- 1Department of Entomology and Wildlife Ecology, University of Delaware, Newark, DE, USA
- 2US Forest Service, Northern Research Station, Newark, DE, USA
- 3Department of Mathematical Modeling, Statistics and Bioinformatics, Ghent University, Ghent, Belgium
Loss of habitat to urbanization is a primary cause of population declines as human-dominated landscapes expand at increasing rates. Understanding how the relative effects of different conservation strategies is important to slow population declines for species in urban landscapes. We studied the wood thrush Hylocichla mustelina, a declining forest-breeding Neotropical migratory species, and umbrella species for forest-breeding songbirds, within the urbanized mid-Atlantic United States. We integrated 40 years of demographic data with contemporary metapopulation model simulations of breeding wood thrushes to predict population responses to differing conservation scenarios. We compared four conservation scenarios over a 30-year time period (2014–2044) representing (A) current observed state (Null), (B) replacing impervious surface with forest (Reforest), (C) reducing brown-headed cowbird Molothrus ater parasitism pressure (Cowbird removal), and (D) simultaneous reforesting and cowbird removal. Compared to the Null scenario, the Reforest scenario increased mean annual population trends by 54%, the Remove cowbirds scenario increased mean annual population trends by 38%, and the scenario combining reforestation and cowbird removal increased mean annual population trends by 98%. Mean annual growth rates (λ) per site were greater in the Reforest (λ = 0.94) and Remove cowbirds (λ = 0.92) compared to the Null (λ = 0.88) model scenarios. However, only by combining the positive effects of reforestation and cowbird removal did wood thrush populations stop declining (λ = 1.00). Our results suggest that independently replacing impervious surface with forest habitat around forest patches and removing cowbirds may slow current negative population trends. Furthermore, conservation efforts that combine reforestation and cowbird removal may potentially benefit populations of wood thrushes and other similarly forest-breeding songbird species within urbanized fragmented landscapes that typify the mid-Atlantic United States.
Introduction
The conservation of species is faced with overcoming multiple anthropogenic factors that can negatively affect populations across multiple scales (Jarzyna et al., 2015). Understanding population responses to regional and local factors is essential for defining priorities and achieving conservation goals (Walsh et al., 2015). Using empirically-based integrated population models (IPM; Abadi et al., 2010), predictive site occupancy models (Meineri et al., 2015), and individual-based metapopulation models (Hanski and Gaggiotti, 2004) can improve our understanding and predictions of population responses to conservation efforts. Additionally, spatially-explicit metapopulation models and their extensions (e.g., source-sink models) have been successfully used for this problem, particularly in fragmented landscapes (Pulliam, 1988; Hanski, 1999). Metapopulation ecology has provided a powerful set of tools for applied conservation (Soulé et al., 1988), and is ideally suited for modeling population responses to anthropogenic stressors such as urbanization, habitat loss, and fragmentation (Kawecki, 2004). Particularly in the face of rapidly changing landscapes due to anthropogenic factors, these approaches can help increase the accuracy of population estimates across spatial scales, and help elucidate how multiple interacting drivers may be related to observed population dynamics (review in Walther et al., 2002; Faaborg, 2014).
Since 1966, 49% of Neotropical migratory songbird species have undergone significant population declines (Sauer et al., 2012). These populations are likely negatively affected by a synergy of habitat loss and fragmentation (Hoover et al., 1995; Burke and Nol, 2000; Rushing et al., 2016), which is exacerbated by urbanization (Suarez-Rubio et al., 2011). In previous studies, urbanization has been shown to reduce fecundity in forest-breeding Neotropical migrants (Rodewald et al., 2013), can influence probability of species occupancy (Goodwin and Shriver, 2011), and hence can influence community-level dynamics (Ladin et al., 2016b). Although, research has shown how urbanization can negatively influence populations and alter community dynamics, it remains unclear how populations might respond to particular conservation strategies.
We used long-term demographic data of wood thrushes Hylocichla mustelina, considered an umbrella species for declining forest-breeding migratory songbirds (Simons et al., 1999; Russell et al., 2004), to understand how these species may respond to conservation in human-dominated landscapes. Similar to other forest-obligate species, regional patterns in wood thrush occupancy and abundance are positively related to the amount of forest habitat on the landscape (Smith et al., 2011). As forest habitat is replaced via urbanization, the increasing proportion of impervious surface on the landscape can have profound effects on watersheds, habitat quality, and species occurrence (Kaplan and Ayers, 2000). Impervious surface has been documented as a leading stressor on landbird community structure (Donnelly and Marzluff, 2006; Schlesinger et al., 2008), abundance (Lussier et al., 2006), and dispersal (Whittaker and Marzluff, 2012).
Locally, wood thrush breeding density and fecundity are negatively related to human development (e.g., impervious surface; Phillips et al., 2005; Richmond et al., 2012), and nest parasitism by the brown-headed cowbird Molothrus ater (hereafter cowbird; Hoover et al., 1995; Ladin et al., 2015). Similar to findings from previous studies on the negative effects of Cowbird parasitism on other species (Mayfield, 1977; Brittingham and Temple, 1983; De Groot and Smith, 2001). Ladin et al. (2016a) have recently demonstrated how coincident long-term population trends in Wood Thrushes and Cowbirds has exacerbated negative effects of Cowbirds on Wood Thrush reproduction. Moreover, within our study area located within the mid-Atlantic United States, 97% of 29 forest-breeding species with similar life-history traits to the wood thrush (e.g., migratory, multiple-broods) are actively parasitized by cowbirds (Lowther, 2013). Previous studies of long-term localized wood thrush population demographics (Roth and Johnson, 1993; Holmes, 2011) and regional source-sink dynamics (Lloyd et al., 2005; Tittler et al., 2006) suggest that multiple-scale approaches can help determine linkages between population dynamics and conservation of forest ecosystems (Bonnot et al., 2011).
We constructed an individual-based model for breeding wood thrushes that assumed heterogeneity among site quality and differential inter-site transition probabilities, to evaluate how among- and within-site characteristics influence metapopulation dynamics (Ovaskainen and Hanski, 2004). We used 40 years of demographic information and contemporary count and population genetics data to evaluate population vital rates in relation to four conservation scenarios. We predicted that conservation scenarios would have a positive effect on population growth rates, however, we were unsure of the magnitudes of respective population responses. Here we present a predictive metapopulation model that evaluates alternative conservation strategies that can be used as a tool in guiding conservation and management decisions for wood thrushes and other similarly forest-breeding migratory species.
Materials and Methods
Study Area and Species
Our study took place in the mid-Atlantic United States on the boundary of the Piedmont plateau and Atlantic Coastal Plain physiographic regions (Fenneman and Johnson, 1946), within the White Clay Creek and Christina River watersheds. We randomly located 21 sites within 18 distinct forest fragments in and near Newark, DE, USA (39°41′1N 75°44′58W; Table 1). Each site lay along an edge adjacent to non-forest land cover to control for edge effects. Sites ranged in size from 2.1 to 16.3 ha, and included Ecology Woods (Bray et al., 1965), where an ongoing 40-year demographic study on breeding wood thrushes occur. Wood thrushes breed in deciduous forest from southeastern Canada throughout the eastern United States and winter in Central America (Evans et al., 2011). Throughout their breeding range, wood thrushes are typically found nesting in under- and mid-story vegetation within forest patches ranging in size from 1 to >1000 ha (Keller and Yahner, 2007), often raise two broods per season (Evans et al., 2011), and exhibit a high degree of breeding site fidelity (Roth and Johnson, 1993).
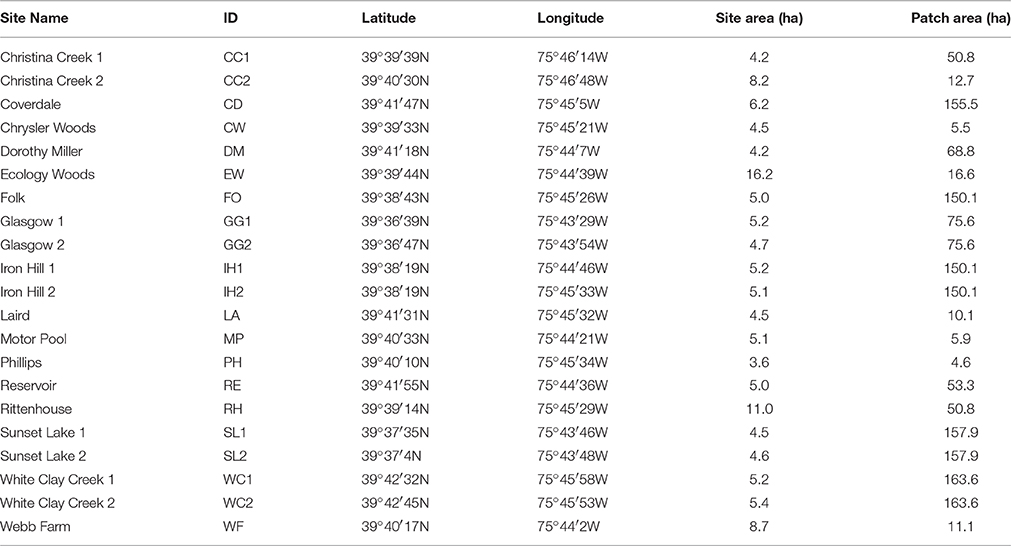
Table 1. List of 21 study site names, IDs, locations, and site and patch areas (hectares) in and near Newark, Delaware, USA.
Demographic Data Collection
We discovered and monitored active wood thrush nests every 2–3 days (Martin and Geupel, 1993). We recorded the numbers of wood thrush and cowbird eggs and nestlings present during each nest check. For each nest, we recorded the location (UTM coordinates), height (m), plant species, and subsequently the distance to the non-forest edge using ArcMap 10.1 (ESRI, 2011). We considered nests parasitized if at least one cowbird egg was present in the nest. Within our study area, cowbirds were detected at all sites, and we used logistic regression to test for effects of distance to edge (m), site, and month (i.e., May, June, July) on cowbird parasitism occurrence, and found no effects for distance to edge (z-value = −0.895, P = 0.371), or for site or month (all P > 0.998). We captured and sampled adult wood thrushes using mist nets (36 mm mesh size), and 6–10 day old nestlings by hand extraction from nests (Federal Bird Banding permit #: 23475) between 6 May and 15 August 2011–2013. We fitted all birds with aluminum US Geological Service bands, and adults were given unique color-band combinations to allow future identification by sight.
Demographic Parameters
We estimated wood thrush demographic parameters (i.e., adult survival, recruitment, fecundity, and immigration) using an integrated population model (IPM; Kéry and Schaub, 2012; Schaub et al., 2013). We used 40 years (1973–2013) of mark-recapture, population census, and fecundity data containing information from 2,592 marked individuals and 1,692 nests collected annually within Ecology Woods (EW), a 16-ha forest patch within our study area (Roth and Johnson, 1993), to run a female-based, age-structured population model (Ladin et al., 2016a) using the R package “R2WinBUGS” (Sturtz et al., 2005) and WinBUGS (ver. 1.4.3; Lunn et al., 2000). We used data from 1974 to 2013 on cowbird parasitism of wood thrush nests, which has increased by over 400% over the past four decades, to build regression models correlating cowbird parasitism pressure (i.e., mean proportion of parasitized nests and mean number of cowbird eggs per nest) with IPM-estimated wood thrush survival, fecundity, and recruitment (see Ladin et al., 2016a for details). We then computed contemporary demographic parameters (mean ± SD), by averaging 10 years of IPM annual estimates from 2002 to 2012 for adult survival, fecundity, recruitment, and immigration (Table 2).
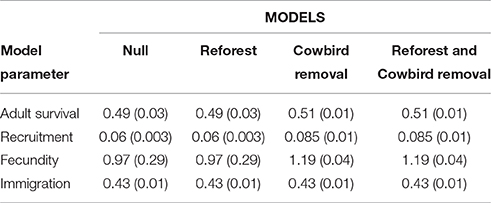
Table 2. Parameter estimate means (SD) used in four metapopulation model scenarios for wood thrushes breeding in small urban forest fragments in and near Newark, Delaware, USA.
Transition Matrix
To determine transition probabilities among sites, we genotyped individuals that were sampled over 3 years during the 2011–2013 breeding seasons at five microsatellite loci and estimated relatedness between all site pairs. In order to genotype individuals, we collected blood samples via brachial artery puncture using 27 gauge, 1.27 cm syringes in heparinized capillary tubes (75 μL) according to IACUC regulations, under permit #:1129-2012-2. We sealed capillary tubes containing blood with crito-seal clay and stored immediately on ice in the field. We then transferred blood samples to 1.5 mL cryo-vials and stored frozen at −80°C within 2–3 h of collection. We extracted deoxyribonucleic acid (DNA) from blood samples using Qiagen DNeasy kit (Qiagen, Venlo, Netherlands), and used polymerase chain reaction (PCR) in Bio Rad T100 thermal cyclers (Bio Rad, Hercules, CA, USA) to amplify five neutral polymorphic microsatellite loci, that have been previously used in genetic studies of wood thrush (Dawson et al., 1997; Lisle Gibbs et al., 1999; Evans et al., 2008). We analyzed florescent dye-labeled PCR products at the Delaware Biotechnology Institute using fragment analysis on a ABI Prism 3130XL Genetic Analyzer (Applied Biosystems, Foster City, CA, USA). We used the program BayesAss (Rannala, 2013) to estimate genetic distances and compute transition probabilities of wood thrushes among sites (Wilson and Rannala, 2003). Pair-wise site transition probabilities were then averaged between all site pairings and used to construct a transition matrix (see Appendix S2).
Wood Thrush Occupancy and Abundance Estimates
We conducted fixed-radius point count surveys (Buckland, 1987; Ralph et al., 1995) between 30 min before and 5 h after dawn from 1 May to 15 August 2011–2012 at one central point in each of the 21 sites. We visited each site 5 times per year and conducted a 10-min point count recording all birds detected within 0–50 m. We analyzed point count data using the “unmarked” package (Fiske and Chandler, 2011) in R (R Development Core Team, 2014) to estimate site occupancy (MacKenzie et al., 2002) and abundance (Royle, 2004). We used Akaike's information criterion (AIC; Akaike, 1974) with ΔAIC values ≤2 as a criterion to compare models predicting wood thrush site occupancy and abundance. In order to test for potential drivers of wood thrush site occupancy based on findings from previous studies (James et al., 1984; Donovan and Flather, 2002), we included the detection covariate (ordinal day) and the following site-level covariates: year, forest patch size (ha), proportion of impervious surface within 500 m buffer, proportion of agricultural land cover within 500 m buffer, mean forest site age, mean number of woody stems, and mean exchangeable soil calcium (Ca) (meq/100 g) in models. We computed the following site-level covariates [i.e., forest patch size (ha), proportion impervious surface within 500 m buffer, and proportion of agricultural land cover within 500 m buffer] in ArcMap (ver. 10.1) using the National Land Cover Data (NLCD) 2006 raster data. We compared 11 models to capture potential effects of patch-level covariates on wood thrush site occupancy. We extrapolated a population estimate to establish the initial conditions for model simulations by multiplying the estimated wood thrush density (i.e., individuals per ha) by the total area (ha) of forest habitat within our study area. Given documented even sex-ratios of males and females from previous studies (Roth and Johnson, 1993), we halved this number to estimate the number of females on the landscape.
Metapopulation Model Structure
We modeled wood thrush metapopulation dynamics among 21 discrete sites within our study area as a discrete-time Markov process in Mathematica 10.1 (Wolfram Research, 2014) and created an additional (22nd) site to represent emigration (i.e., removal from the study area). By drawing from distributions based on empirically-derived demographic parameters, model simulations mimicked a stochastic process (containing temporal variation from long-term data) where the probability of future states of the population were dependent on the current state at a given time step t (Caswell and Cohen, 1991; Verboom et al., 1991; Hanski and Ovaskainen, 2003).
We simulated four conservation scenarios by varying metapopulation model parameters based on regression functions that represented Scenario (A) the Null scenario, akin to no conservation efforts under current observed conditions (hereafter “Null”), Scenario (B) replacing impervious surface with forest (hereafter “Reforest”), Scenario (C) reducing cowbird parasitism pressure (hereafter “Cowbird removal”), and Scenario (D) coincident replacing impervious surface with forest and reducing cowbird parasitism (hereafter “Reforest and Cowbird removal”; see Figure 1 for conceptual diagram). All model simulations consisted of 10,000 iterations, and were run for 30 years into the future with = 0.03 as a calibration term that we adjusted so Null scenario trends mimicked that of current BBS-estimated trends within Delaware. We chose a 30-year timeframe based on the Null scenario performance, where after 30 years, the local population reached near-extinction levels. All simulations started from an initial distribution of birds across sites following the current observed distribution of birds (2011–2013) among 13 known occupied sites and 9 empty sites (including the idealized 22nd site representing emigration).
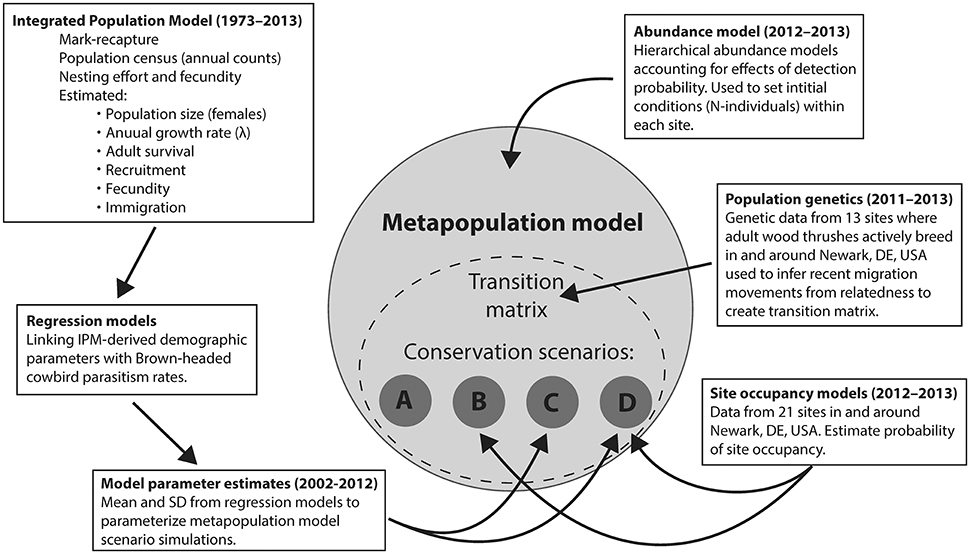
Figure 1. Conceptual diagram depicting data inputs (years shown in parentheses), analyses, and various modeling approaches used in parameterizing metapopulation model framework for comparison among conservation scenarios: (A) Null, (B) Reforest, (C) Cowbird removal, and (D) Reforest and Cowbird removal.
We initialized the model by distributing 400 birds (based on abundance estimates) by observed relative proportions among the 21 sites. At each time step (years), the number of birds per site for all 22 sites, was computed by multiplying the number of birds per site i at time step t (which we rounded to the nearest integer) by the transition probabilities of individuals moving between site i and any of the other sites, and then subsequently multiplying independently by adult survival, recruitment, and immigration rates to compute the number of returning adults, recruited individuals, and immigrants, respectively, with the equations:
where is the number of birds in site i and , , and were computed for each site i at each time step t, by randomly drawing values from uniform distributions with means and 95% CIs taken from IPM-estimated means for adult survival, recruitment, and immigration rates from 2002 to 2012. We defined recruited individuals as birds that were born and subsequently returned to the study area as sexually mature (≥1 year old). For each of 22 sites, the total number of birds at time (t + 1) was computed with the equation:
We computed the annual growth rate lambda (λi) within each site i with the equation:
where are the total number of birds per site i (from Equation 4) at time step (t + 1) and t, respectively. To estimate metapopulation annual growth rate (λ) for each model scenario, we summed the number of birds across 21 sites at each time step (Equation 4), and divided the total number of birds in our study area at time step t + 1 (Nt+1) by the total number of birds from the previous time step t (Nt). We calculated mean annual growth rates (λi) per year at each site i before averaging across 21 sites (excluding emigrants in site 22) for each ensemble of 10,000 model iterations. We calculated the probability of lambda (λi ≥ 1) for each scenario by tallying the number of sites where the local annual growth rate was equal to or >1 at each time step and dividing by the total number of sites. We estimated population trends (mean % annual change) for each model scenario using the equation:
where Nf is the number of birds at the final time step, No is the number of birds at the initial time step (to), and T is the total number of years the model is run for. We estimated mean annual site occupancy by dividing the number of occupied sites per year (defined as having at least two individuals) by the number of years.
After testing simulation-derived demographic data for departures from normality with a Shapiro–Wilk test, we used one-way analysis of variance (ANOVA) with Tukey's post-hoc tests to test for differences in population trend, mean annual growth rate (λ), and a multiple pair-wise comparison test (Marascuilo, 1966) for differences in site occupancy among the four model scenarios.
Model Scenario Descriptions
Scenario (A) Null
Null scenario parameters at each time step were derived independently for each site by drawing randomly from uniform distributions constrained by upper and lower 95% CI around the means of IPM annual estimates from 2002 to 2012 in EW for adult survival, recruitment, and fecundity (i.e., number of fledglings per female). Similarly, we estimated the proportion of immigrants for the metapopulation at each time step by randomly drawing from a uniform distribution with a mean and SD taken from IPM estimates. To model current observed conditions within the study area, we limited breeding (i.e., set fecundity = 0), and constrained immigration rates (i.e., 1% of total birds per site) to occupy currently unoccupied sites at (to), while allowing birds to immigrate at estimated rates into currently occupied sites and to reproduce at currently estimated levels of fecundity.
Scenario (B) Reforest (Replace Impervious Surface)
We modeled the effect of replacing the proportion of impervious surface with forest habitat on wood thrush metapopulation dynamics (based on results from predictive site occupancy models) by allowing 90% of total individuals at each time step to enter into and effectively breed (i.e., no reduction of fecundity) in previously unoccupied sites. These adjustments in the likelihood of site occupancy follow from concomitant reductions in impervious surface from ca. 0.8 (Null scenario) to around 0.05 using a predictive site occupancy model fit using the R package “unmarked” (Fiske and Chandler, 2011). Adult survival, recruitment, and fecundity were all estimated using the methods described above for the Null scenario.
Scenario (C) Cowbird Removal
We based our modeled reduction in cowbird parasitism pressure on beta regression models by increasing the probability of adult survival corresponding to a reduction in the proportion of cowbird nests parasitized from 0.65 (current observed parasitism rate) to range between (0.25 and 0.05). We increased estimated mean fecundity (i.e., number of fledglings per female) from 0.97 (current observed fecundity) to 1.19 using a linear model representing a predictive relationship between fecundity and the mean number of cowbird eggs laid per nest. We additionally increased mean recruitment based on the linear model relationship between the proportion of cowbird parasitized nests and annual number of recruited individuals. In this model scenario, immigration probabilities and fecundity in initially unoccupied sites were the same as in the Null scenario.
Scenario (D) Reforest and Cowbird Removal
We modeled the simultaneous effects of reducing impervious surface and cowbird parasitism pressure by using regression-based models to estimate according increases in immigration probabilities to all sites, and allowed breeding in all sites (as described in the Reforest scenario above). Under this model scenario, we used parameters estimated for adult survival, fecundity, and recruitment following the methods described above for the Cowbird removal scenario, and immigration probabilities were estimated as a function of reduced proportion of impervious surface (as stated above).
Results
Occupancy Model Results
The impervious surface model had the most support (Akaike weight = 0.62) as indicated by AIC model comparison (Appendix S1). Site occupancy was negatively related to the proportion of impervious surface within a 500 m buffer around forest sites (Figure 2A), and differed from the null model according to the likelihood ratio test (Kent, 1982) (χ2 = 11.6, df = 1, P < 0.01). From significant correlation between impervious surface within a 500 m buffer around sites and wood thrush site occupancy, we derived the equation:
representing the predicted probability of patch occupancy (y) given the proportion of impervious surface (x) within a 500 m buffer around a forest patch (Figure 2A).
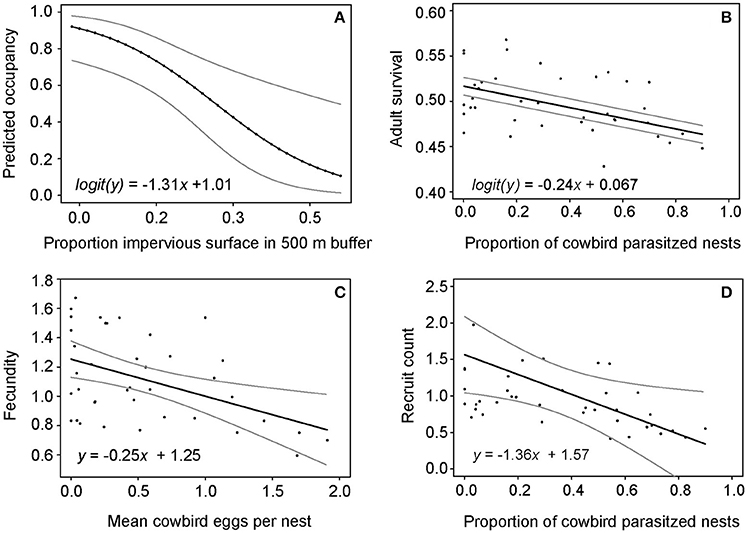
Figure 2. Regression models showing relationships between (A) proportion of impervious surface (within 500 m buffer around sites) and site occupancy, (B) proportion of cowbird-parasitized nests and adult wood thrush survival, (C) mean cowbird eggs laid per nest and wood thrush fecundity (mean fledglings per female), and (D) proportion of cowbird parasitized nests and mean number of recruited individuals per year.
Regression Model Results
Adult wood thrush survival from 1974 to 2012 was negatively related to the proportion of parasitized nests (χ2 = 80.1, df = 3, P < 0.01; Figure 2B). We inferred the following equation from the beta regression model of the negative relationship between adult wood thrush survival and the proportion of cowbird parasitized nests:
where y is annual adult survival and x is the proportion of cowbird parasitized wood thrush nests (Figure 2B), to estimate a mean adult survival (0.51 ± 0.01) corresponding to a simulated reduction in the proportion of parasitized nests by 62% (Table 2).
Wood thrush annual fecundity was negatively related to mean cowbird eggs laid per nest (F = 9.72, df = 37, P < 0.01; Figure 2C). We used linear regression in the “stats” R package and found that wood thrush annual fecundity (i.e., number of offspring produced per female) was negatively related to mean cowbird eggs laid per nest (F = 9.72, df = 37, P < 0.01). With the governing linear regression equation:
where y is predicted wood thrush fecundity and x is the mean cowbird eggs laid per nest (Figure 2C), we estimated a mean fecundity (1.19 ± 0.04) given the simulated reduction in mean number of cowbird eggs per nest from 1.18 to 0.5 (Table 2).
The mean number of annually recruited wood thrushes was also negatively related to the proportion of parasitized nests (F = 5.61, df = 37, P < 0.05), resulting in the following linear model equation:
where y is the number of recruited birds and x is proportion of parasitized nests (Figure 2D). We simulated increases in annual wood thrush recruitment rates by randomly drawing values from a uniform distribution with mean and SD of 0.085 ± 0.01 (Table 2).
Transition Matrix
We estimated transition probabilities among sites inferred from mean relatedness of birds among sites using microsatellite data from 177 genotyped adult wood thrushes sampled from 13 discrete sites (see Appendix S2). The final normalized transition matrix contained estimated transition probabilities from the 13 sampled sites, transition probabilities of 0.008 and 0.85 for leaving and remaining in a site, respectively, for the 8 initially unoccupied sites, and a 22nd site representing emigration out of the study area (see example transition matrix in Table 3).
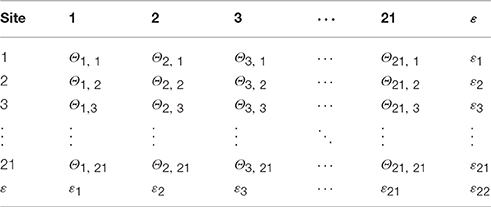
Table 3. Example transition matrix containing probabilities (Θ) of dispersal movement among 21 discrete forest sites, and functional emigration (ε) from the study area.
Metapopulation Simulation Results
Metapopulation-wide annual growth rates (λ) (mean ± SD) differed among all model scenarios (F = 567.1, df = 3, P < 0.001; Table 4), and were lowest for the Null scenario (0.88 ± 0.01) after 30 years (Table 4, Figure 3). The annual growth rate for the Cowbird removal scenario (0.92 ± 0.02) was lower than in the Reforest scenario (0.94 ± 0.01), and both were lower than the combined Reforest and Cowbird removal scenario (1.0 ± 0.01; Table 4, Figure 3).
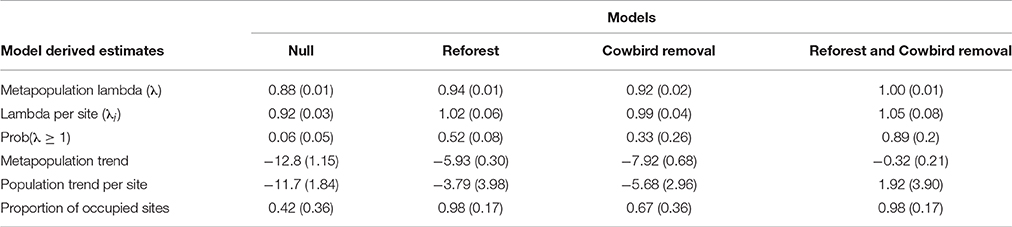
Table 4. Model-estimated means (SD) for metapopulation lambda (λ), lambda per site (λi), probability λ ≥ 1, annual population trend (%), and the proportion of occupied sites for four model scenarios.
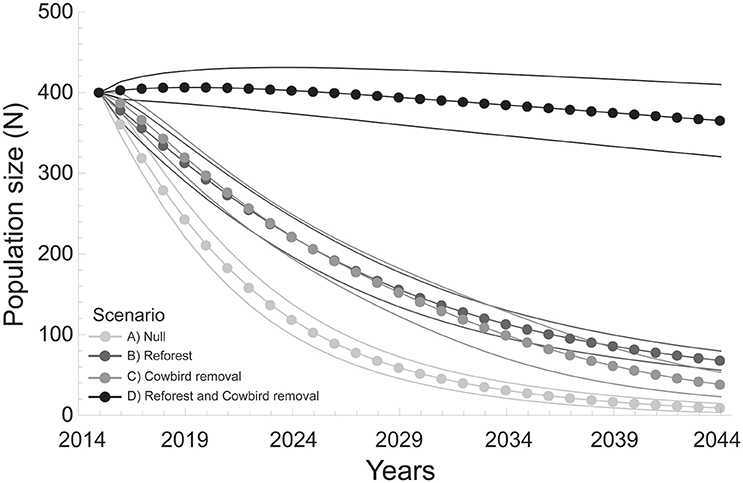
Figure 3. Wood thrush population size (N) estimates (mean and 95% CI) over a 30-year period under four model scenarios: (A) Null (light gray), (B) Reforest (dark gray), (C) Cowbird removal (gray), and (D) Reforest and Cowbird removal (black).
Annual growth rates per site (λi) (mean ± SD) differed among model scenarios (F = 46.3, df = 3, P < 0.001). Mean growth rates per site for the Null scenario (0.92 ± 0.03) were lower than all other scenarios, and mean growth rate per site for the Cowbird removal scenario (0.99 ± 0.04) was lower than both the Reforest scenario (1.02 ± 0.06), and the combined Reforest and Cowbird removal scenario (1.05 ± 0.08; Table 4). Additionally, we found that the probability that site-level mean annual growth rates (λi) were >1 over the 30-year simulation period was low under the Null scenario (0.06 ± 0.05), and higher probabilities were observed for Cowbird removal, Reforest, and combined Reforest and Cowbird removal scenarios (0.33 ± 0.26, 0.52 ± 0.08, and 0.89 ± 0.2, respectively; Table 4, Figure 3).
Annual population trend estimates (mean ± SE) differed among the four model scenarios (F = 61.1, df = 3, P < 0.001; see Appendix S3). Trend estimates were lower for the Null scenario (−11.7 ± 0.40) compared to all other scenarios, and both Cowbird removal (−5.68±0.65) and Reforest (−3.79±0.87) scenarios were lower than the combined Reforest and Cowbird removal scenario (1.92 ± 0.85; Table 4).
The proportion of occupied sites were similar under the Reforest scenario and the combined Reforest and Cowbird removal scenario (0.98 ± 0.17), and both were greater than the Null scenario (0.42 ± 0.36) and the Cowbird removal scenario (0.67 ± 0.36; χ2, df = 3, 7.815), which did not differ (Table 4).
The relationship of combinations of proportions of impervious surface (ranging from 0 to 0.35) and cowbird index (ranging between 0 and 0.5) to mean annual growth rate per site (λi) is shown in Figure 4. Mean annual growth rate (λi) was lowest (<0.94) when both impervious surface and cowbird index were 0.35 and 0.5, respectively (Figure 4). Annual growth rate was stable (λ = 1) between 0.05 and 0.15 impervious surface over the range of cowbird index values, and mean annual growth rate was positive (λ > 1) between 0.02 and 0.10 impervious surface over the range of cowbird index values (Figure 4).
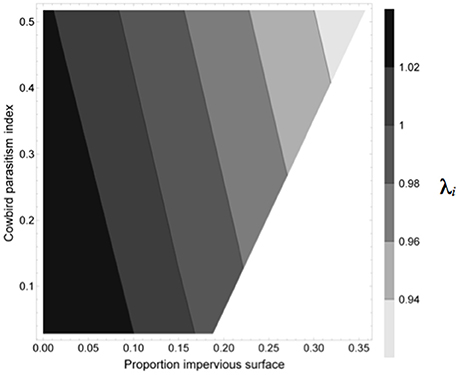
Figure 4. Contour plot showing the relationship of combinations of proportions of impervious surface and index of cowbird parasitism (i.e., proportion of cowbird parasitized nests × number of cowbird eggs per nest) to mean lambda per site (λi).
Discussion
Simulated conservation scenarios had a positive effect on wood thrush population growth rates. Our results suggest that coincident replacement of impervious surface with forest habitat and reducing cowbird parasitism rates would have the greatest benefit for wood thrush populations. Although both conservation measures (Reforest scenario and Cowbird removal scenario) had positive effects on wood thrush population growth, they were insufficient to stabilize population growth when implemented individually. We think wood thrush conservation efforts in the New England/mid-Atlantic region could combine local and regional strategies to reduce current rates of regional population declines and stabilize the population within the urbanized mid-Atlantic USA.
The implementation plan for the New England/mid-Atlantic Coast Bird Conservation Region 30 (BCR 30; characterized by a highly developed landscape), has identified habitat loss, degradation, and fragmentation as the greatest threat to bird species, and has designated wood thrushes as a species of “Highest Priority” with a regional population estimate 1/3 lower than objective goals (Atlantic Coast Joint Venture, 2012). We estimated that 23% of all forest habitat within BCR 30 (590,794 ha), is contained in forest fragments ≤20 ha that can provide valuable breeding and habitat for wood thrushes and other forest-breeding birds. Given observed differences in wood thrush occupancy across a range of patch sizes (Keller and Yahner, 2007; MacIntosh et al., 2011), we think that solely focusing conservation efforts on larger forest patches would be a mistake in attempting to achieve established population goals for wood thrushes in BCR 30.
We compared population trends among model scenarios and 40 years of empirical data from EW (−2.79), which were similar to Breeding Bird Survey (BBS) route regression trend estimates (1966–2012) for the New England/mid-Atlantic Coast (−2.77) and the state of Delaware (−2.1) (Sauer et al., 2012). However, more recent trends (from 2002 to 2012) have decreased to −8.42 in EW, and to −3.73 and −10.52 for the New England/mid-Atlantic Coast and Delaware, respectively (Sauer et al., 2012). In silico population trends for 30 years into the future were similar for Null in EW (−11.5), and for the overall study area (−12.8). This confirmed continuity among regional BBS, locally-observed, and model-predicted population trends which supports our comparison of population-trend estimates under modeled conservation scenarios. Given differences among growth rates throughout the breeding range (Sauer et al., 2012), conservation efforts for wood thrushes should address both regional and local factors that likely drive source-sink dynamics (Keagy et al., 2005).
Negative effects of urbanization and impervious surface on wood thrushes and likely other forest-breeding birds may be a key driver of site occupancy (Longcore and Jones, 1969; Roth and Johnson, 1993, this study). Our findings indicate that reducing the proportion of impervious surface from 0.60 (in Null) to 0.05 (Scenarios B and D), would increase site occupancy by 57% and the probability of (λ ≥ 1) by 88.5%. We found an upper threshold of 0.17 for the proportion of impervious surface where mean growth rate per site was positive (λi ≥ 1).
Despite known challenges regarding habitat loss and increasing rates of impervious surface in urban areas (Nowak and Greenfield, 2012), reforestation and “greening” of urbanized landscapes provide many plausible and beneficial opportunities for helping populations of forest-breeding migratory species (De Sousa, 2014; Haase et al., 2014). Existing strategies to mitigate current rates of habitat loss include tools and methods for guiding future sustainable design and development of urban areas (Le Roux et al., 2014; Scott and Lennon, 2016).
However, we should not be limited in our creativity or by our ability to work within existing urban areas (Madre et al., 2014). Several examples of successful reforestation within urban areas exist including long-term (20-year) benefits of reforestation within New York City parks where reforestation efforts led to increases in tree species diversity, forest structure complexity, and native tree regeneration (Simmons et al, 2016). Additionally, initiatives for maintaining urban green spaces and keeping pace with rates of human development have become important priorities within existing city plans (Tan et al., 2013; Norton et al., 2015).
Cowbird parasitism can also have negative effects on songbird species by reducing fecundity and can contribute to population-level declines (Mayfield, 1977; Kilpatrick, 2002). In addition, cowbird parasitism can have less obvious relationships to wood thrush population dynamics such as adult survival, perhaps due to adults incurring increased energetic costs related to provisioning cowbird nestlings (Ladin et al., 2016a). Simulations of cowbird removal showed that reducing the proportion of parasitized nests from 0.65 (Null) to between 0.25 and 0.0 (Cowbird removal), resulted in a 4.3% increase in metapopulation-wide annual growth rate (λ), a 7.1% increase in mean annual growth rates per site (λi), and an 82% increase in the probability that λ ≥ 1. Additionally, we observed a 37% increase in site occupancy under the Cowbird removal scenario. Given the positive trend for cowbirds in the New England/mid-Atlantic (1.2; Sauer et al., 2012) and demonstrated efficacy of cowbird removal for benefiting host species (e.g., Kirtland's warblers Dendroica kirtlandii; Siegle and Ahlers, 2004; McLeod and Koronkiewicz, 2014), we propose that cowbird removal be tested in the field to evaluate model predictions.
While empirically-based studies on how populations will respond to potential conservation strategies are critical starting points aiding in the design and implementation of conservation efforts, there are several other factors that can influence (potentially in unpredictable ways) intended outcomes from the application of conservation strategies that should be considered. For instance, how populations are likely to respond to climate change both on breeding and wintering grounds within spatially-explicit areas must be considered in concert with other factors that are examined (Giannini et al., 2015; Regos et al., 2015). One broadly-applicable consideration would be how species distribution shifts, and variation within species among populations to climate change might influence responses to specific conservation actions within a given area (Hällfors et al., 2016). Given the centrality of latitude for the location of our study area within the breeding range of wood thrushes in the eastern United States, species distribution shifts are likely to have little to minor effects on the performance of our model scenarios. However, for situations where conservation actions are targeting populations located at the margins of species distribution ranges, this should be directly accounted for (Hampe and Petit, 2005). Also, due to weak migratory connectivity between breeding and wintering areas found for wood thrushes, it will be hard to predict how climate- and landscape-change on wintering grounds may influence (via carry-over effects) population-limiting responses during breeding (Rushing et al., 2016). We are aware of additional and difficult-to-predict population responses that our model does not account for that include likely increases in human population growth rates, as well as, synergistic effects among climate change, increased human population densities in urban areas, and continued resultant land cover change due to increased rates of urbanization. However, our model does indirectly capture some of the inherent variation in wood thrush population responses to these factors through our inclusion of “noise” from long-term empirical relationships that end up being propagated within model predictions of the conservation scenarios we compared.
Our predicted population responses of wood thrushes to conservation scenarios could be used to estimate growth rates in other mid-Atlantic locations. However, estimated transition probabilities (which we held constant over time) do not account for other potentially contributing factors to wood thrush population dynamics, and ignore variation in habitat quality over time. Future models could improve these shortcomings by modifying transition probabilities and emigration rates through time according to predicted changes in habitat quality. Predicting bird population responses to future land use change (Martinuzzi et al., 2015), and mitigating negative effects of urbanization using sustainable landscape planning tools (Mason et al., 2007; Lerman et al., 2014; Gagné et al., 2015) will aid the conservation of wood thrushes and other forest-obligates. In general, we advocate for future studies using integrated population models and other empirically-based model simulations to evaluate potential population responses to conservation efforts.
Ethics Statement
We followed recommended guidelines for ethical scientific research and publishing conduct provided by the University of Delaware's Center for Science, Ethics and Public Policy.
Author Contributions
ZL was lead author on the study, and lead data collection and analyses. VD was involved in study design, study area establishment, and review of manuscript drafts. JB helped develop modeling framework for metapopulation models. RR was responsible for long-term data collection and review of manuscript drafts. WS was responsible for data management, budgetary management, study design, and review of manuscript drafts.
Conflict of Interest Statement
The authors declare that the research was conducted in the absence of any commercial or financial relationships that could be construed as a potential conflict of interest.
Acknowledgments
We thank the Northern Research Station of the US Forest Service, McIntire-Stennis Forestry Research Program, and the University of Delaware for funding. We thank cooperators from the University of Delaware, City of Newark Municipal parks, and White Clay Creek State Park for study site accessibility. We thank S. Adalsteinnson, J. Buler, A. Colavecchio, D. Delaney, D. Ecker, N. Hengst, S. Mkheidze, K. Pastirik, C. Rega, J. Richardson, K. Serno, and M. Walker for help with field work and data collection.
Supplementary Material
The Supplementary Material for this article can be found online at: http://journal.frontiersin.org/article/10.3389/fevo.2016.00122
References
Abadi, F., Gimenez, O., Ullrich, B., Arlettaz, R., and Schaub, M. (2010). Estimation of immigration rate using integrated population models. J. Appl. Ecol. 47, 393–400. doi: 10.1111/j.1365-2664.2010.01789.x
Akaike, H. (1974). A new look at the statistical model identification. IEEE Trans. Autom. Control 19, 716–723. doi: 10.1109/TAC.1974.1100705
Atlantic Coast Joint Venture (2012). New England/mid-Atlantic Coast Bird Conservation Region (BCR 30) Implementation Plan. Hadley, MA.
Bonnot, T. W., Thompson, F. R. III, and Millspaugh, J. J. (2011). Extension of landscape-based population viability models to ecoregional scales for conservation planning. Biol. Conserv. 144, 2041–2053. doi: 10.1016/j.biocon.2011.04.026
Bray, D. F., Catts, E. P., Jones, R. E., Longcore, J. R., Lesser, C. A., Preiss, F. J., et al. (1965). Wildland Ecology and Urban Impact. Research Report. Newark, DE: University of Delaware.
Brittingham, M. C., and Temple, S. A. (1983). Have cowbirds caused forest songbirds to decline? Bioscience 33, 31–35. doi: 10.2307/1309241
Buckland, S. T. (1987). On the variable circular plot method of estimating animal density. Biometrics 43, 363–384. doi: 10.2307/2531819
Burke, D. M., and Nol, E. (2000). Landscape and fragment size effects on reproductive success of forest-breeding birds in Ontario. Ecol. Appl. 10, 1749–1761. doi: 10.1890/1051-0761(2000)010[1749:LAFSEO]2.0.CO;2
Caswell, H., and Cohen, J. E. (1991). Disturbance, interspecific interaction and diversity in metapopulations. Biol. J. Linn. Soc. 42, 193–218. doi: 10.1111/j.1095-8312.1991.tb00560.x
Dawson, R. J., Gibbs, H. L., Hobson, K. A., and Yezerinac, S. M. (1997). Isolation of microsatellite DNA markers from a passerine bird, Dendroica petechia (the yellow warbler), and their use in population studies. Heredity 79, 506–514. doi: 10.1038/hdy.1997.190
De Groot, K. L., and Smith, J. N. M. (2001). Community-wide impacts of a generalist brood parasite, the Brown-headed Cowbird (Molothrus ater). Ecology 82, 868–881. doi: 10.2307/2680205
De Sousa, C. (2014). The greening of urban post-industrial landscapes: past practices and emerging trends. Local Environ. 19, 1049–1067. doi: 10.1080/13549839.2014.886560
Donnelly, R., and Marzluff, J. M. (2006). Relative importance of habitat quantity, structure, and spatial pattern to birds in urbanizing environments. Urban Ecosyst. 9, 99–117. doi: 10.1007/s11252-006-7904-2
Donovan, T. M., and Flather, C. H. (2002). Relationships among North American songbird trends, habitat fragmentation, and landscape occupancy. Ecol. Appl. 12, 364–374. doi: 10.2307/3060948
Evans, M., Gow, E., Roth, R. R., Johnson, M. S., and Underwood, T. J. (2011). Wood Thrush (Hylocichla mustelina). The Birds of North America Online. Ithaca, NY: Cornell Laboratory of Ornithology.
Evans, M. L., Stutchbury, B. J., and Woolfenden, B. E. (2008). Off-territory forays and genetic mating system of the Wood Thrush (Hylocichla mustelina). Auk 125, 67–75. doi: 10.1525/auk.2008.125.1.67
Faaborg, J. (2014). Saving Migrant Birds: Developing Strategies for the Future. Austin, Tx: University of Texas Press.
Fenneman, N. M., and Johnson, D. W. (1946). Physical Divisions of the United States. Reston, VA: US Geological Survey map prepared in cooperation with the Physiographic Commission.
Fiske, I., and Chandler, R. (2011). Unmarked: An R package for fitting hierarchical models of wildlife occurrence and abundance. J. Stat. Softw. 43, 1–23. doi: 10.18637/jss.v043.i10
Gagné, S. A., Eigenbrod, F., Bert, D. G., Cunnington, G. M., Olson, L. T., Smith, A. C., et al. (2015). A simple landscape design framework for biodiversity conservation. Landsc. Urban Plan. 136, 13–27. doi: 10.1016/j.landurbplan.2014.11.006
Giannini, T. C., Tambosi, L. R., Acosta, A. L., Jaffé, R., Saraiva, A. M., Imperatriz-Fonseca, V. L., et al. (2015). Safeguarding ecosystem services: a methodological framework to buffer the joint effect of habitat configuration and climate change. PLoS ONE 10:e0129225. doi: 10.1371/journal.pone.0129225
Lisle Gibbs, H., Tabak, L. M., and Hobson, K. (1999). Characterization of microsatellite DNA loci for a neotropical migrant songbird, the Swainson's thrush (Catharus ustulatus). Mol. Ecol. 8, 1551–1551. doi: 10.1046/j.1365-294x.1999.00673.x
Goodwin, S. E., and Shriver, W. G. (2011). Effects of traffic noise on occupancy patterns of forest birds. Conserv. Biol. 25, 406–411. doi: 10.1111/j.1523-1739.2010.01602.x
Haase, D., Frantzeskaki, N., and Elmqvist, T. (2014). Ecosystem services in urban landscapes: practical applications and governance implications. AMBIO 43, 407–412. doi: 10.1007/s13280-014-0503-1
Hällfors, M. H., Liao, J., Dzurisin, J., Grundel, R., Hyvärinen, M., Towle, K., et al. (2016). Addressing potential local adaptation in species distribution models: implications for conservation under climate change. Ecol. Appl. 26, 1154–1169. doi: 10.1890/15-0926
Hampe, A., and Petit, R. J. (2005). Conserving biodiversity under climate change: the rear edge matters. Ecol. Lett. 8, 461–467. doi: 10.1111/j.1461-0248.2005.00739.x
Hanski, I., and Ovaskainen, O. (2003). Metapopulation theory for fragmented landscapes. Theor. Popul. Biol. 64, 119–127. doi: 10.1016/S0040-5809(03)00022-4
Hanski, I. A., and Gaggiotti, O. E. (2004). Ecology, Genetics and Evolution of Metapopulations. London: Academic Press.
Holmes, R. T. (2011). Avian population and community processes in forest ecosystems: long-term research in the Hubbard Brook experimental forest. For. Ecol. Manag. 262, 20–32. doi: 10.1016/j.foreco.2010.06.021
Hoover, J. P., Brittingham, M. C., and Goodrich, L. J. (1995). Effects of forest patch size on nesting success of wood thrushes. Auk 112, 146–155. doi: 10.2307/4088774
James, F. C., Johnston, R. F., Wamer, N. O., Niemi, G. J., and Boecklen, W. J. (1984). The Grinnellian niche of the wood thrush. Am. Nat. 124, 17–47. doi: 10.1086/284250
Jarzyna, M. A., Porter, W. F., Maurer, B. A., Zuckerberg, B., and Finley, A. O. (2015). Landscape fragmentation affects responses of avian communities to climate change. Glob. Change Biol. 21, 2942–2953. doi: 10.1111/gcb.12885
Kaplan, M. B., and Ayers, M. (2000). Impervious Surface Cover: Concepts and Thresholds. Basis and Background in Support of the Water Quality and Watershed Management Rules. Trenton, NJ: New Jersey Department of Environmental Protection.
Kawecki, T. D. (2004). “Ecological and evolutionary consequences of source-sink population dynamics,” in Ecology, Genetics, and Evolution of Metapopulations, eds I. Hanski and O. Gaggiotti (Amsterdam: Elsevier), 387–414. doi: 10.1016/B978-012323448-3/50018-0
Keagy, J. C., Schreiber, S. J., and Cristol, D. A. (2005). Replacing sources with sinks: when do populations go down the drain? Restor. Ecol. 13, 529–535. doi: 10.1111/j.1526-100x.2005.00066.x
Keller, G. S., and Yahner, R. H. (2007). Seasonal forest-patch use by birds in fragmented landscapes of south-central Pennsylvania. Wilson J. Ornithol. 119, 410–418. doi: 10.1676/04-034.1
Kent, J. T. (1982). Robust properties of likelihood ratio tests. Biometrika 69, 19–27. doi: 10.2307/2335849
Kéry, M., and Schaub, M. (2012). Bayesian Population Analysis Using WinBUGS: A Hierarchical Perspective. Waltham, MA: Academic Press.
Kilpatrick, A. M. (2002). Variation in growth of Brown-headed Cowbird (Molothrus ater) nestlings and energetic impacts on their host parents. Can. J. Zool.-Rev. Can. Zool. 80, 145–153. doi: 10.1139/z01-217
Ladin, Z. S., D'Amico, V., Baetens, J. M., Roth, R. R., and Shriver, W. G. (2016a). Long-term dynamics in local host-parasite interactions linked to regional population trends. Ecosphere 7:e01420. doi: 10.1002/ecs2.1420
Ladin, Z. S., D'Amico, V., Jaisi, D. P., and Shriver, W. G. (2015). Is brood parasitism related to host nestling diet and nutrition? Auk Ornithol. Adv. 132, 717–734.
Ladin, Z. S., Higgins, C., Schmit, J. P., Sanders, G., Johnson, M. J., Weed, A. S., et al. (2016b). Using regional bird community dynamics to evaluate ecological integrity within national parks. Ecosphere 7:e01464. doi: 10.1002/ecs2.1464
Lerman, S. B., Nislow, K. H., Nowak, D. J., DeStefano, S., King, D. I., and Jones-Farrand, D. T. (2014). Using urban forest assessment tools to model bird habitat potential. Landsc. Urban Plan. 122, 29–40. doi: 10.1016/j.landurbplan.2013.10.006
Le Roux, D. S., Ikin, K., Lindenmayer, D. B., Blanchard, W., Manning, A. D., and Gibbons, P. (2014). Reduced availability of habitat structures in urban landscapes: implications for policy and practice. Landsc. Urban Plan. 125, 57–64. doi: 10.1016/j.landurbplan.2014.01.015
Lloyd, P., Martin, T. E., Redmond, R. L., Langner, U., and Hart, M. M. (2005). Linking demographic effects of habitat fragmentation across landscapes to continental source-sink dynamics. Ecol. Appl. 15, 1504–1514. doi: 10.1890/04-1243
Longcore, J., and Jones, R. E. (1969). Reproductive success of the wood thrush in a Delaware Woodlot. Wilson Bull. 81, 396–406.
Lowther, P. E. (2013). Lists of Victims and Hots of the Parasitic Cowbirds (Molothrus). Available online at: http://www.fieldmuseum.org/sites/default/files/Molothrus_hosts-26aug2013.pdf
Lunn, D. J., Thomas, A., Best, N., and Spiegelhalter, D. (2000). WinBUGS-a Bayesian modelling framework: concepts, structure, and extensibility. Stat. Comput. 10, 325–337. doi: 10.1023/A:1008929526011
Lussier, S. M., Enser, R. W., Dasilva, S. N., and Charpentier, M. (2006). Effects of habitat disturbance from residential development on breeding bird communities in riparian corridors. Environ. Manage. 38, 504–521. doi: 10.1007/s00267-005-0088-3
MacIntosh, T., Stutchbury, B. J. M., and Evans, M. L. (2011). Gap-crossing by Wood Thrushes (Hylocichla mustelina) in a fragmented landscape. Can. J. Zool. 89, 1091–1097. doi: 10.1139/z11-090
MacKenzie, D. I., Nichols, J. D., Lachman, G. B., Droege, S., Andrew Royle, J., and Langtimm, C. A. (2002). Estimating site occupancy rates when detection probabilities are less than one. Ecology 83, 2248–2255. doi: 10.1890/0012-9658(2002)083[2248:ESORWD]2.0.CO;2
Madre, F., Vergnes, A., Machon, N., and Clergeau, P. (2014). Green roofs as habitats for wild plant species in urban landscapes: first insights from a large-scale sampling. Landsc. Urban Plan. 122, 100–107. doi: 10.1016/j.landurbplan.2013.11.012
Marascuilo, L. A. (1966). Large-sample multiple comparisons. Psychol. Bull. 65, 280. doi: 10.1037/h0023189
Martin, T., and Geupel, G. (1993). Nest-monitoring plots - methods for locating nests and monitoring success. J. Field Ornithol. 64, 507–519.
Martinuzzi, S., Withey, J. C., Pidgeon, A. M., Plantinga, A. J., McKerrow, A. J., and Williams, S. G. (2015). Future land-use scenarios and the loss of wildlife habitats in the southeastern United States. Ecol. Appl. 25, 160–171. doi: 10.1890/13-2078.1
Mason, J., Moorman, C., Hess, G., and Sinclair, K. (2007). Designing suburban greenways to provide habitat for forest-breeding birds. Landsc. Urban Plan. 80, 153–164. doi: 10.1016/j.landurbplan.2006.07.002
McLeod, M. A., and Koronkiewicz, T. J. (2014). Comparison of capture and escape rates between different types of portable cowbird traps. Wildl. Soc. Bull. 38, 611–618. doi: 10.1002/wsb.420
Meineri, E., Deville, A.-S., Grémillet, D., Gauthier-Clerc, M., and Béchet, A. (2015). Combining correlative and mechanistic habitat suitability models to improve ecological compensation. Biol. Rev. 90, 314–329. doi: 10.1111/brv.12111
Norton, B. A., Coutts, A. M., Livesley, S. J., Harris, R. J., Hunter, A. M., and Williams, N. S. G. (2015). Planning for cooler cities: a framework to prioritise green infrastructure to mitigate high temperatures in urban landscapes. Landsc. Urban Plan. 134, 127–138. doi: 10.1016/j.landurbplan.2014.10.018
Nowak, D. J., and Greenfield, E. J. (2012). Tree and impervious cover change in U.S. cities. Urban For. Urban Green. 11, 21–30. doi: 10.1016/j.ufug.2011.11.005
Ovaskainen, O., and Hanski, I. (2004). “Metapopulation dynamics in highly fragmented landscapes,” in Ecology, Genetics, and Evolution of Metapopulations, eds I. Hanski, and O. Gaggiotti (Amsterdam: Elsevier), 73–103. doi: 10.1016/B978-012323448-3/50006-4
Phillips, J., Nol, E., Burke, D., and Dunford, W. (2005). Impacts of housing developments on Wood Thrush nesting success in hardwood forest fragments. Condor 107, 97–106. doi: 10.1650/7560
Pulliam, H. R. (1988). Sources, sinks, and population regulation. Am. Nat. 132, 652–661. doi: 10.1086/284880
Ralph, C. J., Sauer, J. R., and Droege, S. (eds.). (1995). Monitoring Bird Populations by Point Counts. Gen. Tech. Rep. PSW-GTR-149. Albany, CA: Pacific Southwest Research Station, Forest Service, US. Department of Agriculture.
Rannala, B. (2013). BayesAss Edition 3.0 User's Manual. Accessed Online 27. Available online at: sourceforge.mirrorservice.org/b/ba/bayesass/BA3/3.0.4/docs/BA3Manual.pdf
R Development Core Team (2014). A Language and Environment for Statistical Computing. R Foundation for Statistical Computing (Vienna). Available online at: http://www.R-project.org
Regos, A., D'Amen, M., Titeux, N., Herrando, S., Guisan, A., and Brotons, L. (2015). Predicting the future effectiveness of protected areas for bird conservation in Mediteranian ecosystems under climate change and novel fire regime scenarios. Divers. Distrib. 22, 83–96. doi: 10.1111/ddi.12375
Richmond, S., Nol, E., and Burke, D. (2012). Local-versus landscape-scale effects on the demography of three forest-breeding songbirds in Ontario, Canada. Can. J. Zool. 90, 815–828. doi: 10.1139/z2012-051
Rodewald, A. D., Kearns, L. J., and Shustack, D. P. (2013). Consequences of urbanizing landscapes to reproductive performance of birds in remnant forests. Biol. Conserv. 160, 32–39. doi: 10.1016/j.biocon.2012.12.034
Roth, R., and Johnson, R. (1993). Long-term dynamics of a wood thrush population breeding in a forest fragment. Auk 110, 37–48.
Royle, J. A. (2004). N-mixture models for estimating population size from spatially replicated counts. Biometrics 60, 108–115. doi: 10.1111/j.0006-341X.2004.00142.x
Rushing, C. S., Ryder, T. B., and Marra, P. P. (2016). Quantifying drivers of population dynamics for a migratory bird throughout the annual cycle. Proc. R. Soc. B 283:20152846. doi: 10.1098/rspb.2015.2846
Russell, R. E., Moore, J. E., Miller, M. S., Sutton, T. M., and Knapp, S. M. (2004). “Selecting surrogate species for ecological assessments in land-use planning: a case study in the Upper Wabash River Basin,” in Conserving Biodiversity in Agricultural Landscapes: Model-Based Planning Tools, eds R. K. Swihart, and J. E. Moore (West Lafayette, IN: Purdue University Press), 181–214.
Sauer, J. R., Hines, J. E., Fallon, J. E., Pardieck, K. L., Ziolkowski, D. J. Jr., and Link, W. A. (2012). The North American Breeding Bird Survey, Results and Analysis 1966–2011. Version 07.03. 2013. Laurel, MD: USGS Patuxent Wildlife Research Center.
Schaub, M., Jakober, H., and Stauber, W. (2013). Strong contribution of immigration to local population regulation: evidence from a migratory passerine. Ecology 94, 1828–1838. doi: 10.1890/12-1395.1
Schlesinger, M. D., Manley, P. N., and Holyoak, M. (2008). Distinguishing stressors acting on land bird communities in an urbanizing environment. Ecology 89, 2302–2314. doi: 10.1890/07-0256.1
Scott, M., and Lennon, M. (2016). Nature-based solutions for the contemporary city. Plan. Theory Prac. 17, 267–300. doi: 10.1080/14649357.2016.1158907
Siegle, R., and Ahlers, D. (2004). Brown-Headed Cowbird Management Techniques Manual. US Department of the Interior, Bureau of Reclamation, Technical Service Center, Ecological Planning and Assessment.
Simmons, B. L., Hallett, R. A., Sonti, N. F., Auyeung, D. S. N., and Lu, J. W. (2016). Long-term outcomes of forest restoration in an urban park. Restor. Ecol. 24, 109–118. doi: 10.1111/rec.12281
Simons, T. R., Rabenold, K. N., Buehler, D. A., Collazo, J. C., and Franzreb, K. E. (1999). “The role of indicator species: Neotropical migratory songbirds,” in Ecosystem Management for Sustainability: Principles and Practices Illustrated by a Regional Biosphere Reserve Cooperative, ed J. D. Peine (Boca Raton, FL: CRC Press), 187–208.
Smith, A. C., Fahrig, L., and Francis, C. M. (2011). Landscape size affects the relative importance of habitat amount, habitat fragmentation, and matrix quality on forest birds. Ecography 34, 103–113. doi: 10.1111/j.1600-0587.2010.06201.x
Soulé, M. E., Bolger, D. T., Alberts, A. C., Wrights, J., Sorice, M., and Hill, S. (1988). Reconstructed dynamics of rapid extinctions of chaparral-requiring birds in urban habitat islands. Conserv. Biol. 2, 75–92. doi: 10.1111/j.1523-1739.1988.tb00337.x
Sturtz, S., Ligges, U., and Gelman, A. E. (2005). R2WinBUGS: a package for running WinBUGS from R. J. Stat. Softw. 12, 1–16. doi: 10.18637/jss.v012.i03
Suarez-Rubio, M., Leimgruber, P., and Renner, S. C. (2011). Influence of exurban development on bird species richness and diversity. J. Ornithol. 152, 461–471. doi: 10.1007/s10336-010-0605-x
Tan, P. Y., Wang, J., and Sia, A. (2013). Perspectives on five decades of the urban greening of Singapore. Cities 32, 24–32. doi: 10.1016/j.cities.2013.02.001
Tittler, R., Fahrig, L., and Villard, M.-A. (2006). Evidence of large-scale source-sink dynamics and long-distance dispersal among wood thrush populations. Ecology 87, 3029–3036. doi: 10.1890/0012-9658(2006)87[3029:EOLSDA]2.0.CO;2
Verboom, J., Lankester, K., and Metz, J. A. (1991). Linking local and regional dynamics in stochastic metapopulation models. Biol. J. Linn. Soc. 42, 39–55. doi: 10.1111/j.1095-8312.1991.tb00550.x
Walsh, J. C., Dicks, L. V., and Sutherland, W. J. (2015). The effect of scientific evidence on conservation practitioners' management decisions. Conserv. Biol. 29, 88–98. doi: 10.1111/cobi.12370
Walther, G.-R., Post, E., Convey, P., Menzel, A., Parmesan, C., Beebee, T. J., et al. (2002). Ecological responses to recent climate change. Nature 416, 389–395. doi: 10.1038/416389a
Whittaker, K. A., and Marzluff, J. M. (2012). “Post-fledging mobility in an urban landscape,” in Urban Bird Ecology and Conservation. Stud Avian Biol (no. 45), eds C. A. Lepczyk and P. S. Warren (Berkeley, CA: University of California Press), 183–198. doi: 10.1525/california/9780520273092.003.0012
Keywords: brood parasite, brown-headed cowbird, Hylocichla mustelina, impervious surface, metapopulation, Molothrus ater, urbanization, wood thrush
Citation: Ladin ZS, D'Amico V, Baetens JM, Roth RR and Shriver WG (2016) Predicting Metapopulation Responses to Conservation in Human-Dominated Landscapes. Front. Ecol. Evol. 4:122. doi: 10.3389/fevo.2016.00122
Received: 31 August 2016; Accepted: 03 October 2016;
Published: 19 October 2016.
Edited by:
Caroline Isaksson, Lund University, SwedenReviewed by:
Jari Niemelä, University of Helsinki, FinlandAndrea Olive, University of Toronto, Canada
Copyright © 2016 Ladin, D'Amico, Baetens, Roth and Shriver. This is an open-access article distributed under the terms of the Creative Commons Attribution License (CC BY). The use, distribution or reproduction in other forums is permitted, provided the original author(s) or licensor are credited and that the original publication in this journal is cited, in accordance with accepted academic practice. No use, distribution or reproduction is permitted which does not comply with these terms.
*Correspondence: Zachary S. Ladin, emFjaEB1ZGVsLmVkdQ==