO2 from CO2-loaded monoethanolamine (MEA) and blended monoethanolamine – methyl-diethanolamine (MEA-MDEA) solutions. Energy 179, 475–489. doi:10.1016/j.energy.2019.04.174
Alivand, M. S., Mazaheri, O., Wu, Y., Stevens, G. W., Scholes, C. A., and Mumford, K. A. (2020). Catalytic solvent regeneration for energy-efficient CO2 capture. ACS Sustain. Chem. Eng. 8 (51), 18755–18788. doi:10.1021/acssuschemeng.0c07066
AR5 Synthesis Report: Climate Change (2014). “Summary for policymakers,” in Climate change 2013 – the physical science basis: Working group I contribution to the fifth assessment report of the intergovernmental panel on climate change, ed. C. Intergovernmental panel on climate. (Cambridge: Cambridge University Press), 1–30.
Barzagli, F., Giorgi, C., Mani, F., and Peruzzini, M. (2018). Reversible carbon dioxide capture by aqueous and non-aqueous amine-based absorbents: A comparative analysis carried out by 13C nmr spectroscopy. Appl. Energy 220, 208–219. doi:10.1016/j.apenergy.2018.03.076
Barzagli, F., and Mani, F. (2021). Direct CO2 air capture with aqueous 2-(ethylamino)ethanol and 2-(2-aminoethoxy)ethanol: 13C NMR speciation of the absorbed solutions and study of the sorbent regeneration improved by a transition metal oxide catalyst. Inorganica Chim. Acta 518, 120256. doi:10.1016/j.ica.2021.120256
Benhelal, E., Shamsaei, E., and Rashid, M. I. (2021). Challenges against CO(2) abatement strategies in cement industry: A review. J. Environ. Sci. (China) 104, 84–101. doi:10.1016/j.jes.2020.11.020
Bhatti, U. H., Shah, A. K., Hussain, A., Khan, H. A., Park, C. Y., Nam, S. C., et al. (2020). Catalytic activity of facilely synthesized mesoporous HZSM-5 catalysts for optimizing the CO2 desorption rate from CO2-rich amine solutions. Chem. Eng. J. 389, 123439. doi:10.1016/j.cej.2019.123439
Bhatti, U. H., Shah, A. K., Kim, J. N., You, J. K., Choi, S. H., Lim, D. H., et al. (2017). Effects of transition metal oxide catalysts on MEA solvent regeneration for the post-combustion carbon capture process. ACS Sustain. Chem. Eng. 5 (7), 5862–5868. doi:10.1021/acssuschemeng.7b00604
Bhatti, U. H., Sivanesan, D., Lim, D. H., Nam, S. C., Park, S., and Baek, I. H. (2018). Metal oxide catalyst-aided solvent regeneration: A promising method to economize post-combustion CO2 capture process. J. Taiwan Inst. Chem. Eng. 93, 150–157. doi:10.1016/j.jtice.2018.05.029
Bhatti, U. H., Sivanesan, D., Nam, S., Park, S. Y., and Baek, I. H. (2019). Efficient Ag2O–Ag2CO3 catalytic cycle and its role in minimizing the energy requirement of amine solvent regeneration for CO2 capture. ACS Sustain. Chem. Eng. 7 (12), 10234–10240. doi:10.1021/acssuschemeng.9b01709
Bhown, A. S., and Freeman, B. C. (2011). Analysis and status of post-combustion carbon dioxide capture technologies. Environ. Sci. Technol. 45 (20), 8624–8632. doi:10.1021/es104291d
Bui, M., Adjiman, C. S., Bardow, A., Anthony, E. J., Boston, A., Brown, S., et al. (2018). Carbon capture and storage (CCS): the way forward. Energy and Environ. Sci. 11 (5), 1062–1176. doi:10.1039/c7ee02342a
Caplow, M. J. J. o. t. A. C. S. (1968). Kinetics of carbamate formation and breakdown. Kinet. carbamate Form. breakdown 90, 6795–6803. doi:10.1021/ja01026a041
Carson, J. K., Marsh, K. N., and Mather, A. E. (2000). Enthalpy of solution of carbon dioxide in (water + monoethanolamine, or diethanolamine, orN-methyldiethanolamine) and (water + monoethanolamine +N-methyldiethanolamine) atT= 298.15 K. J. Chem. Thermodyn. 32 (9), 1285–1296. doi:10.1006/jcht.2000.0680
Chen, C., Huang, H., Yu, Y., Shi, J., He, C., Albilali, R., et al. (2018). Template-free synthesis of hierarchical porous carbon with controlled morphology for CO2 efficient capture. Chem. Eng. J. 353, 584–594. doi:10.1016/j.cej.2018.07.161
Chuenphan, T., Yurata, T., Sema, T., and Chalermsinsuwan, B. (2022). Techno-economic sensitivity analysis for optimization of carbon dioxide capture process by potassium carbonate solution. Energy 254, 124290. doi:10.1016/j.energy.2022.124290
Davy, R. (2009). Development of catalysts for fast, energy efficient post combustion capture of CO2 into water; an alternative to monoethanolamine (MEA) solvents. Energy Procedia 1 (1), 885–892. doi:10.1016/j.egypro.2009.01.118
Decardi-Nelson, B., Akachuku, A., Osei, P., Srisang, W., Pouryousefi, F., and Idem, R. (2017). A flexible and robust model for low temperature catalytic desorption of CO2 from CO2-loaded amines over solid acid catalysts. Chem. Eng. Sci. 170, 518–529. doi:10.1016/j.ces.2016.12.068
Doney, S. C., Fabry, V. J., Feely, R. A., and Kleypas, J. A. (2009). Ocean acidification: the other CO2Problem. Ocean. Acidif. 1 (1), 169–192. doi:10.1146/annurev.marine.010908.163834
Dou, B., Song, Y., Liu, Y., and Feng, C. (2010). High temperature CO2 capture using calcium oxide sorbent in a fixed-bed reactor. J. Hazard. Mater. 183 (1), 759–765. doi:10.1016/j.jhazmat.2010.07.091
Du, M., Feng, B., An, H., Liu, W., and Zhang, L. (2011). Effect of addition of weak acids on CO2 desorption from rich amine solvents. Korean J. Chem. Eng. 29, 362–368. doi:10.1007/s11814-011-0184-4
Feng, B., Du, M., Dennis, T. J., Anthony, K., and Perumal, M. J. (2010). Reduction of energy requirement of CO2 desorption by adding acid into CO2-loaded solvent. Energy and Fuels 24 (1), 213–219. doi:10.1021/ef900564x
Finotello, A., Bara, J. E., Camper, D., and Noble, R. D. (2008). Room-temperature ionic liquids: temperature dependence of gas solubility selectivity. Industrial Eng. Chem. Res. 47 (10), 3453–3459. doi:10.1021/ie0704142
Foster, G. L., and Rohling, E. J. (2013). Relationship between sea level and climate forcing by CO 2 on geological timescales Proc. Natl. Acad. Sci. U. S. A. 110 (4), 1209–1214. doi:10.1073/pnas.1216073110
Freund, P. (2003). Making deep reductions in CO2 emissions from coal-fired power plant using capture and storage of CO2. Proc. Institution Mech. Eng. Part A J. Power Energy 217 (1), 1–7. doi:10.1243/095765003321148628
Fu, L., Ren, Z., Si, W., Ma, Q., Huang, W., Liao, K., et al. (2022). Research progress on CO2 capture and utilization technology. J. CO2 Util. 66, 102260. doi:10.1016/j.jcou.2022.102260
Gao, H., Huang, Y., Zhang, X., Bairq, Z. A. S., Huang, Y., Tontiwachwuthikul, P., et al. (2020). Catalytic performance and mechanism of SO42−/ZrO2/SBA-15 catalyst for CO2 desorption in CO2-loaded monoethanolamine solution. Appl. Energy 259, 114179. doi:10.1016/j.apenergy.2019.114179
Godin, J., Liu, W., Ren, S., and Xu, C. C. (2021). Advances in recovery and utilization of carbon dioxide: A brief review. J. Environ. Chem. Eng. 9 (4), 105644. doi:10.1016/j.jece.2021.105644
Goff, G. S., and Rochelle, G. T. (2004). Monoethanolamine degradation: O2 mass transfer effects under CO2 capture conditions. Industrial Eng. Chem. Res. 43 (20), 6400–6408. doi:10.1021/ie0400245
Haszeldine, R. S. (2009). Carbon capture and storage: how green can black Be? Science 325 (5948), 1647–1652. doi:10.1126/science.1172246
Herzog, H., Drake, E., and Adams, E. (2012). CO capture, reuse, and storage technologies 2 for mitigating global climate change.
Huang, Y., Zhang, X., Luo, X., Gao, H., Bairq, Z. A. S., Tontiwachwuthikul, P., et al. (2021). Catalytic performance and mechanism of meso–microporous material β-SBA-15-supported FeZr catalysts for CO2 desorption in CO2-loaded aqueous amine solution. Industrial Eng. Chem. Res. 60 (6), 2698–2709. doi:10.1021/acs.iecr.0c03767
Idem, R., Shi, H., Gelowitz, D., and Tontiwachwuthikul, P. (2011). Catalytic method and apparatus for separating a gaseous component from an incoming gas stream". US).
Javad Kalbasi, R., Mansouri, S., and Mazaheri, O. (2018). In situ polymerization of poly(vinylimidazole) into the pores of hierarchical MFI zeolite as an acid–base bifunctional catalyst for one-pot C–C bond cascade reactions. Res. Chem. Intermed. 44 (5), 3279–3291. doi:10.1007/s11164-018-3306-3
Ji, L., Yu, H., Yu, B., Jiang, K., Grigore, M., Wang, X., et al. (2018). Integrated absorption–mineralisation for energy-efficient CO2 sequestration: reaction mechanism and feasibility of using fly ash as a feedstock. Chem. Eng. J. 352, 151–162. doi:10.1016/j.cej.2018.07.014
Kerr, R. A. (2006). Climate change. Yes, it's been getting warmer in here since the CO2 began to rise. Science, 312(5782), 1854–1854. doi:10.1126/science.312.5782.1854
Lai, Q., Toan, S., Assiri, M. A., Cheng, H., Russell, A. G., Adidharma, H., et al. (2018). Catalyst-TiO(OH)2 could drastically reduce the energy consumption of CO2 capture. Nat. Commun. 9 (1), 2672. doi:10.1038/s41467-018-05145-0
Le Moullec, Y., Neveux, T., Azki, A. A., Chikukwa, A., and Hoff, K. A. (2014). Process modifications for solvent-based post combustion CO2 capture. Energy Procedia 63, 1470–1477. doi:10.1016/j.egypro.2014.11.156
Lepaumier, H., Picq, D., and Carrette, P. L. (2009). New amines for CO2 capture. I. Mechanisms of amine degradation in the presence of CO2. Industrial Eng. Chem. Res. - IND ENG CHEM RES 48, 9061–9067. doi:10.1021/ie900472x
Lepaumier, H., Silva, E., Einbu, A., Grimstvedt, A., Knudsen, J., Zahlsen, K., et al. (2011). Comparison of MEA degradation in pilot-scale with lab-scale experiments. Energy Procedia 4, 1652–1659. doi:10.1016/j.egypro.2011.02.037
Li, K., Leigh, W., Feron, P., Yu, H., and Tade, M. (2016). Systematic study of aqueous monoethanolamine (MEA)-based CO2 capture process: techno-economic assessment of the MEA process and its improvements. Appl. Energy 165, 648–659. doi:10.1016/j.apenergy.2015.12.109
Li, K., Yu, H., Feron, P., Tade, M., and Wardhaugh, L. (2015). Technical and energy performance of an advanced, aqueous ammonia-based CO2 capture technology for a 500 MW coal-fired power station. Environ. Sci. Technol. 49 (16), 10243–10252. doi:10.1021/acs.est.5b02258
Li, T. C., Yang, C. N., Tantikhajorngosol, P., Sema, T., and Tontiwachwuthikul, P. (2022). Experimental investigations of CO2 absorption and catalyst-aided CO2 desorption performance of several different amines blending with a promoter. Chem. Eng. Sci. 264, 118177. doi:10.1016/j.ces.2022.118177
Li, T., Yu, Q., Barzagli, F., Li, C. e., Che, M., Zhang, Z., et al. (2023). Energy efficient catalytic CO2 desorption: Mechanism, Technological progress and Perspective.
Liang, Z., Idem, R., Tontiwachwuthikul, P., Yu, F., Liu, H., and Rongwong, W. (2016). Experimental study on the solvent regeneration of a CO2-loaded MEA solution using single and hybrid solid acid catalysts. AIChE J. 62 (3), 753–765. doi:10.1002/aic.15073
Liang, Z. W., Rongwong, W., Liu, H. L., Fu, K. Y., Gao, H. X., Cao, F., et al. (2015). Recent progress and new developments in post-combustion carbon-capture technology with amine based solvents. Int. J. Greenh. Gas Control 40, 26–54. doi:10.1016/j.ijggc.2015.06.017
Lin, Y.-J., and Rochelle, G. T. (2016). Approaching a reversible stripping process for CO2 capture. Chem. Eng. J. 283, 1033–1043. doi:10.1016/j.cej.2015.08.086
Liu, G., Yuan, H., Li, X., Li, K., Mao, L., and Zhang, G. (2022). Tailoring the properties of self-assembled carbonic anhydrase supraparticles for CO2 capture. ACS Sustain. Chem. Eng. 10 (37), 12374–12385. doi:10.1021/acssuschemeng.2c03740
Liu, H., Zhang, X., Gao, H., Liang, Z., Idem, R. O., Tontiwachwuthikul, P. J. I., et al. (2017). Investigation of CO2 regeneration in single and blended amine solvents with and without catalyst. Ind. Eng. Chem. Res. 56, 7656–7664. doi:10.1021/acs.iecr.7b00778
Luis Míguez, J., Porteiro, J., Pérez-Orozco, R., Patiño, D., and Rodríguez, S. (2018). Evolution of CO2 capture technology between 2007 and 2017 through the study of patent activity. Appl. Energy 211, 1282–1296. doi:10.1016/j.apenergy.2017.11.107
McGurk, S. J., Martín, C. F., Brandani, S., Sweatman, M. B., and Fan, X. (2017). Microwave swing regeneration of aqueous monoethanolamine for post-combustion CO2 capture. Appl. Energy 192, 126–133. doi:10.1016/j.apenergy.2017.02.012
Mercure, J. F., Pollitt, H., Viñuales, J. E., Edwards, N. R., Holden, P. B., Chewpreecha, U., et al. (2018). Macroeconomic impact of stranded fossil fuel assets. Nat. Clim. Change 8 (7), 588–593. doi:10.1038/s41558-018-0182-1
Montzka, S. A., Dlugokencky, E. J., and Butler, J. H. (2011). Non-CO2 greenhouse gases and climate change. Nature 476 (7358), 43–50. doi:10.1038/nature10322
Muchan, P., Saiwan, C., Narku-Tetteh, J., Idem, R., Supap, T., and Tontiwachwuthikul, P. (2017). Screening tests of aqueous alkanolamine solutions based on primary, secondary, and tertiary structure for blended aqueous amine solution selection in post combustion CO2 capture. Chem. Eng. Sci. 170, 574–582. doi:10.1016/j.ces.2017.02.031
Muchan, P., Saiwan, C., and Nithitanakul, M. (2022). Carbon dioxide adsorption/desorption performance of single- and blended-amines-impregnated MCM-41 mesoporous silica in post-combustion carbon capture. Clean. Energy 6 (3), 424–437. doi:10.1093/ce/zkac020
Nakrak, S., Chalermsinsuwan, B., Tontiwachwuthikul, P., Gao, H., Liang, Z., and Sema, T. (2023). Comparative mass transfer performance of CO2 absorption using highly-concentrated AMP-PZ-MEA ternary amines solvent. Energy Rep. 9, 1–7. doi:10.1016/j.egyr.2023.05.219
Narku-Tetteh, J., Muchan, P., Saiwan, C., Supap, T., and Idem, R. (2017). Selection of components for formulation of amine blends for post combustion CO2 capture based on the side chain structure of primary, secondary and tertiary amines. Chem. Eng. Sci. 170, 542–560. doi:10.1016/j.ces.2017.02.036
Oyenekan, B. A. J. D., and Gradworks, T.-. (2007). Modeling of strippers for CO2 capture by aqueous amines.
Oyenekan, B. A., and Rochelle, G. T. (2006). Energy performance of stripper configurations for CO2 capture by aqueous amines. Industrial Eng. Chem. Res. 45 (8), 2457–2464. doi:10.1021/ie050548k
Peters, G. P., Andrew, R. M., Boden, T., Canadell, J. G., Ciais, P., Le Quéré, C., et al. (2013). The challenge to keep global warming below 2 °C. Nat. Clim. Change 3 (1), 4–6. doi:10.1038/nclimate1783
Rao, A. B., and Rubin, E. S. (2019). A technical, economic, and environmental assessment of amine-based CO2 capture technology for power plant greenhouse gas control. Environ. Sci. Technol. 20 (20), 4467–4475. doi:10.1021/es0158861
Rashid, M., Benhelal, E., Farhang, F., Oliver, T., Stockenhuber, M., and Kennedy, E. (2020). Application of a concurrent grinding technique for two-stage aqueous mineral carbonation. J. CO2 Util. 42, 101347. doi:10.1016/j.jcou.2020.101347
Rashid, M. I., Benhelal, E., Anderberg, L., Farhang, F., Oliver, T., Rayson, M. S., et al. (2022). Aqueous carbonation of peridotites for carbon utilisation: A critical review. Environ. Sci. Pollut. Res. 29 (50), 75161–75183. doi:10.1007/s11356-022-23116-3
Rhodes, C. J. (2016). The 2015 Paris climate change conference: cop21. Sci. Prog. 99 (1), 97–104. doi:10.3184/003685016x14528569315192
Rochelle, G. T. (2009). Amine scrubbing for CO2 capture. Science 325 (5948), 1652–1654. doi:10.1126/science.1176731
Romeo, L. M., Espatolero, S., and Bolea, I. (2008). Designing a supercritical steam cycle to integrate the energy requirements of CO2 amine scrubbing. Int. J. Greenh. Gas Control 2 (4), 563–570. doi:10.1016/j.ijggc.2008.03.002
Sakwattanapong, R., Aroonwilas, A., and Veawab, A. (2005). Behavior of reboiler heat duty for CO2 capture plants using regenerable single and blended alkanolamines. Industrial Eng. Chem. Res. - IND ENG CHEM RES 44, 4465–4473. doi:10.1021/ie050063w
Schreiber, A., Zapp, P., and Kuckshinrichs, W. (2009). Environmental assessment of German electricity generation from coal-fired power plants with amine-based carbon capture. Int. J. Life Cycle Assess. 14 (6), 547–559. doi:10.1007/s11367-009-0102-8
Scott, V., Gilfillan, S., Markusson, N., Chalmers, H., and Haszeldine, R. S. (2013). Last chance for carbon capture and storage. Nat. Clim. Change 3 (2), 105–111. doi:10.1038/nclimate1695
Shah, B., Shah, M., Shah, V., and Prajapati, M. (2023). An Anatomized study on the progress and prospects of CO2 utilization technology. Case Stud. Chem. Environ. Eng. 8, 100381. doi:10.1016/j.cscee.2023.100381
Shakerian, F., Kim, K.-H., Szulejko, J. E., and Park, J.-W. (2015). A comparative review between amines and ammonia as sorptive media for post-combustion CO2 capture. Appl. Energy 148, 10–22. doi:10.1016/j.apenergy.2015.03.026
Shi, H. C., Zheng, L. N., Huang, M., Zuo, Y. H., Kang, S. F., Huang, Y. D., et al. (2018). Catalytic-CO2-Desorption studies of DEA and DEA-MEA blended solutions with the aid of Lewis and bronsted acids. Industrial Eng. Chem. Res. 57 (34), 11505–11516. doi:10.1021/acs.iecr.8b00961
Shi, H., Idem, R., Naami, A., Gelowitz, D., and Tontiwachwuthikul, P. (2014a). Catalytic solvent regeneration using hot water during amine based CO2 capture process. Energy Procedia 63, 273–278. doi:10.1016/j.egypro.2014.11.029
Shi, H., Naami, A., Idem, R., and Tontiwachwuthikul, P. (2014b). Catalytic and non catalytic solvent regeneration during absorption-based CO2 capture with single and blended reactive amine solvents. Int. J. Greenh. Gas Control 26, 39–50. doi:10.1016/j.ijggc.2014.04.007
Singh, P., and Versteeg, G. F. (2008). Structure and activity relationships for CO2 regeneration from aqueous amine-based absorbents. Process Saf. Environ. Prot. 86 (5), 347–359. doi:10.1016/j.psep.2008.03.005
Srisang, W., Pouryousefi, F., Osei, P. A., Decardi-Nelson, B., Akachuku, A., Tontiwachwuthikul, P., et al. (2018). CO2 capture efficiency and heat duty of solid acid catalyst-aided CO2 desorption using blends of primary-tertiary amines. Int. J. Greenh. Gas Control 69, 52–59. doi:10.1016/j.ijggc.2017.12.010
Srisang, W., Pouryousefi, F., Osei, P. A., Decardi-Nelson, B., Akachuku, A., Tontiwachwuthikul, P., et al. (2017). Evaluation of the heat duty of catalyst-aided amine-based post combustion CO2 capture. Chem. Eng. Sci. 170, 48–57. doi:10.1016/j.ces.2017.01.049
Sun, R., Shen, J., Grasby, S. E., Zhang, J., Chen, J., Yang, C., et al. (2022). CO2 buildup drove global warming, the Marinoan deglaciation, and the genesis of the Ediacaran cap carbonates. Precambrian Res. 383, 106891. doi:10.1016/j.precamres.2022.106891
Uyanga, I. J., and Idem, R. O. (2007). Studies of SO2- and O2-induced degradation of aqueous MEA during CO2 capture from power plant flue gas streams. Industrial Eng. Chem. Res. 46 (8), 2558–2566. doi:10.1021/ie0614024
Wang, H., Tang, S., Zhong, S., and Liang, B. (2023). An investigation of the enhancing effect of solid particle surface on the CO2 desorption behavior in chemical sorption process with MEA solution[J]. CIESC J. 74 (4), 1539–1548. doi:10.11949/0438-1157.20221565
Wang, M., Joel, A. S., Ramshaw, C., Eimer, D., and Musa, N. M. (2015). Process intensification for post-combustion CO2 capture with chemical absorption: A critical review. Appl. Energy 158, 275–291. doi:10.1016/j.apenergy.2015.08.083
Wang, M., Lawal, A., Stephenson, P., Sidders, J., and Ramshaw, C. (2011). Post-combustion CO2 capture with chemical absorption: A state-of-the-art review. Chem. Eng. Res. Des. 89 (9), 1609–1624. doi:10.1016/j.cherd.2010.11.005
Wang, Y., Zhao, L., Otto, A., Robinius, M., and Stolten, D. (2017). A review of post-combustion CO2 capture technologies from coal-fired power plants. Energy Procedia 114, 650–665. doi:10.1016/j.egypro.2017.03.1209
Wei, Y., Parmentier, T. E., de Jong, K. P., and Zečević, J. (2015). Tailoring and visualizing the pore architecture of hierarchical zeolites. Chem. Soc. Rev. 44 (20), 7234–7261. doi:10.1039/C5CS00155B
Xia, C., Ye, B., Jiang, J., and Shu, Y. (2020). Prospect of near-zero-emission IGCC power plants to decarbonize coal-fired power generation in China: implications from the GreenGen project. J. Clean. Prod. 271, 122615. doi:10.1016/j.jclepro.2020.122615
Xiao, M., Liu, H., Idem, R., Tontiwachwuthikul, P., and Liang, Z. (2016). A study of structure–activity relationships of commercial tertiary amines for post-combustion CO2 capture. Appl. Energy 184, 219–229. doi:10.1016/j.apenergy.2016.10.006
Xing, L., Wei, K., Li, Y., Fang, Z., Li, Q., Qi, T., et al. (2021). TiO2 coating strategy for robust catalysis of the metal–organic framework toward energy-efficient CO2 capture. Environ. Sci. Technol. 55 (16), 11216–11224. doi:10.1021/acs.est.1c02452
Xu, Y., Jin, B., Jiang, H., Li, L., and Wei, J. (2020). Investigation of the regeneration of a CO2-loaded ammonia solution with solid acid catalysts: A promising alternative for reducing regeneration energy. Fuel Process. Technol. 205, 106452. doi:10.1016/j.fuproc.2020.106452
Yang, H., Xu, Z., Fan, M., Gupta, R., Slimane, R. B., Bland, A. E., et al. (2008). Progress in carbon dioxide separation and capture: A review. J. Environ. Sci. 20 (1), 14–27. doi:10.1016/S1001-0742(08)60002-9
Yousef, A. M., Eldrainy, Y. A., El-Maghlany, W. M., and Attia, A. (2017). Biogas upgrading process via low-temperature CO2 liquefaction and separation. J. Nat. Gas Sci. Eng. 45, 812–824. doi:10.1016/j.jngse.2017.07.001
Yu, C.-H., Huang, C.-H., and Tan, C.-S. (2012). A review of CO2 capture by absorption and adsorption. Aerosol Air Qual. Res. 12 (5), 745–769. doi:10.4209/aaqr.2012.05.0132
Zhang, X., Hong, J., Liu, H., Luo, X., Olson, W., Tontiwachwuthikul, P., et al. (2018a). SO42−/ZrO2 supported on γ-Al2O3 as a catalyst for CO2 desorption from CO2-loaded monoethanolamine solutions. AIChE J. 64 (11), 3988–4001. doi:10.1002/aic.16380
Zhang, X., Huang, Y., Gao, H., Luo, X., Liang, Z., and Tontiwachwuthikul, P. (2019). Zeolite catalyst-aided tri-solvent blend amine regeneration: an alternative pathway to reduce the energy consumption in amine-based CO2 capture process. Appl. Energy 240, 827–841. doi:10.1016/j.apenergy.2019.02.089
Zhang, X., Huang, Y., Yang, J., Gao, H., Huang, Y., Luo, X., et al. (2020). Amine-based CO2 capture aided by acid-basic bifunctional catalyst: advancement of amine regeneration using metal modified MCM-41. Chem. Eng. J. 383, 123077. doi:10.1016/j.cej.2019.123077
Zhang, X., Liu, H., Liang, Z., Idem, R., Tontiwachwuthikul, P., Jaber Al-Marri, M., et al. (2018b). Reducing energy consumption of CO2 desorption in CO2-loaded aqueous amine solution using Al2O3/HZSM-5 bifunctional catalysts. Appl. Energy 229, 562–576. doi:10.1016/j.apenergy.2018.07.035
Zhang, X., Zhang, R., Liu, H., Gao, H., and Liang, Z. (2018c). Evaluating CO2 desorption performance in CO2-loaded aqueous tri-solvent blend amines with and without solid acid catalysts. Appl. Energy 218, 417–429. doi:10.1016/j.apenergy.2018.02.087
Zhang, X., Zhang, X., Liu, H., Li, W., Xiao, M., Gao, H., et al. (2017). Reduction of energy requirement of CO2 desorption from a rich CO2-loaded MEA solution by using solid acid catalysts. Appl. Energy 202, 673–684. doi:10.1016/j.apenergy.2017.05.135
Zhang, Z., Yan, Y., Zhang, L., Chen, Y., and Ju, S. (2014). CFD investigation of CO2 capture by methyldiethanolamine and 2-(1-piperazinyl)-ethylamine in membranes: part B. Effect of membrane properties. J. Nat. Gas Sci. Eng. 19, 311–316. doi:10.1016/j.jngse.2014.05.023
Zhao, M., Minett, A. I., and Harris, A. T. (2013). A review of techno-economic models for the retrofitting of conventional pulverised-coal power plants for post-combustion capture (PCC) of CO2. Energy and Environ. Sci. 6 (1), 25–40. doi:10.1039/C2EE22890D
Zhao, Y., Zhang, Y., Liu, Q., Guo, X., Cao, Y., Xu, N., et al. (2023). Energy-efficient carbon dioxide capture using piperazine (PZ) activated EMEA+DEEA water lean solvent: performance and mechanism. Sep. Purif. Technol. 316, 123761. doi:10.1016/j.seppur.2023.123761
Keywords: global warming, carbon capture, chemical absorption, MEA solution, high energy consumption, catalytic regeneration
Citation: Yang Z, Shen Y, Yang H, Yi H, Guo H and Zhang X (2023) A review of CO2 catalytic regeneration research based on MEA solution. Front. Energy Res. 11:1257218. doi: 10.3389/fenrg.2023.1257218
Received: 12 July 2023; Accepted: 21 August 2023;
Published: 01 September 2023.
Edited by:
Jared Taylor, Nova Chemicals, CanadaReviewed by:
Yisong Wang, Northeastern University, ChinaMuhammad Imran Rashid, University of Engineering and Technology, Lahore, Pakistan
Copyright © 2023 Yang, Shen, Yang, Yi, Guo and Zhang. This is an open-access article distributed under the terms of the Creative Commons Attribution License (CC BY). The use, distribution or reproduction in other forums is permitted, provided the original author(s) and the copyright owner(s) are credited and that the original publication in this journal is cited, in accordance with accepted academic practice. No use, distribution or reproduction is permitted which does not comply with these terms.
*Correspondence: Zhiyuan Yang, eWFuZ3p5QHNobXR1LmVkdS5jbg==
†These authors have contributed equally to this work