- 1School of Animal Biology, University of Western Australia, Perth, WA, Australia
- 2University of Western Australia Oceans Institute, University of Western Australia, Perth, WA, Australia
- 3Lions Eye Institute, University of Western Australia, Perth, WA, Australia
Light detection not only forms the basis of vision (via visual retinal photoreceptors), but can also occur in other parts of the body, including many non-rod/non-cone ocular cells, the pineal complex, the deep brain, and the skin. Indeed, many of the photopigments (an opsin linked to a light-sensitive 11-cis retinal chromophore) that mediate color vision in the eyes of vertebrates are also present in the skin of animals such as reptiles, amphibians, crustaceans and fishes (with related photoreceptive molecules present in cephalopods), providing a localized mechanism for light detection across the surface of the body. This form of non-visual photosensitivity may be particularly important for animals that can change their coloration by altering the dispersion of pigments within the chromatophores (pigment containing cells) of the skin. Thus, skin coloration may be directly color matched or “tuned” to both the luminance and spectral properties of the local background environment, thereby facilitating behavioral functions such as camouflage, thermoregulation, and social signaling. This review examines the diversity and sensitivity of opsin-based photopigments present in the skin and considers their putative functional roles in mediating animal behavior. Furthermore, it discusses the potential underlying biochemical and molecular pathways that link shifts in environmental light to both photopigment expression and chromatophore photoresponses. Although photoreception that occurs independently of image formation remains poorly understood, this review highlights the important role of non-visual light detection in facilitating the multiple functions of animal coloration.
Introduction
Almost all taxa, ranging from simple single-celled organisms to vertebrates, have evolved to cope with exposure to, or utilize photic stimuli derived from sunlight. As a result, many species exhibit some form of light sensitivity (Wolken and Mogus, 1979). Fundamental responses to light are particularly well known in marine invertebrates and include orientation of the body, localized movement of appendages, withdrawal or “shadow reflexes” and locomotion (reviewed by Steven, 1963). For example, a change in light intensity stimulates tail withdrawal in annelid worms, as well as the opening and closing of the siphon in sea squirts (reviewed by Wolken, 1988). The fundamental role of light in guiding non-visual animal behavior is evident from the finding that photokinetic responses (locomotory responses to light) are observed in ancestral taxa or at very early life stages before the eyes are fully functional. For example, zebrafish larvae that have been enucleated and had their pineal gland ablated at 5 days post-fertilization display increased locomotory activity on exposure to light (Fernandes et al., 2012). Photosensitivity has also been reported in eyeless fishes such as the Mexican cave tetra (Astyanax mexicanus) (Langecker, 1989), the Arabian barb (Garra barreimiae) (Timmermann and Plath, 2009), and the Somalian blind cavefish (Phreatichthys andruzzii) (Tarttelin et al., 2012). Despite the complete absence of eyes, optic nerve and associated brain structures, the Somalian blind cavefish displays photophobic behaviors that are mediated by light-sensitive rod-like visual pigments present in the brain (Tarttelin et al., 2012). Conversely, photoresponses, such as entrainment of peripheral clocks by external light, appear to be absent in P. andruzzii due to mutations identified in two non-visual pigment genes, a melanopsin (opn4) and a teleost multiple tissue (tmt) opsin, whereas both photoentrainment and these candidate pigments remain intact in zebrafish (Danio rerio) (Cavallari et al., 2011). It is likely, however, that various species of cavefish possess many more visual and non-visual opsin classes (Tobler et al., 2010; Tarttelin et al., 2012; Meng et al., 2013; Yang et al., 2016), so the pigments that mediate the entrainment of tissue-specific peripheral circadian clocks are yet to be determined. Nonetheless, light plays a fundamental role in the behavior of almost all organisms, with detection mechanisms that are likely to be universally mediated by opsin-based photopigments and the biochemical photocascades they activate.
In addition to visual processes, light also induces important physiological responses in the majority of animals, such as pupil constriction, pineal melatonin suppression, and the entrainment of circadian rhythms (Freedman et al., 1999; Lucas et al., 2001; Rea et al., 2002; Semo et al., 2003). Circadian rhythms, which oscillate over ~24 h, allow animals to respond to temporal events in the environment when it is generally most beneficial to do so (e.g., synchronicity of the daily sleep/wake cycle to coincide with the availability of food); however, biological rhythms may be regulated over longer (and shorter) time periods (e.g., circannual cycles oscillate over a year) and are critical for the timing of major biological events such as maturation, reproduction and migration (Foster and Hankins, 2002). Pioneering research with house sparrows (Passer domesticus) in the 1960-1970s revealed that development of the testes is under photoperiodic control and is maintained even when the eyes and pineal gland are removed (Menaker et al., 1970). With the general exception of mammalian vertebrates, light detection is not only performed by the eye, but by photoreceptive cells present in other regions of the body, including the pineal complex, the deep brain and the skin (reviewed by Vigh et al., 2002; Davies et al., 2010, 2014, 2015; Hankins et al., 2014). This additional light sense is referred to as “extraocular photoreception” (Millott, 1968), or more generally as “non-visual photoreception” since the eye performs many functions that do not involve classical image-formation. The skin or dermis is a particularly important site of photoreception as it is the first part of the body to receive light and typically presents a large surface area for photon capture. Classic studies of dermal light detection with a variety of organisms such as hydroids, flatworms, anemones, lampreys, blind cave fish, and many others, have revealed that the skin is not only sensitive to changes in light intensity, but also to different wavelengths (Steven, 1963). Simple dermal photoreception was previously thought to be limited to primitive animals, with more specialized organs such as the eye considered the main sites for light detection in complex organisms. However, it is becoming increasingly clear that this is not the case, and the underlying mechanisms of light detection by the skin are surprisingly sophisticated and critical to animal behavior and survival. Recently it was shown that isolated cnidarian tentacles (Hydra vulgaris) can distinguish between, and respond to, various wavelengths (i.e., colors) across the visible spectrum despite lacking structures that resemble traditional photoreceptors (Guertin and Kass-Simon, 2015). However, the photosensory pigments and pathways that are involved in dermal non-visual light detection remain to be fully determined.
In poikilothermic animals, non-visual photoreceptors can occur in specialist pigment containing cells, known as chromatophores (Table 1). Chromatophores contain the pigments that are responsible for generating the body coloration of amphibians, reptiles, crustaceans, insects cephalopods, and fishes, but also mediate changes in coloration observed in these animals (Parker, 1948; Bagnara and Hadley, 1973; Umbers et al., 2014). The notion that chromatophores might respond directly to changes in ambient light is of particular relevance to behavioral and evolutionary ecologists due to the important functional role of coloration in thermoregulation, social communication, sexual selection, and predator avoidance (Cott, 1940; Stevens and Merilaita, 2009; Stuart-Fox and Moussalli, 2009; Stevens, 2016). Depending on the species, individuals respond to changes in the light environment by either aggregating or dispersing the pigments located in their chromatophores, causing a change in the area of body coloration (Figure 1). Thus, the identification of photoreceptors in the skin potentially provides a localized mechanism of light detection for specific regions of the body that is independent from image-forming visual processes. Indeed, regulatory independence from ocular photoreception is advantageous for rapid dermal changes in color, due to the lack of requirement for complex neural or endocrine signaling pathways involving the eye, brain, and skin (the exception being cephalopods as discussed below). In addition, direct dermal photosensitivity could supplement any photic input received by the retina and/or provide luminance information in regions outside the field of view or outside the spectral range of the visual photoreceptors. This is likely to be particularly important when the head of the animal is hidden or buried, but other parts of the body, such as the tail, are exposed to environmental light. The ability to detect changes in environmental light also provides animals with important temporal information about their surroundings that can be used to regulate behavioral activities, such as foraging and reproductive behaviors, as well as regulating the cyclic changes in body coloration that are commonly observed in poikilotherms (Caswell, 1950).
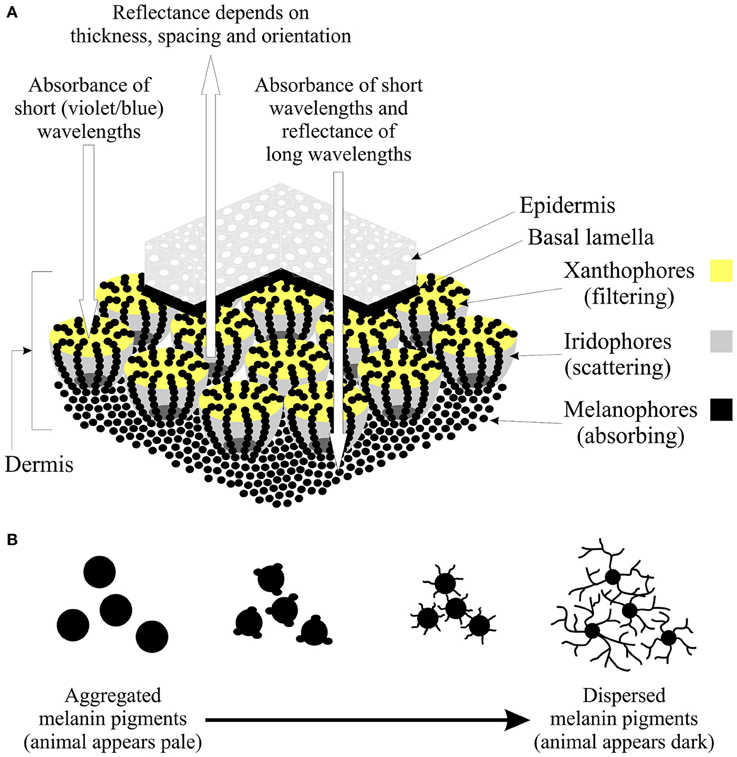
Figure 1. The structure and function of chromatophores. (A) Illustration of a section of skin showing the dermal chromatophore unit (modified from Bagnara et al., 1968; Bagnara and Hadley, 1973) and how the interaction of the chromatophores results in the observed reflectance spectrum of a color patch. The upper layers of xanthophores and erythrophores act to filter light and preferentially absorb short wavelengths (violets and blues). The underlying iridophores may reflect all incident light or partially scatter light depending on the thickness, spacing, and orientation of the platelets. Any remaining light is absorbed by the melanophores or reflected back through the upper chromatophores by a basal layer of leucophores (if present). If the platelets of the iridophores are arranged to reflect light of all wavelengths equally, the animal will appear silver, yellow, orange or red depending on the pigment composition of the xanthophores (Grether et al., 2004). If the platelets are arranged so that light is partly reflected, long-wavelength light is absorbed by the melanophores, while short-wavelengths are reflected. This results in the animal appearing blue if no xanthophores are present, or yellow-green, green or blue-green if xanthophores are present (Grether et al., 2004). In the context of color change, note that the dendritic processes of the melanophores extend up and terminate above the iridophores. (B) During color change on dark backgrounds, the iridophores may aggregate (leading to a reduction in the surface area of reflectance), while melanin pigments move into the dendritic processes to cover the iridophores (Bagnara and Hadley, 1973). The overall effect is a change in the shape of the melanophore. Thus, melanin pigments are aggregated when the animal is placed on a pale background, making the animal appear pale, while pigments are dispersed on a dark background, thereby darkening the skin of the animal.
The aim of this review is to provide a brief overview of the structure and classification of chromatophores, before considering the evidence for light-mediated changes in skin coloration that occur independently of the eyes. In this review, different classes of chromatophores will be examined within the context of whether they contain different types of opsin-based photoreceptor, before considering the molecular mechanisms that underpin and regulate dermal light detection. Finally, the types of information that light detection by the skin can potentially provide will be discussed, as well as how dermal photoreception may guide a variety of behavioral and physiological responses in animals. Although there are a number of molecules that are responsible for photoreception in animals, such as the blue light-sensitive cryptochromes that play a major role in the circadian clock, this review focuses on opsins. Being functionally diverse, having a broad spectral range with absorbance maxima ~360-625 nm (depending on chromophore usage), as well as being expressed in peripheral tissues such as the skin, opsin pigments (especially visual opsins) are ideal candidates for the molecular basis of dermal photosensitivity; however, their light detection mechanisms are poorly understood outside of their roles in image-forming vision. This review finds that in many studies, the cell types in which the opsins are expressed have not been identified; thus, the general phrase “light detection by the skin” is used to encompass a range of potential photoreceptive sites located within the dermal area.
Structure and Classification of Chromatophores
The body color patterns of animals such as amphibians, fishes, reptiles, and cephalopods are generated and altered using specialist pigment-containing chromatophores that absorb or reflect light (Fujii, 1993; Skold et al., 2016). These cells are generally classified as follows based on their color, which in turn reflects the pigments they contain (Table 1): (1) brown/black melanophores that contain melanin; (2) erythrophores (red) colored by various carotenoids; and (3) xanthophores, which are colored yellow by the presence of pteridine pigments. Two further chromatophore classes exist that generate color due to the light-reflecting structural properties of the purine platelets they contain, namely iridophores (silvery and iridescent) and leucophores (white). In poikilothermic vertebrates, the basic dermal chromatophore comprises three layers: basal melanophores that absorb light of all wavelengths, an iridophore layer that reflects light, and an outer layer of xanthophores and erythrophores that absorb short-wavelength light (Bagnara and Hadley, 1973; Grether et al., 2004; Figure 1). The relative amounts of each chromatophore type, their density and state of dispersion or aggregation determine the color of a particular skin patch (Bagnara and Hadley, 1973; Grether et al., 2004).
The chromatophores of cephalopods differ from those of other animals in their morphology; the pigment granules are contained in an elastic sac that is controlled by radial muscles and nerves connected directly to the brain (Messenger, 2001). In fishes, reptiles, and amphibians, color changes have historically been categorized as rapid “physiological color change” that is mediated by the motile activity of the chromatophores, or “morphological color change,” which refers to color change that occurs more slowly and involves the production or absence of pigments (Bagnara and Hadley, 1973; Fujii and Oshima, 1986; Fujii, 1993). In practice, this distinction is not based on distinct mechanisms and is, thus, somewhat arbitrary. The underlying mechanisms of physiological color change are best understood for the melanophores of fishes, where chromatophores were initially considered to respond primarily to the intensity of light and secondarily, or indirectly via melanocyte stimulating hormone (MSH) produced by the pituitary gland and melatonin secreted by the pineal complex (Bagnara and Hadley, 1973). Nonetheless, several studies have re-examined photoreception in chromatophores (melanophores in particular) and demonstrated that they are also sensitive to particular wavelengths of light.
Melanophore Responses to Environmental Light
The possibility of non-visual photosensitivity was first suggested by Karl von Frisch in 1911, when it was noted that common minnows (Phoxinus phoxinus) change their skin coloration in response to light, even when the eyes and pineal organs had been removed, leading to the suggestion that light detection occurred in the deep regions of the brain (von Frisch, 1911). This was followed by work with mosquitofish in the 1940s, which demonstrated that fish placed on a dark background for several weeks disperse melanin in the skin to darken their coloration, while those placed on a white background aggregate melanin and appear pale (Sumner, 1935a). Furthermore, experiments with both bird and fish predators revealed the protective value of this color change, as fish that matched their background were less likely to be attacked than mismatched fish (Sumner, 1935a,b). Such camouflage strategies are not limited to vertebrates; indeed, a number of studies in invertebrates have also demonstrated body color changes that serve to match the local background [e.g., shore crab (Carcinus maenas) juveniles (Stevens et al., 2014a,b)].
It has sometimes been assumed that the color change response is mediated by the eye, based on the ratio of light coming from above relative to that reflected off the substrate below (Sugimoto, 2002). However, a number of studies has revealed that chromatophore responses to light are maintained in denervated tissue preparations (Fujii, 2000). For example, in the cryprinid fish Zacco temmincki, the melanophores in excised scales aggregate when exposed to the dark and disperse on exposure to light, where the level of aggregation depends on the light intensity (Iga and Takabatake, 1983). Other studies have examined the spectral sensitivity of fish melanophores, showing for example, that the optimum wavelength for melanosome dispersion in Z. temmincki is 525 nm (Hiroyuki et al., 1988). Studies with reptiles have also revealed that blinded animals can change color for background matching. One such investigation showed that Moorish geckos (Tarentola mauritanica) exhibit the same skin darkening response when placed on a black background, irrespective of whether the eyes were covered or not. By contrast, no color change was observed when the thorax was obscured, suggesting that light detection occurs in this region (Fulgione et al., 2014). Subsequent molecular and histological analyzes revealed that a light sensitive visual pigment was present in the skin tissues of T. mauritanica and showed higher levels of expression in the flanks than on the back or belly (Fulgione et al., 2014). In this case, the pigment was identified as the short-wavelength-sensitive-1 (SWS1, but also known as OPN1SW) opsin (Figure 2), although it is currently unknown if this pigment is sensitive to ultraviolet (UV) or violet wavelengths.
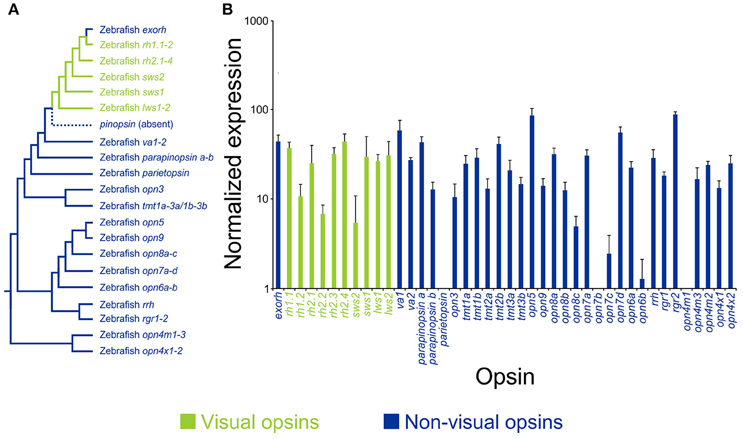
Figure 2. Opsin diversity and expression in the skin of a model vertebrate. (A) A representative phylogenetic tree of vertebrate visual (green) and non-visual (blue) pigment genes, showing the presence of 42 opsin sequences in the model teleost species, zebrafish (Danio rerio), including pinopsin (absent in teleosts, but found in other non-mammalian lineages). Opsin gene classes are divided into extra-ocular rhodopsin (exorh), rhodopsin-like-1 (rh1) rod opsins (rh1.1 and rh1.2), rhodopsin-like-2 (rh2) cone opsins (rh2.1, rh2.2, rh2.3, and rh2.4), short-wavelength-sensitive-2 (sws2) cone opsin, short-wavelength-sensitive-1 (sws1) cone opsin, long-wavelength-sensitive (lws) cone opsins (lws1 and lws2), vertebrate ancient (va) opsins (va1 and va2), parapinopsins (parapinopsin a and parapinopsin b), parietopsin, encephalopsin/panopsin (opn3), teleost multiple tissue (tmt) opsins (tmt1a, tmt1b, tmt2a, tmt2b, tmt3a, and tmt3b), neuropsin (opn5), novel opsins (opn6, opn7, opn8, and opn9), retinal pigment epithelium-derived rhodopsin homolog (rrh; also known as peropsin), retinal pigment epithelium (RPE) retinal G protein receptors (rgr) (rgr1 and rgr2), mammalian-like melanopsins (opn4m) (opn4m1, opn4m2, and opn4m3), and Xenopus-like melanopsins (opn4x) (opn4x1 and opn4x2). (B) Gene expression profiling of adult zebrafish opsin transcripts in the skin using the NanoString nCounter assay. Relative opsin gene expression is presented as bar charts on a log10 scale. Each column represents a different opsin, which is color-coded as described above. Modified from Davies et al. (2015).
A number of studies have shown that blind or eyeless Xenopus laevis (Imai and Takahashi, 1971), the Mexican axolotl Ambystoma mexicanum (Epp, 1972), and hybrids of Astyanax mexicanus generated by crossing surface and Pachón cavefish pleiotropic forms (Gross et al., 2016), display a permanently darker phenotype. This suggests that there is an association between light detection by the eye and the production of body pigmentation; however, by contrast, many species that dwell in light restricted environments (e.g., cave animals) are often blind or visually impaired and present with a loss of pigmentation or an albino phenotype (e.g., many A. mexicanus populations) (Culver, 1982; Jeffery, 2009a,b), so the underlying mechanisms that are currently debated are likely to be very complex. For example, a zebrafish mutant (i.e., “lakritz” or “lak”) that lacks the ath5 gene [which is present in retinal ganglion cells (RGCs)] is unable to distribute melanin pigment, remains permanently darkened, and is unable to exhibit background matching (Kay et al., 2001), suggesting a central role of the eyes (or at least this gene) in pigment aggregation. By contrast, further evidence from zebrafish suggests pigment dispersion (i.e., skin darkening) occurs independently of the eyes (Shiraki et al., 2010). Dermal melanophores in zebrafish larvae, which naturally develop a characteristic horizontal banding body pattern and are thought to protect again UV damage, responded to illumination from 2 days post-fertilization by rapidly dispersing melanin granules, whereas delayed pigment aggregation occurred at 3 days post-fertilization (Shiraki et al., 2010). Furthermore, enucleation abolished pigment aggregation, but not granule dispersal. These data suggest, at least in zebrafish, that pigment dispersion and aggregation are eye-independent and eye-dependent, respectively (Shiraki et al., 2010). The authors stated a number of possible candidate pigments; however, they did not provide any evidence for their expression in skin tissues except for two out of the five melanopsin (opn4) genes (Davies et al., 2011), a pigment class first identified from dermal melanophores of the African clawed frog, Xenopus laevis (Provencio et al., 1998). More recently, a critical study demonstrated that zebrafish possess 42 opsin genes in their genome, including many previously unpublished novel pigments (Figure 2; Davies et al., 2015). Surprisingly, the authors demonstrated that 38 of these genes, encompassing all the known visual pigments and most non-visual opsins, were expressed in the skin (Figure 2; Davies et al., 2015). It is likely that many of these pigments are involved in multiple non-visual tasks (e.g., modulation of peripheral clocks, photoisomerization, UV detection, and DNA repair); nonetheless, it would not be unexpected if many of these light-activated proteins also played a photosensory role in localized pigment dispersion, a hypothesis that warrants further investigation. Of particular interest is the observation that ~40% of non-mammalian opsin gene classes are conserved in the mammalian lineage (Davies et al., 2015), some of which (e.g., OPN1SW; rhodopsin-1, RH1; panopsin, OPN3, and neuropsin, OPN5) are expressed in dermal tissue of mice (Kojima et al., 2011; de Assis et al., 2016) and humans (Tsutsumi et al., 2009; Kim et al., 2013; Haltaufderhyde et al., 2015). Their functional roles are currently unknown; however, it has been suggested that they might be involved in the detection of solar radiation and may induce photo-protective cellular and behavioral mechanisms.
Responses of Other Chromatophores to Light
In addition to melanophores, there is evidence that other types of chromatophore exist that may display a direct response to light that is independent from ocular photoreception (Oshima, 2001). For example, the reflectance of the iridophores that form the iridescent “neon” lateral stripe of neon tetras (e.g., Paracheirodon innesi) changes from a blue-green coloration during the day to a violet-blue color at night, even in decapitated tetras (Lythgoe and Shand, 1982). This color change occurs by altering the thickness of the cytoplasmic layer between the guanine platelets and commences a few minutes after the experimental lighting is switched on, with the full transition from blue-green to violet-blue being complete within 25–35 min. Immunofluorescence staining revealed that light detection by the iridophores is regulated by opsin-based visual pigments (Lythgoe et al., 1984); however, which pigment classes might be involved remained undetermined until a 2006 study identified the dermal expression of a rod opsin (rhodopsin-1; rh1) gene and two cone opsin (i.e., rhodopsin-like-2 (rh2) class) genes in iridophores most photosensitive to 500 nm (Kasai and Oshima, 2006).
In Nile tilapia (Oreochromis niloticus), erythrophore pigments in denervated caudal tissue aggregated when exposed to light within two wavelength ranges, 400–440 nm and 550–600 nm, and dispersed when exposed to wavelengths between 470 and 530 nm (Oshima and Yokozeki, 1999), a spectrally antagonistic photosensitive mechanism that was subsequently confirmed and functionally characterized (Chen et al., 2015) and shown to be influenced by different chromatic backgrounds (Chen et al., 2014). The authors of the earlier study suggested that the wavelength range for optimum dispersion coincides with the wavelength of maximum sunlight intensity (i.e., 500 nm) during peak reproductive activity for this species in spring and summer (Oshima and Yokozeki, 1999). Nonetheless, such changes could also occur due to seasonal variation in the intensity and wavelength of light in the aquatic environment (e.g., due to changes in the humic (organic matter) content of the water). Molecular analyzes have shown some consistency between these studies as both medium-wavelength-sensitive (rh2) and long-wavelength-sensitive (lws) opsin genes were identified (Ban et al., 2005). More recently, a greater number of opsins were found in Nile tilapia fin erythrophores (as well as melanophores), where single-cell analyzes revealed diverse expression patterns: some cells expressed single opsins (e.g., sws1 or rh2b in melanophores; sws1 or one of the three rh2 subclasses in erythrophores), while others appeared to co-express multiple pigment genes (Chen et al., 2013). Xanthophores from medaka (Oryzias latipes) also showed a direct and rapid (within 30 s) aggregation response to light that was higher in the summer compared to the winter, which maximally responded to wavelengths of 410–420 nm (Oshima et al., 1998).
Non-Visual Light Detection in Cephalopods
In cephalopods, expansion of the chromatophores causes the pigment sac to stretch and decrease in thickness, thereby increasing the surface area of pigmentation and causing the skin to change color (Cloney and Florey, 1968). Expansion and contraction of the chromatophores in cephalopods is primarily controlled by visual input and information from the central nervous system (CNS) (Messenger, 2001), yet early studies suggested that dermal chromatophores could be stimulated directly by light. Chromatophores in isolated skin preparations of the opalescent inshore squid (Loligo opalescens, now renamed as Doryteuthis opalescens) undergo spontaneous pigment expansions that are enhanced by light when tissue samples have been kept in the dark for several hours (Florey, 1966). Similarly, the common octopus (Octopus vulgaris), can develop a mottled skin pattern within 1 s after exposure to a light pulse, following the removal of the supraoesophageal brain and in the absence of any optic input (Packard and Brancato, 1993). More recently, studies with isolated mantle sections of the California two-spot octopus (Octopus bimaculoides) have revealed that bright light initiates rapid expansion of the chromatophores, causing these organs to become fully expanded within 5 s of the onset of the light stimulus (Ramirez and Oakley, 2015). Furthermore, this “light-activated chromatophore expansion” response occurred most rapidly when blue light (470–480 nm) was used as the stimulus, when testing a wavelength range of 400–660 nm. The spectral sensitivity of the photopigment present in the eyes of O. vulgaris is maximally sensitive to 474 nm, which suggests that activation of the same opsin, and induction of a suitable phototransduction cascade in the skin, is a likely mechanism for localized light detection in this species (Ramirez and Oakley, 2015).
Mechanisms of Non-Visual Light Detection
Biologists have long noted that spectral sensitivities of multiple dermal light responses match that of the visual pigments utilized in the same organism, thus suggesting that the same photopigment/s might be involved (Steven, 1963). Such are the cases where both rod and cone pigments have been identified in dermal chromatophores in multiple species, as discussed above. Only relatively recently, however, have researchers begun to investigate the specific mechanisms of extraretinal photoreception, which is probably due to the apparent complexity of the underlying molecular pathways. In some cases, novel light transduction pathways have been elucidated; in Drosophila melanogaster larvae for example, a distinct class of sensory neurons (class IV dendritic aborization neurons) that cover almost the entire body wall are photosensitive, facilitating a unique mechanism of light detection (based on a gustatory G-protein coupled receptor, Gr28b) that mediates light avoidance behaviors (Xiang et al., 2010). In larval sponges such as Amphimedon queenslandica that do not possess opsins or a nervous system, phototactic swimming behavior may be mediated by cryptochromes, such as Aq-Cry2 that is expressed in the pigment ring eyes and has an absorption spectrum that is similar to that of phototactic behaviors (i.e., ~450 nm) (Rivera et al., 2012). Whether these non-opsin-based photosensory mechanisms function in ways that are unique to these organisms or are more widespread in the animal kingdom remains to be determined.
In marine annelids such as Nereis diversicolor and Platynereis dumerilii, dermal photoreceptors are present on the parapodia and can control the shadow reflex when the head is removed (Gwilliam, 1969). More recently, it has been shown that P. dumerilii possess non-cephalic photoreceptive cells that express “rhabdomeric-type” opsins (see below) that are orthologous to two pigment genes expressed in the teleost eye and other peripheral tissues (i.e., mammalian-like melanopsin opn4m2 and Xenopus-like melanopsin opn4x2), suggesting that non-cephalic photoreception may be more common than previously thought (Backfisch et al., 2013). In Xenopus laevis larvae, which have been well studied with respect to melanophore responses to environmental light, variation in skin pigmentation for background adaptation is regulated by light-dependent changes in the number of neurons in the deep brain that express the neurotransmitter dopamine (Dulcis and Spitzer, 2008). Recent work suggests that non-ocular photoreception may utilize both opsins and/or cryptochromes as photosensitive molecules; the isolated nervous system of X. laevis tadpoles produces swimming activity when stimulated by short-wavelength (400 nm) light; a motor response that appears to be mediated by neuropsin (opn5) and/or cryptochrome 1 (cry 1) in the caudal diencephalon (Currie et al., 2016). It is likely that a number of interacting photoreceptive molecules, combined with their respective underlying neural and hormonal pathways, contribute both to changes in animal coloration and diverse photosensitive behaviors.
An increasing number of studies suggest that non-visual light detection can be mediated by closely related opsin classes to those responsible for photoreception in the retina. For example, non-classical visual opsin-like pigments (sometimes referred to as “ciliary-type” or “c-opsins,” as opposed to “rhabdomeric-type” or “r-opsins”) are expressed in photoreceptor cells found in the tube feet, spines, and epidermis of sea urchins, and in the spines of brittle stars and starfish (Burke et al., 2006; Raible et al., 2006; Sodergren et al., 2006; Ullrich-Lüter et al., 2013; Delroisse et al., 2014, 2016), as well as classical non-visual opsin-like pigments (“rhabdomeric-like” or “r-opsins”) in sea urchin tube feet (Burke et al., 2006; Raible et al., 2006; Sodergren et al., 2006; Lesser et al., 2011). Several species of Hydra (members of Cnidaria, which contain sea anemones, corals, and jellyfish) lack eyes or ocelli, but possess a “rhodopsin-like” protein in the ectodermal layer (Musio et al., 2001). Specifically, the presence of opsins appears to induce a phototransduction cascade that regulates the discharge of the cnidocyte or “stinging cell,” where secretions are ejected for prey capture, defense, and locomotion (Kass-Simon and Scappaticci, 2002). Subsequent behavioral studies have revealed that cnidocyte discharge rates were significantly higher under dim light conditions than under bright light, suggesting that their function might be related to diurnal feeding cycles, the detection of shadows caused by prey, or phototaxis (Plachetzki et al., 2012).
In cephalopods, with probably the most mesmerizing displays of rapid color change mediated by chromatophores, several studies have revealed that opsins that are more related to craniate melanopsin (Davies et al., 2010, 2014) [which are also responsible for vision in molluscs and arthropods and confusingly labeled as “rhodopsins” (Davies et al., 2012a)], are also present in the skin of cuttlefish Sepia officinalis and O. bimaculoides, and the longfin squid (D. pealeii) (Kingston et al., 2015a; Kingston and Cronin, 2016). In S. officinalis, opsin transcripts detected in the dorsal fin are molecularly and functionally identical to invertebrate-type rhodopsins identified in the retina (Mäthger et al., 2010). Similarly, opsins present in the skin of the dorsal mantle of O. bimaculoides are almost identical to those expressed in the eyes of the animal (Ramirez and Oakley, 2015). However, to demonstrate that extraocular opsins function in photoreception, it is not only necessary to confirm the expression of these photosensitive molecules in other body regions, but to determine the presence of other components that are involved in the phototransduction signaling pathway. Unlike vertebrate visual phototransduction that comprises pigment activation of transducin (a G protein trimer), which in turn induces the activity of phosphodiesterase (PDE) and hyperpolarization of photoreceptors through closure of cyclic nucleotide-gated (CNG) ion channels (Fain et al., 2010; Lamb, 2013), the invertebrate visual signaling cascade involves Gq-type activation, phospholipase C (PLC), the messengers inositol 1,4,5-triphosphate (IP3), and diacylglycerol (DAG), which results in cellular depolarization mediated by the opening of transient receptor potential (TRP) channels (Montell, 2012). A recent study with several species of cuttlefish (S. officinalis, S. latimanus) and squid (D. pealeii) revealed that a number of these latter components were present in dermal chromatophores, including retinochrome (an invertebrate opsin-like protein that catalyzes the conversion of the chromophore from all-trans retinal to 11-cis retinal), G proteins, and TRP channels, with each mRNA transcript being identical to those found in the retina of these species (Kingston et al., 2015b). Similarly, G proteins and PLC have been identified in the skin of the octopus, O. bimaculoides (Speiser et al., 2014). Collectively, these studies provide good evidence that dermal opsins in invertebrate chromatophores respond to light independently of the CNS and use the same visual phototransduction cascade present in the retina. It is unclear, however, whether these same visual phototransduction pathways operate in the chromatophores of other animals that can change their coloration.
If light detection by the skin is mediated by the same visual opsins and phototransduction pathways that occur in the retina, the sensitivity of dermal photoreceptors to changes in light intensity will generally be quite limited, when compared to the vast natural variation in illumination that occurs during the diel light cycle. Indeed, natural light varies over 8 log units between sunlight and starlight (Land and Nilsson, 2012), and the photosensitivity of visual pigments is highly restricted by comparison to non-visual pigments (Figure 3). Specifically, simple non-directional photoreceptors, such as those present in the skin, are predicted to be sensitive to the entire intensity range of natural luminance. This suggests that there are other photosensitive pigments involved in irradiance detection, besides the visual opsins. Melanopsin, which is a light-sensitive pigment restricted to the RGCs of mammals (Provencio et al., 2000, 2002; Pires et al., 2007, 2009; Davies et al., 2010, 2012c, 2014; Hankins et al., 2014), but expressed in a multitude of tissues in most, if not all, non-mammalian taxa (Provencio et al., 1998; Bellingham et al., 2002, 2006; Drivenes et al., 2003; Jenkins et al., 2003; Chaurasia et al., 2005; Koyanagi et al., 2005; Frigato et al., 2006; Grone et al., 2007; Cheng et al., 2009; Davies et al., 2010, 2011, 2012b, 2014, 2015; Hankins et al., 2014), is a likely candidate as it was initially identified in amphibian dermal melanophores (Provencio et al., 1998). Indeed, the vertebrate melanopsin signaling pathway is very similar to that utilized by invertebrate retinal and dermal opsins (Isoldi et al., 2005; Contin et al., 2006; Graham et al., 2008), although there are notable differences (Panda et al., 2005; Peirson and Foster, 2006; Hughes et al., 2012; Davies et al., 2014). In X. laevis, light activates melanopsin and causes the dispersion of melanin within the melanophores (Isoldi et al., 2005). Melanopsin is known to regulate circadian rhythms by photoentrainment (Freedman et al., 1999; Semo et al., 2003) and may be present in the dermis in conjunction with visual opsins, thereby providing parallel light detection pathways to allow animals to discriminate among changes in irradiance associated with the time of day and those related to the local external environment (e.g., water depth, where both the intensity and spectral range of downwelling light diminish from the surface to the mesopelagic zone, Jerlov, 1976; Denton, 1990). This would also facilitate changes in coloration associated with photoperiod, which are relatively well known in lizards (Underwood, 1985; Binkley et al., 1987). Bearded dragons (Pogona vitticipe), for example, maintain a cyclic rhythm of skin color change that is maintained when the animals are held in constant darkness (Fan et al., 2014).
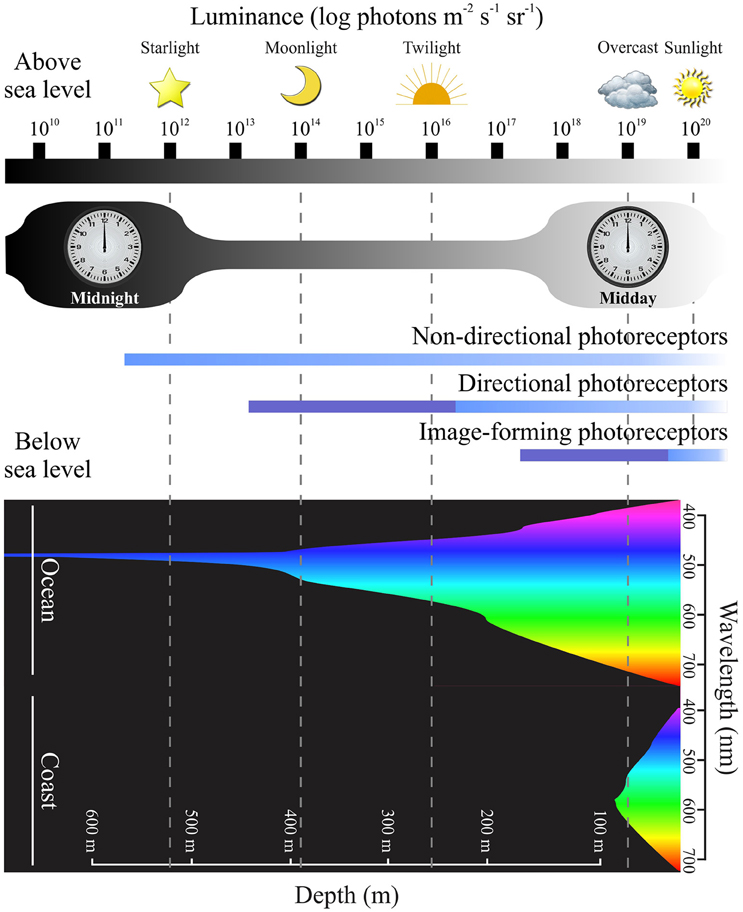
Figure 3. Photoreceptor sensitivity and environmental luminance. A diagram showing the range of light intensities present during the diel light cycle, with luminance plotted on a logarithmic scale over 10 log units (modified from Nilsson, 2009). In the upper part of the figure, changes in light levels are indicated at different time points (e.g., midday vs. midnight) and under diverse environmental conditions [e.g., from starlight (1012 photons m−2 s−1 sr−1) to sunlight (1020 photons m−2 s−1 sr−1]). The lower part of the figure demonstrates how downwelling light becomes less intense, with a more restricted spectral range, as water depth increases (Jerlov, 1976; Denton, 1990) in oceanic and coastal regions (modified from Speight and Henderson, 2010; Davies et al., 2012a). Equivalent luminance levels of light above water compared to below sea level are shown by dotted lines (modified from Nilsson, 2009). The functional range of different photoreceptor types are indicted in the middle of the figure, such that non-directional photoreceptors (e.g., those expressing melanopsin pigments) are operational over a broad range of light intensities compared to rods and cones that express visual pigments. Directional and image-forming photoreceptor membranes (i.e., outer segments) are arranged in stacked structures that permit an increase in photosensitivity (dark blue) compared to unstacked structures (light blue) (modified from Nilsson, 2009). Differences between photoreceptor sensitivities suggest that dermal chromatophores that contain (or co-express) visual and non-visual pigments might be able to detect a wide range of wavelengths and light intensities, thereby providing the molecular basis for body color changes as discussed in the main text.
Functions of Non-Visual Light Detection by the Skin
Photoreceptors that are not involved with image-forming vision have the potential to provide critical information about the spectral characteristic of any habitat within which an organism lives. At a fundamental level, the ability to detect changes in the intensity and wavelength of light can instruct a species with temporal information about the time of day, the weather (e.g., presence of cloud cover), the time of year, and the lunar cycle. In addition, the level of ambient light provides important cues regarding local surroundings, such as water depth and the presence of shading from nearby objects or other animals (e.g., predators or prey) (Shand and Foster, 1999; Nilsson, 2009). These essential cues allow animals to time their daily or seasonal activities: for example, to forage when diurnally migrating plankton are present, to stay hidden during the times of day when predators are active, or to remain buried or move to deeper waters at the time of day when the intensity of UV light reaches a peak (Nilsson, 2009). These simple sensory tasks require non-directional sensitivity to light and represent the simplest form of irradiance detection (Nilsson, 2009). Sensitivity to directional light is the next evolutionary step, serving as the basis for phototactic responses and the control of light-dependent body orientation (Nilsson, 2009). The advantages of phototactic responses include an better chance of encountering photosynthetic prey, increased probability of gamete encounters, and enhanced larval dispersal in marine invertebrates (reviewed by Jékely, 2009).
In complex organisms such as cephalopods, dermal photosensitivity may provide an explanation for how animals can rapidly adjust their coloration for both social signaling and camouflage, thereby providing optimal patterning despite not possessing classical ocular color vision (Mäthger et al., 2010). In the neon tetra, changes in the spectral properties of the iridocytes may allow fish to appear conspicuous during the day and cryptic at night (Lythgoe and Shand, 1982). Light detection by photoreceptors present in particularly vulnerable body parts, such as the tails of sea snakes, may aid their ability to detect dark crevices to remain concealed from predators (Zimmerman and Heatwole, 1990). Whichever behaviors are exhibited, individual chromatophores may function and sense light independently, or operate in conjunction with nearby receptors to provide regionalized light detection, thereby providing supplementary information to that received by the retina (Kingston et al., 2015b).
Conclusions
Non-visual light detection is likely to play an important role in the behavior of animals, yet information regarding function, as well as the pigments and phototransduction components involved, is generally lacking. While a great deal of work has highlighted the role of melanopsin in photoentrainment (e.g., the regulation of the sleep/wake cycle and the timing of seasonal reproductive events), there is a paucity of studies examining the function and regulation of other light-sensitive pigments. Although a number of investigations propose the involvement of some visual pigments in dermal photoreception, most of the non-visual opsin classes have been largely ignored.
Dermal light sensitivity is of particular importance as the skin is the primary surface encountered by photons. Additionally, the pigment cells that are sensitive to light (i.e., chromatophores) are not only responsible for generating animal coloration, but can be used to directly control changes in skin pigmentation. Thus, dermal light detection provides a direct link between the ambient light environment and the use of color patterns for functional roles such as thermoregulation, social signaling, and camouflage. The presence of spectrally distinct classes of photopigment in the chromatophores of some animals suggests that they may function to detect intensity and wavelength shifts in irradiance associated with the diurnal period, or at specific times of the day (e.g., dawn and dusk). This would allow animals to optimize changes in coloration according to the light environment at a given diel time point, month, or year. The direct control of chromatophore responses by light, however, could potentially conflict with the hormonal regulation of color patterns in specific contexts such as aggressive signaling (Kelley et al., 2016). Therefore, further studies are required to investigate the function and regulation of chromatophores, both in varying light environments and in different behavioral scenarios.
Author Contributions
All authors listed, have made substantial, direct and intellectual contribution to the work, and approved it for publication.
Conflict of Interest Statement
The authors declare that the research was conducted in the absence of any commercial or financial relationships that could be construed as a potential conflict of interest.
Acknowledgments
WD is supported by the Australian Research Council (FT110100176 and DP140102117). JK would like to acknowledge support from the University of Western Australia.
References
Armstrong, T. N., Cronin, T. W., and Bradley, B. P. (2000). Microspectrophotometric analysis of intact chromatophores of the Japanese medaka, Oryzias latipes. Pigment Cell Res. 13, 116–119. doi: 10.1034/j.1600-0749.2000.130210.x
Backfisch, B., Veedin Rajan, V. B., Fischer, R. M., Lohs, C., Arboleda, E., Tessmar-Raible, K., et al. (2013). Stable transgenesis in the marine annelid Platynereis dumerilii sheds new light on photoreceptor evolution. Proc. Natl. Acad. Sci. U.S.A. 110, 193–198. doi: 10.1073/pnas.1209657109
Bagnara, J. T., and Hadley, M. E. (1973). Chromatophores and Color Change. Englewood Cliffs, NJ: Prentice-Hall.
Bagnara, J. T., Taylor, J. D., and Hadley, M. E. (1968). The dermal chromatophore unit. J. Cell Biol. 38, 67–79. doi: 10.1083/jcb.38.1.67
Ban, E., Kasai, A., Sato, M., Yokozeki, A., Hisatomi, O., and Oshima, N. (2005). The signaling pathway in photoresponses that may be mediated by visual pigments in erythrophores of Nile tilapia. Pigment Cell Res. 18, 360–369. doi: 10.1111/j.1600-0749.2005.00267.x
Bellingham, J., Chaurasia, S. S., Melyan, Z., Liu, C., Cameron, M. A., Tarttelin, E. E., et al. (2006). Evolution of melanopsin photoreceptors: discovery and characterization of a new melanopsin in nonmammalian vertebrates. PLoS Biol. 4:e254. doi: 10.1371/journal.pbio.0040254
Bellingham, J., Whitmore, D., Philp, A. R., Wells, D. J., and Foster, R. G. (2002). Zebrafish melanopsin: isolation, tissue localisation and phylogenetic position. Brain Res. Mol. Brain Res. 107, 128–136. doi: 10.1016/S0169-328X(02)00454-0
Binkley, S., Reilly, K. B., Hermida, V., and Mosher, K. (1987). Circadian rhythm of color change in Anolis carolinensis: reconsideration of regulation, especially the role of melatonin in dark-time pallor. Pineal Res. 5, 133–151.
Burke, R. D., Angerer, L. M., Elphick, M. R., Humphrey, G. W., Yaguchi, S., Kiyama, T., et al. (2006). A genomic view of the sea urchin nervous system. Dev. Biol. 300, 434–460. doi: 10.1016/j.ydbio.2006.08.007
Caswell, H. H. (1950). Rhythmic color change in the lizard Xantusia vigilis. Copeia 1950, 87–91. doi: 10.2307/1438950
Cavallari, N., Frigato, E., Vallone, D., Fröhlich, N., Lopez-Olmeda, J. F., Foà, A., et al. (2011). A blind circadian clock in cavefish reveals that opsins mediate peripheral clock photoreception. PLoS Biol. 9:e1001142. doi: 10.1371/journal.pbio.1001142
Chaurasia, S. S., Rollag, M. D., Jiang, G., Hayes, W. P., Haque, R., Natesan, A., et al. (2005). Molecular cloning, localization and circadian expression of chicken melanopsin (Opn4): differential regulation of expression in pineal and retinal cell types. J. Neurochem. 92, 158–170. doi: 10.1111/j.1471-4159.2004.02874.x
Chen, S. C., Hornsby, M. A., Robertson, R. M., and Hawryshyn, C. W. (2014). The influence of chromatic background on the photosensitivity of tilapia erythrophores. Biol. Open 3, 117–120. doi: 10.1242/bio.20146742
Chen, S. C., Robertson, R. M., and Hawryshyn, C. W. (2013). Possible involvement of cone opsins in distinct photoresponses of intrinsically photosensitive dermal chromatophores in tilapia Oreochromis niloticus. PLoS ONE 8:e70342. doi: 10.1371/journal.pone.0070342
Chen, S. C., Xiao, C., Troje, N. F., Robertson, R. M., and Hawryshyn, C. W. (2015). Functional characterisation of the chromatically antagonistic photosensitive mechanism of erythrophores in the tilapia Oreochromis niloticus. J. Exp. Biol. 218, 748–756. doi: 10.1242/jeb.106831
Cheng, N., Tsunenari, T., and Yau, K. W. (2009). Intrinsic light response of retinal horizontal cells of teleosts. Nature 460, 899–903. doi: 10.1038/nature08175
Cloney, R. A., and Florey, E. (1968). Ultrastructure of cephalopod chromatophore organs. Cell Tissue Res. 89, 250–280. doi: 10.1007/bf00347297
Contin, M. A., Verra, D. M., and Guido, M. E. (2006). An invertebrate-like phototransduction cascade mediates light detection in the chicken retinal ganglion cells. FASEB J. 20, 2648–2650. doi: 10.1096/fj.06-6133fje
Currie, S. P., Doherty, G. H., and Sillar, K. T. (2016). Deep-brain photoreception links luminance detection to motor output in Xenopus frog tadpoles. Proc. Natl. Acad. Sci. U.S.A. 113, 6053–6058. doi: 10.1073/pnas.1515516113
Davies, W. I., Collin, S. P., and Hunt, D. M. (2012a). Molecular ecology and adaptation of visual photopigments in craniates. Mol. Ecol. 21, 3121–3158. doi: 10.1111/j.1365-294X.2012.05617.x
Davies, W. I., Foster, R. G., and Hankins, M. W. (2014). The Evolution and Function of Melanopsin in Craniates. New York, NY: Springer.
Davies, W. I., Tamai, T. K., Zheng, L., Fu, J. K., Rihel, J., Foster, R. G., et al. (2015). An extended family of novel vertebrate photopigments is widely expressed and displays a diversity of function. Genome Res. 25, 1666–1679. doi: 10.1101/gr.189886.115
Davies, W. I., Tay, B. H., Zheng, L., Danks, J. A., Brenner, S., Foster, R. G., et al. (2012b). Evolution and functional characterisation of Melanopsins in a deep-sea chimaera (elephant shark, Callorhinchus milii). PLoS ONE 7:e51276. doi: 10.1371/journal.pone.0051276
Davies, W. I., Zheng, L., Hughes, S., Tamai, T. K., Turton, M., Halford, S., et al. (2011). Functional diversity of melanopsins and their global expression in the teleost retina. Cell. Mol. Life Sci. 68, 4115–4132. doi: 10.1007/s00018-011-0785-4
Davies, W. L., Foster, R. G., and Hankins, M. W. (2012c). Focus on molecules: melanopsin. Exp. Eye Res. 97, 161–162. doi: 10.1016/j.exer.2010.07.020
Davies, W. L., Hankins, M. W., and Foster, R. G. (2010). Vertebrate ancient opsin and melanopsin: divergent irradiance detectors. Photochem. Photobiol. Sci. 9, 1444–1457. doi: 10.1039/c0pp00203h
de Assis, L. V., Moraes, M. N., Da Silveira Cruz-Machado, S., and Castrucci, A. M. (2016). The effect of white light on normal and malignant murine melanocytes: a link between opsins, clock genes, and melanogenesis. Biochim. Biophys. Acta 1863, 1119–1133. doi: 10.1016/j.bbamcr.2016.03.001
Delroisse, J., Mallefet, J., and Flammang, P. (2016). De novo adult transcriptomes of two european brittle stars: spotlight on opsin-based photoreception. PLoS ONE 11:e0152988. doi: 10.1371/journal.pone.0152988
Delroisse, J., Ullrich-Lüter, E., Ortega-Martinez, O., Dupont, S., Arnone, M. I., Mallefet, J., et al. (2014). High opsin diversity in a non-visual infaunal brittle star. BMC Genomics 15:1035. doi: 10.1186/1471-2164-15-1035
Denton, E. J. (1990). Light and Vision at Depths Greater Than 200 m. Cambridge: Cambridge University Press.
Drivenes, Ø., Søviknes, A. M., Ebbesson, L. O., Fjose, A., Seo, H. C., and Helvik, J. V. (2003). Isolation and characterization of two teleost melanopsin genes and their differential expression within the inner retina and brain. J. Comp. Neurol. 456, 84–93. doi: 10.1002/cne.10523
Dulcis, D., and Spitzer, N. C. (2008). Illumination controls differentiation of dopamine neurons regulating behaviour. Nature 456, 195–201. doi: 10.1038/nature07569
Epp, L. G. (1972). Development of pigmentation in the eyeless mutant of the Mexican axolotl, Ambystoma mexicanum, Shaw. J. Exp. Zool. 181, 169–180. doi: 10.1002/jez.1401810204
Fain, G. L., Hardie, R., and Laughlin, S. B. (2010). Phototransduction and the evolution of photoreceptors. Curr. Biol. 20, R114–124. doi: 10.1016/j.cub.2009.12.006
Fan, M., Stuart-Fox, D., and Cadena, V. (2014). Cyclic colour change in the bearded dragon Pogona vitticeps under different photoperiods. PLoS ONE 9:e111504. doi: 10.1371/journal.pone.0111504
Fernandes, A. M., Fero, K., Arrenberg, A. B., Bergeron, S. A., Driever, W., and Burgess, H. A. (2012). Deep brain photoreceptors control light-seeking behavior in zebrafish larvae. Curr. Biol. 22, 2042–2047. doi: 10.1016/j.cub.2012.08.016
Florey, E. (1966). Nervous control and spontaneous activity of the chromatophores of a cephalopod, Loligo opalescens. Comp. Biochem. Physiol. 18, 305–324. doi: 10.1016/0010-406X(66)90189-7
Foster, R. G., and Hankins, M. W. (2002). Non-rod, non-cone photoreception in the vertebrates. Prog. Retin. Eye Res. 21, 507–527. doi: 10.1016/S1350-9462(02)00036-8
Freedman, M. S., Lucas, R. J., Soni, B., Von Schantz, M., Muñoz, M., David-Gray, Z., et al. (1999). Regulation of mammalian circadian behavior by non-rod, non-cone, ocular photoreceptors. Science 284, 502–504. doi: 10.1126/science.284.5413.502
Frigato, E., Vallone, D., Bertolucci, C., and Foulkes, N. S. (2006). Isolation and characterization of melanopsin and pinopsin expression within photoreceptive sites of reptiles. Naturwissenschaften 93, 379–385. doi: 10.1007/s00114-006-0119-9
Fujii, R. (1993). “Cytophysiology of fish chromatophores,” in International Review of Cytology, eds K. Jeon, M. Friedlander, and J. Jarvik (Amsterdam: Academic Press), 191–255.
Fujii, R. (2000). The regulation of motile activity in fish chromatophores. Pigment cell research/sponsored by the European Society for Pigment Cell Research and the International Pigment Cell Soc. 13, 300–319. doi: 10.1034/j.1600-0749.2000.130502.x
Fujii, R., and Oshima, N. (1986). Control of chromatophore movements in teleost fishes. Zool. Sci. 3, 13–47.
Fulgione, D., Trapanese, M., Maselli, V., Rippa, D., Itri, F., Avallone, B., et al. (2014). Seeing through the skin: dermal light sensitivity provides cryptism in moorish gecko. J. Zool. 294, 122–128. doi: 10.1111/jzo.12159
Graham, D. M., Wong, K. Y., Shapiro, P., Frederick, C., Pattabiraman, K., and Berson, D. M. (2008). Melanopsin ganglion cells use a membrane-associated rhabdomeric phototransduction cascade. J. Neurophysiol. 99, 2522–2532. doi: 10.1152/jn.01066.2007
Grether, G. F., Kolluru, G. R., and Nersissian, K. (2004). Individual colour patches as multicomponent signals. Biol. Rev. Camb. Philos. Soc. 79, 583–610. doi: 10.1017/S1464793103006390
Grone, B. P., Sheng, Z., Chen, C. C., and Fernald, R. D. (2007). Localization and diurnal expression of melanopsin, vertebrate ancient opsin, and pituitary adenylate cyclase-activating peptide mRNA in a teleost retina. J. Biol. Rhythms 22, 558–561. doi: 10.1177/0748730407308285
Gross, J. B., Powers, A. K., Davis, E. M., and Kaplan, S. A. (2016). A pleiotropic interaction between vision loss and hypermelanism in Astyanax mexicanus cave x surface hybrids. BMC Evol. Biol. 16:145. doi: 10.1186/s12862-016-0716-y
Guertin, S., and Kass-Simon, G. (2015). Extraocular spectral photosensitivity in the tentacles of Hydra vulgaris. Comp. Biochem. Physiol. A Mol. Integr. Physiol. 184, 163–170. doi: 10.1016/j.cbpa.2015.02.016
Gwilliam, G. F. (1969). Electrical responses to photic stimulation in the eyes and nervous system of nereid polychaetes. Biol. Bull. 136, 385–397. doi: 10.2307/1539683
Haltaufderhyde, K., Ozdeslik, R. N., Wicks, N. L., Najera, J. A., and Oancea, E. (2015). Opsin expression in human epidermal skin. Photochem. Photobiol. 91, 117–123. doi: 10.1111/php.12354
Hankins, M. W., Davies, W. I., and Foster, R. G. (2014). The Evolution of Non-visual Photopigments in the Central Nervous System of Vertebrates. New York, NY: Springer.
Hiroyuki, N., Ikuo, T., and Tetsuro, I. (1988). Spectral sensitivity of melanophores of a freshwater teleost, Zacco temmincki. Comp. Biochem. Physiol. A Physiol. 90, 147–149. doi: 10.1016/0300-9629(88)91020-1
Hughes, S., Hankins, M. W., Foster, R. G., and Peirson, S. N. (2012). Melanopsin phototransduction: slowly emerging from the dark. Prog. Brain Res. 199, 19–40. doi: 10.1016/B978-0-444-59427-3.00002-2
Iga, T., and Takabatake, I. (1983). Melanophores of Zacco temmincki (Teleostei) are light sensitive. J. Exp. Zool. 227, 9–14. doi: 10.1002/jez.1402270103
Imai, K., and Takahashi, H. (1971). Changes in the melanophorotropic function of the pituitary gland accompanying blindness in Xenopus laevis daudin. Dev. Growth Differ. 12, 253–264. doi: 10.1111/j.1440-169X.1971.00253.x
Isoldi, M. C., Rollag, M. D., Castrucci, A. M., and Provencio, I. (2005). Rhabdomeric phototransduction initiated by the vertebrate photopigment melanopsin. Proc. Natl. Acad. Sci. U.S.A. 102, 1217–1221. doi: 10.1073/pnas.0409252102
Jeffery, W. R. (2009a). Chapter 8. Evolution and development in the cavefish Astyanax. Curr. Top. Dev. Biol. 86, 191–221. doi: 10.1016/S0070-2153(09)01008-4
Jeffery, W. R. (2009b). Regressive evolution in Astyanax cavefish. Annu. Rev. Genet. 43, 25–47. doi: 10.1146/annurev-genet-102108-134216
Jékely, G. (2009). Evolution of phototaxis. Philos. Trans. R. Soc. Lond. B Biol. Sci. 364, 2795–2808. doi: 10.1098/rstb.2009.0072
Jenkins, A., Muñoz, M., Tarttelin, E. E., Bellingham, J., Foster, R. G., and Hankins, M. W. (2003). VA opsin, melanopsin, and an inherent light response within retinal interneurons. Curr. Biol. 13, 1269–1278. doi: 10.1016/S0960-9822(03)00509-8
Kasai, A., and Oshima, N. (2006). Light-sensitive motile iridophores and visual pigments in the neon tetra, Paracheirodon innesi. Zool. Sci. 23, 815–819. doi: 10.2108/zsj.23.815
Kass-Simon, G., and Scappaticci, J. A. A. (2002). The behavioral and developmental physiology of nematocysts. Can. J. Zool. 80, 1772–1794. doi: 10.1139/z02-135
Kay, J. N., Finger-Baier, K. C., Roeser, T., Staub, W., and Baier, H. (2001). Retinal ganglion cell genesis requires lakritz, a Zebrafish atonal Homolog. Neuron 30, 725–736. doi: 10.1016/S0896-6273(01)00312-9
Kelley, J. L., Rodgers, G. M., and Morrell, L. J. (2016). Conflict between background matching and social signalling in a colour-changing freshwater fish. R. Soc. Open Sci. 3:160040. doi: 10.1098/rsos.160040
Kim, H. J., Son, E. D., Jung, J. Y., Choi, H., Lee, T. R., and Shin, D. W. (2013). Violet light down-regulates the expression of specific differentiation markers through Rhodopsin in normal human epidermal keratinocytes. PLoS ONE 8:e73678. doi: 10.1371/journal.pone.0073678
Kingston, A. C., and Cronin, T. W. (2016). Diverse distributions of extraocular opsins in crustaceans, cephalopods, and fish. Integr. Comp. Biol. doi: 10.1093/icb/icw022. [Epub ahead of print].
Kingston, A. C. N., Kuzirian, A. M., Hanlon, R. T., and Cronin, T. W. (2015b). Visual phototransduction components in cephalopod chromatophores suggest dermal photoreception. J. Exp. Biol. 218, 1596–1602. doi: 10.1242/jeb.117945
Kingston, A. C., Wardill, T. J., Hanlon, R. T., and Cronin, T. W. (2015a). An unexpected diversity of photoreceptor classes in the longfin squid, Doryteuthis pealeii. PLoS ONE 10:e0135381. doi: 10.1371/journal.pone.0135381
Kojima, D., Mori, S., Torii, M., Wada, A., Morishita, R., and Fukada, Y. (2011). UV-sensitive photoreceptor protein OPN5 in humans and mice. PLoS ONE 6:e26388. doi: 10.1371/journal.pone.0026388
Koyanagi, M., Kubokawa, K., Tsukamoto, H., Shichida, Y., and Terakita, A. (2005). Cephalochordate melanopsin: evolutionary linkage between invertebrate visual cells and vertebrate photosensitive retinal ganglion cells. Curr. Biol. 15, 1065–1069. doi: 10.1016/j.cub.2005.04.063
Lamb, T. D. (2013). Evolution of phototransduction, vertebrate photoreceptors and retina. Prog. Retin. Eye Res. 36, 52–119. doi: 10.1016/j.preteyeres.2013.06.001
Langecker, T. G. (1989). Studies on the light reaction of epigean and cave populations of Astyanax fasciatus (Characidae, Pisces). Mem. Biospeol. 16, 169–176.
Lesser, M. P., Carleton, K. L., Böttger, S. A., Barry, T. M., and Walker, C. W. (2011). Sea urchin tube feet are photosensory organs that express a rhabdomeric-like opsin and PAX6. Proc. Biol. Sci. 278, 3371–3379. doi: 10.1098/rspb.2011.0336
Lucas, R. J., Douglas, R. H., and Foster, R. G. (2001). Characterization of an ocular photopigment capable of driving pupillary constriction in mice. Nat. Neurosci. 4, 621–626. doi: 10.1038/88443
Lythgoe, J. N., and Shand, J. (1982). Changes in spectral reflexions from the iridophores of the neon tetra. J. Physiol. 325, 23–34. doi: 10.1113/jphysiol.1982.sp014132
Lythgoe, J. N., Shand, J., and Foster, R. G. (1984). Visual pigment in fish iridocytes. Nature 308, 83–84. doi: 10.1038/308083a0
Mäthger, L. M., Roberts, S. B., and Hanlon, R. T. (2010). Evidence for distributed light sensing in the skin of cuttlefish, Sepia officinalis. Biol. Lett. 6, 600–603. doi: 10.1098/rsbl.2010.0223
Menaker, M., Roberts, R., Elliott, J., and Underwood, H. (1970). Extraretinal light perception in the sparrow. 3. The eyes do not participate in photoperiodic photoreception. Proc. Natl. Acad. Sci. U.S.A. 67, 320–325. doi: 10.1073/pnas.67.1.320
Meng, F., Zhao, Y., Postlethwait, J. H., and Zhang, C. (2013). Differentially-expressed opsin genes identified in Sinocyclocheilus cavefish endemic to China. Curr. Zool. 59, 170–174. doi: 10.1093/czoolo/59.2.170
Messenger, J. B. (2001). Cephalopod chromatophores: neurobiology and natural history. Biol. Rev. Camb. Philos. Soc. 76, 473–528. doi: 10.1017/S1464793101005772
Montell, C. (2012). Drosophila visual transduction. Trends Neurosci. 35, 356–363. doi: 10.1016/j.tins.2012.03.004
Musio, C., Santillo, S., Taddei-Ferretti, C., Robles, L. J., Vismara, R., Barsanti, L., et al. (2001). First identification and localization of a visual pigment in Hydra (Cnidaria, Hydrozoa). J. Comp. Physiol. A 187, 79–81. doi: 10.1007/s003590100180
Nilsson, D.-E. (2009). The evolution of eyes and visually guided behaviour. Philos. Trans. R. Soc. Lond. B Biol. Sci. 364, 2833–2847. doi: 10.1098/rstb.2009.0083
Oshima, N. (2001). Direct reception of light by chromatophores of lower vertebrates. Pigment Cell Res. 14, 312–319. doi: 10.1034/j.1600-0749.2001.140502.x
Oshima, N., Nakata, E., Ohta, M., and Kamagata, S. (1998). Light-induced pigment aggregation in xanthophores of the medaka, Oryzias latipes. Pigment Cell Res. 11, 362–367. doi: 10.1111/j.1600-0749.1998.tb00495.x
Oshima, N., and Yokozeki, A. (1999). Direct control of pigment aggregation and dispersion in tilapia erythrophores by light. Zool. Sci. 16, 51–54. doi: 10.2108/zsj.16.51
Packard, A., and Brancato, D. (1993). Some responses of Octopus chromatophores to light. J. Physiol. 459, 429.
Panda, S., Nayak, S. K., Campo, B., Walker, J. R., Hogenesch, J. B., and Jegla, T. (2005). Illumination of the melanopsin signaling pathway. Science 307, 600–604. doi: 10.1126/science.1105121
Parker, G. H. (1948). Animal Colour Changes and Their Neurohumours. Cambridge: Cambridge University Press.
Peirson, S., and Foster, R. G. (2006). Melanopsin: another way of signaling light. Neuron 49, 331–339. doi: 10.1016/j.neuron.2006.01.006
Pires, S. S., Hughes, S., Turton, M., Melyan, Z., Peirson, S. N., Zheng, L., et al. (2009). Differential expression of two distinct functional isoforms of melanopsin (Opn4) in the mammalian retina. J. Neurosci. 29, 12332–12342. doi: 10.1523/JNEUROSCI.2036-09.2009
Pires, S. S., Shand, J., Bellingham, J., Arrese, C., Turton, M., Peirson, S., et al. (2007). Isolation and characterization of melanopsin (Opn4) from the Australian marsupial Sminthopsis crassicaudata (fat-tailed dunnart). Proc. Biol. Sci. 274, 2791–2799. doi: 10.1098/rspb.2007.0976
Plachetzki, D. C., Fong, C. R., and Oakley, T. H. (2012). Cnidocyte discharge is regulated by light and opsin-mediated phototransduction. BMC Biol. 10:17. doi: 10.1186/1741-7007-10-17
Provencio, I., Jiang, G., De Grip, W. J., Hayes, W. P., and Rollag, M. D. (1998). Melanopsin: an opsin in melanophores, brain, and eye. Proc. Natl. Acad. Sci. U.S.A. 95, 340–345. doi: 10.1073/pnas.95.1.340
Provencio, I., Rodriguez, I. R., Jiang, G., Hayes, W. P., Moreira, E. F., and Rollag, M. D. (2000). A novel human opsin in the inner retina. J. Neurosci. 20, 600–605.
Provencio, I., Rollag, M. D., and Castrucci, A. M. (2002). Photoreceptive net in the mammalian retina. This mesh of cells may explain how some blind mice can still tell day from night. Nature 415, 493. doi: 10.1038/415493a
Raible, F., Tessmar-Raible, K., Arboleda, E., Kaller, T., Bork, P., Arendt, D., et al. (2006). Opsins and clusters of sensory G-protein-coupled receptors in the sea urchin genome. Dev. Biol. 300, 461–475. doi: 10.1016/j.ydbio.2006.08.070
Ramirez, M. D., and Oakley, T. H. (2015). Eye-independent, light-activated chromatophore expansion (LACE) and expression of phototransduction genes in the skin of Octopus bimaculoides. J. Exp. Biol. 218, 1513–1520. doi: 10.1242/jeb.110908
Rea, M. S., Bullough, J. D., and Figueiro, M. G. (2002). Phototransduction for human melatonin suppression. J. Pineal Res. 32, 209–213. doi: 10.1034/j.1600-079X.2002.01881.x
Rivera, A. S., Ozturk, N., Fahey, B., Plachetzki, D. C., Degnan, B. M., Sancar, A., et al. (2012). Blue-light-receptive cryptochrome is expressed in a sponge eye lacking neurons and opsin. J. Exp. Biol. 215, 1278–1286. doi: 10.1242/jeb.067140
Semo, M., Peirson, S., Lupi, D., Lucas, R. J., Jeffery, G., and Foster, R. G. (2003). Melanopsin retinal ganglion cells and the maintenance of circadian and pupillary responses to light in aged rodless/coneless (rd/rd cl) mice. Eur. J. Neurosci. 17, 1793–1801. doi: 10.1046/j.1460-9568.2003.02616.x
Shand, J., and Foster, R. G. (1999). “The extraretinal photoreceptors of non-mammalian vertebrates,” in Adaptive Mechanisms in the Ecology of Vision, eds S. N. Archer, M. B. A. Djamgoz, E. R. Loew, J. C. Partridge, and S. Vallerga (Dordrecht: Kluwer Academic Publishers), 197–222.
Shiraki, T., Kojima, D., and Fukada, Y. (2010). Light-induced body color change in developing zebrafish. Photochem. Photobiol. Sci. 9, 1498–1504. doi: 10.1039/c0pp00199f
Sköld, H. N., Aspengren, S., Cheney, K. L., and Wallin, M. (2016). Fish chromatophores–from molecular motors to animal behavior. Int. Rev. Cell Mol. Biol. 321, 171–219. doi: 10.1016/bs.ircmb.2015.09.005
Sodergren, E., Weinstock, G. M., Davidson, E. H., Cameron, R. A., Gibbs, R. A., Angerer, R. C., et al. (2006). The genome of the sea urchin Strongylocentrotus purpuratus. Science 314, 941–952. doi: 10.1126/science.1133609
Speight, M. R., and Henderson, P. A. (2010). Marine Ecology: Concepts and Applications. Chichester: Wiley-Blackwell.
Speiser, D. I., Pankey, M. S., Zaharoff, A. K., Battelle, B. A., Bracken-Grissom, H. D., Breinholt, J. W., et al. (2014). Using phylogenetically-informed annotation (PIA) to search for light-interacting genes in transcriptomes from non-model organisms. BMC Bioinformatics 15:350. doi: 10.1186/s12859-014-0350-x
Steven, D. M. (1963). The dermal light sense. Biol. Rev. Camb. Philos. Soc. 38, 204–240. doi: 10.1111/j.1469-185X.1963.tb00783.x
Stevens, M. (2016). Color change, phenotypic plasticity, and camouflage. Front. Ecol. Evol. 4:51. doi: 10.3389/fevo.2016.00051
Stevens, M., Lown, A. E., and Wood, L. E. (2014a). Camouflage and individual variation in shore crabs (Carcinus maenas) from different habitats. PLoS ONE 9:e115586. doi: 10.1371/journal.pone.0115586
Stevens, M., Lown, A. E., and Wood, L. E. (2014b). Color change and camouflage in juvenile shore crabs Carcinus maenas. Front. Ecol. Evol. 2:14. doi: 10.3389/fevo.2014.00014
Stevens, M., and Merilaita, S. (2009). Animal camouflage: current issues and new perspectives. Philos. Trans. R. Soc. Lond. B. Biol. Sci. 364, 423–427. doi: 10.1098/rstb.2008.0217
Stuart-Fox, D., and Moussalli, A. (2009). Camouflage, communication and thermoregulation: lessons from colour changing organisms. Philos. Trans. R. Soc. Lond. B. Biol. Sci. 364, 463–470. doi: 10.1098/rstb.2008.0254
Sugimoto, M. (2002). Morphological color change in fish: regulation of pigment cell density and morphology. Microsc. Res. Tech. 58, 496–503. doi: 10.1002/jemt.10168
Sumner, F. B. (1935a). Evidence for the protective value of changeable coloration in fishes. American Naturalist 69, 245–266. doi: 10.1086/280597
Sumner, F. B. (1935b). Studies of protective color change: III Experiments with fishes both as predators and prey. Proc. Natl. Acad. Sci. U.S.A. 21, 345–353. doi: 10.1073/pnas.21.6.345
Tarttelin, E. E., Frigato, E., Bellingham, J., Di Rosa, V., Berti, R., Foulkes, N. S., et al. (2012). Encephalic photoreception and phototactic response in the troglobiont Somalian blind cavefish Phreatichthys andruzzii. J. Exp. Biol. 215, 2898–2903. doi: 10.1242/jeb.071084
Timmermann, M., and Plath, M. (2009). Phototactic response and light sensitivity in an epigean and a hypogean population of a barb (Garra barreimiae, Cyprinidae). Aquatic Ecol. 43, 539–547. doi: 10.1007/s10452-008-9173-z
Tobler, M., Coleman, S. W., Perkins, B. D., and Rosenthal, G. G. (2010). Reduced opsin gene expression in a cave-dwelling fish. Biol. Lett. 6, 98–101. doi: 10.1098/rsbl.2009.0549
Tsutsumi, M., Ikeyama, K., Denda, S., Nakanishi, J., Fuziwara, S., Aoki, H., et al. (2009). Expressions of rod and cone photoreceptor-like proteins in human epidermis. Exp. Dermatol. 18, 567–570. doi: 10.1111/j.1600-0625.2009.00851.x
Ullrich-Lüter, E. M., D'aniello, S., and Arnone, M. I. (2013). C-opsin expressing photoreceptors in echinoderms. Integr. Comp. Biol. 53, 27–38. doi: 10.1093/icb/ict050
Umbers, K. D. L., Fabricant, S. A., Gawryszewski, F. M., Seago, A. E., and Herberstein, M. E. (2014). Reversible colour change in Arthropoda. Biol. Rev. 89, 820–848. doi: 10.1111/brv.12079
Underwood, H. (1985). Pineal melatonin rhythms in the lizardAnolis carolinensis: effects of light and temperature cycles. J. Comp. Physiol. A 157, 57–65. doi: 10.1007/BF00611095
Vigh, B., Manzano, M. J., Zádori, A., Frank, C. L., Lukats, A., Röhlich, P., et al. (2002). Nonvisual photoreceptors of the deep brain, pineal organs and retina. Histol. Histopathol. 17, 555–590.
von Frisch, K. (1911). Beitrage zur physiologie der pigmentzellen in der fischhaut. Pflug. Arch. Eur. J. Physiol. 138, 319–387. doi: 10.1007/BF01680752
Wolken, J. J. (1988). Photobehavior of marine invertebrates: extraocular photoreception. Comp. Biochem. Physiol. C Comp. Pharmacol. 91, 145–149. doi: 10.1016/0742-8413(88)90180-6
Wolken, J. J., and Mogus, M. A. (1979). Extra-ocular photosensitivity. Photochem. Photobiol. 29, 189–196. doi: 10.1111/j.1751-1097.1979.tb09281.x
Xiang, Y., Yuan, Q., Vogt, N., Looger, L. L., Jan, L. Y., and Jan, Y. N. (2010). Light-avoidance-mediating photoreceptors tile the Drosophila larval body wall. Nature 468, 921–926. doi: 10.1038/nature09576
Yang, J., Chen, X., Bai, J., Fang, D., Qiu, Y., Jiang, W., et al. (2016). The Sinocyclocheilus cavefish genome provides insights into cave adaptation. BMC Biol. 14:1. doi: 10.1186/s12915-015-0223-4
Keywords: photosensitivity, skin, chromatophore, aggregation, dispersion, opsin, camouflage, background matching
Citation: Kelley JL and Davies WIL (2016) The Biological Mechanisms and Behavioral Functions of Opsin-Based Light Detection by the Skin. Front. Ecol. Evol. 4:106. doi: 10.3389/fevo.2016.00106
Received: 17 May 2016; Accepted: 16 August 2016;
Published: 30 August 2016.
Edited by:
Chuan-Chin Chiao, National Tsing Hua University, TaiwanReviewed by:
Ronald Hamilton Douglas, City University London, UKKristin Tessmar-Raible, University of Vienna, Austria
Copyright © 2016 Kelley and Davies. This is an open-access article distributed under the terms of the Creative Commons Attribution License (CC BY). The use, distribution or reproduction in other forums is permitted, provided the original author(s) or licensor are credited and that the original publication in this journal is cited, in accordance with accepted academic practice. No use, distribution or reproduction is permitted which does not comply with these terms.
*Correspondence: Jennifer L. Kelley, amVubmlmZXIua2VsbGV5QHV3YS5lZHUuYXU=