- 1Oceanographic Institute, University of São Paulo, São Paulo, Brazil
- 2Division of Biological and Environmental Science and Engineering (BESE), Red Sea Research Center, King Abdullah University of Science and Technology (KAUST), Thuwal, Saudi Arabia
Symbiodinium are dinoflagellate photosynthetic algae that associate with a diverse array of marine invertebrates, and these relationships are comprehensively documented for adult animal hosts. Conversely, comparatively little is known about the associations during larval development of animal hosts, although four different metazoan phyla (Porifera, Cnidaria, Acoelomorpha, and Mollusca) produce larvae associated with Symbiodinium. These phyla represent considerable diversities in larval forms, manner of symbiont acquisition, and requirements on the presence of symbionts for successful metamorphosis. Importantly, the different requirements are conveyed by specific symbiont types that are selected by the host animal larvae. Nevertheless, it remains to be determined whether these associations during larval stages already represent mutualistic interactions, as evident from the relationship of Symbiodinium with their adult animal hosts. For instance, molecular studies suggest that the host larval transcriptome is nearly unaltered after symbiont acquisition. Even so, a symbiosis-specific gene has been identified in Symbiodinium that is expressed in larval host stages, and similar genes are currently being described for host organisms. However, some reports suggest that the metabolic exchange between host larvae and Symbiodinium may not cover the energetic requirements of the host. Here, we review current studies to summarize what is known about the association between metazoan larvae and Symbiodinium. In particular, our aim was to gather in how far the mutualistic relationship present between adult animals hosts and Symbiodinium is already laid out at the time of symbiont acquisition by host larvae. We conclude that the mutualistic relationship between animal hosts and algal symbionts in many cases is not set up during larval development. Furthermore, symbiont identity may influence whether a mutualism can be established during host larval stages.
Introduction
Marine invertebrates typically present indirect development and their generally planktonic larvae act as the dispersive stage. This is a particularly important adaptation, as it directly influences species distribution and abundance, and also enables geographical range expansion and recovery of populations (Strathmann et al., 2002). Many factors influence larval development and its dispersal, such as oceanographic conditions, predation avoidance by planktivorous organisms, energy reserves, and feeding abilities (Pechenik, 1990). As a response to such wide array of scenarios, there is a significant diversity of larval forms presented by metazoan organisms (Wray and Raff, 1991). Certain larval types are planktotrophic and required to feed, thus presenting specialized organs, while others are lecithotrophic and can survive through metamorphosis while relying solely on endogenous reserves (Thorson, 1950; Pechenik, 1999).
Despite the diversity of requirements for different marine invertebrate larvae, certain larval forms not only require to feed in the plankton, but also acquire symbionts (Fitt et al., 1986; Mieog et al., 2009; Garcia Ramos and Banaszak, 2014). Many metazoan phyla, especially scleractinian corals within the Cnidaria, engage in a mutualistic symbiosis with the photosynthetic dinoflagellates in the genus Symbiodinium (Stat et al., 2006; Venn et al., 2008). The majority of these corals are broadcast spawners and newly-formed planula larvae, while lecithotrophic, must acquire their symbionts horizontally (Harrison and Wallace, 1990; Baird et al., 2009; Harrison, 2011). The planktonic larvae acquire different free-living Symbiodinium strains from the environmental pool before selecting one or more specific strains at a later stage (Belda-Baillie et al., 1999; Weis et al., 2001; Coffroth and Santos, 2005).
Further, although numerous studies are available on animal-dinoflagellate symbioses, there is still an overall paucity of knowledge on this relationship during host larval development, especially in regard to metabolite exchange and symbiosis-related differential gene expression. In fact, this paucity of information raises the question as to whether the mutualistic nature of this relationship is already present during host larval development or becomes established at a later stage. Likewise, while there is much information on the impacts of climate change and anthropogenic influence on adult and symbiotic coral reef organisms (Hoegh-Guldberg, 1999; Hughes et al., 2003; Hoegh-Guldberg et al., 2007), comparatively little is known about the impacts of these environmental changes on Symbiodinium-associated animal larvae. Therefore, here we aim to compile, describe, and interpret the available data on metazoan larva-Symbiodinium associations, with emphasis on the ecological, molecular and biochemical relationship, evolution, and impact of climate change.
Marine Invertebrate Larvae Associated with Symbiodinium
Four different metazoan phyla produce larval forms that associate with Symbiodinium: Porifera, Cnidaria, Acoelomorpha, and Mollusca (Table 1). Two protist taxa are also known to associate with Symbiodinium, Foraminifera (Pochon and Gates, 2010), and Ciliophora (Mordret et al., 2016). Invertebrate larvae acquire symbionts in two different ways: vertically (transmitted to the offspring in parental oocytes) or horizontally by oral ingestion during larval development. Both strategies have their advantages. Vertical transmission ensures that a successful symbiont strain is supplied to the larvae (Wilkinson and Sherratt, 2001), while horizontal transmission allows for the larvae to acquire a diversity of symbionts, some of which may be more compatible with different environmental conditions encountered after dispersal (Baird et al., 2007).
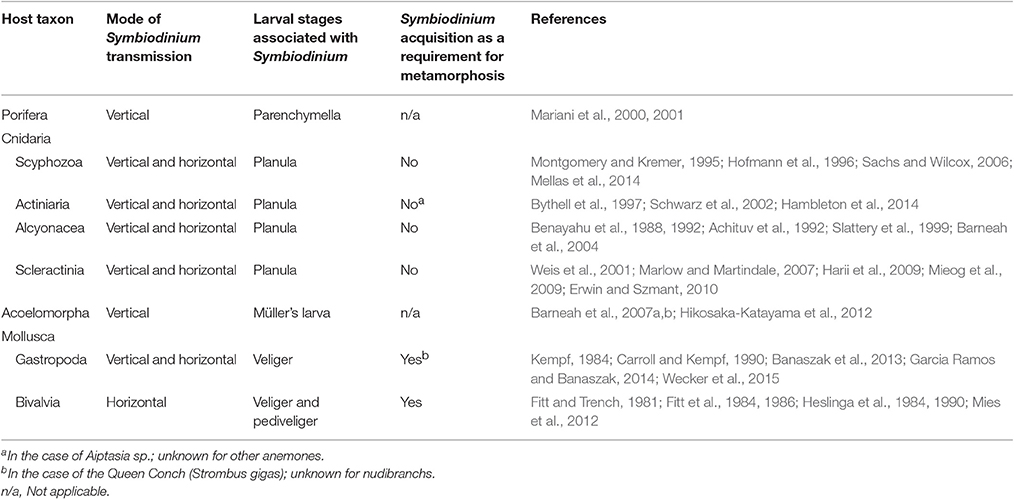
Table 1. Host taxa and their larval stages associated with Symbiodinium, also describing mode of symbiont transmission and addressing whether the presence of Symbiodinium in larval tissues is critical for successfully attaining metamorphosis.
Sponges and acoelomorph worms are the two groups in which particularly little is known about their association with Symbiodinium, especially during larval development. Within sponges, the association with Symbiodinium seems to be largely restricted to the family Clionaidae (Hill et al., 2011) and parenchymella larvae contain parentally-seeded symbionts (Mariani et al., 2000, 2001). In the case of acoelomorph worms, the genus Waminoa harbors two different symbiotic dinoflagellates, Symbiodinium and Amphidinium, which are also transmitted vertically (Barneah et al., 2007b). Other acoelomorph genera are known to associate with Symbiodinium (Trench and Winsor, 1987), but no information regarding their larval development has been reported.
In the case of cnidarians, while broad knowledge on their association with Symbiodinium is available, there are still relatively few studies on the larva-Symbiodinium association (Rodriguez-Lanetty et al., 2006; Voolstra et al., 2009b; Schnitzler and Weis, 2010; Wolfowicz et al., 2016). Approximately 80-90% of reef-building coral species (Scleractinia) are broadcast spawners and acquire symbionts horizontally (Harrison and Wallace, 1990; Baird et al., 2009; Harrison, 2011). On the other hand, many of the less common brooding species transmit symbionts vertically, most notably in the family Pocilloporidae (Baird et al., 2009). In the case of soft coral species (Alcyonacea), the incidence of vertical transmission seems to be higher (Benayahu et al., 1988; Achituv et al., 1992) than for scleractinians. Coral larvae are typically lecithotrophic (Morse et al., 1996) and while symbiont acquisition may improve host fitness, it does not seem to be a requirement for metamorphosis (Schwarz et al., 1999; Mieog et al., 2009). In the case of other cnidarians such as anemones and jellyfish, there is still very little information on the role of Symbiodinium in their larval ecology.
Among mollusks, giant clams (subfamily Tridacninae) are possibly the most conspicuous species associated with Symbiodinium. In this group symbiont acquisition is horizontal (Fitt and Trench, 1981) and a requirement for successful metamorphosis (Mies et al., 2017a). Differently from other metazoan hosts, symbionts are only acquired during the second larval stage (veliger), as the first larval stage is a non-feeding trochophore (Fitt et al., 1984; Heslinga et al., 1984). To date, giant clams are also the only known Symbiodinium hosts that during larval development do not move symbionts from the digestive tract or equivalent to endodermal tissues (Schwarz et al., 2002; Marlow and Martindale, 2007; Huang et al., 2008), as they remain in the digestive tract throughout the larval development (Fitt et al., 1986; Hirose et al., 2006). The other mollusk group associated with Symbiodinium is Gastropoda. The Queen Conch Strombus gigas is the largest among them to do so and symbiont acquisition is also horizontal and apparently required for metamorphosis (Garcia Ramos and Banaszak, 2014). While a large diversity of nudibranchs (mainly aeolids) are known to associate with Symbiodinium, there is a scarcity of larval experiments, possibly due to their typically restricted diet (Dionísio et al., 2013). The only exception is Berghia stephanieae, a species that maintains Symbiodinium in dorsal extensions of the digestive diverticulum. Its lecithotrophic veliger larvae undergo metamorphosis in the absence of Symbiodinium (Carroll and Kempf, 1990; Kempf, 1991), but recent studies suggest that this species does not engage in a mutualistic relationship (Mies et al., 2017c). While nudibranchs usually acquire symbionts horizontally (Loh et al., 2006; Burghardt and Wägele, 2014; Ahmadian et al., 2016), a case of vertical transmission has been recently reported (Wecker et al., 2015).
Symbiodinium Diversity
Symbiodinium dinoflagellates may be found in two different life stages, a planktonic and free-living zoospore or a symbiotic and non-motile coccoid cyst (Freudenthal, 1962; Schoenberg and Trench, 1980; Stat et al., 2006). Thus, by presenting a free-living stage, the mutualistic association is not obligate for Symbiodinium (Stat et al., 2006). Species within this genus are morphologically cryptic and identification is performed through the use of molecular biology techniques (LaJeunesse et al., 2012; Arif et al., 2014). Based on ribosomal, plastid and mitochondrial phylogenies, Symbiodinium are divided in nine clades (A–I, Figure 1) and many subclades (Rowan and Powers, 1991; LaJeunesse, 2001; Pochon et al., 2014). Species-level diversity is still being assessed, mainly through sequencing of the internal transcribed spacer 2 (ITS2, see LaJeunesse and Trench, 2000), either by denaturing gradient gel electrophoresis (DGGE) based separation of ITS2 genomic copies (LaJeunesse and Trench, 2000; LaJeunesse, 2002; Thornhill et al., 2006) or by next-generation sequencing based elucidation of ITS2 diversity (Arif et al., 2014; Batovska et al., 2016; Hume et al., 2016; Ziegler et al., 2017). As a result, many new taxa and/or strains have been described recently (LaJeunesse et al., 2012; Hume et al., 2015; Lee et al., 2015; Ziegler et al., 2017), with probably more than 100 extant species being present in the genus Symbiodinium (LaJeunesse, 2001). Next-generation sequencing methods also allow to approach cryptic diversity in Symbiodinium-host relationships, i.e., the presence of rare, background Symbiodinium taxa that might be missed by genotyping methods with a lower resolution. This approach holds a dual premise: firstly, intragenomic allelic diversity of the ITS2 marker allows to resolve differences within and between individuals and species previously not possible (Batovska et al., 2016); secondly, in the case of Symbiodinium, deep sequencing of ITS2 diversity may uncover symbiont associations previously not detected (Arif et al., 2014; Boulotte et al., 2016). However, the contribution of low abundant Symbiodinium taxa to host resilience remains to be determined (Lee et al., 2016). Regardless of these considerations, the highest species diversity is found within clade C, that dominates Indo-Pacific coral reefs and is also one of the most abundant clades in the Atlantic (LaJeunesse, 2005). Host species tend to associate with a single Symbiodinium strain (Goulet, 2006), but multiple symbiont taxa (of different clades) may be found within a single host specimen (Rowan and Powers, 1991; Carlos et al., 2000; Baker, 2003). The type of Symbiodinium is a relevant factor for holobiont fitness, in both adult and larval stages (Schwarz et al., 1999; Mieog et al., 2009). While it has been reported that there is host life stage-specificity for symbiont strains, as juvenile and adult acroporid corals actively associate with different clades (Abrego et al., 2009), there is no life-stage specificity reported for larval stages yet.
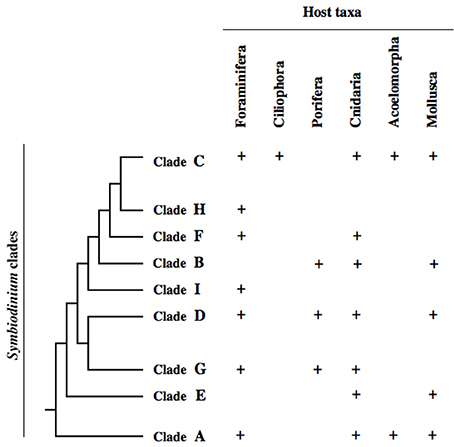
Figure 1. Relationship between Symbiodinium clades (based on a multigene phylogeny–see Pochon et al., 2014) and their distribution in host taxa according to Stat et al. (2006); Barneah et al. (2007b), and Pochon et al. (2014).
Symbiont Acquisition and Larval Response
Animal larvae acquire symbionts from different sources, but mainly from the water column and sediment (Adams et al., 2009; Cumbo et al., 2012; Nitschke et al., 2016). The amount of Symbiodinium cells acquired is highly variable among species and may vary according to environmental conditions. For acroporid corals Cumbo et al. (2012) report less than 10 symbiont cells acquired by larvae, while Harii et al. (2009) registered an average of 50-60 symbiont cells, but with high variability. Mies et al. (2017c) report about 30 cells for both giant clam and nudibranch larvae and more than 100 for coral larvae.
Animal hosts are able to select Symbiodinium strains through post-phagocytic recognition mechanisms involving lectin/glycan interactions (Koike et al., 2004; Wood-Charlson et al., 2006; Fransolet et al., 2012). These mechanisms are also active during larval development and reports also show that coral (Bay et al., 2011) and gastropod (Garcia Ramos and Banaszak, 2014) larvae actively select symbionts. Desired Symbiodinium species are kept while undesired ones suffer apoptosis (Dunn and Weis, 2009). However, there are reports that coral larvae may have no preference for different symbiont strains (Cumbo et al., 2012). In a study testing the offer of a homologous and two heterologous strains to Fungia scutaria larvae, there was no statistical difference in acquisition among strains, except for one of the heterologous strains that had significantly lower acquisition (Rodriguez-Lanetty et al., 2004). Interestingly, homologous strains have been shown to distribute differently in larval tissues than heterologous strains (Rodriguez-Lanetty et al., 2006), and it has also been reported that homologous symbionts establish a more benefitting symbiosis (Trench et al., 1981; Schwarz et al., 1999; Dunn and Weis, 2009). However, Mies et al. (2017c) found no differences in survival in coral, nudibranch, and giant clam larvae associated with both homologous and heterologous Symbiodinium clades. Further, a recent study by Biquand et al. (2017) suggests that at least part of the specificity in the host-symbiont relationship may be determined by symbiont cell size, where Symbiodinium strains with a larger cell size are less likely to establish a symbiotic relationship than taxa with a smaller cell size using the laboratory model Aiptasia (Baumgarten et al., 2015) and corals. Nevertheless, the presence of symbionts in larval tissues (see Figure 2) may significantly contribute to its development. Giant clam larvae infected with Symbiodinium grow significantly larger than aposymbiotic larvae (Fitt et al., 1986; Mies et al., 2012) and F. scutaria larvae settle earlier in the presence of symbionts (Schwarz et al., 1999). However, higher growth not always suggests the establishment of symbiosis as some coral larvae have been shown to digest the symbionts (Titlyanov et al., 1998).
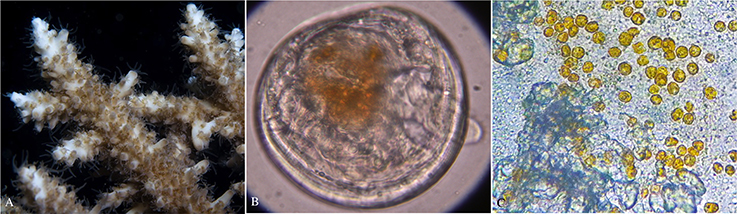
Figure 2. Symbiodinium cells in both adult and larval host tissues. (A) Reef-building acroporid coral with brown coloration due to the presence of Symbiodinium, (B) Symbiodinium cells (orange-brownish area) in the digestive tract of a pediveliger larva of the giant clam Tridacna crocea, and (C) Symbiodinium cells in the gastrovascular cavity of a planula larva (squashed under a microscope) of the reef-building coral Mussismilia hispida.
Biochemical and Molecular Relationship
The metabolite exchange between Symbiodinium and its adult host has been extensively documented. The host supplies the symbiont with CO2 and other compounds for cellular synthesis such as nitrogen and phosphorus (Trench, 1979; Allemand et al., 1998; Leggat et al., 2003; Weis et al., 2008). In turn, the symbiont supplies the host with more than 90% of its metabolic requirement, in the form of organic compounds including glucose, glycerol, fatty acids, and amino acids (Muscatine, 1990; Grant et al., 1997; Papina et al., 2003; Burriesci et al., 2012). Still, very little is known about the metabolite exchange between Symbiodinium and metazoan larvae. To our knowledge. Kopp et al. (2016) is the only study to date confirming active metabolite exchange by showing that labeled 13C and 15N are translocated from symbiont to Pocillopora damicornis larvae. However, that same study found that these compounds contribute rather insignificantly to the larval nutrition. Other reports show that Symbiodinium types within clades A and C associated with coral and giant clam larvae produce a higher amount of the fatty acid DHA (docosahexaenoic acid, 22:6ω3–Mies et al., 2017c) and that Symbiodinium associated with giant clam larvae express a glycerol-synthesizing gene (Mies et al., 2017b), but in neither of those cases translocation was effectively confirmed.
One of the main issues hindering the better understanding of this relationship is the lack of molecular studies and information for Symbiodinium, also addressed in Leggat et al. (2011). The genomes for types within clades A, B, and F (Shoguchi et al., 2013; Lin et al., 2015; Aranda et al., 2016), transcriptomes for types within clades A-D (Bayer et al., 2012; Ladner et al., 2012; Parkinson et al., 2016) and the plastid genome for type B1 (Mungpakdee et al., 2014) and type C3 (Barbrook et al., 2014) have been sequenced in recent years, but further information, especially on metabolic pathways, is still missing. However, a few experiments investigated differential gene expression in coral larvae with and without symbionts, all of which reported the absence of or rather small gene expression differences in the transcriptomes of symbiotic hosts when compared to the aposymbiotic state (deBoer et al., 2007; Voolstra et al., 2009b; Schnitzler and Weis, 2010). Only recently, a study by Mohamed et al. (2016) detected a transient period of differential expression involving a limited number of genes (3% of assayed transcriptome) 4 h after the exposure of Acropora digitifera planula larvae to a competent strain of Symbiodinium. While yet to be applied for larval studies, symbiosis-specific genes have been identified recently in Aiptasia anemones (Bucher et al., 2016; Wolfowicz et al., 2016). From the symbiont perspective, apart from the identification of protein kinases that may be involved in the establishment of symbiosis (Rosic et al., 2014), a symbiosis-specific gene was identified in Symbiodinium clade A, an H+-ATPase (Bertucci et al., 2010). This gene is the only symbiosis-specific gene that had its expression verified in Symbiodinium associated with invertebrate larvae (Mies et al., 2017b,c). The expression of this gene was confirmed in the veliger larvae of the giant clams Tridacna crocea and T. maxima and in the planula larvae of the hermatypic coral Mussismilia hispida, suggesting that symbiotic interactions are present.
Evolutionary Perspective on Symbiosis Establishment
Symbiodinium is found in association with a diversity of polyphyletic hosts, which differ regarding aspects of symbiont uptake and symbiosis maintenance. Hence, the symbiosis between animal hosts and Symbiodinium likely formed independently on multiple occasions. The ability of Symbiodinium as well as other dinoflagellates to establish symbioses with such a diversity of hosts may derive from their evolutionary background as recently suggested by Aranda et al. (2016). Parasitism is common in many extant dinoflagellates (Kuperman and Matey, 1999) as well as their closest relatives, the apicomplexans (Shoguchi et al., 2013; Lin et al., 2015). It is thus possible that a putative parasitic evolutionary background of Symbiodinium facilitated the infection of different hosts and their subsequent proliferation as endosymbionts. Hence, while the initial symbiosis of metazoan hosts and Symbiodinium may have been of parasitic nature, these symbioses evolved toward mutualistic interactions over time. Indeed, Aranda et al. (2016) recently confirmed adaptations in Symbiodinium, which may have facilitated the nutrient exchange with their hosts, such as an enrichment in carbon and nitrogen transporter domains. Yet, or as evidence to the derived mutualism notion, even extant metazoan-Symbiodinium associations are not exclusively mutualistic interactions (Stat et al., 2008; Lesser et al., 2013). Rather these interactions may exist along a continuum ranging from parasitism to mutualism depending on the identity of the host and symbiont (Sachs and Wilcox, 2006; Stat et al., 2008). Hence, the degree of mutualism between these partners may ultimately dependent on the specificity of this association and the ability of the host to select for beneficial symbionts. Further, as the performance of Symbiodinium types depends on their host as well as environmental conditions, different hosts may select for different symbionts (Baker, 2003; Mieog et al., 2009). Thereby, the large diversity of metazoan hosts found in association with Symbiodinium likely contributed to the rapid divergence found in this genus (Pochon et al., 2006; Hume et al., 2016).
Impact of Climate Change
Climate change is having a significant impact on coral reef ecosystems, particularly because of ocean acidification and the rise in seawater temperature (Hoegh-Guldberg, 1999; Hughes et al., 2003; Pandolfi et al., 2003; Hoegh-Guldberg et al., 2007). While the effects of ocean acidification have been investigated independently in marine invertebrate larvae and Symbiodinium (Brading et al., 2011; Nakamura et al., 2011; Waldbusser et al., 2013; Rivest and Hofmann, 2014), they yet need to be assessed on invertebrate larva-Symbiodinium associations. Nevertheless, it is known that the effects of ocean acidification on cultured (free-living) Symbiodinium is phylotype-specific, with some strains (such as A13 and A2) in fact benefitting from it and increasing their photosynthetic capacity (Brading et al., 2011). As it has also been shown that symbiont presence may mitigate the combined effects of acidification and hypoxia in non-calcifying hosts (Klein et al., 2017), it would be interesting to verify whether the same would happen during host larval development.
In contrast, the bleaching phenomenon, defined by the loss of Symbiodinium cells from host tissue due to rising seawater surface temperatures has been well documented (Brown, 1997; Hoegh-Guldberg, 1999; Fitt et al., 2001). For adult and larval hosts alike, the presence of symbionts is generally regarded as positive for coral reef larvae development and fitness, but under thermal stress it may have the opposite effect. High temperatures cause the symbionts to become a source of reactive oxygen species (ROS), which leads to significantly higher larval mortality (Weis, 2008; Baird et al., 2009; Yakovleva et al., 2009; Schnitzler et al., 2012). Some of the effects of ROS include DNA damage and higher rates of antioxidant activity (Yakovleva et al., 2009; Nesa et al., 2012).
The bleaching threshold for corals and coral larvae alike is usually only 1.0–1.5°C above the prevailing maximum temperature (Edmunds et al., 2005; Mieog et al., 2009). At this time, the expression of heat-shock proteins and oxidative-stress genes becomes detectable (DeSalvo et al., 2008, 2010; Rodriguez-Lanetty et al., 2009; Meyer et al., 2011). Data by Mies et al. (2017c) show that coral and giant clam larvae from Vietnam bleach at 29°C and hardly survive past 32°C. However, the highly-tolerant larvae of Pocillopora damicornis (from Okinawa, Japan) have been shown to withstand a temperature of 32°C, despite losing the majority of its symbiont cells (Haryanti et al., 2015). Nevertheless, the bleaching threshold may be greatly influenced by host and symbiont physiology and genetics, and also by the oceanographic conditions in situ (Roik et al., 2016). The symbiont type associated with the larvae may also influence bleaching intensity, as coral and giant clam larvae associated with Symbiodinium types within A and C bleach at lower rates than types within clades B, D, E, and F (Mies et al., 2017c). However, further investigation is required, especially for strains in clade D, which increase the adult host temperature tolerance threshold and become dominant during bleaching events (Berkelmans and van Oppen, 2006; Jones et al., 2008; Mieog et al., 2009; Ladner et al., 2012). Taken together, the effects of climate change on larval-symbiont associations are not clear at present, but increasing seawater temperatures may lead to increased loads of ROS, which in turn may limit the association of certain symbiont types with host larvae.
Is There a Mutualism from the Start?
Symbiosis is usually defined as the long-term interaction between two different organisms and mutualism is defined as a beneficial relationship for both parties. Considering the information presented in the previous sections, it remains to be determined whether a mutualistic symbiosis is in place for most metazoan larva-Symbiodinium associations. According to Davy et al. (2012), the establishment of symbiosis between Symbiodinium and its host is a complex process that involves several steps: symbiont uptake, phagocytosis by host cells (in the case of intracellular relationship), function integration, symbiont reproduction, and long-term persistence. Therefore, for hosts in their larval development, the establishment of symbiosis would have to follow the steps in Figure 3, which include symbiont acquisition, transfer of symbionts from gut to endodermal tissue, metabolite exchange and symbiont reproduction and persistence.
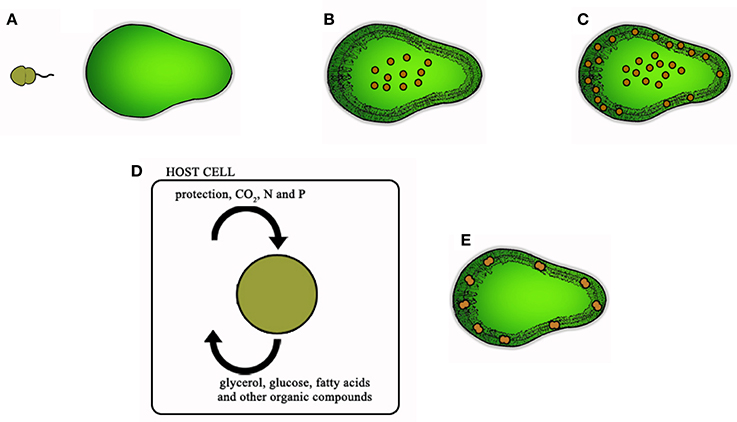
Figure 3. Steps for the establishment of a mutualistic relationship between Symbiodinium and metazoan larvae, using a coral planula as an example: (A) larval acquisition of free-living Symbiodinium zoospore, (B) symbiont inside gastrovascular cavity immediately after acquisition, (C) symbiont transfer to endodermal tissues, (D) metabolite/favor exchange, and (E) symbiont reproduction and long-term persistence.
The vast majority of experiments performed on invertebrate larva-Symbiodinium associations only visually observed the acquisition of symbionts and no metabolite exchange was detected, which fails to meet the criteria and events described above and shown in Figure 3. Especially considering reports that some larvae may digest the symbionts instead of engaging in a symbiotic relationship (Titlyanov et al., 1998; Mies et al., 2017c). The available gene expression studies are inconclusive in regard to whether the observed gene expression changes in coral larvae are specific to the symbiont exposure (deBoer et al., 2007; Voolstra et al., 2009a; Schnitzler and Weis, 2010; Mohamed et al., 2016). For instance, it is unclear if these changes are related to a mutualistic relationship or to the mere presence of a foreign algal body. Yet, the reports of higher production of fatty acids by Symbiodinium associated with coral and giant clam larvae (Mies et al., 2017c) and increases in larval caloric content and competence due to symbiont presence (Ben-David-Zaslow and Benayahu, 1998) suggest metabolite exchange. To date, perhaps the most compelling confirmation of a symbiosis between metazoan larvae and Symbiodinium may come from the expression of symbiosis-specific marker genes and the translocation of organic compounds. In this regard, the studies by Mies et al. (2017b,c) and Kopp et al. (2016) may provide critical support. These studies show the expression of a putative symbiosis-specific gene by Symbiodinium associated with coral (Mussismilia hispida) and giant clam (Tridacna crocea and T. maxima) larvae and the transfer of labeled carbon and nitrogen from symbiont to coral planulae (Pocillopora damicornis), respectively. However, these authors also point out that the energetic intake from this is insignificant for larvae nutrition, supporting that the initial symbiotic relationship might not be mutualistic in nature. Hence, the search for marker genes that could potentially disentangle different states in the symbiotic relationship (i.e., from an initial parasitic or commensal to a mutualistic interaction) is warranted.
Taken together, hosting Symbiodinium may be of no or little direct benefit to early larval animal host life stages. Yet, a mutualistic relationship is present in adult invertebrate–Symbiodinium associations. Thus, a gradual transition of the nature of the symbiotic association toward mutualism has to occur at one point during host development. In this context, the selection of symbionts within the host may increase the relative abundance of beneficial symbionts (Wolfowicz et al., 2016). Further, the rate of carbon translocation per symbiont may increase as the density of symbionts increases in relation to the host cells. The translocation of carbon by Symbiodinium requires the availability of excess carbon (Ezzat et al., 2015). In mature symbioses, it is suggested that this is achieved by the constant nitrogen limitation of symbiont growth (Falkowski et al., 1993; Pogoreutz et al., 2017). Yet, it is questionable whether algal symbionts are nitrogen-limited during early larval host stages. Nitrogen from host metabolism is an important source for algal nutrition (Rädecker et al., 2015). However, the ratio of symbiont to host biomass is significantly higher in early larval stages than in adult hosts (Muscatine et al., 1998). Thus, significantly more host-derived nitrogen may be available to Symbiodinium during early larval development. While this would enable a rapid proliferation of symbionts, it would inhibit the availability of excess carbon available for translocation to the host. Hence, the onset of nitrogen limitation and subsequent initiation of carbon translocation may only occur once the density of symbionts in association with the host has passed a certain threshold. This scenario, although speculative at this point, would explain the transition toward mutualistic interactions during the maturation of the symbiosis between Symbiodinium and marine animal invertebrates.
Conclusions
Our review provides an analysis on the relationship between Symbiodinium and marine invertebrate larvae. The information compiled and discussed shows that there is significant heterogeneity in larval response to initial infection by the symbiont, even within the same host taxon. Some species may digest symbionts while others actively engage in metabolite exchange and certain larval forms apparently select symbiont types while others do not. Symbiont presence may be imperative for the larval development and recruitment of certain host groups, while symbiont identity and homology may improve larval fitness and contribute to thermal tolerance. However, for the majority of cases investigated, the mutualistic relationship cannot be unequivocally confirmed. Rather, from the current available data, we suggest a gradual transition from parasitism/commensalism to mutualism, both on evolutionary and larval development time scales.
Author Contributions
All authors listed, have made substantial, direct and intellectual contribution to the work, and approved it for publication.
Conflict of Interest Statement
The authors declare that the research was conducted in the absence of any commercial or financial relationships that could be construed as a potential conflict of interest.
Acknowledgments
We would like to thank Arthur Güth and Linda Waters for their important inputs that greatly contributed to the production of this manuscript, and also Anna Roik for the acroporid coral photograph and Juliana Ali for the illustrations.
References
Abrego, D., van Oppen, M. J., and Willis, B. L. (2009). Highly infectious symbiont dominates initial uptake in coral juveniles. Mol. Ecol. 18, 3518–3531. doi: 10.1111/j.1365-294X.2009.04275.x
Achituv, Y., Benayahu, Y., and Hanania, J. (1992). Planulae brooding and acquisition of zooxanthellae in Xenia macrospiculata (Cnidaria: Octocorallia). Helgoländer Meeresuntersuchungen 46, 301–310. doi: 10.1007/BF02367101
Adams, L. M., Cumbo, V. R., and Takabayashi, M. (2009). Exposure to sediment enhances primary acquisition of Symbiodinium by asymbiotic coral larvae. Mar. Ecol. Prog. Ser. 377, 149–156. doi: 10.3354/meps07834
Ahmadian, R., Burghardt, I., and Shepherd, U. L. (2016). Embryonic development of the solar-powered nudibranch Phyllodesmium lizardensis (Gastropoda: Nudibranchia). Molluscan Res. 36, 285–289. doi: 10.1080/13235818.2016.1150770
Allemand, D., Furla, P., and Bénazet-Tambutté, S. (1998). Mechanisms of carbon acquisition for endosymbiont photosynthesis in Anthozoa. Can. J. Bot. 76, 925–941. doi: 10.1139/b98-086
Aranda, M., Li, Y., Liew, Y. J., Baumgarten, S., Simakov, O., Wilson, M., et al. (2016). Genomes of coral dinoflagellate symbionts highlight evolutionary adaptations conducive to a symbiotic lifestyle. Sci. Rep. 6:39734. doi: 10.1038/srep39734
Arif, C., Daniels, C., Bayer, T., Banguera-Hinestroza, E., Barbrook, A., Howe, C. J., et al. (2014). Assessing Symbiodinium diversity in scleractinian corals via next-generation sequencing-based genotyping of the ITS2 rDNA region. Mol. Ecol. 23, 4418–4433. doi: 10.1111/mec.12869
Baird, A. H., Cumbo, V. R., Leggat, W., and Rodriguez-Lanetty, M. (2007). Fidelity and flexibility in coral symbioses. Mar. Ecol. Prog. Ser. 347, 307–309. doi: 10.3354/meps07220
Baird, A. H., Guest, J. R., and Willis, B. L. (2009). Systematic and biogeographical patterns in the reproductive biology of scleractinian corals. Annu. Rev. Ecol. Evol. Syst. 40, 551–571. doi: 10.1146/annurev.ecolsys.110308.120220
Baker, A. C. (2003). Flexibility and specificity in coral-algal symbiosis: diversity, ecology, and biogeography of Symbiodinium. Annu. Rev. Ecol. Evol. Syst. 34, 661–689. doi: 10.1146/annurev.ecolsys.34.011802.132417
Banaszak, A. T., García Ramos, M., and Goulet, T. L. (2013). The symbiosis between the gastropod Strombus gigas and the dinoflagellate Symbiodinium: an ontogenic journey from mutualism to parasitism. J. Exp. Mar. Bio. Ecol. 449, 358–365. doi: 10.1016/j.jembe.2013.10.027
Barbrook, A. C., Voolstra, C. R., and Howe, C. J. (2014). The chloroplast genome of a Symbiodinium sp. clade C3 isolate. Protist 165, 1–13. doi: 10.1016/j.protis.2013.09.006
Barneah, O., Brickner, I., Hooge, M., Weis, V. M., and Benayahu, Y. (2007a). First evidence of maternal transmission of algal endosymbionts at an oocyte stage in a triploblastic host, with observations on reproduction in Waminoa brickneri (Acoelomorpha). Invertebr. Biol. 126, 113–119. doi: 10.1111/j.1744-7410.2007.00082.x
Barneah, O., Brickner, I., Hooge, M., Weis, V. M., LaJeunesse, T. C., and Benayahu, Y. (2007b). Three party symbiosis: acoelomorph worms, corals and unicellular algal symbionts in Eilat (Red Sea). Mar. Biol. 151, 1215–1223. doi: 10.1007/s00227-006-0563-2
Barneah, O., Weis, V. M., Perez, S., and Benayahu, Y. (2004). Diversity of dinoflagellate symbionts in Red Sea soft corals: mode of symbiont acquisition matters. Mar. Ecol. Prog. Ser. 275, 89–95. doi: 10.3354/meps275089
Batovska, J., Cogan, N. O., Lynch, S. E., and Blacket, M. J. (2016). Using next-generation sequencing for DNA barcoding: capturing allelic variation in ITS2. G3 Genes Genomes Genet. 7, 19–29. doi: 10.1534/g3.116.036145
Baumgarten, S., Simakov, O., Esherick, L. Y., Liew, Y. J., Lehnert, E. M., Michell, C. T., et al. (2015). The genome of Aiptasia, a sea anemone model for coral symbiosis. Proc. Natl. Acad. Sci. U.S.A. 112, 11893–11898. doi: 10.1073/pnas.1513318112
Bay, L. K., Cumbo, V. R., Abrego, D., Kool, J. T., Ainsworth, T. D., and Willis, B. L. (2011). Infection dynamics vary between Symbiodinium types and cell surface treatments during establishment of endosymbiosis with coral larvae. Diversity 3, 356–374. doi: 10.3390/d3030356
Bayer, T., Aranda, M., Sunagawa, S., Yum, L. K., DeSalvo, M. K., Lindquist, E., et al. (2012). Symbiodinium transcriptomes: genome insights into the dinoflagellate symbionts of reef-building corals. PLoS ONE 7:e35269. doi: 10.1371/journal.pone.0035269
Belda-Baillie, C. A., Sison, M., Silvestre, V., Villamor, K., Monje, V., Gomez, E. D., et al. (1999). Evidence for changing symbiotic algae in juvenile tridacnids. J. Exp. Mar. Bio. Ecol. 241, 207–221. doi: 10.1016/S0022-0981(99)00079-9
Benayahu, Y., Achituv, Y., and Berner, T. (1988). Embryogenesis and acquisition of algal symbionts by planulae of Xenia umbellata (Octocorallia: Alcyonacea). Mar. Biol. 100, 93–101. doi: 10.1007/BF00392959
Benayahu, Y., Weil, D., and Malik, Z. (1992). Entry of algal symbionts into oocytes of the coral Litophyton arboreum. Tissue Cell 24, 473–482. doi: 10.1016/0040-8166(92)90063-D
Ben-David-Zaslow, R., and Benayahu, Y. (1998). Competence and longevity in planulae of several species of soft corals. Mar. Ecol. Prog. Ser. 163, 235–243. doi: 10.3354/meps163235
Berkelmans, R., and van Oppen, M. J. (2006). The role of zooxanthellae in the thermal tolerance of corals: a “nugget of hope” for coral reefs in an era of climate change. Proc. R. Soc. B. Biol. Sci. 273, 2305–2312. doi: 10.1098/rspb.2006.3567
Bertucci, A., Tambutté, E., Tambutté, S., Allemand, D., and Zoccola, D. (2010). Symbiosis-dependent gene expression in coral-dinoflagellate association: cloning and characterization of a P-type H+-ATPase gene. Proc. R. Soc. B. Biol. Sci. 277, 87–95. doi: 10.1098/rspb.2009.1266
Biquand, E., Okubo, N., Aihara, Y., Rolland, V., Hayward, D. C., Hatta, M., et al. (2017). Acceptable symbiont cell size differs among cnidarian species and may limit symbiont diversity. ISME J. doi: 10.1038/ismej.2017.17. [Epub ahead of print].
Boulotte, N. M., Dalton, S. J., Carroll, A. G., Harrison, P. L., Putnam, H. M., Peplow, L. M., et al. (2016). Exploring the Symbiodinium rare biosphere provides evidence for symbiont switching in reef-building corals. ISME J. 10, 1–9. doi: 10.1038/ismej.2016.54
Brading, P., Warner, M. E., Davey, P., Smith, D. J., Achterberg, E. P., and Suggett, D. J. (2011). Differential effects of ocean acidification on growth and photosynthesis among phylotypes of Symbiodinium (Dinophyceae). Limnol. Oceanogr. 56, 927–938. doi: 10.4319/lo.2011.56.3.0927
Brown, B. E. (1997). Adaptations of reef corals to physical environmental stress. Adv. Mar. Biol. 31, 221–299. doi: 10.1016/S0065-2881(08)60224-2
Bucher, M., Wolfowicz, I., Voss, P. A., Hambleton, E. A., and Guse, A. (2016). Development and Symbiosis establishment in the cnidarian endosymbiosis model Aiptasia sp. Sci. Rep. 6:19867. doi: 10.1038/srep19867
Burghardt, I., and Wägele, H. (2014). The symbiosis between the “solar-powered” nudibranch Melibe engeli Risbec, 1937 (Dendronotoidea) and Symbiodinium sp. (Dinophyceae). J. Molluscan Stud. 80, 508–517. doi: 10.1093/mollus/eyu043
Burriesci, M. S., Raab, T. K., and Pringle, J. R. (2012). Evidence that glucose is the major transferred metabolite in dinoflagellate-cnidarian symbiosis. J. Exp. Biol. 215, 3467–3477. doi: 10.1242/jeb.070946
Bythell, J. C., Douglas, A. E., Sharp, V. A., Searle, J. B., and Brown, B. E. (1997). Algal genotype and photoacclimatory responses of the symbiotic alga Symbiodinium in natural populations of the sea anemone Anemonia viridis. Proc. R. Soc. B Biol. Sci. 264, 1277–1282. doi: 10.1098/rspb.1997.0176
Carlos, A. A., Baillie, B. K., and Maruyama, T. (2000). Diversity of dinoflagellate symbionts (zooxanthellae) in a host individual. Mar. Ecol. Prog. Ser. 195, 93–100. doi: 10.3354/meps195093
Carroll, D. J., and Kempf, S. C. (1990). Laboratory culture of the aeolid nudibranch Berghia verrucicornis (Mollusca, Opisthobranchia): some aspects of its development and life history. Biol. Bull. 179, 243–253. doi: 10.2307/1542315
Coffroth, M. A., and Santos, S. R. (2005). Genetic diversity of symbiotic dinoflagellates in the genus Symbiodinium. Protist 156, 19–34. doi: 10.1016/j.protis.2005.02.004
Cumbo, V. R., Baird, A. H., and van Oppen, M. J. H. (2012). The promiscuous larvae: flexibility in the establishment of symbiosis in corals. Coral Reefs 32, 111–120. doi: 10.1007/s00338-012-0951-7
Davy, S. K., Allemand, D., and Weis, V. M. (2012). Cell Biology of cnidarian-dinoflagellate Symbiosis. Microbiol. Mol. Biol. Rev. 76, 229–261. doi: 10.1128/MMBR.05014-11
deBoer, M. L., Krupp, D. A., and Weis, V. M. (2007). Proteomic and transcriptional analyses of coral larvae newly engaged in symbiosis with dinoflagellates. Comp. Biochem. Physiol. Part D Genomics Proteomics 2, 63–73. doi: 10.1016/j.cbd.2006.11.003
DeSalvo, M. K., Sunagawa, S., Voolstra, C. R., and Medina, M. (2010). Transcriptomic responses to heat stress and bleaching in the elkhorn coral Acropora palmata. Mar. Ecol. Prog. Ser. 402, 97–113. doi: 10.3354/meps08372
DeSalvo, M. K., Voolstra, C. R., Sunagawa, S., Schwarz, J. A., Stillman, J. H., Coffroth, M. A., et al. (2008). Differential gene expression during thermal stress and bleaching in the Caribbean coral Montastraea faveolata. Mol. Ecol. 17, 3952–3971. doi: 10.1111/j.1365-294X.2008.03879.x
Dionísio, G., Rosa, R., Leal, M. C., Cruz, S., Brandão, C., Calado, G., et al. (2013). Beauties and beasts: a portrait of sea slugs aquaculture. Aquaculture 408–409, 1–14. doi: 10.1016/j.aquaculture.2013.04.033
Dunn, S. R., and Weis, V. M. (2009). Apoptosis as a post-phagocytic winnowing mechanism in a coral-dinoflagellate mutualism. Environ. Microbiol. 11, 268–276. doi: 10.1111/j.1462-2920.2008.01774.x
Edmunds, P. J., Gates, R. D., Leggat, W., Hoegh-Guldberg, O., and Allen-Requa, L. (2005). The effect of temperature on the size and population density of dinoflagellates in larvae of the reef coral Porites astreoides. Invertebr. Biol. 124, 185–193. doi: 10.1111/j.1744-7410.2005.00018.x
Erwin, P. M., and Szmant, A. M. (2010). Settlement induction of Acropora palmata planulae by a GLW-amide neuropeptide. Coral Reefs 29, 929–939. doi: 10.1007/s00338-010-0634-1
Ezzat, L., Maguer, J.-F., Grover, R., and Ferrier-Pagès, C. (2015). New insights into carbon acquisition and exchanges within the coral–dinoflagellate symbiosis under and supply. Proc. R. Soc. B Biol. Sci. 282:20150610. doi: 10.1098/rspb.2015.0610
Falkowski, P. G., Dubinsky, Z., Muscatine, L., and McCloskey, L. (1993). Population control in symbiotic corals. Bioscience 43, 606–611. doi: 10.2307/1312147
Fitt, W. K., Brown, B. E., Warner, M. E., and Dunne, R. P. (2001). Coral bleaching: interpretation of thermal tolerance limits and thermal thresholds in tropical corals. Coral Reefs 20, 51–65. doi: 10.1007/s003380100146
Fitt, W. K., Fisher, C. R., and Trench, R. K. (1984). Larval biology of tridacnid clams. Aquaculture 39, 181–195. doi: 10.1016/0044-8486(84)90265-5
Fitt, W. K., Fisher, C. R., and Trench, R. K. (1986). Contribution of the symbiotic dinoflagellate Symbiodinium microadriaticum to the nutrition, growth and survival of larval and juvenile tridacnid clams. Aquaculture 55, 5–22. doi: 10.1016/0044-8486(86)90051-7
Fitt, W. K., and Trench, R. K. (1981). Spawning, development, and acquisition of zooxanthellae by Tridacna squamosa (Mollusca, Bivalvia). Biol. Bull. 161, 213–235. doi: 10.2307/1540800
Fransolet, D., Roberty, S., and Plumier, J. C. (2012). Establishment of endosymbiosis: the case of cnidarians and Symbiodinium. J. Exp. Mar. Bio. Ecol. 420–421, 1–7. doi: 10.1016/j.jembe.2012.03.015
Freudenthal, H. D. (1962). Symbiodinium gen. nov. and Symbiodinium microadriaticum sp. nov., a zooxanthella: taxonomy, life cycle, and morphology. J. Protozool. 9, 45–52. doi: 10.1111/j.1550-7408.1962.tb02579.x
Garcia Ramos, M., and Banaszak, A. T. (2014). Symbiotic association between Symbiodinium and the gastropod Strombus gigas: larval acquisition of symbionts. Mar. Biotechnol. 16, 193–201. doi: 10.1007/s10126-013-9536-x
Goulet, T. L. (2006). Most corals may not change their symbionts. Mar. Ecol. Prog. Ser. 321, 1–7. doi: 10.3354/meps321001
Grant, A. J., Rémond, M., People, J., and Hinde, R. (1997). Effects of host-tissue homogenate of the scleractinian coral Plesiastrea versipora on glycerol metabolism in isolated symbiotic dinoflagellates. Mar. Biol. 128, 665–670. doi: 10.1007/s002270050133
Hambleton, E. A., Guse, A., and Pringle, J. R. (2014). Similar specificities of symbiont uptake by adults and larvae in an anemone model system for coral biology. J. Exp. Biol. 217, 1613–1619. doi: 10.1242/jeb.095679
Harii, S., Yasuda, N., Rodriguez-Lanetty, M., Irie, T., and Hidaka, M. (2009). Onset of symbiosis and distribution patterns of symbiotic dinoflagellates in the larvae of scleractinian corals. Mar. Biol. 156, 1203–1212. doi: 10.1007/s00227-009-1162-9
Harrison, P. L. (2011). “Sexual reproduction of scleractinian corals,” in Coral Reefs: An Ecosystem in Transition, eds Z. Dubinsky and N. Stambler (Heidelberg: Springer), 59–85.
Harrison, P. L., and Wallace, C. C. (1990). “Reproduction, dispersal and recruitment of scleractinian corals,” in Ecosystems of the World, ed Z. Dubinsky (Amsterdam: Elsevier), 133–208.
Haryanti, D., Yasuda, N., Harii, S., and Hidaka, M. (2015). High tolerance of symbiotic larvae of Pocillopora damicornis to thermal stress. Zool. Stud. 54:52. doi: 10.1186/s40555-015-0134-7
Heslinga, G. A., Perron, F. E., and Orak, O. (1984). Mass culture of giant clams (F. Tridacnidae) in Palau. Aquaculture 39, 197–215. doi: 10.1016/0044-8486(84)90266-7
Heslinga, G. A., Watson, T. C., and Isamu, T. (eds.). (1990). Giant Clam Farming. Honolulu.: Pacific Fisheries Development Foundation (NMFS/NOAA).
Hikosaka-Katayama, T., Koike, K., Yamashita, H., Hikosaka, A., and Koike, K. (2012). Mechanisms of maternal inheritance of dinoflagellate symbionts in the acoelomorph worm Waminoa litus. Zool. Sci. 29, 559–567. doi: 10.2108/zsj.29.559
Hill, M., Allenby, A., Ramsby, B., Schönberg, C., and Hill, A. (2011). Symbiodinium diversity among host clionaid sponges from Caribbean and Pacific reefs: evidence of heteroplasmy and putative host-specific symbiont lineages. Mol. Phylogenet. Evol. 59, 81–88. doi: 10.1016/j.ympev.2011.01.006
Hirose, E., Iwai, K., and Maruyama, T. (2006). Establishment of the photosymbiosis in the early ontogeny of three giant clams. Mar. Biol. 148, 551–558. doi: 10.1007/s00227-005-0119-x
Hoegh-Guldberg, O. (1999). Climate change, coral bleaching and the future of the world's coral reefs. Mar. Freshw. Res. 50, 839–866. doi: 10.1071/MF99078
Hoegh-Guldberg, O., Mumby, P. J., Hooten, A. J., Steneck, R. S., Greenfield, P., Gomez, E., et al. (2007). Coral reefs under rapid climate change and ocean acidification. Science 318, 1737–1742. doi: 10.1126/science.1152509
Hofmann, D. K., Fitt, W. K., and Fleck, J. (1996). Checkpoints in the life-cycle of Cassiopea spp.: control of metagenesis and metamorphosis in a tropical jellyfish. Int. J. Dev. Biol. 40, 331–338.
Huang, H. J., Wang, L. H., Chen, W. N. U., Fang, L. S., and Chen, C. S. (2008). Developmentally regulated localization of endosymbiotic dinoflagellates in different tissue layers of coral larvae. Coral Reefs 27, 365–372. doi: 10.1007/s00338-007-0337-4
Hughes, T. P., Baird, A. H., Bellwood, D. R., Card, M., Connolly, S. R., Folke, C., et al. (2003). Climate change, human impacts, and the resilience of coral reefs. Science 301, 929–933. doi: 10.1126/science.1085046
Hume, B. C., D'Angelo, C., Smith, E. G., Stevens, J. R., Burt, J., and Wiedenmann, J. (2015). Symbiodinium thermophilum sp. nov., a thermotolerant symbiotic alga prevalent in corals of the world's hottest sea, the Persian/Arabian Gulf. Sci. Rep. 5:8562. doi: 10.1038/srep08562
Hume, B. C., Voolstra, C. R., Arif, C., D'Angelo, C., Burt, J. A., Eyal, G., et al. (2016). Ancestral genetic diversity associated with the rapid spread of stress-tolerant coral symbionts in response to Holocene climate change. Proc. Natl. Acad. Sci. U.S.A. 113, 4416–4421. doi: 10.1073/pnas.1601910113
Jones, A. M., Berkelmans, R., van Oppen, M. J., Mieog, J. C., and Sinclair, W. (2008). A community change in the algal endosymbionts of a scleractinian coral following a natural bleaching event: field evidence of acclimatization. Proc. R. Soc. B. Biol. Sci. 275, 1359–1365. doi: 10.1098/rspb.2008.0069
Kempf, S. C. (1984). Symbiosis between the zooxanthella Symbiodinium (= Gymnodinium) microadriaticum (Freudenthal) and four species of nudibranchs. Biol. Bull. 166, 110–126. doi: 10.2307/1541435
Kempf, S. C. (1991). A “primitive” symbiosis between the aeolid nudibranch Berghia verrucicornis (A. Costa, 1867) and a zooxanthella. J. Molluscan Stud. 57, 75–85.
Klein, S. G., Pitt, K. A., Nitschke, M. R., Goyen, S., Welsh, D. T., Suggett, D. J., et al. (2017). Symbiodinium mitigate the combined effects of hypoxia and acidification on a non-calcifying organism. Glob. Chang. Biol. doi: 10.1111/gcb.13718. [Epub ahead of print].
Koike, K., Jimbo, M., Sakai, R., Kaeriyama, M., Muramoto, K., Ogata, T., et al. (2004). Octocoral chemical signaling selects and controls dinoflagellate symbionts. Biol. Bull. 207, 80–86. doi: 10.2307/1543582
Kopp, C., Domart-Coulon, I., Barthelemy, D., and Meibom, A. (2016). Nutritional input from dinoflagellate symbionts in reef-building corals is minimal during planula larval life stage. Sci. Adv. 2:e1500681. doi: 10.1126/sciadv.1500681
Kuperman, B. I., and Matey, V. E. (1999). Massive infestation by Amyloodinium ocellatum (Dinoflagellida) of fish in a highly saline lake, Salton Sea, California, USA. Dis. Aquat. Organ. 39, 65–73. doi: 10.3354/dao039065
Ladner, J. T., Barshis, D. J., and Palumbi, S. R. (2012). Protein evolution in two co-occurring types of Symbiodinium: an exploration into the genetic basis of thermal tolerance in Symbiodinium clade D. BMC Evol. Biol. 12:217. doi: 10.1186/1471-2148-12-217
LaJeunesse, T. C. (2001). Investigating the biodiversity, ecology, and phylogeny of endosymbiotic dinoflagellates in the genus Symbiodinium using the ITS region: in search of a “species” level marker. J. Phycol. 37, 866–880. doi: 10.1046/j.1529-8817.2001.01031.x
LaJeunesse, T. C. (2002). Diversity and community structure of symbiotic dinoflagellates from Caribbean coral reefs. Mar. Biol. 141, 387–400. doi: 10.1007/s00227-002-0829-2
LaJeunesse, T. C. (2005). “Species” radiations of symbiotic dinoflagellates in the Atlantic and Indo-Pacific since the Miocene-Pliocene transition. Mol. Biol. Evol. 22, 570–581. doi: 10.1093/molbev/msi042
LaJeunesse, T. C., Parkinson, J. E., and Reimer, J. D. (2012). A genetics-based description of Symbiodinium minutum sp. nov. and S. psygmophilum sp. nov. (dinophyceae), two dinoflagellates symbiotic with cnidaria. J. Phycol. 48, 1380–1391. doi: 10.1111/j.1529-8817.2012.01217.x
LaJeunesse, T. C., and Trench, R. K. (2000). Biogeography of two species of Symbiodinium (Freudenthal) inhabiting the intertidal sea anemone Anthopleura elegantissima (Brandt). Biol. Bull. 199, 126–134. doi: 10.2307/1542872
Lee, M. J., Jeong, H. J., Jang, S. H., Lee, S. Y., Kang, N. S., Lee, K. H., et al. (2016). Most low-abundance “Background” Symbiodinium spp. Are transitory and have minimal functional significance for symbiotic corals. Microb. Ecol. 71, 771–83. doi: 10.1007/s00248-015-0724-2
Lee, S. Y., Jeong, H. J., Kang, N. S., Jang, T. Y., Jang, S. H., and Lajeunesse, T. C. (2015). Symbiodinium tridacnidorum sp. nov., a dinoflagellate common to Indo-Pacific giant clams, and a revised morphological description of Symbiodinium microadriaticum Freudenthal, emended Trench & Blank. Eur. J. Phycol. 50, 155–172. doi: 10.1080/09670262.2015.1018336
Leggat, W., Buck, B. H., Grice, A., and Yellowlees, D. (2003). The impact of bleaching on the metabolic contribution of dinoflagellate symbionts to their giant clam host. Plant Cell Environ. 26, 1951–1961. doi: 10.1046/j.0016-8025.2003.01111.x
Leggat, W., Yellowlees, D., and Medina, M. (2011). Recent progress in Symbiodinium transcriptomics. J. Exp. Mar. Bio. Ecol. 408, 120–125. doi: 10.1016/j.jembe.2011.07.032
Lesser, M. P., Stat, M., and Gates, R. D. (2013). The endosymbiotic dinoflagellates (Symbiodinium sp.) of corals are parasites and mutualists. Coral Reefs 32, 603–611. doi: 10.1007/s00338-013-1051-z
Lin, S., Cheng, S., Song, B., Zhong, X., Lin, X., Li, W., et al. (2015). The Symbiodinium kawagutii genome illuminates dinoflagellate gene expression and coral symbiosis. Science 350, 691–694. doi: 10.1126/science.aad0408
Loh, W. K. W., Cowlishaw, M., and Wilson, N. G. (2006). Diversity of Symbiodinium dinoflagellate symbionts from the Indo-Pacific sea slug Pteraeolidia ianthina (Gastropoda: Mollusca). Mar. Ecol. Prog. Ser. 320, 177–184. doi: 10.3354/meps320177
Mariani, S., Piscitelli, M. P., and Uriz, M.-J. (2001). Temporal and spatial co-occurrence in spawning and larval release of Cliona viridis (Porifera: Hadromerida). J. Mar. Biol. Assoc. U.K. 81, 565–567. doi: 10.1017/S0025315401004246
Mariani, S., Uriz, M. J., and Turon, X. (2000). Larval bloom of the oviparous sponge Cliona viridis: coupling of larval abundance and adult distribution. Mar. Biol. 137, 783–790. doi: 10.1007/s002270000400
Marlow, H. Q., and Martindale, M. Q. (2007). Embryonic development in two species of scleractinian coral embryos: Symbiodinium localization and mode of gastrulation. Evol. Dev. 9, 355–367. doi: 10.1111/j.1525-142X.2007.00173.x
Mellas, R. E., McIlroy, S. E., Fitt, W. K., and Coffroth, M. A. (2014). Variation in symbiont uptake in the early ontogeny of the upside-down jellyfish, Cassiopea spp. J. Exp. Mar. Bio. Ecol. 459, 38–44. doi: 10.1016/j.jembe.2014.04.026
Meyer, E., Aglyamova, G. V., and Matz, M. V. (2011). Profiling gene expression responses of coral larvae (Acropora millepora) to elevated temperature and settlement inducers using a novel RNA-Seq procedure. Mol. Ecol. 20, 3599–3616. doi: 10.1111/j.1365-294x.2011.05205.x
Mieog, J. C., Olsen, J. L., Berkelmans, R., Bleuler-Martinez, S. A., Willis, B. L., and van Oppen, M. J. (2009). The roles and interactions of symbiont, host and environment in defining coral fitness. PLoS ONE 4:e6364. doi: 10.1371/journal.pone.0006364
Mies, M., Braga, F., Scozzafave, M. S., Lemos, D., and Sumida, P. Y. G. (2012). Early development, survival and growth rates of the giant clam Tridacna crocea (Bivalvia: Tridacnidae). Braz. J. Oceanogr. 60, 129–135. doi: 10.1590/S1679-87592012000200003
Mies, M., Scozzafave, M. S., Braga, F., and Sumida, P. Y. G. (2017a). Giant Clams. eds R. Calado, I. Olivotto, M. P. Oliver, and G. J. Holt (Oxford: Wiley-Blackwell Publishing), 510–535
Mies, M., Van Sluys, M. A., Metcalfe, C. J., and Sumida, P. Y. G. (2017b). Molecular evidence of symbiotic activity between Symbiodinium and Tridacna maxima larvae. Symbiosis 72, 13–22. doi: 10.1007/s13199-016-0433-8
Mies, M., Voolstra, C. R., Castro, C. B., Pires, D. O., Calderon, E. N., and Sumida, P. Y. G. (2017c). Expression of a symbiosis-specific gene in Symbiodinium type A1 associated with coral, nudibranch and giant clam larvae. R. Soc. Open Sci. 4:170253. doi: 10.1098/rsos.170253
Mohamed, A. R., Cumbo, V., Harii, S., Shinzato, C., Chan, C. X., Ragan, M. A., et al. (2016). The transcriptomic response of the coral Acropora digitifera to a competent Symbiodinium strain: the symbiosome as an arrested early phagosome. Mol. Ecol. 25, 3127–3141. doi: 10.1111/mec.13659
Montgomery, M. K., and Kremer, P. M. (1995). Transmission of symbiotic dinoflagellates through the sexual cycle of the host scyphozoan Linuche unguiculata. Marine Biol. 124, 147–155. doi: 10.1007/BF00349156
Mordret, S., Romac, S., Henry, N., Colin, S., Carmichael, M., Berney, C., et al. (2016). The symbiotic life of Symbiodinium in the open ocean within a new species of calcifying ciliate (Tiarina sp.). ISME J. 10, 1424–1436. doi: 10.1038/ismej.2015.211
Morse, A. N. C., Iwao, K., Baba, M., Shimoike, K., Hayashibara, T., and Omori, M. (1996). An ancient chemosensory mechanism new life to coral reefs. Biol. Bull. 191, 149–154. doi: 10.2307/1542917
Mungpakdee, S., Shinzato, C., Takeuchi, T., Kawashima, T., Koyanagi, R., Hisata, K., et al. (2014). Massive gene transfer and extensive RNA editing of a symbiotic dinoflagellate plastid genome. Genome Biol. Evol. 6, 1408–1422. doi: 10.1093/gbe/evu109
Muscatine, L. (1990). “The role of symbiotic algae in carbon and energy flux in coral reefs,” in Ecosystems of the World, ed Z. Dubinsky (Amsterdam: Elsevier), 75–87.
Muscatine, L., Ferrier-Pagès, C., Blackburn, A., Gates, R. D., Baghdasarian, G., and Allemand, D. (1998). Cell-specific density of symbiotic dinoflagellates in tropical anthozoans. Coral Reefs 17, 329–337. doi: 10.1007/s003380050133
Nakamura, M., Ohki, S., Suzuki, A., and Sakai, K. (2011). Coral larvae under ocean acidification: survival, metabolism, and metamorphosis. PLoS ONE 6:e14521. doi: 10.1371/journal.pone.0014521
Nesa, B., Baird, A. H., Harii, S., Yakovleva, I., and Hidaka, M. (2012). Algal symbionts increase DNA damage in coral planulae exposed to sunlight. Zool. Stud. 51, 12–17.
Nitschke, M. R., Davy, S. K., and Ward, S. (2016). Horizontal transmission of Symbiodinium cells between adult and juvenile corals is aided by benthic sediment. Coral Reefs 35, 335–344. doi: 10.1007/s00338-015-1349-0
Pandolfi, J. M., Bradbury, R. H., Sala, E., Hughes, T. P., Bjorndal, K. A., Cooke, R. G., et al. (2003). Global trajectories of the long-term decline of coral reef ecosystems. Science 301, 955–958. doi: 10.1126/science.1085706
Papina, M., Meziane, T., and van Woesik, R. (2003). Symbiotic zooxanthellae provide the host-coral Montipora digitata with polyunsaturated fatty acids. Comp. Biochem. Physiol. Part B Biochem. Mol. Biol. 135, 533–537. doi: 10.1016/S1096-4959(03)00118-0
Parkinson, J. E., Baumgarten, S., Michell, C. T., Baums, I. B., LaJeunesse, T. C., and Voolstra, C. R. (2016). Gene expression variation resolves species and individual strains among coral-associated dinoflagellates within the genus Symbiodinium. Genome Biol. Evol. 8, 665–680. doi: 10.1093/gbe/evw019
Pechenik, J. A. (1990). Delayed metamorphosis by larvae of benthic marine invertebrates: does it occur? Is there a price to pay? Ophelia 32, 63–94. doi: 10.1080/00785236.1990.10422025
Pechenik, J. A. (1999). On the advantages and disadvantages of larval stages in benthic marine invertebrate life cycles. Mar. Ecol. Prog. Ser. 177, 269–297. doi: 10.3354/meps177269
Pochon, X., and Gates, R. D. (2010). A new Symbiodinium clade (Dinophyceae) from soritid foraminifera in Hawai'i. Mol. Phylogenet. Evol. 56, 492–497. doi: 10.1016/j.ympev.2010.03.040
Pochon, X., Montoya-Burgos, J. I., Stadelmann, B., and Pawlowski, J. (2006). Molecular phylogeny, evolutionary rates, and divergence timing of the symbiotic dinoflagellate genus Symbiodinium. Mol. Phylogenet. Evol. 38, 20–30. doi: 10.1016/j.ympev.2005.04.028
Pochon, X., Putnam, H. M., and Gates, R. D. (2014). Multi-gene analysis of Symbiodinium dinoflagellates: a perspective on rarity, symbiosis, and evolution. PeerJ 2:e394. doi: 10.7717/peerj.394
Pogoreutz, C., Rädecker, N., Cárdenas, A., Gärdes, A., Voolstra, C. R., and Wild, C. (2017). Sugar enrichment provides evidence for a role of nitrogen fixation in coral bleaching. Glob. Change Biol. doi: 10.1111/gcb.13695. [Epub ahead of print].
Rädecker, N., Pogoreutz, C., Voolstra, C. R., Wiedenmann, J., and Wild, C. (2015). Nitrogen cycling in corals: the key to understanding holobiont functioning? Trends Microbiol. 23, 490–497. doi: 10.1016/j.tim.2015.03.008
Rivest, E. B., and Hofmann, G. E. (2014). Responses of the metabolism of the larvae of Pocillopora damicornis to ocean acidification and warming. PLoS ONE 9:e96172. doi: 10.1371/journal.pone.0096172
Rodriguez-Lanetty, M., Harii, S., and Hoegh-Guldberg, O. (2009). Early molecular responses of coral larvae to hyperthermal stress. Mol. Ecol. 18, 5101–5114. doi: 10.1111/j.1365-294X.2009.04419.x
Rodriguez-Lanetty, M., Krupp, D. A., and Weis, V. M. (2004). Distinct ITS types of Symbiodinium in Clade C correlate with cnidarian/dinoflagellate specificity during onset of symbiosis. Mar. Ecol. Prog. Ser. 275, 97–102. doi: 10.3354/meps275097
Rodriguez-Lanetty, M., Wood-Charlson, E. M., Hollingsworth, L. L., Krupp, D. A., and Weis, V. M. (2006). Temporal and spatial infection dynamics indicate recognition events in the early hours of a dinoflagellate/coral symbiosis. Mar. Biol. 149, 713–719. doi: 10.1007/s00227-006-0272-x
Roik, A., Röthig, T., Roder, C., Ziegler, M., Kremb, S. G., and Voolstra, C. R. (2016). Year-long monitoring of physico-chemical and biological variables provide a comparative baseline of coral reef functioning in the central Red Sea. PLoS ONE 11:e0163939. doi: 10.1371/journal.pone.0163939
Rosic, N., Ling, E. Y., Chan, C. K., Lee, H. C., Kaniewska, P., Edwards, D., et al. (2014). Unfolding the secrets of coral-algal symbiosis. ISME J. 9, 1–13. doi: 10.1038/ismej.2014.182
Rowan, R., and Powers, D. A. (1991). Molecular genetic identification of symbiotic dinoflagellates (zooxanthellae). Mar. Ecol. Prog. Ser. 71, 65–73. doi: 10.3354/meps071065
Sachs, J. L., and Wilcox, T. P. (2006). A shift to parasitism in the jellyfish symbiont Symbiodinium microadriaticum. Proc. R. Soc. B Biol. Sci. 273, 425–429. doi: 10.1098/rspb.2005.3346
Schnitzler, C. E., Hollingsworth, L. L., Krupp, D. A., and Weis, V. M. (2012). Elevated temperature impairs onset of symbiosis and reduces survivorship in larvae of the Hawaiian coral, Fungia scutaria. Mar. Biol. 159, 633–642. doi: 10.1007/s00227-011-1842-0
Schnitzler, C. E., and Weis, V. M. (2010). Coral larvae exhibit few measurable transcriptional changes during the onset of coral-dinoflagellate endosymbiosis. Mar. Genomics 3, 107–116. doi: 10.1016/j.margen.2010.08.002
Schoenberg, D. A., and Trench, R. K. (1980). Genetic variation in Symbiodinium (= Gymnodinium) microadriaticum Freudenthal, and specificity in its symbiosis with marine invertebrates. I. Isoenzyme and soluble protein patterns of axenic cultures of Symbiodinium microadriaticum. Proc. R. Soc. B Biol. Sci. 207, 405–427. doi: 10.1098/rspb.1980.0031
Schwarz, A., Weis, M., and Potts, C. (2002). Feeding behavior and acquisition of zooxanthellae by planula larvae of the sea anemone Anthopleura elegantissima. Mar. Biol. 140, 471–478. doi: 10.1007/s00227-001-0736-y
Schwarz, J. A., Krupp, D. A., and Weis, V. M. (1999). Late larval development and onset of symbiosis in the scleractinian coral Fungia scutaria. Biol. Bull. 196, 70–79. doi: 10.2307/1543169
Shoguchi, E., Shinzato, C., Kawashima, T., Gyoja, F., Mungpakdee, S., Koyanagi, R., et al. (2013). Draft assembly of the symbiodinium minutum nuclear genome reveals dinoflagellate gene structure. Curr. Biol. 23, 1399–1408. doi: 10.1016/j.cub.2013.05.062
Slattery, M., Hines, G. A., Starmer, J., and Paul, V. J. (1999). Chemical signals in gametogenesis, spawning, and larval settlement and defense of the soft coral Sinularia polydactyla. Coral Reefs 18, 75–84. doi: 10.1007/s003380050158
Stat, M., Carter, D., and Hoegh-Guldberg, O. (2006). The evolutionary history of Symbiodinium and scleractinian hosts-symbiosis, diversity, and the effect of climate change. Perspect. Plant Ecol. Evol. Syst. 8, 23–43. doi: 10.1016/j.ppees.2006.04.001
Stat, M., Morris, E., and Gates, R. D. (2008). Functional diversity in coral-dinoflagellate symbiosis. Proc. Natl. Acad. Sci. U.S.A. 105, 9256–9261. doi: 10.1073/pnas.0801328105
Strathmann, R. R., Hughes, T. P., Kuris, A. M., Lindeman, K. C., Morgan, S. G., Pandolfi, J. M., et al. (2002). Evolution of local recruitment and its consequences for marine populations. Bull. Mar. Sci. 70, 377–396.
Thornhill, D. J., LaJeunesse, T. C., Kemp, D. W., Fitt, W. K., and Schmidt, G. W. (2006). Multi-year, seasonal genotypic surveys of coral-algal symbioses reveal prevalent stability or post-bleaching reversion. Mar. Biol. 148, 711–722. doi: 10.1007/s00227-005-0114-2
Thorson, G. (1950). Reproductive and larval ecology of marine bottom invertebrates. Biol. Rev. Camb. Philos. Soc. 25, 1–45. doi: 10.1111/j.1469-185X.1950.tb00585.x
Titlyanov, E. A., Titlyanova, T. V., Loya, Y., and Yamazato, K. (1998). Degradation and proliferation of zooxanthellae in planulae of the hermatypic coral Stylophora pistillata. Mar. Biol. 130, 471–477. doi: 10.1007/s002270050267
Trench, R. K. (1979). The cell biology of plant-animal symbiosis. Annu. Rev. Plant Physiol. 30, 485–531. doi: 10.1146/annurev.pp.30.060179.002413
Trench, R. K., Wethey, D. S., and Porter, J. W. (1981). Observations on the symbiosis with zooxanthellae among the Tridacnidae (Mollusca, Bivalvia). Biol. Bull. 161, 180–198. doi: 10.2307/1541117
Trench, R. K., and Winsor, H. (1987). Symbiosis with dinoflagellates in two pelagic flatworms, Amphiscolops sp. and Haplodiscus sp. Symbiosis 3, 1–22.
Venn, A. A., Loram, J. E., and Douglas, A. E. (2008). Photosynthetic symbioses in animals. J. Exp. Bot. 59, 1069–1080. doi: 10.1093/jxb/erm328
Voolstra, C. R., Schnetzer, J., Peshkin, L., Randall, C. J., Szmant, A. M., and Medina, M. (2009a). Effects of temperature on gene expression in embryos of the coral Montastraea faveolata. BMC Genomics 10:627. doi: 10.1186/1471-2164-10-627
Voolstra, C. R., Schwarz, J. A., Schnetzer, J., Sunagawa, S., Desalvo, M. K., Szmant, A. M., et al. (2009b). The host transcriptome remains unaltered during the establishment of coral-algal symbioses: FAST TRACK. Mol. Ecol. 18, 1823–1833. doi: 10.1111/j.1365-294X.2009.04167.x
Waldbusser, G. G., Brunner, E. L., Haley, B. A., Hales, B., Langdon, C. J., and Prahl, F. G. (2013). A developmental and energetic basis linking larval oyster shell formation to acidification sensitivity. Geophys. Res. Lett. 40, 2171–2176. doi: 10.1002/grl.50449
Wecker, P., Fournier, A., Bosserelle, P., Debitus, C., Lecellier, G., and Berteaux-Lecellier, V. (2015). Dinoflagellate diversity among nudibranchs and sponges from French Polynesia: insights into associations and transfer. C. R. Biol. 338, 278–283. doi: 10.1016/j.crvi.2015.01.005
Weis, V. M. (2008). Cellular mechanisms of Cnidarian bleaching: stress causes the collapse of symbiosis. J. Exp. Biol. 211, 3059–3066. doi: 10.1242/jeb.009597
Weis, V. M., Davy, S. K., Hoegh-Guldberg, O., Rodriguez-Lanetty, M., and Pringle, J. R. (2008). Cell biology in model systems as the key to understanding corals. Trends Ecol. Evol. 23, 369–376. doi: 10.1016/j.tree.2008.03.004
Weis, V. M., Reynolds, W. S., DeBoer, M. D., and Krupp, D. A. (2001). Host-symbiont specificity during onset of symbiosis between the dinoflagellates Symbiodinium spp. and planula larvae of the scleractinian coral Fungia scutaria. Coral Reefs 20, 301–308. doi: 10.1007/s003380100179
Wilkinson, D. M., and Sherratt, T. N. (2001). Horizontally acquired mutualisms, an unsolved problem in ecology? Oikos 92, 377–384. doi: 10.1034/j.1600-0706.2001.920222.x
Wolfowicz, I., Baumgarten, S., Voss, P. A., Hambleton, E. A., Voolstra, C. R., Hatta, M., et al. (2016). Aiptasia sp. larvae as a model to reveal mechanisms of symbiont selection in cnidarians. Sci. Rep. 6:srep32366. doi: 10.1038/srep32366
Wood-Charlson, E. M., Hollingsworth, L. L., Krupp, D. A., and Weis, V. M. (2006). Lectin/glycan interactions play a role in recognition in a coral/dinoflagellate symbiosis. Cell. Microbiol. 8, 1985–1993. doi: 10.1111/j.1462-5822.2006.00765.x
Wray, G. A., and Raff, R. A. (1991). The evolution of developmental strategy in marine invertebrates. Trends Ecol. Evol. 6, 45–50. doi: 10.1016/0169-5347(91)90121-D
Yakovleva, I. M., Baird, A. H., Yamamoto, H. H., Bhagooli, R., Nonaka, M., and Hidaka, M. (2009). Algal symbionts increase oxidative damage and death in coral larvae at high temperatures. Mar. Ecol. Prog. Ser. 378, 105–112. doi: 10.3354/meps07857
Keywords: symbiosis, zooxanthellae, coral reef, larval ecology, planula, veliger
Citation: Mies M, Sumida PYG, Rädecker N and Voolstra CR (2017) Marine Invertebrate Larvae Associated with Symbiodinium: A Mutualism from the Start? Front. Ecol. Evol. 5:56. doi: 10.3389/fevo.2017.00056
Received: 09 March 2017; Accepted: 15 May 2017;
Published: 30 May 2017.
Edited by:
Tilmann Harder, University of Bremen, GermanyReviewed by:
Michael Leonard Judge, Manhattan College, United StatesPunyasloke Bhadury, Indian Institute of Science Education and Research Kolkata, India
Copyright © 2017 Mies, Sumida, Rädecker and Voolstra. This is an open-access article distributed under the terms of the Creative Commons Attribution License (CC BY). The use, distribution or reproduction in other forums is permitted, provided the original author(s) or licensor are credited and that the original publication in this journal is cited, in accordance with accepted academic practice. No use, distribution or reproduction is permitted which does not comply with these terms.
*Correspondence: Miguel Mies, bWlndWVsLm1pZXNAdXNwLmJy