- Parasitology, Zoology, University of Otago, Otago, New Zealand
Scientific and public interest in host manipulation by parasites has surged over the past few decades, resulting in an exponential growth of cases where potential behavioral manipulation has been identified. However, these studies dwarf the number of genuine attempts to elucidate the mechanistic processes behind this behavioral manipulation. Ultimately, this imbalance has slowed progress in the study of the mechanisms underlying host manipulation. As it stands, research suggests that the mechanisms of host manipulation fall into three categories: immunological, genomic/proteomic and neuropharmacological, and forth potential category: symbioant-mediated manipulation. After exploration of the literature pertaining to these four pathways, four major trends become evident. First and foremost, there is a severe disconnect between the observed molecular and behavioral shifts in a parasitized host. Indeed, very rarely a study demonstrates that molecular changes observed in a host are the result of active manipulation by the resident parasite, or that these molecular changes directly result in behavioral manipulation that increases the parasite's fitness. Secondly, parasites may often employ multiple pathways in unison to achieve control over their hosts. Despite this, current scientific approaches usually focus on each manipulation pathway in isolation rather than integrating them. Thirdly, the relative amount of host-parasite systems yet to be investigated in terms of molecular manipulation is staggering. Finally, as a result of the aforementioned trends, guiding mechanisms or principles for the multiple types of behavioral manipulation are yet to be found. Researchers should look to identify the manipulative factors required to generate the molecular changes seen in hosts, while also considering the “multi-pronged” approach parasites are taking to manipulate behavior. Assessing gene expression and its products during transitional periods in parasites may be a key methodological approach for tackling these recent trends in the host manipulation literature.
Introduction
“I did not direct my life. I didn't design it. I never made decisions. Things always came up and made them for me. That's what life is.”
B. F. Skinner on behavior
The control parasites can demonstrate when realigning their host's goals with their own cannot be understated, with many parasitologists regarding hosts as an extended phenotype of the parasite (Adamo, 2013; Hughes, 2013; Poulin and Maure, 2015). Integrating this statement and the quote above, suggests Skinner (partially by semantic accident) was well ahead of his time in assessment of behavior and its origin. A common ideology among behaviorists, juxtaposed to Skinner, is that animals are solely in control of their own behavior (Sih et al., 2015), with the mind being viewed as the “last bastion of freedom.” However, considering parasites make up over 40% of the Earth's biodiversity, being prevalent in a vast taxonomic range of hosts from insects to humans (Poulin, 2010, 2011b; Thomas et al., 2010; Houte et al., 2013; Poulin and Maure, 2015) and their apparent control over host behavior, it is clear that this ideology has not anticipated the numerous parasite species that began raiding this supposed “last bastion” a long time ago.
The effects of host manipulation by parasites can range from a subtle change in pre-existing traits, such as Toxoplasma gondii's ability to encourage risk-taking behaviors in its hosts (Webster et al., 2013), to entirely new behaviors (Thomas et al., 2005; Poulin, 2011a; Adamo, 2013; Poulin and Maure, 2015). For example, ants infected with the trematode Dicrocoelium dendriticum will leave the safety of the colony to perch on grass blade tips, where their susceptibility to predation from the trematode's definitive host is greatly increased (Thomas et al., 2011). The list of induced behavioral changes that are potentially beneficial to the parasite's fitness is extensive, and while correct identification of these behavioral modifications is important, one of the most fascinating question we can ask is how the parasites are achieving this. More specifically, what are the true proximate triggers for host manipulation, and how, at a molecular level, are these behavioral manipulations achieved?
Currently, three major physiological pathways have been suggested by which parasites manipulate their host (Adamo, 2013). Firstly, some parasites manipulate their hosts by altering the communication between the immune and central nervous system (CNS) (Friberg et al., 2010; Hakimi and Cannella, 2011; Boucias and Pendland, 2012). Secondly, some may operate via proteomic (Helluy, 1983; Biron et al., 2005, 2006; Ponton et al., 2006; Hakimi and Cannella, 2011; Rahman and McFadden, 2011; McDonough and Rodriguez, 2012) and/or genomic mechanisms (Lüder et al., 2009; Hari Dass and Vyas, 2014; Sivakumar et al., 2014). Finally, parasites may secrete molecules that directly interact with the CNS to alter neuronal activity (Klein, 2003; Adamo, 2012, 2013; Lafferty and Shaw, 2013). Note, however, that parasites can interact with the hosts' brain on a structural level as well, destroying areas of the brain (Lafferty and Shaw, 2013; Libersat and Gal, 2013), but in the interest of remaining cohesive the major focus here will be on micro-level manipulation via molecular mechanisms. Symbiont-mediated behavioral manipulation could also be another potential major pathway for parasite behavioral manipulation, but research on this phenomenon is still in its infancy currently (Dheilly et al., 2015).
The first part of this review will attempt to collate the literature under each major and potential pathway. The critical analysis of the literature in the first part of this review will culminate in the second part, which will directly address several trends in the host manipulation literature. Ultimately, this is aimed to give the reader a comprehensive understanding of the strengths and weaknesses in the current host manipulation literature and suggest directions for future research. The review will primarily draw from three main sources: a special issue of the Journal of Experimental Biology concerning host manipulation, a literature compilation on helminth parasites by Poulin and Maure (2015) and the Web of Science search engine (search terms: parasite* AND behavior* AND mechanis* AND manipula* OR effect*, starting with the most relevant since 2017).
Immunological Host Manipulation
Immunological Manipulation of Vertebrates
Bidirectional connections between the immune and CNS are evolutionarily ancient, being present in both invertebrates and vertebrates (Ottaviani and Franceschi, 1996; Adamo, 2006, 2008; Dantzer et al., 2008). Upon infection by a parasite, the immune system releases cytokines as part of the neuroinflammation process, typically targeting the brain to result in “sickness behaviors” (Hart, 1988; Dantzer, 2004). These mostly depressive behaviors direct energy away from non-essential activities, such as reproduction or socializing, to enhance the chances of full recovery (Hart, 1988; Dantzer, 2004). Given some parasites can regulate these immune factors, in particular cytokines, to evade the host's immune system (Friberg et al., 2010; Hakimi and Cannella, 2011; Boucias and Pendland, 2012), the neuroimmune hypothesis suggests that transitioning from evasion to immunological manipulation of behavior would be parsimonious (Adamo, 2013). Essentially, under the neuroimmune hypothesis, the immune system can be seen as an impressionable middleman between the parasite and the brain, with evidence suggesting immunological manipulation might, in some cases be easier than neurological manipulation, given defense mechanisms such as the blood brain barrier (Dantzer et al., 2008; Nation, 2008).
A growing body of evidence in vertebrate hosts suggests that chronic neuroinflammation, induced by cerebral parasites, results in neural disruption and irregular behavior (Hemachudha et al., 2002; Klein, 2003; Bentivoglio and Kristensson, 2007; Sciutto et al., 2007; Hamilton et al., 2008). The infamous T. gondii, an intracellular parasite of neurons, is an exemplar in this case. T. gondii encysts into the brain of rats, resulting in an attraction to feline urine and hyper-aggression in infected individuals (Vyas et al., 2007; House et al., 2011), subsequently increasing the chance that T. gondii will be transferred to a feline definitive host (Vyas et al., 2007; House et al., 2011). T. gondii infection, during its active phase, results in the release of a cascade of cytokines including gamma interferons and proinflammatory mediators that are toxic to neurons (Henriquez et al., 2009). T. gondii's immunological manipulation also leads to microglia activation and through this nitric oxide (NO) release, which impacts on neurite outgrowth and is a known neuromodulator (Rozenfeld et al., 2003).
The gastrointestinal nematode Toxocara canis is another popular model species regarding vertebrate immunological manipulation. T. canis is highly prevalent in canines globally and can spread to humans via its widespread environmental contamination of feces (Holland and Smith, 2006). Studies show that T. canis can migrate to the brain in humans, and its presence here is more common than originally anticipated (Hill et al., 1985; Salvador et al., 2010). The neurological impacts of T. canis on humans are far from being fully understood, but initial evidence from experimental infection of mice show pro- and anti-inflammatory cytokine levels peak with increasing infection levels of T. canis in mice brains (Holland and Hamilton, 2013). However, no empirical proof correlates this cytokine change with a behavioral change in the host that is adaptive for T. canis.
The trypanosome Leishmania amazonensis is another parasite that can live in the brain of vertebrates (including humans) and has been associated with behavioral changes in its host (Petersen and Greenlee, 2011; Maia et al., 2015). Recently a study found that experimentally infected mice consistently had several key cytokines (IFN-y, IL-1,4,6,10) down regulated, while tumor necrosis factor alpha (TNF-α) was upregulated in the prefrontal cortex (Portes et al., 2016). Furthermore, this cytokine manipulation was correlated with increased anxiety in the hosts (Portes et al., 2016). Although this may appear as evidence for the neuroimmune hypothesis, the adaptive value for the parasite of increased host anxiety seems dubious relative to the predator-philic behavior induced by Toxoplasma in rats (Vyas et al., 2007; House et al., 2011). Therefore, it is more parsimonious to suggest the anxiety response may be a pathological side effect, induced by the parasite, or a sickness behavior evoked by the host, rather than adaptive behavioral manipulation. Causative empirical evidence is needed to conclude either way.
Cytokine-dependent manipulations similar to those reported in Toxoplasma, Toxocara, and Leishmania infected individuals are seen in other host-parasite systems (Flegr et al., 1996; Webster, 2007; Kannan et al., 2010). Adaptive value of behavioral manipulation aside, none of these studies have empirically connected the reported cytokine manipulation to an adaptive behavioral change in the host, nor have they identified any specific factors produced by the parasite that affect cytokine expression. In lay terms, we know infection results in cytokine expression changes in the host, but not what specific aspect of this infection causes the resulting cytokine level changes. Further studies are needed to confirm the specific identity and function of factors that regulate these cytokines. The present lack of understanding of how these molecular changes come about in the host as a result of parasite infection is a universal problem in host manipulation literature is detrimental to the field. Given this missing information, parasitic manipulation of cytokines is still more likely to be used to solely evade immunological defenses of the host rather than to manipulate behavior as well in vertebrates.
A core argument for immunological manipulation by parasites is that it enables them to bypass brain defense systems (Dantzer et al., 2008; Nation, 2008). However, this idea appears conflicting, as much of the evidence for the neuroimmune hypothesis in vertebrates, comes from cerebral parasites (Hemachudha et al., 2002; Klein, 2003; Bentivoglio and Kristensson, 2007; Sciutto et al., 2007; Hamilton et al., 2008; Henriquez et al., 2009; Petersen and Greenlee, 2011; Holland and Hamilton, 2013; Portes et al., 2016). Logic suggests that a parasite already present in the brain of a host will not have to by-pass the brains defenses in order to manipulate host behavior. Again, this questions whether cytokine manipulation is primarily a general response of the host or something specifically directed by the parasite to manipulate behavior. Overall the neuroimmune hypothesis remains theoretical at best in vertebrates.
Immunological Manipulation of Invertebrates
In simpler invertebrate hosts, the behavioral changes as a result from cytokine manipulation appear to align more directly with that of the parasites goals, and the connection between the immunological mechanism and the resulting behavioral change appears to be more direct (Helluy and Thomas, 2010; Helluy, 2013). For example, the parasitic wasp Cotesia congregata exploits immune-neural connections of its host, Manduca sexta (caterpillar), suppressing feeding and locomotion during the larval egression phase (Adamo, 1997, 2005). Considering the caterpillars' voracious appetite this behavioral shift is significant. The wasp larvae disrupt the removal of octopamine (OA), a neuromodulator tightly linked with immune response to infection (Dunphy and Downer, 1994), in the hemolymph of the caterpillar. Subsequently, this results in desynchronization between parts of the caterpillar's brain, rendering it unable to swallow (Miles and Booker, 2000; Adamo, 2005). The larvae may also boost the production of a cytokine that acts as a paralytic peptide, which immobilizes the caterpillar (Skinner et al., 1991; Adamo, 2013). This reduction in digestion and locomotion in the caterpillar provides the wasp larvae with a static environment, which greatly increases the chance of successful egression from the caterpillar (Adamo, 1998).
Interestingly, this hypothetical increase in production of the paralytic peptide may not be the only factor contributing to loss of locomotive control in the wasp-caterpillar system. Adult wasps are also known to inject venom into the CNS of insects, resulting in the loss of self-driven movement, ultimately enabling the wasp to continue its reproductive cycle (Libersat et al., 2009). Currently, empirical evidence from the jewel wasp-cockroach host-parasite system suggests a component of this venom alters the activity of neuron populations involved in regulating OA, and thus locomotive ability (Rosenberg et al., 2006). If we contrast this to the larval wasp disrupting OA breakdown in the caterpillar, it may suggest altering OA does not have merely digestive, but also locomotor effects similar to that of adult wasp venom on the cockroach.
Continuing this line of thinking, the source of this OA disruption in the wasp larvae-caterpillar system and thus digestive and locomotor paralysis may stem from a potential larval form of the adult venom being pumped into the host's circulation during the ejection phase rather than paralytic cytokine modulation. The paralytic cytokines in the larval wasp-caterpillar system are thought to heal wounds via physiological (Yu et al., 1999) and behavioral changes (Skinner et al., 1991). Therefore, the hypothesized elevated presence of the paralytic cytokines during larval ejection may just be a pathological reaction to the damage caused by the larva egressing from the host. As of yet there is no empirical evidence to suggest these cytokines are elevated during this phase, or that the larvae adaptively manipulate them to begin with. Utilizing the wasp venom's properties for host locomotor control during larval and adult life stages seems more parsimonious than developing two different mechanisms for the same paralytic function.
Given the lack of mechanistic understanding of how both the OA manipulation and the hypothesized cytokine production boost occur during the wasp-caterpillar interaction, it is difficult to empirically conclude whether these effects are immunological reactions of the host to infection, or adaptive manipulations by the parasite. Logically, the chance of an immunological reactionary response (i.e., increase in paralytic cytokine levels) from the caterpillar aligning almost perfectly with that of the wasp's reproductive strategy would be extremely low. Adaptive manipulation of OA by the wasp larvae venom seems more parsimonious in this case given the venom's effects in other host-wasp systems. Further mechanistic studies looking at the fundamental source of these OA and potential cytokine changes in the wasp-caterpillar system are needed to build a more complete picture and empirically confirm adaptive manipulation. Also, looking at the role of potential OA manipulation in other parasite-host systems that involve significant control of movement, such as the hairworm-cricket/grasshopper systems, could establish OA as a key gateway molecule for parasitic control of movement.
A further example of invertebrate immunological manipulation comes from the schistosome parasite Trichobilharzia ocellata, which infects the snail Lymnaea stagnalis. The parasite secretes compounds that subvert the snail immune system, allowing it to persist within the snail (de Jong-Brink et al., 1997, 2001). Interestingly, the knock-on effects of this immune manipulation result in decreased investment in reproduction by the snail. The restructuring in the allocation of energy may favor the parasite's growth over the host's own fitness. Specifically, the parasite releases an antigonadotropic cytokine called schistosomin. To date, the neurophysiological effects of schistosomin are among the best understood relative to other compounds involved in host manipulation. Once schistosomin binds to neuroendocrine cells it potentially disrupts the binding of gonadotropic hormones to receptors, and/or a component of the signal transduction system, resulting in a reduction in the release of egg-laying hormone. Schistosomin may also enhance adenylate cyclase activity in neurons, resulting in changes in innervation of the male copulatory system. Considering the snail is hermaphroditic, the fact that schistosomin can potentially influence both female and male reproductive systems suggests a very close evolutionary relationship between the worm and the snail.
The manipulation of sensorimotor processes of gammarids (Amphipoda) by acanthocephala is another major area of research regarding invertebrate host manipulation. When infected gammarids are disturbed they display photophilic behaviors, actively swimming toward the surface and clinging to debris. The opposite behavior is exhibited in their uninfected counterparts, which swim down into the benthic layer (Bethel and Holmes, 1973, 1974; Rauque et al., 2011). This behavioral manipulation of infected gammarid's makes them more susceptible to avian predators, increasing trophic transmission opportunities for the parasite (Bethel and Holmes, 1977). Experimental injections of serotonin (5-HT) in uninfected gammarids provoked identical photophilic and clinging behaviors as seen in infected gammarids (Helluy and Holmes, 1990). This result has been replicated in other acanthocephala-gammarid host parasite systems as well (Tain et al., 2006).
Collectively these studies indicate that 5-HT plays a major role in modulating sensorimotor pathways in gammarids. However, given the high concentrations of 5-HT required to elicit the photophilic behaviors, it is doubtful the parasites (based on energetic costs) release that amount of 5-HT directly into the surrounding host tissues (Thomas et al., 2005; Adamo, 2012). Instead, convergent evidence suggests the involvement of immune defense systems upstream of the 5-HT-induced sensorimotor changes (Helluy, 2013). Upon infection, the prophenoloxidase (proPO) cascade is activated in gammarids leading to the production of melanin and the active enzyme PO (Cerenius and Söderhäll, 2004; Nappi and Christensen, 2005). The melanin is used to encapsulate parasites resulting in their death (Nappi and Christensen, 2005). In acanthocephalan-gammarid associations we see a decrease in PO followed by a surge in 5-HT levels (Lefevre et al., 2009). This could indicate that subversion of the host immune system results in the 5-HT surge necessary to trigger sensorimotor manipulation (Helluy, 2013). However, this is likely to be only a small part of the picture. Many other host defense mechanisms such as cytokine or toll signaling pathways are yet to be investigated with regards to gammarids.
Tumor Necrosis Factor Family: A Key Player in Immunological Manipulation?
Research indicates that the TNF family of cytokines may be a fruitful avenue of research when it comes to immunological manipulation in host-parasite systems. Several studies, spanning multiple invertebrates, have described TNF as a key pro-inflammatory cytokine associated with response to parasitic infection (Mekata et al., 2010; Vidal, 2010; Wang et al., 2012). Eiger, a member of the TNF family, is a key component of the neuroinflammatory response in vertebrates and has been implicated in the process of melanisation (Fearon and Locksley, 1996; Igaki et al., 2002; Mabery and Schneider, 2010). A study by Qin et al. (2007) also found that TNF-α, generated by parasitic immune activation, can cross the blood brain barrier and kill specific neuronal populations in mice. Considering that L. amazonensis upregulates TNF-α in mice (as previously discussed), this suggests TNF-α regulation and the resulting neuronal degeneration in the pre-frontal cortex as a potential key aspect of immunological manipulation. Holistically, this apparent overlap in the importance of TNF (and cytokines in general) across taxa suggests that promising future research will be inspired by the similarity of neuroimmune systems between invertebrates and vertebrates, and in the convergence of host manipulation mechanisms across distantly related phyla.
Proteomic and Genomic Host Manipulation
Proteomic Manipulation
Compared to immunological host manipulation and its proposed “middle man” approach, directly altering gene and protein expression in the host CNS appears to be a very blunt and direct mechanism. One of the most prominent cases comes from a line of research conducted on hairworms (Nematomorpha). These endoparasites infect a wide range of arthropod hosts (Poulin, 2010, 2011a; Thomas et al., 2010), inducing intense hydrophilic behaviors in their otherwise hydrophobic hosts (Thomas et al., 2002; Poulin, 2011a). A dramatic case of this induced behavioral switch, comes from the cricket Nemobius sylvestris. When infected with the hairworm Paragordius tricuspidatus, and once the parasite has achieved reproductive maturity, the cricket essentially commits suicide in large bodies of water (Biron et al., 2006). Once there, the hairworm emerges from the cricket and reproduces in this aquatic environment, thus increasing its chances of transmission to further hosts (Biron et al., 2006).
Given the extreme behavioral change induced by Nematomorph infection, and the apparent convergence of the manipulated phenotype across arthropod hosts it is perhaps unsurprising that the potential causative mechanisms have been investigated. Research has focused on two separate host-parasite relationships (the cricket mentioned above, and a grasshopper species infected with a similar hairworm) in which the hosts exhibit the same hydrophilic behavioral tendencies when infected (Biron et al., 2005, 2006). Using 2D gel electrophoresis and mass spectrometry, with successive characterization of both the host and parasite proteomes, investigators have found differential protein expression linked to neurotransmitter activities in the CNS of both arthropods at different time stages of infection (Biron et al., 2005, 2006). This provides a strong case for the host proteome being manipulated during infection in these two host-parasite systems. Interestingly, evidence suggests that the hairworm induces apoptosis in the grasshopper but inhibits this process in the cricket (Biron and Loxdale, 2013). Both of these tactics have the potential to disrupt the host's CNS function (Klein, 2003; James and Green, 2004). However, the connection between infection and the molecular /behavioral change in the host still remains unclear, with no concrete evidence yet to suggest a connection between the two.
Revisiting the gammarid parasite system; it is noted, that two studies also investigated the effects of trematode infection on host proteome expression. The two gammarid species investigated were Gammarus insensibilis and Gammarus pulex. It was found that 13 and 8% of the gammarid proteome, respectively, was differentially expressed in the presence of the hairworm parasite (Ponton et al., 2006). Many of the proteins manipulated by the trematode were implicated in playing crucial roles in 5-HT production (Helluy, 1983; Ponton et al., 2006). Furthermore, results of these proteomic studies indicated that arginine kinase is differentially expressed in infected over uninfected gammarid brains (Ponton et al., 2006). Arginine kinase is a regulator of nitric oxide production (Mori and Gotoh, 2000). As discussed, nitric oxide can also be regulated by the immune system and is a known neuromodulator (Rozenfeld et al., 2003). This corroborates results of previous studies concerning possible immunological manipulation and the resulting change in CNS 5-HT levels, suggesting parasites are using multiple manipulative avenues to gain control over host behavior.
Similar levels of proteomic manipulation, were also found in Artemia-cestode associations. Here, according to the researchers, the parasite demonstrated adept control over its host's behavior, in order to increase its chances of trophic transmission (Amat et al., 1991; Sánchez et al., 2006, 2007). Specifically, two peptides were found to be consistently down-regulated in a single Artemia species experimentally infected with 3 different species of cestode (Sánchez et al., 2009). Since the same behavioral manipulation was seen across all experimental infections, these two peptides are strong potential candidates for involvement in the manipulative process. However, as addressed before with regard to immunological manipulation, multiple gaps in our understanding of the full process of host manipulation hinder attempts to make scientifically robust conclusions as to whether these peptides actually contribute to the behavioral manipulation.
Genomic Manipulation
The field of epigenetics has been receiving increasing interest in the field of host-parasite systems. Epigenetic mechanisms alter gene expression without altering the DNA code itself and such changes can be inherited (Poulin and Thomas, 2008; Gómez-Díaz et al., 2012). As such, epigenetic changes induced by a parasite can potentially alter the hosts behavior as well as the offspring of the host. Conceptually, the behavioral changes induced in the host's offspring would enable an increased probability of transmission for the parasites own progeny (Poulin and Thomas, 2008). Most of this research has focused on intracellular protozoan parasites, specifically, Theileria, Toxoplasma, and Plasmodium (Lüder et al., 2009; Hari Dass and Vyas, 2014; Sivakumar et al., 2014; Cheeseman and Weitzman, 2015). The epigenetic programming by the parasite falls into 3 categories: the epigenator, the epigenetic initiator and the epigenetic maintainer (Berger et al., 2009). In order to establish stably heritable states of genetic suppression, the pathogen must possess mechanisms that progress logically from the epigenator to the maintainer to have a lasting structural influence on host's DNA (Berger et al., 2009; Cheeseman and Weitzman, 2015).
Recently, extensive studies indicate that several intracellular parasite species meet these criteria, implying that in these cases, infection can have a direct impact on gene expression (Lüder et al., 2009; Hari Dass and Vyas, 2014; Sivakumar et al., 2014; Cheeseman and Weitzman, 2015). This in turn has a variety of adaptive consequences for the parasite. For example,McMaster et al. (2016) concluded that hosts infected with Leishmania (previously implicated in immunological manipulation) systematically had the activity of their macrophages down regulated as a result of the pathogen's epigenetic mechanisms. However, the hypothesis of trans-generational behavioral effects from genomic modulation is yet to be empirically supported in intracellular parasites (Poulin and Thomas, 2008; Gómez-Díaz et al., 2012).
Perhaps the strongest evidence to date, for epigenetic modulation affecting host behavior comes from the increased release of testicular testosterone in T. gondii infected male rats (Lim et al., 2013; Vyas, 2013). Testosterone crosses the blood brain barrier in the infected host to have a wide variety of effects including hypomethylation of the arginine vasopressin gene within the posterodorsal medial amygdala resulting in elevated vasopressin expression that may alter behavior of male rats (Auger et al., 2011; Hari Dass and Vyas, 2014; Abdulai-Saiku and Vyas, 2017). Interestingly, testosterone has been implicated in acanthocephalan-gammarid host manipulation and other host-parasite systems as well (Klein, 2004; Lewis et al., 2016). This suggests that the epigenetic mechanism found in Toxoplasma may be present at a similar level in these other systems. However, failure to replicate the behavioral effects of testosterone in female hosts implies this mechanism of host manipulation struggles to account for the sexually dimorphic effects of testosterone (Lewis et al., 2016; Abdulai-Saiku and Vyas, 2017).
Evidence that macro-parasites, such as nematodes and cestodes, using epigenetic mechanisms to control their hosts, is currently limited, although given the extensive proteomic impact these parasites can have on their hosts, the most parsimonious explanation may be genomic manipulation. Indeed, two recent studies suggest that extracellular parasites can have wide reaching impact on their host's genome (Feldmeyer et al., 2016; Geffre et al., 2017), suggesting gene regulation is a key factor in host manipulation. While in both cases compelling evidence is provided for potential gene-induced behavioral manipulation, the researchers could only establish correlation and not causation for the effect of gene regulation on host behavior. Again, this emphasizes the need to empirically connect infection with molecular and behavioral changes in the host.
Holistically, the apparent connection between gene expression and behavior is becoming clearer as the field of behavioral genetics develops. For example, the foraging (for) gene encodes protein kinase G (PKG), which is potentially involved in the phosphorylation of multiple neuropeptides and hormones (Fitzpatrick and Sokolowski, 2004). Modulation of PKG levels has been consistently linked with regulation of locomotor activity across multiple insect species (Fitzpatrick and Sokolowski, 2004; Houte et al., 2013). Given that multiple other “locomotor” gene targets exist as well as the for gene in insects (Houte et al., 2013), and the commonality of locomotor behavioral manipulation in host-parasite systems, gene manipulation could be a realistic approach to manipulating locomotion.
However, two baculovirus species that alter the locomotive activity of their hosts, appear to bypass gene regulation in favor of releasing protein tyrosine phosphatase (PTP), a dephosphorylation enzyme, into the host (Kamita et al., 2005; van Houte et al., 2012). Consequently, the PTP is thought to dephosphorylate PKG. This alters the activity of PKG, and thus phosphorylation of its downstream neuropeptides/hormones, resulting in alteration of behavior. As a result, PTP has been put forward as a potential key protein involved in behavioral manipulation (Houte et al., 2013). A large amount of work will need to be done to validate this suggestion however. Aside from the various mechanistic studies required to validate the multiple steps in this theoretical framework, a key assumption must be verified here. Evidence for this hypothesis is based on the correlation between the expression level of the PTP gene in the parasite and the presence of the behavioral modification. The assumption here is that PTP level in the host correlates with parasite PTP gene expression. Given PTP is a crucial enzyme for physiological processes (Mustelin, 2007), it is possible PTP knockouts in the parasite may adversely affect its functioning. Therefore, abnormal functioning might be the reason for loss of the behavioral manipulation ability, rather than no PTP being released into the host. Essentially, correlating parasite gene expression with host behavioral manipulation can be very informative, but the product of that gene expression must be quantified in the host also. On the whole, the PTP-PKG system is a logical and elegant solution to understanding locomotor (and potentially other) behavioral manipulation, but it needs empirical evidence that confirms what happens to the PTP once it is expressed.
Integrating Immune and Proteomic/Genomic Pathways
Some studies indicate that blocking or overexpressing genes may be tightly linked to immunological manipulation (Hakimi and Cannella, 2011; Rahman and McFadden, 2011; McDonough and Rodriguez, 2012). It is common for intracellular pathogenic species to release compounds directly into their own host cell and the surrounding cells to regulate gene expression (McDonough and Rodriguez, 2012). Through the modulation of critical second messenger pathways within cells, such as cAMP, changes in gene and protein expression occur in the host that can manipulate the host's immune system (i.e., cytokine production) (Hakimi and Cannella, 2011; Rahman and McFadden, 2011; McDonough and Rodriguez, 2012). A classic example of this comes from T. gondii, which secretes protein kinase IKK into host cells (Hakimi and Cannella, 2011), resulting in increased phosphorylation of IκB-α, triggering the activation of the transcription factor NF-κB (Rahman and McFadden, 2011). This transcription factor plays a known role in immunological gene and protein expression as well as neural signaling (Meffert et al., 2003; Gilmore, 2006). Integration of genomic and immunological pathways to deter immunological defenses and potentially manipulate their host's behavior, is a classic example of the “multi-pronged” approach parasites may take when manipulating host behavior (Adamo, 2013).
Neuropharmacological Host Manipulation
Monoamines and Hormones
Neuropharmacology is the study of a drug's action on the nervous system. Mounting evidence suggests that parasites can secrete neuromodulators (hormones or neurotransmitters), that impact upon the functioning of the host's CNS (Klein, 2003; Adamo, 2012, 2013; Lafferty and Shaw, 2013). This can potentially result in the manipulation or creation of behaviors that assist in parasite transmission into suitable environments or a definitive host (Moore, 2002). The production and application of substances that alter CNS functioning is not restricted to a parasite's manipulative effort. Indeed, a host's own body does this automatically to enable neuronal plasticity, and thus adapt its behavior to unpredictable environments (Huber, 2005). Given enough evolutionary time, parasites could have adapted to exploit receptor-binding sites that enable plasticity, thus giving them a window into behavioral manipulation of their hosts.
Neuromodulators are capable of achieving long-term physiological effects (Lafferty and Shaw, 2013). In particular, parasites commonly use the potent monoamine neurotransmitters dopamine (DA), serotonin and octopamine with conservation in structure and function across the vast range of host taxa that parasites manipulate (Pflüger and Stevenson, 2005; Shaw et al., 2009; Helluy, 2013; Libersat and Gal, 2013; McConkey et al., 2013; Vyas, 2013; Webster et al., 2013). These monoamines are also used by hosts to regulate a wide variety of brain functions from stress to reproduction (Libersat and Pflueger, 2004; Nelson and Trainor, 2007; Øverli et al., 2007). As discussed earlier, hormones such as testosterone are also thought to play a key role in neuropharmological manipulation (Auger et al., 2011; Hari Dass and Vyas, 2014; Abdulai-Saiku and Vyas, 2017).
Challenges in Assessing Neurochemical Behavioral Manipulation
In some host-parasite systems it remains unclear whether parasites alter CNS function via the direct secretion of neuromodulators, or if they subtly secrete a factor that manipulates the host into producing more neuromodulator indirectly. Consider that the average disparity between host and parasite body sizes is quite large (Poulin and Maure, 2015) and neuromodulator concentrations need to be high in the host to generate the behavioral shifts seen in experimental injection studies (Helluy and Holmes, 1990). Given this, it would appear the indirect option should be the most parsimonious in most host-parasite systems, as it would be too energetically costly for the parasites to produce the required levels of neuromodulator directly. Increasingly this seems to be the case in host parasite systems (Thomas et al., 2005; Adamo, 2012; Houte et al., 2013; Libersat and Gal, 2014). For example, looking back to the jewel wasp-cockroach parasite-host system, evidence suggests OA is regulated through indirect mechanisms (Rosenberg et al., 2006). This is surprising considering, as a host-parasite system, it would be one of the most likely to directly manipulate neuromodulator levels in the host, given the relatively similar body sizes between the host and parasite, and the precise control over where the wasp can target its manipulation (Libersat and Gal, 2014).
The potential for direct alteration of monoamines in the jewel wasp-cockroach system may not be completely squandered however. If we look at the induction of compulsive grooming in the cockroach after injection of wasp venom into the CNS, evidence suggests a dopamine-like substance in the venom triggers the grooming behavior (Weisel-Eichler et al., 1999). Indeed, experimentally injecting dopamine into the hemolymph of an unstung cockroach triggers a similar behavior (Weisel-Eichler and Libersat, 2002). However, incorporating this evidence into the argument for indirect over direct monoamine manipulation mechanisms is complicated for multiple reasons. In particular, no study quantifies how much of this dopamine-like substance is delivered on average per sting. Therefore, the volume delivered could range from an extremely low to a relatively high amount. Given the small disparity in body size between the cockroach and wasp relative to most other host-parasite systems, it is entirely possible a relatively large quantity of dopamine could be delivered to the cockroach CNS, with comparatively little energy expenditure from the parasite.
Conversely, a low amount of dopamine could be just as effective, given the wasp consistently targets the sub and supra-esophageal ganglion of the cockroach. If this is indeed the target site for its manipulation of behavior, consider the reduction in distance and thus dilution of the dopamine if the wasp releases it very close to its targeted manipulation site, rather than non-specifically adding it into circulation further away. Applying this rationale to other host-parasite systems provides a potential counter argument to the indirect over direct method of monoamine manipulation. However, when we look at the placement of cerebral parasites such as T. gondii in the brain, it appears to be random, with no placement in a specific area (McConkey et al., 2013). This suggests either being located in the CNS renders the release of low amounts of monoamines sufficient to produce behavioral modification, or indirect mechanisms such as influencing neuronal populations is the more adaptive answer.
On top of all this, the cockroach's compulsive grooming behavior may be a pathological side effect of infection, rather than an adaptive behavioral manipulation. The best hypothesis posits that the grooming keeps the cockroach in place while the latter paralytic phase of the venom kicks in allowing the wasp to easily relocate the cockroach upon its return visit (Libersat and Gal, 2014). However, it is probable that the grooming may be a side effect of the component in the venom, which alters the activity of dopamine neurons as well as its target OA neurons in the CNS. The dopamine-like substance present in the venom could be an artifact of evolution (vestigial), a by-product of venom production or have an adaptive function in the first sting the wasp uses to initially paralyze the legs of the cockroach, but no adaptive function in the second sting. Given we only know it is present in the venom but not its concentration, the first two alternative suggestions cannot be ruled out. Also, it is possible that the locomotor effects of the venom, i.e., paralysis of the legs, and loss of ability to initiate movement, may overlap with the compulsive grooming behavior. Therefore, the grooming behavior may not be necessary to keep the cockroach in place, since the other two effects of the venom may keep the cockroach sedentary regardless. Ultimately, it is clear the jewel wasp-cockroach system is difficult to place in the indirect vs. direct monoamine manipulation debate. In truth, it may suggest that in some systems, parasites are often capable of using both direct and indirect mechanisms simultaneously to adaptively alter monoamine levels in their host.
A further conundrum faced by parasitologists investigating neuropharmological mechanisms is that neuromodulator effects applied experimentally to the host, in absence of the parasite, are often very different to the behavioral changes evoked by the parasite itself (Adamo, 2013). This has been encountered across many studies that experimentally replicate the neuromodulator change associated with behavioral manipulation in the absence of a parasite. For example, the cysts formed by T. gondii have been shown to secrete tyrosine hydroxylase, a rate-limiting enzyme in the synthesis of dopamine (Cooper et al., 2003; Gonzalez et al., 2007; Prandovszky et al., 2011). In vitro, neurons containing cysts were found to secrete more DA than neurons without (Prandovszky et al., 2011). On a superficial level this implies that up-regulation of DA may result in increased attraction of rodents to the urine of felines. However, increasing dopamine release via the drug L-DOPA in non-infected rodents results in the opposite behavior to those of infected rodents (Cooper et al., 2003; Jaunarajs et al., 2011). As mentioned previously, Toxoplasma is involved in immunological manipulation (Rozenfeld et al., 2003; Henriquez et al., 2009) and studies show that Toxoplasma has influence over testicular testosterone secretion as well (Vyas, 2013). This indicates that multiple mechanisms may contribute toward behavioral manipulation in Toxoplasma (Webster et al., 2013).
The final case of behavioral manipulation presented here is very interesting. It has no potential direct impact on neuronal functioning, and therefore would be best described as pharmacological manipulation. Furthermore, it does not use monoamines or hormones. Mosquitoes are known to be attracted to humans infected with malaria-inducing Plasmodium spp. parasites. This facilitates the Plasmodium spp. transmission to the mosquito, its definitive host (Cornet et al., 2013; Batista et al., 2014). Until very recently the mechanism for this behavioral manipulation was unknown. Plasmodium falciparum releases an isoprenoid precursor into the red blood cells of its human host, resulting in an increased release of carbon dioxide, monoterpenes and aldehydes. Based on experimental evidence, this combination of molecules is hypothesized to attract and subsequently affect infection susceptibility via gene transcription modulation of mosquito hosts (Emami et al., 2017).
Symbiont-Mediated Manipulation
Viruses as Vectors for Behavioral Manipulation
Symbiont-mediated manipulation of host behavior involves a close relationship between two parasitic species that may directly result in behavioral manipulation of their target host. The only scenario that has empirical support for symbiont-mediated manipulation is the relationship between a macro-parasite and the viruses that use it as a vector. Essentially, the macro-parasite harnesses the virus' manipulative abilities, while the virus uses the macro-parasites as delivery device (Dheilly et al., 2015). Let us look at the ladybeetle-wasp host-parasite system: the best and (currently) only empirically confirmed example of symbiont-mediated manipulation. The wasp injects its eggs into the ladybeetle. After roughly 20 days a single larva egresses from the host and spins a cocoon on the ventral surface of the beetle. After a week, an adult wasp emerges from the cocoon, and the life cycle repeats. During this period, the beetle is completely immobilized, serving as protective structure for the cocoon. The virus (DcPV) may be responsible for the paralysis, as evidence suggests it causes lysis of glia cells and thus neuropathy of the cerebral ganglion. Crucially, the wasp injects DcPV with the eggs into the beetle (Dheilly et al., 2015). Here we can see the mutualistic relationship the wasp and DcPV share. The wasp gets an adaptive behavioral manipulation while the virus gets a vector.
Viral transfer during contact of parasites and hosts is a very common occurrence across a wide range of host-parasite systems (Lipsitch et al., 1996). Also, examples of viruses helping parasites persist in their host are now surfacing along with the recent conceptualization of the holobiome (Ives et al., 2011; Fichorova et al., 2012; Dheilly, 2014). Therefore, there is a distinct possibility that symbiont-mediated manipulation is not just restricted to the wasp-ladybeetle system. In fact, it may provide an elegant answer to a troubling phenomenon: the suppression of the Varroa sensitive hygiene (VSH) behavior in honeybees. Bees displaying VSH behavior remove Varroa-mite infected bee pupae from the hive, however very few bees utilize this behavior. Given the adaptive value of this behavior, why only some bees display VSH is puzzling (Harbo and Harris, 2009). However, considering that Varroa transfers multiple viruses to the bee while it feeds on the hemolymph (Kevan et al., 2006), symbiont-mediated manipulation (similar to the wasp-ladybeetle system) resulting in the suppression of VSH behavior may be a potential answer here. Considering the critical importance of bees to society, and Varroa's role in colony collapse disorder (Martin, 2001), this possibility may warrant further investigation.
Symbiogenesis: A Potential Evolutionary Outcome of Symbiont-Mediated Manipulation
Given the mutualistic relationship between DcPV and the wasp, we can draw tentative parallels with the symbiogenesis theory: that mitochondria and chloroplasts (and potentially other organelles) were once free-living prokaryotic organisms that became assimilated by eukaryotes (Sapp et al., 2002). Ultimately, this comparison between symbiont-mediated manipulation and the symbiogenesis theory suggests that over evolutionary time, a virus using a parasite as a vector may start to lose its pre-symbiosis traits until eventually it becomes an element only capable of behavioral manipulation and self-replication. Consider that mitochondria were once free-living bacteria, and over a vast amount of evolutionary time they were whittled down to two basic functions: producing ATP and self-replication. Given this potential parallel between DcPV and the mitochondria (and other organelles) and the recent advances in the holobiome theory, is it not reasonable to consider symbiogenesis as a potential evolutionary outcome of the DcPV-wasp system? Furthermore, may single or multiple (semi) complete processes of symbiogenesis have occurred before in systems similar to the ladybug-wasp system? This could imply that assimilated, “stripped back” viruses may be a potential element in initiating behavioral manipulation in other host-parasite systems.
Trends in Host Manipulation Research
The Imbalance between Identification and Mechanistic Studies
The literature on host manipulation shows a strong preference toward identification of behavioral manipulation and/or its ecological significance in various host-parasite systems, over elucidation of the mechanisms behind this manipulation (hereafter, these are referred to as “identification” and “mechanistic” studies). For example, of the 221 empirical studies focusing on trophically-transmitted helminth (worm) parasites from 1973 to 2014 (dataset from Poulin and Maure's, 2015 review), only 20 of the studies were classed as mechanistic, while the rest were identification studies. Additionally, this disparity between mechanistic and identification studies appears to be widening with time. The amount of identification studies on helminth parasites appears to roughly follow an exponential trend, while mechanistic studies appear to be following a weakly increasing linear trend (Figure 1). If helminth studies can be used as a marker for host manipulation research in general, then these trends suggest identification studies will completely overshadow mechanistic studies in the future.
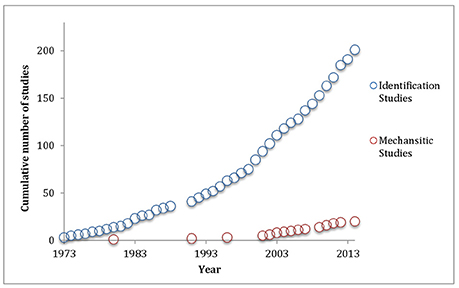
Figure 1. Temporal trend in the cumulative number of studies that identify parasite-host manipulation (n = 201) and those that attempt to elucidate the mechanism behind said manipulation (n = 20) in helminth studies from 1973 to 2014 (data from Poulin and Maure, 2015).
The large and widening gap between the number of identification and mechanistic studies may explain the current difficulty to claim adaptive behavioral manipulation in host parasite systems. As without empirical causative evidence, which identification studies cannot provide, the chance that behavioral manipulation may be a pathological side effect of infection rather than adaptive cannot be ignored. Mechanistic studies can nullify the possibility of pathological side effects, because proving these behavioral changes are the direct result of molecular manipulation implies causation, not correlation. Therefore, this difficulty to claim adaptive behavioral manipulation may stem from a lack of mechanistic studies providing causative evidence. Adding another perspective to this argument, it is thought that to confirm adaptive manipulation, evidence that the parasite expends energy during behavioral manipulation is required (Perrot-Minnot and Cézilly, 2013). Methods that correlate energy expenditure of the parasite during potential adaptive behavioral changes in the host may provide a reliable indicator of active manipulation by the parasite.
Specializing in a Sea of Host-Parasite Systems
Identification studies in helminth systems have indicated potential behavioral manipulation in 135 host-parasite species combinations, while mechanistic studies have only looked at the mechanism behind 17 of these combinations (extracted from Poulin and Maure's, 2015 review). Also, there is an estimated 300,000 uninvestigated helminth parasite species infecting vertebrates alone (Dobson et al., 2008). Furthermore, it is apparent that the majority of mechanistic studies are focused on a few host-parasite systems that usually have immediate consequences for humans. Collectively, this implies that scientists are only looking at the tip of an enormous iceberg of host-parasite interactions. Furthermore, when they do look, it's primarily to identify rather than mechanistically understand behavioral manipulation in anthropocentric or readily observable host-parasite systems. Considering this, the fact that principles or guiding mechanisms for the different types of host manipulation have not yet been uncovered yet is reasonable, given so many host-parasite systems remain uninvestigated.
This lack of foundational direction in the host manipulation literature is evident in host-parasite systems that have absorbed most of the research effort. For example, Toxoplasma-host systems are one of the most studied in the field of parasitology, and while considerable progress has been made on this model system, it still faces the same struggles as lesser-studied systems. Specifically, there is a need to integrate the multiple identified mechanisms and to better connect the evidence for the reported molecular changes to the process of behavioral manipulation. Undoubtedly, Toxoplasma is the poster child of host manipulation, and has consequently drawn a lot of funding and attention for the field. While host-Toxoplasma systems that have the potential for adaptive behavioral manipulation will continue to contribute significantly to the field, dead-end host-Toxoplasma systems may have limited potential. The human-Toxoplasma system is a particularly good example, with little mechanistic basis for a multitude of reported correlative behavioral effects resulting from infection. Parasitologists might consider directing research effort away from host-parasite systems such as human-Toxoplasma and toward systems with potential adaptive behavioral manipulation.
Furthermore, parasitologists should not be discouraged from investigating new host-parasite systems for adaptive behavioral manipulation. Finding convergence in manipulative mechanisms across evolutionary distant parasite species would be a significant finding. For example, finding that PTP is crucial in multiple other host-parasite systems where behavioral manipulation occurs would greatly contribute to establishing PTP as a gateway for behavioral manipulation. Similarly, expanding on the hairworm-grasshopper/cricket systems to other hairworm-host systems (of which there a many) and finding similar modulation of protein levels would be noteworthy, suggesting a convergence in the mechanisms used to generate hydrophilic behavior. Essentially, establishing similar potential mechanisms within types of behavioral manipulation (i.e., locomotor adjustment, aggression, hydrophilia) will progress the field from hypotheses to theories of behavioral manipulation. However, it is important to keep in mind that behavioral manipulation has arisen multiple times over the course of evolution. Therefore, it is important to not seek convergence in mechanisms where parsimony is significantly compromised. The ultimate goal here should be to establish working hypotheses on where the different types behavioral modifications first evolved, and the initial mutations that eventually lead to the different types of behavioral manipulations.
Fully Realizing a Multi-Dimensional Phenomenon
From the previous sections, it is clear that comprehensively summarizing the major pathways of host manipulation in isolation is not possible. Furthermore, the further researchers delve into the mechanisms of host manipulation, the more apparent this integration and reliance on multiple pathways becomes. Toxoplasma is a textbook example of this phenomenon, having multiple effects on all the major pathways of manipulation. The integrated action of these pathways is clear from past research (not just Toxoplasma) and appears to be a crucial component in the manipulation of host behavior by parasites. Given this, the analysis of 50 mechanistic studies on parasite behavioral manipulation published between 1990 and 2017 yields surprising results (Figure 2). Only 7 of the mechanistic studies directly mentioned, or considered as an alternative hypothesis, all of the major pathways in host manipulation within the context of their study. Fourteen studies considered another pathway other than their focal pathway, while 29 of the studies in the dataset only considered their focal pathway, ignoring the other pathways.
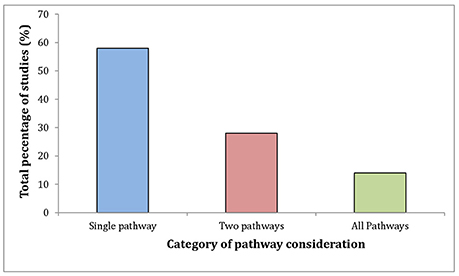
Figure 2. Consideration of the major pathways in host manipulation: Gene/proteomic, neuropharmological and immunological, from 1990 to 2017 in mechanistic studies of host manipulation. Studies were selected using the Web of Science database (search terms: parasite* AND behavior* AND mechanis* AND manipula* OR effect*), and classified based on their consideration of the pathways of host manipulation. To qualify for “consideration,” studies had to either directly address the pathway, or consider it as an alternative hypothesis relative to their original target pathway. 58% (n = 29) of papers considered a single pathway, while 28% (n = 14) of studies considered two pathways and only 14% (7) of papers considered all 3 major pathways.
Considering the results in Figure 2 highlight the tight focus many mechanistic studies take to host manipulation, and that evidence suggests parasites are using multiple pathways to manipulate behavior, we can see a mismatch between the mindsets of the researcher and the parasite. Ultimately this could be one of the main barriers to progress in host manipulation research. Therefore, in future studies, researchers should consider paying greater attention to the possibility of integration between their focal pathway and the other major pathways of manipulation. Also, a greater focus should be placed on the interactions between pathways that enable host manipulation.
Conversely, a more critical consideration of other pathways is necessary, since molecular alterations in a single pathway could just as likely be pathological side effects of infection. We need greater awareness of this possibility in hosts with complex physiology, as the literature on vertebrate immunological manipulation has demonstrated. Parasites may have to rely on more elaborate mechanisms to establish themselves and manipulate behavior in complex hosts. Consequently, physiologically complex hosts may increase the chances of an observed molecular change in the host being a pathological side effect, rather than a manipulative effort from the parasite.
Redefining the Term Proximate in the Host Manipulation Literature
As suggested multiple times in this review, the crux of past host manipulation research is the disconnected evidence for molecular manipulation throughout the steps of host manipulation. Simply put, a functional explanation for any of the molecular changes seen in infected hosts is rare. If we follow the conceptual framework laid out for a more detailed explanation of host manipulation in Figure 3, to directly manipulate host behavior, parasites must be releasing “manipulative factor/s” (see Figure 3 for definition) that directly interact with the major pathways of host manipulation resulting in the observed molecular changes in infected hosts (see Figure 3, step 3). The generation and maintenance of a source for these manipulative factors (see Figure 3 for definition) is also a necessary fundamental step in host manipulation (see Figure 3, step 2).
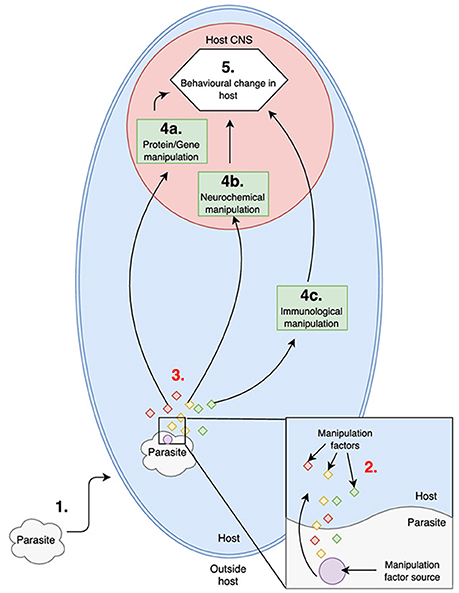
Figure 3. Depiction of the known (1,4,5) and hypothetical (2,3) major steps in a parasite manipulating host behavior adaptively.
Key: Numbers represent known and potential (highlighted in red) major fundamental steps in host manipulation.
(1) Establishment of parasite in host (location of parasite will vary depending of host-parasite system i.e., CNS, muscle, digestive tract).
(2) Source (potentially multiple different sources) of manipulation factors activates at a given time during the parasite's development cycle, releasing manipulation factors into the host.
(3) Manipulation factors exert their effects on one or more of the major pathways in host manipulation.
(4) Molecular change in the host (a- gene or protein modulation of expression/frequency; b- manipulation of neurochemicals such as serotonin or dopamine; c- manipulation of the host immune system) as a result of the manipulative factors released by the parasite.
(5) Host behavior changes as a result of the parasites manipulative effort. Induced behavior directly increases the parasites fitness.
Glossary-
Manipulative factor: Any molecule/substance released by the parasite that alters the normal functioning of one or more of the major identified pathways for host manipulation, resulting in a molecular shift in the host which ultimately changes the host behavior for the benefit of the parasite.
Manipulative factor source: A structure (organelle, membrane, gland etc.) which generates manipulative factors for the parasite to use in host manipulation.
Until very recently, with elucidation of the mechanism behind the attraction of mosquitoes to malaria infected humans (Emami et al., 2017), and the potential mechanism behind baculoviruses effects on host locomotion (Houte et al., 2013), there had been no reported attempts to elucidate step 3, and no attempts at all for step 2. Intracellular pathogenic species are also known to release compounds into the surrounding host tissue, but none of these compounds has yet to be exclusively linked to manipulative effort. Identification of manipulation factors, and their source, is the crucial components missing from host manipulation research. For example, consider that step 2 (venom gland) and step 3 (venom) in the jewel wasp-cockroach system were undoubtedly confirmed via observation, and that this system is arguably the best understood and supported case of adaptive behavioral manipulation. Consequently, proving manipulation factors and their source exist could be a major step toward generating causative empirical evidence for host manipulation and ruling out pathological side effects in favor of adaptive manipulation. Mechanistic studies continually suggest that molecular change in the host is the proximate mechanism for behavioral manipulation, when in fact manipulation factors and their source should be considered the true proximate mechanisms.
Recently, Hébert et al. (2017) have provided a potential avenue for identifying these potential manipulation factors and their source. They found that major genome wide reprogramming events in Schistocephalus solidus (cestode) were associated in transitioning to its intermediate fish host and then to its final avian host. Following the products of genes switched on during transition from intermediate to final host could potentially lead researchers to the manipulative factors (and their source) produced by parasites. In future, establishing genomic changes during transitions in other manipulative parasites and revealing the products of these reprogramming events, would help the field of host manipulation immeasurably. Thus, identifying the truly proximate mechanisms of host manipulation. This could give parasitologists a new avenue for confirming behavioral manipulation, and significantly advance our understanding of how parasites control their host's behavior.
However, it is important to consider that this approach is restricted by a lack of knowledge of the temporal aspects of host manipulation; i.e., do the molecular changes required for behavioral manipulation occur rapidly, or take shape over a prolonged period of time, or involve a combination of temporally-blended changes? Changes in gene expression in the parasite just prior to host transition (Hébert et al., 2017) suggest a rapid temporal profile for behavioral manipulation is possible, at least in this host-parasite system. Yet if a gradual temporal profile for behavioral manipulation takes place in other host-parasite systems, the rapid gene expression changes during host transition should be not be associated with potential behavioral manipulation mechanisms. Instead, genes that slowly alter their expression, or switch on early in development, will be more likely candidates in these host-parasite systems. Ultimately, this points to another caveat in the host manipulation literature that is hindering progress: a limited understanding of the temporal profiles of host behavioral manipulation.
Concluding Remarks
Considering the obstacles facing host manipulation research and the vast sea of uninvestigated host-parasite systems, the field of host manipulation is still in its infancy. This is further emphasized by the relative lack of mechanistic studies in the field. Furthermore, the few mechanistic studies that have attempted to provide causative evidence have mostly focused upon identifying molecular differences between uninfected and infected hosts without providing a functional explanation for said changes. A need for an integrated consideration of the different major pathways of manipulation is another factor that is likely hindering progress. Essentially, all of these factors have resulted in a lack of strong causative evidence for behavioral manipulation by parasites. As such, the field continues to suffer from indecision as to whether host effects represent adaptive manipulation or pathological side effects of infection. Overcoming this barrier is likely to be fundamental to future progress in this field.
The recent identification of a potential manipulative factor in human-Plasmodium (Emami et al., 2017) and baculovirus-insect systems is a promising start. To continue this progress, analyzing the gene expression of a parasite during transitional periods, may direct researchers toward potential manipulative factors and their source. Moreover, adopting this approach in host-parasite systems that show convergence in the type of behavioral manipulation across distantly related taxa of hosts and parasites could be very beneficial, and may potentially help construct guiding principles/mechanisms for host manipulation.
Author Contributions
The author confirms being the sole contributor of this work and approved it for publication.
Conflict of Interest Statement
The author declares that the research was conducted in the absence of any commercial or financial relationships that could be construed as a potential conflict of interest.
Acknowledgments
Thank you to the Otago University parasitology lab and Zoology department for providing much needed evaluation and constructive criticism of this work. Firstly thank you to Robert Poulin who provided me with this opportunity, and for his expert mentorship. Thank you to Olwyn Friesen, Bromwen Presswell, Adele Parli and Steve Evans. Finally, thank you to Allan Herbison from the Otago physiology department for expert advice as well.
References
Abdulai-Saiku, S., and Vyas, A. (2017). Loss of predator aversion in female rats after Toxoplasma gondii infection is not dependent on ovarian steroids. Brain Behav. Immun. 65, 95–98. doi: 10.1016/j.bbi.2017.04.005
Adamo, S. A. (1997). “How parasites alter the behavior of their insect hosts,” in Parasites and Pathogens, ed N. E. Beckage (Boston, MA: Springer), 231–245.
Adamo, S. A. (1998). Feeding suppression in the tobacco hornworm, Manduca sexta: costs and benefits to the parasitic wasp Cotesia congregata. Can. J. Zool. 76, 1634–1640. doi: 10.1139/z98-105
Adamo, S. A. (2005). Parasitic suppression of feeding in the tobacco hornworm, Manduca sexta: parallels with feeding depression after an immune challenge. Arch. Insect Biochem. Physiol. 60, 185–197. doi: 10.1002/arch.20068
Adamo, S. A. (2006). Comparative psychoneuroimmunology: evidence from the insects. Behav. Cogn. Neurosci. Rev. 5, 128–140. doi: 10.1177/1534582306289580
Adamo, S. A. (2008). Bidirectional connections between the immune system and the nervous system in insects. Insect Immunol. 129–149. doi: 10.1016/B978-012373976-6.50008-2
Adamo, S. A. (2012). “The strings of the puppet master: how parasites change host behaviour,” in Parasitic Manipulation, eds D. Hughes and F. Thomas (Oxford: Oxford University Press), 36–51.
Adamo, S. A. (2013). Parasites: evolution's neurobiologists. J. Exp. Biol. 216, 3–10. doi: 10.1242/jeb.073601
Amat, F., Gozalbo, A., Navarro, J. C., Hontoria, F., and Varó, I. (1991). Some aspects of Artemia biology affected by cestode parasitism. Hydrobiologia 212, 39–44. doi: 10.1007/BF00025985
Auger, C. J., Coss, D., Auger, A. P., and Forbes-Lorman, R. M. (2011). Epigenetic control of vasopressin expression is maintained by steroid hormones in the adult male rat brain. Proc. Natl. Acad. Sci. U.S.A. 108, 4242–4247. doi: 10.1073/pnas.1100314108
Batista, E. P., Costa, E. F., and Silva, A. A. (2014). Anopheles darlingi (Diptera: Culicidae) displays increased attractiveness to infected individuals with Plasmodium vivax gametocytes. Parasit. Vectors 7:251. doi: 10.1186/1756-3305-7-251
Bentivoglio, M., and Kristensson, K. (2007). Neural–immune interactions in disorders of sleep-wakefulness organization. Trends Neurosci. 30, 645–652. doi: 10.1016/j.tins.2007.09.004
Berger, S. L., Kouzarides, T., Shiekhattar, R., and Shilatifard, A. (2009). An operational definition of epigenetics. Genes Dev. 23, 781–783. doi: 10.1101/gad.1787609
Bethel, W. M., and Holmes, J. C. (1973). Altered evasive behavior and responses to light in amphipods harboring acanthocephalan cystacanths. J. Parasitol. 59, 945–956. doi: 10.2307/3278623
Bethel, W. M., and Holmes, J. C. (1974). Correlation of development of altered evasive behavior in Gammarus lacustris (Amphipoda) harboring cystacanths of Polymorphus paradoxus (Acanthocephala) with the infectivity to the definitive host. J. Parasitol. 60, 272–274. doi: 10.2307/3278463
Bethel, W. M., and Holmes, J. C. (1977). Increased vulnerability of amphipods to predation owing to altered behavior induced by larval acanthocephalans. Can. J. Zool. 55, 110–115. doi: 10.1139/z77-013
Biron, D. G., and Loxdale, H. D. (2013). Host–parasite molecular cross- talk during the manipulative process of a host by its parasite. J. Exp. Biol. 216, 148–160. doi: 10.1242/jeb.073825
Biron, D. G., Marché, L., Ponton, F., Loxdale, H. D., Galéotti, N., Renault, L., et al. (2005). Behavioural manipulation in a grasshopper harbouring hairworm: a proteomics approach. Proc. R. Soc. Lond. B Biol. Sci. 272, 2117–2126. doi: 10.1098/rspb.2005.3213
Biron, D. G., Ponton, F., Marché, L., Galeotti, N., Renault, L., Demey-Thomas, E., et al. (2006). ‘Suicide' of crickets harbouring hairworms: a proteomics investigation. Insect Mol. Biol. 15, 731–742. doi: 10.1111/j.1365-2583.2006.00671.x
Boucias, D., and Pendland, J. C. (2012). Principles of Insect Pathology. New York, NY: Springer Science and Business Media.
Cerenius, L., and Söderhäll, K. (2004). The prophenoloxidase-activating system in invertebrates. Immunol. Rev. 198, 116–126. doi: 10.1111/j.0105-2896.2004.00116.x
Cheeseman, K., and Weitzman, J. B. (2015). Host–parasite interactions: an intimate epigenetic relationship. Cell. Microbiol. 17, 1121–1132. doi: 10.1111/cmi.12471
Cooper, J. R., Bloom, F. E., and Roth, R. H. (2003). The Biochemical Basis of Neuropharmacology. Oxford: Oxford University Press.
Cornet, S., Nicot, A., Rivero, A., and Gandon, S. (2013). Malaria infection increases bird attractiveness to uninfected mosquitoes. Ecol. Lett. 16, 323–329. doi: 10.1111/ele.12041
Dantzer, R. (2004). Cytokine-induced sickness behaviour: a neuroimmune response to activation of innate immunity. Eur. J. Pharmacol. 500, 399–411. doi: 10.1016/j.ejphar.2004.07.040
Dantzer, R., O'Connor, J. C., Freund, G. G., Johnson, R. W., and Kelley, K. W. (2008). From inflammation to sickness and depression: when the immune system subjugates the brain. Nat. Rev. Neurosci. 9, 46–56. doi: 10.1038/nrn2297
de Jong-Brink, M., Bergamin-Sassen, M., and Solis Soto, M. (2001). Multiple strategies of schistosomes to meet their requirements in the intermediate snail host. Parasitology 123, 129–141. doi: 10.1017/S0031182001008149
de Jong-Brink, M., Hoek, R. M., Lageweg, W., and Smit, A. B. (1997). “Schistosome parasites induce physiological changes in their snail hosts by interfering with two regulatory systems, the internal defense system and the neuroendocrine system,” in Parasites and Pathog: Effects on Host Hormones and Behavior, ed N. E. Beckage (Springer, Boston, MA), 57–75. doi: 10.1007/978-1-4615-5983-2_3
Dheilly, N. M. (2014). Holobiont–Holobiont interactions: redefining host–parasite interactions. PLoS Pathog. 10:e1004093. doi: 10.1371/journal.ppat.1004093
Dheilly, N. M., Maure, F., Ravallec, M., Galinier, R., Doyon, J., Duval, D., et al. (2015). Who is the puppet master? Replication of a parasitic wasp-associated virus correlates with host behaviour manipulation. Proc. R. Soc. Lond. B Biol. Sci. 282:20142773. doi: 10.1098/rspb.2014.2773
Dunphy, G. B., and Downer, R. G. (1994). Octopamine, a modulator of the haemocytic nodulation response of non-immune Galleria mellonella larvae. J. Insect Physiol. 40, 267–272. doi: 10.1016/0022-1910(94)90050-7
Emami, S. N., Lindberg, B. G., Hua, S., Hill, S. R., Mozuraitis, R., Lehmann, P., et al. (2017). A key malaria metabolite modulates vector blood seeking, feeding, and susceptibility to infection. Science 355, 1076–1080. doi: 10.1126/science.aah4563
Fearon, D. T., and Locksley, R. M. (1996). The instructive role of innate immunity in the acquired immune response. Science 272:50. doi: 10.1126/science.272.5258.50
Feldmeyer, B., Mazur, J., Beros, S., Lerp, H., Binder, H., and Foitzik, S. (2016). Gene expression patterns underlying parasite-induced alterations in host behaviour and life history. Mol. Ecol. 25, 648–660. doi: 10.1111/mec.13498
Fichorova, R. N., Lee, Y., Yamamoto, H. S., Takagi, Y., Hayes, G. R., Goodman, R. P., et al. (2012). Endobiont viruses sensed by the human host – beyond conventional antiparasitic therapy. PLoS ONE 7:e48418. doi: 10.1371/journal.pone.0048418
Fitzpatrick, M. J., and Sokolowski, M. B. (2004). In search of food: exploring the evolutionary link between cGMP-dependent protein kinase (PKG) and behaviour. Integr. Comp. Biol. 44, 28–36. doi: 10.1093/icb/44.1.28
Flegr, J., Zitková, Š., Kodym, P., and Frynta, D. (1996). Induction of changes in human behaviour by the parasitic protozoan Toxoplasma gondii. Parasitology 113, 49–54. doi: 10.1017/S0031182000066269
Friberg, I. M., Bradley, J. E., and Jackson, J. A. (2010). Macroparasites, innate immunity and immunoregulation: developing natural models. Trends Parasitol. 26, 540–549. doi: 10.1016/j.pt.2010.06.010
Dobson, A., Lafferty, K. D., Kuris, A. M., Hechinger, R. F., and Jetz, W. (2008). Homage to linnaeus: how many parasites? How many hosts? Proc. Natl. Acad. Sci. U.S.A. 105(Suppl. 1), 11482–11489. doi: 10.1073/pnas.0803232105
Geffre, A. C., Liu, R., Manfredini, F., Beani, L., Kathirithamby, J., Grozinger, C. M., et al. (2017). Transcriptomics of an extended phenotype: parasite manipulation of wasp social behaviour shifts expression of caste-related genes. Proc. R. Soc. B Biol. Sci. 284:20170029. doi: 10.1098/rspb.2017.0029
Gilmore, T. D. (2006). Introduction to NF-κB: players, pathways, perspectives. Oncogene 25, 6680–6684. doi: 10.1038/sj.onc.1209954
Gómez-Díaz, E., Jordà, M., Peinado, M. A., and Rivero, A. (2012). Epigenetics of host–pathogen interactions: the road ahead and the road behind. PLoS Pathog. 8:e1003007. doi: 10.1371/journal.ppat.1003007
Gonzalez, L. E., Rojnik, B., Urrea, F., Urdaneta, H., Petrosino, P., Colasante, C., et al. (2007). Toxoplasma gondii infection lower anxiety as measured in the plus-maze and social interaction tests in rats: a behavioral analysis. Behav. Brain Res. 177, 70–79. doi: 10.1016/j.bbr.2006.11.012
Hakimi, M. A., and Cannella, D. (2011). Apicomplexan parasites and subversion of the host cell microRNA pathway. Trends Parasitol. 27, 481–486. doi: 10.1016/j.pt.2011.07.001
Hamilton, C. M., Brandes, S., Holland, C. V., and Pinelli, E. (2008). Cytokine expression in the brains of Toxocara canis-infected mice. Parasite Immunol. 30, 181–185. doi: 10.1111/j.1365-3024.2007.01002.x
Harbo, J. R., and Harris, J. W. (2009). Responses to Varroa by honey bees with different levels of Varroa Sensitive Hygiene. J. Apic. Res. 48, 156–161. doi: 10.3896/IBRA.1.48.3.02
Hari Dass, S. A., and Vyas, A. (2014). Toxoplasma gondii infection reduces predator aversion in rats through epigenetic modulation in the host medial amygdala. Mol. Ecol. 23, 6114–6122. doi: 10.1111/mec.12888
Hart, B. L. (1988). Biological basis of the behavior of sick animals. Neurosci. Biobehav. Rev. 12, 123–137. doi: 10.1016/S0149-7634(88)80004-6
Hébert, F. O., Grambauer, S., Barber, I., Landry, C. R., and Aubin-Horth, N. (2017). Major host transitions are modulated through transcriptome-wide reprogramming events in Schistocephalus solidus, a threespine stickleback parasite. Mol. Ecol. 26, 1118–1130. doi: 10.1111/mec.13970
Helluy, S. (1983). Host -parasite relations of the trematode Microphallus papillorobustus (Rankin 1940). III Factors involved in the behavioral changes of the Gammarus, intermediate hosts and predatortests. Ann. Parasitol. Hum. Comp. 59, 41–56.
Helluy, S. (2013). Parasite-induced alterations of sensorimotor pathways in gammarids: collateral damage of neuroinflammation? J. Exp. Biol. 216, 67–77. doi: 10.1242/jeb.073213
Helluy, S., and Holmes, J. C. (1990). Serotonin, octopamine, and the clinging behavior induced by the parasite Polymorphus paradoxus (Acanthocephala) in Gammarus lacustris (Crustacea). Can. J. Zool. 68, 1214–1220. doi: 10.1139/z90-181
Helluy, S., and Thomas, F. (2010). Parasitic manipulation and neuroinflammation: evidence from the system Microphallus papillorobustus (Trematoda)-Gammarus (Crustacea). Parasit. Vectors 3:1. doi: 10.1186/1756-3305-3-38
Hemachudha, T., Laothamatas, J., and Rupprecht, C. E. (2002). Human rabies: a disease of complex neuropathogenetic mechanisms and diagnostic challenges. Lancet Neurol. 1, 101–109. doi: 10.1016/S1474-4422(02)00041-8
Henriquez, S. A., Brett, R., Alexander, J., Pratt, J., and Roberts, C. W. (2009). Neuropsychiatric disease and Toxoplasma gondii infection. Neuroimmunomodulation 16, 122–133. doi: 10.1159/000180267
Hill, I. R., Denham, D. A., and Scholtz, C. L. (1985). Toxocara canis larvae in the brain of a British child. Trans. R. Soc. Trop. Med. Hyg. 79, 351–354. doi: 10.1016/0035-9203(85)90378-5
Holland, C. V., and Hamilton, C. M. (2013). The significance of cerebral toxocariasis: a model system for exploring the link between brain involvement, behaviour and the immune response. J. Exp. Biol. 216, 78–83. doi: 10.1242/jeb.074120
House, P. K., Vyas, A., and Sapolsky, R. (2011). Predator cat odors activate sexual arousal pathways in brains of Toxoplasma gondii infected rats. PLoS ONE 6:e23277. doi: 10.1371/journal.pone.0023277
Houte, S., Ros, V. I., and Oers, M. M. (2013). Walking with insects: molecular mechanisms behind parasitic manipulation of host behaviour. Mol. Ecol. 22, 3458–3475. doi: 10.1111/mec.12307
Huber, R. (2005). Amines and motivated behaviors: a simpler systems approach to complex behavioral phenomena. J. Comp. Physiol. A 191, 231–239. doi: 10.1007/s00359-004-0585-5
Hughes, D. (2013). Pathways to understanding the extended phenotype of parasites in their hosts. J. Exp. Biol. 216, 142–147. doi: 10.1242/jeb.077461
Igaki, T., Kanda, H., Yamamoto-Goto, Y., Kanuka, H., Kuranaga, E., Aigaki, T., et al. (2002). Eiger, a TNF superfamily ligand that triggers the Drosophila JNK pathway. EMBO J. 21, 3009–3018. doi: 10.1093/emboj/cdf306
Ives, A., Ronet, C., Prevel, F., Ruzzante, G., Fuertes-Marraco, S., Schutz, F., et al. (2011). Leishmania RNA virus controls the severity of mucocutaneous leishmaniasis. Science 331, 775–778. doi: 10.1126/science.1199326
James, E. R., and Green, D. R. (2004). Manipulation of apoptosis in the host–parasite interaction. Trends Parasitol. 20, 280–287. doi: 10.1016/j.pt.2004.04.004
Jaunarajs, K. L. E., Angoa-Perez, M., Kuhn, D. M., and Bishop, C. (2011). Potential mechanisms underlying anxiety and depression in Parkinson's disease: consequences of l-DOPA treatment. Neurosci. Biobehav. Rev. 35, 556–564. doi: 10.1016/j.neubiorev.2010.06.007
Kamita, S. G., Nagasaka, K., Chua, J. W., Shimada, T., Mita, K., Kobayashi, M., et al. (2005). A baculovirus-encoded protein tyrosine phosphatase gene induces enhanced locomotory activity in a lepidopteran host. Proc. Natl. Acad. Sci. U.S.A. 102, 2584–2589. doi: 10.1073/pnas.0409457102
Kannan, G., Moldovan, K., Xiao, J. C., Yolken, R. H., Jones-Brando, L., and Pletnikov, M. V. (2010). Toxoplasma gondii strain- dependent effects on mouse behaviour. Folia Parasitol. 57:151. doi: 10.14411/fp.2010.019
Kevan, P. G., Hannan, M. A., and Ostiguy, N. (2006). A summary of the Varroa-virus disease complex in honey bees. Am. Bee J. 146, 694–697.
Klein, S. L. (2003). Parasite manipulation of the proximate mechanisms that mediate social behavior in vertebrates. Physiol. Behav. 79, 441–449. doi: 10.1016/S0031-9384(03)00163-X
Klein, S. L. (2004). Hormonal and immunological mechanisms mediating sex differences in parasite infection. Parasite Immunol. 26, 247–264. doi: 10.1111/j.0141-9838.2004.00710.x
Lafferty, K. D., and Shaw, J. C. (2013). Comparing mechanisms of host manipulation across host and parasite taxa. J. Exp. Biol. 216, 56–66. doi: 10.1242/jeb.073668
Lefevre, T., Adamo, S. A., Biron, D. G., Misse, D., Hughes, D., and Thomas, F. (2009). Invasion of the body snatchers: the diversity and evolution of manipulative strategies in host–parasite interactions. Adv. Parasitol. 68, 45–83. doi: 10.1016/S0065-308X(08)00603-9
Lewis, S. E., Freund, J. G., Wankowski, J. L., and Baldridge, M. G. (2016). Correlations between estrogen and testosterone concentrations, pairing status and acanthocephalan infection in an amphipod. J. Zool. 298, 241–248. doi: 10.1111/jzo.12309
Libersat, F., Delago, A., and Gal, R. (2009). Manipulation of host behavior by parasitic insects and insect parasites. Annu. Rev. Entomol. 54, 189–207. doi: 10.1146/annurev.ento.54.110807.090556
Libersat, F., and Gal, R. (2013). What can parasitoid wasps teach us about decision-making in insects? J. Exp. Biol. 216, 47–55. doi: 10.1242/jeb.073999
Libersat, F., and Gal, R. (2014). Wasp voodoo rituals, venom-cocktails, and the zombification of cockroach hosts. Integr. Comp. Biol. 54, 129–142. doi: 10.1093/icb/icu006
Libersat, F., and Pflueger, H. J. (2004). Monoamines and the orchestration of behavior. Bioscience 54, 17–25. doi: 10.1641/0006-3568(2004)054[0017:MATOOB]2.0.CO;2
Lim, A., Kumar, V., Hari Dass, S. A., and Vyas, A. (2013). Toxoplasma gondii infection enhances testicular steroidogenesis in rats. Mol. Ecol. 22, 102–110. doi: 10.1111/mec.12042
Lipsitch, M., Siller, S., and Nowak, M. A. (1996). The evolution of virulence in pathogens with vertical and horizontal transmission. Evolution 50, 1729–1741. doi: 10.1111/j.1558-5646.1996.tb03560.x
Lüder, C. G., Stanway, R. R., Chaussepied, M., Langsley, G., and Heussler, V. T. (2009). Intracellular survival of apicomplexan parasites and host cell modification. Int. J. Parasitol. 39, 163–173. doi: 10.1016/j.ijpara.2008.09.013
Mabery, E. M., and Schneider, D. S. (2010). The Drosophila TNF ortholog eiger is required in the fat body for a robust immune response. J. Innate Immun. 2, 371–378. doi: 10.1159/000315050
Maia, C. S. F., Monteiro, M. C., Gavioli, E. C., Oliveira, F. R., Oliveira, G. B., and Romão, P. R. T. (2015). Neurological disease in human and canine leishmaniasis–clinical features and immunopathogenesis. Parasite Immunol. 37, 385–393. doi: 10.1111/pim.12203
Martin, S. J. (2001). The role of Varroa and viral pathogens in the collapse of honeybee colonies: a modelling approach. J. Appl. Ecol. 38, 1082–1093. doi: 10.1046/j.1365-2664.2001.00662.x
McConkey, G. A., Martin, H. L., Bristow, G. C., and Webster, J. P. (2013). Toxoplasma gondii infection and behaviour–location, location, location? J. Exp. Biol. 216, 113–119. doi: 10.1242/jeb.074153
McDonough, K. A., and Rodriguez, A. (2012). The myriad roles of cyclic AMP in microbial pathogens: from signal to sword. Nat. Rev. Microbiol. 10, 27–38. doi: 10.1038/nrmicro2688
McMaster, W. R., Morrison, C. J., and Kobor, M. S. (2016). Epigenetics: a new model for intracellular parasite–host cell regulation. Trends Parasitol. 32, 515–521. doi: 10.1016/j.pt.2016.04.002
Meffert, M. K., Chang, J. M., Wiltgen, B. J., Fanselow, M. S., and Baltimore, D. (2003). NF-κB functions in synaptic signaling and behavior. Nat. Neurosci. 6, 1072–1078. doi: 10.1038/nn1110
Mekata, T., Sudhakaran, R., Okugawa, S., Inada, M., Kono, T., Sakai, M., et al. (2010). A novel gene of tumor necrosis factor ligand superfamily from kuruma shrimp, Marsupenaeus japonicus. Fish Shellfish Immunol. 28, 571–578. doi: 10.1016/j.fsi.2009.12.020
Miles, C. I., and Booker, R. (2000). Octopamine mimics the effects of parasitism on the foregut of the tobacco hornworm Manduca sexta. J. Exp. Biol. 203, 1689–1700.
Mori, M., and Gotoh, T. (2000). Regulation of nitric oxide production by arginine metabolic enzymes. Biochem. Biophys. Res. Commun. 275, 715–719. doi: 10.1006/bbrc.2000.3169
Mustelin, T. (2007). Protein tyrosine phosphatases. Lymphocyte Signal. Transduct. 584:53. doi: 10.1007/0-387-34132-3_5
Nappi, A. J., and Christensen, B. M. (2005). Melanogenesis and associated cytotoxic reactions: applications to insect innate immunity. Insect Biochem. Mol. Biol. 35, 443–459. doi: 10.1016/j.ibmb.2005.01.014
Nelson, R. J., and Trainor, B. C. (2007). Neural mechanisms of aggression. Nat. Rev. Neurosci. 8, 536–546. doi: 10.1038/nrn2174
Øverli, Ø., Sørensen, C., Pulman, K. G., Pottinger, T. G., Korzan, W., Summers, C. H., et al. (2007). Evolutionary background for stress- coping styles: relationships between physiological, behavioral, and cognitive traits in non-mammalian vertebrates. Neurosci. Biobehav. Rev. 31, 396–412. doi: 10.1016/j.neubiorev.2006.10.006
Ottaviani, E., and Franceschi, C. (1996). The neuroimmunology of stress from invertebrates to man. Prog. Neurobiol. 48, 421–440. doi: 10.1016/0301-0082(95)00049-6
Perrot-Minnot, M. J., and Cézilly, F. (2013). Investigating candidate neuromodulatory systems underlying parasitic manipulation: concepts, limitations and prospects. J. Exp. Biol. 216, 134–141. doi: 10.1242/jeb.074146
Petersen, C. A., and Greenlee, M. H. W. (2011). Neurologic manifestations of Leishmania spp. infection. J. Neuroparasitol. 2, 1–5. doi: 10.4303/jnp/N110401
Pflüger, H. J., and Stevenson, P. A. (2005). Evolutionary aspects of octopaminergic systems with emphasis on arthropods. Arthropod Struct. Dev. 34, 379–396. doi: 10.1016/j.asd.2005.04.004
Ponton, F., Lefèvre, T., Lebarbenchon, C., Thomas, F., Loxdale, H. D., Marché, L., et al. (2006). Do distantly related parasites rely on the same proximate factors to alter the behaviour of their hosts? Proc. R. Soc. Lond. B Biol. Sci. 273, 2869–2877. doi: 10.1098/rspb.2006.3654
Portes, A., Giestal-de-Araujo, E., Fagundes, A., Pandolfo, P., de Sá Geraldo, A., Lira, M. L. F., et al. (2016). Leishmania amazonensis infection induces behavioral alterations and modulates cytokine and neurotrophin production in the murine cerebral cortex. J. Neuroimmunol. 301, 65–73. doi: 10.1016/j.jneuroim.2016.11.003
Poulin, R. (2010). Parasite manipulation of host behavior: an update and frequently asked questions. Adv. Stud. Behav. 41, 151–186. doi: 10.1016/S0065-3454(10)41005-0
Poulin, R. (2011a). The many roads to parasitism: a tale of convergence. Adv. Parasitol. 74, 1–40. doi: 10.1016/B978-0-12-385897-9.00001-X
Poulin, R., and Maure, F. (2015). Host manipulation by parasites: a look back before moving forward. Trends Parasitol. 31, 563–570. doi: 10.1016/j.pt.2015.07.002
Poulin, R., and Thomas, F. (2008). Epigenetic effects of infection on the phenotype of host offspring: parasites reaching across host generations. Oikos 117, 331–335. doi: 10.1111/j.2007.0030-1299.16435.x
Prandovszky, E., Gaskell, E., Martin, H., Dubey, J. P., Webster, J. P., and McConkey, G. A. (2011). The neurotropic parasite Toxoplasma gondii increases dopamine metabolism. PLoS ONE 6:e23866. doi: 10.1371/journal.pone.0023866
Qin, L., Wu, X., Block, M. L., Liu, Y., Breese, G. R., Hong, J. S., et al. (2007). Systemic LPS causes chronic neuroinflammation and progressive neurodegeneration. Glia 55, 453–462. doi: 10.1002/glia.20467
Rahman, M. M., and McFadden, G. (2011). Modulation of NF-κB signalling by microbial pathogens. Nat. Rev. Microbiol. 9, 291–306. doi: 10.1038/nrmicro2539
Rauque, C. A., Paterson, R. A., Poulin, R., and Tompkins, D. M. (2011). Do different parasite species interact in their effects on host fitness? A case study on parasites of the amphipod Paracalliope fluviatilis. Parasitology 138, 1176–1182. doi: 10.1017/S0031182011000928
Rosenberg, L. A., Pflüger, H. J., Wegener, G., and Libersat, F. (2006). Wasp venom injected into the prey's brain modulates thoracic identified monoaminergic neurons. Dev. Neurobiol. 66, 155–168. doi: 10.1002/neu.20203
Rozenfeld, C., Martinez, R., Figueiredo, R. T., Bozza, M. T., Lima, F. R., Pires, A. L., et al. (2003). Soluble factors released by Toxoplasma gondii-infected astrocytes down-modulate nitric oxide production by gamma interferon -activated microglia and prevent neuronal degeneration. Infect. Immun. 71, 2047–2057. doi: 10.1128/IAI.71.4.2047-2057.2003
Salvador, S., Ribeiro, R., Winckler, M. I., Ohlweiler, L., and Riesgo, R. (2010). Pediatric neurotoxocariasis with concomitant cerebral, cerebellar, and peripheral nervous system involvement: case report and review of the literature. J. Pediatr. 86, 531–534. doi: 10.1590/S0021-75572010000600015
Sánchez, M. I., Georgiev, B. B., and Green, A. J. (2007). Avian cestodes affect the behaviour of their intermediate host Artemia parthenogenetica: an experimental study. Behav. Process. 74, 293–299. doi: 10.1016/j.beproc.2006.11.002
Sánchez, M. I., Georgiev, B. B., Nikolov, P. N., Vasileva, G. P., and Green, A. J. (2006). Red and transparent brine shrimps (Artemia parthenogenetica): a comparative study of their cestode infections. Parasitol. Res. 100, 111–114. doi: 10.1007/s00436-006-0248-2
Sánchez, M. I., Thomas, F., Perrot-Minnot, M. J., Biron, D. G., Bertrand-Michel, J., and Missé, D. (2009). Neurological and physiological disorders in Artemia harboring manipulative cestodes. J. Parasitol. 95, 20–24. doi: 10.1645/GE-1550.1
Sapp, J., Carrapiço, F., and Zolotonosov, M. (2002). Symbiogenesis: the hidden face of Constantin Merezhkowsky. Hist. Philos. Life Sci. 413–440. doi: 10.1080/03919710210001714493
Sciutto, E., Chavarria, A., Fragoso, G., Fleury, A., and Larralde, C. (2007). The immune response in Taenia solium cysticercosis: protection and injury. Parasite Immunol. 29, 621–636. doi: 10.1111/j.1365-3024.2007.00967.x
Shaw, J. C., Korzan, W. J., Carpenter, R. E., Kuris, A. M., Lafferty, K. D., Summers, C. H., et al. (2009). Parasite manipulation of brain monoamines in California killifish (Fundulus parvipinnis) by the trematode Euhaplorchis californiensis. Proc. R. Soc. Lond. B Biol. Sci. 276, 1137–1146. doi: 10.1098/rspb.2008.1597
Sih, A., Mathot, K. J., Moirón, M., Montiglio, P. O., Wolf, M., and Dingemanse, N. J. (2015). Animal personality and state–behaviour feedbacks: a review and guide for empiricists. Trends Ecol. Evol. 30, 50–60. doi: 10.1016/j.tree.2014.11.004
Sivakumar, T., Hayashida, K., Sugimoto, C., and Yokoyama, N. (2014). Evolution and genetic diversity of Theileria. Infect. Genet. Evol. 27, 250–263. doi: 10.1016/j.meegid.2014.07.013
Skinner, W. S., Dennis, P. A., Li, J. P., Summerfelt, R. M., Carney, R. L., and Quistad, G. B. (1991). Isolation and identification of paralytic peptides from hemolymph of the lepidopteran insects Manduca sexta, Spodoptera exigua, and Heliothis virescens. J. Biol. Chem. 266, 12873–12877.
Tain, L., Perrot-Minnot, M. J., and Cézilly, F. (2006). Altered host behaviour and brain serotonergic activity caused by acanthocephalans: evidence for specificity. Proc. R. Soc. Lond. B Biol. Sci. 273, 3039–3045. doi: 10.1098/rspb.2006.3618
Thomas, F., Adamo, S., and Moore, J. (2005). Parasitic manipulation: where are we and where should we go? Behav. Process. 68, 185–199. doi: 10.1016/j.beproc.2004.06.010
Thomas, F., Brodeur, J., Maure, F., Franceschi, N., Blanchet, S., and Rigaud, T. (2011). Intraspecific variability in host manipulation by parasites. Infect. Genet. Evol. 11, 262–269. doi: 10.1016/j.meegid.2010.12.013
Thomas, F., Poulin, R., and Brodeur, J. (2010). Host manipulation by parasites: a multidimensional phenomenon. Oikos 119, 1217–1223. doi: 10.1111/j.1600-0706.2009.18077.x
Thomas, F., Schmidt-Rhaesa, A., Martin, G., Manu, C., Durand, P., and Renaud, F. (2002). Do hairworms (Nematomorpha) manipulate the water seeking behaviour of their terrestrial hosts?. J. Evol. Biol. 15, 356–361. doi: 10.1046/j.1420-9101.2002.00410.x
van Houte, S., Ros, V. I., Mastenbroek, T. G., Vendrig, N. J., Hoover, K., Spitzen, J., et al. (2012). Protein tyrosine phosphatase-induced hyperactivity is a conserved strategy of a subset of baculoviruses to manipulate lepidopteran host behavior. PLoS ONE 7:e46933. doi: 10.1371/journal.pone.0046933
Vidal, M. (2010). The dark side of fly TNF: an ancient developmental proof reading mechanism turned into tumor promoter. Cell Cycle 9, 3851–3856. doi: 10.4161/cc.9.19.13280
Vyas, A. (2013). Parasite -augmented mate choice and reduction in innate fear in rats infected by Toxoplasma gondii. J. Exp. Biol. 216, 120–126. doi: 10.1242/jeb.072983
Vyas, A., Kim, S. K., Giacomini, N., Boothroyd, J. C., and Sapolsky, R. M. (2007). Behavioral changes induced by Toxoplasma infection of rodents are highly specific to aversion of cat odors. Proc. Natl. Acad. Sci.U.S.A. 104, 6442–6447. doi: 10.1073/pnas.0608310104
Wang, P. H., Wan, D. H., Pang, L. R., Gu, Z. H., Qiu, W., Weng, S. P., et al. (2012). Molecular cloning, characterization and expression analysis of the tumor necrosis factor (TNF) superfamily gene, TNF receptor superfamily gene and lipopolysaccharide-induced TNF-α factor (LITAF) gene from Litopenaeus vannamei. Dev. Comp. Immunol. 36, 39–50. doi: 10.1016/j.dci.2011.06.002
Webster, J. P. (2007). The effect of Toxoplasma gondii on animal behavior: playing cat and mouse. Schizophr. Bull. 33, 752–756. doi: 10.1093/schbul/sbl073
Webster, J. P., Kaushik, M., Bristow, G. C., and McConkey, G. A. (2013). Toxoplasma gondii infection, from predation to schizophrenia: can animal behaviour help us understand human behaviour? J. Exp. Biol. 216, 99–112. doi: 10.1242/jeb.074716
Weisel-Eichler, A., Haspel, G., and Libersat, F. (1999). Venom of a parasitoid wasp induces prolonged grooming in the cockroach. J. Exp. Biol. 202, 957–964.
Weisel-Eichler, A., and Libersat, F. (2002). Are monoaminergic systems involved in the lethargy induced by a parasitoid wasp in the cockroach prey? J. Comp. Physiol. A Neuroethol. Sens. Neural Behav. Physiol. 188, 315–324. doi: 10.1007/s00359-002-0305-y
Keywords: parasite, pathogen, host, behavior, manipulation, molecular, proximate, mechanism
Citation: Herbison REH (2017) Lessons in Mind Control: Trends in Research on the Molecular Mechanisms behind Parasite-Host Behavioral Manipulation. Front. Ecol. Evol. 5:102. doi: 10.3389/fevo.2017.00102
Received: 05 July 2017; Accepted: 17 August 2017;
Published: 11 September 2017.
Edited by:
David Georges Biron, Centre National de la Recherche Scientifique (CNRS), FranceReviewed by:
Frederic Libersat, Ben-Gurion University of the Negev, Beersheba, IsraelShelley Adamo, Dalhousie University, Canada
Copyright © 2017 Herbison. This is an open-access article distributed under the terms of the Creative Commons Attribution License (CC BY). The use, distribution or reproduction in other forums is permitted, provided the original author(s) or licensor are credited and that the original publication in this journal is cited, in accordance with accepted academic practice. No use, distribution or reproduction is permitted which does not comply with these terms.
*Correspondence: Ryan E. H. Herbison, cnlhbl9oZXJiaUBob3RtYWlsLmNvbQ==