- 1Ethologie Animale et Humaine (UMR 6552) – Centre National de la Recherche Scientifique, Université de Rennes 1, Rennes, France
- 2Centre d'Ecologie Fonctionnelle et Evolutive (UMR 5175), Centre National de la Recherche Scientifique – Université de Montpellier –Université Paul-Valéry Montpellier – EPHE, Montpellier, France
- 3Developmental Ethology and Cognitive Psychology Group, Centre des Sciences du Goût (UMR 6265 CSGA), Centre National de la Recherche Scientifique-Université de Bourgogne-Franche-Comté, Dijon, France
- 4Department of Arts and Sciences, Université de Nîmes, Nîmes, France
- 5Centre National de la Recherche Scientifique, Ethologie Animale et Humaine (UMR 6552) – Université de Rennes 1, Rennes, France
- 6Département Scientifique et Pédagogique, Planète Sauvage, Port-Saint-Père, France
A large part of the literature on sensory perception and behavior in dolphins is devoted to its well-developed vocal and echolocation abilities. In this review, we aim to augment current knowledge by examining the literature on dolphins' entire “Merkwelt” (which refers to everything a subject perceives, creating a crucial part of the subject's Umwelt). We will show that despite extensive knowledge on audition, aspects such as context relatedness, the social function of vocalizations or socio-sexual recognition, remain poorly understood. Therefore, we propose areas for further lines of investigation. Recent studies have shown that the sensory world of dolphins might well be much more diverse than initially thought. Indeed, although underwater and aerial visual systems differ in dolphins, they have both been shown to be important. Much debated electro- and magnetoreception appear to be functional senses according to recent studies. Finally, another neglected area is chemoreception. We will summarize neuroanatomical and physiological data on olfaction and taste, as well as corresponding behavioral evidence. Taken together, we will identify a number of technical and conceptual reasons for why chemosensory data appear contradictory, which is much debated in the literature. In summary, this article aims to provide both an overview of the current knowledge on dolphin perception, but also offer a basis for further discussion and potential new lines of research.
Dolphin's Umwelt
Sensory perception is essential for the survival of organisms, be it for the detection of (un)favorable physical conditions, the presence/absence of food or predators, the detection of communication signals or the recognition of social partners. It is crucial for any species to perceive regularities and changes in the properties of their abiotic and biotic environment.
The perception of an organism's local environment is one part of a living being's Umwelt (von Uexküll, 1909). The literal translation of Umwelt from German to English is “environment,” but the typical biological meaning is better described as an organism's “subjective universe” (Chien, 2006). Initially, appropriate sensory receptors have to be able to detect the characteristics of surrounding objects, contexts and conspecifics (von Uexküll, 1909). After being perceived, information concerning the object is further processed through corresponding neural structures and a specific meaning is attributed to each stimulus depending on the context or the subject's internal state. Everything an organism perceives creates its Merkwelt (English translation: perceptual world). For the sake of completeness: the other part of its Umwelt is the action a living being is taking on its environment according to the meaning that was previously attributed to the perceived stimuli. Everything an organism does creates its Wirkwelt (English translation: active world).
Sensory receptors and perceptual processing structures are critical in the perception of the environment, thus a species' body plan determines the Umwelt (von Uexküll, 1934). Although several species can share the same environment, each has its own Umwelt as sensory abilities may differ from one species to another. Even within the same species, individuals do not necessarily share identical Umwelten because of morphoanatomical differences caused by genetic defects or events during ontogeny (e.g., a blind and a seeing person may share the same environment, but not the same Umwelt).
It is difficult to determine a species' Umwelt from an external point of view because we, as humans, also possess our own Umwelt. By simply transferring our perception of reality to another species, we do not respect the subjectivity of a specific organism. Indeed, an object that might be meaningful from the human point of view may be meaningless to another species (Delfour, 2010) either because it does not possess the according receptors to perceive the object's feature or because the object, although it can be perceived, does not have an importance for this species. Therefore, an unbiased study of a species' sensory perception and behavior is necessary.
When it comes to sensory perception, cetaceans are particularly informative because they underwent a drastic change in lifestyle in the course of evolution. This mammalian order is currently considered as having evolved about 47 million years ago (MYA) from a small deer-like ancestor (Thewissen et al., 2009), moving from a terrestrial lifestyle back to an aquatic environment. This evolutionary reversal in habitat caused extensive, yet slow rate changes in anatomy, neuroanatomy, physiology, and behavior (Gatesy et al., 2013). The results of this transformation are overtly seen in the baleen whales (Mysticeti) and the toothed whales (Odontoceti). These two suborders are very different in terms of morphology, feeding ecology and behavior, wherefore knowledge gained about mysticete species can be generalized to odontocete species (and vice versa) only with caution, if at all. Therefore, a general “cetacean Umwelt” does not exist. A species-specific perspective is required to understand the Umwelt. The odontocete family Delphinidae includes the best-studied cetacean species; therefore, they present a suitable model to outline their Umwelt. The analysis of the dolphin's perceived environment will begin with a review of some of the sensory abilities of dolphins, namely audition, equilibrioception, vision, somatosensory perception, electroreception, magnetoreception and chemoreception. Each sense is described in a section that comprises anatomical, physiological and behavioral data, followed by propositions for further lines of investigation. Whenever possible, precise data refer to the bottlenose dolphin (Tursiops truncatus), but for a broader view other members of the family of Delphinidae are included, as well as information that are true for Delphinidae or Odontoceti in general. For those sensory modalities where little literature is available for dolphins, this review includes other cetacean species.
Audition
Current Knowledge on Audition
Most research efforts on dolphin sensory systems over the past 50 years has been devoted to the study of audition (reviewed in Au et al., 2000), namely the ability to detect oscillations of pressure transmitted through air, water or another medium. Hearing in cetaceans has been evaluated mostly by auditory evoked potentials (e.g., Mooney et al., 2015) or behavioral audiograms (e.g., Kastelein et al., 2003).
The sounds that are perceived can originate from prey, predators or conspecifics. Beside echolocation, some delphinids are known to detect their prey by passive listening (Barros, 1993; Gannon et al., 2005), meaning that they use the sounds produced by their prey to locate it. Noise-producing fish make up a large part of the bottlenose dolphin's diet (Barros and Wells, 1998). Indeed, it was suggested that the cetacean ancestor developed high-frequency hearing to locate sound-producing fish already in Eocene and based on this ability echolocation evolved in Oligocene odontocetes enabling the location of silent prey (Fahlke et al., 2011).
Sharks (Heithaus, 2001) and orcas (Orcinus orca; Constantine et al., 1998) occasionally attack dolphins. However, not all orcas are hunting mammals (there are also fish-eating orcas) and other cetacean species seem to be able to discriminate between mammal- and fish-eating orcas. The playback of vocalizations of fish-eating orcas elicited an increase in group size in pilot whales (Globicephala melas) and a strong attraction toward the sound (Curé et al., 2012), whereas the playback of vocalizations of mammal-eating orcas prompted a clear avoidance response in beaked whales (Mesoplodon densirostris; Tyack et al., 2011).
Beside natural sound sources such as prey, predators or conspecifics, cetaceans are also exposed to and disturbed by anthropogenic noises originating from military and seismic survey sonars (e.g., Jepson et al., 2003; Piantadosi and Thalmann, 2004), boat noise (e.g., Buckstaff, 2004), or drilling (e.g., Bailey et al., 2010). After loud noise exposure, several cetacean species show a hearing threshold shift, which can be temporary or permanent, meaning a noise-induced hearing loss (e.g., Mooney et al., 2009a; Finneran and Schlundt, 2010; Mann et al., 2010).
Anatomical Data on Audition
The anatomy of the odontocete ear is exclusively adapted for underwater hearing and differs from that of terrestrial mammals: the outer ear pinna as sound collector is replaced with the lower jaw, and the tympanic membrane as sound transmitter is replaced with a thin and large tympanic bone plate (Hemilä et al., 2010). The primary sound perception pathway is considered to be that the lower jaw receives the sound energy and transmits it through fatty tissue located in the mandibular canal (mandibular fat pad) up to the tympanic plate, with best auditory sensitivity at the middle of the lower jaw (Møhl et al., 1999). The mandibular fat pad is composed of triacylglycerol being similar in density, and thus acoustic impedance, to water (Varanasi and Malins, 1971). Middle and inner ear are located together in the tympano-periotic complex that is surrounded by air cushions, which acoustically isolate the ear from the skull (Cranford et al., 2010). Bone density of the tympano-periotic complex increases rapidly during the first months of life, which possibly reflects the importance of hearing for dolphins (Cozzi et al., 2015).
Physiological Data on Audition
Acoustic impulses are transmitted from the ear to the brain via the cochlear nerve, part of the vestibulocochlear nerve (cranial nerve (CN) VIII; Figure 1). Dolphins seem to process auditory impulses in at least two brain areas. The primary auditory cortex is believed to be located in the suprasylvian gyrus along the vertex of the hemispheres, lateral and adjacent to the primary visual cortex (Popov et al., 1986). In addition, a recent study found that the auditory cortex also exists in the temporal lobe (Berns et al., 2015). Odontocetes tend to have a 10-octave functional hearing range with peak sensitivity between 40 and 80 kHz (Wartzok and Ketten, 1999). In the bottlenose dolphin, hearing ranges up to 150 kHz, with optimal sensitivity within 10–80 kHz (Houser and Finneran, 2006). Dolphins, like cetaceans in general, have good directional hearing. Generally, the direction from which a sound originates may be determined by the difference in arrival time of a sound to each of two ears. As this interaural time difference is calculated by , the increased sound velocity in water (compared to air) leads to a reduction in the interaural time difference (compared to air). Cetaceans can compensate for this effect as they have relatively large heads and therefore a naturally large interaural distance, thus increasing the interaural time difference (Nummela and Thewissen, 2008).
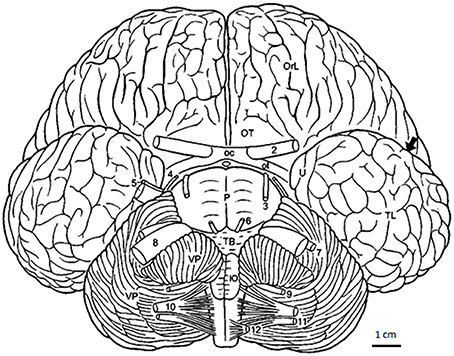
Figure 1. Bottlenose dolphin brain in basal aspect (after Langworthy, 1932, modified after Pilleri and Gihr, 1970; Morgane and Jacobs, 1972). Arrow pointing into sylvian cleft. Ot, optic tract; OT, olfactory tubercle; TL, temporal lobe; U, uncus; VP, ventral paraflocculus; 2-12, cranial nerves; 2, optic nerve; 3, oculomotor nerve; 4, trochlear nerve; 5, trigeminal nerve; 6, abducens nerve; 8, vestibulocochlear nerve; 9, glossopharijngeus nerve; 10, vagus nerve; 11, accessory nerve; 12, hypoglossals nerve. Scale: 1 cm.
Behavioral Data on Audition
Traditionally, research on audition in dolphins has focused on echolocation and communication. Dolphins produce three different categories of vocalizations: clicks, burst-pulsed sounds and whistles (reviewed in Janik, 2009). Clicks are short broadband signals that can exceed 100 kHz and are mostly used for echolocation. Burst-pulsed sounds consist of highly directional, rapid click trains: for example, the bray calls generated by the bottlenose dolphin (Janik, 2000a), the so-called “squawks,” “yelps,” and “barks” (Schultz et al., 1995), as well as “moans” or “rasps” (Caldwell and Caldwell, 1967). The distinction between echolocation clicks and burst-pulsed sounds is not always easy. Whistles are tonal, frequency-modulated signals with fundamental frequencies lying between 800 Hz (Schultz and Corkeron, 1994) and 28.5 kHz (May-Collado and Wartzok, 2008), and often several harmonics. Whistles and burst-pulsed sounds can be produced simultaneously (Janik, 2009). This corresponds with the generally accepted concept that there are two sites of sound production that can be controlled independently (Dormer, 1979). They are composed of two identical sound producing structures consisting of fatty dorsal bursae within a pair of phonic lips, one in the left and one in the right nasal passage (Cranford et al., 1996). A recent study suggested that the two dolphin brain hemispheres, which sleep independently (Lyamin et al., 2008), may also act independently when it comes to coordinating prey capture and communication with simultaneously emitted echolocation clicks and social sounds (Ridgway et al., 2015).
Echolocation
An important function of sound for odontocetes is echolocation (or biosonar), where they emit short sound pulses (clicks) and listen for returning echoes to generate an auditory representation of their surroundings for navigation and foraging (Madsen and Surlykke, 2013). As shorter wavelengths have a better spatial resolution, and wavelength is inversely proportional to frequency, high frequencies are better suitable for detecting small objects compared to low frequencies. Consistently, species inhabiting acoustically complex inshore and river waters use higher frequencies for echolocation (>100 kHz) than near- and offshore species (<100 kHz) that inhabit low object-density environments (Wartzok and Ketten, 1999). Rapid auditory temporal processing facilitates echolocation and sound location (Mooney et al., 2009b). While echolocating, dolphins are able to hear (Li et al., 2011), to adjust their hearing (Nachtigall and Supin, 2008), as well as being able to process the heard echoes and vocalize while still echolocating (Ridgway et al., 2012). Furthermore, dolphins possess several mechanisms for gain control (reviewed in Supin and Nachtigall, 2013). Echolocation is so effective in bottlenose dolphins that they can still detect a small object of less than 8 cm at distances of over 110 m (Au and Snyder, 1980) and discriminate objects by using spectrum shape of the echo, as well as its peak and center frequency (DeLong et al., 2006). Bottlenose dolphins start to echolocate at the early age of around 2 months (Carder, 1983), supporting the hypothesis that the structures involved in hearing develop early in life due to the importance of these inputs for survival and development (Cozzi et al., 2015).
Communication
In a habitat where vision is not always possible, acoustic signals provide a good communication channel, even for over long distances. Most delphinids use whistles for communication, but some species use pulsed sounds (e.g., Commerson's dolphin, Cephalorhynchus commersonii; Yoshida et al., 2014). Why some delphinid and other odontocete species [e.g., the family of Phocoenidae (porpoises), the pygmy sperm whale, Kogia breviceps, and the genus of Pontoporia] do not produce whistles, but only pulse sounds was connected to the orca predation risk. It was hypothesized that species with high orca predation risk were subject to a selective pressure favoring vocalizations restricted to sounds that orcas hear poorly or not at all (i.e., below 2 and above 100 kHz; Morisaka and Connor, 2007). On the other side, orcas adapt their vocal behavior to the prey they are hunting: transient orcas that feed on marine mammals (a prey with sensitive underwater hearing) vocalize less and reduce their vocal activity before and during hunting compared to resident orcas that feed on fish (a prey with poor hearing abilities; Deecke et al., 2005).
Most studies on delphinid communication are concerned with whistles because they are thought to play an important role in social interactions (Díaz López, 2011). Whistles have varying numbers of harmonics and delphinids can distinguish between whistles with and without harmonics (Yuen et al., 2007). Whereas the fundamental frequency is relatively omnidirectional, higher order harmonics are directional (Lammers and Au, 2003). Bottlenose dolphins can discriminate tonal sounds that differ in frequency by only 0.2–0.8% (Thompson and Herman, 1975), but they seem to pay more attention to frequency modulation than to the absolute frequency (Ralston and Herman, 1995). The active space (i.e., the transmission range over which a signal can be detected by conspecifics) for bottlenose dolphins' whistles is determined as 10–20 km for frequencies below 12 kHz (Janik, 2000b). However, the active space of a sound depends (among other factors such as its frequency) on bottom substrate and water depth. For example, the same call is perceived at less than 200 m in a shallow sea grass area of 1.6 m depth, but up to more than 6 km in a sandy bottom area of 3.5 m depth (Quintana-Rizzo et al., 2006).
Bottlenose dolphins are known for their production of signature whistles, which are individually distinctive whistles that do not depend on the individual's voice features, but the whistles' frequency contour (reviewed in Janik and Sayigh, 2013). These are used for individual recognition, for example between mother and offspring (Sayigh et al., 1998). A proof of the dolphins' capacity to discriminate even complex frequency modulations is their call matching which has been experimentally tested in wild dolphins and it seems possible that dolphins use these copies (i.e., mimicking sounds) as referential vocal labels in order to address each other (King and Janik, 2013). Furthermore, dolphins seem to be capable of remembering the signature whistles of other individuals for at least 20 years (Bruck, 2013).
Perspectives on Audition
Although there is a multitude of studies concerning the vocal communication of delphinids, many questions remain unresolved that often due to technical and methodological constraints, such as individually assigned recordings and unlimited access to the animals. The latter concern greatly influences those studies with direct observation of free-ranging cetaceans, which are not always easy to detect and to follow. Technical assistance is necessary to identify the vocalizing individual as it is not possible to visually assign a vocalization to its emitter (i.e., dolphins do not open their mouths to vocalize and or systematically produce any other visible correlate of vocalizing). Although such approaches do exist, they are often expensive and/or possibly disturbing to the animals, as the device is generally secured to the body (e.g., Johnson and Tyack, 2003; Blomqvist and Amundin, 2004). For instance, most studies only tag one or a few individuals, which may result in limited data that does not appropriately address a particular research question. This technique might not be suitable for studies that want to test the social function of vocalizations or communication rules during vocal exchanges as this is difficult, if not impossible, when only one group member is tagged.
Alternatively, individuals can be temporarily restrained to record individually assigned vocalizations (e.g., Watwood et al., 2005), but this particular context does not allow for a broad range of vocalizations to be recorded and further studied as stress strongly influences the pattern and content of vocalizations. For example, bottlenose dolphins are thought to encode their level of stress via the whistle rate (Caldwell et al., 1990) and an alteration of acoustic parameters, while keeping the overall frequency modulation pattern constant (Esch et al., 2009). Another, less invasive technique is triangulation, where the location of the sound source is determined by recording with two or more hydrophones and then calculating the origin of the sound. Therefore, simultaneous visual information is necessary to identify the individual that is present at the location calculated as the sound source, making this technique sometimes difficult to apply to free-ranging dolphins.
With regards to echolocation, there is an approach that might yield some insightful results: the “echolocation visualization and interface system,” which can visualize echolocation signals and be used as an “acoustically operated ‘touch screen”’ (Amundin et al., 2008). The echolocation signals of dolphins are recorded with hydrophones when those clicks are aimed at a semitransparent screen. Subsequently, the recorded acoustic signals are translated into a corresponding visual image that is projected on the location where the echolocation signals contact the screen: this leads to immediate visual feedback (Amundin et al., 2008). Another approach is eavesdropping on echolocation signals of conspecifics (reviewed in Gregg et al., 2007). In a behavioral experiment, a bottlenose dolphin was able to correctly choose an object in a matching-to-sample task by eavesdropping on the echoes produced by the echolocation signals of a conspecific (Xitco and Roitblat, 1996). However, the ecological implications of this passive echolocation remain unknown.
Equilibrioception
Current Knowledge on Equilibrioception
Equilibrioception is the sense of balance, which provides information about the body's movement. Due to physical differences, more three-dimensional movements are possible in water compared to land, which lead to an increased importance of equilibrioception for aquatic species.
Anatomical Data on Equilibrioception
In vertebrates in general, the sensory organ of balance is the vestibular system in the inner ears. Linear movement and gravity are detected by the two otolith organs, one in the utricle and the other in the saccule, which are located in the vestibule (Rabbitt et al., 2004). Rotational movements are detected by the three membranous semicircular ducts, which are enclosed by the three bony semicircular canals (anterior, posterior, and lateral; Graf, 1988). The otolith organ seems to be well developed and fully functional in cetaceans, with a thicker membrane compared to other mammals (Solntseva, 2001). The semicircular canal system of cetaceans is smaller relative to body size when compared to other mammals. In bottlenose dolphins, the mean radius of curvature of the three canals is 1.1 mm (Spoor and Thewissen, 2008). For comparison: in greater kudus (Tragelaphus strepsiceros), an Artiodactyla species with a similar body mass, the mean radius of curvature of the three canals is 3.5 mm (Spoor and Thewissen, 2008). Furthermore, the cetacean lateral canal is the largest and the posterior canal the smallest of the three semicircular canals (reviewed in Spoor and Thewissen, 2008). In bottlenose dolphins, the relative size of the anterior, posterior and lateral canal is 34, 28, and 38%, respectively. This is in contrast to other mammals where the lateral canal is the smallest if the three canals.
The size reduction concerns only the semicircular canals, and not the entire inner ear of cetaceans, as their cochlea is similar in size relative to body mass when compared to other mammals (Spoor et al., 2002). Is was proposed that this size reduction is due to the dominant auditory function of the inner ear and thus a limited space for the vestibular system (Boenninghaus, 1903). Another explanation for the comparatively small semicircular canal system in cetaceans concerns its sensitivity: the smaller the semicircular canal system, the less sensitive it is. What seems disadvantageous at first sight might be favorable for species with increased head movements, as is the case for cetaceans due to their swimming movement and fused cervical vertebrae (leading to a mostly immobile neck that no longer compensates for body movement to stabilize the head). Here, reduced sensitivity of the sense of balance might help to avoid an overstimulation of the semicircular canal system, which would otherwise lead to disorienting effects (Spoor et al., 2002). Consistent with this hypothesis is the fact that size reduction is more pronounced in Odontoceti, which are more agile compared to Mysticeti (Spoor and Thewissen, 2008).
Physiological Data on Equilibrioception
It is presumed that equilibrioception in dolphins works physiologically similar to other species: impulses from the vestibular system are transmitted to the brain via the vestibular nerve, part of the vestibulocochlear nerve (CN VIII). The information is then processed in the vestibular brain stem nuclei, which transmit neural signals to motor nuclei in order to generate reflexive movements of the eyes and/or other body parts in order to stabilize the body (Sipla and Spoor, 2008).
Vision
Current Knowledge on Vision
Another important sense to perceive the environment is vision, which constitutes the ability to detect variations in the intensity and wavelength of light. When light passes through water it is differently absorbed, refracted and scattered depending on the wavelength of the light, as well as the concentration and type of dissolved material in the water. In shallow waters, longer wavelengths of the light spectrum are common, whereas only shorter wavelengths can penetrate well into deeper layers of water (Wartzok and Ketten, 1999). In general, light decreases with depth.
Anatomical and Physiological Data on Vision
As in all cetaceans, dolphin eyes are located laterally (directed ventronasally), allowing a panoramic vision with a 120–130° visual field, and can be moved independently from each other (Mass and Supin, 2009). Several anatomical structures inside the eyes protect them from mechanical damage (e.g., a thickened cornea to resist water pressure) or cooling (Mass and Supin, 2009). Furthermore, a secretion produced by the Harderian gland protects the eyes from the high concentration of salt in marine water (Dawson et al., 1972, 1987). Bottlenose dolphins have good underwater and in-air vision with a visual acuity of 12.6 min of visual angle from a distance of 2.5 m (Herman et al., 1975), probably due to their asymmetric double-slit pupils (Rivamonte, 2009) and excellent distance estimation (Mobley and Helweg, 1990). Under low-light conditions this pupil is round and roughly U-shaped in bright light conditions (Mass and Supin, 2009). In general, the lens of the cetacean eye is very strong and more similar to those of fish compared to the lens of terrestrial mammals (Wartzok and Ketten, 1999).
In cetaceans, visual sensitivity is maximized by a high density of photoreceptors (400,000 cells/mm2 in bottlenose dolphins; Dral, 1977), as well as a tapetum lucidum (i.e., a reflective layer behind the retina that increases the amount of light absorbed by the photoreceptors; Dawson, 1980). Both rod and cone photoreceptors have been described in the retina of bottlenose dolphins (Perez et al., 1972), with absorption maxima of 488 and 524 nm for the rod and cone pigments, respectively, which are both short-wavelength shifted compared to many terrestrial mammals (Fasick et al., 1998). However, bottlenose dolphins only possess long/middle-wavelength-sensitive L-cones but no short-wavelength-sensitive S-cones, thus they are thought to lack the common dichromatic vision typical of many terrestrial mammals and may, therefore, be colorblind (Simons, 1977; Fasick et al., 1998; Peichl et al., 2001). However, under mesopic conditions, where both rods and cones are active, bottlenose dolphins (as well as other so-called monochromatic cetaceans) might exhibit “conditional dichromacy” and a rudimentary form of color vision (Davies et al., 2012), as hypothesized for wobbegong sharks (Theiss et al., 2012).
The dolphin retina possesses (partly giant) ganglion cells (Perez et al., 1972) with a density of up to 670 cells/mm2 (Mass and Supin, 1995). The retinal ganglion cells receive visual information from the photoreceptors via inter-retinal neurons and transmit them through the optic nerve (CN II). The optic nerve has a low fiber density (50,000 fibers/mm2 compared to >220,000 fibers/mm2 in monkeys), which in bottlenose dolphin comprise of 150,000–180,000 optic fibers in total (Mass and Supin, 2009).
Visual impulses are transmitted by the optic nerve to the midbrain, the thalamus and the cerebral cortex (superior colliculus, lateral geniculate nucleus and primary visual cortex) where they are processed further (Glezer et al., 1995).
Behavioral Data on Vision
Tested in a visual-matching task, the patterns of perceptual similarities for two-dimensional forms of dolphins was found to be similar to those of chimpanzees and humans (Tomonaga et al., 2014). Contrarily to the previously mentioned hypothesis of color blindness in dolphins (Peichl et al., 2001), a behavioral experiment showed that a bottlenose dolphin had two peaks in spectral sensitivity and that it could discriminate between two wavelengths with equal brightness (Griebel and Schmid, 2002). These findings are consistent with the “conditional dichromacy” hypothesis (Davies et al., 2012) mentioned above. The debate on the evolution and underlying mechanisms of cetacean color vision is still ongoing (Griebel and Peichl, 2003; Meredith et al., 2013).
Delphinids use their good sense of sight in a variety of contexts, from social interactions to prey capture. In short-range communication, visual displays are known to play an important role for delphinids. Postures are thought to signal intent and demeanor of the signal emitter (Dudzinski, 1996). The S-posture, in which the dolphin's body is bent into an S-shape (head pointing down, pectoral fins stretched out), is often associated with aggressive behaviors that include sexual interactions and disciplinary behavior toward juveniles (Dudzinski, 1996; Bojanowski, 2002). The S-posture is consistent with aggressive behaviors in other cetaceans too (e.g., humpback whales, Megaptera novaeangliae), which might be comparable with the arched head and neck position known in many terrestrial mammals during displays of aggression (Dudzinski, 1996). The dolphin's head-to-head posture is often accompanied by jaw claps, hits, tail hits and “squawks” (burst-pulsed sounds) that are thought to express irritation or anger (Dudzinski, 1996). Jaw claps and head jerks are also described by Connor et al. (2000) as aggressive behaviors. Furthermore, these authors describe a distinct posture, in which the dolphin arches the head and flukes down, which may be used to threaten another dolphin.
Vision also mediates non-aggressive interactions. Affiliation between individuals is, among others, expressed by proximity and synchronous movements (Connor et al., 2000). Another visual display occurs in reproductive contexts; for example, when dolphins present their genital region to sexually attract a mating partner (Tyack, 2000).
There is some evidence that dolphins use pointing gestures (Xitco et al., 2001) and that complex behaviors such as foraging techniques are taught by action imitation that in turn require observation and good vision (Bender et al., 2009; Abramson et al., 2013).
Beside conspecifics, cetaceans use vision for the inspection of their surroundings, both in water and air. A common behavior of several cetacean species is spyhopping, i.e., surfacing vertically and lifting the head out of the water (e.g., Ford, 1984; Whitehead and Weilgart, 1991; Jensen et al., 2013), that seems to serve the inspection of objects above water (Madsen and Herman, 1980). When inspecting objects or humans, familiarity of the object/human to dolphins influences their behavior: dolphins show a visual laterality, using the left eye when looking at familiar objects and the right eye when looking at unfamiliar objects (Blois-Heulin et al., 2012). Furthermore, their gaze lasts longer when viewing unfamiliar humans compared to those that are familiar (Thieltges et al., 2011). Dolphins use their accurate vision, for example, when catching fish in air after they have hit them firmly with their fluke (Wells et al., 1987). Some foraging behaviors of the bottlenose dolphin were also found to be lateralized, meaning a localization of function or activity on one side of the body in preference to the other (e.g., Silber and Fertl, 1995; Lewis and Schroeder, 2003). It was suggested that the observed right-sided lateralization in dolphins (but also whales) when foraging may be associated with the visual perception of prey (Karenina et al., 2016).
Perspectives on Vision
Color vision is another topic that appears worth of further analyses. Knowing which opsin-based photopigments are expressed in the cetacean retina and their corresponding spectral sensitivities only suggest the potential for color vision. However, behavioral experiments are critical to understanding the functional consequences of the suggested colorblindness or hypothesized “conditional dichromacy” that might exist in many so-called marine mammal monochromats. For example, the normally white ventral side of bottlenose dolphins can be remarkable pink in periods of high sexual activity (personal observation of the authors), which might be used as a reproductive visual cue. How males react to a female whose abdomen is colored pink (either in the field or altered experimentally) is unknown and worthy of further study.
Somatosensory Perception
Current Knowledge on Somatosensory Perception
Somatosensory systems comprise the perception of touch (via pressure and strokes), pain (nociception), temperature (thermoreception), and body position (kinesthesis and proprioception). Several different receptor types are involved (including mechanoreceptors, nociceptors, and thermoreceptors) that are located in the dermis, muscles and joints. Aquatic species can perceive water movement through their mechanoreceptors (e.g., Dehnhardlt et al., 1998). The ability to know the relative body position of an organism is crucial for an air-breathing animal that lives in a three-dimensional underwater habitat in order to orient itself toward the surface even when no visual cues are available and to feel whether the blowhole is above the water (to ensure respiration).
Anatomical Data on Somatosensory Perception
The skin of bottlenose dolphins is furrowed by small ridges that are circumferentially oriented in the anterior part of the body (head to dorsal fin) and more obliquely positioned in the posterior part of the body (dorsal fin to caudal fin). The function of these ridges has been implicated in tactile sensing (Shoemaker and Ridgway, 1991), hydrodynamics (Ridgway and Carder, 1993) or both. In the region of the blowhole, large numbers of mechanoreceptors are present that are thought to serve in the perception of pressure changes that occur when the whale/dolphin breaks through the water surface in order to ensure that the blowhole is opened for respiration only after surfacing (Bryden and Molyneux, 1986). Most odontocetes possess vibrissae (i.e., sensory hair), especially on the rostrum of newborns, losing them shortly after birth (Ling, 1977). Thus, adult dolphins possess hairless vibrissal/follicle crypts on the rostrum, except for the Amazon River dolphin (Inia geoffrensis) where the presence of rostral sensory hairs continues into adulthood (Dehnhardt and Mauck, 2008). In general, vibrissae are more common in mysticetes (e.g., Drake et al., 2015).
Physiological Data on Somatosensory Perception
Cetacean skin is well innervated and very sensitive to touch (Tyack, 2000). Skin sensitivity was examined by studies using either somatosensory evoked potentials (e.g., Lende and Welke, 1972) or the galvanic skin response (e.g., Kolchin and Bel'kovich, 1973). Dolphins are most sensitive on their heads (corners of the mouth, eyes, snout, melon, and the area around the blowhole), reaching a sensitivity comparable to human fingertips or lips (Ridgway and Carder, 1990). Somatosensory information is processed in the postcruciate gyrus of the cerebral cortex (Supin et al., 2001).
Behavioral Data on Somatosensory Perception
Dolphins are able to perceive pressures as small as 10 mg/mm2 around the blowhole and the eyes (Kolchin and Bel'kovich, 1973). Besides the surrounding water, somatic stimuli can originate from objects in the environment. Rubbing occurs in both captive and free-ranging cetaceans. Delphinids were found rubbing their bodies on particular substrates (e.g., pebbles, sand, or along rocky edges; Smith et al., 1992; Whitehead et al., 2004; Rossi-Santos and Wedekin, 2006), which may possibly have a role in pleasure, hygiene (Dudzinski et al., 2012), or might even be a result of play behavior (Kuczaj et al., 2006).
Touch is also an important short-range communication signal utilized during play, sexual, maternal and social contexts, and involves the entire body (Dudzinski et al., 2009a). Tactile contacts between dolphin conspecifics can be observed during aggressive interactions (including biting etc.), but are also common in affiliative contexts (Paulos et al., 2008; Dudzinski et al., 2009b, 2010, 2012). Affiliation between individuals is expressed by proximity and physical contact (Connor et al., 2000), which includes contact swimming, gentle stroking with the pectoral fin or rubbing against another individual. Sakai et al. (2006) reported that flipper rubbing in wild Indo-Pacific bottlenose dolphins (Tursiops aduncus) is an affiliative behavior, which could be a quantitative measure of social relationships among individuals. Tamaki et al. (2006) reported that flipper rubbing might contribute to restore friendly relationships between former opponents or reduce conflicts. Thus, flipper rubbing may be the cetacean equivalent of primate grooming (Tamaki et al., 2006; Connor, 2007). People working with delphinids in captivity suggest that petting is appreciated by the animals and, therefore, could be used as a reinforcer in training (Dudzinski et al., 2009a).
Perspectives on Somatosensory Perception
Most odontocetes possess vibrissae, especially on the rostrum, right from birth (Ling, 1977). It would be informative to test whether these perioral hairs serve a tactile function in the context of suckling, when acoustic (echolocation) and visual abilities of young dolphins are not fully developed.
Electroreception
Current Knowledge on Electroreception
Electroreception is the ability to detect an electric field. Electric stimuli can originate from both abiotic and biotic sources. Bioelectric fields are generated for instance by all muscle movement and the water medium provides ideal conditions for conducting electrical currents, although the spread of these stimuli is far less in the marine environment compared to freshwater (Czech-Damal et al., 2013). In active electroreception, the animal itself generates an electric field and senses distortion of this field from objects of varying conductivity present in its habitat; for example, in electric eels (Electrophorus electricus; Souza et al., 2007). In passive electroreception, the animal perceives electric fields generated by an object that is located in close vicinity (Czech-Damal et al., 2012); for example, prey detection by elasmobranchs (Kalmijn, 1971) that possess electroreceptors called ampullae of Lorenzini (Murray, 1960).
Behavioral Data on Electroreception
So far, there is only one study on electroreception in cetaceans, which analyzes the behavioral response of a trained Guiana dolphin (Sotalia guianensis) toward electrical stimuli. The dolphin has been found to be sensitive to weak electric currents, such as those emitted by the muscles of prey fish buried in the sediment (Czech-Damal et al., 2012). The electroreceptors are probably situated within the hairless vibrissal crypts on the rostrum (Czech-Damal et al., 2013). As a control, these vibrissal crypts were covered with a plastic shell to prevent contact with seawater. After such treatment, the dolphin could not detect the electric stimuli, whereas a plastic shell that did not impede seawater from contacting the vibrissal crypts did not affect the dolphin's ability to detect electric stimuli (Czech-Damal et al., 2012).
Perspectives on Electroreception
Electroreceptors as found in the Guiana dolphin (Czech-Damal et al., 2012), however, further investigation is essential in other dolphin species to determine their broad functional role in electroreception. Bottlenose dolphins, for example, possess vibrissal crypts on their rostrum, but their involvement in electroreception has not been assessed. Passive electroreception could function as a supplementary sense to echolocation during benthic feeding (Roitblat et al., 1995), which is not uncommon in bottlenose dolphins (Rossbach and Herzing, 1997).
Magnetoreception
Current Knowledge on Magnetoreception
Magnetoreception is the ability to perceive a magnetic field. The Earth's magnetic field is a dipole field that is generated by the Earth's fluid outer iron core (Wiltschko and Wiltschko, 1995), providing a consistent source of directional information (Winklhofer, 2010). Its intensity ranges from over 60,000 nanoteslas (nT) near the magnetic poles to 30,000 nT at the magnetic equator, but shows minimum values below 26,000 nT at the east coast of South America. In the ocean, the magnetic topography (i.e., variation in the magnetic field) is regular and stable long-term, with “magnetic hills” (i.e., local higher total intensities) and “magnetic valleys” (i.e., local lower intensities) quasi-symmetrically arranged on both sides of the mid-oceanic ridge. There are some anomalies that run parallel on opposite sides of the ridge and some that are found in a perpendicular orientation (reviewed in Walker and Dennis, 2005). Differently magnetized rocks can cause such local anomalies (Wiltschko and Wiltschko, 1995). Besides spatial variation, the geomagnetic field also shows temporal variation caused by solar electromagnetic radiation (leading to regular daily variations) or sun spot activity (leading to irregular fluctuations called magnetic storms; Wiltschko and Wiltschko, 1995).
Physiological Data on Magnetoreception
There are two main mechanisms that underpin the perception of a magnetic field, namely those that are based on induction or are reliant on magnetite (reviewed in Wiltschko and Wiltschko, 1995). Induction-based perception assumes that the electric field, which is generated by the magnetic field, is detected by electroreceptors; it is dependent on the conductivity of the surrounding medium, thus salt water provides a suitable conductive medium. By contrast, magnetite-based perception is mediated by ferromagnetic particles such as magnetite (iron oxide). These miniature magnets align themselves in the magnetic field and are connected to the central nervous system; however, the exact pathways of signal transmission are still unclear (Lohmann and Johnsen, 2000). Magnetite has been found in the dura mater of both bottlenose (Bauer et al., 1985) and short-beaked common dolphins (Delphinus delphis), where nerve fibers have been identified adjacent to the surface of the iron oxide particles (Zoeger et al., 1981).
Behavioral Data on Magnetoreception
Magnetoreception is commonly used for navigation, i.e., animal orientation based on the geomagnetic field (reviewed in Wiltschko and Wiltschko, 1995). Observations of free-ranging cetaceans show some evidence of magnetoreception-based navigation. For example, fin whale (Balaenoptera physalus) migration routes are correlated with low geomagnetic intensity (Walker et al., 1992) and offshore cetacean live strandings seem to occur where valleys in the geomagnetic field cross the coast (Klinowska, 1985; Kirschvink et al., 1986). However, foraging routes of wild short-beaked common dolphins do not seem to be influenced by the geomagnetic field (Hui, 1994). Generally, there are few studies that test magnetoreception in dolphins. Kuznetsov (1999) reported that neurovegetative responses in dolphins, such as the electrocardiogram, galvanic skin responses and respiration, are altered by changes in the magnetic field. The author interpreted this as “a high sensitivity of the dolphin to changes in the permanent magnetic field (a ‘magnetic sense’)”. However, as this study is only presented as an abstract, it is difficult to evaluate both the data and conclusions of the work. When captive bottlenose dolphins were exposed to a magnetic field created in their pool by an induction coil (magnetic field strength unknown), they did not show any differential response (Bauer et al., 1985). Even during a series of conditioning experiments using two-choice discrimination and go/no-go designs (magnetic field strength: 37 μT), the dolphins did not show any indication of magnetic discrimination (Bauer et al., 1985). However, Bauer et al. (1985) admitted “experiments that constrain the subject in time and place may be putting significant limits on appropriate orientation.” In a recent study, we conducted an experiment that neither confined dolphins spatially to a given position (as, for example, during a go/no-go experiment), nor demanded a direct response (as it is the case in conditioning experiments), but rather observed their spontaneous reaction toward magnetized and demagnetized devices. Dolphins approached the device with shorter latency when it contained a strongly magnetized neodymium block (magnetic field strength of 1.2 T) compared to a control demagnetized block that was identical in form and density and, therefore, undistinguishable through echolocation (Kremers et al., 2014). This finding suggests that dolphins may be able to discriminate the two stimuli used in our study based on their magnetic properties.
Perspectives on Magnetoreception
The mechanisms underpinning magnetoreception still need to be studied in dolphins, as no primary magnetoreceptors have been identified unequivocally (Lohmann and Johnsen, 2000; Winklhofer, 2010). To date, only a magnetite-based system has been proposed in cetaceans (Walker et al., 1992). Since the geomagnetic field is on average 4.5 μT strong (Wiltschko and Wiltschko, 1995), it is not clear whether or not dolphins are sensitivity enough to perceive and use geomagnetic cues for navigation. As such, further studies concerning the magnetic perception threshold, as well as the possible influence of the orientation of the magnetic field, on dolphin behavior awaits to be tested. Finally, it is still unclear whether magnetic fields are attractive or repulsive to dolphins. Such information could be important for the development of repellent devices that could, for example, protect fishing nets from foraging dolphins and simultaneously decrease the dolphins' risk of entanglement in those nets.
Chemoreception
Compared to the other senses, chemoreception has drawn little empirical attention in marine mammals and its functional status in cetaceans remains unknown. The different modalities of chemoreception (i.e., olfaction, vomerolfaction, gustation, and trigeminal sensation) are sometimes difficult to tell apart in aquatic animals due to less clear physiochemical selectivity of stimuli conveyed by water (Hemilä and Reuter, 2008). Moreover, it is possible that chemoreceptors of aquatic mammals are found on unexpected body parts compared to terrestrial mammals. Cetaceans might possess chemoreceptors allowing them to sense all types of substances carried in either water or air (Hemilä and Reuter, 2008). However, chemoreceptive structures known from terrestrial mammals may be modified, displaced, reduced or absent in extant cetaceans due to evolutionary adaptation to an aquatic environment. The latter appears to be the case for the vomeronasal organ and related accessory olfactory tracts (Thewissen, 2009), although a recent study found some evidence for the potential presence of a vomeronasal organ in a neonate gray whale (Eschrichtius robustus; Kienle et al., 2015). Accordingly, the following sections will only focus on olfaction and gustation.
Current Knowledge on Olfaction
Olfaction is traditionally defined as the ability to detect airborne volatile compounds, viz. compounds having a molecular weight below 400 Dalton (Hemilä and Reuter, 2008; Mollo et al., 2014). In terrestrial mammals, odorants have to dissolve in the mucus covering the olfactory epithelium inside the nasal cavity, where they adhere to binding proteins that in turn activate olfactory receptors (ORs) located on the cilia of sensory neurons that transmit impulses to the brain via the olfactory nerve (CN I). However, olfactorily active stimuli can be conveyed by water, as shown, for example, in the fetuses of mammalian terrestrial species that react to acute chemical stimulations and detect the chemosensory qualities of their amniotic environment (Schaal and Orgeur, 1992). Thus, even when the detected chemicals are waterborne, the modality has to be considered as olfaction if the neural transmission pathway involves CN I (e.g., Hara, 1994). The important point here is that olfaction can be fully functional under aquatic conditions. For instance, several marine (Davis et al., 2006; DeBose et al., 2008) and freshwater (Hara, 2006) fish species are known to use odorants as social or foraging cues and display a specific behavior called “sniffing” or “coughing” to drive water into the olfactory sacs, thus increasing the supply to the olfactory epithelium (Nevitt, 1991). In addition, the olfactory modality might not necessarily require receptor cells that are exclusively located within the nasal cavity.
Anatomical Data on Olfaction
The nasal cavity is not considered to be involved in olfaction in odontocetes as it accommodates parts of their echolocation system and because the cribriform plate of the ethmoid bone, as well as the ethmoturbinals, are absent (Breathnach, 1960). The main and accessory olfactory tracts are absent in toothed whales and considerably reduced or absent in baleen whales (Breathnach, 1960; Oelschläger, 2008). Furthermore, CN I appears to vanish during early ontogeny in both of these species (Oelschläger and Buhl, 1985).
By contrast, other studies imply that cetaceans may possess neural structures involved in olfaction. Chemoreceptor cells were found in the nasal cavity (frontal and vestibular sacs) of harbor porpoises (Phocoena phocoena; Behrmann, 1989), perhaps enabling some kind of odor sensation. Odontoceti were found to possess a well-developed olfactory tubercle (Oelschläger and Oelschläger, 2009). In bowhead whales (Balaena mysticetus), a complex olfactory bulb and olfactory tracts are present and more than half of the OR genes are intact, suggesting a potentially functional sense of smell (Thewissen et al., 2011; Kishida et al., 2015a). However, OR genes are reported to be functionally reduced by pseudogenization in Odontoceti (Kishida et al., 2007). Bottlenose dolphins possess only two class I and ten class II OR genes that are intact, as well as a single vomeronasal receptor type 1 gene (Kishida et al., 2015b).
Current Knowledge on Gustation
Gustation is the ability to detect waterborne compounds such as hydrophilic substances (i.e., organic acids, amino acids, or nucleotides), but also traces of all sorts of miscible or hydrophobic compounds (Hemilä and Reuter, 2008), that are ingested with prey or during social interactions. Gustation provides information about water or food materials already in the mouth, through taste bud receptor cells that are located on the tongue, palate, epiglottis, esophagus and duodenum (Purves et al., 2001).
Anatomical Data on Gustation
No taste buds were found on the tongue or other areas in the oral cavity of various odontocete species (Kuznetzov, 1990). However, the number and age of individuals investigated are often unknown or very limited; therefore, these findings remain unconvincing. Nevertheless, several authors have suggested that cetaceans in general and odontocetes in particular should exhibit taste sensation (e.g., Pfeiffer et al., 2001). Taste buds were indeed found in younger individuals of the same species that were previously described as absent in adults (Yamasaki et al., 1978; Behrmann, 1988; Kuznetzov, 1990). Other studies did not describe taste buds, but found marginal vallate papillae on the tongue of dolphins, known to be potential locations of taste buds (Kastelein and Dubbeldam, 1990; Werth, 2007), as well as cells that resemble von Ebner's glands (also called gustatory glands) that might be important for chemoreception (Ferrando et al., 2010).
Physiological Data on Gustation
It was proposed that in dolphins the well-developed trigeminal nerve (CN V; Oelschläger, 2008) might provide a pathway to transmit impulses from the oral cavity to the brain, called trigeminal chemoreception (Kuznetzov, 1990). In mammals, CN V innervates the oral and nasal cavities, as well as the eyes, and responds especially to chemical irritants (Silver and Finger, 2009). Unlike other mammals, where CN VII innervates the lingual taste buds (Purves et al., 2001), this nerve does not seem to be involved in dolphin chemoreception, but rather in acoustic signal production (Oelschläger, 2008). However, CN V, just like CN VII, is able to excite gustatory neurons in the nucleus of the solitary tract in the brainstem of other mammals (Purves et al., 2001; Boucher et al., 2003), so it might be involved in taste perception in cetaceans.
Taste receptor genes are reported to be mostly pseudogenized in Odontoceti: in bottlenose dolphins, sweet, umami, bitter and sour taste receptor genes are non-functional, whereas salty taste receptor genes are intact and potentially have functional roles in gustation (Jiang et al., 2013; Feng et al., 2014; Kishida et al., 2015b). Recent molecular findings suggest that this reduction of gustatory abilities in cetaceans occurred between the Artiodactyla-Cetacea and the Odontoceti-Mysticeti evolutionary divisions (Kishida et al., 2015b).
Behavioral Data on Gustation
Behavioral studies have shown that bottlenose dolphins can perceive the sour and bitter tastes of citric acid and quinine sulfate/hydrochloride dehydrate solutions, respectively, nearly as well as humans (Nachtigall and Hall, 1984; Friedl et al., 1990; Kuznetzov, 1990). Moreover, they were able to detect the salty taste of sodium chloride solution (Friedl et al., 1990; Kuznetzov, 1990). Studies that test the perception of sweet stimuli are contradictory, stating that dolphins are able to perceive the sweet taste of sucrose solution (Friedl et al., 1990) or not (Kuznetzov, 1990). In addition to these simple tastants, dolphins were able to detect complex tastants, such as those conveyed in conspecific urine and feces (Kuznetzov, 1990). Kuznetzov (1990) proposed the term “quasi-olfaction” to describe the chemical sense in dolphins that combines characteristics of both gustation and olfaction. Recent behavioral studies suggest that dolphins are able to detect airborne odors and discriminate between different flavors (Kremers et al., 2016).
Perspectives on Chemoreception
Generally, the air above the ocean is chemically less rich compared to air above land, wherefore the chemical environment for air-breathing aquatic species is less diverse compared to terrestrial species: indeed, this lack of chemical variation might have been one of the main drivers that led to the evolution of more specialized sensory abilities in aquatic species. Water birds, for example, show adaption to their aquatic environment in their olfactory receptor complement compared to land birds, with OR families 2/13, 51, and 52 (that were correlated with aquatic adaptations) being expanded (Khan et al., 2015). Just as other marine species are able to detect chemical compounds and exploit them as source of information (e.g., from conspecifics, prey, and predators), it is not unreasonable to hypothesize that dolphins might use a similar sensory system, either by detecting airborne molecules when they surface or waterborne cues. Indeed, fish emit chemical cues that are perceived by other fish and used for the detection of conspecifics, prey and predators, as well as analyzing the chemical profile of water to direct locomotion (Hara, 1994; Hirvonen et al., 2000). Although it is widely accepted that dolphins use echolocation to locate prey, it may be possible that dolphins also use chemical cues to identify and assess the quality of prey. These questions have not been investigated so far, which is probably due to technical issues and the availability of animals for testing.
Extrapolations from anatomical data in determining actual sensory capacities may have been over-interpreted and should be revised accordingly. For example, the reduced size of chemoreceptive organs in some cetaceans do not exclude that a given species may exhibit specialized sensitivity to particular chemical cues (Pihlström et al., 2005; Nummela et al., 2013). As the chemical senses in dolphins are not yet sufficiently delineated, all chemosensory modalities (i.e., olfaction, gustation, and trigeminal sensation) are potentially involved. Behavioral studies might be a good approach to investigate the functional status of chemoreception in dolphins as anatomical and genetic studies have yielded conflicted and controversial results. Similarly, it was proposed that sweet taste perception in hummingbirds (who lack the specific corresponding taste receptor genes) is enabled through unrelated taste receptors that have undergone a change in function (Baldwin et al., 2014). Thus, the simple absence or pseudogenization of taste or olfactory receptor genes should not be the sole basis for proposing firm conclusions that relate to the chemosensory abilities of a particular species: appropriately conducted and controlled behavioral studies should be included.
In general, go/no-go tasks are an elegant way to investigate perceptual abilities as the behaviors displayed by dolphins in response to internal (e.g., pleasure/liking, aversion, interest, fright etc.) or external stimuli can be subtle. However, these experiments require that dolphins are trained, thus preventing the investigation of spontaneous responses or preferences. Similar to experiments conducted to test gustatory stimuli, detection thresholds for airborne stimuli could be determined using go/no-go tasks. Furthermore, dolphins could be trained to react to the presence of an odor with a certain response (e.g., choosing one of two proposed symbols or buttons) and to the absence of another, using positive reinforcement.
Although dolphinariums provide a good opportunity to train dolphins involving several research tasks, there are a number of constraints: for example, training sessions and actual presentations for public viewing limit the time available for experimentation. Furthermore, some methods that work well in captivity are not applicable in the field. For instance, in contrast to captive dolphins, wild dolphins cannot be trained; therefore, other methods may have to be developed for the same research tasks. While chemoreception may be tested in captive dolphins by using odor sources close to the pool or ice cubes (see Kremers et al., 2016), floating dispensers (such as the ones used for chlorine tablets in swimming pools) may be adopted for wild dolphins. For olfactory studies, the substance to be tested is simply placed inside the device. For gustatory studies, the substance is contained within large ice cubes, which themselves are placed within the device. When submerged into water, this allows for a slow release of the tastant through holes located at the bottom of the device. The behavior of the dolphins toward the device, such as their distance to it or their approach latency, can then be analyzed and compared with other tested substances or controls. Given the fact that such observations are made at the surface of the water body, they are often inaccurate due to the shallow angle of the observer; therefore, it might be helpful to use drones (i.e., small unmanned aerial vehicle that are remotely controlled) equipped with cameras to record animal movements from above. An aerial view with an approximately perpendicular angle has the advantage that even animals under the water surface are visible (as long as they are not too deep). Furthermore, the use of drones would permit an increased distance between the boat and the device, thereby reducing the potential disturbance to the test subjects.
It would be informative to test different chemical cues within diverse experimental contexts. As dolphins prefer fish species with a high energy density (Spitz et al., 2010), it would be insightful to investigate if food choices are dependent on chemical cues. Therefore, a test with high vs. low energy density fish would be revealing. Another substance to test is dimethyl sulfide (DMS), which is released by phytoplankton when being grazed on by zooplankton (Dacey and Wakeham, 1986), and used by predators (including at least one other marine mammal, the harbor seal Phoca vitulina vitulina) to find their prey (Nevitt et al., 1995; Kowalewsky et al., 2006; Wright et al., 2011). As phytoplankton attracts zooplankton and zooplankton in turn attracts fish, the ability to detect DMS might allow dolphins to find fish. Indeed, prey detection by using chemical cues has already been suggested for bowhead whales (Thewissen et al., 2011).
Another approach would be to test for chemical cues in social and reproductive contexts, as individual recognition or mate detection (e.g., female receptiveness) could be chemically mediated as is common in many other species. Therefore, the use of urine (given the fact that dolphins seem to be able to detect urine and feces; Kuznetzov, 1990) and/or excretions from the urogenital glands could be used as stimuli (e.g., those obtained from known and unknown individuals or receptive and non-receptive females). The idea of odor-mediated sexual behavior was previously suggested for spinner dolphins (Stenella longirostris; Norris, 1991) and exploratory behaviors such as “genital inspections” have also been reported (Norris and Dohl, 1980; Herzing, 1996). In a behavioral and endocrinological study of three female bottlenose dolphins, that were observed during three conceptive estrous cycles, Muraco and Kuczaj (2015) found that reproductive behaviors increased with estradiol and luteinizing hormone levels. During estrus, females received more behavioral attention than they initiated, including an investigatory behavior (“genital tracking”, as defined by the authors of the corresponding study) and having their genital slit being touched by the rostrum of another dolphin (“goose,” as defined by the authors of the corresponding study). Thus, the authors suggested that dolphins might be able to gain information about the physiological state of another dolphin (e.g., during reproduction) by using chemosensory abilities.
Finally, it seems worth investigating whether some repulsive stimuli are inherent or acquired. Chemical stimuli that are potentially involved in eliciting negative responses might be compounds associated with natural predators (e.g., orcas, a natural predator of dolphins), or intensively irritating, tasting or smelling substances such as capsaicin or putrescine. A possible application could be the development of a device to repel dolphins from fishing nets or areas with high boat traffic by using an effectively repulsive substance, thus minimizing adverse human-dolphin interactions.
Further Questions and Potentially Promising Approaches on Perception in Dolphins
As the previous sections have illustrated, there is a huge amount of knowledge on sensory perception in dolphins. Nevertheless, as always in research, each finding raises new questions that require further experimentation. One promising line of research is cross-modal perception, which describes the interaction between two or more different sensory modalities (i.e., the ability to relate information received from one sense with information obtained from another). Probably the best-studied example of cross-modal perception in dolphins concerns their ability to link auditory and visual cues. Dolphins are able to recognize objects visually that were previously inspected by echolocation and vice versa (e.g., Herman et al., 1998; DeLong et al., 2000; Hoffmann-Kuhnt et al., 2008). Furthermore, dynamic information about movement, in addition to stationary objects, can be perceived across those two senses (Kuczaj et al., 2008).
So far, cross-modal perception in dolphins was only investigated with regards to the interaction between audition and vision within the context of object and movement recognition. However, other senses and contexts should also be investigated. Possible valid questions may concern if dolphins are able to link information in the context of: (1) individual recognition of conspecifics between audition (signature whistles), vision (individually distinctive physical features) and/or chemoreception (chemical profile); (2) communication between audition (e.g., jaw claps, tail hits or “squawks”) and vision (body postures); (3) prey location between audition (returning echo) and electroreception (electric field generated by prey); and (4) food evaluation between audition (returning echo), somatosensory perception (haptic characteristics of prey) and/or chemoreception (flavor characteristics of prey).
Conclusion
Although intensively studied for decades, many facets of dolphin biology remain unknown. Without doubt, this is partly due to the difficulties researchers encounter when studying marine mammals, especially in the field. Generally, hearing is considered to be the most important sensory modality, not only in dolphins but also in odontocetes in general (e.g., Thewissen, 2009), as it is involved in navigation, prey location, and communication. This has led to the majority of studies addressing questions related to hearing, sound production, echolocation and related communicative activities. By contrast, other sensory modalities are considered to be less important (e.g., Marriott et al., 2013) and, therefore, have become physically reduced or may even be absent due to complex trade-offs between different sensory modalities (Nummela et al., 2013). This approach appears biased and runs the risk of distorting knowledge or oversimplifying the degree by which dolphins, and other cetacean, might possess and utilize a potentially diverse array of senses. Therefore, the sensory perception of cetaceans, and in particular the dolphin, should be revisited, especially regarding the study of those modalities that have been largely neglected, namely electroreception, magnetoreception and chemoreception.
Author Contributions
DK, AL, and MH prepared the main text of the manuscript. All authors contributed to the preparation of the article, in the form of discussion and critical comments, and reviewed the manuscript.
Funding
This work was supported by the Centre National de la Recherche Scientifique (Groupement de Recherche 2822), by the Agence Nationale de la Recherche (grant ORILANG to AL) and the Institut Universitaire de France. Furthermore, it was funded by the Association Nationale de la Recherche et de la Technologie (CIFRE grant #2010/0471 to DK).
Conflict of Interest Statement
The authors declare that the research was conducted in the absence of any commercial or financial relationships that could be construed as a potential conflict of interest.
Acknowledgments
We thank Planète Sauvage for their support and Françoise Joubaud for logistic help.
References
Abramson, J. Z., Hernández-Lloreda, V., Call, J., and Colmenares, F. (2013). Experimental evidence for action imitation in killer whales (Orcinus orca). Anim. Cogn. 16, 11–22. doi: 10.1007/s10071-012-0546-2
Amundin, M., Starkhammar, J., Evander, M., Almqvist, M., Lindström, K., and Persson, H. W. (2008). An echolocation visualization and interface system for dolphin research. J. Acoust. Soc. Am. 123, 1188–1194. doi: 10.1121/1.2828213
Au, W. W. L., Popper, A. N., and Fay, R. R. (2000). Hearing by Whales and Dolphins (Springer Handbook of Auditory Research). New York, NY: Springer-Verlag.
Au, W. W. L., and Snyder, K. J. (1980). Long-range target detection in open waters by an echolocating Atlantic bottlenose dolphin (Tursiops truncatus). J. Acoust. Soc. Am. 68, 1077–1084. doi: 10.1121/1.384993
Bailey, H., Senior, B., Simmons, D., Rusin, J., Picken, G., and Thompson, P. M. (2010). Assessing underwater noise levels during pile-driving at an offshore windfarm and its potential effects on marine mammals. Mar. Poll. Bull. 60, 888–897. doi: 10.1016/j.marpolbul.2010.01.003
Baldwin, M. W., Toda, Y., Nakagita, T., O'Connell, M. J., Klasing, K. C., Misaka, T., et al. (2014). Evolution of sweet taste perception in hummingbirds by transformation of the ancestral umami receptor. Science 345, 929–933. doi: 10.1126/science.1255097
Barros, N. B. (1993). Feeding Ecology and Foraging Strategies of Bottlenose Dolphins on the Central East Coast of Florida. Coral Gables: University of Miami, Dissertation.
Barros, N. B., and Wells, R. S. (1998). Prey and feeding patterns of resident bottlenose dolphins (Tursiops truncatus) in Sarasota Bay, Florida. J. Mammal. 79, 1045–1059. doi: 10.2307/1383114
Bauer, G. B., Fuller, M., Perry, A., Dunn, J. R., and Zoeger, J. (1985). “Magnetoreception and biomineralization of magnetite in cetaceans,” in Magnetite Biomineralization and Magnetoreception in Organisms: A New Biomagnetism, eds J. L. Kirschvink, D. S. Jones, and B. J. MacFadden (New York, NY: Plenum Press), 489–507.
Behrmann, G. (1988). The peripheral nerve ends in the tongue of the harbour porpoise Phocoena phocoena (Linne, 1758). Aquat. Mammals 14, 107–112.
Behrmann, G. (1989). The olfactory regions in the nose of the harbour porpoise Phocoena phocoena (Linne, 1758). Aquat. Mammals 15, 130–133.
Bender, C. E., Herzing, D. L., and Bjorklund, D. F. (2009). Evidence of teaching in Atlantic spotted dolphins (Stenella frontalis) by mother dolphins foraging in the presence of their calves. Anim. Cogn. 12, 43–53. doi: 10.1007/s10071-008-0169-9
Berns, G. S., Cook, P. F., Foxley, S., Jbabdi, S., Miller, K. L., and Marino, L. (2015). Diffusion tensor imaging of dolphin brains reveals direct auditory pathway to temporal lobe. Proc. R. Soc. B 282:20151203. doi: 10.1098/rspb.2015.1203
Blois-Heulin, C., Crével, M., Böye, M., and Lemasson, A. (2012). Visual laterality in dolphins: importance of the familiarity of stimuli. BMC Neurosci. 13:9. doi: 10.1186/1471-2202-13-9
Blomqvist, C., and Amundin, M. (2004). An acoustic tag for recording directional pulsed ultrasounds aimed at free-swimming bottlenose dolphins (Tursiops truncatus) by conspecifics. Aquat. Mammals 30, 345–356. doi: 10.1578/AM.30.3.2004.345
Boenninghaus, G. (1903). Das Ohr des Zahnwales, Zugleich ein Beitrag zur Theorie der Schalleitung. Eine Biologische Studie. Jena: Fischer Verlag.
Bojanowski, E. (2002). Vocal Behaviour in Bottlenose Dolphins (Tursiops truncatus): Ontogeny and Contextual Use in Specific Interactions. Berlin: Freie Universität Berlin, Dissertation.
Boucher, Y., Simons, C. T., Faurion, A., Azérad, J., and Carstens, E. (2003). Trigeminal modulation of gustatory neurons in the nucleus of the solitary tract. Brain Res. 973, 265–274. doi: 10.1016/S0006-8993(03)02526-5
Breathnach, A. S. (1960). The cetacean nervous system. Biol. Rev. 35, 187–230. doi: 10.1111/j.1469-185X.1960.tb01414.x
Bruck, J. N. (2013). Decades-long social memory in bottlenose dolphins. Proc. R. Soc. B 280:20131726. doi: 10.1098/rspb.2013.1726
Bryden, M. M., and Molyneux, G. S. (1986). “U1trastrucrure of encapsulated mechanoreceptor organs in the region of the nares,” in Research on Dolphins, eds M. M. Bryden and R. Harrison (Oxford: Clarendon Press), 99–107.
Buckstaff, K. C. (2004). Effects of watercraft noise on the acoustic behaviour of bottlenose dolphins, Tursiops truncatus, in Sarasota Bay, Florida. Mar. Mam. Sci. 20, 709–725. doi: 10.1111/j.1748-7692.2004.tb01189.x
Caldwell, M. C., and Caldwell, D. K. (1967). “Intraspecific transfer of information via the pulsed sound in captive odontocete cetaceans,” in Animal Sonar Systems: Biology and Bionics, ed R. G. Bullock (Jouy-en-Josas: Laboratoire Physiologie Acoustique), 879–936.
Caldwell, M. C., Caldwell, D. K., and Tyack, P. L. (1990). “Review of the signature-whistle-hypothesis for the Atlantic bottlenose dolphin,” in The Bottlenose Dolphin, eds S. Leatherwood and R. R. Reeves (San Diego, CA: Academic Press), 199–234.
Carder, D. A. (1983). Apparent echolocation by a sixty-day-old bottlenosed dolphin, Tursiops truncatus. J. Acoust. Soc. Am. 74, S74. doi: 10.1121/1.2021123
Chien, J.-P. (2006). Of animals and men: a study of Umwelt in Uexküll, Cassirer, and Heidegger. Concentric Lit. Cult. Stud. 32, 57–79.
Connor, R. C. (2007). Dolphin social intelligence: complex alliance relationships in bottlenose dolphins and a consideration of selective environments for extreme brain size evolution in mammals. Philos. Trans. R. Soc. Lond. B 362, 587–602. doi: 10.1098/rstb.2006.1997
Connor, R. C., Wells, R. S., Mann, J., and Read, A. J. (2000). “The bottlenose dolphin: social relationships in a fission-fusion society,” in Cetacean Societies: Field Studies of Dolphins and Whales, eds J. Mann, R. C. Connor, P. L. Tyack, and H. Whitehead (Chicago, IL: University of Chicago Press), 91–126.
Constantine, R., Visser, I., Buurman, D., Buurman, R., and Mfadden, B. (1998). Killer whale (Orcinus orca) predation on dusky dolphins (Lagenorhynchus obscurus) in Kaikoura, New Zealand. Mar. Mam. Sci. 14, 324–330. doi: 10.1111/j.1748-7692.1998.tb00721.x
Cozzi, B., Podestà, M., Vaccaro, C., Poggi, R., Mazzariol, S., Huggenberger, S., et al. (2015). Precocious ossification of the tympanoperiotic bone in fetal and newborn dolphins: an evolutionary adaptation to the aquatic environment? Anat. Rec. 298, 1294–1300. doi: 10.1002/ar.23120
Cranford, T. W., Amundin, M., and Norris, K. S. (1996). Functional morphology and homology in the odontocete nasal complex: implications for sound generation. J. Morphol. 228, 223–285.
Cranford, T. W., Krysl, P., and Amundin, M. (2010). A new acoustic portal into the odontocete ear and vibrational analysis of the tympanoperiotic complex. PLoS ONE 5:e11927. doi: 10.1371/journal.pone.0011927
Curé, C., Antunes, R., Samarra, F., Alves, A. C., Visser, F., Kvadsheim, P. H., et al. (2012). Pilot whales attracted to killer whale sounds: acoustically-mediated interspecific interactions in cetaceans. PLoS ONE 7:e52201. doi: 10.1371/journal.pone.0052201
Czech-Damal, N. U., Dehnhardt, G., Manger, P., and Hanke, W. (2013). Passive electroreception in aquatic mammals. J. Comp. Physiol. A 199, 555–563. doi: 10.1007/s00359-012-0780-8
Czech-Damal, N. U., Liebschner, A., Miersch, L., Klauer, G., Hanke, F. D., Marshall, C., et al. (2012). Electroreception in the Guiana dolphin (Sotalia guianensis). Proc. R. Soc. B 279, 663–668. doi: 10.1098/rspb.2011.1127
Dacey, J. W. H., and Wakeham, S. G. (1986). Oceanic dimethylsulfide: production during zooplankton grazing on phytoplankton. Science 233, 1314–1315. doi: 10.1126/science.233.4770.1314
Davies, W. I. L., Collin, S. P., and Hunt, D. M. (2012). Molecular ecology and adaptation of visual photopigments in craniates. Mol. Ecol. 21, 3121–3158. doi: 10.1111/j.1365-294X.2012.05617.x
Davis, M. W., Spencer, M. L., and Ottmar, M. L. (2006). Behavioral responses to food odor in juvenile marine fish: acuity varies with species and fish length. J. Exp. Mar. Biol. Ecol. 328, 1–9. doi: 10.1016/j.jembe.2005.04.029
Dawson, W. W. (1980). “The cetacean eye,” in Cetacean Behavior: Mechanisms and Functions, ed L. M. Herman (New York, NY: Wiley Interscience), 54–99.
Dawson, W. W., Birndorf, L., and Perez, J. (1972). Gross anatomy and optics of the dolphin eye. Cetology 10, 1–11.
Dawson, W. W., Schroeder, J. P., and Sharpe, S. N. (1987). Corneal surface properties of two marine mammal species. Mar. Mam. Sci. 3, 186–197. doi: 10.1111/j.1748-7692.1987.tb00161.x
DeBose, J. L., Lema, S. C., and Nevitt, G. A. (2008). Dimethylsulfoniopropionate as a foraging cue for reef fishes. Science 319, 1356. doi: 10.1126/science.1151109
Deecke, V. B., Ford, J. K. B., and Slater, P. J. (2005). The vocal behaviour of mammal-eating killer whales: communicating with costly calls. Anim. Behav. 69, 395–405. doi: 10.1016/j.anbehav.2004.04.014
Dehnhardlt, G., Mauck, B., and Bleckmann, H. (1998). Seal whiskers detect water movements. Nature 394, 235–236. doi: 10.1038/28303
Dehnhardt, G., and Mauck, B. (2008). “Mechanoreception in secondarily aquatic vertebrates,” in Sensory Evolution on the Threshold: Adaption in Secondarily Aquatic Mammals, eds J. G. M. Thewissen and S. Nummela (Berkeley; Los Angeles, CA: University of California Press), 295–314.
DeLong, C. M., Au, W. W. L., and Harley, H. E. (2000). Acoustic analysis of objects ensonified by a bottlenose dolphin during a cross−modal matching task. J. Acoust. Soc. Am. 108, 2635. doi: 10.1121/1.4743809
DeLong, C. M., Au, W. W. L., Lemonds, D. W., Harley, H. E., and Roitblat, H. L. (2006). Acoustic features of objects matched by an echolocating bottlenose dolphin. J. Acoust. Soc. Am. 119, 1867–1879. doi: 10.1121/1.2161434
Díaz López, B. (2011). Whistle characteristics in free-ranging bottlenose dolphins (Tursiops truncatus) in the Mediterranean Sea: influence of behaviour. Mamm. Biol. 76, 180–189. doi: 10.1016/j.mambio.2010.06.006
Dormer, K. J. (1979). Mechanism of sound production and air recycling in delphinids: cineradiographic evidence. J. Acoust. Soc. Am. 65, 229–239. doi: 10.1121/1.382240
Drake, S. E., Crish, S. D., George, J. C., Stimmelmayr, R., and Thewissen, J. G. M. (2015). Sensory hairs in the bowhead whale, Balaena mysticetus (Cetacea, Mammalia). Anat. Rec. 298, 1327–1335. doi: 10.1002/ar.23163
Dral, A. D. G. (1977). “On the retinal anatomy of cetacea,” in Functional Anatomy of Marine Mammals, Vol. 3, ed R. J. Harrison (London: Academic Press), 86–87.
Dudzinski, K. M. (1996). Communication and Behavior in the Atlantic Spotted Dolphins (Stenella frontalis): Relationship between Vocal and Behavioral Activities. College Station: Texas A and M University, Dissertation.
Dudzinski, K. M., Gregg, J. D., Paulos, R. D., and Kuczaj, S. A. (2010). A comparison of pectoral fin contact behaviour for three distinct dolphin populations. Behav. Process. 84, 559–567. doi: 10.1016/j.beproc.2010.02.013
Dudzinski, K. M., Gregg, J. D., Ribic, C. A., and Kuczaj, S. A. (2009b). A comparison of pectoral fin contact between two different wild dolphin populations. Behav. Process. 80, 182–190. doi: 10.1016/j.beproc.2008.11.011
Dudzinski, K. M., Melillo-Sweeting, J. G., Melillo-Sweeting, K., Seay, B., Levengood, A., and Kuczaj, S. A. (2012). Tactile contact exchanges between dolphins: self-rubbing versus inter-individual contact in three species from three geographies. Int. J. Comp. Psychol. 25, 21–43.
Dudzinski, K. M., Thomas, J. A., and Gregg, J. D. (2009a). “Communication in marine mammals,” in Encyclopedia of Marine Mammals, 2nd Edn., eds W. F. Perrin, B. Würsig, and J. G. M. Thewissen (Burlington, MA: Academic Press), 260–269.
Esch, H. C., Sayigh, L. S., Blum, J. E., and Wells, R. S. (2009). Whistles as potential indicators of stress in bottlenose dolphins (Tursiops truncatus). J. Mammal. 90, 638–650. doi: 10.1644/08-MAMM-A-069R.1
Fahlke, J. M., Gingerich, P. D., Welsh, R. C., and Wood, A. R. (2011). Cranial asymmetry in Eocene archaeocete whales and the evolution of directional hearing in water. Proc. Natl. Acad. Sci. U.S.A. 108, 14545–14548. doi: 10.1073/pnas.1108927108
Fasick, J. I., Cronin, T. W., Hunt, D. M., and Robinson, P. R. (1998). The visual pigments of the bottlenose dolphin (Tursiops truncatus). Vis. Neurosci. 15, 643–651. doi: 10.1017/S0952523898154056
Feng, P., Zheng, J., Rossiter, S. J., Wang, D., and Zhao, H. (2014). Massive losses of taste receptor genes in toothed and baleen whales. Genome Biol. Evol. 6, 1254–1265. doi: 10.1093/gbe/evu095
Ferrando, T., Caresano, F., Ferrando, S., Gallus, L., Wurty, M., and Tagliafierro, G. (2010). The tongue morphology and lingual gland histochemistry of Ligurian Sea odontocetes. Mar. Mam. Sci. 26, 588–601. doi: 10.1111/j.1748-7692.2010.00383.x
Finneran, J. J., and Schlundt, C. E. (2010). Frequency-dependent and longitudinal changes in noise-induced hearing loss in a bottlenose dolphin (Tursiops truncatus) (L). J. Acoust. Soc. Am. 128, 567–570. doi: 10.1121/1.3458814
Ford, J. K. B. (1984). Call Traditions and Dialects of Killer Whales (Orcinus orca) in British Columbia. Vancouver: University of British Columbia, Dissertation.
Friedl, W. A., Nachtigall, P. E., Moore, P. W. B., Chun, N. K. W., Haun, J. E., Hall, R. W., et al. (1990). “Taste reception in the Pacific bottlenose dolphin (Tursiops truncatus gilli) and the California sea lion (Zalophus californianus),” in Sensory Abilities of Cetaceans, eds J. A. Thomas and R. A. Kastelein (New York, NY: Plenum Press), 447–454.
Gannon, D. P., Barros, N. B., Nowacek, D. P., Read, A. J., Waples, D. M., and Wells, R. S. (2005). Prey detection by bottlenose dolphins (Tursiops truncatus): an experimental test of the passive listening hypothesis. Anim. Behav. 69, 709–720. doi: 10.1016/j.anbehav.2004.06.020
Gatesy, J., Geisler, J. H., Chang, J., Buell, C., Berta, A., Meredith, R. W., et al. (2013). A phylogenetic blueprint for a modern whale. Mol. Phylogenet. Evol. 66, 479–506. doi: 10.1016/j.ympev.2012.10.012
Glezer, I. I., Hof, P. R., Istomin, V. V., and Morgane, P. J. (1995). “Comparative immunocytochemistry of calcium-binding protein-positive neurons in visual and auditory systems of cetaceans and primate brains,” in Sensory Systems of Aquatic Mammals, eds R. A. Kastelein, J. A. Thomas, and P. E. Nachtigall (Woerden: De Spil Publishers), 477–513.
Graf, W. (1988). Motion detection in physical space and its peripheral and central representation. Ann. N.Y. Acad. Sci. 545, 154–169. doi: 10.1111/j.1749-6632.1988.tb19561.x
Gregg, J. D., Dudzinski, K. M., and Smith, H. V. (2007). Do dolphins eavesdrop on the echolocation signals of conspecifics? Int. J. Comp. Psychol. 20, 65–88.
Griebel, U., and Peichl, L. (2003). Colour vision in aquatic mammals – facts and open questions. Aquat. Mammals 29, 18–30. doi: 10.1578/016754203101024040
Griebel, U., and Schmid, A. (2002). Spectral sensitivity and color vision in the bottlenose dolphin (Tursiops truncatus). Mar. Freshwater Behav. Physiol. 35, 129–137. doi: 10.1080/1023624021000014716
Hara, T. J. (1994). Olfaction and gustation in fish: an overview. Acta Physiol. Scand. 152, 207–217. doi: 10.1111/j.1748-1716.1994.tb09800.x
Hara, T. J. (2006). Feeding behaviour in some teleosts is triggered by single amino acids primarily through olfaction. J. Fish Biol. 68, 810–825. doi: 10.1111/j.0022-1112.2006.00967.x
Heithaus, M. R. (2001). Predator-prey and competitive interactions between sharks (order Selachii) and dolphins (suborder Odontoceti): a review. J. Zool. 253, 53–68. doi: 10.1017/S0952836901000061
Hemilä, S., Nummela, S., and Reuter, T. (2010). Anatomy and physics of the exceptional sensitivity of dolphin hearing (Odontoceti: Cetacea). J. Comp. Physiol. A 196, 165–179. doi: 10.1007/s00359-010-0504-x
Hemilä, S., and Reuter, T. (2008). “The physics and biology of olfaction and taste,” in Sensory Evolution on the Threshold: Adaption in Secondarily Aquatic Mammals, eds J. G. M. Thewissen and S. Nummela (Berkeley; Los Angeles, CA: University of California Press), 29–33.
Herman, L. M., Pack, A. A., and Hoffmann-Kuhnt, M. (1998). Seeing through sound: dolphins (Tursiops truncatus) perceive the spatial structure of objects through echolocation. J. Comp. Psychol. 112, 292–305. doi: 10.1037/0735-7036.112.3.292
Herman, L. M., Peacock, M. F., Yunker, M. P., and Madsen, C. J. (1975). Bottlenosed dolphin: double-slit pupil yields equivalent aerial and underwater diurnal acuity. Science 189, 650–652. doi: 10.1126/science.1162351
Herzing, D. L. (1996). Vocalizations and associated underwater behavior of free-ranging Atlantic spotted dolphins, Stenella frontalis, and bottlenose dolphins, Tursiops truncatus. Aquat. Mammals 22, 61–79.
Hirvonen, H., Ranta, E., Piironen, J., Laurila, A., and Peuhkuri, N. (2000). Behavioral response of naïve Artic charr young to chemical cues from salmonid and non-salmonid fish. Oikos 88, 191–199. doi: 10.1034/j.1600-0706.2000.880121.x
Hoffmann-Kuhnt, M., Chitre, M. A., Seekings, P. J., and Abel, G. (2008). Acoustics of shape recognition by a dolphin in a cross-modal matching-to-sample paradigm. J. Acoust. Soc. Am. 123:3361. doi: 10.1121/1.2933955
Houser, D. S., and Finneran, J. J. (2006). A comparison of underwater hearing sensitivity in bottlenose dolphins (Tursiops truncatus) determined by electrophysiological and behavioral methods. J. Acoust. Soc. Am. 120, 1713–1722. doi: 10.1121/1.2229286
Hui, C. A. (1994). Lack of association between magnetic patterns and the distribution of free-ranging dolphins. J. Mammal. 75, 399–405. doi: 10.2307/1382559
Janik, V. M. (2000b). Source levels and estimated active space of bottlenose dolphins (Tursiops truncatus) whistles in the Moray Firth, Scotland. J. Comp. Physiol. A 186, 673–680. doi: 10.1007/s003590000120
Janik, V. M. (2000a). Food-related bray calls in wild bottlenose dolphins (Tursiops truncatus). Proc. R. Soc. B 267, 923–927. doi: 10.1098/rspb.2000.1091
Janik, V. M. (2009). “Acoustic communication in delphinids,” in Advances in the Study of Behaviour, Vol. 40, eds M. Naguib and V. M. Janik (Burlington, MA: Academic Press), 123–157.
Janik, V. M., and Sayigh, L. S. (2013). Communication in bottlenose dolphins: 50 years of signature whistle research. J. Comp. Physiol. A 199, 479–489. doi: 10.1007/s00359-013-0817-7
Jensen, A.-L. M., Delfour, F., and Carter, T. (2013). Anticipatory behavior in captive bottlenose dolphins (Tursiops truncatus): a preliminary study. Zoo Biol. 32, 436–444. doi: 10.1002/zoo.21077
Jepson, P. D., Arbelo, M., Deaville, R., Patterson, I. A. P., Castro, P., Baker, J. R., et al. (2003). Gas-bubble lesions in stranded cetaceans. Nature 425, 575–576. doi: 10.1038/425575a
Jiang, P., Josue, J., Li, X., Glaser, D., Li, W., Brand, J. G., et al. (2013). Major taste loss in carnivorous mammals. Proc. Natl. Acad. Sci. U.S.A. 109, 4956–4961. doi: 10.1073/pnas.1118360109
Johnson, M. P., and Tyack, P. L. (2003). A digital acoustic recording tag for measuring the response of wild marine mammals to sound. IEEE J. Oceanic Eng. 28, 3–12. doi: 10.1109/JOE.2002.808212
Karenina, K., Giljov, A., Ivkovich, T., and Malashichev, Y. (2016). Evidence for the perceptual origin of right-sided feeding biases in cetaceans. Anim. Cogn. 19, 239–243. doi: 10.1007/s10071-015-0899-4
Kastelein, R. A., Hagedoorn, M., Au, W. W. L., and De Haan, D. (2003). Audiogram of a striped dolphin (Stenella coeruleoalba). J. Acoust. Soc. Am. 113, 1130–1141. doi: 10.1121/1.1532310
Kastelein, R., and Dubbeldam, J. L. (1990). Marginal papillae on the tongue of the harbour porpoise (Phocoena phocoena), bottlenose dolphin (Tursiops truncatus) and Commerson's dolphin (Cephalorhynchus commersonii). Aquat. Mammals 15, 158–170.
Khan, I., Yang, Z., Maldonado, E., Li, C., Zhang, G., Gilbert, M. T. P., et al. (2015). Olfactory receptor subgenomes linked with broad ecological adaptations in Sauropsida. Mol. Biol. Evol. 32, 2832–2843. doi: 10.1093/molbev/msv155
Kienle, S. S., Ekdale, E. G., Reidenberg, J. S., and Deméré, T. A. (2015). Tongue and hyoid musculature and functional morphology of a neonate gray whale (cetacea, mysticeti, Eschrichtius robustus). Anat. Rec. 298, 660–674. doi: 10.1002/ar.23107
King, S. L., and Janik, V. M. (2013). Bottlenose dolphins can use learned vocal labels to address each other. Proc. Natl. Acad. Sci. U.S.A. 110, 13216–13221. doi: 10.1073/pnas.1304459110
Kirschvink, J. L., Dizon, A. E., and Westphal, J. A. (1986). Evidence from strandings for geomagnetic sensitivity in cetaceans. J. Exp. Biol. 120, 1–24.
Kishida, T., Kubota, S., Shirayama, Y., and Fukami, H. (2007). The olfactory receptor gene repertoire in secondary-adapted marine vertebrates: evidence for reduction of the functional proportion in cetaceans. Biol. Lett. 3, 428–430. doi: 10.1098/rsbl.2007.0191
Kishida, T., Thewissen, J. G. M., Hayakawa, T., Imai, H., and Agata, K. (2015b). Aquatic adaptation and the evolution of smell and taste in whales. Zool. Lett. 1, 9. doi: 10.1186/s40851-014-0002-z
Kishida, T., Thewissen, J. G. M., Usip, S., Suydam, R. S., and George, J. C. (2015a). Organization and distribution of glomeruli in the bowhead whale olfactory bulb. Peer J. 28:e897. doi: 10.7717/peerj.897
Klinowska, L. (1985). Cetacean live stranding sites relate to geomagnetic topography. Aquat. Mammals 1, 27–32.
Kolchin, S. P., and Bel'kovich, V. M. (1973). Tactile sensitivity in Delphinus delphis. Zoologichesky ZhurnaI 52, 620–622.
Kowalewsky, S., Dambach, M., Mauck, B., and Dehnhardt, G. (2006). High olfactory sensitivity for dimethyl sulphide in harbour seals. Biol. Lett. 2, 106–109. doi: 10.1098/rsbl.2005.0380
Kremers, D., López Marulanda, J., Hausberger, M., and Lemasson, A. (2014). Behavioural evidence of magnetoreception in dolphins: detection of experimental magnetic fields. Naturwissenschaften 101, 907–911. doi: 10.1007/s00114-014-1231-x
Kremers, D., Célérier, A., Schaal, B., Campagna, S., Trabalon, M., Böye, M., et al. (2016). Sensory perception in cetaceans: Part II — Promising experimental approaches to study chemoreception in dolphins. Front. Ecol. Evol. 4:50. doi: 10.3389/fevo.2016.00050
Kuczaj, S. A., Makecha, R. N., Trone, M., Paulos, R. D., and Ramos, J. A. (2006). The role of peers in cultural transmission and cultural innovation: evidence from dolphin calves. Int. J. Comp. Psychol. 19, 223–240.
Kuczaj, S., Solangi, M., Hoffland, T., and Romagnoli, M. (2008). Recognition and discrimination of human actions across the senses of echolocation and vision in the bottlenose dolphin: evidence for dolphin cross-modal integration of dynamic information. Int. J. Comp. Psychol. 21, 84–95.
Kuznetsov, V. B. (1999). Vegetative reactions of dolphins to a change in the permanent magnetic field. Biofizika 44, 496–502 [article in Russian, only abstract available in English].
Kuznetzov, V. B. (1990). “Chemical sense of dolphins: quasi-olfaction,” in Sensory Abilities of Cetaceans, eds J. A. Thomas and R. A. Kastelein (New York, NY: Plenum Press), 481–503.
Lammers, M. O., and Au, W. W. L. (2003). Directionality in the whistles of Hawaiian spinner dolphins (Stenella longirostris): a signal feature to cue direction of movement? Mar. Mam. Sci. 19, 249–264. doi: 10.1111/j.1748-7692.2003.tb01107.x
Langworthy, O. R. (1932). A description of the central nervous system of the porpoise (Tursiops truncatus). J. Comp. Neurol. 54, 437–499. doi: 10.1002/cne.900540204
Lende, R. A., and Welke, W. I. (1972). An unusual sensory area in the cerebral neocortex of the bottlenose dolphin, Tursiops truncatus. Brain Res. 45, 555–560. doi: 10.1016/0006-8993(72)90482-9
Lewis, J. S., and Schroeder, W. W. (2003). Mud plume feeding, a unique foraging behavior of the bottlenose dolphin in the Florida Keys. Gulf Mexico Sci. 21, 92–97.
Li, S., Nachtigall, P. E., and Breese, M. (2011). Dolphin hearing during echolocation: evoked potential responses in an Atlantic bottlenose dolphin (Tursiops truncatus). J. Exp. Biol. 214, 2027–2035. doi: 10.1242/jeb.053397
Ling, J. K. (1977). “Vibrissae of marine mammals,” in Functional Anatomy of Marine Mammals, Vol. 3, ed R. J. Harrison (London: Academic Press), 387–415.
Lohmann, K. J., and Johnsen, S. (2000). The neurobiology of magnetoreception in vertebrate animals. Trends Neurosci. 23, 153–159. doi: 10.1016/S0166-2236(99)01542-8
Lyamin, O. I., Manger, P. R., Ridgway, S. H., Mukhametov, L. M., and Siegel, J. M. (2008). Cetacean sleep: an unusual form of mammalian sleep. Neurosci. Biobehav. Rev. 32, 1451–1484. doi: 10.1016/j.neubiorev.2008.05.023
Madsen, P. T., and Herman, L. M. (1980). “Social and ecological correlates of vision and visual appearance,” in Cetacean Behavior: Mechanisms and Functions, ed L. M. Herman (New York, NY: Wiley Interscience), 101–147.
Madsen, P. T., and Surlykke, A. (2013). Functional convergence in bat and toothed whale biosonar. Physiology 28, 276–283. doi: 10.1152/physiol.00008.2013
Mann, D., Hill-Cook, M., Manire, C., Greenhow, D., Montie, E., Powell, J., et al. (2010). Hearing loss in stranded odontocete dolphins and whales. PLoS ONE 5:e13824. doi: 10.1371/journal.pone.0013824
Marriott, S., Cowan, E., Cohen, J., and Hallock, R. M. (2013). Somatosensation, echolocation, and underwater sniffing: adaptions allow mammals without traditional olfactory capabilities to forage for food underwater. Zool. Sci. 30, 69–75. doi: 10.2108/zsj.30.69
Mass, A. M., and Supin, A. Y. (1995). “Retinal resolution in the bottlenose dolphin (Tursiops truncatus),” in Sensory Systems of Aquatic Mammals, eds R. A. Kastelein, J. A. Thomas, and P. E. Nachtigall (Woerden: De Spil Publishers), 419–427.
Mass, A. M., and Supin, A. Y. (2009). “Vision” in Encyclopedia of Marine Mammals, 2nd Edn., eds W. F. Perrin, B. Würsig, and J. G. M. Thewissen (Burlington, MA: Academic Press), 1200–1211.
May-Collado, L. J., and Wartzok, D. (2008). A comparison of bottlenose dolphin whistles in the Atlantic Ocean: factors promoting whistle variation. J. Mammal. 89, 1229–1240. doi: 10.1644/07-MAMM-A-310.1
Meredith, R. W., Gatesy, J., Emerling, C. A., York, V. M., and Springer, M. S. (2013). Rod monochromacy and the coevolution of cetacean retinal opsins. PLoS Genet. 9:e1003432. doi: 10.1371/journal.pgen.1003432
Mobley, J. R., and Helweg, D. A. (1990). “Visual ecology and cognition in cetaceans,” in Sensory Abilities of Cetaceans, eds J. A. Thomas and R. A. Kastelein (New York, NY: Plenum Press), 519–536.
Møhl, B., Au, W. W., Pawloski, J., and Nachtigall, P. E. (1999). Dolphin hearing: relative sensitivity as a function of point of application of a contact sound source in the jaw and head region. J. Acoust. Soc. Am. 105, 3421–3424. doi: 10.1121/1.426959
Mollo, E., Fontana, A., Roussis, V., Polese, G., Amodeo, P., and Ghiselin, M. T. (2014). Sensing marine biomolecules: smell, taste, and the evolutionary transition from aquatic to terrestrial life. Front. Chem. 2:92. doi: 10.3389/fchem.2014.00092
Mooney, T. A., Nachtigall, P. E., Taylor, K. A., Rasmussen, M. H., and Miller, L. A. (2009b). Auditory temporal resolution of a wild white-beaked dolphin (Lagenorhynchus albirostris). J. Comp. Physiol. A 195, 375–384. doi: 10.1007/s00359-009-0415-x
Mooney, T. A., Nachtigall, P. E., and Vlachos, S. (2009a). Sonar-induced temporary hearing loss in dolphins. Biol. Lett. 5, 565–567. doi: 10.1098/rsbl.2009.0099
Mooney, T. A., Yang, W.-C., Yu, H.-Y., Ketten, D. R., and Jen, I.-F. (2015). Hearing abilities and sound reception of broadband sounds in an adult Risso's dolphin (Grampus griseus). J. Comp. Physiol. A 201, 751–761. doi: 10.1007/s00359-015-1011-x
Morgane, P. J., and Jacobs, M. S. (1972). “Comparative anatomy of the cetacean nervous system,” in Functional Anatomy of Marine Mammals, ed R. J. Harrison (London: Academic Press), 117–244.
Morisaka, T., and Connor, R. C. (2007). Predation by killer whales (Orcinus orca) and the evolution of whistle loss and narrow-band high frequency clicks in odontocetes. J. Evol. Biol. 20, 1439–1458. doi: 10.1111/j.1420-9101.2007.01336.x
Muraco, H., and Kuczaj, S. A. I. I. (2015). Conceptive estrus behavior in three bottlenose dolphins (Tursiops truncatus). Anim. Behav. Cogn. 2, 30–48. doi: 10.12966/abc.02.03.2015
Murray, R. W. (1960). Electrical sensitivity of the ampullae of Lorenzini. Nature 187, 957. doi: 10.1038/187957a0
Nachtigall, P. E., and Hall, R. W. (1984). Taste reception in the bottlenose dolphin. Acta Zool. Fennica 172, 147–148.
Nachtigall, P. E., and Supin, A. Y. (2008). A false killer whale adjusts its hearing when it echolocates. J. Exp. Biol. 211, 1714–1718. doi: 10.1242/jeb.013862
Nevitt, G. A. (1991). Do fish sniff? A new mechanism of olfactory sampling in pleuronectid flounders. J. Exp. Biol. 157, 1–18.
Nevitt, G. A., Veit, R. R., and Kareiva, P. (1995). Dimethyl sulphide as a foraging cue for Antarctic procellariiform seabirds. Nature 376, 680–682. doi: 10.1038/376680ao
Norris, K. S. (1991). Dolphin Days: The Life and Times of the Spinner Dolphin. New York: W. W. Norton.
Norris, K. S., and Dohl, T. P. (1980). Behavior of the Hawaiian spinner dolphin, Stenella longirostris. Fish. Bull. 77, 821–849.
Nummela, S., Pihlström, H., Puolamäki, K., Fortelius, M., Hemilä, S., and Reuter, T. (2013). Exploring the mammalian sensory space: co-operations and trade-offs among senses. J. Comp. Physiol. A 199, 1077–1092. doi: 10.1007/s00359-013-0846-2
Nummela, S., and Thewissen, J. G. M. (2008). “The physics of sound in air and water,” in Sensory Evolution on the Threshold: Adaption in Secondarily Aquatic Mammals, eds J. G. M. Thewissen and S. Nummela (Berkeley; Los Angeles, CA: University of California Press), 175–182.
Oelschläger, H. H. A. (2008). The dolphin brain – a challenge for synthetic neurobiology. Brain Res. Bull. 75, 450–459. doi: 10.1016/j.brainresbull.2007.10.051
Oelschläger, H. H. A., and Buhl, E. H. (1985). Development and rudimentation of the peripheral olfactory system in the harbor porpoise, Phocoena phocoena (Mammalia: Cetacea). J. Morphol. 184, 351–360. doi: 10.1002/jmor.1051840309
Oelschläger, H. H. A., and Oelschläger, J. S. (2009). “Brain,” in Encyclopedia of Marine Mammals, 2nd edn., eds W. F. Perrin, B. Würsig, and J. G. M. Thewissen (Burlington, MA: Academic Press), 134–149.
Paulos, R. D., Dudzinski, K., and Kuczaj, S. A. (2008). The role of touch in select social interactions of Atlantic spotted dolphin (Stenella frontalis) and Indo-Pacific bottlenose dolphin (Tursiops aduncus). J. Ethol. 26, 153–164. doi: 10.1007/s10164-007-0047-y
Peichl, L., Behrmann, G., and Kroger, R. H. H. (2001). For whales and seals the ocean is not blue: a visual pigment loss in marine mammals. Eur. J. Neurosci. 13, 1–10. doi: 10.1046/j.0953-816x.2001.01533.x
Perez, J. M., Dawson, W. W., and Landau, D. (1972). Retinal anatomy of the bottlenose dolphin (Tursiops truncatus). Cetology 11, 1–11.
Pfeiffer, D. C., Wang, A., Nicolas, J., and Pfeiffer, C. J. (2001). Lingual ultrastructure of the long-finned pilot whale (Globicephala melas). Anat Histol. Embryol. 30, 359–365. doi: 10.1046/j.1439-0264.2001.00352.x
Piantadosi, C. A., and Thalmann, E. D. (2004). Pathology: whales, sonar and decompression sickness. Nature 428, 716–717. doi: 10.1038/nature02527a
Pihlström, H., Fortelius, M., Hemilä, S., Forsman, R., and Reuter, T. (2005). Scaling of mammalian ethmoid bones can predict olfactory organ size and performance. Proc. R. Soc. B 272, 957–962. doi: 10.1098/rspb.2004.2993
Pilleri, G., and Gihr, M. (1970). The central nervous system of the Mysticete and Odontocete whales. Invest. Cetacea 2, 890–128.
Popov, V. V., Ladygina, T. F., and Supin, A. Y. (1986). Evoked potentials of the auditory cortex of the porpoise, Phocoena phocoena. J. Comp. Physiol. A 158, 705–711. doi: 10.1007/BF00603828
Purves, D., Augustine, G. J., Fitzpatrick, D., Katz, L. C., LaMantia, S., McNamara, J. O., et al. (2001). Neuroscience, 2nd Edn. Sunderland: Sinauer Ass.
Quintana-Rizzo, E., Mann, D. A., and Wells, R. S. (2006). Estimated communication range of social sounds used by bottlenose dolphins (Tursiops truncatus). J. Acoust. Soc. Am. 120, 1671–1683. doi: 10.1121/1.2226559
Rabbitt, R. D., Damiano, E. R., and Wallace Grant, J. (2004). “Biomechanics of the semicircular canals and otolith organs,” in The Vestibular System, eds S. M. Highstein, R. R. Fay, and A. N. Popper (New York, NY: Springer-Verlag), 153–201.
Ralston, J. V., and Herman, L. M. (1995). Perception and generalization of frequency contours by a bottlenose dolphin (Tursiops truncatus). J. Comp. Psychol. 109, 268–277. doi: 10.1037/0735-7036.109.3.268
Ridgway, S. H., and Carder, D. A. (1990). “Tactile sensitivity, somatosensory responses, skin vibrations, and the skin surface ridges of the bottlenose dolphin, Tursiops truncatus,” in Sensory Abilities of Cetaceans, eds J. A. Thomas, and R. A. Kastelein (New York, NY: Plenum Press), 163–179.
Ridgway, S. H., and Carder, D. A. (1993). Features of dolphin skin with potential hydrodynamic importance. Eng. Med. Biol. Mag., IEEE 12, 83–88. doi: 10.1109/51.232347
Ridgway, S. H., Elsberry, W. R., Blackwood, D. J., Kamolnick, T., Todd, M., Carder, D. A., et al. (2012). Vocal reporting of echolocation targets: dolphins often report before click trains end. J. Acoust. Soc. Am. 131, 593–598. doi: 10.1121/1.3664074
Ridgway, S., Samuelson, D., Van Alstyne, K., and Price, D. (2015). On doing two things at once: dolphin brain and nose coordinate sonar clicks, buzzes, and emotional squeals with social sounds during fish capture. J. Exp. Biol. 218, 3987–3995. doi: 10.1242/jeb.130559
Rivamonte, L. A. (2009). Bottlenose dolphin (Tursiops truncatus) double-slit pupil asymmetries enhance vision. Aquat. Mammals 35, 269–280. doi: 10.1578/AM.35.2.2009.269
Roitblat, H. L., Au, W. W. L., Nachtigall, P. E., Shizumura, R., and Moons, G. (1995). Sonar recognition of targets embedded in sediment. Neural Net. 8, 1263–1273. doi: 10.1016/0893-6080(95)00052-6
Rossbach, K. A., and Herzing, D. L. (1997). Underwater observations of benthic-feeding bottlenose dolphins (Tursiops truncatus) near Grand Bahama Island, Bahamas. Mar. Mam. Sci. 13, 498–504. doi: 10.1111/j.1748-7692.1997.tb00658.x
Rossi-Santos, M. R., and Wedekin, L. L. (2006). Evidence of bottom contact behavior by estuarine dolphins (Sotalia guianensis) on the eastern coast of Brazil. Aquat. Mammals 32, 140–144. doi: 10.1578/AM.32.2.2006.140
Sakai, M., Hishii, T., Takeda, S., and Kohshima, S. (2006). Flipper rubbing behaviors in wild bottlenose dolphins (Tursiops aduncus). Mar. Mam. Sci. 22, 966–978. doi: 10.1111/j.1748-7692.2006.00082.x
Sayigh, L. S., Tyack, P. L., Wells, R. S., Solow, A. R., Scott, M. D., and Irvine, A. B. (1998). Individual recognition in wild bottlenose dolphins: a field test using playback experiments. Anim. Behav. 57, 41–50. doi: 10.1006/anbe.1998.0961
Schaal, B., and Orgeur, P. (1992). Olfaction in utero: can the rodent model be generalized? Quart. J. Exp. Psychol. 44B, 245–278.
Schultz, K. W., Cato, D. H., Corkeron, P. J., and Bryden, M. M. (1995). Low frequency narrow-band sounds produced by bottlenose dolphins. Mar. Mam. Sci. 11, 503–509. doi: 10.1111/j.1748-7692.1995.tb00673.x
Schultz, K. W., and Corkeron, P. J. (1994). Interspecific differences in whistles produced by inshore dolphins in Moreton Bay, Queensland, Australia. Can. J. Zool. 72, 1061–1068. doi: 10.1139/z94-143
Shoemaker, P. A., and Ridgway, S. H. (1991). Cutaneous ridges in odontocetes. Mar. Mam. Sci. 7, 66–74. doi: 10.1111/j.1748-7692.1991.tb00551.x
Silber, G. K., and Fertl, D. (1995). Intentional beaching by bottlenose dolphins (Tursiops truncatus) in the Colorado River Delta, Mexico. Aquat. Mammals 21, 183–186.
Silver, W. L., and Finger, T. E. (2009). The anatomical and electrophysiological basis of peripheral nasal trigeminal chemoreception. Ann. N.Y. Acad. Sci. 1170, 202–205. doi: 10.1111/j.1749-6632.2009.03894.x
Simons, D. (1977). Analysis of an experiment on colour vision in dolphins. Aquatic Mammals 5, 27–33.
Sipla, J. S., and Spoor, F. (2008). “The physics and physiology of balance,” in Sensory Evolution on the Threshold: Adaption in Secondarily Aquatic Mammals, eds J. G. M. Thewissen and S. Nummela (Berkeley; Los Angeles, CA: University of California Press), 227–232.
Smith, T. G., Aubin, D. J. S., and Hammill, M. O. (1992). Rubbing behaviour of belugas, Delphinapterus leucas, in a high Arctic estuary. Can. J. Zool. 70, 2405–2409. doi: 10.1139/z92-322
Solntseva, G. N. (2001). Comparative analysis of vestibular system development in various groups of mammals living under different environmental conditions. Russian J. Dev. Biol. 32, 171–174. doi: 10.1023/A:1016659521966
Souza, M. L. S., Freitas, C. F., Domingos, M.-A. O., Nunes-Tavares, N., Hasson-Voloch, A., Nasciutti, L. E., et al. (2007). Identification and distribution of chondroitin sulfate in the three electric organs of the electric eel, Electrophorus electricus (L.). Comp. Biochem. Physiol. B 146, 227–233. doi: 10.1016/j.cbpb.2006.10.107
Spitz, J., Mourocq, E., Leauté, J.-P., Quéro, J.-C., and Ridoux, V. (2010). Prey selection by the common dolphin: fulfilling high energy requirements with high quality food. J. Exp. Marine Biol. Ecol. 390, 73–77. doi: 10.1016/j.jembe.2010.05.010
Spoor, F., Bajpai, S., Hussain, S. T., Kumar, K., and Thewissen, J. G. M. (2002). Vestibular evidence for the evolution of aquatic behavior in early cetaceans. Nature 417, 163–166. doi: 10.1038/417163a
Spoor, F., and Thewissen, J. G. M. (2008). “Comparative and functional anatomy of balance in aquatic mammals,” in Sensory Evolution on the Threshold: Adaption in Secondarily Aquatic Mammals, eds J. G. M. Thewissen and S. Nummela (Berkeley; Los Angeles, CA: University of California Press), 257–284.
Supin, A. Y., and Nachtigall, P. E. (2013). Gain control in the sonar of odontocetes. J. Comp. Physiol. A 199, 471–478. doi: 10.1007/s00359-012-0773-7
Supin, A. Y., Popov, V. V., and Mass, A. M. (2001). The Sensory Physiology of Aquatic Mammals. New York, NY: Springer-Verlag. doi: 10.1007/978-1-4615-1647-7
Tamaki, N., Morisaka, T., and Taki, M. (2006). Does body contact contribute towards repairing relationships? The association between flipper-rubbing and aggressive behavior in captive bottlenose dolphins. Behav. Process. 73, 209–215. doi: 10.1016/j.beproc.2006.05.010
Theiss, S. M., Davies, W. I. L., Collin, S. P., Hunt, D. M., and Hart, N. S. (2012). Cone monochromacy and visual pigment spectral tuning in wobbegong sharks. Biol. Lett. 8, 1019–1022. doi: 10.1098/rsbl.2012.0663
Thewissen, J. G. M. (2009). “Sensory biology: overview,” in Encyclopedia of Marine Mammals, 2nd Edn., eds W. F. Perrin, B. Würsig, and J. G. M. Thewissen (Burlington, MA: Academic Press), 1003–1005.
Thewissen, J. G. M., Cooper, L. N., George, J. C., and Bajpai, S. (2009). From land to water: the origin of whales, dolphins, and porpoises. Evol. Educ. Outreach 2, 272–288. doi: 10.1007/s12052-009-0135-2
Thewissen, J. G. M., George, J., Rosa, C., and Kishida, T. (2011). Olfaction and brain size in the bowhead whale (Balaena mysticetus). Mar. Mam. Sci. 27, 282–294. doi: 10.1111/j.1748-7692.2010.00406.x
Thieltges, H., Lemasson, A., Kuczaj, S., Böye, M., and Blois-Heulin, C. (2011). Visual laterality in dolphins when looking at (un)familiar humans. Anim. Cogn. 14, 303–308. doi: 10.1007/s10071-010-0354-5
Thompson, R. K. R., and Herman, L. M. (1975). Underwater frequency discrimination in the bottlenosed dolphin (1-140 khz) and the human (1-8 khz). J. Acoust. Soc. Am. 57, 943–948. doi: 10.1121/1.380513
Tomonaga, M., Uwano, Y., and Saito, T. (2014). How dolphins see the world: a comparison with chimpanzees and humans. Sci. Rep. 4:3717. doi: 10.1038/srep03717
Tyack, P. L. (2000). “Functional aspects of cetacean communication,” in Cetacean Societies: Field Studies of Dolphins and Whales, eds J. Mann, R. C. Connor, P. L. Tyack, and H. Whitehead (Chicago, IL: The University of Chicago Press), 270–307.
Tyack, P. L., Zimmer, W. M. X., Moretti, D., Southall, B. L., Claridge, D. E., Durban, J. W., et al. (2011). Beaked whales respond to simulated and actual navy sonar. PLoS ONE 6:e17009. doi: 10.1371/journal.pone.0017009
Varanasi, U., and Malins, D. C. (1971). Unique lipids of the porpoise Tursiops gilli: differences in triacyl glycerols and wax esters of acoustic (mandibular canal and melon) and blubber tissues. Biochem. et Biophys. Acta: Lipids Lipid Metab. 231, 415–418. doi: 10.1016/0005-2760(71)90158-5
von Uexküll, J. (1934). Streifzüge durch die Umwelten von Tieren und Menschen: ein Bilderbuch unsichtbarer Welten. Berlin: Springer-Verlag.
Walker, M. M., and Dennis, T. E. (2005). Role of the magnetic sense in the distribution and abundance of marine animals. Mar. Ecol. Prog. Ser. 287, 295–307.
Walker, M. M., Kirschvink, J. L., Ahmed, G., and Diction, A. E. (1992). Evidence that fin whales respond to the geomagnetic field during migration. J. Exp. Biol. 171, 67–78.
Wartzok, D., and Ketten, D. R. (1999). “Marine mammal sensory systems,” in Biology of Marine Mammals, eds J. Reynolds and S. Rommel (Washington, DC: Smithsonian Institution Press), 117–175.
Watwood, S. L., Owen, E. C. G., Tyack, P. L., and Wells, R. S. (2005). Signature whistle use by temporarily restrained and free-swimming bottlenose dolphins, Tursiops truncatus. Anim. Behav. 69, 1373–1386. doi: 10.1016/j.anbehav.2004.08.019
Wells, R. S., Scott, M. D., and Irvine, A. B. (1987). “The social structure of free-ranging bottlenose dolphins” in Current Mammalogy, Vol. 1, ed H. Genoways (New York, NY: Plenum Press), 247–305.
Werth, A. J. (2007). Adaptions of the cetacean hyolingual apparatus for aquatic feeding and thermoregulation. Anat. Rec. 290, 546–568. doi: 10.1002/ar.20538
Whitehead, H., Rendell, L., Osborne, R. W., and Würsig, B. (2004). Culture and conservation of non-humans with reference to whales and dolphins: review and new directions. Biol. Cons. 118, 205–218. doi: 10.1016/j.biocon.2004.03.017
Whitehead, H., and Weilgart, L. (1991). Patterns of visually observable behaviour and vocalizations in groups of female sperm whales. Behaviour 118, 275–296. doi: 10.1163/156853991X00328
Winklhofer, M. (2010). Magnetoreception. J. R. Soc. Interface 7, S131–S134. doi: 10.1098/rsif.2010.0010.focus
Wright, K. L. B., Pichegru, L., and Ryan, P. G. (2011). Penguins are attracted to dimethyl sulphide at sea. J. Exp. Biol. 214, 2509–2511. doi: 10.1242/jeb.058230
Xitco, M. J., Groy, J. D., and Kuczaj, S. A. (2001). Spontaneous pointing by bottlenose dolphins (Tursiops truncatus). Anim. Cogn. 4, 115–123. doi: 10.1007/s100710100107
Xitco, M. J., and Roitblat, H. L. (1996). Object recognition through eavesdropping: passive echolocation in bottlenose dolphins. Anim. Learn. Behav. 24, 355–365. doi: 10.3758/BF03199007
Yamasaki, F., Komatsu, S., and Kamiya, T. (1978). Taste buds in the pits at the posterior dorsum of the tongue of Stenella coeruleoalba. Sci. Rep. Whales Res. Instit. 30, 285–290.
Yoshida, Y. M., Morisaka, T., Sakai, M., Iwasaki, M., Wakabayashi, I., Seko, A., et al. (2014). Sound variation and function in captive Commerson's dolphins (Cephalorhynchus commersonii). Behav. Process. 108, 11–19. doi: 10.1016/j.beproc.2014.08.017
Yuen, M. M. L., Nachtigall, P. E., Breese, M., and Vlachos, S. A. (2007). The perception of complex tones by a false killer whale (Pseudorca crassidens). J. Acoust. Soc. Am. 121, 1768–1774. doi: 10.1121/1.2436640
Keywords: cetaceans, Delphinidae, Tursiops truncatus, audition, vision, electroreception, magnetoreception, chemoreception
Citation: Kremers D, Célérier A, Schaal B, Campagna S, Trabalon M, Böye M, Hausberger M and Lemasson A (2016) Sensory Perception in Cetaceans: Part I—Current Knowledge about Dolphin Senses As a Representative Species. Front. Ecol. Evol. 4:49. doi: 10.3389/fevo.2016.00049
Received: 04 January 2016; Accepted: 24 April 2016;
Published: 11 May 2016.
Edited by:
Wayne Iwan Lee Davies, University of Western Australia, AustraliaReviewed by:
Paul Manger, University of the Witwatersrand, South AfricaTakushi Kishida, Kyoto University, Japan
Copyright © 2016 Kremers, Célérier, Schaal, Campagna, Trabalon, Böye, Hausberger and Lemasson. This is an open-access article distributed under the terms of the Creative Commons Attribution License (CC BY). The use, distribution or reproduction in other forums is permitted, provided the original author(s) or licensor are credited and that the original publication in this journal is cited, in accordance with accepted academic practice. No use, distribution or reproduction is permitted which does not comply with these terms.
*Correspondence: Alban Lemasson, YWxiYW4ubGVtYXNzb25AdW5pdi1yZW5uZXMxLmZy