- 1Centre for Ecology and Conservation, College of Life and Environmental Sciences, University of Exeter, Cornwall, UK
- 2Hawkesbury Institute for the Environment, Western Sydney University, Penrith, NSW, Australia
- 3School of Natural Sciences and Psychology, Liverpool John Moores University, Liverpool, UK
- 4School of Life, Sport and Social Sciences, Edinburgh Napier University, Edinburgh, UK
- 5Department of Zoology, University of Cambridge, Cambridge, UK
Oxidative damage has been proposed as a potential mechanism underlying a life history tradeoff between survival and reproduction. However, evidence that reproduction is associated with increased oxidative damage is equivocal, and some studies have found that breeding females exhibit reduced, rather than elevated, levels of oxidative damage compared to equivalent non-breeders. Recently it was hypothesized that oxidative damage could have negative impacts on developing offspring, and that mothers might down-regulate oxidative damage during reproduction to shield their offspring from such damage. We tested this hypothesis through a longitudinal study of adult survival, reproduction, and oxidative damage in wild banded mongooses (Mungos mungo) in Uganda. High levels of oxidative damage as measured by malondialdehyde (MDA) were associated with reduced survival in both sexes. Levels of protein carbonyls were not linked to survival. Mothers showed reduced levels of MDA during pregnancy, and individuals with higher MDA levels gestated fewer offspring and had lower pup survival. These results suggest that maternal oxidative damage has transgenerational costs, and are consistent with the idea that mothers may attempt to shield their offspring from particularly harmful types of oxidative damage during pregnancy. We suggest that further advance in understanding of life history variation could benefit from theoretical and empirical exploration of the potential transgenerational costs of reproduction.
Introduction
A tenet of life history theory is the idea that investment in reproduction is costly to survival (Kirkwood and Rose, 1991; Hammers et al., 2013; Moore et al., 2013). Reproductive effort is inversely related to lifespan across taxa (Kirkwood and Rose, 1991), and within species (Hammers et al., 2013), but the mechanisms proposed to underlie this trade-off are controversial. One leading hypothesis (the oxidative stress theory of aging; OSTA) suggests that energy expensive activities, such as reproduction, can result in oxidative damage to cells and tissues, thereby reducing somatic function and survival (Harman, 1956; Beckman and Ames, 1998; Selman et al., 2012). Evidence that reproduction increases oxidative damage is equivocal, however (Speakman and Selman, 2011; Selman et al., 2012; Metcalfe and Monaghan, 2013; Speakman and Garratt, 2013). A recent meta-analysis of studies of female birds and mammals showed that there is a positive association between reproductive effort (i.e., offspring number) and oxidative damage, while paradoxically, levels of oxidative damage in certain tissues are lower in breeders compared to non-breeders of similar age and condition—the opposite pattern to that expected under OSTA (Blount et al., 2015).
We recently hypothesized that previous findings concerning the relationship between reproduction and oxidative damage may be reconciled if maternal oxidative damage levels have transgenerational impacts on offspring fitness. Specifically, where developing offspring are sensitive to maternal oxidative damage (for example, via impaired placental function or reduced milk yield; Gupta et al., 2007; Al-Gubory et al., 2010) breeding females may gain from pre-emptive reduction of oxidative damage to shield their offspring from oxidative insults (Blount et al., 2015). The predicted relationship between reproductive effort and oxidative damage will then depend on the balance between maternal and transgenerational impacts of oxidative damage, and the degree to which mothers are selected to shield their offspring from harm. In the Supplementary Material we use a simple optimization model of oxidative defense given transgenerational costs to make explicit this verbal argument. The model shows that mothers may be selected to reduce oxidative damage during pregnancy at the cost of damage levels during non-breeding periods; and that mothers with higher levels of damage during reproductive periods should produce smaller litters and fewer surviving young (Supplementary Figure S1).
We used data from a long-term study of wild banded mongooses in Uganda to test (1) whether markers of oxidative damage and oxidative defense predicted variation in adult survival; (2) whether markers of oxidative damage and defense were consistent with known sex differences in survival; (3) whether oxidative damage varied longitudinally with reproductive status in females and males; and (4) whether there were detectable costs of oxidative damage to developing offspring. Banded mongooses are small (ca. 1.5 kg) highly social mammals that live in extended family groups of 10–30 individuals. Groups produce on average 4 litters per year, with multiple males and females reproducing in each breeding event (see Materials and Methods). Unusually for a mammal, males live longer than females in this species (Cant et al., 2016). We measured blood levels of oxidative damage to lipids and proteins, and markers of enzymatic and non-enzymatic antioxidant defenses longitudinally for 251 individuals over 5 years. Specifically, we measured plasma levels of malondialdehyde (MDA) as a marker of lipid peroxidation, and plasma protein carbonyls as a marker of oxidative damage to proteins. We also measured whole blood levels of superoxide dismutase (SOD)—which catalyzes the dismutation of superoxide into oxygen and hydrogen peroxide and is an important component of intracellular antioxidant defenses, and plasma levels of vitamin E (α-tocopherol)—a dietary non-enzymatic antioxidant capable of breaking the chain of lipid peroxidation (Halliwell and Gutteridge, 2007).
Our results indicate that oxidative damage can explain patterns of adult and offspring survival in the wild, and provide support for the recent suggestion (Blount et al., 2015) that mothers may gain from shielding their offspring from harm caused by oxidative damage during sensitive developmental windows. We suggest that future advance in life history theory would benefit from an approach that takes into account potential transgenerational impacts of the mechanisms underlying life history trade-offs.
Materials and Methods
Study Population
Data were collected from a wild population of the banded mongoose (Mungos mungo) living on and around the Mweya peninsula, Queen Elizabeth National Park, Uganda. The population has been studied since 1995, and detailed data on life-history, births and deaths have been recorded as part of long-term data collection (Cant et al., 2013, and references therein). Unusually for a mammal, females have a shorter average lifespan than males (38 vs. 42 months; Cant et al., 2016) with the maximum observed lifespan in this population being 11 years for a female, and 12 years for a male. Females also start reproducing earlier than males, at around 1 year of age (Cant et al., 2013), whereas males have to “queue” in order to reproduce (Nichols et al., 2010). Annual mortality rate is around 15% for adults, and females give birth to 8 litters on average during their entire lifetime (Cant et al., 2016; Vitikainen, unpublished data). Banded mongooses are cooperative breeders with a mixed mating system and collaborative rearing of communal litters. Oestrus is synchronized within groups, and occurs 3–4 times per year with a majority of females reproducing in each event. During oestrus males mateguard females, with oldest males fathering most of the offspring (Nichols et al., 2010). Females give birth synchronously to a communal litter which is then cooperatively cared for by all group members (Hodge et al., 2011; Cant et al., 2013), and accurate data on parentage requires assignment by genotyping.
We used two measures to estimate breeding success in relation to oxidative stress markers. First, a portable ultrasound scanner was used to measure fetal litter size during pregnancy. Second, a panel of 43 microsatellite loci was used to assign pups to mothers and to calculate the probability that a pregnant mother had surviving offspring at the time when the communal litter emerged from the den (for details of the genetic analyses see Sanderson et al., 2015). We measured rainfall as a proxy for resource abundance as it is strongly correlated with the availability of arthropod prey, and is a predictor of female reproductive success (Nichols et al., 2012b). Weather data were recorded daily by the Mweya weather station. Monthly rainfall varied between 0 and 140 mm (mean 47.3, SD 32.6) and we used total rainfall 30 days before sampling as a proxy for resource availability (Nichols et al., 2012a).
Trapping and Collection of Blood Samples
During years 2009–2013, 251 adult (>1 year old) mongooses were trapped using baited Tomahawk live traps (Tomahawk Live Trap Co., Tomahawk, Wisconsin, USA), and immobilized using isoflurane (IsoFlo®, Abbott Laboratories). All animals were weighed and females examined for signs of lactation and pregnancy (Cant et al., 2013). Suspected pregnancies were confirmed and fetuses counted using a portable veterinary ultrasound machine (SIUI CTS-900V, Fisher Biomedical).
Altogether 489 blood samples (volume 100–500 μl) were collected from anesthetised mongooses from the jugular vein, within 5 min following anesthesia, using a 25G needle and syringe and immediately transferred into a 3 ml EDTA BD Vacutainer®. A whole blood sample aliquot was immediately frozen for analysis of SOD, and plasma for the remaining assays was extracted by centrifugation of whole blood at 2000 × g for 4 min (Spectrafuge mini centrifuge, Sigma Aldrich, UK) at room temperature. All samples were snap-frozen within 10 min of collection, stored at −80°C, and analyzed within 6 months of collection. Samples were transported from Uganda to our UK laboratory using a cryogenic shipper (Taylor-Wharton CX100, Jencons, UK).
Ethics
All research procedures received prior approval from Uganda Wildlife Authority (UWA) and Uganda National Council for Science and Technology (UNCST), and adhered to the Guidelines for the Treatment of Animals in Behavioral Research and Teaching, published by the Association for the Study of Animal Behavior. All research was approved by the Ethical Review Committee of the University of Exeter.
Analyses of Oxidative Damage and Antioxidant Defenses
Determination of Malondialdehyde (MDA)
Plasma malondialdehyde was determined using high performance liquid chromatography (HPLC) with a fluorescence detector (RF2000; Dionex). All chemicals were HPLC grade, and chemical solutions were prepared using ultra-pure water (Milli-Q Synthesis; Millipore, Watford, UK). We followed the method in Nussey et al. (2009) with some modifications; see Supplementary Material for details.
Determination of Protein Carbonyls
Plasma protein carbonyls were measured using a colorimetric assay (Protein Carbonyl Assay Kit, Cayman Chemical Co., Ann Arbor, MI, USA), using a Spectramax M2 plate reader (Molecular Devices Corp., Sunnyvale, CA, USA). Due to limitations in the amount of plasma available as well as the high protein content of samples, 50 μl of plasma was used in the sample and control tubes instead of the 200 μl recommended in the kit instructions. Carbonyl content in samples was expressed in nmol/mg protein in the controls; repeatability of the plate assay was 87.9%.
Determination of Vitamin E (α-Tocopherol)
A 10 μl aliquot of plasma was mixed with 10 μl of 5% NaCl and 20 μl EtOH, then vortexed for 20 s. Hexane (600 μl) was added and the mixture was vortexed for a further 20 s, then centrifuged for 4 min (13,000 × g). An aliquot (500 μl) of the hexane phase was drawn off and evaporated to dryness under a vacuum and the residue re-dissolved in 150 μl MeOH. Samples (20 μl) were injected into a Dionex HPLC (Dionex Corporation, California, USA). Separation utilized a 3 μ C18 reverse-phase column (15 × 4.6 mm) (Spherisorb S30DS2; Phase separations, Clwyd, UK), with a mobile phase of MeOH:water (97:3 v/v) at a flow rate of 1.1 mL min−1. Fluorescence detection (Dionex RF2000) was performed at 295 nm (excitation) and 330 nm (emission). The α-tocopherol peak was identified and quantified by comparison with a standard solution of α-tocopherol (T3251 Sigma-Aldrich) in MeOH. Concentrations of α-tocopherol are reported as μg mL−1 plasma.
Determination of Superoxide Dismutase (Sod) Activity
Superoxide dismutase was measured in whole blood samples, using the Cayman chemicals superoxide dismutase assay kit. Samples were prepared according to the kit instructions, with blood samples being diluted 1:200 with sample buffer before assaying. Total SOD was quantified relative to a bovine erythrocyte SOD standard supplied with the kit, using a molecular devices plate reader (Spectramax M2; Molecular Devices, USA). Repeatability of the assay was 70.2%. One unit is defined as the amount of enzyme needed to exhibit 50% dismutation of the superoxide radical; results are expressed as enzyme activities in units/ml.
Statistical Analyses
We examined the relationship between life-history variation and markers of oxidative stress by testing whether marker levels showed patterns with (1) adult survival or (2) age, sex or reproductive state of individuals. As malondialdehyde (MDA) showed patterns with both reproduction and survival (see Results) we further tested for the potential for transgenerational effects predicted by our model (Supplementary Material) by (3) examining the relationship between plasma levels of MDA in pregnant mothers and number and survival of her offspring.
Limited sample volume precluded analyzing all markers in all samples, and sample size as well as the last sampling point varied between the markers. We therefore analyzed marker data separately in order to maximize sample size and power of within-individual analysis for each marker, and corrected p-values for multiple testing for each of the separate analyses using false discovery rate (FDR, Benjamini and Hochberg, 1995); sample sizes and total number of individuals included in each analysis are listed in supplementary data tables 3 and 4 in the Supplementary Material. Additionally, we used a PCA with varimax rotation to examine patterns of covariation between markers of oxidative damage and antioxidant defenses (Hõrak and Cohen, 2010) in the 74 samples where data for all four markers were available (Supplementary Table S1).
Survival Analyses
We tested for effects of oxidative stress on survival with number of months survived after the last sampling event as the response, and blood levels of each marker as the (continuous) predictors of survival, and age and sex at sampling as covariates, using Cox regression as implemented in IBM SPSS Statistics for Macintosh, Version 19.0 (Supplementary Table S2).
Effects of Age, Sex, and Breeding Status on Markers of Oxidative Damage and Antioxidant Defences
We investigated whether marker levels changed with age, sex, and group reproductive status (females pregnant or non-pregnant). We then examined effects of age, reproductive state (lactation and pregnancy), body mass and rainfall (a proxy for resource availability; Nichols et al., 2012a,b), on marker levels separately for females. All included variables and their interactions are given in Supplementary Tables S3, S4.
As Vitamin E, SOD and MDA showed an apparent change with group reproductive status, we analyzed a subset of individuals to investigate whether the apparent changes in marker levels with group reproductive state also held within individuals.
Specifically we analyzed those individuals that had been sampled during group pregnancy as well as longitudinally within 3 months of group pregnancy, either before, after, or both). For this analysis we used a repeated-measures ANOVA as implemented in IBM SPSS Statistics for Macintosh, Version 19.0.
Oxidative Damage, litter size, and Survival
To investigate the potential of MDA in mediating transgenerational effects we investigated the relationship between oxidative damage and fetal litter size by using a linear mixed model (LMM) with fetus number, female age and lipid MDA during pregnancy as predictors, and female and group identity as random factors. We then used a generalized linear mixed model (GLMM) with binomial error structure to investigate whether oxidative damage predicted the probability that a pregnant female had any surviving offspring at the time of litter emergence from the den. This model had female age, fetus number and MDA during pregnancy as predictors, and group and individual as random terms. To account for repeated measures, we fitted (LMM) and (GLMM) using restricted maximum likelihood (REML) and included individual and group identity as random effects in all analyses (Bates et al., 2015). The initial models included all factors and their second order interactions. We removed non-significant interactions from the final models to enable assessing significance of the main terms not involved in interactions (Engqvist, 2005), but the models were not simplified further and we report the χ2 and p values of the full model in Supplementary Tables S3, S4. The standard output from a linear mixed model does not differentiate between-subjects and within-subjects effects, such as individual reaction norms vs. individual quality or population level change due to selective disappearance (sensu Nussey et al., 2008). We therefore used within-subject centering, as described in van de Pol and Wright (2009), to examine the relative contribution of within- vs. between-individual variance to explain population level changes in MDA, α-tocopherol and protein carbonyls. These analyses only included individuals with multiple blood samples. We performed these analyses in R 3.0.2 (R Core Team, 2015), fitting GLMMs using the lme4 package (Bates et al., 2015).
Results
Oxidative Damage and Adult Survival
Oxidative lipid damage (MDA) predicted survival in both sexes (remaining lifespan at last blood sampling: Cox regression, Hazard ratio = 2.049, 95 CI = 1.453–2.890, N = 166, adjusted p = 0.00016, Figure 1) whereas protein carbonyls (PC) were not associated with survival (see Supplementary Table S2). Opposite to the pattern expected under the OSTA, plasma levels of MDA declined with age [LMM, individual and group as random effects: = 8.03, p = 0.0046; N = 331; Figure 2A, Supplementary Table S3A]. This was almost entirely driven by a within-individual decline in MDA (within-subject centering, excluding individuals only sampled once: estimate of within-individual change: −0.018 ± 0.004; between-individual estimate: −0.001 ± 0.002: difference between the two: t = 4.3, p < 0.001, N = 238 samples of 88 individuals). A similar pattern of decline with age was observed in protein carbonyls [GLMM, individual and group as random effects: = 3.82, p = 0.051; N = 331; Supplementary Figure S2, Supplementary Table S3A]; however, this was not significant after correcting for false discovery rate (Benjamini and Hochberg, 1995). Variation in PC also seemed to be driven by within-individual change (within-individual estimate: −0.003 ± 0.001, between-individual: −0.001 ± 0.0006) but the difference between the slopes was not significant (t = 0.96, p = 0.33, N = 216 samples of 80 individuals).
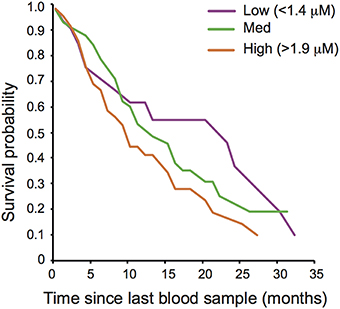
Figure 1. Oxidative damage and adult post-sampling survival of wild banded mongooses. Plasma oxidative lipid damage (malondialdehyde; MDA) concentration predicted adult survival. Survival curves are drawn for illustrative purposes for individuals with low (>1.4 μM), medium and high (>1.9 μM) levels of MDA.
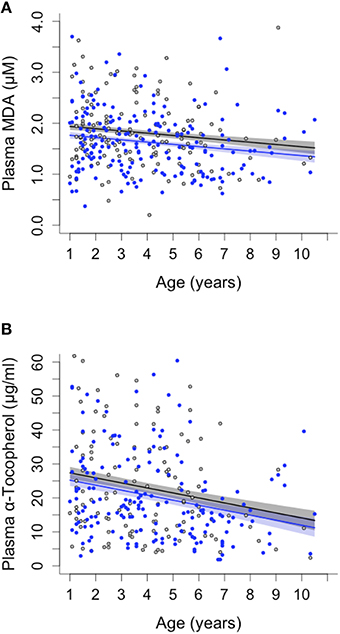
Figure 2. Sex differences and age-related changes in plasma malondialdehyde (MDA) and plasma vitamin E (α-tocopherol) concentration in banded mongooses. (A) MDA decreased with age of individuals and was consistently lower in male (blue) than in female (black) banded mongooses. (B) Vitamin E (α-tocopherol) decreased with age in both sexes. Plots show LMM model predicted values ± SE controlling for random effects of individual and group, and the raw data.
Neither of the measured antioxidants was associated with survival (Supplementary Table S2). Blood levels of SOD activity also showed no pattern with age (Supplementary Table S3B), whereas α-tocopherol decreased with age in both sexes [ = 13.1, p = 0.0003; N = 289; Figure 2B, Supplementary Table S3B]. As with the pattern for MDA, the decline in α-tocopherol was driven by a within-individual decline with age (within-subject centering: estimate of within-individual change: −0.454 ± 0.081; between-individual estimate: −0.07 ± 0.033, difference between estimates: t = 4.399, p < 0.001).
Sex Differences in Oxidative Damage
Females had higher levels of oxidative lipid damage (MDA) than males at all ages [GLMM, age: = 6.29, p = 0.012; N = 331; Figure 2A, Supplementary Table S3B]. This is consistent with the predictions of the OSTA, given our finding that MDA predicted lifespan and that female banded mongooses have shorter lifespans than males (Cant et al., 2016). Blood protein carbonyls, SOD and α-tocopherol showed no overall sex differences (Supplementary Table S3B).
Oxidative Damage and Reproductive Status
Females had lower levels of plasma lipid damage (MDA) during pregnancy [non-pregnant: 1.92 ± 0.0796 μM, pregnant 1.69 ± 0.076 μM, mean ± SE; GLMM: breeding status: = 6.64, p = 0.009; N = 140] and longitudinal analysis spanning pre-, during and post-breeding periods confirmed this was due to a within-individual decline in MDA during pregnancy [repeated-measures ANOVA: F(2, 97) = 6.73, p = 0.002; post-hoc tests before vs. pregnant: p = 0.006; pregnant vs. after: p = 0.007; Figure 3A, Supplementary Table S3].
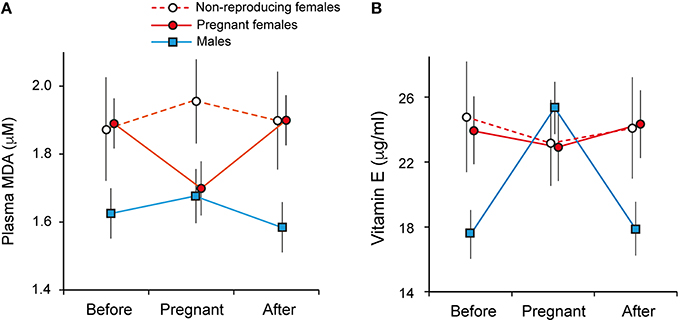
Figure 3. Oxidative damage and reproductive state in banded mongooses. (A) Plasma malondialdehyde (MDA) was lower in females during breeding, and (B) males had higher levels of vitamin E (α-tocopherol) during group breeding. Data points represent mean ± SE of longitudinally sampled individuals during pregnancy and in a non-pregnant state 3 months pre- and post-breeding.
In males, there was no change in MDA levels across the phases of the reproductive cycle (Figure 3A). However, plasma levels of α-tocopherol (vitamin E) were higher in males during group pregnancy across individuals [breeding: 24.8 ± 1.61 μg/ml, non-breeding: 17.22 ± 1.59 μg/ml, mean ± SE; GLMM: sex*group breeding status: = 9.37, p = 0.002; N = 289, Supplementary Table S3B] and longitudinal analysis showed this was due to an increase during breeding [repeated measures ANOVA: F(2, 166) = 7.95, p = 0.001; post-hoc tests: before vs. during breeding, p = 0.003; breeding vs. after, p = 0.003; Figure 3B]. Across the population, breeding was associated with elevated levels of SOD in both sexes [GLMM: group breeding status = 4.82, p = 0.028, Supplementary Table S3B]. However, this result was not significant after correcting for multiple testing (FDR, Benjamini and Hochberg, 1995) and within individuals there were no significant changes associated with breeding in either sex (Supplementary Figure S2; pairwise t-tests all p > 0.15).
Oxidative Damage and Offspring Survival
Females with higher plasma levels of MDA during pregnancy carried fewer fetuses, as measured by ultrasound at weeks 2–4 of gestation, and the decline in fetus number with increasing MDA was steeper in older females [Female age*MDA: = 7.71, p = 0.006; N = 58; Figure 4A]. Similarly, higher plasma levels of MDA during pregnancy were associated with lower litter survival to emergence, controlling for initial litter size, at approximately 1 month of age [MDA: = 3.96, p = 0.047; Fetal litter size: = 0.016, p = 0.89; age: = 2.015, p = 0.156; N = 58; Figure 4B].
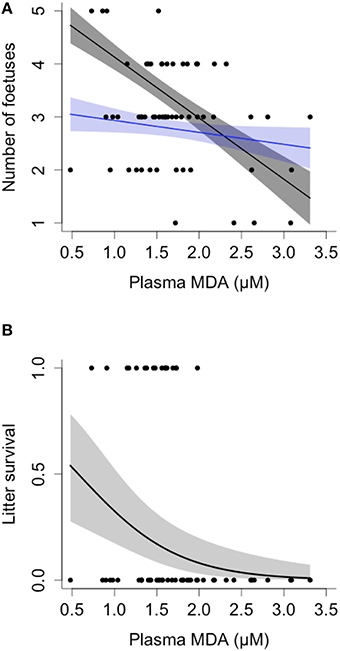
Figure 4. Oxidative damage, fetal litter size, and offspring survival in banded mongooses. (A) Mothers exhibiting higher levels of malondialdehyde (MDA) during gestation carried fewer fetuses, as measured via ultrasound. Oldest females had the steepest decline in fetal litter size; model values are plotted for females at 2 (blue) and 5 years of age (gray) for illustrative purposes. (B) Mothers with higher levels of MDA during gestation had reduced litter survival after taking into account fetal litter size, see text for details of the analysis. Plots show GLMM values ± SE and raw data.
Discussion
Our results provide clear evidence of a link between oxidative damage and survival in a wild animal population, and support the idea that maternal oxidative damage has transgenerational impacts on the fitness of developing young. A striking result is that variation in plasma MDA predicted individual and sex differences in adult survival, and MDA levels during pregnancy were linked to offspring number and survival, whereas plasma protein carbonyls showed no such patterns. Many authors have pointed out that measurement of multiple markers of oxidative damage is necessary to advance understanding of the mechanisms underlying life history trade-offs, because some markers may be more informative than others, and the long-term consequences of different forms of oxidative damage may differ amongst tissues and across the course of life (e.g., Monaghan et al., 2009; Selman et al., 2012; Speakman et al., 2015). Our results highlight the importance of linking markers to fitness effects, and suggest that plasma MDA marks a form of oxidative damage that is associated with functional impairment and negative fitness outcomes under natural conditions. Oxidative lipid damage is implicated in a variety of human pathologies such as cirrhosis (Moustafa et al., 2009) and chronic stroke (Bir et al., 2006), and increased levels of MDA have prognostic value in outcomes and survival from types of cancer (e.g., Salzman et al., 2009) and sepsis (Lorente et al., 2013). However, we are aware of only one other study to date that has linked variation in a marker of oxidative damage (plasma MDA) to a proxy for survival (recruitment probability) in a wild animal (Noguera et al., 2012). Apart from the results that we have presented, there are to our knowledge no other data comparing fitness correlates of two or more oxidative damage markers in a natural population. In order to understand the role of oxidative damage in life history evolution, there is a need for more studies which investigate links between individual damage markers and fitness outcomes (Selman et al., 2012; Speakman and Garratt, 2013; Blount et al., 2015; Speakman et al., 2015).
There is no consistent evidence that oxidative damage increases with age in birds or mammals in the wild (Selman et al., 2012), and our study supports this pattern for the banded mongoose. In our longitudinal dataset, the population-level decline in plasma MDA with age was attributable mostly to a within-individual decline, which suggests that within-individual processes rather than selective disappearance account for the change. A within-individual decline in oxidative damage with age could reflect declining metabolic rate (as in humans, Roberts and Rosenberg, 2006; primates, Roth et al., 2004; and laboratory rodents, Even et al., 2001, although we note that ROS production does not always correlate with metabolic rate; see Speakman et al., 2015), or, potentially, upregulation of endogenous antioxidant activity or increased exogenous antioxidant intake with age. However, vitamin E was also found to decrease with age. As higher levels of MDA were also associated with poorer survival, selective disappearance of individuals that are less able to clear damage could also explain why across the population there is no damage accumulation with age.
Our finding that mothers with high plasma levels of oxidative damage gestated fewer offspring is as expected if mothers adjust litter size according to the potential for transgenerational oxidative harm. As predicted by our model (Supplementary Material; Supplementary Figure S1), females with higher levels of oxidative damage during pregnancy produced fewer fetuses and had lower litter survival. The fetal litter size of older females declined more sharply with increasing MDA than that of younger females. This could reflect senescent decline in the efficiency of shielding mechanisms with advancing age. In our model, allowing both baseline mortality and the offspring sensitivity parameter d to increase with age results in an interaction similar to that seen in Figure 4A. The negative relationship we observed between a measure of “intrinsic” oxidative damage (plasma MDA) and fetal litter size parallels findings in other taxa that mothers exposed to extrinsic sources of damage (e.g., heavy metals) or other environmental stressors produce fewer offspring and have reduced reproductive success (Hendrickx et al., 2003; Räsänen et al., 2005). In both these cases, the potential for maternal damage to carry over to depress offspring survival shifts the optimal maternal reproductive strategy toward fewer, larger offspring, with lower total reproductive success overall (Hendrickx et al., 2003; Räsänen et al., 2005).
The negative relationship between oxidative damage and fetal litter size observed here appears to conflict with studies which have shown a positive relationship between offspring number and oxidative damage (reviewed in Blount et al., 2015). However, the majority of previous studies of oxidative damage and reproductive effort focus on postnatal investment: lactation in mammals, and provisioning in birds (Blount et al., 2015). The costs of gestating additional embryos are likely to be relatively small compared to costs of lactation or provisioning (e.g., Clutton-Brock et al., 1989; Speakman, 2008) and therefore the observed pattern is more likely to reflect quality differences and allocation decisions among females, rather than increase in reproductive effort per se. As banded mongooses are cooperative breeders with communal lactation of pups, females can effectively uncouple investment pre- and post-birth in this species, which may also explain why there was no increase in oxidative damage during lactation (Supplementary Table S4). The negative association between plasma levels of MDA and fetal litter size seems likely to reflect quality differences between mothers, with those that had lower levels of oxidative damage being able to successfully gestate more offspring. Alternatively, it is possible that females carrying more fetuses were also able to, or chose to invest more in limiting oxidative damage or in protecting their offspring from damage transmission; conversely, females with high levels of damage may adjust litter size in order to save resources or to protect themselves.
We found no effects of female weight, parity, or rainfall (an index of food supply) on any of the markers of oxidative damage or antioxidant defense (Supplementary Table S4). However, plasma levels of MDA during pregnancy predicted offspring survival to emergence from the den, which strongly suggests that maternal oxidative state has important consequences for reproductive success (Figure 4B); the effect was independent from the decline in fetus number with increasing damage. Increased levels of MDA are predictive of pregnancy outcomes in humans (Al-Gubory et al., 2010) and complications during pregnancy such as pre-eclampsia (Sharma et al., 2006) and intra-uterine growth restriction (Biri et al., 2007), and our study is consistent with the usefulness of MDA as a marker of survival and fecundity also in wild mammals.
We found no evidence that increased reproductive investment incurred future costs to females in terms of elevated levels of damage and therefore reduced survival. On the contrary, females with low levels of damage survived longer and also were more fecund, pointing to the importance of variation in quality among individuals. A positive relationship between reproduction and future survival is expected where females vary considerably in quality or access to resources, since high quality females may be able to divert more resources to offspring production without compromising their somatic function (the “big house big car” effect, Reznick et al., 2000).
At present it is unclear what mechanisms might be involved in reducing oxidative damage in breeding females. This is unlikely to be due to dietary changes during pregnancy, as females showed no consistent changes in plasma levels of α-tocopherol (vitamin E) during pregnancy, as might be expected if pregnancy was associated with a change in dietary intake of lipophilic compounds such as vitamin E. However, plasma lipid levels become elevated during pregnancy in humans (Knopp et al., 2012) and pregnancy is associated with changes in the lipid composition of plasma (Otto et al., 2001), so a dilution effect or changes toward compounds more robust to oxidation are both potential mechanisms by which transferrable damage could be reduced in pregnant females.
Such reductions in oxidative damage could also arise as a consequence of mitochondrial uncoupling, reducing ROS production (Brand, 2000). However, uncoupling protein gene expression is downregulated or unchanged during lactation (Speakman, 2008), most likely because uncoupling would result in reduced efficiency of energy production at a time when energetic demands are likely to be particularly high (Blount et al., 2015). Rather, laboratory studies of mice, gerbils, and voles suggest that decreased oxidative damage is associated with increased antioxidant defenses (e.g., Garratt et al., 2011; Yang et al., 2013; Xu et al., 2014). In breeding females, antioxidants could be increased as a result of elevated oestradiol, which activates signaling cascades that increase the activity of antioxidant enzymes such as SOD (Viña et al., 2005; Baeza et al., 2010). Indeed, SOD and the non-enzymatic antioxidant glutathione (GSH, one of the most abundant thiols) have specifically been linked to reduced oxidative damage in breeding rodents (Garratt et al., 2011, 2013; Xu et al., 2014).
We found no significant associations between blood levels of the antioxidants SOD or α-tocopherol (vitamin E) and oxidative damage. Cross-sectionally, SOD increased marginally during breeding, but within-individual analyses indicated a pattern only in non-reproductive females (Supplementary Figure S2). We might hypothesize that SOD activity is up-regulated during the breeding season, but becomes depleted in reproductive individuals compared with those that do not reproduce. This serves to highlight that variation in antioxidant levels can be difficult to interpret (Monaghan et al., 2009; Selman et al., 2012), as lowered levels can result from downregulation or reduced dietary intake, as well as depletion caused by oxidative stress. In males only, we observed an increase in plasma levels of α-tocopherol during group breeding, which may point to an increased importance of this antioxidant during reproduction. The mating season is typically associated with heightened testosterone levels in males, posing an additional oxidative challenge (e.g., Aydilek et al., 2004). Furthermore, sperm plasma membranes mainly consist of polyunsaturated fats which are highly susceptible to oxidative damage caused by ROS (Moustafa et al., 2004). Indeed, there is considerable evidence from laboratory studies of humans and animal models that α-tocopherol has an important role in protecting sperm membrane phospholipids against peroxidation, and thereby helps maintain functional fertility (Agarwal et al., 2005; Almbro et al., 2011). Notably, the males were measured on average one month after the mating, which would be well past the point of greatest need for sperm protection from oxidative damage. We do not have measures from males during oestrus, but it is possible that the increase in plasma levels is only detectable after the greatest peak in oxidative challenge, possibly owing to a time-lag in its release from storage tissues. Data linking blood level dynamics of α-tocopherol and fertility are currently lacking for wild animals. This would be a valuable direction for further study.
Conclusion
Despite growing interest among evolutionary biologists in transgenerational effects (Uller, 2008; Badyaev and Uller, 2009; Jablonka and Raz, 2009; Day and Bonduriansky, 2011), only a few models have begun to consider the evolution of the effects themselves rather than focusing on their consequences, and these have largely treated such effects as adaptive mechanisms of information transfer (Shea et al., 2011; Uller and Pen, 2011; Leimar and McNamara, 2015) rather than as a consequence of damage accumulation and management. At the same time, models of oxidative stress and damage accumulation have focused on individual schedules of mortality and fecundity, and have largely ignored its potential transgenerational impacts (Munch and Mangel, 2006; McNamara et al., 2009). The trade-off between within-generational and trans-generational costs has thus yet to be incorporated into life history theory.
Where developing offspring are sensitive to maternal oxidative state, an important component of the cost of reproduction for females may be a requirement to invest in oxidative shielding of offspring, not only oxidative self-defense (Blount et al., 2015). To conclusively test this hypothesis will require an experimental approach to manipulate reproductive effort or oxidative damage, neither of which is possible in our long-term study of a natural population of banded mongooses. Nevertheless, our longitudinal data strongly suggest the existence of trans-generational costs of maternal oxidative damage. Across species, the further development of formal models would help to predict more generally how transgenerational costs of reproduction and strategies to mitigate this damage are likely to coevolve with other life history traits. For example, taxa in which parental investment is relatively small or short in duration may be less likely candidates to invest in oxidative damage reduction during reproduction. A transgenerational approach to the costs of reproduction may advance our understanding of the mechanisms and evolution of life history variation within and across species.
Author Contributions
MC, JB, and EV designed research; EV, JB, JS, HM, JG, and SH collected data; EV and FT analyzed data; JS and HN carried out genetic analyses; JB and CM conducted oxidative stress assays and analyses; MC and RJ wrote the theoretical model; EV, MC, and JB drafted the paper with input from the other authors.
Funding
This work was funded by Natural Environment Research Council (NERC), grant NE/G019657/1 to MC and JB, and the European Research Council (ERC) (Grant number 309249) to MC.
Conflict of Interest Statement
The authors declare that the research was conducted in the absence of any commercial or financial relationships that could be construed as a potential conflict of interest.
Acknowledgments
We are grateful to Uganda Wildlife Authority and Uganda National Council for Science and Technology for permission to carry out our research. We thank the wardens of Queen Elizabeth National Park for logistical support. We thank Francis Mwanguhya, Solomon Kyabulima, Kenneth Mwesige, Robert Businge, Matthew Bell, Neil Jordan, Corsin Mueller, Roman Furrer, and David Jansen for help in the field; and Wendy Campbell for help with sample shipping. We thank Darren Croft and Dan Nussey for comments on the manuscript. Data used in the analyses are available at 10.6084/m9.figshare.3178510.
Supplementary Material
The Supplementary Material for this article can be found online at: http://journal.frontiersin.org/article/10.3389/fevo.2016.00058
References
Agarwal, A., Prabakaran, S. A., and Said, T. M. (2005). Prevention of oxidative stress injury to sperm. J. Androl. 26, 654–660. doi: 10.2164/jandrol.05016
Al-Gubory, K. H., Fowler, P. A., and Garrel, C. (2010). The roles of cellular reactive oxygen species, oxidative stress and antioxidants in pregnancy outcomes. Int. J. Biochem. Cell Biol. 42, 1634–1650. doi: 10.1016/j.biocel.2010.06.001
Almbro, M., Dowling, D. K., and Simmons, L. W. (2011). Effects of vitamin E and beta-carotene on sperm competitiveness. Ecol. Lett. 14, 891–895. doi: 10.1111/j.1461-0248.2011.01653.x
Aydilek, N., Aksakal, M., and Karakilçik, A. Z. (2004). Effects of testosterone and vitamin E on the antioxidant system in rabbit testis. Andrologia 36, 277–281. doi: 10.1111/j.1439-0272.2004.00618.x
Badyaev, A. V., and Uller, T. (2009). Parental effects in ecology and evolution: mechanisms, processes and implications. Philos. Trans. R. Soc. B. Biol. Sci. 364, 1169–1177. doi: 10.1098/rstb.2008.0302
Baeza, I., Fdez-Tresguerres, J., Ariznavarreta, C., and la Fuente, D. E. M. (2010). Effects of growth hormone, melatonin, oestrogens and phytoestrogens on the oxidized glutathione (GSSG)/reduced glutathione (GSH) ratio and lipid peroxidation in aged ovariectomized rats. Biogerontology 11, 687–701. doi: 10.1007/s10522-010-9282-7
Bates, D., Mächler, M., Bolker, B., and Walker, S. (2015). Fitting linear mixed-effects models using lme4. J. Stat. Softw. 67, 1–48. doi: 10.18637/jss.v067.i01
Beckman, K. B., and Ames, B. N. (1998). The free radical theory of aging matures. Physiol. Rev. 78, 547–581. Available online at: http://physrev.physiology.org/content/78/2/547.full
Benjamini, Y., and Hochberg, Y. (1995). Controlling the false discovery rate: a practical and powerful approach to multiple testing. J. R. Stat. Soc. Ser. B. 57, 289–300. doi: 10.2307/2346101
Bir, L. S., Demir, S., Rota, S., and Köseoğlu, M. (2006). Increased serum malondialdehyde levels in chronic stage of ischemic stroke. Tohoku J. Exp. Med. 208, 33–39. doi: 10.1620/tjem.208.33
Biri, A., Bozkurt, N., Turp, A., Kavutcu, M., Himmetoglu, O., and Durak, I. (2007). Role of oxidative stress in intrauterine growth restriction. Gynecol. Obstet. Invest. 64, 187–192. doi: 10.1159/000106488
Blount, J. D., Vitikainen, E. I. K., Stott, I., and Cant, M. A. (2015). Oxidative shielding and the cost of reproduction. Biol. Rev. Camb. Philos. Soc. 91, 483–497. doi: 10.1111/brv.12179
Brand, M. D. (2000). Uncoupling to survive? The role of mitochondrial inefficiency in ageing. Exp. Gerontol. 35, 811–820. doi: 10.1016/S0531-5565(00)00135-2
Cant, M. A., Nichols, H. J., Thompson, F., and Vitikainen, E. I. K. (2016). “Banded mongooses: demography, life-history and social behaviour,” in Cooperative Breeding in Vertebrates, Chapter 18, eds W. D. Koenig and J. L. Dickinson (Cambridge: Cambridge University Press), 318–337. doi: 10.1017/CBO9781107338357.019
Cant, M. A., Vitikainen, E. I. K., and Nichols, H. J. (2013). Demography and social evolution of banded mongooses. Adv. Study Behav. 45, 407–445. doi: 10.1016/B978-0-12-407186-5.00006-9
Clutton-Brock, T. H., Albon, S. D., and Guinness, F. E. (1989). Fitness costs of gestation and lactation in wild mammals. Nature 337, 260–262. doi: 10.1038/337260a0
Day, T., and Bonduriansky, R. (2011). A unified approach to the evolutionary consequences of genetic and nongenetic inheritance. Am. Nat. 178, E18–E36. doi: 10.1086/660911
Engqvist, L. (2005). The mistreatment of covariate interaction terms in linear model analyses of behavioural and evolutionary ecology studies. Anim. Behav. 70, 967–971. doi: 10.1016/j.anbehav.2005.01.016
Even, P. C., Rolland, V., Roseau, S., Bouthegourd, J. C., and Tomé, D. (2001). Prediction of basal metabolism from organ size in the rat: relationship to strain, feeding, age, and obesity. Am. J. Physiol. Regul. Integr. Comp. Physiol. 280, R1887–R1896. doi: 10.1016/0026-0495(73)90035-8
Garratt, M., Pichaud, N., King, E. D. A., and Brooks, R. C. (2013). Physiological adaptations to reproduction. I. Experimentally increasing litter size enhances aspects of antioxidant defence but does not cause oxidative damage in mice. J. Exp. Biol. 216, 2879–2888. doi: 10.1242/jeb.082669
Garratt, M., Vasilaki, A., Stockley, P., McArdle, F., Jackson, M., and Hurst, J. L. (2011). Is oxidative stress a physiological cost of reproduction? An experimental test in house mice. Proc. Biol. Sci. 278, 1098–1106. doi: 10.1098/rspb.2010.1818
Gupta, S., Agarwal, A., Banerjee, J., and Alvarez, J. G. (2007). The role of oxidative stress in spontaneous abortion and recurrent pregnancy loss: a systematic review. Obstet. Gynecol. Surv. 62, 335–347. doi: 10.1097/01.ogx.0000261644.89300.df
Halliwell, B., and Gutteridge, J. (2007). Free Radicals in Biology and Medicine, 4th Edn. Oxford: Oxford University Press.
Hammers, M., Richardson, D. S., Burke, T., and Komdeur, J. (2013). The impact of reproductive investment and early-life environmental conditions on senescence: support for the disposable soma hypothesis. J. Evol. Biol. 26, 1999–2007. doi: 10.1111/jeb.12204
Harman, D. (1956). Aging: a theory based on free radical and radiation chemistry. J. Gerontol. 11, 298–300.
Hendrickx, F., Maelfait, J.-P., Speelmans, M., and Van Straalen, N. M. (2003). Adaptive reproductive variation along a pollution gradient in a wolf spider. Oecologia 134, 189–194. doi: 10.1007/s00442-002-1031-4
Hodge, S. J., Bell, M. B. V., and Cant, M. A. (2011). Reproductive competition and the evolution of extreme birth synchrony in a cooperative mammal. Biol. Lett. 7, 54–56. doi: 10.1098/rsbl.2010.0555
Hõrak, P., and Cohen, A. (2010). How to measure oxidative stress in an ecological context: methodological and statistical issues. Funct. Ecol. 24, 960–970. doi: 10.1111/j.1365-2435.2010.01755.x
Jablonka, E., and Raz, G. (2009). Transgenerational epigenetic inheritance: prevalence, mechanisms, and implications for the study of heredity and evolution. Q. Rev. Biol. 84, 131–176. doi: 10.1086/598822
Kirkwood, T. B., and Rose, M. R. (1991). Evolution of senescence: late survival sacrificed for reproduction. Philos. Trans. R. Soc. Lond. B. Biol. Sci. 332, 15–24. doi: 10.1098/rstb.1991.0028
Knopp, R. H., Bonet, B., and Zhu, X. (2012). “Lipid metabolism in pregnancy,” in Principles of Perinatal–Neonatal Metabolism, ed R. M. Cowett (New York, NY: Springer), 221–258.
Leimar, O., and McNamara, J. M. (2015). The evolution of transgenerational integration of information in heterogeneous environments. Am. Nat. 185, E55–E69. doi: 10.1086/679575
Lorente, L., Martín, M. M., Abreu-González, P., Domínguez-Rodríguez, A., Labarta, L., Díaz, C., et al. (2013). Prognostic value of malondialdehyde serum levels in severe sepsis: a multicenter study. PLoS ONE. 8:e53741. doi: 10.1371/journal.pone.0053741
McNamara, J. M., Houston, A. I., Barta, Z., Scheuerlein, A., and Fromhage, L. (2009). Deterioration, death and the evolution of reproductive restraint in late life. Proc. R. Soc. B. Biol. Sci. 276, 4061–4066. doi: 10.1098/rspb.2009.0959
Metcalfe, N. B., and Monaghan, P. (2013). Does reproduction cause oxidative stress? An open question. Trends Ecol. Evol. 28, 347–350. doi: 10.1016/j.tree.2013.01.015
Monaghan, P., Metcalfe, N. B., and Torres, R. (2009). Oxidative stress as a mediator of life history trade-offs: mechanisms, measurements and interpretation. Ecol. Lett. 12, 75–92. doi: 10.1111/j.1461-0248.2008.01258.x
Moore, M. J., DiStefano, R. J., and Larson, E. R. (2013). An assessment of life-history studies for USA and Canadian crayfishes: identifying biases and knowledge gaps to improve conservation and management. Freshwater Sci. 32, 1276–1287. doi: 10.1899/12-158.1
Moustafa, A. H. A., Ali, E. M. M., Mohamed, T. M., and Abdou, H. I. (2009). Oxidative stress and thyroid hormones in patients with liver diseases. Eur. J. Int. Med. 20, 703–708. doi: 10.1016/j.ejim.2009.08.003
Moustafa, M. H., Sharma, R. K., Thornton, J., Mascha, E., Abdel-Hafez, M. A., Thomas, A. J., et al. (2004). Relationship between ROS production, apoptosis and DNA denaturation in spermatozoa from patients examined for infertility. Hum. Reprod. 19, 129–138. doi: 10.1093/humrep/deh024
Munch, S. B., and Mangel, M. (2006). Evaluation of mortality trajectories in evolutionary biodemography. Proc. Natl. Acad. Sci. U.S.A. 103, 16604–16607. doi: 10.1073/pnas.0601735103
Nichols, H. J., Amos, W., Bell, M. B. V., Mwanguhya, F., Kyabulima, S., and Cant, M. A. (2012a). Food availability shapes patterns of helping effort in a cooperative mongoose. Anim. Behav. 83, 1377–1385. doi: 10.1016/j.anbehav.2012.03.005
Nichols, H. J., Amos, W., Cant, M. A., Bell, M. B. V., and Hodge, S. J. (2010). Top males gain high reproductive success by guarding more successful females in a cooperatively breeding mongoose. Anim. Behav. 80, 649–657. doi: 10.1016/j.anbehav.2010.06.025
Nichols, H. J., Bell, M. B. V., Hodge, S. J., and Cant, M. A. (2012b). Resource limitation moderates the adaptive suppression of subordinate breeding in a cooperatively breeding mongoose. Behav. Ecol. 23, 635–642. doi: 10.1093/beheco/ars008
Noguera, J. C., Kim, S. Y., and Velando, A. (2012). Pre-fledgling oxidative damage predicts recruitment in a long-lived bird. Biol. Lett. 8, 61–63. doi: 10.1098/rsbl.2011.0756
Nussey, D. H., Coulson, T., Festa-Bianchet, M., and Gaillard, J. M. (2008). Measuring senescence in wild animal populations: towards a longitudinal approach. Funct. Ecol. 22, 393–406. doi: 10.1111/j.1365-2435.2008.01408.x
Nussey, D. H., Pemberton, J. M., Pilkington, J. G., and Blount, J. D. (2009). Life history correlates of oxidative damage in a free-living mammal population. Funct. Ecol. 23, 809–817. doi: 10.1111/j.1365-2435.2009.01555.x
Otto, S. J., van Houwelingen, A. C., Badart-Smook, A., and Hornstra, G. (2001). Changes in the maternal essential fatty acid profile during early pregnancy and the relation of the profile to diet. Am. J. Clin. Nutr. 73, 302–307. Available online at: http://ajcn.nutrition.org/content/73/2/302.long
R Core Team (2015). R: A Language and Environment for Statistical Computing. Vienna: R Foundation for Statistical Computing. Available online at: http://www.R-project.org/
Räsänen, K., Laurila, A., and Merilä, J. (2005). Maternal investment in egg size: environment- and population-specific effects on offspring performance. Oecologia 142, 546–553. doi: 10.1007/s00442-004-1762-5
Reznick, D., Nunney, L., and Tessier, A. (2000). Big houses, big cars, superfleas and the costs of reproduction. Trends Ecol. Evol. 15, 421–425. doi: 10.1016/S0169-5347(00)01941-8
Roberts, S. B., and Rosenberg, I. (2006). Nutrition and aging: changes in the regulation of energy metabolism with aging. Physiol. Rev. 86, 651–667. doi: 10.1152/physrev.00019.2005
Roth, G. S., Mattison, J. A., Ottinger, M. A., Chachich, M. E., Lane, M. A., and Ingram, D. K. (2004). Aging in rhesus monkeys: relevance to human health interventions. Science 305, 1423–1426. doi: 10.1126/science.1102541
Salzman, R., Pácal, L., Tomandl, J., Kanková, K., Tóthová, E., Gál, B., et al. (2009). Elevated malondialdehyde correlates with the extent of primary tumor and predicts poor prognosis of oropharyngeal cancer. Anticancer Res. 29, 4227–4231. Available online at: http://ar.iiarjournals.org/content/29/10/4227.long
Sanderson, J. L., Wang, J., Vitikainen, E. I. K., Cant, M. A., and Nichols, H. J. (2015). Banded mongooses avoid inbreeding when mating with members of the same natal group. Mol. Ecol. 24, 3738–3751. doi: 10.1111/mec.13253
Selman, C., Blount, J. D., Nussey, D. H., and Speakman, J. R. (2012). Oxidative damage, ageing, and life-history evolution: where now? Trends Ecol. Evol. 27, 570–577. doi: 10.1016/j.tree.2012.06.006
Sharma, J. B., Sharma, A., Bahadur, A., Vimala, N., Satyam, A., and Mittal, S. (2006). Oxidative stress markers and antioxidant levels in normal pregnancy and pre-eclampsia. Int. J. Gynecol. Obstet. 94, 23–27. doi: 10.1016/j.ijgo.2006.03.025
Shea, N., Pen, I., and Uller, T. (2011). Three epigenetic information channels and their different roles in evolution. J. Evolut. Biol. 24, 1178–1187. doi: 10.1111/j.1420-9101.2011.02235.x
Speakman, J. R. (2008). The physiological costs of reproduction in small mammals. Philos. Trans. R. Soc. Lond. B. Biol. Sci. 363, 375–398. doi: 10.1098/rstb.2007.2145
Speakman, J. R., Blount, J. D., Bronikowski, A. M., Buffenstein, R., Isaksson, C., Kirkwood, T. B. L., et al. (2015). Oxidative stress and life histories: unresolved issues and current needs. Ecol. Evol. 5, 5745–5757. doi: 10.1002/ece3.1790
Speakman, J. R., and Garratt, M. (2013). Oxidative stress as a cost of reproduction: beyond the simplistic trade-off model. Bioessays 36, 93–106. doi: 10.1002/bies.201300108
Speakman, J. R., and Selman, C. (2011). The free-radical damage theory: accumulating evidence against a simple link of oxidative stress to ageing and lifespan. Bioessays 33, 255–259. doi: 10.1002/bies.201000132
Uller, T. (2008). Developmental plasticity and the evolution of parental effects. Trends Ecol Evol. 23, 432–438. doi: 10.1016/j.tree.2008.04.005
Uller, T., and Pen, I. (2011). A theoretical model of the evolution of maternal effects under parent-offspring conflict. Evolution 65, 2075–2084. doi: 10.1111/j.1558-5646.2011.01282.x
van de Pol, M., and Wright, J. (2009). A simple method for distinguishing within- versus between-subject effects using mixed models. Anim. Behav. 77, 753–758. doi: 10.1016/j.anbehav.2008.11.006
Viña, J., Borrás, C., Gambini, J., Sastre, J., and Pallardó, F. V. (2005). Why females live longer than males? Importance of the upregulation of longevity-associated genes by oestrogenic compounds. FEBS Lett. 579, 2541–2545. doi: 10.1016/j.febslet.2005.03.090
Xu, Y.-C., Yang, D.-B., Speakman, J. R., and Wang, D.-H. (2014). Oxidative stress in response to natural and experimentally elevated reproductive effort is tissue dependent. Funct. Ecol. 28, 402–410. doi: 10.1111/1365-2435.12168
Yang, D.-B., Xu, Y.-C., Wang, D.-H., and Speakman, J. R. (2013). Effects of reproduction on immuno-suppression and oxidative damage, and hence support or otherwise for their roles as mechanisms underpinning life history trade-offs, are tissue and assay dependent. J. Exp. Biol. 216, 4242–4250. doi: 10.1242/jeb.092049
Keywords: life history, oxidative stress, maternal effects, aging, early-life effects, litter size, tradeoffs, cooperative breeding
Citation: Vitikainen EIK, Cant MA, Sanderson JL, Mitchell C, Nichols HJ, Marshall HH, Thompson FJ, Gilchrist JS, Hodge SJ, Johnstone RA and Blount JD (2016) Evidence of Oxidative Shielding of Offspring in a Wild Mammal. Front. Ecol. Evol. 4:58. doi: 10.3389/fevo.2016.00058
Received: 08 March 2016; Accepted: 05 May 2016;
Published: 18 May 2016.
Edited by:
Geoffrey E. Hill, Auburn University, USACopyright © 2016 Vitikainen, Cant, Sanderson, Mitchell, Nichols, Marshall, Thompson, Gilchrist, Hodge, Johnstone and Blount. This is an open-access article distributed under the terms of the Creative Commons Attribution License (CC BY). The use, distribution or reproduction in other forums is permitted, provided the original author(s) or licensor are credited and that the original publication in this journal is cited, in accordance with accepted academic practice. No use, distribution or reproduction is permitted which does not comply with these terms.
*Correspondence: Emma I. K. Vitikainen, ZS5pLmsudml0aWthaW5lbkBleGV0ZXIuYWMudWs=;
Michael A. Cant, bS5hLmNhbnRAZXhldGVyLmFjLnVr;
Jonathan D. Blount, ai5kLmJsb3VudEBleGV0ZXIuYWMudWs=